- 1Laboratory of Waste and Biomass, Department of Applied Bioeconomy, Wrocław University of Environmental and Life Sciences, Wrocław, Poland
- 2Shanghai Key Laboratory of Atmospheric Particle Pollution and Prevention (LAP3), Department of Environmental Science and Engineering, Fudan University, Shanghai, China
- 3Shanghai Technical Service Platform for Pollution Control and Resource Utilization of Organic Wastes, Shanghai, China
- 4Shanghai Institute of Pollution Control and Ecological Security, Shanghai, China
- 5Department of Agricultural and Biosystems Engineering, Iowa State University, Ames, IA, United States
Carbon monoxide (CO) is ubiquitous in the environment. In this literature review, the biological CO transformations in ecosystems were summarized as an inspiration for the biorefinery industry. Specifically, for the first time, information about CO fate in soil, water, and the atmosphere was collected, and CO impact on plants, animals, and humans was discussed. The review also addresses the need to develop new solutions to implementing circular bioeconomy and highlights the potential of CO use in biologically mediated processes as an untapped valuable resource. Specific key areas of research were identified as 1) development of water-gas shift (WGS) bioreaction, 2) electricity production during bioelectrochemical syngas conversion (BESs), and 3) electro-fermentation (EF) as a source of added-value biochemicals and fuels.
Introduction
Human’s pursuit of knowing and understanding the mechanisms and interdependencies in the environment certainly applies to carbon monoxide (CO). CO is a colorless, odorless, and highly flammable gas. It is considered toxic and threatens air quality and human health (Mahajan and Jagtap, 2020; Ramezani et al., 2020). The harmful effects of CO on higher living organisms are well-documented based primarily on the formation of carboxyhemoglobin (Ruth-Sahd et al., 2011). CO can also be considered an indirect greenhouse gas (GHG). CO reacts with OH radicals in the atmosphere, depleting OH reservoirs, which can effectively control the primary of GHGs such as methane. Atmospheric CO balance can also contribute to the formation of tropospheric ozone (Rozante et al., 2017). On the other hand, the lack of CO classification as a greenhouse gas has led to its omission in many environmental studies, leaving a considerable gap in knowledge about its formation’s exact conditions and pathways.
However, CO links and participates in many processes and reactions in the ecosphere, being a part of water, soil, and atmospheric chemistry. The topic of CO formation and consumption in various ecosystems seemed to be well-known, and most of the literature on the subject was published in the 20th century. However, the knowledge about CO gained earlier has the potential to be used in innovative and future-oriented concepts related to gas processing. The natural presence of CO in all elements of the environment has provoked people to ask questions about the possibility of using CO for industrial purposes in biological-inspired processes–in the area of biorefinery.
The concept of biorefinery attracts the widespread interest of researchers, industry, and policy makers, being a part of circular economy, which is currently one of the most significant environmental undertakings and challenges of the 21st century. However, the transition from a linear to a circular model requires a high level of awareness, well-established, science-based, and transdisciplinary approaches that acknowledge multiple ramifications that can be linked into a closed loop. Solutions for moving to green and sustainable production and energy systems from conventional fossil sources are needed. The biorefinery concept is well-positioned to address the increasing demand for energy and materials, natural resource depletion, and societal ecological awareness, with CO as one of the promising chemicals for these purposes. If subjected to biological processing, CO can become a source of energy, fuels such as H2, and biochemicals (Santoro et al., 2017).
In this literature review, the biological CO transformations in ecosystems were summarized as an inspiration for the biorefinery industry. Specifically, information about CO fate in soil, water, and the atmosphere was collected, and CO impact on plants, animals, and humans was discussed. The review also addresses the need to develop new solutions to implementing circular bioeconomy and highlights the potential of CO use in biologically mediated processes as a valuable resource. Here, for the first time, we aimed to address the questions:
1) Is it possible to treat CO in biologically-mediated biorefinery processes using microorganisms despite its toxic nature?
2) What are the possible pathways of using CO in biologically mediated processes?
3) What are the risks associated with the CO considered for a biorefinery feedstock?
The Fate of CO in the Environment
CO Fate in the Atmosphere
CO is one of the most common air pollutants, and its concentration in contaminated continental air masses reaches up to several ppmv (Badr and Probert, 1994). CO is emitted to the atmosphere through anthropogenic processes, such as biomass combustion, fossil fuels, waste incineration, industrial processes, and transport. Additional contributors are natural sources (oceans, soils, plants, forest fires), atmospheric oxidation of CH4, and other non-CH4 hydrocarbons (NMHC) (Badr and Probert, 1995; Tarr et al., 1995; Schade and Crutzen, 1999; Bruhn et al., 2013). CH4 oxidation has the largest share of these sources, producing approximately 700 Tg-CO·yr−1 (Bergamaschi et al., 2000; Monson and Holland, 2001); fossil fuel combustion together with biofuel use and other industrial emissions are responsible for 500–900 Tg-CO·yr−1, while biomass burning–for 400–800 Tg (Duncan et al., 2007). Photochemical CO production due to the oxidation of naturally emitted and anthropogenic NMHC equals 450 and 110 Tg-CO·yr−1, respectively, (Rozante et al., 2017). In general, global CO levels rose from the Industrial Revolution until 1980; then a gradual decrease in its concentration, especially in the Northern Hemisphere, was observed in measurements from the global surface network from the National Oceanic and Atmospheric Administration (NOAA), caused by both the use of catalytic converters in cars and technological advances in combustion since 2000 (Bakwin et al., 1994; Voiland, 2015; Gaubert et al., 2017). More recently, the downward trends in CO observed for both the Northern and Southern Hemispheres have shown good consistency with long-term trends in bottom-up emissions in Europe, the United States, and China; where an improvement in combustion efficiency and a reduction in emissions from anthropogenic sources was observed (Gaubert et al., 2017). It is worth noting, however, that despite its main global sources, atmospheric CO levels show spatial as well as seasonal variability. While the oxidation of CH4, a gas that is evenly distributed around the world, provides a similarly constant CO background of around 25 ppb, the remaining emission groups depend on space-time aspects. Thus, inter alia, CO from fossil fuels shows higher levels in the northern mid-latitudes, mainly in winter, while biomass combustion in tropical continents contributes to higher CO concentrations in the summer, during the dry season, along with the rainforests (Andreae et al., 2012). Additionally, the spatial variation in CO concentration is also characteristic on a smaller scale, e.g., in urban areas, where it depends not only on meteorological conditions or thermal inversion but also directly on atmospheric turbulence and traffic intensity (Oliveira et al., 2003).
Nevertheless, the determination of the atmospheric CO concentration is still a challenge; despite the variety of analytical techniques available, there is still remaining uncertainty. Therefore, in addition to the most commonly used methods, such as gas chromatographic combined with a mercuric oxide (HgO) reduction detection or a flame ionization detector (FID), non-dispersive infrared absorption (NDIR), vacuum ultra-violet resonance fluorescence (VURF), and tuneable diode lasers spectroscopy (TDLS), comparative studies were also performed (Ou-Yang et al., 2009; Zellweger et al., 2012). In addition to the analytical methods of CO measurement, further possibilities were gradually added, such as the use of closed path Fourier Transform Infrared (FTIR) absorption, cavity-enhanced off-axis integrated cavity output spectroscopy (ICOS), or multi-path quantum cascade laser (QCL) absorption in the mid-infrared range, and cavity ring-down spectroscopy (CRDS) in the near-infrared range (Zellweger et al., 2012).
CO is mainly utilized by the tropospheric reaction with the OH hydroxyl radical (Logan et al., 1981; Khalil and Rasmussen, 1984; Badr and Probert, 1995). CO is oxidized to CO2 in the stratosphere, where it migrates via convection, turbulence, and mixing (Seiler and Warneck, 1972; Seiler, 1974). The reaction is fast and independent of temperature; thanks to it, the residence time of CO in the atmosphere is relatively short, from 2 weeks to 3 months (Rozante et al., 2017; Rakitin et al., 2021). Soils and oceans are also involved in CO capture (Ingersoll et al., 1974; Conrad et al., 1982) and higher plants and algae (Krall and Tolbert, 1957; Chappelle, 1962).
Taking into account the characteristics of greenhouse gases, CO is not considered one of them as it is not capable of absorbing infrared radiation (Rozante et al., 2017). However, because of the primary mechanism for removing atmospheric CO by reaction with the OH radical, CO is recognized as an essential trace gas that controls the oxidative ability of the atmosphere (Bruhn et al., 2013). An increase in CO concentration in the troposphere causes changes in the distribution and amount of OH; reactions of the radical with CO and CH4 constitute about 97% of its destruction (Logan et al., 1981; Levine et al., 1985; Badr and Probert, 1995). The change in the atmospheric OH affects the concentration of other gases, including CH4 and O3 (Hameed et al., 1980). Thus, CO indirectly affects the energy budget of the atmosphere (Evans and Puckrin, 1995), increasing the concentration of GHGs and the time of their utilization in the troposphere, as well as controlling the transfer to the stratosphere, which in turn has an impact on stratospheric O3 (Ramanathan et al., 1985; Thompson, 1992; Bruhn et al., 2013). The radiative forcing from CO is estimated more than from N2O and halogenated hydrocarbons (Rakitin et al., 2021).
