- 1Nanjing Institute of Environmental Science, Ministry of Ecology and Environment, Nanjing, China
- 2State Environmental Protection Key Laboratory of Pesticide Environmental Assessment and Pollution Control, Nanjing, China
- 3Medical School, Southeast University, Nanjing, China
- 4Jiangsu Collaborative Innovation Center of Atmospheric Environment and Equipment Technology, Nanjing University of Information Science and Technology, Nanjing, China
Using Caenorhabditis elegans as an animal model, the possible toxic effects of six insecticides (dinotefuran, thiamethoxam, thiacloprid, nitenpyram, acetamiprid, and sulfoxaflor) commonly used in agriculture on sensory perception were examined. The sensory behaviors of thermotaxis, avoidance of copper ion, chemotaxis to NaCl, and chemotaxis to diacetyl were measured to investigate the damage on sensory perceptions in nematodes exposed to the examined insecticides in the range of micrograms per liter (μg/L). Exposure to dinotefuran, thiamethoxam, thiacloprid, nitenpyram, acetamiprid, or sulfoxaflor at concentrations of 10–100 μg/L resulted in severe deficits in sensory perceptions to temperature, copper ion, NaCl, and diacetyl. The relative neurotoxicity of the six insecticides examined to C. elegans were shown as dinotefuran > thiamethoxam > thiacloprid > nitenpyram > acetamiprid > sulfoxaflor. Moreover, post-treatment with the antioxidant ascorbate effectively suppressed the production of reactive oxygen species and damages of sensory perceptions induced by the six insecticides, indicating that the activation of oxidative stress can act as an important cellular contributor to the observed damage of the examined insecticides in affecting sensory perceptions. Our data highlighted the potential toxicity of the six insecticides at low concentrations in inducing sensory disturbance to environmental organisms.
Introduction
The application of insecticides has made great contributions to the development of agriculture around the world. Among insecticides, most of the neonicotinoid insecticides are considered as classic neurotoxins since they can irreversibly target to nicotinic acetylcholine receptors and cause paralysis and eventual death in most arthropods rather than in vertebrates (Matsuda et al., 2005; Bradford et al., 2020). Moreover, diamide insecticides could damage muscle contraction via activating ryanodine receptors and releasing stored calcium from the sarcoendoplasmic reticulum, which is an important mechanism to control lepidopteran pests (Cordova et al., 2006). Sulfoximine insecticides (such as sulfoxaflor) could kill insects by damaging Ca2+ homeostasis in muscle cells (Guo et al., 2019).
After release into the environment, insecticides potentially cause toxicity to environmental organisms. As reported in previous studies, organochlorines, organophosphates, carbamates, pyrethroids, and neonicotinoid insecticides could cause damage on reproduction, feeding, and avoidance of predation in poultry populations (Walker, 2003). In addition, exposure to insecticides also poses a great threat to some soil organisms—for example, cycloxaprid, a novel neonicotinoid insecticide, could induce neurotoxicity in earthworms, such as neurological dysfunctions, stress responses, and damage on calcium binding (Qi et al., 2018).
Due to the properties of short lifespan, small size, short life cycle, and high sensitivity to pollutants (Leung et al., 2008; Haegerbaeumer et al., 2018; Queirós et al., 2019; Wang, 2020; Zhang et al., 2022), Caenorhabditis elegans has been used as an ideal model for examining the response to environmental toxicants or stresses (Wang et al., 2021a; Liu et al., 2021c; Sun et al., 2021). Recently, it was reported that some neonicotinoid insecticides could cause a dysfunction in the development, reproduction, and locomotion behaviors of C. elegans (Bradford et al., 2020). Moreover, C. elegans is an important animal model for the developmental study of nervous systems because of its simple nervous system structure composed of 302 neurons in adult hermaphrodite (Bargmann, 1998; Corsi et al., 2015). C. elegans is helpful for investigating different functions of the nervous system, such as learning and memory (Ardiel and Rankin, 2010; Bargmann et al., 1993). In addition, C. elegans is a powerful animal model for assessing neurotoxicity induced by different toxicants (Wang, 2019; Liu et al., 2021a)—for example, in C. elegans, the development of dopaminergic nervous systems and their functions (such as sensory perception behaviors) were found to be affected by exposure to nanoparticles (Qu and Wang, 2020). Considering the fact that ∼80% of C. elegans proteome has human homologous genes, specifically neurodevelopmental genes (Riddle et al., 1997), C. elegans is an important animal model for both biomedical and environmental toxicology. Due to the availability of some disease models (Moy et al., 2009; Griffin et al., 2017), C. elegans is also useful for pharmaceutical screening.
Sensory perception is an important function for organisms to sense and detect the existence and alteration of environmental stimuli. Nevertheless, the possible toxic mode of action on sensory perception behaviors induced by exposure to insecticides remains largely unclear in organisms. Thus, the aim of this study is to compare the possible damage of six insecticides (acetamiprid, nitenpyram, thiacloprid, thiamethoxam, dinotefuran, and sulfoxaflor) on the sensory perception behaviors of C. elegans as representative for other nematodes. Among them, acetamiprid, nitenpyram, thiacloprid, thiamethoxam, and dinotefuran are neonicotinoid insecticides. Sulfoxaflor belongs to sulfoximine insecticides. C. elegans has been widely used for the assessment of pesticide toxicity (Gomez-Eyles et al., 2009; Roh and Choi, 2011; Ruan et al., 2012; Du et al., 2015; Yu et al., 2020). The well-described backgrounds of development and functions of the nervous system of C. elegans provide a strong support for this research. The sensory perception behaviors of thermotaxis, avoidance to copper ion, chemotaxis to NaCl, and chemotaxis to diacetyl were examined in this study. Thermotaxis reflects a sensory perception to physical stimuli. The chemotaxis to NaCl and avoidance to copper reflect a gustatory perception to soluble chemicals. The chemotaxis to diacetyl indicates an olfactory perception to volatile chemicals. Our results demonstrated the potential of exposure to the examined insecticides in the range of micrograms per liter (μg/L) in causing damage on sensory perceptions to different degrees in nematodes. The obtained data highlights the toxicity of long-term exposure to the examined insecticides at low concentrations in inducing damage on sensory perceptions in environmental organisms.
Materials and Methods
Reagents
The standards for six insecticides (Figure 1) were obtained from Shanghai Pesticide Research Institute Co. (Shanghai, China). Acetamiprid (purity, 98.0%), nitenpyram (purity, 99.1%), thiacloprid (purity, 99.4%), thiamethoxam (purity, 98.0%), dinotefuran (purity, 99.0%), and sulfoxaflor (purity, 99.7%) were dissolved in distilled water to obtain stock solutions (1 g/L). The working concentrations of the examined insecticides are shown in Supplementary Table S1.
Strains and Maintenance
The wild-type (N2) nematodes were obtained from Caenorhabditis Genetics Center. Nematode growth medium (NGM) plates seeded with Escherichia coli OP50 were used to maintain the examined worms (Brenner, 1974). To obtain age-synchronous L1-larvae nematodes, the gravid worms were lysed using a bleaching mixture solution containing 2% HOCl and 0.45 M NaOH to release enough eggs from the body (Yang et al., 2021b). After that, the eggs were allowed to develop into L1-larvae on new NGM plates.
