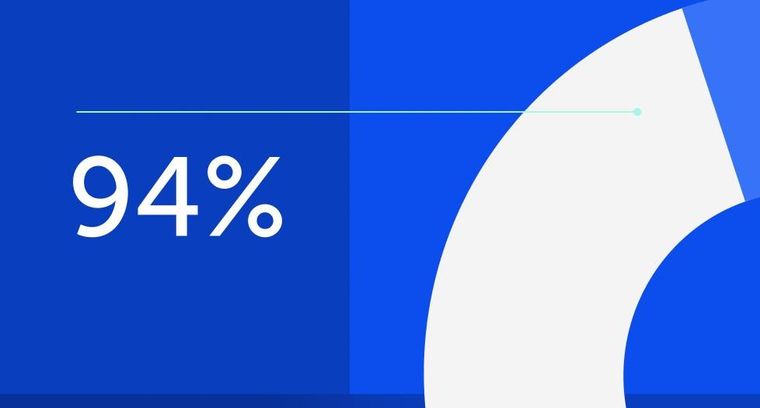
94% of researchers rate our articles as excellent or good
Learn more about the work of our research integrity team to safeguard the quality of each article we publish.
Find out more
ORIGINAL RESEARCH article
Front. Environ. Sci., 01 April 2022
Sec. Environmental Informatics and Remote Sensing
Volume 10 - 2022 | https://doi.org/10.3389/fenvs.2022.848085
Land use and land cover (LULC) change can have detrimental effects on water quality. In Belize, agricultural expansion creates the risk of increased sediment load and excess nutrients in runoff water, while deforestation removes potential infiltration sites for this outflow. Climate change and evolving precipitation rates can intensify the quantity of runoff, further enabling the flow of sediments and excess nutrients out to the lagoon surrounding the Belize Barrier Reef Reserve System (BBRRS). This study sought to estimate potential impacts on future water quality in Belize by first modeling LULC change through 2090 across Belize’s major watersheds based on observed trends from 2008 to 2018. Those LULC projections were subsequently combined with soil type data, elevation, and precipitation rates into a hydrologic model to produce runoff flow estimates as a proxy for water quality. The two Coupled Model Intercomparison Project phase 6 (CMIP-6) scenarios employed in the study represented bookend climate change scenarios, and both indicated generally lower precipitation rates in Belize over the next century due to climate change. The most extreme scenario predicted a 46% decrease in precipitation. When holding LULC change constant, these climate scenarios projected a decrease in runoff, suggesting a positive relationship between precipitation and runoff. In contrast with the northern watersheds, the southern watersheds are projected to experience greater decreases in annual rainfall and runoff by 2090. When holding climate constant, runoff increased by approximately 2.8% in the Conservation-focused LULC scenario by 2090, which was 28% lower than the Business as Usual scenario, and 42% lower than the Development scenario. The study’s integration of CMIP6 climate scenarios into LULC and hydrologic modeling provides a more holistic view of the future of Belize’s water quality and supports the long-term planning efforts of local decision-making agencies.
The environmental and economic impacts of runoff and water pollution in Belize are high given the ecological importance of the Belize Barrier Reef Reserve System (BBRRS), the second largest barrier reef in the world. This highlights the need for a comprehensive understanding of both future land use and land cover (LULC) conversions and climate change trends in Belize. In terms of LULC, expanding agricultural practices and climate change impacts have lasting environmental consequences at the expense of forests worldwide (Hecht, 1993; Graesser et al., 2015; Lawrence and Vandecar, 2015). In Belize, it is estimated that approximately 60% of approximately 9,500 registered farmers are smallholder farmers, many of whom practice milpa agriculture (Ramirez et al., 2013). Milpa agriculture traditionally involves intensively cultivating a plot of land and subsequently leaving that land fallow for 8–10 years to allow for soil regeneration (Richardson, 2009; Drexler, 2020). In recent years, Belizean farmers have shortened the fallow period to approximately 2–3 years, and the resulting soil degradation has heightened issues of erosion and agricultural runoff (Arnason et al., 1982; Ramirez et al., 2013). Belize’s largest export crops consist of sugarcane, citrus, and bananas, with the former being grown on large-scale plantations that make heavy use of chemical inputs that have enduring impacts on water and soil health (Cherrington et al., 2010; Ramirez et al., 2013; Chicas et al., 2015). Additionally, global aggregate demand for meat is projected to grow at a rate of 1.3% until 2050 (Bruinsma, 2003). The amount of pasture land and production worldwide have increased in response to this rising demand (Wassenaar et al., 2007; Patterson, 2016), including in Belize (Merrill, 2010). The impacts of deforestation and pasture expansion on soil erosivity and runoff have been well documented (Sanchez et al., 2002; Merten and Minella, 2013). Evolving demands from both local and global markets have the potential to dictate LULC conversions and impact the runoff outflow in Belize.
Forests can combat issues of erosivity and runoff, as they act as a natural water filter as tree roots absorb nutrient pollution that may travel from nearby agricultural areas. Trees utilize the nitrogen and phosphorus for growth which would otherwise have washed into the nearby water sources which can result in degraded water quality and harmful algal blooms. According to Lee 2009, there were significant decreases in excess nutrients once agricultural runoff passed through wooded areas. Forests also slow the movement of water over land. Tree canopies can slow the rate at which falling precipitation reaches the surface and the high porosity soils soak up much of the water that does make its way to the surface (Neary et al., 2009). Deforestation removes these natural filtration systems, leaving the rivers susceptible to increased pollution and erosion.
Climate change impacts may also exacerbate water quality issues (Costa and Foley, 1997; Arnell et al., 2003; Gómez-Martínez et al., 2021). For instance, much of Belize’s coast lies at sea level, leaving the area particularly susceptible to sea-level rise, severe storm surges, and coastal erosion. The small nation ranks within the top five percent of 182 countries in the German Watch’s Climate Risk Index in terms of economic losses caused by climate-related disasters (Fund, 2018). Additionally, Belize is located in the tropics; while precipitation rates are generally increasing in the northern hemisphere, there has been a negative trend in tropical regions (Neelin et al., 2006). Studies based on precipitation trends between 1960 and 2005 indicate that Belize is projected to experience an average decrease of 120.5 millimeters per year. Along with decreasing precipitation, the Central American region is also projected to see an increase in average temperature of 3.5 degrees Celsius by 2099 (Richardson, 2009). This increase in temperature combined with less precipitation leaves the region at a higher risk of drought. The increased risk of tropical storms of greater intensity in Belize can further worsen the runoff potential of the region, where degraded soils are vulnerable to erosion (Richardson, 2009).
As was previously mentioned, increasing runoff and decreasing water quality can also directly impact the BBRRS (Cho, 2005). Starting only a few hundred meters from the mainland coast, the BBRRS was listed as a UNESCO World Heritage Site in 1996. It was placed on the List of World Heritage in Danger in 2009 mainly due to the threats posed by mangrove cutting and the facilitation of sale, lease, and development of lands within the property, to which concerns about offshore oil concessions were added a year later (Gibson, 2011; Gould, 2017). In 2017, Belize became the first country to combat the threat of offshore drilling by imposing a moratorium on petroleum exploration in the maritime zone of Belize (Root, 2018). The BBRRS was removed from the List of World Heritage in Danger in 2018 and that same year, it enacted mangrove protection regulations (IUCN, 2018).
Despite the protections and moratorium in place, the barrier reef is subject to environmental threats. Like coral reefs in general, the BBRRS is sensitive to water temperature, chemistry, microbial threats, and clarity. High temperatures and excess amounts of nutrients in the water can cause them to expel their symbiotic algae and turn white, a phenomenon known as coral bleaching (Hoegh-Guldberg, 1999; Loka et al., 2001; Donovan et al., 2020). Decreases in coral richness, abundance, diversity, density, and percent cover has been documented in the BBRRS in areas with higher temperature regimes as well as increases in mass bleaching events (Baumann et al., 2016; Baumann et al., 2019). Sediments and high turbidity in the water can also inhibit sunlight, a necessary input for many of the organisms in the reef (Goatley et al., 2016). Anthropogenic pollution sources previously investigated in Belize include microfibers in coral tissues in the BBRRS (Soto et al., 2009; Oldenburg et al., 2021), marine traffic (Callejas et al., 2021), and nutrients from riverine runoff (Soto et al., 2009; Lapointe et al., 2021). Reducing marine pollution, including runoff containing land-based nutrients and other pollutants, is Target 14.1 of the United Nations Sustainable Development Goal 14 “Life Below Water” (United Nations, 2018).
