- State Key Laboratory of Crop Biology, College of Horticultural Science and Engineering, Shandong Agricultural University, Tai’an, China
Apple replant disease (ARD) is a common disease in apple producing areas, and more and more evidence shows that soil-borne pathogens are the main factor. However, most of the drugs used to kill microorganisms are not friendly to the environment. Therefore, there is an urgent need to identify a method that can effectively eliminate these harmful microorganisms and to construct a microbial community structure that is conducive to plant growth in the soil. Herein, we use four different application technologies: foliar spraying, foliar soaking, root soaking, and soil soaking, to examine the inhibitory effect of zinc oxide nanoparticles (ZnO-NPs) on ARD. This study found that they all promoted the growth of Malus hupehensis Rehd. seedlings, and the plant height was 1.09 times, 1.15 times, 1.26 times, and 1.36 times higher that of the control, respectively. Soil soaking had the best promotion effect, and the changes in the soil microbial community structure after root soaking were analyzed. After treatment with ZnO-NPs, the abundances of Neocosmospora, Gibberella, and Fusarium were reduced, whereas the abundances of Tausonia, Chaetomium, and Mrakia were increased. The copy numbers of Fusarium solani and Fusarium oxysporum were 55.7 and 68.9% lower in the ZnO-NPs treatment group than those in the control group, respectively. This study found that after ZnO-NPs were applied to the soil, a new microbial community structure that was conducive to plant growth was formed to overcome ARD. In summary, ZnO-NPs, as a green chemical reagent, can overcome ARD, and it can also be applied to other continuous crops.
Introduction
The consequences of long-term intensive monocultures are that crops produce autotoxic compounds, reduce biodiversity levels, decrease food production, increase pest infections, and reduce soil carbon and nitrogen, thereby affecting the health of the crops and the microbial community structure (Nicola et al., 2018; Cavael et al., 2019). The resulting decline in crop yield is known as replant disease. China is the world’s largest cultivator of apples (Wang et al., 2016). With few land resources and outdated orchards, farmers tend to grow fruit trees of the same species, which can cause apple replant diseases (ARD) (Li et al., 2020). The causes of ARD are complex, generally involving biotic and abiotic factors (Politycka and Adamska, 2003). Abiotic factors include soil pH, orchard age, plant autotoxins, nutrient imbalances, and unfavorable external environments (Mai, 1981; Traquair, 1984), whereas biotic factors include nematodes, bacteria, fungi, and other unknown agents (Yim et al., 2013), with the latter considered as the main cause of replant disease. Franke-Whittle et al. (2015) reported that Acremonium, Cylindrocarpon, and Fusarium are strongly associated with replant disease, whereas Mazzola. (1998) demonstrated that fungi are a major causative factor of Washington ARD. A recent study found that Fusarium is the main pathogen causing ARD in China (Sheng et al., 2020).
The survival rate of trees with replant disease is low. These trees also possess significantly shortened internodes, reduced biomass production, and root and root tip necrosis (Mazzola and Manici, 2012; Grunewaldt-Stöcker et al., 2019), which lead to low fruit yields and poor fruit quality, and without human intervention, ARD can reduce profits by 50% (Van Schoor et al., 2009). For instance, in Washington, replant disease can decrease the total revenue of each acre by $40,000 every decade (Smith, 1995). In the absence of crop rotation options, chemical control is the best way to control ARD caused by soil-borne pathogens (Mai, 1981), and when the soil is disinfected, plant growth is improved significantly (Yim et al., 2013). Broad-spectrum fumigants, such as methyl bromide and chloropicrin, have been used since the 1900s for the disinfection of soil to overcome replant disease (Willett et al., 1994). However, methyl bromide is a toxic gas, which threatens human health and destroys the ozone layer. According to the “Montreal Protocol,” an international treaty that aims to protect the ozone layer, developed and developing countries stopped using methyl bromide in 2005 or shortly thereafter. In China, the use of methyl bromide ceased in 2018. However, the identification a safe and effective alternative to methyl bromide is proving to be a challenge.
Recently, nanoparticles have been extensively studied due to their antifungal property (Sirelkhatim et al., 2015) and defense ability (Sofy et al., 2020); however, the optimal inhibitory concentration is not known. Dimkpa et al. (2013) reported that ZnO-NPs could inhibit fungi at the concentrations ranging from 100 to 500 mg/kg, whereas González-Merino et al. (2021) demonstrated that ZnO-NPs at a concentration of 1,600 mg/kg had the best inhibitory effect against Fusarium isolated from tomato. In addition, ZnO-NPs can alter the soil microbial community at low concentrations such as 10 mg/kg (Xu et al., 2017). Therefore, this study determined the optimal concentration of ZnO-NPs, and then used different application methods: foliar spraying, foliar soaking, root soaking, and soil soaking to determine the best treatment based on the phenotype of the plant. In addition, high-throughput sequencing of soil microorganisms was performed to investigate how ZnO-NPs can overcome ARD by affecting the soil microbial community.
Materials and Methods
Experimental Materials
The pot experiment was carried out at the National Apple Central Experimental Station (36°9′29″N, 117°9′4″E) located at the Panhe Campus of Shandong Agricultural University, Tai’an City, Shandong Province from March to October 2021. The soil used in the experiment was taken from the 34-year-old Fuji Apple Orchard in Tanqingwan Village, Manzhuang Town, Tai’an City, Shandong Province (36°5′27″N, 117°3′14″E). The average annual temperature and rainfall were approximately 12.9°C and 697 mm, respectively. The specific physical and chemical properties of the soil are given in Table 1.
ZnO-NPs with a particle diameter of 30 ± 10 nm were purchased from Macklin Biochemical Co., Ltd. (Shanghai, China). In preliminary experiments, we found that a concentration of 250 mg/L ZnO-NPs had the best antifungal effect (Figure 1). Therefore, 250 mg of ZnO-NPs was weighed and resuspended in 1 L of deionized water (18 MΩ cm), followed by sonication with an ultrasonic device (100 W, 45 kHZ) for 60 min and adjustment of the pH to 7.0.
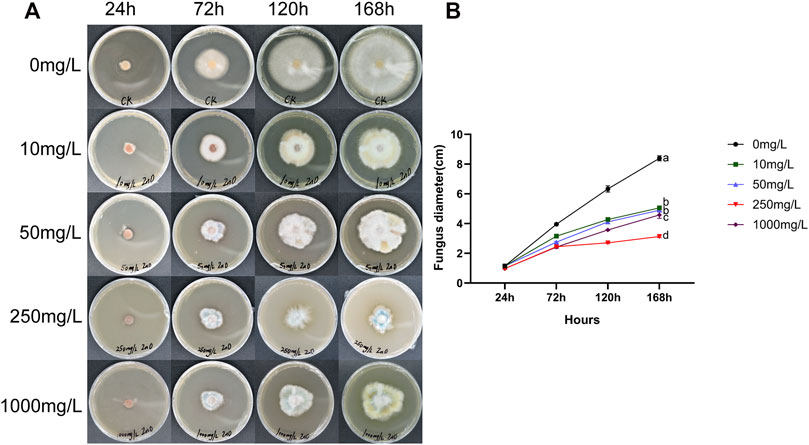
FIGURE 1. After adding ZnO-NPs to PDA medium, the inhibitory effect on the growth of F. proliferatum. (A) Growth of F. proliferatum at 24, 72, 120, 168 h after treatment with different concentrations of ZnO-NPs. (B) Diameter variation curve of F. proliferatum after treatment with different concentrations of ZnO-NPs. There is a significant difference between the mean values of different treatments using one-way ANOVA and Duncan’s multiple range test (p < 0.05). Error bars indicate ± S.E. (n = 3).
We used Malus. hupehensis (Pamp.) Rehd. var. Pingyiensis (hereafter referred to as M. hupehensis Rehd.), a common rootstock of apple, as the test material. M. hupehensis Rehd. seeds were soaked in water and mixed with an appropriate amount of fine sand. In January 2021, they were layered at approximately 4°C for 30 days. After the seeds germinated and became white, they were sown in plastic seedling trays. By mid-April, the seedlings were transplanted when they reached the six-leaf-stage.
Experimental Design and Treatment
The test was divided into six treatments, including the control (CK1) and high standard control (CK2, methyl bromide fumigation), methyl bromide fumigation treatment can effectively prevent ARD (Smith, 1994). Wang et al. (2021) reported that the biomass of M. hupehensis Rehd. seedlings after treated with methyl bromide fumigation was significantly higher than the control soil. The ZnO-NPs suspension (250 mg/L) was applied in four different ways: foliar spraying (T1), foliar soaking (T2), root soaking (T3), and soil soaking (T4). Methyl bromide fumigation was carried out for 26 days before transplantation: methyl bromide is hydrolyzed to methanol and the bromide ion, with a half-life of 20–26 days (Kaushik, 2021). The soaked soil was treated for 7 days before transplantation, and the uniform suspension was slowly poured into the mud pot containing the old orchard soil. To prevent the interference of the external environment from causing unpredictable errors, the mud pots were sealed with a white plastic film. T1, T2, and T3 were carried out before transplantation, and the mud pots were sealed with a plastic film to prevent the suspension from entering the soil. There are ten replicates for each treatment. A M. hupehensis Rehd. seedling with uniform growth was planted in each pot, and the administration of fertilizer and water was identical across the groups. Three pots of seedlings with similar growth patterns were selected, the top soil in the mud pot was removed, and the soil around the rhizosphere with a depth of 10–30 cm was collected on 20 July 2021. The mixed soil samples were passed through a 5 mm sieve to remove visible organisms, stones, and other debris, packed, and sealed in a bag that was brought back to the lab. A portion of the fresh soil was stored in a −80°C freezer for high-throughput sequencing, and the other portion was used for the determination of microorganism abundance. Finally, the remaining soil samples were air dried in a ventilated area for the determination of soil enzyme activity.