Exposure
Nematodes were exposed to six insecticides with the addition of OP50 (4 × 106 colony-forming units, CFUs) from L1-larvae to adult stage (adult day-1, approximately 4.5 days). The age-synchronous L1-larvae were used for the exposure. Insecticides were diluted into the examined concentrations using K-medium to determine their effects on different sensory perception behaviors.
Thermotaxis Assay
Radial temperature gradient was employed to perform the thermotaxis assay as described by Ye et al. (2008). A steeper temperature gradient in the range from 17°C (at the center) to 25°C (at the periphery) was prepared. To generate this radial temperature gradient, a vial containing frozen acetic acid was put on the bottom of 9-cm culture plates, and the culture plates were incubated at 26°C (the preferred temperature for nematodes) for 90 min. After exposure to insecticides and the following washing with M9 buffer, the individual worms were transferred on the agar surface of the prepared 9-cm culture plates having a thermal gradient. On the agar surface, the worms were allowed to move for 2 h. After removing the worms, the traces of movement that the examined worms left on the agar surface were captured by camera. The percentages of worms performing isothermal tracking (IT) were counted. Approximately 30 nematodes were examined for each exposure. Three replicates were performed.
Avoidance of Copper
Under normal conditions, the nematodes will avoid the copper on the NGM assay plate (Sambongi et al., 2000). An assay of avoidance of Cu2+ was performed as described previously (Sambongi et al., 2000). A 9-cm culture plate was divided into four equal parts. Among these four equal parts, normal NGM medium was added into two opposite sides. Meanwhile, NGM medium containing 100 μM Cu2+ was added into the other two opposite sides. After exposure to the insecticides and the following washing with M9 buffer, the worms were transferred onto the surface of Cu2+-free parts for 1 h. The avoidance index was calculated as the value of the number of worms on NGM containing Cu2+/total number of worms. Approximately 100 nematodes were examined for each exposure. Three replicates were performed.
Chemotaxis to NaCl
Chemotaxis to NaCl (a water-soluble chemoattractant) was performed as described previously (Saeki et al., 2001). An agar plug containing NaCl (100 mM) was placed on the off-center surface of an agar plate prepared with agar (20 g/L), potassium phosphate (5 mM, pH 6.0), CaCl2 (1 mM), and MgSO4 (1 mM). After overnight treatment, the NaCl plug was removed. In order to anesthetize the nematodes, 1 μl sodium azide (0.5 M) was added on position 4 cm away from NaCl plug position (control) and NaCl plug position. After 1 h, the chemotaxis index (CI) was calculated as the value of (number of worms within 1.5 cm of center of NaCl spot — number of worms within 1.5 cm of the control spot) / (total number of worms). Approximately 100 nematodes were examined for each exposure. Three replicates were performed.
Chemotaxis to Diacetyl
Chemotaxis to diacetyl was performed as described previously (Li et al., 2011). One microliter of diacetyl (10−2) was added on the surface of the assay plates. Meanwhile, 1 μl sodium azide (0.5 M) was added on diacetyl position and the position 4 cm away from the diacetyl position (control). After 1 h, the CI was calculated as the value of (number of worms within 1.5 cm of the center of the diacetyl spot—number of worms within 1.5 cm of the control spot) / (total number of worms). Approximately 100 nematodes were examined for each exposure. Three replicates were performed.
Reactive Oxygen Species Production
Reactive oxygen species (ROS) production was used to reflect the activation of oxidative stress (Liu et al., 2021b). To detect the production of ROS, the control and exposed nematodes were labeled with CM-H2DCFDA (1 µM) in the dark for 3 h (Yang et al., 2021a). After that, the nematodes were washed with M9 buffer three times. C. elegans was then mounted on a 2% agar pad and analyzed for their fluorescence signals at 510 nm (emission filter)/488 nm (excitation wavelength) using laser scanning confocal microscopy. The intestinal fluorescent intensities were determined by normalization against the autofluorescence. For each treatment, 50 nematodes were used. Three replicates were performed.
Pharmacological Assay
The nematodes were first exposed to six insecticides (100 μg/L) with the addition of OP50 (4 × 106 CFUs) from L1-larvae to adult day-1. After that, the nematodes were treated with 10 mM ascorbate (an antioxidant) for 24 h. Three replicates were performed.
Lethality Assay
For the lethality assay, 100 worms were added into each well in a 24-well plate with 250 μl insecticide at concentrations of 0.1, 1, 10, or 100 mg/L. After exposure, the nematodes were transferred to a fresh NGM plate. After gently touching with a needle, the nematodes showing immobilization without recovery were considered dead. Three replicates were performed in each exposure.
The LC50 and 95% confidence interval of the examined insecticides were analyzed using SPSS 13.0 software. The LC50 and 95% confidence interval of the examined insecticides are shown in Supplementary Table S2.
Body Bend Assay
Body bend refers to the alteration in bending direction at the mid-body as described by Wang et al. (2021b). Forty nematodes were analyzed per treatment. Three replicates were performed.
Statistical Analysis
Statistical analysis was performed using SPSS 12.0 software. The probability level of 0.01 (**) was considered to be statistically significant. The one-way analysis of variance, followed by post-hoc Bonferroni test, was performed for group comparisons.
Results
Toxicity Comparison of Six Insecticides in Affecting Thermotaxis
The first sensory perception response to the exposure of six insecticides examined was thermotaxis (Figure 2A). At the concentration of 1 μg/L, none of the six insecticides induced significant changes in thermotaxis in nematodes. However, after exposure to all the examined insecticides at concentrations of 10–100 μg/L, the thermotaxis ability of nematodes decreased significantly compared to the control (p < 0.01) (Figure 2B), and the order for the toxicity of the six insecticides in reducing thermotaxis was dinotefuran > thiamethoxam > thiacloprid > nitenpyram > acetamiprid > sulfoxaflor (Figure 2B).