CO Fate in Soil
CO is constantly supplied to the atmosphere, which results from both natural and anthropogenic sources. Despite the significant amount of gas emitted, its concentration in the atmosphere does not seem to increase (Bartholomew and Alexander, 1979). It’s because natural processes are responsible for utilizing CO shortly after its release (Inman et al., 1971).
Soil is considered one of the main sinks of atmospheric CO, responsible for 40% of total consumption (Seiler, 1978). However, soils can also be a CO source in the global CO cycle, as noted, especially in the savannas and deserts (Conrad and Seiler, 1985a; Kuhlbusch et al., 1998). For this reason, CO uptake by soils is a net flux consisting simultaneously of consumption and production (Figure 1) (Seiler, 1978; King and Crosby, 2002; Bruhn et al., 2013; van Asperen et al., 2015; Pihlatie et al., 2016). Simultaneous chemical, physical and microbiological processes (Kuhlbusch et al., 1998) depend on many climatic, biological, and physical soil factors, making the equilibrium CO vary between a few ppbv up to hundreds of ppbv (parts per billion by volume) (Conrad and Seiler, 1979; Conrad and Seiler, 1980b; Conrad and Seiler, 1982a). The most important soil parameters include water content, temperature, organic matter content, pH, soil type, the depth of CO consumption horizon, and CO concentration in the gas phase (Potter et al., 1996). Even small changes in this balancing between CO production and soil uptake can severely impact tropospheric chemistry (Moxley and Smith, 1998b).
Soils have been analyzed for the production and consumption of CO for over 40 years. The soil processes were considered for most CO budget analyses in the atmosphere (Crutzen and Gidel, 1983; Conrad, 1996). Soil CO absorption studies were conducted in static and dynamic chamber systems to investigate gross and net exchanges or both (Ingersoll et al., 1974; Conrad and Seiler, 1985b; Sanhueza et al., 1994a). CO consumption was defined as a first-order process, while CO production is a zero-order process for CO (Conrad and Seiler, 1982a; Duggin and Cataldo, 1985; King, 1999b). Gross CO exchanges were determined mainly based on 14CO oxidation, while both gross production and consumption were estimated using models and empirical estimates of net uptake rate constants and steady-state headspace CO concentrations (Bartholomew and Alexander, 1981; King, 1999b).
CO uptake by soils is mainly due to the activity of microorganisms (Inman et al., 1971; Conrad and Seiler, 1980b; Sanhueza et al., 1998; Whalen and Reeburgh, 2001). The biological nature of the CO consumption has demonstrated, among others, the fact that CO use activity in autoclaved soils disappears and returns when they are inoculated again with fresh and non-sterile soil (Inman et al., 1971; Ingersoll et al., 1974; Liebl and Seiler, 1976; Seiler, 1978). However, CO uptake may be limited at elevated CO levels due to microbial metabolic limitations [above 1,000 ppbv, (Bartholomew and Alexander, 1979)]. When CO levels reach lower values that are closer to atmospheric levels of this gas (100–200 ppbv), indicators of CO consumption by soils are higher than its production into the atmosphere (Potter et al., 1996).
The process of CO consumption by microorganisms is considered a result of several mechanisms. CO can be metabolized and incorporated into cellular material, catabolicly oxidized to CO2 by autotrophs as a source of energy or carbon, and used as an additional electron donor by heterotrophic microorganisms; the first of these processes appearing to be dominant (Bartholomew and Alexander, 1979). The assumption that CO uptake by soils is based on the metabolism of the microorganisms is confirmed by research using antibiotics that caused immediate inhibition of this process. For this reason, it is assumed that the CO consumption is controlled by the anabolic activity of microorganisms that consume it during growth and not in the case of protein biosynthesis (Conrad and Seiler, 1980b). In this way, microorganisms are responsible for removing CO from 10% to as much as 50% of its global emissions, depending on sources (Heichel, 1973; Liebl and Seiler, 1976; King, 1999a).
CO consumption is correlated with the total organic carbon present in soil (Inman et al., 1971; Ingersoll et al., 1974). It is associated with organic matter as a source of energy and carbon by CO oxidizing microorganisms (Heichel, 1973; Kiessling and Meyer, 1982; Meyer and Schlegel, 1983; Moxley and Smith, 1998a). The opposite tendency was observed by (Conrad and Seiler, 1985b), proving that the rate of CO uptake depends only on soil enzymes and microorganisms that are not limited by the available organic matter. It is also worth emphasizing that the CO exchange between soil and atmosphere is mainly determined by the top 5 cm of soil (King, 1999a); concentration profiles of this gas do not show a significant amount at greater depth, and below 10 cm they are close to zero (Sanhueza et al., 1998). CO consumption and production processes in these few upper centimeters of soil are also most vulnerable to changes in temperature and humidity and other factors such as fires (Kuhlbusch et al., 1998).
Soil CO consumption based on the activity of microorganisms can occur both under aerobic and anaerobic conditions; in the latter case, it occurs at a lower rate (Conrad and Seiler, 1980b). It is assumed that there are anaerobic sites in the soil in which certain groups of microorganisms break down CO without the presence of O2 (Zavarzin and Nozhevnikova, 1977). This is confirmed by observations of CO consumption in anaerobic conditions in fresh soil, which was just directed to incubation in an anaerobic atmosphere. Anaerobic CO consumption then increased by 15%, while aerobic CO consumption decreased by 40% (Conrad and Seiler, 1980b). The conversion of 14CO to CO2 in anaerobic conditions has also been noted (Bartholomew and Alexander, 1979).
CO absorption rates are controlled by environmental factors such as humidity and temperature (Conrad and Seiler, 1985b; Moxley and Smith, 1998b; King, 1999a). Soil absorption of this gas reaches its maximum value under optimal humidity and temperature conditions. However, these processes have not been extensively studied for various soil types (Moxley and Smith, 1998b; Sun et al., 2018). CO consumption was reported under temperature conditions in the range of 20–30°C with an optimum uptake at 30°C (Inman et al., 1971; Heichel, 1973; Ingersoll et al., 1974; Liebl and Seiler, 1976; King, 1999a). The temperature reactions of the CO flux between soil and atmosphere are complex and include the consumption of this gas and its production by an abiotic path (Conrad and Seiler, 1985b). According to (King, 1999a), CO formation processes become more and more important at temperatures above 30°C and become dominant when the temperature exceeds 35°C. Data reported by (Scharffe et al., 1990) agree with this observation, according to which 30°C is the temperature limit at which the ability to consume CO is exceeded. In addition (Inman et al., 1971) found that at 40°C, CO was about 30% higher in the presence of light than in the dark. Soil heating above 40°C resulted in CO production (Inman et al., 1971; Ingersoll et al., 1974).
Due to the possibility of lower than optimal humidity of the annual soil moisture range in natural conditions, field observations of the impact of this parameter on CO flux are challenging (Sun et al., 2018). Nevertheless, it has been investigated that wetland soils have low CO uptake (Moxley and Smith, 1998b). This tendency may be caused by limiting O2 diffusion by filling the soil pores with water and hindering gas transport. Reduced water content causes an increase in CO consumption up to an optimum value at soil moisture in the range of 30–60% (King, 1999a). In addition, CO absorption ceases when the soil water freezes or the soil is desiccated (Potter et al., 1996; Moxley and Smith, 1998b). A renewed increase in humidity in dry soils leads to the restoration of CO uptake. However, the hysteresis becomes visible. Water stress contributes to this, resulting from increased water content to optimal values immediately after its low level (King, 1999a).
The combination of the above factors contributes to the seasonality of CO consumption in soils. CO intake shows daily variability as a function of CO concentration changes in the atmosphere and temperature (King, 1999b). For this reason, soil can be seen as a source of CO during the day, while it absorbs it at night (Conrad and Seiler, 1982a). In addition, changing weather conditions also affect soil moisture, which translates into the activity of microorganisms. For this reason, lower CO uptake rates in winter were observed when the microbial activity is relatively small (Conrad and Seiler, 1980b).
CO production in soils is mainly abiotic, although there are also reports of the production of this gas in laboratory conditions by root microorganisms (Conrad and Seiler, 1985b; Zepp et al., 1997; King and Crosby, 2002). The non-biological nature of CO formation is confirmed by the tendency to increase it after autoclaving or other sterilization processes of soil samples, such as the use of heat, ultraviolet light, or fumigation (Smith et al., 1973; Conrad and Seiler, 1980b; Conrad and Seiler, 1982a). CO emission is based on chemical and biochemical reactions leading to loss of organic matter by rotting, lysis of microorganisms, and oxidation of dead microbiological material (Conrad and Seiler, 1980b). Heat treatment of dry soil stimulates the efficiency of CO production, which is caused by the breakdown of complex organic substances into simpler particles–including plant residues (Conrad and Seiler, 1982a).
Photochemical production has an important share, as evidenced by the linear relationship of CO emissions from the intensity of solar radiation and the lack of this gas production in the dark (Seiler et al., 1978; Conrad and Seiler, 1980b; Yonemura et al., 2000; van Asperen et al., 2015). Illumination of mineral soils, litter, and plant tissue with light at < 400 nm wavelength stimulates CO production (Valentine and Zepp, 1993). There are also conflicting reports regarding this parameter; (Conrad and Seiler, 1985c) observed CO production both in light and in the dark, which they explained by moisture rather than the photochemical nature of CO forming processes. The stimulating effect on CO production was, among others, an increase in soil moisture–those saturated with water emitted CO into the atmosphere (Conrad et al., 1988). Higher moisture may involve the activation of soil enzymes and dormant microorganisms, which may multiply and reach other habitats in the soil (Conrad and Seiler, 1985b). In addition, moist organic matter is better for microorganisms compared to a dry substrate for CO production.