ZnO In Vitro Antifungal Ability
To examine the antifungal activity of ZnO-NPs, different concentrations (0, 10, 50, 250, 1000 mg/L) of the ZnO-NPs suspension were added to potato dextrose agar (PDA) medium. In order to disperse the suspension evenly into the PDA medium, we immediately added the freshly prepared ZnO-NPs suspensions into PDA medium after dispersion. The suspension was mixed for 10 min to obtain a dispersed suspension (Shang et al., 2020) and then pour the suspension into the disposable flat plate. After the suspension has cooled down completely, fresh cake of Fusarium proliferatum (10 mm diameter) were cut and transfer to the center of PDA plates, and its growth was observed at 24, 72, 120, and 168 h.
Soil Microbial Quantity
The uniformly mixed fresh soil samples were analyzed for microorganisms using the dilution plating technique according to a standardized method. In brief, 10 g of soil was added into 90 g of sterile double-distilled water and mixed in a shaker set at a suitable speed. The fungi were cultivated on PDA plates, and the bacteria were cultivated on Luria broth/agar plates (Kinghunt Biological Co., Nantong, China) according to the manufacturer’s instructions. The soil was serially diluted and the plating technique was used to determine the number of bacteria and fungi (Ujjainiya et al., 2021), and bacteria were counted for 1 day (24 h) and fungi were counted for 2 days (48 h).
Soil Enzyme Activity
Soil urease activity was measured using the sodium phenate-sodium hypochlorite colorimetric method. First, weigh 5 g of the air-dried soil sample and place it in a 50 ml erlenmeyer flask, add 1 ml of toluene, and shake until the mixture is uniform. After waiting for 15 min, add 10% urea solution and 10 ml of citric acid buffer to the Erlenmeyer flask, shake well and incubate at 37°C for 24 h. Filter 1 ml of the filtrate to a 50 ml flask. Add 4 ml of sodium phenate solution and 3 ml of sodium hypochlorite solution, slowly stand for 20 min, dilute the mixture to 50 ml, and use a spectrophotometer to compare the color at 578 nm (the blue color of indophenol remains stable). Urease activity is calculated by subtracting the absorbance value of the sample from the difference in the absorbance value of the control sample, and the ammonia nitrogen content is calculated according to the standard curve.
The formula for determining soil urease (Ure) activity was as follows:
Where a is the concentration of ammonium-nitrogen obtained from the standard curve (mg/ml), V is the volume of the chromatic liquid (50 ml), n is the separation multiple, and m is the weight of the drying soil (g).
Soil neutral phosphatase activity was measured using a colorimetric assay with disodium phenyl phosphate. First, weigh 5 g of air-dried soil sample and place it in a 200 ml erlenmeyer flask, and add 2.5 ml of toluene. After shaking well for 15 min, add 20 ml of 0.5% phenyl disodium phosphate. Incubate at 37°C for 24 h, add 100 ml of 0.3% aluminum sulfate solution to the flask and filter. Then suck 3 ml of the filtrate into a 50 ml volumetric flask, and add 5 ml buffer and four drops of chlorinated dibromo-p-benzoquinone imine reagent to each bottle. Dilute the solution to the scale line, and perform colorimetric determination after 30 min, and compare the color at 660 nm with a spectrophotometer. Take 1, 3, 5, 7, 9, 11, 13 ml of phenolic working solution for color development, measure the volume, and draw a standard curve. After reaching the color stability, draw a standard curve by colorimetry. Phosphatase activity is expressed in micrograms per gram of soil phenol content.
The formula for determining soil neutral phosphatase (Pho) activity was as follows:
Where a is the concentration of phenol obtained from the standard curve (mg/ml), V is the volume of the chromatic liquid (50 ml), n is the separation multiple, and m is the weight of the drying soil (g).
Sucrase activity was measured using the 3,5-dinitrosalicylic acid colorimetric method. First, weigh 5 g of air-dried soil sample and place it in a 50 ml Erlenmeyer flask, and inject 10 ml of 1% starch solution. Add 10 ml of phosphate buffer with pH 5.6 and five drops of toluene, shake well, incubate at 37°C for 24 h, filter the suspension after incubation. Pour 1 ml of filtrate into a 50 ml measuring flask, add 2 ml of 3,5-dinitrosalicylic acid solution, heat it in a boiling water bath for 5 min, and then place the measuring flask in running water to cool. After diluting to 50 ml, perform color comparison on a 508 nm spectrophotometer. Take glucose solution as standard (Chen et al., 2021).
The formula for determining soil sucrase (Suc) activity was as follows:
Where a is the concentration of glucose obtained from the standard curve (mg/ml), V is the volume of the chromatic liquid (50 ml), n is the separation multiple, and m is the weight of the drying soil (g).
Plant Biomass
Three M. hupehensis Rehd. seedlings with similar growth patterns were selected, and the height, ground diameter, and fresh weight of the plants were measured with a ruler, a vernier caliper, and an electronic balance, respectively. Thereafter, the seedlings were placed into a paper bag and stored in an oven set at 80°C. When they were completely dried, the dry weight of the plants was determined.
DNA Extraction and Real-Time Quantitative Analysis of F. solani and F. oxysporum
Total genomic DNA was extracted and purified using the E. Z.N.A. Soil DNA kit (Omega Bio-tek, Norcross, GA, United States) according to the manufacturer’s instructions. The CFX Connect system (Bio-Rad, Hercules, CA, United States) was used to determine the expression levels of F. solani genes in the soil by real-time quantitative PCR. The primers were FR (5′-GGCCTGAGGGTTGTAATG-3′), FF (5′-CGAGTTATACAACTCATCAACC-3′), JR (5′-GAACGCGAATTAACGC-GAGTC-3′), and JF (5′-CATACCACTTGTTGTCTCGGC3′). The reactions were performed according to the instructions of the SYBR Premix Ex Taq kit (TaKaRa Biotech Co., Ltd., Dalian, China). Each 25-μl reaction contained 1.5 μl of DNA, 12.5 μl of SYBR Premix Ex Taq II, 1 μl of each primer, and 9 μl of sterile double-distilled water. The thermal cycling parameters were as follows: pre-denaturation at 95°C for 30 s, followed by 40 cycles of denaturation at 95°C for 5 s and annealing at 60°C for 30 s. A final extension at 72°C for 10 min was also included.
DNA Extraction and High-Throughput Sequencing Analysis
DNA was extracted using the Fast DNA SPIN Soil kit (MP Biomedicals, Solon, OH, United States) and quantified using the NanoDrop spectrophotometer (Thermo Fisher Scientific, Waltham, MA, United States). The fungal internal transcribed spacer (ITS) region was double-end sequenced on the Illumina MiSeq platform (San Diego, CA, United States). PCR amplification of the 16S rRNA gene was conducted using the primers 515F (5′-GTGCCAGCMGCCGCGGTAA-3′) and 926R (5′-CCGTCAATTCMTTTGAGTTT-3′). The sequences of the primers were ITS1F (5′-CTTGGTCATTTAGAGGAAGTAA-3′) and ITS2 (5′-GCTGCGTTCTTCATCGATGC-3′) (Amato et al., 2013). Trans Start Fast Pfu DNA polymerase was used for PCR amplification in the GeneAmp 9700 PCR system (Applied Biosystems, Foster City, CA, United States). Each 20-μl reaction system contained 0.8 μl of DNA (final concentration, 10 ng), 4 μl of 5× FastPfu buffer, 2 μl of 2.5 mM dNTPs, 0.8 μl of each primer (5 μM), 0.4 μl of FastPfu polymerase, 0.2 μl of BSA, and sterile double-distilled water to a final volume of 20 μl. The thermal cycling conditions were as follows: pre-denaturation at 95°C for 3 min, followed by 35 cycles of denaturation at 95°C for 30 s, annealing at 55°C for 30 s, and extension at 72°C for 45 s. A final extension at 72°C for 10 min was also included.
Statistical Analysis
Data were presented as mean ± standard deviation of triplicate cultures. Analysis of variance was performed using SPSS 23.0 software (SPSS Inc., Chicago, IL, United States), and significant differences were detected by Duncan’s new complex range method. p < 0.05 was considered statistically significant. Adobe Illustrator CC 2018 (San Jose, CA, United States) was used to draw schematic diagrams and typesetting pictures and GraphPad Prism 8.0 (Origin Lab Corp., San Diego, CA, United States) was used to make a bar chart. Fungi abundances in the soil after different treatments were determined using R language and Circos 0.67–7 software. Based on the operational taxonomic unit (OTU) results, the diversities of Shannon, Chao, Ace, and Simpson indices were calculated by Mothur software. Principal coordinates analysis (PCoA) was performed using R language.