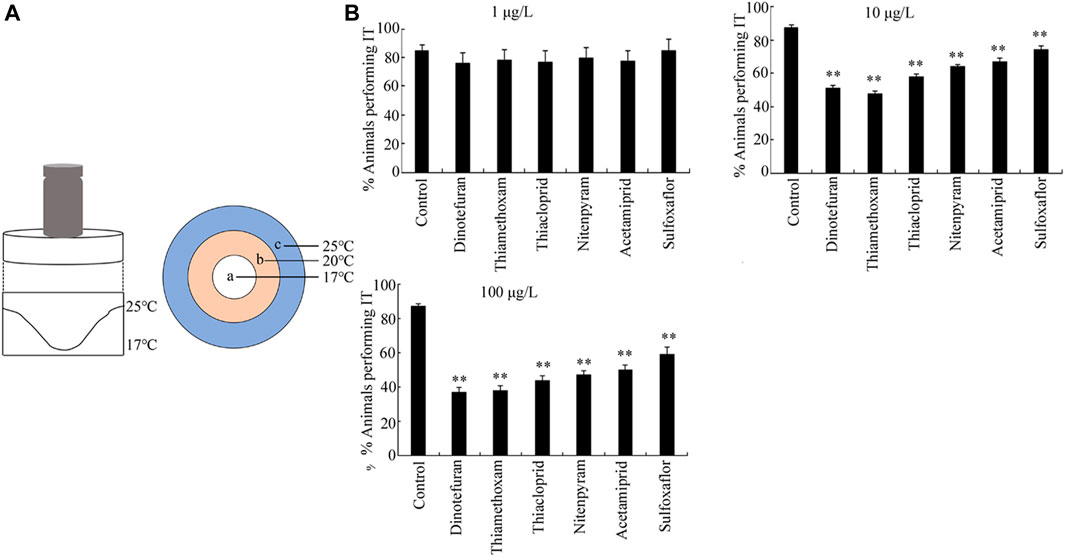
FIGURE 2. Toxicity of insecticides in affecting thermotaxis. (A) Assay model for thermotaxis. (B) Toxicity of the six insecticides examined in affecting thermotaxis ability. IT, isothermal tracking. Nematodes were exposed to insecticide with the addition of OP50 from L1-larvae to adult day-1. Bars represent means ± SD. **p < 0.01 vs. control.
Toxicity Comparison of Six Insecticides in Affecting Avoidance of Copper Ion
The second sensory perception response to the exposure of six insecticides examined was avoidance of copper ion (Figure 3A). Exposure to the six insecticides examined at the concentration of 1 μg/L did not significantly affect the avoidance index (Figure 3B). However, after exposure at concentrations of 10–100 μg/L, all the six insecticides examined could cause a significant increase in the avoidance index compared to the control (p < 0.01) (Figure 3B). At concentrations of 10–100 μg/L, the order for the toxicity of the six insecticides in reducing ability to avoid copper ion was dinotefuran > thiamethoxam > thiacloprid > nitenpyram > acetamiprid > sulfoxaflor (Figure 3B).
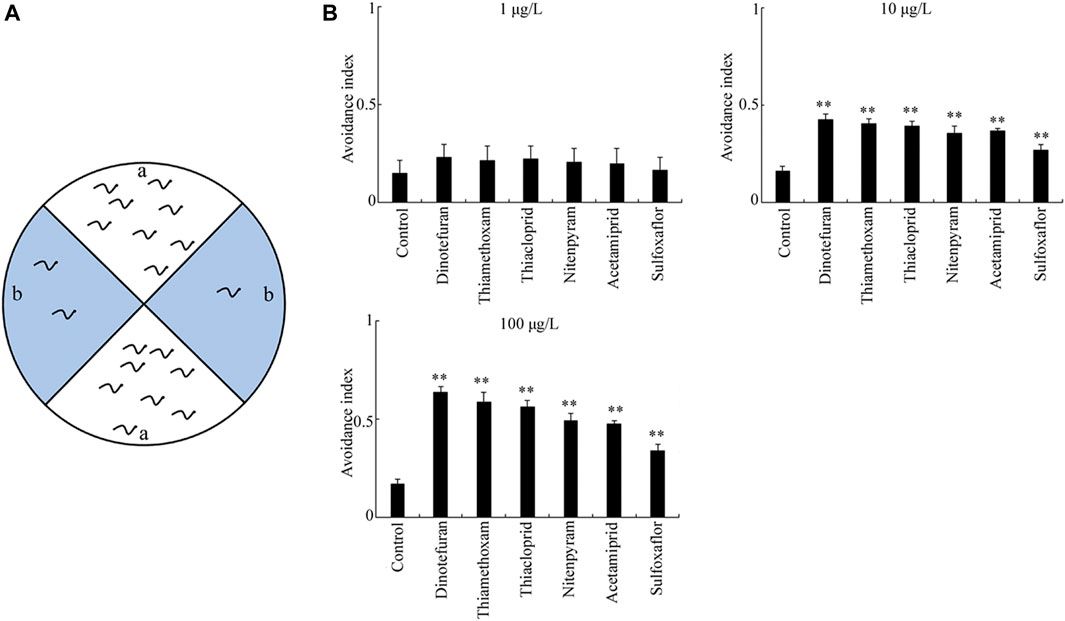
FIGURE 3. Toxicity of insecticides in affecting avoidance of copper ion. (A) Assay model for avoidance of copper ion. (B) Toxicity of the six insecticides examined in affecting the avoidance of copper ion. Nematodes were exposed to insecticide with the addition of OP50 from L1-larvae to adult day-1. Bars represent means ± SD. **p < 0.01 vs. control.
Toxicity Comparison of Six Insecticides in Affecting Chemotaxis to NaCl
The third sensory perception response to the exposure of six insecticides examined was chemotaxis to NaCl (Figure 4A). After exposure at the concentration of 1 μg/L, dinotefuran, thiamethoxam, thiacloprid, nitenpyram, acetamiprid, and sulfoxaflor did not alter chemotaxis to NaCl obviously (Figure 4B). However, after exposure at concentrations of 10–100 μg/L, all the examined insecticides significantly decreased the chemotaxis to NaCl compared to the control (p < 0.01) (Figure 4B). Additionally, after exposure at concentrations of 10–100 μg/L, the order for the toxicity of the six insecticides in inhibiting chemotaxis to NaCl was dinotefuran > thiamethoxam > thiacloprid > nitenpyram > acetamiprid > sulfoxaflor (Figure 4B).
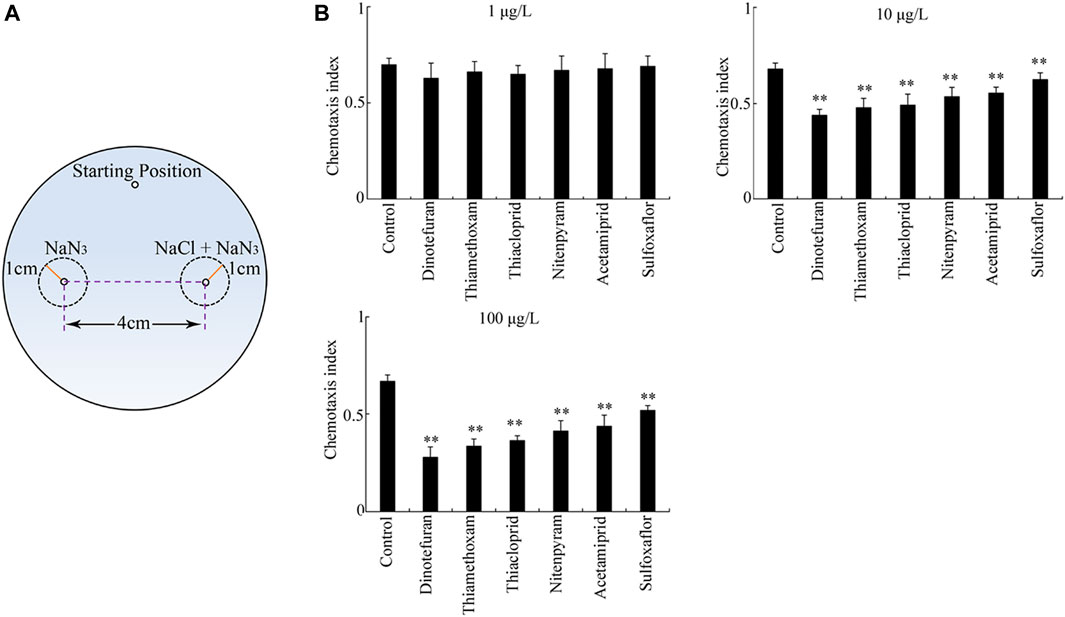
FIGURE 4. Toxicity of insecticides in affecting chemotaxis to NaCl. (A) Assay model for chemotaxis to NaCl. (B) Toxicity of the six insecticides examined in affecting chemotaxis to NaCl. Nematodes were exposed to insecticide with the addition of OP50 from L1-larvae to adult day-1. Bars represent means ± SD. **p < 0.01 vs. control.