The fragility of the reef makes long-term coastal planning an integral part of its conservation. In 1998, the government of Belize passed the Coastal Zone Management Act to mitigate issues surrounding rapid development, overfishing, and rapid population growth (CZMAI, 2016). CZMAI is the leading authority in the nation’s management of coastal resources and is responsible for the development of the mandated Integrated Coastal Zone Management Plan (ICZMP). The goal of the ICZMP involves providing a set of recommended actions to ensure sustainable use of coastal resources with conservation and social and economic needs in mind. It provides a planning framework for national action to improve management of coastal and marine resources, in order to maintain their integrity and ecosystem service benefits (CZMAI, 2016). The plan was approved by national government in 2016 and requires updating every 4 years. The most recent ICZP released in 2016 developed projected scenarios for 2025 based on three approaches: conservation, informed management, and development. Studies investigating anthropogenic impacts to water quality and marine resources is vital to CZMAI’s long-term coastal planning and management.
With the importance of LULC and climate change impacts in mind, the objectives of this study were to 1) model three different land use and land cover LULC change scenarios from 2030 to 2090, 2) integrate those LULC products with climate change predictions in the Nonpoint Source Pollution and Erosion Comparison Tool (N-SPECT), and 3) produce predicted runoff outputs through 2090 to assess water quality at the discharge sites (pour points) of Belize’s watersheds (Eslinger et al., 2012). The integration of the CMIP6 climate projections into the hydrologic modeling contributes to the relevance of the model outputs to ongoing long-term planning in Belize.
The study area consisted of the full extent of Belize’s watersheds, including most of mainland Belize, and the headwaters of some transboundary watersheds that are located in Mexico’s Yucatan Peninsula and in northeastern Guatemala (Figure 1). Fourteen of the watersheds analyzed were major and one was minor, and the selection of these particular watersheds for analysis was largely due to their proximity to the locations of our pourpoints. Belize is a sparsely populated country of less than 400,000 people with an area of about 2.297 million hectares (Factbook, 2020). It experiences a subtropical climate with an average of 1,500 mm of annual precipitation (National Meteorological Service of Belize, 2022 ). It is also one of the more heavily forested countries in the Latin American and Caribbean (LAC) region, with 62.7% forest cover (Cherrington et al., 2010). Furthermore, 36.6% of Belize’s terrestrial territory and 19.8% of its marine territory are designated protected areas (Mitchell et al., 2017). The clearing of Belize’s forests and the downstream degradation of the BBRRS could negatively affect tourism, one of its largest industries, as the BBRRS is one of the primary tourist attractions to the country (Nuenninghoff et al., 2015).
FIGURE 1. Map of the study area extent, including elevation (meters above sea level), watershed boundaries, waterways, and pour points of major rivers (i.e., where the rivers discharge into the sea).
This study comprised three main analyses (Figure 2). First, the LULC from 2008 to 2018 was projected over the study area using Clark Labs’ Terrset Land Change Modeler to discover historic trends and model future LULC scenarios (Eastman, 2020). The second portion consisted of employing N-SPECT, a hydrologic model by the National Oceanic and Atmospheric Administration (NOAA) that incorporated the LULC projections along with data on soil types, elevation, and precipitation rates that reflect climate change impacts in order to generate runoff estimates. The third was to investigate all combinations of LULC change scenarios and climate change scenarios. The 2030–2090 climate change model runs were sourced from the Coupled Model Intercomparison Project Phase 6 (CMIP6). The resulting runoff estimates functioned as a proxy for water quality.
Historical LULC maps and input variables were developed in order to run the LULC model in the Land Change Modeler (LCM) platform. The LCM platform is a “land planning and decision support system” that enables users to easily model land cover change, perform driver variable selection, and conduct statistical analyses of the modeled changes (Eastman, 2020). A 500-meter annual LULC product (MCD12Q1) derived from the Moderate Resolution Imaging Spectroradiometer (MODIS) was employed to produce 2008 and 2018 LULC maps (Friedl and Sulla-Menashe, 2018). This product was selected given its availability from 2000 to the present, as well as end user decision-making agencies’ familiarity with the product and its ability to represent the general landscape of our large study region. The International Geosphere-Biosphere Programme (IGBP) classification product was acquired through the cloud-based Google Earth Engine platform (Gorelick et al., 2017). The six classes selected for this study from the 17 available in the IGBP scheme were also compatible with the National Oceanic and Atmospheric Agency’s (NOAA) Coastal Change Analysis Program (C-CAP) classes, necessary for the subsequent water quality assessment in N-SPECT (Table 1). The IGBP classification was reclassified accordingly in ArcGIS Desktop 10.7. A value of 0 was defined as the background value for both LULC maps before they were converted to float-type rasters and imported into the LCM software, where they were converted to TerrSet’s proprietary.rst files for use in the model. The watershed boundaries were sourced from the Biodiversity and Environmental Resource System of Belize (Meerman and Clabaugh, 2017).
TABLE 1. International Geosphere–Biosphere Programme (IGBP) classification scheme reclassified to the six classes for this study’s assessment.
In order to consider the effects of protected areas in the model, the parameters were initially set to only allow land cover change to occur outside of the protected area boundaries. Within sustainable mixed-use areas, some limited changes were allowed within the boundaries. The International Union for Conservation of Nature (IUCN) categories of regulatory levels were used to identify mixed-use areas (UNEPWCMC and IUCN, 2020). National protected area shapefiles for Mexico and Guatemala were acquired from Protected Planet and subset to the study area (Lawrence and Vandecar, 2015). Protected area shapefiles for Belize were acquired from the Biodiversity and Environmental Resource Data System of Belize (Meerman and Clabaugh, 2017), as these data also included privately managed protected areas. Input variables for LULC modeling were selected based on previous LULC modeling work and the consideration of historic and current trends specific to the study region (Cherrington et al., 2020). Except for slope, all variables were in the form of Euclidean Distance, defined as the straight distance from one point to another (Goncalves et al., 2014). The following assumptions led to the selection of the aforementioned input variables (Table 2) (E. Cherrington, personal communication, November 2020):
1) Once a fire used for clearing vegetation was present in an area, it was more likely that said area would not be converted to anything other than crop or pastureland (Morton et al., 2008; Nelson and Chomitz, 2011).
2) Areas surrounding previously cleared areas such as agriculture were more vulnerable to being cleared; consequently, a separate variable was created for areas that were cleared by 2008 (Guild et al., 2004; Morton et al., 2008).
3) Areas near roads or large population centers were assumed to be more likely to be deforested (Mishra et al., 2014; Chomitz and Gray, 2017).
4) Areas with shallow or moderate slopes were also considered more vulnerable to deforestation given that these areas are more suitable to agriculture (Mishra et al., 2014; Gupta and Sharma, 2020).
After the selection of input variables, a transition sub-model was run in Terrset. Transition sub-models are defined as a set of land cover transitions that are influenced by the same drivers of LULC change. Running submodels is necessary to produce the regression equations that define historical changes and that will influence future scenarios (Rodriguez Eraso et al., 2012; Eastman and He, 2020). The Multi-layer Perceptron (MLP) neural network was employed given its capacity to perform well over multiple transition sub-models. The sample size for the model is automatically set to the smallest number of pixels that were converted from one LULC type to another (Sangermano et al., 2010). Although larger sample sizes are typically desired in modelling, artificially setting the sample size to a number larger than the default could result in a skewed training process (Wisz et al., 2008).