Data on the impact of soil pH on the amount of CO stream between soil and atmosphere can be found in the literature. An increase in soil pH causes an increase in this gas stream by stimulating abiological CO production (Conrad and Seiler, 1985a). According to the authors, this abiological CO production in soil follows the Arrhenius equation, and increasing pH causes a decrease in energy and entropy of activation. Studies by (Inman et al., 1971) proved that acidic soils participate in more active CO utilization.
CO formation in soils is also based on the chemical oxidation of humic acids and other phenolic substances in an O2 atmosphere (Miyahara and Takahashi, 1971). One of the most important factors affecting the activation energy of CO production is the physicochemical state of humic acid. Its decrease was noted simultaneously with the increase of dissolved humic acid polymers in soil (Conrad and Seiler, 1985a). The CO production from humic acids is a heat-stimulated reaction (Conrad and Seiler, 1985a). In addition, this reaction was stimulated by the presence of O2, but the addition of chemicals quenching singlet oxygen, superoxide, or hydroxyl radicals did not inhibit it (Conrad and Seiler, 1985a).
The CO flux between soil and atmosphere is also affected by how the soil is cultivated. However, these trends are not clear; some research shows an increase in CO use by arable land compared to non-cultivated soils (Scharffe et al., 1990; Sanhueza et al., 1994b; Sanhueza et al., 1994a). It is explained by the loss of organic matter in the soil and changes in pH and water conditions in cultivated soils (King, 2000). In addition, plowing buries the surface layer of soil and mulch, which reduces CO production in the surface layer and leads to diffusion of CO deep into the soil, where it is consumed by microorganisms (Sanhueza et al., 1994b).
For this reason, forests and native grasslands are seen as areas with lower CO consumption (King, 2000). However, the data obtained in studies of temperate soils underline the minimal impact of agriculture on CO flux. Evidence grows that soil cultivation reduces CO consumption (Moxley and Smith, 1998a; Moxley and Smith, 1998b).
CO Fate in Water
The ocean has been recognized as a source of CO released into the atmosphere since the early 1970s (Swinnerton et al., 1970; Lamontagne et al., 1971). Despite the low ocean share (0.4–9%) among all sources of CO in the atmosphere (Bates et al., 1995), it can constitute up to 50% of the load in the marine boundary layer (Erickson and Taylor, 1992; Stubbins et al., 2006a). The southern hemisphere is particularly important here, in which CO production accounts for almost 60% of the total CO flux from the ocean surface (Erickson, 1989). Surface ocean waters are saturated with CO compared to atmospheric equilibrium, which causes a net flux of this gas at the ocean-atmosphere interface (Linnenbom et al., 1973; Logan et al., 1981; Zuo et al., 1998). The CO emissions to air are controlled mainly by the concentration of this gas in water (Bates et al., 1995). It depends on several factors such as photochemical production, consumption by microorganisms, exchange between air and water, and physical mixing (Figure 2) (Wilson et al., 1970; Conrad et al., 1982; Butler et al., 1987; Jones, 1991). Due to the impact of these factors, CO concentration in waters shows diurnal, seasonal, and regional diversification (Bates et al., 1995).
CO was identified as the second most important product of dissolved organic matter (DOM) photolysis in water bodies (Mopper and Kieber, 2000; Stubbins et al., 2006b). The rate of CO formation is one order of magnitude higher compared to other low molecular weight carbon photoproducts produced under aqueous conditions (Mopper et al., 1991; Zuo and Jones, 1995). It is the photodegradation of the DOM by part of the UV solar radiation that is indicated as the main source of CO from both ocean and sea waters, as well as from the surface of lakes, rivers, wetlands, and coastal waters (Zuo and Jones, 1997; Pos et al., 1998; Zuo et al., 1998; Stubbins et al., 2006a; Blomquist et al., 2012).
The rate of CO production is linearly dependent on the concentration of DOM, mainly derived from the degradation of dead biomass, fragmented organic matter particles, and droppings of living organisms, as well as light absorption and water fluorescence (Zuo and Jones, 1997). Carbonyl compounds and phenols are an important part of the soluble organic matter in waters; they build aqueous fulvic acids, then oxidize to quinones. It is phenols and carbonyls found in the surface layer of water that absorb solar rays, producing CO. Their mechanism as photosensitizers is also a possible pathway, thanks to which solar energy is transferred to other carbonyl compounds (Zuo and Jones, 1997).
Ozone depletion and the resulting increase in UV-B radiation that reaches the earth’s surface have been noted as a factor increasing CO photoproduction in ocean waters (Erickson, 1989; Pos et al., 1998). Additionally, the photodegradation of DOM and thus the associated CO production in waters are affected by dissolved iron. It forms complexes with organic matter (including carbonyls and carboxylates) that are highly photoreactive (Zuo and Hoigne, 1992), which speeds up CO formation. This is confirmed by studies of (Zuo and Jones, 1997), who showed that CO photoproduction increases with increasing Fe (III) concentration.
Due to the dependence of the CO flux on the photolysis processes of soluble organic materials and thus on the light intensity, CO photoproduction in the water surface proceeds according to the daily cycle. Field studies showed maximum CO production in the early afternoon, fall in the evening, and minimum around dawn (Swinnerton et al., 1970; Conrad et al., 1982; Jones, 1991; Bates et al., 1995; Zuo and Jones, 1995; Pos et al., 1998; Xie et al., 2002; Zafiriou et al., 2003; Stubbins et al., 2006a). CO production is also characterized by a time delay relative to light intensity, which draws attention to its mechanism–gas production is initiated by light, and then CO is released within a few hours (Conrad and Seiler, 1980a; Conrad et al., 1982). In addition, the highest daily CO concentration amplitudes were recorded during sunny days, while on rainy days, when the light intensity was lower, the dissolved CO content in water showed lower fluctuations or was constant (Conrad et al., 1982). This demonstrates the short-term residence of CO in the water column of about 3–4 h, strongly coupled with the day-night cycle (Zuo et al., 1998; Tolli and Taylor, 2005).
The correlation between the CO production rate and dissolved organic carbon concentration affects the regional flux. Higher rates of CO formation are observed in coastal places rich in organic matter and other chemical and physical compounds, compared to open oceanic spaces (Lamontagne et al., 1971; Linnenbom et al., 1973; Jones and Amador, 1993; Johnson and Bates, 1996; Zuo et al., 1998). Within the latter, higher CO saturation rates were observed in the waters of the North-West region of Africa and in the equatorial area, which is explained by the activity of equatorial currents mixing surface waters with nutrient-rich waters (Voituriez and Herbland, 1979; Katz et al., 1980; Conrad et al., 1982).
The CO concentration in waters varies with depth. Its gradient can be observed in the euphotic zone, with the highest values at or near the water surface, where the light is not suppressed (Tolli and Taylor, 2005). At greater depths, the CO concentrations decrease, reflecting decreasing light intensity (Seiler, 1978). Below the mixed layer (> 100 m depth), CO concentration is low and constant. The exception is higher concentrations occurring at the water/sediment interface (Swinnerton and Lamontagne, 1974; Johnson and Bates, 1996), as well as small maximum CO concentrations, which are explained by the functioning of microorganisms producing CO very slowly in the dark (Conrad et al., 1982). This indicates a very low net CO exchange between these two zones, which does not significantly affect the daily variability in the surface water layer (Conrad et al., 1982; Mopper et al., 1991; Zuo et al., 1998).
The CO flux from the water surface depends on transfer velocity, which is affected by wind speed and water temperature (Erickson, 1989). The first factor affects the intensity of light–when the wind speed drops to about 2 m s−1 on calm days, the CO concentration increases; the rough surface of the water causes a significant reduction in the amount of CO (Conrad et al., 1982).
Microbiological consumption influences the cyclic daily variability of CO concentration at the water surface (Conrad et al., 1982; Blomquist et al., 2012) and atmospheric exchange (Zuo and Jones, 1995). CO consumption by microorganisms follows the Michaelis-Menten kinetics; the CO oxidation rate increases linearly with increasing CO concentration and depends on the size and type of microbial population (Conrad and Seiler, 1982b; Tolli and Taylor, 2005). However, their species are not known. Chemolitotrophicamonnium oxidizers (Nitrosomonas) and methane oxidizers (methanothrops) have a high affinity for CO in waters, and they are mainly suspected of CO consumption processes (Zuo et al., 1998). The first group includes, for example, Nitrosococcus oceanus and N. europaea, the activity of which is catalyzed by ammonia monooxygenase; on the other hand, in the methanotrophs group, CO oxidation was confirmed in the case of, among others, Methylomonas methanica, Methylococcus capsulatus and Methylomonas albus, and the responsible enzyme was not precisely indicated (Ferenci, 1974; Hubley et al., 1974; Bédard and Knowles, 1989; Jones and Morita, 2011). These microorganisms are capable of oxidizing CO at concentrations <100 nM (Jones et al., 1984).