Toxicity Comparison of Six Insecticides in Affecting Chemotaxis to Diacetyl
The fourth sensory perception response to the exposure of six insecticides determined was chemotaxis to diacetyl (Figure 5A). After exposure at the concentration of 1 μg/L, dinotefuran, thiamethoxam, thiacloprid, nitenpyram, acetamiprid, and sulfoxaflor did not remarkably influence chemotaxis to diacetyl (Figure 5B). Differently from this, the exposure to all the examined insecticides at concentrations of 10–100 μg/L significantly inhibited chemotaxis to diacetyl compared to the control (p < 0.01) (Figure 5B). Moreover, in the concentration range of 10–100 μg/L, the order for the toxicity of the six insecticides in suppressing chemotaxis to diacetyl was dinotefuran > thiamethoxam > thiacloprid > nitenpyram > acetamiprid > sulfoxaflor (Figure 5B).
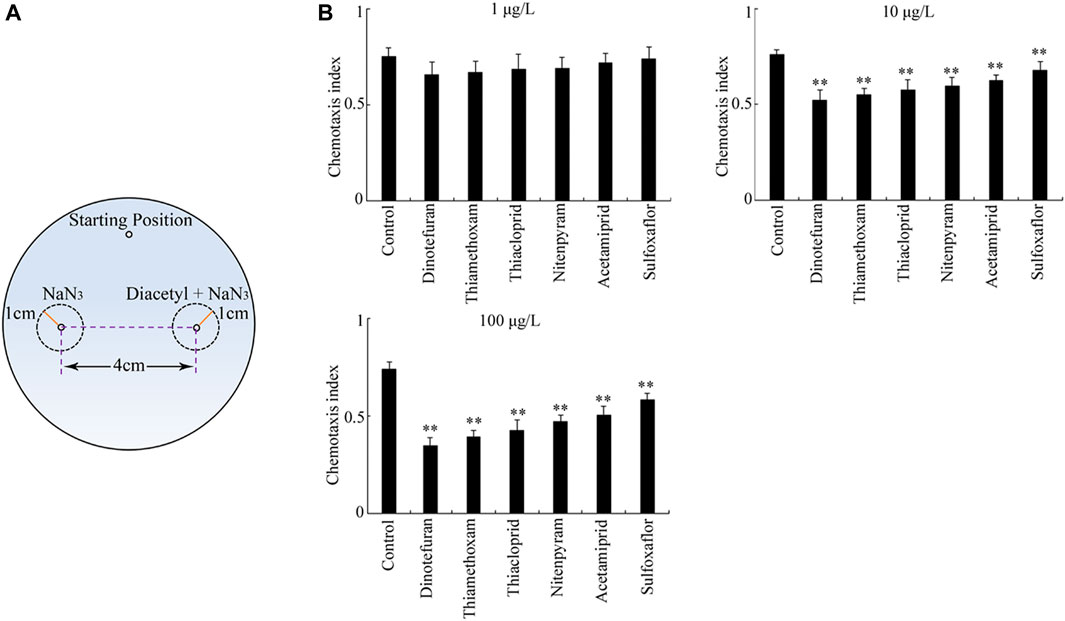
FIGURE 5. Toxicity of insecticides in affecting chemotaxis to diacetyl. (A) Assay model for chemotaxis to diacetyl. (B) Toxicity of the six insecticides examined in affecting chemotaxis to diacetyl. Nematodes were exposed to insecticide with the addition of OP50 from L1-larvae to adult day-1. Bars represent means ± SD. **p < 0.01 vs. control.
Treatment With Antioxidant Suppressed the Damage of Insecticides on Sensory Behaviors
Exposure to the six insecticides (100 μg/L) examined from L1-larvae to adult day-1 caused significant ROS production (p < 0.01) (Figure 6A). In contrast, treatment with 10 mM ascorbate from adult day-1 for 24 h could not induce obvious ROS production. Moreover, after exposure to insecticides, post-treatment with 10 mM ascorbate could obviously suppress ROS production in nematodes exposed to the six insecticides examined (p < 0.01) (Figure 6A).
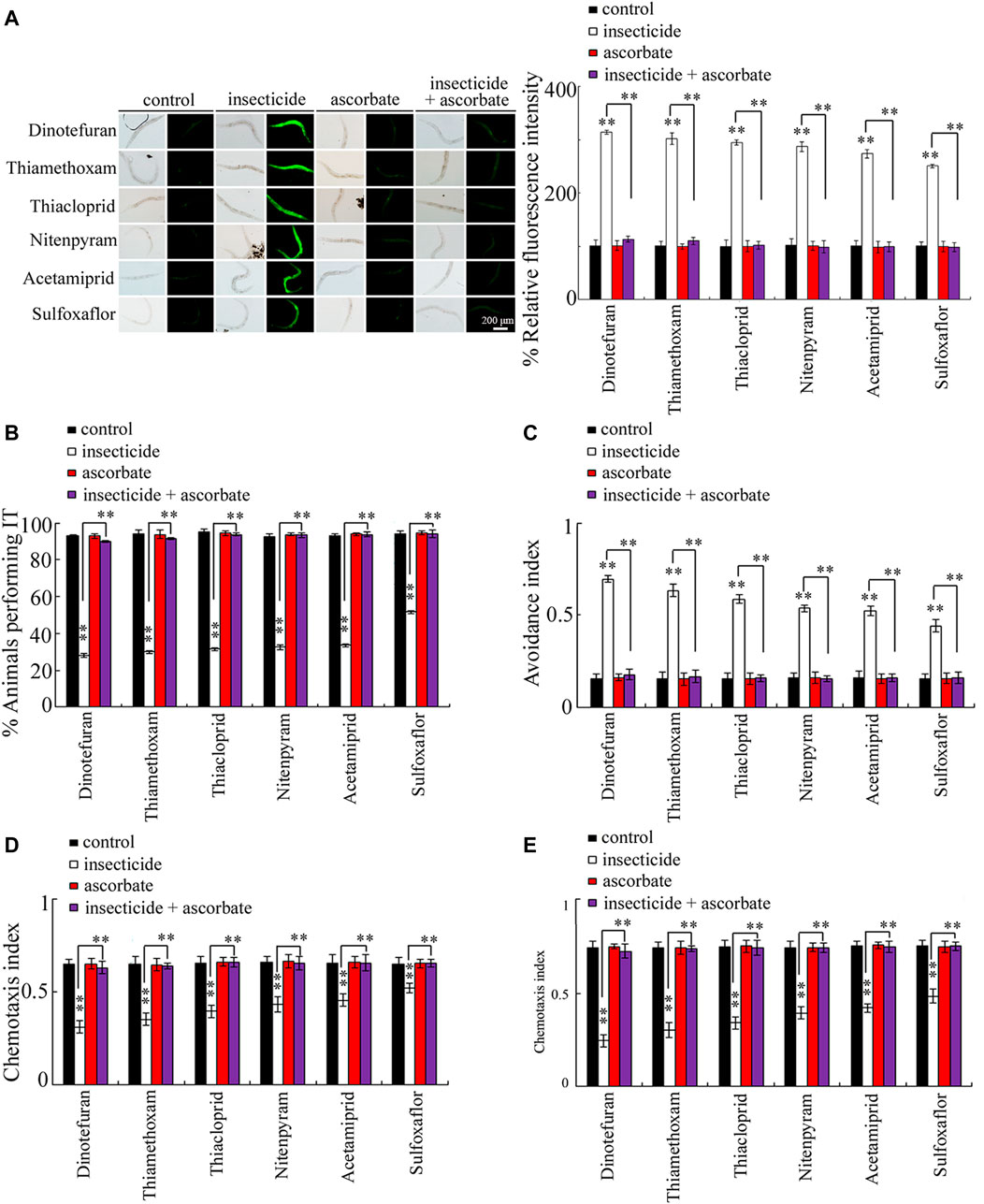
FIGURE 6. Effect of treatment with ascorbate on the toxicity of the examined insecticides in affecting sensory behaviors. (A) Effect of treatment with ascorbate on the toxicity of the examined insecticides in inducing reactive oxygen species production. (B) Effect of treatment with ascorbate on the toxicity of the examined insecticides in affecting thermotaxis ability. (C) Effect of treatment with ascorbate on the toxicity of the examined insecticides in affecting the avoidance of copper ion. (D) Effect of treatment with ascorbate on the toxicity of the examined insecticides in affecting chemotaxis to NaCl. (E) Effect of treatment with ascorbate on the toxicity of the examined insecticides in affecting chemotaxis to diacetyl. Nematodes were first exposed to 100 μg/L insecticides with the addition of OP50 from L1-larvae to adult day-1. After that, the nematodes were treated with 10 mM ascorbate (an antioxidant) for 24 h. Bars represent means ± SD. **p < 0.01 vs. control (if not specifically indicated).
In nematodes, treatment with 10 mM ascorbate alone could not alter the sensory perception of thermotaxis, avoidance of copper ion, chemotaxis to NaCl, and chemotaxis to diacetyl (Figures 6B–E). Moreover, after exposure to insecticides, post-treatment with 10 mM ascorbate could further significantly inhibit the damage induced by exposure to the six insecticides examined in reducing themotaxis, suppressing avoidance of copper ion, decreasing chemotaxis to NaCl, and reducing chemotaxis to diacetyl (Figures 6B–E).
Discussion
Normally, the environmental concentrations of insecticides in surface water were in the range of microgram or nanogram per liter (μg/L or ng/L) (Faria et al., 2020). This is one of the important reasons for our focus on insecticide concentrations in the range of μg/L—to determine the effect of insecticide exposure on sensory perception in this study. Besides this, according to our study, exposure to the six insecticides examined at a concentration of 1–100 mg/L caused significant lethality, but exposure to the six insecticides (0.1 mg/L) examined did not induce significant lethality (Supplementary Figure S1). Thus, the observed damage on sensory perceptions in nematodes exposed to the six insecticides examined at a concentration of ≤100 μg/L might be largely not due to the lethal effect. This is another reason for our focus on insecticide concentrations in the range of μg/L—to determine the effect of insecticide exposure on sensory perception in nematodes.