Results
Effect of Different Concentrations of ZnO-NPs on the Growth of Fusarium proliferatum
Between 24 and 72 h, the growth of fungal hyphae was similar, and there was no significant difference between the time points. Compared with the control at 72–120 h, all treatments had inhibitory effects (Figure 1A), and the best antifungal effect occurred at a concentration of 250 mg/L. The inhibition rate was 63% when fungi were cultured for 168 h. As shown in Figure 1B, the growth rate was the slowest at 250 mg/L, it showed that 250 mg/L ZnO-NPs had the best effect on inhibiting fungi, so it was selected as the best concentration for the pot experiments.
Effects of Different Application Methods of ZnO-NPs on The Quantity of Soil Microorganisms
As shown in Table 2, the number of bacteria in the soil increased and the amount of fungi in the soil decreased significantly after the soil treated by methyl bromide fumigation. Among the four application methods, T4 was the most similar to methyl bromide fumigation, followed by T3. After T1 and T2, the number of fungi in the soil did not change compared with the CK1, but the number of bacteria increased significantly. Compared with the CK1, the number of fungi in the CK2, T1, T2, T3, and T4 groups decreased by 63.2, 21.0, 7.35, 42.3, and 63.2%, and the number of bacteria increased by 103, 442, 736, 93.4, and 126%, respectively.
Effects of Different Application Methods of ZnO-NPs on Gene Copy Numbers of F. solani and F. oxysporum in Soil
Real-time polymerase chain reactions were used for absolute quantitative analysis of the copy numbers of F. solani and F. oxysporum in the soil. All treatments reduced the copy numbers of F. solani and F. oxysporum (Figure 2). The copy numbers of F. solani decreased by 16.5, 28.2, 50.6, 60.7, and 55.7% in T1, T2, T3, T4, and CK2. The copy numbers of F. oxysporum decreased by 28.3, 34.8, 54.9, 61.4, and 68.9% in T1, T2, T3, T4, and CK2.
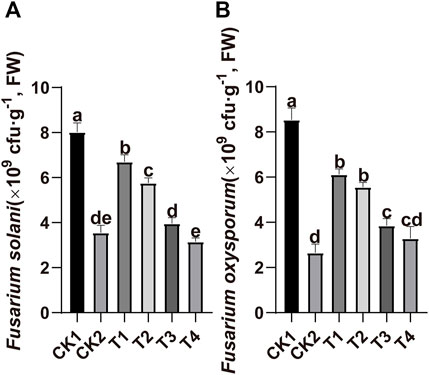
FIGURE 2. Effects of different treatment on the gene copy number of F. solani and F. oxysporum in replant soil. (A) F. solani and (B) F. oxysporum. CK1: control, CK2: high standard control, T1:foliar spraying, T2:foliar soaking, T3: root soaking, T4: soil soaking. The different letters above the bar indicate that there is a significant difference between the mean values of different treatments using one-way ANOVA and Duncan’s multiple range test (p < 0.05). Error bars indicate ± S.E. (n = 3).
Effect of Soaking Soil With ZnO-NPs on Microbial Community
After applying the ZnO-NPs suspension to the soil, high-throughput sequencing revealed that the fungal ACE and Chao indexes changed little compared with the CK1 at the OTU level. The Shannon index was 30.4% lower than that of CK1, and the Simpson index was 303% higher than that of CK1, which was consistent with the change trend of CK2 (Figure 3). The ZnO-NPs treatment significantly changed the structure of the soil microbial community (Figures 4C, 5A), and the top twelve fungal genera with relative abundances were Tausonia, Mortierella, Neocosmospora, Trichocladium, Lophotrichus, Scedosporium, Solicoccozyma, Leuconeurospora, Emericellopsi, Gibberella, Phialemonium, and Fusarium (the three unnamed genera were removed). The relative abundances of Tausonia, Chaetomium, and Mrakia increased by 171, 203, and 720% in T4 and by 84.3, 0.551, and 551% in CK2, respectively, compared with CK1. The relative abundances of Neocosmospora and Gibberella decreased significantly compared with CK1. In particular, the relative abundance of Fusarium after ZnO-NPs treatment was reduced by 84.1% compared with the CK1. Furthermore, compared with the CK1, the relative abundance of Fusarium was also reduced by 86.6% in the CK2 (Figure 4B). The top ten bacteria phylum with relative abundances were Proteobacteria, Actinobacteriota, Acidobacteriota, Chloroflexi, Gemmatimonadota, Bacteroidota, Myxococcota, Firmicutes, Cyanobacteria, Nitrospirota. Compared the three treatments, the difference is the most significant at the Myxococcota, Cyanobacteria, Nitrospirota and Verrucomicrobiota phylum level (Figure 5B). In fungal PCoA analysis, the first principal component was 61.67%, and the second principal component was 23.46% (Figure 6A). In bacteria PCoA analysis, the first principal component was 52.63%, and the second principal component was 24.67% (Figure 6B). The microbial community was roughly divided into three clusters: 1) CK1: control; 2) CK2: high standard control; and 3) T4: soaking soil. The distance heat map showed that CK1 was significantly different from CK2 and T4 at the fungal genus level, and after CK2 and T4 treatments, there was a certain similarity at the fungal genus level (Figure 4A).
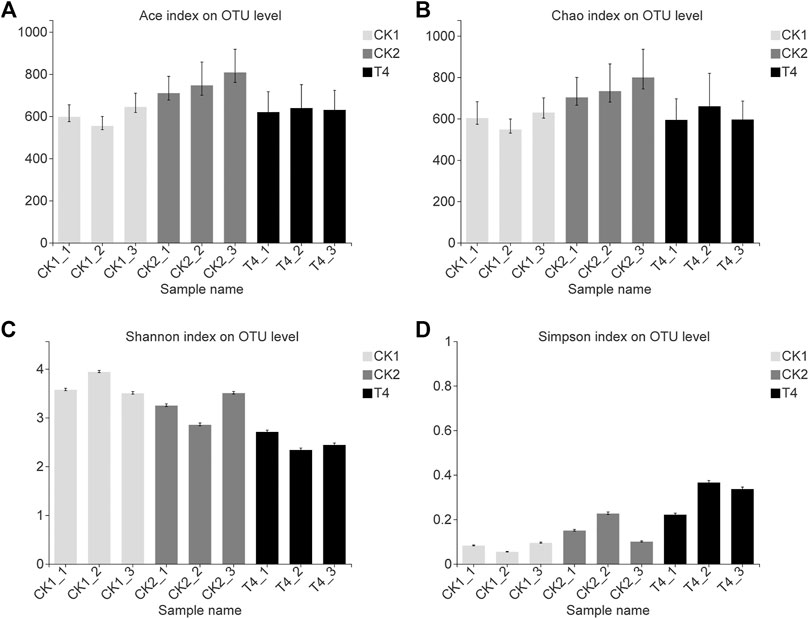
FIGURE 3. Analysis of alpha diversity based on OTU of replant soil fungal. (A) Ace index on OTU level. (B) Chao index on OTU level. (C) Shannon index on OTU level. (D) Simpson index on OTU level. CK1: control, CK2: high standard control, T4: soil soaking. The abscissa is the sample name, and the ordinate is the observed value of a certain index type under the selected classification level. Type I intervals represent the upper and lower limits of the index.
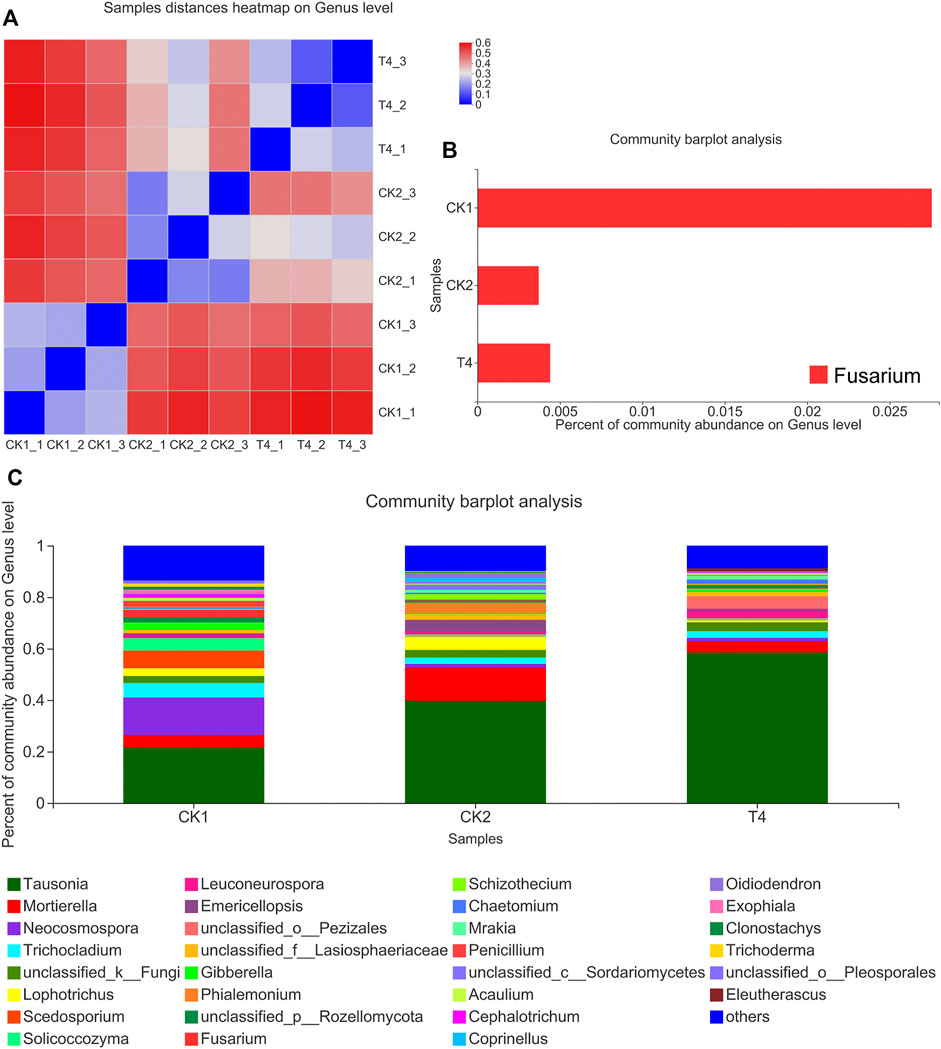
FIGURE 4. Changes of fungal community composition after soaking soil. (A) Samples distances heatmap on Genus level. Both the X and Y axes are samples, and the distance between samples is represented by different color gradients (the right side of the figure is the value represented by the color gradient). (B) Community barplot analysis of single Fusarium. (C) Percent of community abundance on Genus level. The abscissa is the sample name, and the ordinate is the proportion of the species in the sample. The columns of different colors represent different species, and the length of the columns represents the proportion of the species. CK1: control, CK2: high standard control, T4: soil soaking.