For this study, the transitions from Forest to Pasture and Forest to Cropland were included in one transition submodel. Given the physical and hydrological differences between the Pasture and Cropland classes (Guild et al., 2004; Wassenaar et al., 2007), it was important that the model consider changes in both classes so these could be reflected in the modeled future scenarios. Following best practices from previous LULC studies like Wassenaar et al. (2007), some classes were held constant (Urban and Water). The 2008 and 2018 land cover maps demonstrated an apparent loss in the urban class that was due to the initial classification of the “barren” IGBP class within the larger urban class. When classified separately, there was a large decrease of pixels in the barren class, and only 5 pixels in the decadal time series transitioning to the urban class. The model only achieved an overall accuracy of approximately 33% when including transitions in the urban class due to its small sample size, so this class was held constant to focus the model exclusively on the transition from forest to pasture and cropland. It was assumed that there would be little to no expansion or conversion of water bodies. The training and testing data were split so that 50% was for training and 50% was for testing.
Based on commonly used scenarios in other studies, three future LULC scenarios were created that were each defined by different deforestation rates (Merten and Minella, 2013; Ter Steege et al., 2015). These included Conservation, Business as Usual, and Development. The Business as Usual scenario reflected Belize’s status quo annual deforestation rate of 0.6% as defined by Cherrington et al. (2010); in order to create conservative bookend scenarios, the Conservation scenario was defined as a 0.4% annual deforestation rate and was meant to reflect greater environmental protections; and Development scenario reflected an agenda that prioritized economic development and was defined as a 0.8% annual deforestation rate. Future projections of LULC for each of the three scenarios were run for the years 2030, 2050, 2070, and 2090, and outputs for each scenario were converted into hectares to obtain the net change in LULC.
The N-SPECT model requires land cover, precipitation, elevation, soil characteristics, and rainfall factor inputs in order to calculate runoff accumulation (Eslinger et al., 2012). While N-SPECT can output sediment load, Khalili et al. (2015) found that N-SPECT overestimated sediment output by a factor of 3, making sediment outputs unreliable. However, the model was found to be very suitable for runoff volume estimation. It uses the SCS curve number grid to assign a level of infiltration capacity to the soil in order to generate runoff estimates, and it operates on the level of the entire watershed. The projected land cover outputs from the previous section were employed along with elevation derived from the Shuttle Radar Topography Mission (SRTM) at a 90-meter spatial resolution (Lehner et al., 2006). Soil profiles detailing soil infiltration rates were obtained from the Food and Agriculture Organization through the Soil and Terrain Database for Latin America and the Caribbean (SOTERLAC), following best practices set by several other studies on soil erosion in Belize (FAO et al., 1998; Burke and Sugg, 2006; Cherrington et al., 2015; Chicas et al., 2015). Historic precipitation data for 2001, 2008, and 2018 were sourced from the Climate Hazards Center InfraRed Precipitation with Station data (CHIRPS), which is a quasi-global rainfall dataset that incorporates ancillary climatology data, in situ rain station data, and satellite imagery (Funk et al., 2014).
Climate projections for the future 2030–2090 runs were sourced from the Coupled Model Intercomparison Project phase 6 (CMIP-6) of the Inter-governmental Panel on Climate Change, IPCC (Swart et al., 2019), which is a comparison of global climate models that perform experiments to better understand past, present, and future climate changes. These changes can be caused by natural or anthropogenic forces. The global climate models available in this project were modeled using different Shared Socioeconomic Pathways (SSP) and Representative Concentration Pathways (RCP) scenarios, which combine socioeconomic data and land use information with climatic changes through emissions (Swart et al., 2019). From these combinations, the following scenarios were selected: SSP1 RCP2.6 (SSP126), and SSP5 RCP8.5 (SSP585) for this analysis (Table 3). The CanESM5 global climate model was selected because this model had the largest range of precipitation averages between the SSP extremes.
Bilinear resampling was applied to the 500-meter spatial resolution LULC datasets, the 3,600-meter spatial resolution CHIRPS datasets, and the 4,500-meter spatial resolution CMIP-6 datasets. The 39 soil groups in the soil characteristics shapefile were reclassified into 4 hydrologic groups: 1) high infiltration; 2) moderate infiltration; 3) slow infiltration; and 4) very slow infiltration. These correspond with the USGS hydrologic class soil characteristics (Eslinger et al., 2012). The SRTM elevation needed to be burned with an up-to-date river shapefile to ensure correct movement of surface water. Burning the riverways into the elevation data set corrects surface drainage patterns by adjusting the elevation grid that coincide with the vector layer. This was done by utilizing an up to date river shapefile, which was then buffered out to 5, 10, and 20 m. Each buffer was assigned a decreasing value (-25, -15, and -5, respectively) in order to add slope around each river. Watersheds were delineated in N-SPECT using the adjusted SRTM elevation dataset.
The N-SPECT model generated a curve number grid based on the soil characteristics and land cover grid, assigning a value ranging from zero to one hundred that represents the permeability of that cell. This 90-meter grid was used as an estimate for runoff depth. It then calculated the maximum potential retention (R) in inches using Eq. 1. The retention results were subsequently used to calculate initial abstraction in inches using Eq. 2. Abstraction (A) describes the loss of precipitation that can occur before runoff begins, such as water intercepted by vegetation, evaporation, and infiltration. Once these were calculated, N-SPECT used the precipitation grid to calculate annual runoff (Eq. 3). Abstraction and retention were multiplied by the average number of rain days per year, and the units of the final output were converted from inches to volumetric units (Eslinger et al., 2012). Calibration of the rain days in the model was carried out according to the methods set out in Cherrington 2015, and assured appropriate runoff calculations for the specified region [40 days (Burke and Sugg, 2006)].
After applying the testing data, the final LULC transition submodel output had an overall accuracy of 70.59%. The MLP neural network split the samples it had extracted from the LULC maps into training and testing data. The overall skill measure, defined as the measured accuracy minus the accuracy expected by chance, was approximately 0.56. Skill measure ranges from −1 to +1 and indicates the ability of the model to predict the transition or persistence of a pixel better than pure chance would (Sangermano et al., 2010). The minimum sample size of the submodel was 23 because only 23 500 m by 500 m pixels transitioned from Forest to Cropland in the study period, while there were 2,344 pixels that transitioned from Forest to Pasture. Opting to run the merged submodel rather than running only the Forest to Pasture transition led to a decrease in both overall model accuracy and skill measure. However, only the transitions included in the submodel were reflected in LULC projections and analyzing both transitions was fundamental to understanding LULC changes in Belize.
Forested areas were projected to decrease across all watersheds and scenarios (Figure 3). Agricultural expansion often visibly grew from areas that had already been dedicated to agriculture. The patterns of expansion for both pasture and cropland appear to be distinct from each other in that intrusion into forested areas appears to be caused more frequently by pasture expansion than cropland growth, an observation that is supported by other studies conducted in the Central American region (Guild et al., 2004; Wassenaar et al., 2007).
FIGURE 3. Historic and projected LULC transitions across different deforestation scenarios (BAU, CON, DEV). The Agriculture class includes Pasture and Cropland.
The Belize River and Rio Hondo River watersheds were specifically analyzed for a more localized understanding of the magnitude of deforestation projections, with these watersheds representing impacts in both northern Belize and central Belize (Figure 4). Approximately 49% of the Belize River Watershed was forested in 2018, but by 2090 under the Conservation scenario, that area is only projected to be 36%. The Business as Usual 2090 scenario predicted 32% of land would remain forested, and under the Development 2090 scenario, only 28% of land in the watershed was projected to remain forested. In the larger Rio Hondo River watershed, approximately 65% of the watershed was forested in 2018. Only 28% of land was projected to be forested under the Conservation scenario, 19% in the Business as Usual scenario, and a mere 10% of land in this watershed was projected to be forested under the Development scenario by 2090.
FIGURE 4. Belize River Watershed 2008, 2018, and 2090 (Development scenario), Rio Hondo River Watershed 2008, 2018, and 2090 (Development scenario).