Using C. elegans as an animal model, it has been shown that exposure to certain insecticides (such as neonicotinoid insecticides) could result in oxidative stress and damage on reproduction, locomotion behaviors, and growth (Rajini et al., 2008; Han et al., 2017; Kudelska et al., 2017; Zeng et al., 2017; Bradford et al., 2020). In this study, we found that prolonged exposure to insecticides in the range of μg/L potentially caused damage on multiple aspects of sensory perception in nematodes.
Temperature is an important environmental factor for the survival of organisms. Meanwhile, temperature is also an important environmental stimulus for environmental animals to sense. C. elegans has highly sensitive and sophisticated thermosensory mechanisms to detect environmental temperatures (Takeishi et al., 2020). As shown in Figure 2, exposure to neonicotinoid insecticides potentially noticeably affected thermotaxis behavior in nematodes. Sulfoxaflor had the weakest toxic effect on thermotaxis behavior among the six insecticides examined.
Avoidance of copper ion reflects a form of gustatory perception of worms, and this form of gustatory perception is controlled by ASH sensory neurons (Wang, 2019). All the six insecticides examined (10–100 μg/L) suppressed the avoidance of copper and showed the following toxicity order: dinotefuran > thiamethoxam > thiacloprid > nitenpyram > acetamiprid > sulfoxaflor (Figure 3). The heavy metal Cu is neurotoxic for worms (Wang and Xing, 2009; Zhang et al., 2010). These observations suggested that exposure to the examined insecticides in the range of μg/L may cause the difficulty for organisms to avoid harmful compounds or noxious stimuli in the environment. It was also reported that exposure to some insecticides might adversely influence the avoidance of predation in the poultry populations (Walker, 2003).
Chemotaxis towards NaCl is another form of gustatory perception of worms, and ASE sensory neurons controlled this form of gustatory perception (Bargmann and Horvitz, 1991). Similar to the observations on thermotaxis behavior and avoidance of copper ion, these six insecticides at concentrations of 10–100 μg/L caused a remarkable deficit in chemotaxis to NaCl and exhibited the following toxicity order: dinotefuran > thiamethoxam > thiacloprid > nitenpyram > acetamiprid > sulfoxaflor (Figure 4). In honeybees, exposure to thiamethoxam also caused a deficit in sensory perception of sugar (Aliouane et al., 2009).
Chemotaxis to diacetyl reflects the ability of olfactory perception in worm, and this sensory perception is mainly controlled by AWA sensory neurons (Metaxakis et al., 2018). After exposure at concentrations of 10–100 μg/L, the toxicity order for the six insecticides examined in affecting chemotaxis to diacetyl was dinotefuran > thiamethoxam > thiacloprid > nitenpyram > acetamiprid > sulfoxaflor (Figure 5). These observations demonstrated that, in worms exposed to the examined insecticides in the range of μg/L, both gustatory perception and olfactory perception would be noticeably damaged. Besides the four forms of sensory perceptions examined, there are also some other forms of sensory perceptions in C. elegans (Metaxakis et al., 2018). The possible effects of exposure to the examined insecticides on other forms of sensory perceptions still remain unclear in nematodes.