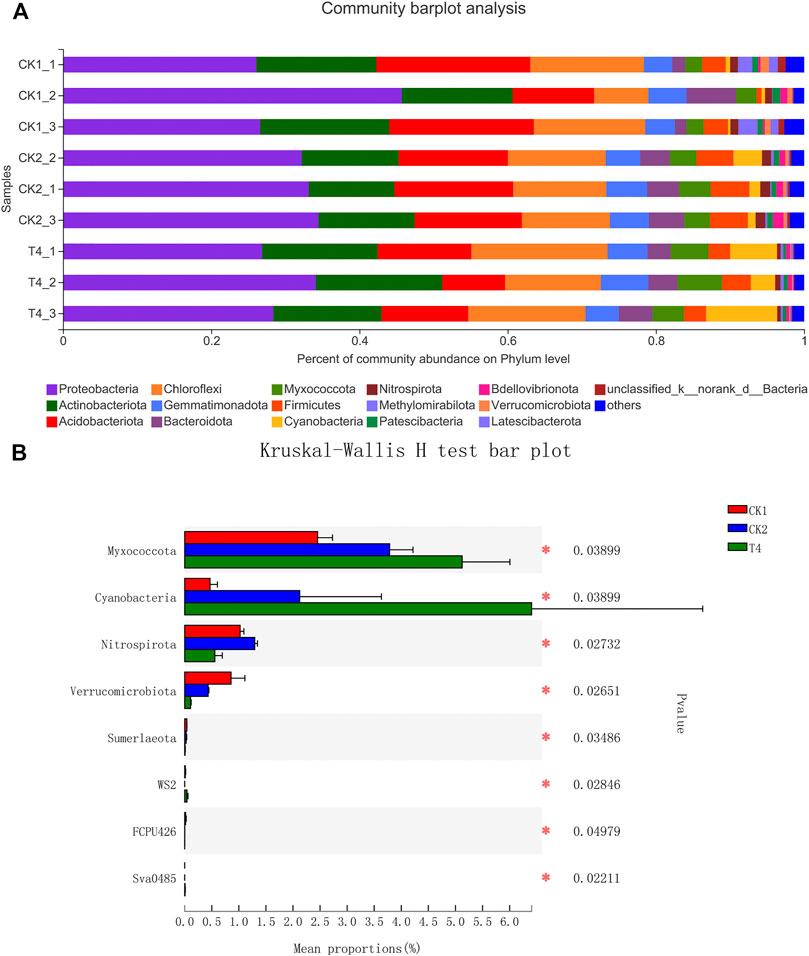
FIGURE 5. Changes of bacteria community composition after soaking soil. (A) Percent of community abundance on Phylum level. The ordinate is the sample name, and the abscissa is the proportion of the species in the sample. The columns of different colors represent different species, and the length of the columns represents the proportion of the species. (B) Kruskal-Wallis H test bar plot. The Y-axis represents the species name at a certain taxonomic level, the X-axis represents the average relative abundance in different groups of species, and the columns with different colors represent different groups; the rightmost is the p value, * 0.01 < p ≤ 0.05, ** 0.001 < p ≤ 0.01, ***p ≤ 0.001. CK1: control, CK2: high standard control, T4: soil soaking.
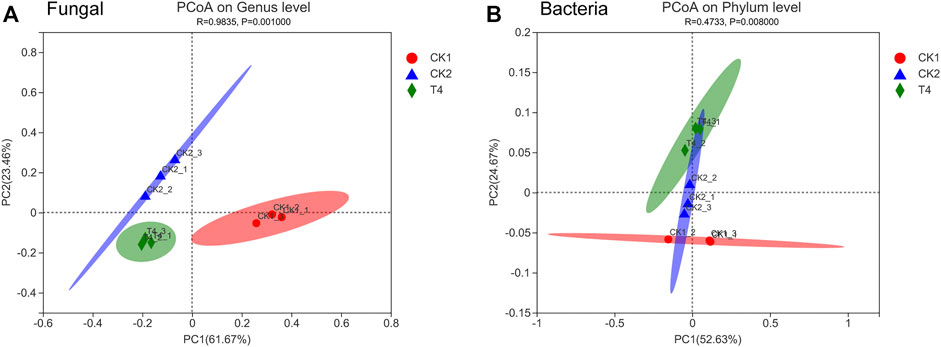
FIGURE 6. Analysis of beta diversity based on Genus level of replant soil fungal and Phylum level of replant soil bacteria. (A) PCoA of fungi and (B) PCoA of bacteria. CK1: control, CK2: high standard control, T4: soil soaking. Principal coordinate analysis (PCoA) was based on the Bray-Curtis distance metric at the genus level, and the results are displayed as a scatter diagram. Different colors and shapes of points indicate different sample groups. The proximity of two sample points is positively related to the similarity of the species compositions of the two samples.
Effects of Different Application Methods of ZnO-NPs on the Soil Enzyme Activities
Different treatments have different effects on soil enzyme activities, as shown in Figure 7. The effects of T1 and T2 on soil enzymes changed little compared with the CK1, while the soil enzyme activities of T3 and T4 changed significantly compared with the CK1, which was similar to the treatment of the CK2. Compared with the CK1, neutral phosphatase, urease, and sucrase were reduced by 32.9, 62.8, and 34.3%, respectively, after soli soaking.
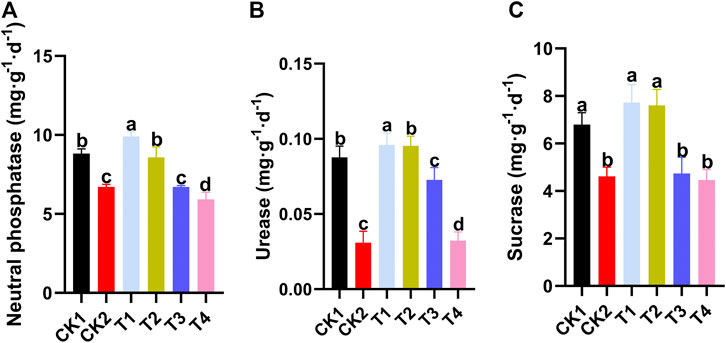
FIGURE 7. Effect of different treatment methods on soil enzyme activity. (A) Neutral phosphatase activity. (B) Urease activity. (C) Sucrase activity. CK1: control, CK2: high standard control, T1:foliar spraying, T2:foliar soaking, T3: root soaking, T4: soil soaking. The different letters above the bar indicate that there is a significant difference between the mean values of different treatments using one-way ANOVA and Duncan’s multiple range test (p < 0.05). Error bars indicate ± S.E. (n = 3).
Effects of Different Application Methods of ZnO-NPs on the Biomass of M. hupehensis Rehd. Seedlings
Different treatments increased the biomass of M. hupehensis Rehd. seedlings (Table 3). The seedling biomass was best after T4 and CK2 treatment. After the treatment, the volume and the quantity of the seedling leaves were increased. After T3 treatment, the seedling height, ground diameter, fresh weight, and dry weight were 1.36 times, 2.05 times, 2.65 times, and 2.75 times that of the CK1, respectively, which was similar to the growth of seedlings after the CK2 treatment, as shown in Figure 8.
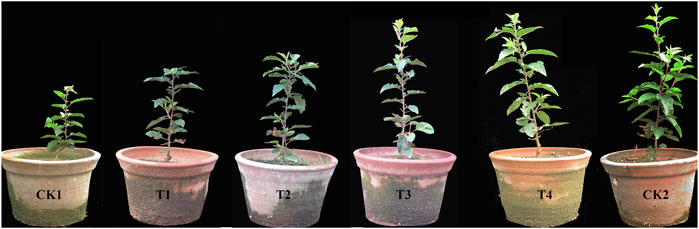
FIGURE 8. Growth of M. hupehensis (Pamp.) Rehd. var. Pingyiensis after different treatments. CK1: control, CK2: high standard control, T1:foliar spraying, T2:foliar soaking, T3: root soaking, T4: soil soaking.