The stark division between forested area and other classes like pasture, cropland, and other vegetation in the Belize River watershed could be attributed to the borders of Chiquibul National Park, a protected area that was accounted for in the model. As for the Rio Hondo River watershed, it likely experienced a greater conversion of forest to other class types given that much of Belize’s industrial agriculture, like sugar cane plantations, take place within the northern Belizean district of Orange Walk that the watershed encompasses (Campbell, 2013). For example, as of 2013, the Tower Hill Sugar Factory in Orange Walk was the only operating sugar mill in Belize (Campbell, 2013). The model posited that more change would occur around pre-existing agricultural areas. The transition to agriculture in the region outside of Belize under the 2090 scenario is predominantly due to deforestation along major roads, such as the Escarcega–Calakmul Highway 186 in Mexico (García-Contreras et al., 2011). Despite the use of similar spatial resolution products in comparable LULC studies like Schulz et al. (2017), the medium 500-meter resolution of the annual MODIS LULC product was a likely source of misclassification in the output model. A LULC classification study by Fisher et al. (2017) found that lower spatial resolution did influence overall model classification accuracy, with a 30 m Landsat model resulting in a 75.1% accuracy compared to the 82.3% accuracy achieved with their 1-m DigitalGlobe dataset. Some of the known errors or limitations within the MCD12Q1 product employed in this study consisted mainly of the underrepresentation of agricultural land (specifically cropland) in the tropics because it was mislabeled as natural vegetation. This was especially prevalent because cropland fields in the tropics are often much smaller than a 500 m MODIS pixel (Friedl and Sulla-Menashe, 2018). Additionally, some grassland areas are classified as savannas or sparse forest, leading to a possible over-representation of the other vegetation class at the expense of agriculture classes. Moreover, rotational milpa agriculture plots are typically about 0.3 ha on average, and this small size led to land classification challenges (Lopez-Ridaura et al., 2021). The secondary regrowth on these milpa plots could be misclassified as other vegetation and forest rather than as part of a rotational agriculture strategy in the MODIS product (Guild et al., 2004; Meerman et al., 2010).
Hydrologic modeling consisted of 41 model iterations that aimed to analyze the isolated and combined effects of land cover and climate change projections on future runoff estimates (Table 4). Generally, model outputs indicated a strong positive relationship between the precipitation rate and the projected runoff volume. Climate change projections across all years and scenarios for our tropical study area predicted an overall decrease in precipitation rates, ranging from 0.3 to 46.2%. Correspondingly, the majority of our model outputs demonstrated decreasing runoff estimates across all watersheds, with the typically drier northern watersheds producing less runoff than the wetter southern watersheds. In terms of land cover, the Development scenario produced the highest runoff estimates when the effects of LULC on model outputs were isolated. Higher runoff estimates correspond with worse water quality in coastal Belize. This supports the idea that forests function as important infiltration sites for water outflow (Lee et al., 2009; Neary et al., 2009; Woo et al., 2019).
The historical annual runoff results in Figure 5 addressed the objective of quantifying typical runoff values by using both historical precipitation trends and historical LULC for all three climatologically varied years: 2001 (moderate year), 2008 (wet year), and 2018 (dry year). In 2001, the southern watersheds produced higher rates of runoff volume, and these volumes increased in 2008 as precipitation averages rose during the wet year. This increase in runoff volume was more substantial in the southern watersheds given that precipitation in that region increased by an average of 13%, resulting in an average increase of 17% in surface runoff volume. By contrast, the northern watersheds only saw an average increase of 4.5% in precipitation, resulting in a 10.5% increase in surface runoff volume. In 2018, precipitation decreased by an average of 25%, resulting in an average decrease of 26% in surface runoff volume.
FIGURE 5. Historical annual runoff for different climatological years: moderate (2001); wet (2008); dry (2018).
Runoff for future scenarios was modeled while holding the 2018 precipitation rate static for each subsequent model run in order to gauge the isolated impact of LULC changes. Figure 6A shows net change in forest loss between 2030 and 2090, which was compared to the change in annual surface runoff for the same time period in Figure 6B. Watersheds experiencing more forest loss also experienced relatively high amounts of surface runoff, as seen in the Rio Hondo, Belize River, and Sarstoon River watersheds. This was true across the Conservation, Business as Usual, and Development scenarios as well. Within the Belize River Watershed, there was a 2.5% increase in runoff when changing from the Business as Usual to the Development scenario. On the other hand, with a transition from the Conservation to Development deforestation rates, there was a 79% increase in runoff. Such findings support the assertion that more deforestation removes infiltration sites and leads to higher runoff volume.
FIGURE 6. (A) Net forest loss from 2030 to 2090. (B) Net change in runoff volume when holding climate constant from 2018 and changing LULC scenarios between 2030 and 2090, normalized by watershed area (ha).
Figures 7A, B show modeled runoff holding 2018 LULC constant to illustrate the impacts of the two bookend SSP climate projections through 2090. The SSP126 projections resulted in minor changes in runoff volume throughout the 70-year period, with a maximum of a 6% decrease in the Rio Hondo watershed. On the other hand, the SSP585 scenario resulted in major decreases in runoff accumulation through 2090, with the Rio Hondo, Belize River, and Sarstoon River watersheds each showing more than a 50% decrease. The SSP585 scenario was defined as the scenario with the more extreme climate change impacts. The lower runoff volume estimates generated under this scenario are likely due to the fact that in this region lower precipitation rates are projected.
FIGURE 7. (A) Net change in runoff using climate scenario SSP126, (B) Net change in runoff using climate scenario SSP585, both normalized by watershed area (ha).
Percent changes in runoff values were based on a comparison to 2018, which was the most recent year available for the land cover product employed in this study. For consistency, the 2018 precipitation rate was also used as a baseline model. Table 5 shows the outputs of models parameterized with changing LULC scenarios and a static climate from 2018. Under these conditions, runoff volume increased across all watersheds and LULC scenarios from 2030 to 2090. In the Belize River Watershed, a critical and highly populated watershed, there was a cumulative increase in runoff volume of 0.2% from 2018 to 2030 under the Conservation LULC scenario. From 2018 to 2090, runoff volume increased by 1.3%. Similar increases occurred within each LULC scenario, with a 2.0% increase in runoff in 2090 under the Business as Usual scenario and a 2.5% increase under the Development scenario. These outputs support the finding established by Lee et al. (2009) vegetated areas such as forests can serve as filtration sites for potential outflow. The combination of decreasing forest cover, the expansion of pasture and cropland, and the static precipitation rate resulted in projections of increasing runoff for all watersheds. Under the Development 2090 scenario, the Belize River Watershed was projected to have a 42.4% decrease in forest cover, a 110.5% increase in pasture land, and a 1,944.7% increase in cropland. These changes resulted in a projected 2.5% cumulative increase in runoff volume between 2018 and 2090. Under the same 2090 scenario, the Rio Hondo River Watershed was projected to see 41.92% decrease in forest cover, a 433.6% increase in pasture land, and a 703.9% increase in cropland, resulting in a 5.6% increase in runoff volume (Figure 4 in Section 4.1).
TABLE 5. N-SPECT model iterations using future LULC and the 2018 precipitation rate. Percentage increases are based on comparison to model outputs from 2018. Watersheds are listed from North to South. Business as Usual (BAU), Conservation (CON), Development (DEV).
Table 6 shows the percent changes in annual surface runoff when the model allowed for changes in both climate and LULC. Under the SSP126 scenario, watersheds south of the Belize River have historically experienced more precipitation than the north; consequently, more precipitation was projected in the south under this climate scenario. Model outputs followed this pattern, with increases in surface runoff projected in southern watersheds and decreases in runoff projected in watersheds north of the Belize River projected in southern watersheds and decreases in runoff projected in watersheds north of the Belize River. While the Development scenario maintained decreasing surface runoff when compared to 2018, these decreases are less than that of the Conservation scenarios due to the fact that there is less forest cover remaining by 2090. While precipitation continued to decrease in these model runs, the increase in deforestation led to higher increases in runoff volume output.
TABLE 6. N-SPECT model iterations using future LULC and SSP126 climate inputs; percentage increases when compared to model runs from 2018. Watersheds are listed from North to South.