After exposure, 100 μg/L of the six insecticides examined obviously decreased the locomotion behavior as reflected by the endpoint of body bend (Supplementary Figure S2). In contrast, dinotefuran, thiamethoxam, thiacloprid, nitenpyram, acetamiprid, and sulfoxaflor at concentrations of ≤100 μg/L did not affect the locomotion behavior (Supplementary Figure S2). Therefore, the observed damage of the six insecticides examined at concentrations ≤100 μg/L on sensory perceptions was not due to the deficit in locomotion behavior.
In nematodes, exposure to insecticides (such as lindane) could induce oxidative stress (Yu et al., 2021). Ascorbate is a normally used antioxidant against oxidative stress in nematodes (Li et al., 2012). We further found that post-treatment with the antioxidant ascorbate could effectively suppress damage by the six insecticides examined in reducing themotaxis, in suppressing avoidance of copper ion, in decreasing chemotaxis toward NaCl, and in reducing chemotaxis toward diacetyl (Figures 6B–E). Meanwhile, post-treatment with the antioxidant ascorbate could also obviously suppress the ROS production induced by exposure to the six insecticides examined (Figure 6A). Therefore, the activation of oxidative stress can act as an important cellular contributor to the observed damage of the examined insecticides in affecting sensory perceptions. In nematodes, the ROS signals are mainly detected in the pharynx and the intestinal cells (Yang et al., 2021a). This implied that the examined insecticides might potentially bind to certain group(s) of biomolecules so as to induce oxidative damage in primary biological barriers, such as pharynx barrier, in nematodes. After that, neurotoxicity on sensory perceptions will be further induced after insecticide exposure—that is, the damage on the examined four forms of sensory perceptions might be largely due to the fact that both sulfoxaflor and neonicotinoid insecticides could cause the activation of oxidative stress. This is also helpful to explain the fact that the sensory perceptions were affected in the same way by the examined insecticides.
The possible adverse effects of insecticides at various aspects have also been determined with other organisms, such as Daphnia magna and Ceriodaphnia dubia (Sheets et al., 2016; Raby et al., 2018). Moreover, it was observed that exposure to neonicotinoid insecticides could result in developmental neurotoxicity (Sheets et al., 2016)—that is, at least at high concentrations, the observed damage on sensory perceptions to temperature, copper ion, NaCl, and diacetyl might also be possibly due to the developmental deficits in related neurons and/or neuronal circuit(s) governing these sensory perceptions in nematodes.
Conclusion
The potential damage on sensory perceptions by exposure to six insecticides (dinotefuran, thiamethoxam, thiacloprid, nitenpyram, acetamiprid, and sulfoxaflor) was compared in C. elegans. Exposure to these insecticides at concentrations of 10–100 μg/L caused severe deficits in sensory perceptions to temperature, copper ion, NaCl, and diacetyl. Activation of oxidative stress acted as an important cellular mechanism for the observed damage of the six insecticides examined in affecting sensory perceptions. Therefore, our results suggested the potential of long-term exposure to the examined insecticides in the range of μg/L in inducing damage on sensory perceptions in organisms.
Data Availability Statement
The original contributions presented in the study are included in the article/Supplementary Material, further inquiries can be directed to the corresponding author.
Author Contributions
RZ and YY contributed to study design, experimentation, and initial draft writing. WZ and DW contributed to experimentation. YB and YW contributed to data analysis and result visualization. YB took charge of funding acquisition. All authors reviewed the final version of the manuscript and approve it for publication.
Funding
This study was funded by the National Key Research and Development Program of China (No. 2018YFC1801105), the Fundamental Research Fund of Central Public Welfare Research Institutions in 2019 (Innovative Team Research Project of New Approach and Application on Substitution Toxicology of Environmental Hormone Substance), and the Open Project of State Environmental Protection Key Laboratory of Pesticide Environmental Assessment and Pollution Control. All sources of funding received for the research have been submitted.
Conflict of Interest
The authors declare that the research was conducted in the absence of any commercial or financial relationships that could be construed as a potential conflict of interest.
Publisher’s Note
All claims expressed in this article are solely those of the authors and do not necessarily represent those of their affiliated organizations or those of the publisher, the editors and the reviewers. Any product that may be evaluated in this article or claim that may be made by its manufacturer is not guaranteed or endorsed by the publisher.
Supplementary Material
The Supplementary Material for this article can be found online at: https://www.frontiersin.org/articles/10.3389/fenvs.2022.859356/full#supplementary-material
References
Aliouane, Y., El Hassani, A. K., Gary, V., Armengaud, C., Lambin, M., and Gauthier, M. (2009). Subchronic Exposure of Honeybees to Sublethal Doses of Pesticides: Effects on Behavior. Environ. Toxicol. Chem. 28, 113–122. doi:10.1897/08-110.1
Ardiel, E. L., and Rankin, C. H. (2010). An Elegant Mind: Learning and Memory in Caenorhabditis Elegans. Learn. Mem. 17, 191–201. doi:10.1101/lm.960510
Bargmann, C. I., and Horvitz, H. R. (1991). Chemosensory Neurons with Overlapping Functions Direct Chemotaxis to Multiple Chemicals in C. Elegans. Neuron 7, 729–742. doi:10.1016/0896-6273(91)90276-6
Bargmann, C. I., Hartwieg, E., and Horvitz, H. R. (1993). Odorant-selective Genes and Neurons Mediate Olfaction in C. Elegans. Cell 74, 515–527. doi:10.1016/0092-8674(93)80053-h
Bargmann, C. I. (1998). Neurobiology of the Caenorhabditis E Genome. Science 282, 2028–2033. doi:10.1126/science.282.5396.2028
Bradford, B. R., Whidden, E., Gervasio, E. D., Checchi, P. M., and Raley-Susman, K. M. (2020). Neonicotinoid-Containing Insecticide Disruption of Growth, Locomotion, and Fertility in Caenorhabditis E. PLoS One 15, e0238637. doi:10.1371/journal.pone.0238637