Discussion
Although researchers have been trying to find a way to overcome ARD, no specific measures have been identified to completely overcome ARD (Cavael et al., 2019). Pasteurization, γ-irradiation, and soil fumigation can all reduce the symptoms of ARD (Borgatta et al., 2018; Fe Lix et al., 2018), indicating that microorganisms are the main causative factors. ZnO-NPs have a significant inhibitory effect on a variety of fungi (Malandrakis et al., 2019), and this study aimed to determine whether ZnO-NPs could be used as a new type of treatment for ARD.
Presently, there are few studies on the antifungal property of ZnO-NPs, and concentrations ranging from 10 to 3,000 mg/L have been reported to have inhibitory effects on fungi or bacteria (Pullagurala et al., 2018). In this study, four different concentrations (10, 50, 250, 1,000 mg/L) were used to inhibit the growth of fungal hyphae. As shown in Figure 1, the growth of F. proliferatum was inhibited, and the fungal hyphae underwent severe deformation, consistent with the results of He et al. (2011) who reported that ZnO-NPs could inhibit the growth of Botrytis cinerea by affecting cell function, thereby resulting in the deformation of fungal hyphae. Surprisingly, ZnO-NPs at a concentration of 250 mg/L had a better antifungal effect than ZnO-NPs at a concentration of 1,000 mg/L. This may have been due to the fact that nanomaterials at high concentrations can aggregate in solution, making the antifungal effect worse (Palmieri et al., 2017).
Soil enzymes play important roles in material circulation and energy flow in soil ecosystems. For instance, they can directly participate in the transformation, circulation, and release of soil nutrients (C, N, S, P) by mediating the biochemical reactions in the decomposition of organic matter. The soil organic matter content, physical and chemical properties, microbial quantity, and microbial activity are the main factors that affect soil enzyme activity (Bowles et al., 2014); therefore, soil enzymes can be used as indicators of the activities of soil microorganisms. In this study, the application of ZnO-NPs to leaves had little effect on the soil enzymes; however, when they were applied to plant roots or soil, the soil enzyme activity was reduced (Eivazi et al., 2018). After releasing nano silver oxide into the soil, and then measuring soil acid phosphatase, β-glucosaminidase, β-glucosidase, and arylsulfatase activities within 1 h and 1 week, it was demonstrated that the activities of the four enzymes were reduced, consistent with our findings. Similarly, Fayuan Wang et al. (2018) reported that ZnO-NPs inhibited the activities of urease, phosphatase, and catalase, whereas Kim et al. (2011) observed that ZnO-NPs inhibited the activities of soil enzymes in pot research. By measuring the number of microorganisms in the soil after it was inoculated with arbuscular mycorrhizal fungi, it was observed that the activities of most soil enzymes increased (Qin et al., 2020). Similarly, Naseby et al. (2010) found that after inoculating pea soil with Trichoderma, the activities of soil acid phosphatase and urease increased. Therefore, the application of ZnO-NPs decreased the number of soil microorganisms, including beneficial microorganisms and harmful microorganisms, and the reduction of microbial activity may be the main reason for the decrease in soil enzyme activity.
Soil microorganisms play important roles in the soil. They can participate in nitrogen fixation, produce hormones, inhibit pathogens, and resist drought (Baum et al., 2015; Jayne and Quigley, 2014). The community composition of the fungal population is strongly affected by plants, which in turn affect plant growth through symbiosis, pathogenicity, and nutrient cycling (Hannula et al., 2017; Wagg et al., 2014). Continuous cropping can increase the abundance of pathogenic fungi in the soil, and then change the fungal community structure, which can adversely affect plant health (Liu et al., 2019). The Illumina MiSeq platform was used to sequence the microorganisms in the soil environment treated with CK1, CK2 and T4, and the statistical analysis of the diversity index of each sample is shown in Figure 3. By analyzing the ACE, Chao, Shannon and Simpson indexes, we found that, compared with CK1, there was no change in the microbial abundance after T4 treatment, but the diversity of the microbial community was reduced to a certain extent. A previous study has reported that soil treatment with 5 mg/ml nano-ZnO reduces the microbial diversity (Ge et al., 2011). In addition, 10 and 1,000 mg/L nano-ZnO also reduce the microbial diversity in poplar leaves (Du et al., 2019). We hope that the replanted soil can achieve the effect of methyl bromide fumigation after treatment with ZnO-NPs, so we analyzed the composition of the soil fungal species at the genus level. In Figures 4A, 6, we obtained surprising results. The community composition of the soil treated with T4 and CK2 was very similar, and there was a significant difference compared with CK1, indicating that treatment with ZnO-NPs achieved the effect we expected, After T4 treatment, the relative abundances of Tausonia, Chaetomium, and Mrakia were increased significantly. It has been reported that the addition of biochar to the soil had a mitigating effect on eggplant Verticillium wilt, among which Tausonia, Chaetomium, Mortierella, and Humicola were the dominant fungi, indicating that they may play a positive role in disease suppression (Ogundeji et al., 2021). After Zhou et al. (2021) added antagonistic bacteria to the soil of winter jujube (Ziziphus jujuba Mill. “Dongzao”) with Botrytis cinerea, Botryosphaeria dothidea, and Colletotrichum gloeosporioides, the relative abundance of Tausonia also increased significantly. However, the specific mechanism behind its effects on plant growth is unknown. Chaetomium can prevent and treat plant diseases and promote plant growth. Many types of Chaetomium can produce antibiotics to treat potato late blight caused by Phytophthora and tomato blight caused by F. oxysporum (Soytong et al., 2001; Shanthiyaa et al., 2013). Furthermore, natural products and fungal metabolites released from Chaetomium can also promote plant growth and induce plant immunity. Xin et al. (2017) reported that Chaetomium globosum D38 promoted the growth and the secondary metabolism of salvia, significantly increasing the accumulation of tanshinone and salvianolic acid. As such, it is a beneficial fungus. High-throughput results showed that the relative abundances of Neocosmospora, Gibberella, and Fusarium decreased significantly, and these three fungi have been reported to induce plant diseases and cause serious economic losses. Dhar et al. (2005) reported that cowpea wilt was caused by Neocosmospora in pot and field experiments. Some diseases are not caused by a single bacterium. For example, citrus dry root rot is a multifactorial disease mainly attributed to Neocosmospora solani as well as other species of Neocosmospora and Fusarium spp (Ezrari et al., 2021). Riaz et al. (2020) identified Neocosmospora as a new pathogen of potato stem rot. Gibberella is a plant pathogenic fungus that produces gibberellins and secondary metabolites such as carotenoids, bikaverin, fusarin, phytotoxins, and mycotoxins (Brückner, 1992; Karov et al., 2009). Bakanae disease in rice is caused by Gibberella, which was first described in Japan and now is widely distributed throughout Asia, Africa, North America, and Italy (Prà et al., 2010). Gongshuai Wang et al. (2018) demonstrated that Fusarium was positively correlated with the severity of ARD and is a causative factor of ARD. After ZnO-NPs treatment, the microbial community in the soil changed, and the number of pathogenic bacteria decreased significantly, so that the plants were protected from the pathogenic fungi. However, through high-throughput sequencing of soil bacteria after treatment with T4 and CK2, we found that the soil bacterial community structure at the phylum level after T4 and CK2 treatments showed only subtle changes compared with CK1. There were significant differences in the relative abundances of Myxococcota, Cyanobacteria and Verrucomicrobiota among the treatments. A previous study has demonstrated that some bacteria, such as Patescibacteria, Chloroflexi, Myxococcota and Bacteroidota, allow tea plants to obtain sufficient nutrients from the soil (Wu et al., 2021). Cyanobacteria are photosynthetic bacteria that are a fundamental component of soil biocrusts, as well as enhance soil function and structure and promote plant growth (Chua et al., 2020). Singh et al. (2011) reported that Cyanobacteria inoculation can promote the growth of rice and increase the resistance of rice.