During the day, sunlight has been shown to significantly inhibit the activity of microorganisms responsible for CO oxidation in the surface water layer (Zuo et al., 1998; Tolli and Taylor, 2005). The opposite situation can be observed at night. The sharp decrease in CO concentration is caused by the lack of CO photoproduction and the resumption of the first-order reaction of gas oxidation by microorganisms. Their activity is no longer inhibited, and the decreasing level of dissolved CO does not cause the supersaturation of enzymes responsible for its consumption (Tolli and Taylor, 2005). The microbiological consumption of CO in situ by converting it to CO2 is the dominant mechanism for the utilization of this gas; it is generally not included in microbial biomass, although there are observations that some autotrophic bacteria may build in CO-C after it has been oxidized. However, they are not necessarily those strains that participated in this process (Tolli and Taylor, 2005).
CO Role for Organisms
CO Impact on Plants
Biosynthesis and photoproduction of CO in plants were observed in the second half of the 20th century (Wilks, 1959; Schade et al., 1999). This compound is formed during oxidative heme catabolism due to the activity of the enzyme heme oxygenase (HO). The result is three products: CO, biliverdin, and free iron Fe2+ (Figure 3). The second one is immediately transformed into bilirubin, while the iron is involved in ferritin induction (Bilban et al., 2008). Among the three isoforms of HO discovered so far, HO-1, HO-2 and HO-3 have been distinguished, the last two of which are characterized by low activity (Maines, 1997).
Additionally, (Muramoto et al., 2002) found AtHO1, plastid heme oxygenase, hemoprotein forming a complex with heme in 1:1 ratio. Their research led to the production of CO by catalyzing the heme to biliverdin IX conversion reaction, which was catalyzed by the AtHO1 mentioned above. In addition to producing CO in plants using HO enzyme-catalyzed reactions, which are the predominant method of producing this compound, researchers also found other ways to form it. (Zilli et al., 2014) observed an increase in CO levels in soybean during the first week after planting, but this effect was not correlated with the increase in HO activity. The authors indicated lipid peroxidation and ureide metabolism as an alternative source of CO in this plant.
Research on the effects of CO on plants covers many aspects of their development, including seed germination. While investigating the influence of gases such as O2, N2, and CO on Setaria faberii, it was observed that the latter might have a twofold influence (Dekker and Hargrove, 2002). Depending on the CO concentration, it stimulated seed germination (it increased from 37 to 56% at 1% CO) or inhibited it (75% CO added resulted in a decrease in germination from 37 to 14%). The authors explain the negative CO effect on seed development by inhibiting the plant’s mitochondrial respiration; in terms of promoting germination, the researchers rejected the idea that this gas acted on a respiratory apparatus, leaning more towards CO interacting with CO, an undefined physiological factor in the seed.
CO has also been found to influence the response of plants to abiotic stress caused by environmental salinity, drought, ultraviolet radiation, and heavy metal pollution (Wang and Liao, 2016). These factors, influencing plant growth, resistance, and yield, can significantly limit their development. One of the most frequently analyzed elements from the above mentioned is salt stress. The researchers conducted experiments on plants such as Triticum aestivum, Oryza sativa, and Cassia obtusifolia, analyzing both their seeds, leaves, and roots (Xu S. et al., 2006; Liu et al., 2007; Xie et al., 2008; Ling et al., 2009; Zhang et al., 2012). Research on the influence of CO on sprouting was conducted by (Liu et al., 2007) by analyzing the reaction of Oryza sativa seeds to the addition of this compound’s donors (hematin and its aqueous solution) under salt stress. CO suppressed the adverse effects of NaCl salinity, mitigating the inhibition of sprouting.
Additionally, CO limited oxidative damage by activating antioxidant enzymes. A similar situation was observed in Cassia obtusifolia seeds and seedlings, where CO ensured cytoprotection, activated anti-oxidant enzymes, and increased osmotic substances (Zhang et al., 2012). In an analysis of the effect of a salted environment on seeds of wheat by (Xu S. et al., 2006), low CO concentrations counteracted lipid peroxidation in germinating seeds by enhancing catalase (CAT), ascorbate peroxidase (APX), superoxide dismutase (SOD), and guaiacol peroxidase (GPOX) activities. Similar to the mitigation of oxidative damage in the seeds of Oryza sativa, CO caused the same effect in the leaves of Triticum aestivum (Huang et al., 2006). When treated with a hematin CO donor, they showed a reversal of chlorophyll breakdown and water loss previously experienced due to the treatment of wheat tissues with NaCl solution. Moreover, the authors noted an increase in enzymes, as reported by (Xu S. et al., 2006).
Additionally, the mechanism of mitigating the inhibition of germination of its seeds under simulated osmotic stress conditions was analyzed by (Liu et al., 2010). The beneficial CO effect based on the endogenous HO/CO signal, possibly integrated with NO, was proven. Triticum aestivum was also analyzed for the effects of salt stress on the roots. (Xie et al., 2008) and (Ling et al., 2009) found that CO was involved in maintaining ion homeostasis and reduced superoxide anion production.
The ability to mitigate its response to environmental pollution with heavy metals such as Hg, Cd, Cu, or Fe is an important aspect of CO activity in the context of increasing plant resistance to stress conditions. These metals, which pose a threat to plants and the organisms consuming them, could be mitigated thanks to both NO and CO (Wang and Liao, 2016). The experiments conducted by (Han et al., 2007) involving Medicago sativa L. plant exposed to mercury (in the form of HgCl2) showed that the increase of lipid peroxidation and limitation of root growth could be inhibited by water solution of CO or hematin. Researchers noted the HO-1 transcript induction cells in Alfalfa after 12–24 h of increased activity of the enzymes glutathione reductase (GR), monodehydroascorbate reductase (MDHAR), and SOD. Plant exposure to Hg in Brassica juncea was also carried out by (Meng et al., 2011), who reported the activation of CAT and APX in addition to the SOD. Additionally, it was proven that reducing the toxic effect of Hg due to the CO activity is also based on proline and reduced non-protein thiols.
The addition of exogenous CO improves the accumulation of chlorophyll, contributing to the prevention of chlorosis as reported by (Kong et al., 2010), investigating the regulation of iron homeostasis by CO in Arabidopsis. Moreover, the CO effect on the plant contributed to the accumulation of NO in the root tips. These results prove not only the beneficial effect of CO on plant adaptation in a Fe-deficient environment but also cross-communication with NO. The role of CO in signaling oxidative damage was also confirmed in the environment with an increased concentration of Cd in Medicago sativa roots (Han et al., 2008). Among the stressors for plant function, researchers also analyzed UV-B radiation, which leads to the formation of reactive oxygen species (ROS). An increase in HO-1 protein expression was noted in the Glycine max L. leaves subjected to irradiation (Yannarelli et al., 2006), demonstrating the mechanism of plant protection tissues against oxidative damage.
The interaction between CO and other signaling molecules, such as NO, phytohormones, or H2S, aroused the broad interest of researchers (Wang and Liao, 2016). It was found that phytohormones can induce various distinct developmental CO-dependent responses in plants. Research by (Guo et al., 2008) on lateral tomato roots (LR) formation showed that CO is partly involved in this process. The researchers, analyzing haem oxygenase-1 (LeHO-1), the source of intracellular CO in tomatoes, found that an increase in proteins and transcripts of LeHO-1 caused a simultaneous increase in LR. This effect was shown for tomato mutants with loss of LeHO-1 function, during which the development of impaired LR was observed. Treatment with CO helped restore LR’s normal development. Further analyses of the CO role in LR development were based on auxin (indole-3-acetic acid, IAA) and the NO mediator (Correa et al., 2004). The tests showed that the influence on the tissues of tomatoes with CO increased the level of IAA in them; however, intracellular NO generation induced by CO was observed in the roots of this plant. Moreover, the activity of CO was inhibited by N-1-naphthylphthalamic acid and carboxy PTIO [cPTIO, [[2-(4-carboxylatophenyl)-4,4,5,5-tetramethyl-2-imidazoline 3-oxide]-1-yloxy] radical], which are known inhibitors of auxin and NO (Fricker, 1999). These observations were also supported by (Cao Z.-Y. et al., 2007; Xuan et al., 2008). The use of naphthylphthalamic acid by the former reduced the HO activity, the CO content was lower, and auxin-mediated induction of cucumber rooting (Cucumis sativus) was inhibited. This effect was mitigated by adding IAA, CO water solution, and hematin (HO-1 activator and CO donor), leading to a reduction in root growth inhibition. Greater CO levels were observed due to the use of IAA and/or hematin and an increase in HO activity (or HO-1 expression). The effect of Zn protoporphyrin IX (ZnPPIX), an inhibitor of HO-1, was also tested (Liu et al., 2007) to determine its inhibitory effect on IAA and hematin. The use of CO aqueous solution did not significantly inhibit accidental rooting of the cucumber. Moreover, the aqueous CO solution increased the endogenous CO content. A similar effect was achieved by (Cao Z. et al., 2007). During their experiments, exogenous CO, dependent on NO, increased the number and length of six lateral roots of Brassica napus L. Yangyou. Analyzes of the influence of CO on plant root growth also concerned the development of root hair and root tip segments [Solanum lycopersicum and Triticum aestivum, respectively, (Xuan et al., 2007; Guo et al., 2009)], as well as adventitious root development [Cucumis sativus and Phaseolus radiates, (Xu J. et al., 2006; Xuan et al., 2008; Xuan et al., 2012; Lin et al., 2014; Cui et al., 2015)]. It has been proven that CO can regulate the expression of target genes CSDNAJ-1 and CSCDPK1/5 (Xuan et al., 2008), restore the ability to develop them after the use of inhibitors during treatment in CH4-rich water (Cui et al., 2015), and take part in with the growth of cucumber adventitious roots in hydrogen-rich water (Lin et al., 2014).