Brenner, S. (1974). The Genetics of Caenorhabditis Elegans. Genetics 77, 71–94. doi:10.1093/genetics/77.1.71
Cordova, D., Benner, E. A., Sacher, M. D., Rauh, J. J., Sopa, J. S., Lahm, G. P., et al. (2006). Anthranilic Diamides: A New Class of Insecticides with a Novel Mode of Action, Ryanodine Receptor Activation. Pestic. Biochem. Physiol. 84, 196–214. doi:10.1016/j.pestbp.2005.07.005
Corsi, A. K., Wightman, B., and Chalfie, M. (2015). A Transparent Window into Biology: A Primer on Caenorhabditis Elegans. Genetics 200, 387–407. doi:10.1534/genetics.115.176099
Du, H., Wang, M., Wang, L., Dai, H., Wang, M., Hong, W., et al. (2015). Reproductive Toxicity of Endosulfan: Implication from Germ Cell Apoptosis Modulated by Mitochondrial Dysfunction and Genotoxic Response Genes in Caenorhabditis Elegans. Toxicol. Sci. 145, 118–127. doi:10.1093/toxsci/kfv035
Faria, M., Wu, X., Luja-Mondragón, M., Prats, E., Gómez-Oliván, L. M., Piña, B., et al. (2020). Screening Anti-predator Behaviour in Fish Larvae Exposed to Environmental Pollutants. Sci. Total Environ. 714, 136759. doi:10.1016/j.scitotenv.2020.136759
Gomez-Eyles, J. L., Svendsen, C., Lister, L., Martin, H., Hodson, M. E., and Spurgeon, D. J. (2009). Measuring and Modelling Mixture Toxicity of Imidacloprid and Thiacloprid on Caenorhabditis Elegans and Eisenia F. Ecotoxicology Environ. Saf. 72, 71–79. doi:10.1016/j.ecoenv.2008.07.006
Griffin, E. F., Caldwell, K. A., and Caldwell, G. A. (2017). Genetic and Pharmacological Discovery for Alzheimer's Disease Using Caenorhabditis E. ACS Chem. Neurosci. 8, 2596–2606. doi:10.1021/acschemneuro.7b00361
Guo, L., Li, C., Liang, P., and Chu, D. (2019). Cloning and Functional Analysis of Two Ca2+-Binding Proteins (CaBPs) in Response to Cyantraniliprole Exposure in Bemisia Tabaci (Hemiptera: Aleyrodidae). J. Agric. Food Chem. 67, 11035–11043. doi:10.1021/acs.jafc.9b04028
Haegerbaeumer, A., Höss, S., Heininger, P., and Traunspurger, W. (2018). Is Caenorhabditis Elegans Representative of Freshwater Nematode Species in Toxicity Testing? Environ. Sci. Pollut. Res. 25, 2879–2888. doi:10.1007/s11356-017-0714-7
Han, Y., Song, S., Wu, H., Zhang, J., and Ma, E. (2017). Antioxidant Enzymes and Their Role in Phoxim and Carbaryl Stress in Caenorhabditis E. Pestic. Biochem. Physiol. 138, 43–50. doi:10.1016/j.pestbp.2017.02.005
Kudelska, M. M., Holden-Dye, L., O'Connor, V., and Doyle, D. A. (2017). Concentration-dependent Effects of Acute and Chronic Neonicotinoid Exposure on the Behaviour and Development of the nematodeCaenorhabditis Elegans. Pest Manag. Sci. 73, 1345–1351. doi:10.1002/ps.4564
Leung, M. C. K., Williams, P. L., Benedetto, A., Au, C., Helmcke, K. J., Aschner, M., et al. (2008). Caenorhabditis Elegans: An Emerging Model in Biomedical and Environmental Toxicology. Toxicol. Sci. 106, 5–28. doi:10.1093/toxsci/kfn121
Li, Y.-X., Wang, Y., Hu, Y.-O., Zhong, J.-X., and Wang, D.-Y. (2011). Modulation of the Assay System for the Sensory Integration of 2 Sensory Stimuli that Inhibit Each Other in Nematode Caenorhabditis Elegans. Neurosci. Bull. 27, 69–82. doi:10.1007/s12264-011-1152-z
Li, Y., Yu, S., Wu, Q., Tang, M., Pu, Y., and Wang, D. (2012). Chronic Al2O3-Nanoparticle Exposure Causes Neurotoxic Effects on Locomotion Behaviors by Inducing Severe ROS Production and Disruption of ROS Defense Mechanisms in Nematode Caenorhabditis E. J. Hazard. Mater. 219-220, 221–230. doi:10.1016/j.jhazmat.2012.03.083
Liu, H., Tian, L., Wang, S., and Wang, D. (2021a). Size-Dependent Transgenerational Toxicity Induced by Nanoplastics in Nematode Caenorhabditis Elegans. Sci. Total Environ. 790, 148217. doi:10.1016/j.scitotenv.2021.148217
Liu, H., Qiu, Y., and Wang, D. (2021b). Alteration in Expressions of Ion Channels in Caenorhabditis Elegans Exposed to Polystyrene Nanoparticles. Chemosphere 273, 129686. doi:10.1016/j.chemosphere.2021.129686
Liu, H., Zhao, Y., Bi, K., Rui, Q., and Wang, D. (2021c). Dysregulated Mir-76 Mediated a Protective Response to Nanopolystyrene by Modulating Heme Homeostasis Related Molecular Signaling in Nematode Caenorhabditis Elegans. Ecotoxicology Environ. Saf. 212, 112018. doi:10.1016/j.ecoenv.2021.112018
Matsuda, K., Shimomura, M., Ihara, M., Akamatsu, M., and Sattelle, D. B. (2005). Neonicotinoids Show Selective and Diverse Actions on Their Nicotinic Receptor Targets: Electrophysiology, Molecular Biology, and Receptor Modeling Studies. Biosci. Biotechnol. Biochem. 69, 1442–1452. doi:10.1271/bbb.69.1442
Metaxakis, A., Petratou, D., and Tavernarakis, N. (2018). Multimodal Sensory Processing in Caenorhabditis Elegans. Open Biol. 8, 180049. doi:10.1098/rsob.180049
Moy, T. I., Conery, A. L., Larkins-Ford, J., Wu, G., Mazitschek, R., Casadei, G., et al. (2009). High-Throughput Screen for Novel Antimicrobials Using a Whole Animal Infection Model. ACS Chem. Biol. 4, 527–533. doi:10.1021/cb900084v
Qi, S., Wang, D., Zhu, L., Teng, M., Wang, C., Xue, X., et al. (2018). Effects of a Novel Neonicotinoid Insecticide Cycloxaprid on Earthworm, Eisenia F. Environ. Sci. Pollut. Res. 25, 14138–14147. doi:10.1007/s11356-018-1624-z
Qu, M., and Wang, D. (2020). Toxicity Comparison between Pristine and Sulfonate Modified Nanopolystyrene Particles in Affecting Locomotion Behavior, Sensory Perception, and Neuronal Development in Caenorhabditis Elegans. Sci. Total Environ. 703, 134817. doi:10.1016/j.scitotenv.2019.134817
Queirós, L., Pereira, J. L., Gonçalves, F. J. M., Pacheco, M., Aschner, M., and Pereira, P. (2019). Caenorhabditis Elegansas a Tool for Environmental Risk Assessment: Emerging and Promising Applications for a "Nobelized Worm". Crit. Rev. Toxicol. 49, 411–429. doi:10.1080/10408444.2019.1626801
Raby, M., Nowierski, M., Perlov, D., Zhao, X., Hao, C., Poirier, D. G., et al. (2018). Acute Toxicity of 6 Neonicotinoid Insecticides to Freshwater Invertebrates. Environ. Toxicol. Chem. 37, 1430–1445. doi:10.1002/etc.4088
Rajini, P. S., Melstrom, P., and Williams, P. L. (2008). A Comparative Study on the Relationship between Various Toxicological Endpoints inCaenorhabditis elegansExposed to Organophosphorus Insecticides. J. Toxicol. Environ. Health A 71, 1043–1050. doi:10.1080/15287390801989002
Riddle, D. L., Blumenthal, T., Meyer, B. J., and Priess, J. R. (1997). C. E II. 2nd edition. Cold Spring Harbor (NY): Cold Spring Harbor Laboratory Press.