After 90 days of treatment, our data showed that all four application methods of ZnO-NPs had a positive impact on plant biomass, including plant height, ground diameter, fresh weight, and dry weight. It has been confirmed that ZnO-NPs had a positive effect on biomass when applied to other species. ZnO-NPs sprayed on the leaves of Sophora sphaerocarpa could increase the weight of fresh leaves and the soluble sugar content of leaves, as well as promote the growth of Sophora sphaerocarpa seedlings (Wan et al., 2020). Borgatta et al. (2018) reported that Cu3(PO4)2·3H2O nanosheets could overcome watermelon wilt caused by Fusarium oxysporum f. sp. Niveum. Our results showed that the soaking method was more effective than the spraying method in overcoming the disease, which may have been caused by the uniformity of the suspension covering the leaves during the treatment. After spraying or soaking the leaves, the number of bacteria in the soil increased significantly compared with the CK1, and it is possible that ZnO-NPs changed the metabolites of the rhizosphere, which increased the number of bacteria around the rhizosphere (Tian et al., 2020). When plants are under external abiotic stress, they can regulate root exudates, and then recruit beneficial microorganisms to overcome and resist the damage (Hartman and Tringe, 2019). A bacterium from the Rhizobium family can interact with legumes to increase the nitrogen fixation ability of plants. In addition to nitrogen fixation, bacteria can also increase the utilization of inorganic and organic phosphorus in the soil (Rodrı́Guez and Fraga, 1999; Rosenblueth et al., 2018). This may be the main reason why leaves exposed to ZnO-NPs increased the plant biomass, and the fertilizer effect brought by ZnO-NPs was not excluded. In our study, when ZnO-NPs were applied to the roots or soil, the plants grew the best. Faizan et al. (2020) reported that soaking tomato roots in a ZnO-NPs solution improved the growth and photosynthetic properties of plants and increased the yield of fruits. Van Schoor et al. (2009) reported that Fusarium may play a role in ARD in South Africa. In China, Fusarium is the main causative factor of ARD (Xiang et al., 2021). In other crops, such as soybeans and potatoes, Fusarium has also been identified as the dominant pathogen in continuous cropping soil (Bai et al., 2015). We found that after these two treatments, the number of soil fungi and the relative abundance of Fusarium were reduced. Therefore, we used qPCR to detect the copy numbers of F. solani and F. oxysporum and found that they were decreased significantly compared with the CK1. As shown in Figure 9, after ZnO-NPs were applied to the soil, eukaryotic cells with pinocytosis can engulf ZnO NPs into cells, and then combine with organelles to cause damage to cells (Neal, 2008). Due to the surface activity of ZnO-NPS, intracellular ROS will be generated spontaneously leading to lipid and DNA damage (Premanathan et al., 2011), damage promotes the accumulation of uptake of nanomaterials, resulting in more severe cytotoxicity (Brayner et al., 2006), ultimately leading to cell death. In our experiments, the number of fungi was significantly reduced after T4 treatment, indicating that ZnO-NPs caused the death of fungal cells. The original soil microorganisms were killed, and after planting Malus hupehensis Rehd. seedlings, new soil microbial communities gradually formed, we performed high-throughput sequencing of soil microbes and found that the newly formed soil microbial communities were significantly different from controls. Collins et al. (2012) also reported that ZnO-NPs can alter soil microbial communities. The relative abundance of harmful fungi in the newly formed soil microbial community significantly decreased, which greatly promoted the growth of Malus hupehensis Rehd. seedlings.
Conclusions
In this study, the effects of different application methods of ZnO-NPs in overcoming ARD were studied through pot experiment. Among them, the effects of soaking the soil with the suspension were the best, which significantly reducing the abundance of pathogens Neocosmospora, Gibberella, and Fusarium, improving the microbial community structure, and promoting the growth of M. hupehensis Rehd. seedlings. This is the first time that nanomaterials have been applied to the study of overcoming ARD, thereby providing new insights for the application of other nanomaterials in the treatment of ARD. Fortunately, nanomaterials have a positive effect on overcoming replant disease. However, field experiments have not been carried out in this study. To further promote the application of nanomaterials, field experiments will be carried out to verify the results. In the future, if there are good results in field experiments, then ZnO-NPs can be used as a new chemical material to overcome ARD.
Data Availability Statement
The datasets presented in this study can be found in online repositories. The names of the repository/repositories and accession number(s) can be found below: NCBI [accession: PRJNA788996 and PRJNA789053].
Author Contributions
LP and ZM conceived and designed the experiment. LZ and WJ performed the experiments. MW, XC, and XS analyzed the data. LP, CY, and ZM wrote the paper. All authors gave their final approval of the submitted and published versions.
Funding
The research was supported by China Agriculture Research System of MOF and MARA (CARS-27), Shandong Agricultural Major Applied Technology Innovation Project (SD2019ZZ008), Qingchuang Science and Technology Support Project of Shandong Colleges and Universities (2019KJF020); Natural Science Foundation of Shandong Province (ZR2020MC131); the National Key Research and Development Program of China (2020YFD1000201), Taishan Scholars Funded Project (NO. ts20190923); the National Natural Science Foundation of China (32072510), the Fruit Innovation Team in Shandong Province, China (SDAIT-06-07).
Conflict of Interest
The authors declare that the research was conducted in the absence of any commercial or financial relationships that could be construed as a potential conflict of interest.
Publisher’s Note
All claims expressed in this article are solely those of the authors and do not necessarily represent those of their affiliated organizations, or those of the publisher, the editors and the reviewers. Any product that may be evaluated in this article, or claim that may be made by its manufacturer, is not guaranteed or endorsed by the publisher.
References
Amato, K. R., Yeoman, C. J., Kent, A., Righini, N., Carbonero, F., Estrada, A., et al. (2013). Habitat Degradation Impacts Black Howler Monkey (Alouatta pigra) Gastrointestinal Microbiomes. Isme J. 7, 1344–1353. doi:10.1038/ismej.2013.16
Bai, L., Cui, J., Jie, W., and Cai, B. (2015). Analysis of the Community Compositions of Rhizosphere Fungi in Soybeans Continuous Cropping Fields. Microbiol. Res. 180, 49–56. doi:10.1016/j.micres.2015.07.007
Baum, C., El-Tohamy, W., and Gruda, N. (2015). Increasing the Productivity and Product Quality of Vegetable Crops Using Arbuscular Mycorrhizal Fungi: A Review. Scientia Horticulturae 187, 131–141. doi:10.1016/j.scienta.2015.03.002
Borgatta, J., Ma, C., Hudson-Smith, N., Elmer, W., Plaza Pérez, C. D., Torre-Roche, R. D. L., et al. (2018). Copper Based Nanomaterials Suppress Root Fungal Disease in Watermelon ( Citrullus lanatus ): Role of Particle Morphology, Composition and Dissolution Behavior. ACS Sust. Chem. Eng. 6, 14847–14856. doi:10.1021/acssuschemeng.8b03379
Bowles, T. M., Acosta-Martínez, V., Lderón, F. C., and Jackson, L. E. (2014). Soil Enzyme Activities, Microbial Communities, and Carbon and Nitrogen Availability in Organic Agroecosystems Across an Intensively-Managed Agricultural Landscape. Soil Biol. Biochem. 68, 252–262. doi:10.1016/j.soilbio.2013.10.004
Brayner, R., Ferrari-Iliou, R., Brivois, N., Djediat, S., Benedetti, M. F., and Fiévet, F. (2006). Toxicological Impact Studies Based on Escherichia coli Bacteria in Ultrafine ZnO Nanoparticles Colloidal Medium. Nano Lett. 6 (4), 866–870. doi:10.1021/nl052326h
Brückner, B. (1992). Regulation of Gibberellin Formation by the Fungus Gibberella fujikuroi. Ciba Found. Symp. 171, 129. doi:10.1002/9780470514344.ch8
Cavael, U., Diehl, K., and Lentzsch, P. (2019). Assessment of Growth Suppression in Apple Production with Replant Soils. Ecol. Indicators 109, 105846. doi:10.1016/j.ecolind.2019.105846
Chen, R., Jiang, W., Duan, Y., Qiao, H., Fan, H., Chen, X., et al. (2021). Effect of Emerging Soil Chemical Amendments on the Replant Soil Environment and Growth of Malus Hupehensis Rehd. Seedlings. ACS Omega 6, 20445–20454. doi:10.1021/acsomega.1c02447
Chua, M., Erickson, T. E., Merritt, D. J., Chilton, A. M., Ooi, M. K., and Muñoz‐Rojas, M. (2020). Bio‐priming Seeds with Cyanobacteria: Effects on Native Plant Growth and Soil Properties. Restoration Ecol. 28, S168–S176. doi:10.1111/rec.13040
Collins, D., Luxton, T., Kumar, N., Shah, S., Walker, V. K., and Shah, V. (2012). Assessing the Impact of Copper and Zinc Oxide Nanoparticles on Soil: A Field Study. PLoS One 7, e42663. doi:10.1371/journal.pone.0042663
Dhar, V., Chaudhary, R., Mishra, S., and Khan, A. (2005). Occurrence of Pigeonpea Wilt Caused by Neocosmospora Vasinfecta. Indian J. Pulses Res. 18, 254–255.