The relationship between the activity of CO, NO, HO, cPTIO, and ZnPP were also investigated by (Cao Z. et al., 2007). Their research tested the HO response to abscisic acid (ABA) in the context of stomatal closure in Vicia faba leaves. Their research proved that the addition of ZnPP or Hb (CO/NO scavenger) blocked this ABA-induced process, while, as in the case of previous authors, the application of hematin or CO aqueous solution increased the CO-generated and stimulated stomatal closure. The authors also declared that the CO generated by HO activity is involved in the stomata closure process and that NO and cyclic guanosine monophosphate (cGMP) act as downstream intermediates. In addition, HO-1/CO was analyzed in the context of programmed cell death (Xie et al., 2014). Observations of ZnPP interactions were performed to test their role during (H2S)-induced cytoprotection. The authors observed that the addition of CO aqueous solution or bilirubin mitigated the negative effect of this HO-1 inhibitor on NaHS responses.
CO Impact on Animals
CO is considered an important endogenous signaling gas with similar properties to NO, produced in their organisms through heme degradation (Dulak and Józkowicz, 2003; Verma et al., 1993). Some of the CO can be generated in vivo by non-enzymatic haem metabolism by hydrogen peroxide or ascorbic acid. CO is then produced by breaking methylene bridges (Dulak and Józkowicz, 2003). The similarity to NO is due, inter alia, to the ability of both of these compounds to bind the iron atom derived from the heme moiety, which is linked to the soluble guanylate cyclase. This binding activates this enzyme and thus stimulates the production of intracellular cGMP (Cao Z. et al., 2007). The relationship between CO and NO in animal organisms has been confirmed in the studies of bovine pulmonary artery endothelial cells, which proved that 11–110 nmol/L of CO in them increases the concentration of released NO (Thom et al., 1997).
Research on the influence of CO on animals was indirectly initiated at the beginning of the 20th century by using them as detectors of vitiated air in mines (Burrell and Seibert, 1914). The analyses were conducted on commonly available animals, such as chickens, dogs, mice, pigeons, rabbits, canaries, and guinea pigs. One of the first reports on the experiments taking place in 1914 in the United Kingdom proved that the most sensitive to the effects of CO were mice and canaries, which meant that they were repeatedly used in rescue operations in mines as organisms experiencing stress caused by CO faster than humans (Burrell and Seibert, 1914).
Most of the studies concerned the effect of high concentrations of CO on animals. Only a few sources reported the effects of prolonged or repeated exposure to CO (Jones et al., 1971), and the results were often contradictory (Preziosi et al., 1970). Analyzes conducted in the mid-20th century showed that 11-weeks exposure of dogs for 6 days a week for 5.5 h to 100 ppm CO caused brain and heart muscle damage (Lewey and Drabkin, 1944). In the latter case, CO poisoning in these animals led to many pathological changes, such as muscle fibers degenerative changes, necrosis, and hemorrhage in the ventricle, associated with a COHb level of 75% (Ehrich et al., 1944). Myocardial changes have also been reported in other animals, such as rabbits, that showed necrosis after 30–60 min exposure to 3,000 ppm CO (Takahashi, 1961) or, inter alia, myofibrillar disintegration after exposure to 100 ppm CO for 4 h (Hugod, 1981). (Preziosi et al., 1970) reported the mortality of the studied dogs of 31%; the remaining surviving animals showed extensive central nervous system and myocardial fiber degeneration pathologies, similar to hypoxia-induced lesions. The authors also noted that the most severe changes occurred in animals exposed to CO for 60 min or more but were less severe than those exposed to short-term exposure at high gas concentrations. In addition, CO can reduce vascular resistance in the coronary vessels of animals, leading to too high blood flow, which has been studied in dogs (Einzig et al., 1980; Kleinert et al., 1980), and it can also reduce myocardial contractility [seen in both dogs and goats, (Erickson and Buckhold, 1972; James et al., 1979)]. Experiments on the exposure of monkeys and dogs to CO also proved that this gas reduces the required threshold leading to ventricular fibrillation and arrhythmia (DeBias et al., 1976; Vanoli et al., 1989). On the other hand, it was later proven that the produced CO dilates blood vessels and prevents platelet aggregation, which maintains tissue microcirculation at an appropriate level (Katori et al., 2002).
CO has also been identified as affecting the respiratory system of animals. Researchers found many changes, including capillary and alveolar epithelial endothelial cell edema in the lungs of rats exposed to 0.5–1% CO (Niden and Schulz, 1965). A higher concentration of 95% led to a 56% decrease in ATP (Bassett and Fisher, 1976). In rabbits, CO exposure resulted in endothelial and epithelial swelling, and additionally, the endothelium was detached from the basement membrane (Fein et al., 1980). A similar effect was also observed in rats (Smialek et al., 1973). Changes caused by CO led to a decrease in dynamic lung compliance and increased resistance in the respiratory tract of animals (Fein et al., 1980). Results opposite to those presented above were obtained by (Musselman et al., 1960). 3-months long exposure of rats, rabbits, and dogs to 50 ppm CO did not cause any observed side effects in these animals. The absence of toxic effects of long-term exposure of rats, dogs, monkeys, and guinea pigs to three different concentrations of CO (51–200 ppm) was also observed by (Jones et al., 1971). No changes in lung tissues were observed in the analyzed dogs, despite being exposed to a 20–30 times higher CO dose than the lethal dose in humans (8–14%) (Fisher et al., 1969). A similar lack of toxic effects in the same animals was observed by (Halebian et al., 1984).
CO also has metabolic effects on animal organisms. It was reported that it changes blood glucose levels in rats (Smith and Penrod, 1940), rabbits (Gothert et al., 1972), and dogs (Schrenk et al., 1932). The hyperglycemia due to CO poisoning was explained by the animals’ livers’ decreased ability to produce and store glycogen. It is also influenced by an increased adrenaline level in the central nervous system and not, as initially thought, disruption of insulin production (von Oettingen, 1944). Additionally, after injection of CO under the skin of rats, catecholamine excretion in the urine significantly increased (400–600%) a few hours after the procedure (Pankow and Ponsold, 1978).
CO can dissolve in tissue fluids, which has been observed in rabbits, guinea pigs (Göthert et al., 1970), rats (Savolainen et al., 1980), and dogs (Coburn et al., 1971) exposed to CO followed by measuring carboxymyoglobin (COMb) levels (Sokal et al., 1984). The COMb concentration after CO exposure is lower than the COHb level in the blood and skeletal muscles. In addition, long-term exposure does not cause CO accumulation in the latter and the extravascular heart compartment (Sokal et al., 1984). Importantly, however, exposure of animal organisms to CO in combination with their workload increases the risk of tissue hypoxia due to the increased tissue level of CO, as shown in studies on rats (Sokal et al., 1986). Additionally, exposure to CO causes changes in specific enzymes in animal organisms, such as plasma leucine aminopeptidase (Katsumata et al., 1980), lactate dehydrogenase, creatine phosphokinase (Penney and Maziarka, 1976), cardiac cytochrome oxidase (Fukui et al., 1987) and cerebral cytochrome oxidase (Savolainen et al., 1980). In the first three enzyme cases, CO increased their activity, and the plasma leucine aminopeptidase level tested in rats exposed to CO depended on the duration of absorption (Katsumata et al., 1980). Enzymes susceptible to CO activity reached a high level of activity, reaching 117 and 132% above controls (lactate dehydrogenase and creatine phosphokinase, respectively, (Penney and Maziarka, 1976).
Behavioral studies of the effects of CO on animals were carried out as early as the second half of the 20th century. These analyses covered a broad spectrum of behaviors but were mainly based on rats, mice, monkeys, and pigeons (Laties and Merigan, 1979). For the first two groups, it was reported that CO interfered with several activities such as food and water intake and activities such as running, swimming, or digging. The eating disorder was associated with exposure of rodents to a CO concentration from 50 ppm for 120 h per week (Stupfel and Bouley, 1970), through 250 ppm per day (Koob et al., 1974; Annau, 1975) to 400–500 ppm (Theodore et al., 1971) and in each case, it was correlated with a reduction in weight gain. The running performance of rats showed a decrease in both long-term exposure of rodents to CO (200 ppm per day) and acute but high gas concentration (700 ppm for 30 min), while in the second case, it was lower (Plevova and Frantik, 1974). CO also limited the mice’s ability to move; in the final stage of the 17-h treatment with CO, the distance covered was shortened from 33% (55 ppm), 50% (84 ppm) to 75% (160 ppm) (Malorny, 1972). A similar inhibitory effect on the activity of animals was observed during rats swimming (over 50% decrease in swimming ability after 1-h and 7-h exposure to CO concentrations of 300 and 100 ppm, respectively), and hamster’s digging [decrease by 75% after exposure to 50 ppm CO, (Malorny, 1972)]. The behavior of the animals’ conditioned reflexes was also analyzed. After the rats were exposed to CO, researchers observed a significant decrease in their responses, including pressing on a lever to obtain food [exposure to 500 ppm CO (Teichner, 1967)]. The animals were also negatively affected with a gas concentration of 250, 500, and 10,000 ppm (Goldberg and Chappell, 1967; Annau, 1975). Analyzes of this type were also carried out on monkeys, which showed reduced responsiveness after being exposed to 383 ppm CO for 1 week (Back and Dominguez, 1968; Theodore et al., 1971).