Forecasts under the SSP585 scenario projected greater decreases in runoff volume, reaching percentages as low as 80%. This was largely due to the fact that this scenario projected extreme climactic impacts, and climate change impacts in this region manifest as a general decrease in precipitation (Table 7). Although SSP585 projected an overall decrease in precipitation, these outputs were compared to 2018, which was a climatologically dry year. Comparing runoff projections with a dry year made some of the watersheds appear to experience increases in runoff estimates. However, had model outputs been compared to a wet year such as 2008, all watersheds would have been projected to experience less runoff volume.
TABLE 7. N-SPECT model iterations using future LULC and SSP585 inputs; percentage increases when compared to model runs from 2018. Watersheds are listed from North to South.
Under the SSP126 and SSP585 climate projections, precipitation is expected to decrease across Belize through 2090, and these decreases resulted in a decrease in runoff volume at all 15 pour points. Both the Belize River and the Rio Hondo watersheds saw an average decrease in precipitation of 40%. In the case of the Belize River, this resulted in a decrease in runoff volume of 51.6%, while the Rio Hondo River watershed saw a decrease in runoff volume of 65.7% (Figure 8). The positive relationship between precipitation and runoff is supported by these findings.
FIGURE 8. Changes in precipitation highlighting the Belize River Watershed and Rio Hondo River Watershed.
Annual surface runoff model results can be grouped by northern and southern watersheds, with northern watersheds being influenced greatly by LULC and the southern ones by precipitation changes. Notably, sugarcane production is prevalent and economically important in the northern lowlands of Belize, comprising about 30% of agricultural area in the country (Chi et al., 2017). Intensively cultivated sugarcane plantations in Belize’s northern districts like Corozal and Orange Walk expand in the LULC projections, contributing more heavily to runoff in the N-SPECT outputs. Smaller agricultural operations like milpa generally occur in predominantly Mayan areas in the southern watersheds (Drexler, 2020). Additionally, the Rio Hondo Watershed produced the largest amounts of both deforestation and annual surface runoff output between 2030 and 2090. This can be attributed not only to the overall size of the watershed, but also to the amount of LULC change occurring in this region. Cropland in the Rio Hondo Watershed increased by 275.6% between 2030 and 2090 under the Business as Usual scenario. Given that sugarcane is the main crop in the neighboring watershed of New River, part of the Rio Hondo’s projected cropland growth may be due to the projected expansion of industrial sugarcane operations (Chi et al., 2017).
Belize’s climate can generally be categorized into a wet and a dry season. During the rainy season, northern Belize experiences an average of 1,524 mm of rainfall per year while southern Belize receives an average of 4,064 mm. The precipitation rate in the south is enhanced by the Intertropical Convergence Zone’s movement northward and the orographic lifting that occurs due to the mountainous terrain (National Meteorological Service of Belize, 2022 ). The increased rainfall in the south explains why the southern watersheds continue experiencing copious amounts of precipitation, despite the fact that the SSP126 and SSP585 scenarios project overall decreases in rainfall for Belize. The southern region also produced higher volumes of runoff when compared to the northern watersheds. Despite the fact that the climate projections applied in this study projected an overall decrease in precipitation for tropical regions such as Belize, these areas are also expected to experience tropical storms of higher intensity (Richardson, 2009).
The projected periods of dry weather before more intense storms have the potential to affect the amount of runoff accumulation entering waterways, as well as increasing the accumulation of nutrients such as phosphorus and nitrogen in soils (Al-Kaisi et al., 2013). In addition to this natural accumulation of nutrients, degraded soils will likely require increased amounts of synthetic fertilizers to maintain crop production. During high rainfall events, the accumulation of nutrients can leach from the soil and increase the risk of erosion (Meerman and Cherrington, 2005). This study employed annual precipitation rates, which provide a generalized approach when modeling non-point source pollution and runoff accumulation; however, they do not allow for the distinction of changes in runoff volume between different seasons, particularly storm and non-storm seasons.
Regarding coral reef impacts, a recent study by Kjerfve et al. (2021) modeled fluvial runoff near the Gulf of Honduras and included the BBRRS in their analysis. They found that there was a constant supply of nutrients such as nitrogen and phosphorus to the BBRRS, and that the reef contained considerable fleshy macroalgae cover because it was subject to breakage from more frequent storms than surrounding areas like Glover’s reef and the Guatemalan coastal reefs. Kjerfve et al. (2021) also found that the same watersheds in our study region were producing high amounts of surface runoff; they listed the Sarstoon River, Moho River, Rio Grande, and Monkey River watersheds as some of their primary contributors to runoff. The Sarstoon River, Moho River, Temash River, and Rio Grande watersheds comprised the four watersheds that produced the most runoff in our study (Figure 7).
Continuing to employ modelling techniques to monitor runoff and impacts of climate change is essential for the conservation of the BBRRS. With increasing runoff projected to occur due to increases in development and deforestation, coral reefs stand to be impacted by land-based stressors such as nutrients and microfibers (Lapointe et al., 2021; Oldenburg et al., 2021). By understanding sources and pollutants released from pour points from land, policy and land management practices can be enacted to mitigate the impacts of anthropogenic stressors on corals. The modelling of different development and climate scenarios arms organizations like CZMAI the tools to bring attention to specific watersheds and regions of the BBRRS most likely to be affected by runoff. This work can help in the update of the nation’s ICZMP. Studies in the future should aim to validate the results of these model outputs as time progresses as well as monitoring the health and diversity of corals.
While this study addressed changes in LULC and climate, both the soil characteristics and elevation variables were held constant throughout all model runs. The conversion of forest to agriculture tends to lead to soil erosion and changes in the chemical composition of the surface soil (Merten and Minella, 2013). Other studies found that the conversion of forest and shrubland to agricultural land held the highest soil erosion rates (Kogo et al., 2020). Elevation would also change over time from natural processes such as sea level changes and erosion. Soil characteristic groups and elevation inputs would need to be adjusted in future modeling efforts to more accurately estimate the amount of runoff that would be entering the waterways.
This study modeled both LULC and runoff estimates using a set of projections that sought to encompass a wide range of potential future scenarios. LULC scenarios were defined by deforestation rates that reflected possible degrees of environmental protections or economic priorities in the region. The historic LULC analysis highlighted the prevalence of agricultural expansion across Belize’s major watersheds, especially the rapid expansion of land devoted to pasture. The initial assumptions about LULC change patterns such as the likelihood of agricultural expansion from previously cleared areas appeared to hold true and support findings by previous studies. Model runs that held climate constant to isolate the effects of changing LULC showed that watersheds with less projected deforestation produced less runoff volume along the coast. Model iterations also predicted that the Belize River watershed could lose approximately 30% of its forested land under the current deforestation rate and 36% with a 0.8% deforestation rate by 2090.
Furthermore, this is the first study to employ the World Climate Research Program’s (WCRP) most recent CMIP6 climate projections for Belize, to the authors’ knowledge. While projections from previous phases of CMIP have supported modeling efforts and decision-making processes, CMIP6 integrates new socioeconomic and concentration pathways that are highly relevant to policymakers (Grose et al., 2020). The SSP scenarios address both climactic and societal variables, and this study employed bookend SSP scenarios for the hydrologic modeling to allow for a wider range of model outputs. The less severe climate change impacts of SSP126 suggested decreased runoff through 2070 in response to lower precipitation rates before subsequently increasing in 2090 once climate forcing is projected to reach its peak; at this time, precipitation would increase once again. SSP585 results predicted a similar trend where the decreasing rates of precipitation through 2090 resulted in decreased runoff volume.
Modeling in N-SPECT along Belize’s coast provided a baseline analysis about how runoff volumes can be impacted by LULC conversions and climate projections. Changes in surface runoff volume were influenced exclusively by variations in LULC and precipitation. The historic surface runoff results demonstrated a strong positive relationship between changes in precipitation rates and runoff volume. The generally wetter southern watersheds experienced greater changes in precipitation and thus produced larger volumes in annual surface runoff. This regional trend can have important implications for the BBRRS as well as for local resilience planning, and the link that this study found between precipitation and runoff could also inform the region’s climate change adaptation strategies. The breadth of these model projections can provide ample opportunities to support decision-makers in their work. Agencies like Belize’s Coastal Zone Management Authority and Institute that are tasked with responding to obstacles to sustainable development can use model outputs in support of their long-term planning efforts. Given the ecological, economic, and social importance of the lagoon, preventative measures that can mitigate climate change-induced damages to water quality and reef health will be a critical investment for the region.