Dimkpa, C. O., Mclean, J. E., Britt, D. W., and Anderson, A. J. (2013). Antifungal Activity of ZnO Nanoparticles and Their Interactive Effect with a Biocontrol Bacterium on Growth Antagonism of the Plant Pathogen Fusarium Graminearum. Biometals 26 (6), 913–924. doi:10.1007/s10534-013-9667-6
Du, J., Zhang, Y., Guo, R., Meng, F., Gao, Y., Ma, C., et al. (2019). Harmful Effect of Nanoparticles on the Functions of Freshwater Ecosystems: Insight into nanoZnO-Polluted Stream. Chemosphere 214 (JAN), 830–838. doi:10.1016/j.chemosphere.2018.09.171
Eivazi, F., Afrasiabi, Z., and Jose, E. (2018). Effects of Silver Nanoparticles on the Activities of Soil Enzymes Involved in Carbon and Nutrient Cycling. Pedosphere 28, 209–214. doi:10.1016/S1002-0160(18)60019-0
Ezrari, S., Mhidra, O., Radouane, N., Tahiri, A., Polizzi, G., Lazraq, A., et al. (2021). Potential Role of Rhizobacteria Isolated from Citrus Rhizosphere for Biological Control of Citrus Dry Root Rot. Plants 10, 872. doi:10.3390/plants10050872
Faizan, M., Faraz, A., and Hayat, S. (2020). Effective Use of Zinc Oxide Nanoparticles through Root Dipping on the Performance of Growth, Quality, Photosynthesis and Antioxidant System in Tomato. J. Plant Biochem. Biotechnol. 29, 553–567. doi:10.1007/s13562-019-00525-z
Wang, F., Adams, C. A., Shi, Z., and Sun, Y. (2018). Combined Effects of ZnO NPs and Cd on Sweet Sorghum as Influenced by an Arbuscular Mycorrhizal Fungus. Chemosphere 209, 421–429. doi:10.1016/j.chemosphere.2018.06.099
Fe Lix, M., Margaux, S., Eva, L., Stefan, P., Andreas, W., and Traud, W. (2018). Induction and Diagnosis of Apple Replant Disease (ARD): A Matter of Heterogeneous Soil Properties? Scientia Horticulturae 241, 167–177. doi:10.1016/j.scienta.2018.06.076
Franke-Whittle, I. H., Manici, L. M., Insam, H., and Stres, B. (2015). Rhizosphere Bacteria and Fungi Associated with Plant Growth in Soils of Three Replanted Apple Orchards. Plant and Soil 395, 317–333. doi:10.1007/s11104-015-2562-x
Ge, Y., Schimel, J. P., and Holden, P. A. (2011). Evidence for Negative Effects of TiO2 and ZnO Nanoparticles on Soil Bacterial Communities. Environ. Sci. Tech. 45 (4), 1659–1664. doi:10.1021/es103040t
Gongshuai Wang, G., Yin, C., Pan, F., Wang, X., Xiang, L., Wang, Y., et al. (2018). Analysis of the Fungal Community in Apple Replanted Soil Around Bohai Gulf. Hortic. Plant J. 4, 175–181. doi:10.1016/j.hpj.2018.05.003
González‐Merino, A. M., Hernández‐Juárez, A., Betancourt‐Galindo, R., Ochoa‐Fuentes, Y. M., Valdez‐Aguilar, L. A., and Limón‐Corona, M. L. (2021). Antifungal Activity of Zinc Oxide Nanoparticles in Fusarium Oxysporum-Solanum lycopersicum Pathosystem under Controlled Conditions. J. Phytopathology 169, 533–544. doi:10.1111/jph.13023
Grunewaldt-Stöcker, G., Mahnkopp, F., Popp, C., Maiss, E., and Winkelmann, T. (2019). Diagnosis of Apple Replant Disease (ARD): Microscopic Evidence of Early Symptoms in Fine Roots of Different Apple Rootstock Genotypes. Scientia Horticulturae 243, 583–594. doi:10.1016/j.scienta.2018.09.014
Hannula, S. E., Morriën, E., De Hollander, M., Van, D. P., Wim, H., Van Veen, J. A., et al. (2017). Shifts in Rhizosphere Fungal Community During Secondary Succession Following Abandonment from Agriculture. Isme J. 11, 2294–2304. doi:10.1038/ismej.2017.90
Hartman, K., and Tringe, S. G. (2019). Interactions Between Plants and Soil Shaping the Root Microbiome Under Abiotic Stress. Biochem. J. 476, 2705–2724. doi:10.1042/BCJ20180615
He, L., Liu, Y., Mustapha, A., and Lin, M. (2011). Antifungal Activity of Zinc Oxide Nanoparticles against Botrytis Cinerea and Penicillium expansum. Microbiol. Res. 166, 207–215. doi:10.1016/j.micres.2010.03.003
Jayne, B., and Quigley, M. (2014). Influence of Arbuscular Mycorrhiza on Growth and Reproductive Response of Plants Under Water Deficit: A Meta-Analysis. Mycorrhiza 24 (2), 109–119. doi:10.1007/s00572-013-0515-x
Karov, I., Mitrev, S., and Arsov, E. (2009). Giberella Fujikuroi (Wollenweber) the New Parasitical Fungus on rice in the Republic of Macedonia. Proc. Nat. Sci. Matica Srpska Novi Sad. 116 , 175–182. doi:10.2298/ZMSPN0916175K
Kaushik, R. (2021). Methyl Bromide: Risk Assessment, Environmental, and Health hazard. Hazardous Gases. Haridwar: Elsevier, 239–250. doi:10.1016/b978-0-323-89857-7.00003-7
Kim, S., Kim, J., and Lee, I. (2011). Effects of Zn and ZnO Nanoparticles and Zn2+ on Soil Enzyme Activity and Bioaccumulation of Zn in Cucumis Sativus. Chem. Ecol. 27, 49–55. doi:10.1080/02757540.2010.529074
Li, X., Mei, W., Fengbing, P., Gongshuai, W., Weitao, J., Yanfang, W., et al. (2020). Transcriptome Analysis Malus Domestica 'M9T337' Root Molecular Responses to Fusarium Solani Infection. Physiol. Mol. Plant Pathol. 113, 101567. doi:10.1016/j.pmpp.2020.101567
Liu, H., Pan, F., Han, X., Song, F., Zhang, Z., Yan, J., et al. (2019). Response of Soil Fungal Community Structure to Long-Term Continuous Soybean Cropping. Front. Microbiol. 9, 3316. doi:10.3389/fmicb.2018.03316
Mai, W. F. (1981). Controlling Replant Diseases of Pome and Stone Fruits in Northeastern United States by Preplant Fumigation. Plant Dis. 65, 859. doi:10.1094/PD-65-859
Malandrakis, A. A., Kavroulakis, N., and Chrysikopoulos, C. V. (2019). Use of Copper, Silver and Zinc Nanoparticles Against Foliar and Soil-Borne Plant Pathogens. Sci. Total Environ. 670, 292–299. doi:10.1016/j.scitotenv.2019.03.210
Mazzola, M. (1998). Elucidation of the Microbial Complex Having a Causal Role in the Development of Apple Replant Disease in Washington. Phytopathology 88, 930–938. doi:10.1094/PHYTO.1998.88.9.930
Mazzola, M., and Manici, L. M. (2012). Apple Replant Disease: Role of Microbial Ecology in Cause and Control. Annu. Rev. Phytopathology 50, 45. doi:10.1146/annurev-phyto-081211-173005
Naseby, D. C., Pascual, J. A., and Lynch, J. M. (2010). Effect of Biocontrol Strains of Trichoderma on Plant Growth, Pythium Ultimum Populations, Soil Microbial Communities and Soil Enzyme Activities. J. Appl. Microbiol. 88, 161–169. doi:10.1046/j.1365-2672.2000.00939.x
Neal, A. L. (2008). What Can Be Inferred from Bacterium–Nanoparticle Interactions about the Potential Consequences of Environmental Exposure to Nanoparticles? Ecotoxicology 17 (5), 362–371. doi:10.1007/s10646-008-0217-x
Nicola, L., Insam, H., Pertot, I., and Stres, B. (2018). Reanalysis of Microbiomes in Soils Affected by Apple Replant Disease (ARD): Old Foes and Novel Suspects lead to the Proposal of Extended Model of Disease Development. Appl. Soil Ecol. 129, 24–33. doi:10.1016/j.apsoil.2018.04.010
Ogundeji, A. O., Li, Y., Liu, X., Meng, L., Sang, P., Mu, Y., et al. (2021). Eggplant by Grafting Enhanced with Biochar Recruits Specific Microbes for Disease Suppression of Verticillium Wilt. Appl. Soil Ecol. 163, 103912. doi:10.1016/j.apsoil.2021.103912
Palmieri, V., Bugli, F., Lauriola, M. C., Cacaci, M., and Spirito, M. (2017). Bacteria Meet Graphene: Modulation of Graphene Oxide Nanosheet Interaction with Human Pathogens for Effective Antimicrobial Therapy. ACS Biomater. Sci. Eng. 3, 619–627. doi:10.1021/acsbiomaterials.6b00812
Politycka, B., and Adamska, D. (2003). Release of Phenolic Compounds from Apple Residues Decomposing in Soil and the Influence of Temperature on Their Degradation. Polish J. Environ. Stud. 12, 95–98.