Accidental animal poisoning with CO is rarely described in the literature. However, due to their cohabitation with people, pets such as cats and dogs are also exposed to elevated CO concentrations due to poor ventilation, faulty heating installations, or fires (Sobhakumari et al., 2018). Few reports are addressing the toxicity threshold of CO for pets, and no evidence of pathology and histological changes induced by CO have been reported (Berent et al., 2005). The analysis showed that after 6–8 h of exposure of cats and dogs to exhaust gases containing CO, the animals were confused, inclined to a lying position, showed stiffness, and suffered from dyspnea (Berent et al., 2005). On the other hand, cats found dead after a fire in their habitats were characterized by visible signs of poisoning, such as irregular red skin spots on the abdominal surface and the auricle, as well as a bright color of blood and muscles (Sobhakumari et al., 2018). Smoke inhalation also caused epileptic seizures in dogs and, as a consequence, their death (Kent et al., 2010).
Additionally, the literature reported human-analogous delayed neurological symptoms in dogs after exposure to smoke (2–6 days after the animals initially improved). Of the dogs tested, 60% either died or were euthanized because of their injuries (Jackson and Drobatz, 2002). Slightly different results were obtained by (Mariani, 2003). The initial acute condition of the Australian shepherd found during the fire showed that dementia and pneumonia were alleviated during 7 days of treatment.
In addition to inducing toxic effects in animal organisms, CO has functional properties. It has been indicated as a potent inhibitor of cell apoptosis (Katori et al., 2002; Liu et al., 2003), which, by activating the cGMP signaling pathway, alleviates vascular disorders, including lesions caused by arterial damage (Liu et al., 2003). In addition to preventing programmed cell death, CO can regulate inflammation (Otterbein, 2002). In mice, this immunoregulatory role of CO was confirmed during the analysis of sensitized to ovalbumin (Chapman et al., 2001), while in miniature swine in ischemia-reperfusion injury lungs (Sahara et al., 2010). By modulating cytokine production and cell proliferation, CO creates protection against stressors and increases organ transplants’ effectiveness in rodents (Nakao et al., 2006). Additionally, it was found that endogenous CO influences contractile responses by regulating vascular tone (Wang et al., 1997). However, the above effects induced by CO require an appropriate and significantly reduced concentration of this compound.
CO Impact on Humans
CO is known to be toxic because of its ability to interfere with oxygen delivery at high concentrations. CO is inhaled from the lungs into the bloodstream. Since the affinity of CO for hemoglobin (Hb) is 230- to 270-times greater than that of oxygen, COHb is formed in erythrocytes (Eq. 1):
The formation of COHb in the blood depends on various factors, including the concentration of inspired CO, duration of exposure, pulmonary ventilation, exercise, and health status (Kinoshita et al., 2020). Tissue hypoxia is the main toxic effect of acute CO poisoning due to the formation of COHb. It causes decreases the oxygen transport capacity, resulting in insufficient oxygenation at the tissue level. Typical symptoms of CO poisoning are headache, dizziness, weakness, vomiting, chest pain, and confusion was often mistaken for flu. Prolonged exposure causes brain damage and death (Adach et al., 2020) (Table 1).
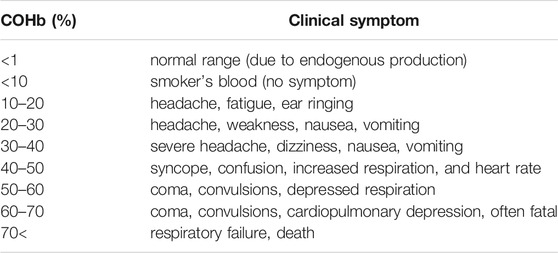
TABLE 1. Levels of carboxyhemoglobin (COHb) saturation (%) and symptoms (Kinoshita et al., 2020).
In urban areas, high concentrations of inhaled CO can have a negative impact on human health. CO is often formed due to the incomplete combustion of carbon-containing compounds, primarily in internal combustion engines. Studies by Mandal et al., 2011 show that between 6 and 20% of homes in London have CO levels above World Health Organization (WHO) upper limits (Table 2) (Mandal et al., 2011). The worldwide incidence of CO poisoning has remained stable during the last 25 years (Kinoshita et al., 2020). Moreover, results from China show that a 1-mg m−3 increase of CO concentrations was associated with ∼2–3% increments in daily years of life lost (YLL) from non-accidental causes, cardiovascular diseases, respiratory diseases, coronary heart disease, stroke, and chronic obstructive pulmonary disease, respectively, (Wang et al., 2021). Associations were more robust in the elderly (≥65 years), females, populations with low education attainment, and those living in southern regions. The role of expired-air CO as an independent marker of 16-y all-cause, cardiovascular, and cancer mortality in a substantial sample of apparently healthy subjects after adjustment for smoking was also evaluated (Bérard et al., 2015). Results provided a better understanding of the potential CO role on mortality and developing new prognostic and therapeutic tools for prevention.
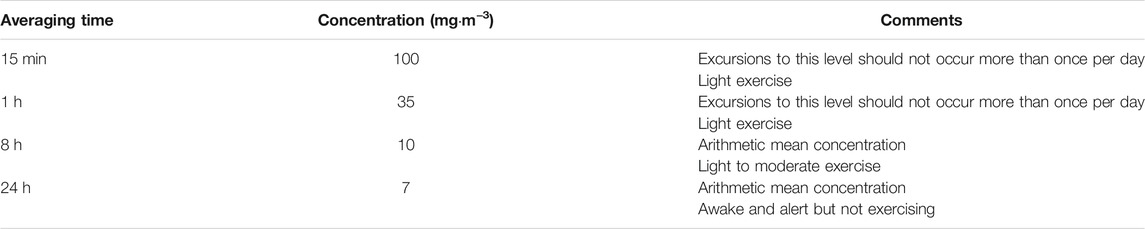
TABLE 2. Indoor carbon monoxide guidelines World Health Organization (WHO) (World Health Organization, 2010).
Additionally, the newest studies show a strong correlation between the concentration of air pollutants such as CO and the number of SARS-CoV-2 cases. A report by (Meo et al., 2021) shows that the PM2.5 concentration increased by 221%, O3 by 20%, and CO concentration increased by 151% after the California wildfire. This was compared with the number of cases, and deaths due to COVID-19 increased by 56.9 and 148.2%. An increase in ambient concentrations of toxic pollutants, which were temporally associated with an increase in the incidence and mortality of COVID-19, could also depend on other reasons, including temperature, humidity, changes in societal patterns of social distancing, and mass gatherings or adherence to wearing masks. Nevertheless, researchers obtain similar results from London (Ayoub Meo et al., 2021). A one AQI unit in the increase in CO level significantly increased the number of cases and deaths by 21.3 and 21.8%, respectively. Air pollutants, such as PM2.5, CO, and O3, are positively associated with increased SARS-CoV-2 cases and daily deaths in London, United Kingdom, but the CO influences the most on new cases.
Despite the toxic effects on the nervous system and the cardiovascular system, CO also plays an essential role in the proper functioning of the human body, where it is also produced endogenously. Like plants and animals, the primary source of endogenous CO in the human body is heme degradation, catalyzed by HO (Olas, 2014). This enzyme breaks up porphyrin in the presence of NADP and molecular oxygen, resulting in the formation of various so-called primary degradation products (HDP): CO, ferrous cations (Fe2+), and biliverdin (Figure 4). The next stage in decay is catalyzed by biliverdin reductase, which begins the reaction of biliverdin to the 2nd-degree metabolite bilirubin in the presence of NADPH and H+. This bilirubin is then secreted into the bile and excreted in the urine (Adach et al., 2020).
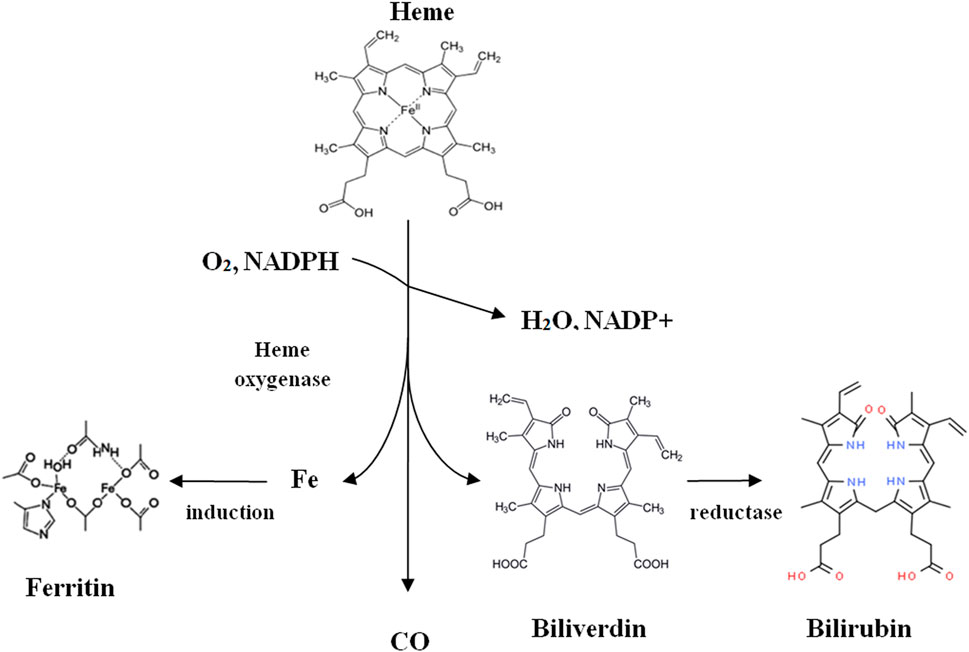
FIGURE 4. Heme catabolism (Adach et al., 2020).