Roh, J.-Y., and Choi, J. (2011). Cyp35a2 Gene Expression Is Involved in Toxicity of Fenitrothion in the Soil Nematode Caenorhabditis Elegans. Chemosphere 84, 1356–1361. doi:10.1016/j.chemosphere.2011.05.010
Ruan, Q.-L., Ju, J.-J., Li, Y.-H., Li, X.-B., Liu, R., Liang, G.-Y., et al. (2012). Chlorpyrifos Exposure Reduces Reproductive Capacity Owing to a Damaging Effect on Gametogenesis in the Nematode Caenorhabditis Elegans. J. Appl. Toxicol. 32, 527–535. doi:10.1002/jat.1783
Saeki, S., Yamamoto, M., and Iino, Y. (2001). Plasticity of Chemotaxis Revealed by Paired Presentation of a Chemoattractant and Starvation in the Nematode Caenorhabditis Elegans. J. Exp. Biol. 204, 1757–1764. doi:10.1242/jeb.204.10.1757
Sambongi, Y., Takeda, K., Wakabayashi, T., Ueda, I., Wada, Y., and Futai, M. (2000). Caenorhabditis E Senses Protons through Amphid Chemosensory Neurons. Neuroreport 11, 2229–2232. doi:10.1097/00001756-200007140-00033
Sheets, L. P., Li, A. A., Minnema, D. J., Collier, R. H., Creek, M. R., and Peffer, R. C. (2016). A Critical Review of Neonicotinoid Insecticides for Developmental Neurotoxicity. Crit. Rev. Toxicol. 46, 153–190. doi:10.3109/10408444.2015.1090948
Sun, L., Li, D., Yuan, Y., and Wang, D. (2021). Intestinal Long Non-coding RNAs in Response to Simulated Microgravity Stress in Caenorhabditis Elegans. Sci. Rep. 11, 1997. doi:10.1038/s41598-021-81619-4
Takeishi, A., Takagaki, N., and Kuhara, A. (2020). Temperature Signaling Underlying Thermotaxis and Cold Tolerance in Caenorhabditis Elegans. J. Neurogenet. 34, 351–362. doi:10.1080/01677063.2020.1734001
Walker, C. H. (2003). Neurotoxic Pesticides and Behavioural Effects upon Birds. Ecotoxicology 12, 307–316. doi:10.1023/a:1022523331343
Wang, D., and Xing, X. (2009). Pre-Treatment with Mild Metal Exposure Suppresses the Neurotoxicity on Locomotion Behavior Induced by the Subsequent Severe Metal Exposure in Caenorhabditis Elegans. Environ. Toxicol. Pharmacol. 28, 459–464. doi:10.1016/j.etap.2009.07.008
Wang, S., Zhang, R., and Wang, D. (2021a). Induction of Protective Response to Polystyrene Nanoparticles Associated with Methylation Regulation in Caenorhabditis Elegans. Chemosphere 271, 129589. doi:10.1016/j.chemosphere.2021.129589
Wang, S., Liu, H., Qu, M., and Wang, D. (2021b). Response of Tyramine and Glutamate Related Signals to Nanoplastic Exposure in Caenorhabditis Elegans. Ecotoxicology Environ. Saf. 217, 112239. doi:10.1016/j.ecoenv.2021.112239
Wang, D. Y. (2019). Target Organ Toxicology in Caenorhabditis E. Singapore: Springer Nature Singapore Pte Ltd.
Wang, D. Y. (2020). Exposure Toxicology in Caenorhabditis E. Singapore: Springer Nature Singapore Pte Ltd.
Yang, Y., Wu, Q., and Wang, D. (2021a). Dysregulation of G Protein-Coupled Receptors in the Intestine by Nanoplastic Exposure in Caenorhabditis E. Environ. Sci. Nano 8, 1019–1028. doi:10.1039/d0en00991a
Yang, Y., Dong, W., Wu, Q., and Wang, D. (2021b). Induction of Protective Response Associated with Expressional Alterations in Neuronal G Protein-Coupled Receptors in Polystyrene Nanoparticle Exposed Caenorhabditis Elegans. Chem. Res. Toxicol. 34, 1308–1318. doi:10.1021/acs.chemrestox.0c00501
Ye, H.-Y., Ye, B.-P., and Wang, D.-Y. (2008). Evaluation of the Long-Term Memory for Thermosensation Regulated by Neuronal Calcium Sensor-1 in Caenorhabditis Elegans. Neurosci. Bull. 24, 1–6. doi:10.1007/s12264-008-0920-x
Yu, Y., Hua, X., Chen, H., Wang, Y. e., Li, Z., Han, Y., et al. (2020). Toxicity of Lindane Induced by Oxidative Stress and Intestinal Damage in Caenorhabditis Elegans. Environ. Pollut. 264, 114731. doi:10.1016/j.envpol.2020.114731
Yu, Y., Chen, H., Hua, X., Wang, Z., Li, L., Li, Z., et al. (2021). Long-Term Toxicity of Lindane through Oxidative Stress and Cell Apoptosis in Caenorhabditis Elegans. Environ. Pollut. 272, 116036. doi:10.1016/j.envpol.2020.116036
Zeng, R., Yu, X., Tan, X., Ye, S., and Ding, Z. (2017). Deltamethrin Affects the Expression of Voltage-Gated Calcium Channel α1 Subunits and the Locomotion, Egg-Laying, Foraging Behavior of Caenorhabditis E. Pestic. Biochem. Physiol. 138, 84–90. doi:10.1016/j.pestbp.2017.03.005
Zhang, Y., Ye, B., and Wang, D. (2010). Effects of Metal Exposure on Associative Learning Behavior in Nematode Caenorhabditis E. Arch. Environ. Contam. Toxicol. 59, 129–136. doi:10.1007/s00244-009-9456-y
Keywords: neurotoxicity, sensory perception, insecticides, Caenorhabditis elegans, oxidative stress
Citation: Zhou R, Yu Y, Zhang W, Wang D, Bai Y, Wang Y and Bu Y (2022) Sensory Disturbance by Six Insecticides in the Range of μg/L in Caenorhabditis elegans. Front. Environ. Sci. 10:859356. doi: 10.3389/fenvs.2022.859356
Received: 21 January 2022; Accepted: 17 February 2022;
Published: 24 March 2022.
Edited by:
Liangang Mao, Institute of Plant Protection (CAAS), ChinaReviewed by:
Neha Vijay Kalmankar, National Centre for Biological Sciences, IndiaQi Rui, Nanjing Agricultural University, China
Copyright © 2022 Zhou, Yu, Zhang, Wang, Bai, Wang and Bu. This is an open-access article distributed under the terms of the Creative Commons Attribution License (CC BY). The use, distribution or reproduction in other forums is permitted, provided the original author(s) and the copyright owner(s) are credited and that the original publication in this journal is cited, in accordance with accepted academic practice. No use, distribution or reproduction is permitted which does not comply with these terms.
*Correspondence: Yuanqing Bu, YnlxQG5pZXMub3Jn
†These authors have contributed equally to this work and share first authorship