Prà, M. D., Tonti, S., Pancaldi, D., Nipoti, P., and Alberti, I. (2010). First Report of Fusarium Andiyazi Associated with Rice Bakanae in Italy. Plant Dis. 94, 1070. doi:10.1094/pdis-94-8-1070a
Premanathan, M., Karthikeyan, K., Jeyasubramanian, K., and Manivannan, G. (2011). Selective Toxicity of ZnO Nanoparticles Toward Gram-Positive Bacteria and Cancer Cells by Apoptosis through Lipid Peroxidation. Nanomedicine: Nanotechnology, Biol. Med. 7 (2), 184–192. doi:10.1016/j.nano.2010.10.001
Pullagurala, V., Adisa, I. O., Rawat, S., Kim, B., Barrios, A. C., Medina-Velo, I. A., et al. (2018). Finding the Conditions for the Beneficial Use of ZnO Nanoparticles Towards Plants-A Review. Environ. Pollut. 241, 1175–1181. doi:10.1016/j.envpol.2018.06.036
Qin, M., Zhang, Q., Pan, J., Jiang, S., Liu, Y., Bahadur, A., et al. (2020). Effect of Arbuscular Mycorrhizal Fungi on Soil Enzyme Activity Is Coupled with Increased Plant Biomass. Eur. J. Soil Sci. 71, 84–92. doi:10.1111/ejss.12815
Riaz, M., Akhtar, N., Khan, S. N., Shakeel, M., and Tahir, A. (2020). Neocosmospora Rubicola: An Unrecorded Pathogen from Pakistan Causing Potato Stem Rot. Sarhad J. Agric. 36, 906–912. doi:10.17582/journal.sja/2020/36.3.906.912
Rodrı́Guez, H., and Fraga, R. (1999). Phosphate Solubilizing Bacteria and Their Role in Plant Growth Promotion. Biotechnol. Adv. 17, 319–339. doi:10.1016/S0734-9750(99)00014-2
Rosenblueth, M., Ormeño-Orrillo, E., López-López, A., Rogel, M. A., Reyes-Hernández, B. J., Martínez-Romero, J. C., et al. (2018). Nitrogen Fixation in Cereals. Front. Microbiol. 9, 1794. doi:10.3389/fmicb.2018.01794
Shang, H., Ma, C., Li, C., White, J. C., Polubesova, T., Chefetz, B., et al. (2020). Copper Sulfide Nanoparticles Suppress Gibberella fujikuroi Infection in Rice (Oryza Sativa L.) by Multiple Mechanisms: Contact-Mortality, Nutritional Modulation and Phytohormone Regulation. Environ. Sci. Nano 7, 2632–2643. doi:10.1039/D0EN00535E
Shanthiyaa, V., Saravanakumar, D., Rajendran, L., Karthikeyan, G., and Raguchander, T. (2013). Use of Chaetomium globosum for Biocontrol of Potato Late Blight Disease. Crop Prot. 52, 33–38. doi:10.1016/j.cropro.2013.05.006
Sheng, Y., Wang, H., Wang, M., Li, H., Xiang, L., Pan, F., et al. (2020). Effects of Soil Texture on the Growth of Young Apple Trees and Soil Microbial Community Structure under Replanted Conditions. Hortic. Plant J. 6, 123–131. doi:10.1016/j.hpj.2020.04.003
Singh, D. P., Prabha, R., Yandigeri, M. S., and Arora, D. K. (2011). Cyanobacteria-mediated Phenylpropanoids and Phytohormones in rice (Oryza Sativa) Enhance Plant Growth and Stress Tolerance. Antonie Van Leeuwenhoek 100 (4), 557–568. doi:10.1007/s10482-011-9611-0
Sirelkhatim, A., Mahmud, S., Seeni, A., Kaus, N., Ling, C. A., Bakhori, S., et al. (2015). Review on Zinc Oxide Nanoparticles: Antibacterial Activity and Toxicity Mechanism. Nano-micro Lett. 7, 219–242. doi:10.1007/s40820-015-0040-x
Smith, T. J. (1994). Successful Management of Orchard Replant Disease in Washington. Acta Horticulturae 363 , 161–168. doi:10.17660/ActaHortic.1994.363.22
Sofy, A. R., Hmed, A. A., Alnaggar, E., Dawoud, R. A., and Sofy, M. (2020). Mitigating Effects of Bean Yellow Mosaic Virus Infection in Faba Bean Using New Carboxymethyl Chitosan-Titania Nanobiocomposites. Int. J. Biol. Macromolecules 163, 1261–1275. doi:10.1016/j.ijbiomac.2020.07.066
Soytong, K., Kanokmedhakul, S., Kukongviriyapa, V., and Isobe, M. (2001). Application of Chaetomium Species (Ketomium) as a New Broad Spectrum Biological Fungicide for Plant Disease Control. Fungal Divers. 7, 1–15.
Tian, L., Shen, J., Sun, G., Wang, B., Ji, R., and Zhao, L. (2020). Foliar Application of SiO2 Nanoparticles Alters Soil Metabolite Profiles and Microbial Community Composition in the Pakchoi (Brassica Chinensis L.) Rhizosphere Grown in Contaminated Mine Soil. Environ. Sci. Tech. 54, 13137–13146. doi:10.1021/acs.est.0c03767
Traquair, J. A. (1984). Etiology and Control of Orchard Replant Problems: A Review. Can. J. Plant Pathol. 6, 54–62. doi:10.1080/07060668409501591
Ujjainiya, P., Choudhary, M., Jatav, H. S., Tokala, V. Y., and Minkina, T. (2021). Impact of Weed Management Practices on Soil Microflora and Dehydrogenase Enzyme Activity under Varying Levels of Nitrogen in Winter Season Onion (Allium cepa L.). Bull. Environ. Contam. Toxicol., 1–7. doi:10.1007/s00128-021-03265-w
Van Schoor, L., Denman, S., and Cook, N. (2009). Characterisation of Apple Replant Disease Under South African Conditions and Potential Biological Management Strategies. Scientia Horticulturae 119, 153–162. doi:10.1016/j.scienta.2008.07.032
Wagg, C., Bender, S. F., Widmer, F., and Van Der Heijden, M. G. (2014). Soil Biodiversity and Soil Community Composition Determine Ecosystem Multifunctionality. Proc. Natl. Acad. Sci. 111, 5266–5270. doi:10.1073/pnas.1320054111
Wan, J., Wang, R., Bai, H., Wang, Y., and Xu, J. (2020). Comparative Physiological and Metabolomics Analysis Reveals that Single-Walled Carbon Nanohorns and ZnO Nanoparticles Affect Salt Tolerance in Sophora Alopecuroides. Environ. Sci. Nano. 7, 2968–2981. doi:10.1039/D0EN00582G
Wang, H., Sheng, Y., Jiang, W., Pan, F., Wang, M., Chen, X., et al. (2021). The Effects of Crop Rotation Combinations on the Soil Quality of Old Apple Orchard. Hortic. Plant J. 8, 1–10. doi:10.1016/j.hpj.2021.04.010
Wang, N., Wolf, J., Zhang, F. S., Systems, P. P., Sciences, D., University, W., et al. (2016). Towards Sustainable Intensification of Apple Production in China—Yield Gaps and Nutrient Use Effi Ciency in Apple Farming Systems. J. Integr. Agric. 15, 716–725. doi:10.1016/s2095-3119(15)61099-1
Willett, M., Smith, T. J., Peterson, A. B., Hinman, H., and Watson, J. W. (1994). Growing Profitable Apple Orchards in Replant Sites: an Interdisciplinary Team Approach in Washington State. Horttechnology 4, 175–181. doi:10.21273/HORTTECH.4.2.175
Wu, T., Qin, Y., and Li, M. (2021). Intercropping of Tea (Camellia Sinensis L.) and Chinese Chestnut: Variation in the Structure of Rhizosphere Bacterial Communities. J. Soil Sci. Plant Nutr. 54, 1–13. doi:10.1007/s42729-021-00513-0
Xiang, L., Wang, M., Pan, F., Wang, G., Jiang, W., Wang, Y., et al. (2021). Transcriptome Analysis Malus Domestica ‘M9T337’ Root Molecular Responses to Fusarium Solani Infection. Physiol. Mol. Plant Pathol. 113, 10156725. doi:10.1016/j.pmpp.2020.101567
Xin, Z., Dong, L., Li, X., Han, T., and Zheng, C. (2017). Endophyte Chaetomium globosum D38 Promotes Bioactive Constituents Accumulation and Root Production in Salvia Miltiorrhiza. Front. Microbiol. 8, 2694. doi:10.3389/fmicb.2017.02694
Xu, J., Luo, X., Wang, Y., and Feng, Y. (2017). Evaluation of Zinc Oxide Nanoparticles on Lettuce (Lactuca sativa L.) Growth and Soil Bacterial Community. Environ. Sci. Pollut. Res. 25, 6026–6035. doi:10.1007/s11356-017-0953-7
Yim, B., Smalla, K., and Winkelmann, T. (2013). Evaluation of Apple Replant Problems Based on Different Soil Disinfection Treatments—Links to Soil Microbial Community Structure? Plant and Soil 366, 617–631. doi:10.1007/s11104-012-1454-6
Keywords: apple replant disease, zinc oxide nanoparticles, microbial community, fusarium, antifungal
Citation: Pan L, Zhao L, Jiang W, Wang M, Chen X, Shen X, Yin C and Mao Z (2022) Effect of Zinc Oxide Nanoparticles on the Growth of Malus hupehensis Rehd. Seedlings. Front. Environ. Sci. 10:835194. doi: 10.3389/fenvs.2022.835194
Received: 14 December 2021; Accepted: 24 January 2022;
Published: 09 February 2022.
Edited by:
Rajni Singh, Amity University, IndiaReviewed by:
Pankaj Sharma, Amity University, IndiaSavita Singh, Babu Shivnath Agrawal College, India
Copyright © 2022 Pan, Zhao, Jiang, Wang, Chen, Shen, Yin and Mao. This is an open-access article distributed under the terms of the Creative Commons Attribution License (CC BY). The use, distribution or reproduction in other forums is permitted, provided the original author(s) and the copyright owner(s) are credited and that the original publication in this journal is cited, in accordance with accepted academic practice. No use, distribution or reproduction is permitted which does not comply with these terms.
*Correspondence: Chengmiao Yin, Y215aW5Ac2RhdS5lZHUuY24=; Zhiquan Mao, bXpoaXF1YW5Ac2RhdS5lZHUuY24=