The original contributions presented in the study are included in the article, further inquiries can be directed to the corresponding author.
VM-A: Methodology, Software, Writing (original draft preparation), Formal Analysis, and Visualization. CE: Methodology, Software, Writing (original draft preparation), Formal Analysis, and Visualization. RG: Conceptualization, Supervision, Reviewing, Editing, and Project Administration. EC: Conceptualization, Supervision, Data Curation, and Project Administration. CL: Reviewing and Editing. DM: Reviewing and Editing. NG: Writing, Reviewing, and Editing. AR: Writing, Reviewing, and Editing. IC: Writing and Reviewing. JJ: Writing and Reviewing. SR: Reviewing.
This project was funded by the NASA Biological Diversity and Ecological Forecasting Program—Grant Number 80NSSC19K0200. Work by JPL co-author CL was carried out at the Jet Propulsion Laboratory, California Institute of Technology, under contract with the National Aeronautics and Space Administration. California Institute of Technology. Government sponsorship acknowledged. Copyright 2021. All rights reserved.
The authors declare that the research was conducted in the absence of any commercial or financial relationships that could be construed as a potential conflict of interest.
All claims expressed in this article are solely those of the authors and do not necessarily represent those of their affiliated organizations, or those of the publisher, the editors and the reviewers. Any product that may be evaluated in this article, or claim that may be made by its manufacturer, is not guaranteed or endorsed by the publisher.
We acknowledge the World Climate Research Programme, which, through its Working Group on Coupled Modelling, coordinated, and promoted CMIP6. We thank the climate modeling groups for producing and making available their model output, the Earth System Grid Federation (ESGF) for archiving the data and providing access, and the multiple funding agencies who support CMIP6 and ESGF.
Al-Kaisi, M. M., Elmore, R. W., Guzman, J. G., Hanna, H. M., Hart, C. E., Helmers, M. J., et al. (2013). Drought Impact on Crop Production and the Soil Environment: 2012 Experiences from Iowa. J. Soil Water Conservation 68, 19A–24A. doi:10.2489/jswc.68.1.19a
Arnason, T., Lambert, J. D. H., Gale, J., Cal, J., and Vernon, H. (1982). Decline of Soil Fertility Due to Intensification of Land Use by Shifting Agriculturists in Belize, Central America. Agro-Ecosystems 8, 27–37. doi:10.1016/0304-3746(82)90012-9
Arnell, N. W., Hudson, D., and Jones, R. (2003). Climate Change Scenarios from a Regional Climate Model: Estimating Change in Runoff in Southern Africa. J. Geophys. Res. 108, e248. doi:10.1029/2002jd002782
Baumann, J., Davies, S., Courtney, T., Aichelman, H., Townsend, J., and Castillo, K. (2016). Characterizing Patch Reef Environments on the Mesoamerican Barrier Reef System Utilizing Historical Sea Surface Temperature Records. PLOS ONE 11 (9), e0162098. doi:10.1016/j.biocon.2014.07.004
Baumann, J. H., Ries, J. B., Rippe, J. P., Courtney, T. A., Aichelman, H. E., Westfield, I., et al. (2019). Nearshore Coral Growth Declining on the Mesoamerican Barrier Reef System. Glob. Change Biol. 25, 3932–3945. doi:10.1111/gcb.14784
Bruinsma, J. (Editor) (2003). World Agriculture : Towards 2015/2030: An FAO Perspective 1st Edn.. London: Routledge. doi:10.4324/9781315083858
Burke, L., and Sugg, Z. (2006). Hydrologic Modeling of Watersheds Discharging Adjacent to the Mesoamerican Reef Analysis Summary - December 1, 2006. World Resources Institute.
Callejas, I. A., Lee, C. M., Mishra, D. R., Felgate, S. L., Evans, C., Carrias, A., et al. (2021). Effect of Covid-19 Anthropause on Water Clarity in the belize Coastal Lagoon. Front. Mar. Sci. 8. doi:10.3389/fmars.2021.648522
Campbell, R. M. (2013). “Transformations in the Belize Sugar Industry: From Colonial Plantations to a Vital Tourist Industry (Dissertation),” (University of North Carolina at Greensboro). Ph.D. thesis.
Cherrington, E. A., Griffin, R. E., Anderson, E. R., Hernandez Sandoval, B. E., Flores-Anderson, A. I., Muench, R. E., et al. (2020). Use of Public Earth Observation Data for Tracking Progress in Sustainable Management of Coastal forest Ecosystems in belize, central america. Remote Sensing Environ. 245, 111798. doi:10.1016/j.rse.2020.111798
Cherrington, E., Ek, E., Cho, P., Howell, B., Hernández Sandoval, B., Anderson, E., et al. (2010). Forest Cover and Deforestation in Belize: 1980–2010 Nature Climate Change.
Cherrington, E., Kay, E., and Waight-Cho, I. (2015). Modelling the Impacts of Climate Change and Land Use Change on Belize’s Water Resources: Potential Effects on Erosion and Runoff. doi:10.13140/RG.2.2.16952.75524
Chi, L., Mendoza-Vega, J., Huerta, E., and Álvarez-Solís, J. D. (2017). Effect of Long-Term Sugarcane (Saccharum spp.) Cultivation on Chemical and Physical Properties of Soils in Belize. Commun. Soil Sci. Plant Anal. 48 (7). doi:10.1080/00103624.2016.1254794
Chicas, S., Omine, K., and Ford, J. (2015). Identifying Erosion Hotspots and Assessing Communities’ Perspectives on the Drivers, Underlying Causes and Impacts of Soil Erosion in Toledo’s Rio Grande Watershed: Belize. Appl. Geogr. 68, 57–67. doi:10.1038/nclimate2502
Cho, L. (2005). Marine Protected Areas: A Tool for Integrated Coastal Management in Belize. Ocean Coastal Manag. 48, 932–947. doi:10.1016/j.ocecoaman.2005.03.007
Chomitz, K. M., and Gray, D. A. (2017). Roads, Land Use, and Deforestation: A Spatial Model Applied to Belize. The Econ. Land Use 10, 289–314. doi:10.4324/9781315240114-20
Costa, M. H., and Foley, J. A. (1997). Water Balance of the Amazon Basin: Dependence on Vegetation Cover and Canopy Conductance. J. Geophys. Res. 102, 23973–23989. doi:10.1038/nclimate250210.1029/97jd01865
Coastal Zone Management Authority and Institute (CZMAI) (2016). Belize Integrated Coastal Zone Management Plan. Belize City: CZMAI.
Donovan, M. K., Adam, T. C., Shantz, A. A., Speare, K. E., Munsterman, K. S., Rice, M. M., et al. (2020). Nitrogen Pollution Interacts with Heat Stress to Increase Coral Bleaching across the Seascape. Proc. Natl. Acad. Sci. USA 117, 5351–5357. doi:10.1073/pnas.1915395117
Drexler, K. A. (2020). Government Extension, Agroecology, and Sustainable Food Systems in Belize Milpa Farming Communities: A Socio-Ecological Systems Approach. J. Agric. Food Syst. Community Dev. 9, 85–97. doi:10.5304/jafscd.2020.093.001
Eastman, J. R., and He, J. (2020). A Regression-Based Procedure for Markov Transition Probability Estimation in Land Change Modeling. Land 9 (11), 407. doi:10.3390/land9110407
Eslinger, D. L., Carter, H., Pendleton, M., Burkhalter, S., and Allen, M. (2012). OpenNSPECT: The Open-Source Nonpoint Source Pollution and Erosion Comparison Tool. Charleston, South Carolina: NOAA Office for Coastal Management.
Factbook, T. W. (2020). Erratum: Effects of Tropical Deforestation on Climate and Agriculture. Available at: : https://www.cia.gov/library/publications/resources/the-world-factbook/index.html.