Recent findings have indicated that heme oxygenases and generation of CO serve as a critical mechanism to maintain the integrity of the physiological function of organs and supported the development of a new paradigm that CO, at low concentrations, functions as a signaling molecule in the body and exerts significant cytoprotection (Adach et al., 2020). Gaseous modulators, such as CO, NO, and H2S, are important physiological mediators in the body. CO has antiapoptotic, signaling, and anti-inflammatory effects; hence, pharmacological agents that can imitate its action may yield therapeutic benefits. Pharmacists and biochemists have extensively studied such practical applications for many years (Kramkowski et al., 2012). Significant amounts of preclinical data indicate that exogenously provided CO can ameliorate I/R injury associated with organ transplantation. A study examining the safety and tolerability of inhaled CO in kidney transplant patients was reported by (Ozaki et al., 2012). However, the basic problem with administering CO is that it exists in gaseous form at room temperature. Although inhalation would commonly result in toxic effects, devices have been invented that strictly control the concentration of inhaled CO and automate the level of its release. The need to avoid the toxic effects of CO administration is not the only complicating aspect of inhalation therapy (Adach et al., 2020).
CO has also held great promise in cancer and other treatments due to its multifaceted regulation of cellular function and the tumor microenvironment. These days, it is growing at a somewhat accelerated pace, including the fast progress in a wide range of intelligent CO donors and CO delivery-related nanoplatforms (Zhou et al., 2020).
Biorefinery Application
The natural processes of CO transformation taking place with the participation of microorganisms in media (e.g., soil or water) inspired researchers to try to restore them under controlled conditions. In this way, biologically-mediated processes compete with inorganic ones. For example, the growing demand for H2 in the 20th and 21st centuries began to be increasingly satisfied by the water-gas shift reaction (WGS) (Reddy and Smirniotis, 2015). This exothermic reaction, taking place according to Equation 2, is carried out at high temperatures and a pressure of 1.0–1.6 MPa (Cui et al., 2019):
Gradually, however, the possibility and advantages of using anaerobic bacteria enabling CO metabolization began to be noticed, indicating the economic and ecological effectiveness of the biologically-mediated WGS (BMWGS). Studies have shown that CO is oxidized to CO2 by a broad range of aerobic and anaerobic microorganisms from various physiological and taxonomic groups, treating CO as an energy or carbon source necessary for growth (Table 3). The majority of those microorganisms use The Wood-Ljungdahl (acetyl-CoA) pathway both in the metabolism and production of CO. The Wood–Ljungdahl pathway is the central metabolism for acetogenic growth, mainly in different types of fermentation by mesophilic and thermophilic bacteria and thermophilic Archaea (Diender et al., 2015).
Thus, the use of bacteria in the BMWGS reaction fits in with the goals of the circular economy by using biomass and bio-waste as raw materials for the production of fuels and organic compounds (Henstra et al., 2007). These raw materials are also available in developing countries where the bioeconomy can be advantageous. Process conditions such as ambient temperature and pressure also influence the biological competitiveness of a method (Alfano and Cavazza, 2018). The advantage of BMWGS is the natural regeneration of microorganisms, which, compared to inorganic catalysts, can quickly replicate their cells to avoid poisoning (Henstra et al., 2007). Notably, from the point of view of industrial use of WGS, this reaction occurs in the dark so that it can be carried out in closed reactors. Thus, for this reason, process costs are minimized, including the elimination of the need for photo-bioreactors (Amos, 2004).
Another beneficial method of CO use with the participation of microorganisms is the Fischer-Tropsch (FT) reaction, taking place at a temperature of 150–300°C and using syngas as a substrate converted catalytically (Maitlis and Klerk, 2013; Selvatico et al., 2016). The primary purpose of FT is to make liquid hydrocarbons by converting CO and H2 mixture (Alfano and Cavazza, 2018). In this reaction, both waste and biomass can be carbon sources (Maitlis and Klerk, 2013), and depending on the material used, the required H2/CO ratio changes (Selvatico et al., 2016). Initially, this reaction, especially in the commercial sector from 1936, used mainly conventional non-renewable substrates such as coal or natural gas to ensure economic viability (Köpke and Simpson, 2020). An example of a mass-scale operation is The Shell Pearl Gas-to-Liquids installation located in Qatar, which since 2012 uses a natural gas well to produce up to 140,000 barrels per day (Shell, 2022). However, due to environmental reasons, based on the concern to reduce carbon emissions, the spectrum of materials used in the FT process has been expanded to various groups of waste, including agricultural, organic industrial, or municipal solid waste; the use of the latter is implemented at the Fulcrum Bioenergy plant, whose production capacity is <1,000 barrels per day (Fackler et al., 2021).
One of the newest technologies aimed at producing sustainable fuels and chemicals using the abundant above-ground carbon feedstocks is gas fermentation. The metabolism of chemolithoautotrophic microorganisms enables the utilization of carbon oxides, including CO (Fast and Papoutsakis, 2012; Claassens et al., 2019). Thanks to this, gas fermentation offers a wide range of potential substrates, including 1) industrial waste gas, e.g., from steel and ferroalloy production, refinery processes, 2) syngas formed from organic waste, biomass residues, and municipal solid waste, as well as 3) CO2 from various processing plants, processes of ethanol production from corn biomass or direct air capture (Köpke and Simpson, 2020). Such a wide variety of input materials, however, makes them compositionally variable, and additionally, the syngas used in the process is characterized by a high level of impurities. They include, among others, heavy metals, nitric oxides, NH3, aromatic compounds, and sulfur compounds (Infantes et al., 2020). While FT processes encounter technical and hence economic problems resulting from the need to provide a purified feedstock to produce high-purity gases, gas fermentation shows high process tolerance to contaminants (Köpke and Simpson, 2020). As in the case of the BMWGS reaction mentioned above, it results from the ability of the microbial catalyst to self-replicate and the process tolerance is based on the binding of pollutants with microorganisms, which are washed out of the reactor after being killed (Köpke and Simpson, 2020). This is facilitated by the continuous process lasting several weeks or months (Fackler et al., 2021). This situation does not lead to the accumulation of unfavorable substances in the process, unlike in traditional chemical thermocatalytic processes. This has its economic consequences, making the fermentation process possible to reduce capital expenditure by keeping the high selectivity of the products despite the variability of the substrates, e.g., by lowering the required level of raw materials (Clomburg et al., 2017). Moreover, this high selectivity increases the conversion efficiency due to the formation of fewer by-products than is the case with catalyzed chemical processes (Fackler et al., 2021). In addition, the features of the process enable the creation of modern facilities, which, unlike the previously adopted classic industrial model, are able to flexibly operate with various groups of microorganisms using one infrastructure. Thus, depending on the type of microbial strain, the same gas composition can lead to a variety of products (Köpke and Simpson, 2020).
Learning about acetogenesis in 1932, willingness to find the added value of fossil sources, and to ensure energy security, were the driving force behind scientific research on syngas fermentation as early as the 1980s. After groundbreaking events, such as the demonstration of the use of gas fermentation to produce ethanol in 1989 and the isolation of the first acetogens producing various compounds in 1990, gas fermentation was considered ready to be attempted on a larger scale (Köpke and Simpson, 2020; Fackler et al., 2021). The top three organic solvents from syngas identified based on their octane values were methanol, ethanol, and iso-propanol (Fackler et al., 2021). The efforts and knowledge gained from the laboratory studies were then transferred to the Bioengineering Resources, Inc., pilot plant in 2003 (which obtained rights to commercialize the technology in 2008), Coskata company (established in 2006, since 2015 as Synata Bio), and INEOS New Planet BioEnergy commercial venture in 2011 (closed in 2016 due to problems related to with a high content of hydrogen cyanide in syngas). Importantly, in 2005, research on C. autoethanogenum at LanzaTech in New Zealand led to the up-scaling of the technology using a 500-L pilot fermenter (Liew et al., 2016) and, consequently, to the creation of a commercial installation in China in 2018, occupying ethanol production with a capacity of 16 million gallons per year, jet fuel and plastic and nylon precursors (Beijing Shougang LanzaTech New Energy Science & Technology Co., Ltd.) (Fackler et al., 2021). The aforementioned growing interest in using municipal solid waste and agricultural waste in the process is also visible. The first of these groups was processed into ethanol by the Japanese company Sekisui Chemical in cooperation with LanzaTech in 2017, which led to the establishment of SEKISUI Bio-Refinery CO., Ltd., a company that verifies the developed technology, 3 years later. One of the above-mentioned shareholders, LanzaTech, also started a joint operation with Indian associates in the same year to implement the process on agricultural substrates (Fackler et al., 2021). Another alternative and innovative course are the in vitro utilization of enzymes present in the BMWGS reaction. The research undertaken concerns hydrogenases; due to the difficulties in understanding the biogenesis of CODH, this enzyme is not yet considered ready to be used on an industrial scale (Alfano and Cavazza, 2018).