FAOISRICUNEPCIP (1998). Soil and Terrain Digital Database for Latin America and the Caribbean at 1:5 Million Scale Land and Water Digital Media Series No. 5. Rome: Food and Agriculture Organization of the United Nations. doi:10.1038/nclimate2502
Fisher, J. R. B., Acosta, E. A., Dennedy-Frank, P. J., Kroeger, T., and Boucher, T. M. (2017). Impact of Satellite Imagery Spatial Resolution on Land Use Classification Accuracy and Modeled Water Quality. Remote Sens Ecol. Conserv 4, 137–149. doi:10.1002/rse2.61
Friedl, M., and Sulla-Menashe, D. (2019). MCD12Q1 MODIS/Terra+Aqua Land Cover Type Yearly L3 Global 500m SIN Grid V006. NASA EOSDIS Land Processes DAAC. doi:10.5067/MODIS/MCD12Q1.006
Funk, C., Peterson, P., Landsfeld, M., Pedreros, D., Verdin, J., Rowland, J., et al. (2014). A Quasi-Global Precipitation Time Series for Drought Monitoring: U.S. Geological Survey. U.S. Geol. Surv. Data Ser. 832, 4. doi:10.3133/ds832
García-Contreras, E. C. J., Acosta-Lugo, G., and Andrade-Hernández, M. (2011). “Maintaining Maya forest Connectivity in a Changing Landscape: Calakmul Biosphere reserve and mexico’s Highway 186,” in 2011 International Conference on Ecology and Transportation (ICOET 2011), Seattle, WA, USA, August 23, 2011.
Gibson, J. (2011). “The Belize Barrier Reef: a World Heritage Site,” in Too Precious to Drill: The Marine Biodiversity of Belize. Editors M L D. Palomares, and D Pauly (Fisheries Centre Research Reports), 8–13.
Goatley, C. H. R., Bonaldo, R. M., Fox, R. J., and Bellwood, D. R. (2016). Sediments and Herbivory as Sensitive Indicators of Coral Reef Degradation. E&S 21. doi:10.5751/ES-08334-210129
Gómez-Martínez, G., Galiano, L., Rubio, T., Prado-López, C., Redolat, D., Paradinas Blázquez, C., et al. (2021). Effects of Climate Change on Water Quality in the Jucar River basin (spain). Water 13 (17). doi:10.3390/w13172424
Gonçalves, D. N. S., Gonçalves, C. d. M., Assis, T. F. d., and Silva, M. A. d. (2014). Analysis of the Difference between the Euclidean Distance and the Actual Road Distance in Brazil. Transportation Res. Proced. 3, 876–885. doi:10.1016/j.trpro.2014.10.066
Gorelick, N., Hancher, M., Dixon, M., Ilyushchenko, S., Thau, D., and Moore, R. (2017). Google Earth Engine: Planetary-Scale Geospatial Analysis for Everyone. Remote Sensing Environ. 202, 18–27. doi:10.1016/j.rse.2017.06.031
Gould, K. A. (2017). Ecotourism under Pressure: The Political Economy of Oil Extraction and Cruise Ship Tourism Threats to Sustainable Development in Belize. Environ. Sociol. 3, 237–247. doi:10.1080/23251042.2017.1308238
Graesser, J., Aide, T. M., Grau, H. R., and Ramankutty, N. (2015). Cropland/pastureland Dynamics and the Slowdown of Deforestation in Latin America. Environ. Res. Lett. 10 (3). doi:10.1088/1748-9326/10/3/034017
Grose, M. R., Narsey, S., Delage, F., Dowdy, A. J., Bador, M., Boschat, G., et al. (2020). Insights from CMIP6 for Australia's Future Climate. Earth’s Future 8 (5). doi:10.1002/essoar.10501525.1
Guild, L. S., Cohen, W. B., and Kauffman, J. B. (2004). Detection of Deforestation and Land Conversion in Rondônia, Brazil Using Change Detection Techniques. Int. J. Remote Sensing 25, 731–750. doi:10.1080/01431160310001598935
Gupta, R., and Sharma, L. K. (2020). Efficacy of Spatial Land Change Modeler as a Forecasting Indicator for Anthropogenic Change Dynamics over Five Decades: A Case Study of Shoolpaneshwar Wildlife Sanctuary, Gujarat, India. Ecol. Indicators 112, 106171. doi:10.1016/j.ecolind.2020.106171
Hecht, S. B. (1993). The Logic of Livestock and Deforestation in Amazonia. BioScience 43, 687–695. doi:10.2307/1312340
Hoegh-Guldberg, O. (1999). Climate Change, Coral Bleaching and the Future of the World's Coral Reefs. Mar. Freshw. Res. 50, 839–866. doi:10.1071/mf99078
International Union for the Conservation of Nature (IUCN) (2018). Second Largest Reef on Earth off ’danger List’, Following IUCN’s Advice. IUCN. Available at: https://www.iucn.org/news/iucn-42whc/201806/second-largest-reef-earth-danger-list-following-iucns-advice.
Khalili, A., Jamali, S., and Morovvati, A. (2015). Investigation of Effective Factors on Runoff Generation and Sediment Yield of Loess Deposits Using Rainfall Simulator. J. Biodiversity Environ. Sci., 80–88.
Kjerfve, B., McField, M., Thattai, D., and Giró, A. (2021). Coral Reef Health in the Gulf of honduras in Relation to Fluvial Runoff, Hurricanes, and Fishing Pressure. Mar. Pollut. Bull. 172, 112865. doi:10.1016/j.marpolbul.2021.112865
Kogo, B. K., Kumar, L., and Koech, R. (2020). Impact of Land Use/cover Changes on Soil Erosion in Western Kenya. Sustainability 12, 9740. doi:10.1038/nclimate250210.3390/su12229740
Lapointe, B. E., Tewfik, A., and Phillips, M. (2021). Macroalgae Reveal Nitrogen Enrichment and Elevated N:p Ratios on the belize Barrier Reef. Mar. Pollut. Bull. 171, 112686. doi:10.1016/j.marpolbul.2021.112686
Lawrence, D., and Vandecar, K. (2015). Erratum: Effects of Tropical Deforestation on Climate and Agriculture. Nat. Clim Change 5, 174. doi:10.1038/nclimate2502
Lee, S.-W., Hwang, S.-J., Lee, S.-B., Hwang, H.-S., and Sung, H.-C. (2009). Landscape Ecological Approach to the Relationships of Land Use Patterns in Watersheds to Water Quality Characteristics. Landscape Urban Plann. 92, 80–89. doi:10.1016/j.landurbplan.2009.02.008
Lehner, B., Verdin, K., and Jarvis, A. (2006). HydroSHEDS Technical Documentation. Washington, DC: World Wildlife Fund US.
Loka, K. Y., Sakai, K. Y., Nakano, Y., and Sambali, H. (2001). Coral Bleaching: The Winners and the Losers. Ecol. Lett. 4, 122–131. doi:10.1046/j.1461-0248.2001.00203.x
Lopez-Ridaura, S., Barba-Escoto, L., Reyna-Ramirez, C. A., Sum, C., Palacios-Rojas, N., and Gerard, B. (2021). Maize Intercropping in the Milpa System. Diversity, Extent and Importance for Nutritional Security in the Western Highlands of Guatemala. Sci. Rep. 11, 3696. doi:10.1038/s41598-021-82784-2
Meerman, J., and Cherrington, E. (2005). Preliminary Survey of Land Degradation in Belize. Ministry of Natural Resources, Local Government, and the Environment. Belize: Belmopan. doi:10.13140/RG.2.1.3880.6480
Meerman, J., and Clabaugh, J. (2017). Biodiversity and Environmental Resource Data System of Belize. Available at: http://www.biodiversity.bz.
Meerman, J., Epting, J., Steininger, M., and Hewson, J. (2010). Forest Cover and Change in Belize Technical Report 2005 - 2011 - 2015. Belmopan, Belize: Belize Tropical Studies.
Merrill, R. (2010). Annual General Meeting Report Tech. rep., Belize Ministry of Agriculture and Fisheries. Yalbac, Cayo.
Merten, G. H., and Minella, J. P. G. (2013). The Expansion of Brazilian Agriculture: Soil Erosion Scenarios. Int. Soil Water Conservation Res. 1, 37–48. doi:10.1016/s2095-6339(15)30029-0
Mishra, V., Rai, P., and Mohan, K. (2014). Prediction of Land Use Changes Based on Land Change Modeler (Lcm) Using Remote Sensing: A Case Study of Muzaffarpur (Bihar), India. J. Geogr. Inst. Cvijic 64, 111–127. doi:10.2298/IJGI1401111M
Mitchell, B. A., Walker, Z., Walker, Z., and Walker, P. (2017). A Governance Spectrum: Protected Areas in Belize. Parks 23, 45–60. doi:10.2305/iucn.ch.2017.parks-23-1bam.en
Morton, D. C., Defries, R. S., Randerson, J. T., Giglio, L., Schroeder, W., and Van Der Werf, G. R. (2008). Agricultural Intensification Increases Deforestation Fire Activity in Amazonia. Glob. Change Biol. 14, 2262–2275. doi:10.1111/j.1365-2486.2008.01652.x
National Meteorological Service of Belize (2022). The Climate of belize. National Meteorological Service of Belize. Available at: http://nms.gov.bz/climate-services/climate-summary/ (Accessed 2022).
Neary, D. G., Ice, G. G., and Jackson, C. R. (2009). Linkages between forest Soils and Water Quality and Quantity. For. Ecol. Manag. 258, 2269–2281. doi:10.1016/j.foreco.2009.05.027
Neelin, J. D., Münnich, M., Su, H., Meyerson, J. E., and Holloway, C. E. (2006). Tropical Drying Trends in Global Warming Models and Observations. Proc. Natl. Acad. Sci. 103, 6110–6115. doi:10.1073/pnas.0601798103
Nelson, A., and Chomitz, K. M. (2011). Effectiveness of Strict vs. Multiple Use Protected Areas in Reducing Tropical forest Fires: A Global Analysis Using Matching Methods. PLoS ONE 6, e22722. doi:10.1371/journal.pone.0022722
Nuenninghoff, S., Lemay, M., Rogers, C., and Martin, D. (2015). Sustainable Tourism in Belize. Inter-American Development Bank: Environment, Rural Development, and Disaster Risk Management Division. Inter-American Development Bank Felipe Herrera Library.
Oldenburg, K., Urban-Rich, J., Castillo, K., and Baumann, J. (2021). Microfiber Abundance Associated with Coral Tissue Varies Geographically on the belize Mesoamerican Barrier Reef System. Mar. Pollut. Bull. 163. doi:10.1016/j.marpolbul.2020.111938
Patterson, C. (2016). Deforestation, Agricultural Intensification, and Farm Resilience in Northern Belize: 1980-2010. Thesis, Doctor of Philosophy. University of Otago.
Ramirez, D., Ordaz, J., Mora, J., Acosta, A., and Serna, B. (2013). Belize: Effects of Climate Change on Agriculture. Mexico: Economic Commission for Latin America and the Caribbean.
Richardson, R. (2009). Belize and Climate Change: The Cost of Inaction. Belmopan, Belize: United Nations Development Programme.
Rodríguez Eraso, N., Armenteras-Pascual, D., and Alumbreros, J. R. (2013). Land Use and Land Cover Change in the Colombian andes: Dynamics and Future Scenarios. J. Land Use Sci. 8, 154–174. doi:10.1080/1747423x.2011.650228
Root, T. (2018). How One Country Is Restoring its Damaged Ocean National Geographic. National Geographic. Available at: https://www.nationalgeographic. com/news/2018/04/belize-restores-coral-reefs-oil-drilling-ban-environment/.
Sánchez, L. A., Ataroff, M., and López, R. (2002). Soil Erosion under Different Vegetation Covers in the Venezuelan Andes. Environmentalist 22, 161–172. doi:10.1023/A:1015389918416
Sangermano, F., Eastman, J. R., and Zhu, H. (2010). Similarity Weighted Instance-Based Learning for the Generation of Transition Potentials in Land Use Change Modeling. Trans. GIS 14, 569–580. doi:10.1111/j.1467-9671.2010.01226.x
Schulz, C., Koch, R., Cierjacks, A., and Kleinschmit, B. (2017). Land Change and Loss of Landscape Diversity at the Caatinga Phytogeographical Domain - Analysis of Pattern-Process Relationships with MODIS Land Cover Products (2001-2012). J. Arid Environments 136, 54–74. doi:10.1016/j.jaridenv.2016.10.004
Soto, I., Andréfouët, S., Hu, C., Muller-Karger, F. E., Wall, C. C., Sheng, J., et al. (2009). Physical Connectivity in the Mesoamerican Barrier Reef System Inferred from 9 Years of Ocean Color Observations. Coral Reefs 28 (2), 415–425. doi:10.1007/s00338-009-0465-0
Swart, N., Cole, J. N., Kharin, V., V., Lazare, M., and Scinocca, J. (2019). Cccma Canesm5 Model Output Prepared for Cmip6 C4mip. Earth System Grid Federation. World Climate Research Programme. doi:10.22033/ESGF/CMIP6.1301
Ter Steege, H., Pitman, N. C., Killeen, T. J., Laurance, W. F., Peres, C. A., Guevara, J. E., et al. (2015). Estimating the Global Conservation Status of More Than 15,000 Amazonian Tree Species. Sci. Adv. 1, e1500936. doi:10.1126/sciadv.1500936
UNEP-WCMC and IUCN (2022). Protected Planet: The World Database on Protected Areas (WDPA) and World Database on Other Effective Area-based Conservation Measures (WD-OECM). Cambridge, United Kingdom: UNEP-WCMC and IUCN. Available at: www.protectedplanet.net (Accessed October 2020).
United Nations (2018). The 2030 Agenda and the Sustainable Development Goals: An Opportunity for Latin america and the Caribbean. Santiago: United Nations, (LC/G.2681-P/Rev.3).
Wassenaar, T., Gerber, P., Verburg, P. H., Rosales, M., Ibrahim, M., and Steinfeld, H. (2007). Projecting Land Use Changes in the Neotropics: The Geography of Pasture Expansion into forest. Glob. Environ. Change 17, 86–104. doi:10.1016/j.gloenvcha.2006.03.007
Wisz, M. S., Hijmans, R. J., Li, J., Peterson, A. T., Graham, C. H., and Guisan, A. (2008). Effects of Sample Size on the Performance of Species Distribution Models. Divers. Distributions 14, 763–773. doi:10.1111/j.1472-4642.2008.00482.x
Keywords: land cover change, hydrology, runoff, CMIP-6, climate change, Belize, N-SPECT, TerrSet
Citation: Martín-Arias V, Evans C, Griffin R, Cherrington EA, Lee CM, Mishra DR, Gomez NA, Rosado A, Callejas IA, Jay JA and Rosado S (2022) Modeled Impacts of LULC and Climate Change Predictions on the Hydrologic Regime in Belize. Front. Environ. Sci. 10:848085. doi: 10.3389/fenvs.2022.848085
Received: 03 January 2022; Accepted: 25 February 2022;
Published: 01 April 2022.
Edited by:
Tianjie Zhao, Aerospace Information Research Institute (CAS), ChinaReviewed by:
Rui Li, Institute of Remote Sensing and Digital Earth (CAS), ChinaCopyright © 2022 Martín-Arias, Evans, Griffin, Cherrington, Lee, Mishra, Gomez, Rosado, Callejas, Jay and Rosado. This is an open-access article distributed under the terms of the Creative Commons Attribution License (CC BY). The use, distribution or reproduction in other forums is permitted, provided the original author(s) and the copyright owner(s) are credited and that the original publication in this journal is cited, in accordance with accepted academic practice. No use, distribution or reproduction is permitted which does not comply with these terms.
*Correspondence: Vanesa Martín-Arias, dm0wMDQzQHVhaC5lZHU=
Disclaimer: All claims expressed in this article are solely those of the authors and do not necessarily represent those of their affiliated organizations, or those of the publisher, the editors and the reviewers. Any product that may be evaluated in this article or claim that may be made by its manufacturer is not guaranteed or endorsed by the publisher.
Research integrity at Frontiers
Learn more about the work of our research integrity team to safeguard the quality of each article we publish.