However, the industrial applications of CO and CO-transforming bacteria are still being explored and described, and so far, several problems related to these processes have been identified.
One of the limitations is the toxicity of CO, which, due to its high affinity to metalloenzymes, may inhibit the growth and catabolic activity of bacteria (Alfano and Cavazza, 2018). The concentration of this gas in the liquid phase greater than 0.15 m mol L−1 decreases the reaction rate, which may result in the complete closure of BMWGS (Amos, 2004). Therefore, researchers point to the need for an in-depth understanding of syngas metabolism, which should allow the development of microorganisms resistant to the toxic effects of CO (Ismail et al., 2008). On the other hand, CO availability to the bacteria could be a problem since their activity potential depends on the CO content. It is necessary to optimize the distribution of microorganisms, the CO concentration in the substrate, and the gas-to-liquid mass transfer (Alfano and Cavazza, 2018). The latter is one of the most problematic aspects affecting the operation of the BMWGS on an industrial scale in large reactors. Due to the low solubility of CO, this reaction is characterized by slow, diffusion-limited mass transfer from the bulk gas into the pores of the catalyst; but the rate of mass transfer is much higher than the rate of mass transfer from a gas into a liquid. In effect, for most reactor configurations, microorganisms have to “wait” for a dose of CO in the solution due to the slow mass transfer rate, which significantly reduces the rate of BMWGS (Amos, 2004). Another potential threat is also CO2, the presence of which at higher process pressure leads to the formation of, among others, carbonic acid. In general, biological WGS is highly active when the pH of the process is in the range of 6.8–8. When it is less than 6.5, the microorganisms are lysed; similar inhibition occurs when pH > 8 (Amos, 2004). Higher molecular weight contaminants may also cause concern when the BMWGS reaction is used in syngas conditioning, which may negatively affect microbial cells (Amos, 2004). An important aspect indicated by the researchers is that BMWGS kinetics can only be determined experimentally (Amos, 2004).
Too high a concentration of CO in the process is also a problem in the case of syngas fermentation, affecting its efficiency. This is based on the inhibitory effect of this gas on the activity of the key enzyme, hydrogenase, and hence the H2 utilization rate (Devarapalli and Atiyeh, 2015). Therefore, in industrial applications, it would be necessary to use fast-response sensors, which by measuring CO and H2 concentrations, would be able to accurately determine them online, enabling high yields of ethanol and stability of the process (Dang et al., 2021). However, currently, dedicated sensors are not available on the market; solutions such as membrane-coated electrochemical sensors in the power industry are not suitable for the CO and H2 concentration measurements required in the process due to the slow reaction time and labor-intensive operation (Dang et al., 2021). Additionally, the challenge for this type of device is the ability to measure both compounds simultaneously; the cross selectivity of CO and H2 is currently not developed, which does not allow for the precise differentiation of these gases by one sensor.
As mentioned earlier, it is necessary to consider the appropriate H2/CO ratio in the FT reaction. The most popular substrate for the production of syngas used is wood and straw, and the syngas produced in this way is characterized by a too low substrates ratio for industrial purposes (Sansaniwal et al., 2017). Therefore, the currently preferred direction is to use the BMWGS reaction and control it to obtain a specific and desired H2/CO ratio with the help of microorganisms (Bukur et al., 2016).
What was mentioned before, syngas fermentation has an advantage over FT processes due to, i.a. higher biocatalysts specificity (Henstra et al., 2007; Wainaina et al., 2018). However, the traditional approach to fermentation is associated with a few significant problems, such as redox imbalance and limited growth and effectiveness of CO-converting microorganisms due to the limited reducing power of the Wood-Ljungdahl pathway (Barbosa et al., 2021). For this reason, in 2009, research on bioelectrochemical syngas conversion (bioelectrochemical systems, BESs) in dedicated systems began (Kim and Chang, 2009). BESs technology, based on integrated biological conversion and production of electricity, uses the activity of electrochemically active bacteria (EAB) capable of the use of insoluble electron acceptors or donors‐anodophiles and cathodophiles‐biocatalysts that are applied at the anode and cathode, respectively, (Figure 5) (Barbosa et al., 2021). In these processes, the chemical energy stored in compounds is transformed into bioenergy; both for electricity production thanks to oxidation (anode) and for production of added-value biochemicals thanks to reduction reactions [cathode, (Logan et al., 2008; Lovley, 2011; Choi and Sang, 2016)].
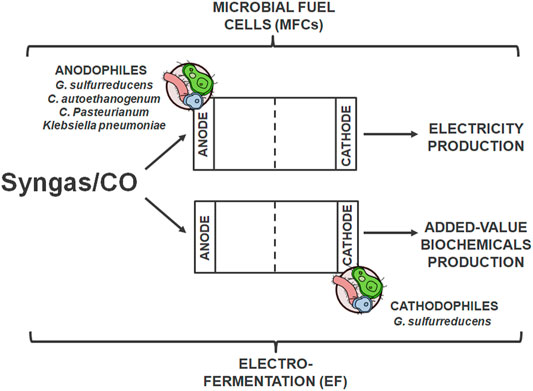
FIGURE 5. Bioelectrochemical syngas conversion in BESs using electrochemically active bacteria (EAB) applied at the anode and cathode to produce bioenergy and biochemicals, respectively.
The first solution, electricity production from syngas/CO using microbial fuel cells (MFCs), is the most popular and tested BESs method (Mehta et al., 2010; Hussain et al., 2011b; Neburchilov et al., 2011; Hussain et al., 2012; Kumar et al., 2017; Santoro et al., 2017). Researchers have proposed three theories about the mechanism of this process. The first is the direct electricity generation by transferring electrons by Fe (III)-reducing carboxydotrophic bacteria to the anode; this process occurs in the one-stage chamber (Mehta et al., 2010). Another assumes that this process is indirectly based on the change of CO to compounds such as H2 or acetate (CO fermentation products, resulting from acetogenic carboxydotrophic microorganisms, such as Alkalibaculum bacchi, C. ljungdahlii, Acetobacterium spp. or C. carboxidivorans). Anodophilic bacteria then convert acetate as a substrate for electricity production in MFCs (e.g., Geobacter sulfurreducens) (Kim and Chang, 2009; Hussain et al., 2011a; Hussain et al., 2014). The third theory allows for the possibility of producing energy from the resulting H2 or converting it and CO2 into acetate (Mehta et al., 2010). Additionally, the influence of temperature on CO in MFCs conversion was analyzed. Greater process efficiency and higher power density were observed in thermophilic conditions (Hussain et al., 2012).
Researchers are increasingly interested in biochemicals production (e.g., CH4, acetate, or H2) in the electrochemically-assisted fermentation process (electro-fermentation, EF) (Rabaey and Rozendal, 2010). This technology is based on the introduction into electric circuits an electrode to provide additional energy and induce the transformation of the substrate into the expected product. Its advantage over conventional fermentation is based on increased production efficiency while reducing costs and increasing product purity (Engel et al., 2019). This is possible thanks to modifying redox balances and fine-tuning metabolic pathways with the need to use special additives (Barbosa et al., 2021).
Analyzes of the use of syngas/CO for the production of biochemicals were initiated in 2010 (Köpke et al., 2010; Nevin et al., 2011), and the process itself is considered future-oriented due to carbon capture and storage in the form of valuable products (Barbosa et al., 2021). Related reactions may become a support for the 4th generation biofuels (Barbosa et al., 2021). Several compounds have been obtained in EF processes using syngas/CO, including, e.g., 1,3-propanediol [G. sulfurreducens, (Moscoviz et al., 2018)], 3-hydroxypropionic acid (3-HP) [Klebsiella pneumoniae, (Kim et al., 2017)], lactate and 2,3-butanediol [Clostridium autoethanogenum, (Kracke et al., 2016)], butanol [C. pasteurianum, (Choi et al., 2014)]. Promisingly, the amounts of these compounds exceeded those obtained with conventional fermentation, for example:
• Production of 1,3-propanediol was improved by the yield of 10% [G. sulfurreducens, (Moscoviz et al., 2018)],
• 1.7-fold enhancement of 3-hydroxypropionic acid (3-HP) production was observed by (Kim et al., 2017),
• Production of lactate and 2,3-butanediol increased by 35-fold and 3-fold, respectively, in electrically enhanced fermentation of fructose (Kracke et al., 2016),
• Improvement of butanol production from glucose and 1,3-propanediol production from glycerol by 20 and 21%, respectively, was noted by (Choi et al., 2014).
A wide spectrum of waste that can be used in biorefinery processes, including municipal, industrial, agricultural waste, etc., is a valuable alternative to the use of fossil resources for the production of fuels, chemicals, or electricity. Above mentioned results indicate the need to develop described technologies and to scale up for industrial applications in the near future. Introducing them to mainline production can bring benefits on the economic and environmental side, possibly through credit and tax incentives. However, these solutions have limitations that affect the economic aspects of implementing it at a commonly used, commercial level. Streams of this waste are abundant, but their characteristic feature is significant dispersion. In order for them to compete with traditional feedstocks in petrochemical processes, they must be delivered to the plant in an integrated manner in quantities that exceed the current capacity (hundreds or thousands of tons per day compared to hundreds of thousands of tons for fossil raw materials). Increased efficiency and competencies of biorefineries largely depend on the availability of these resources, i.e., on a consistent logistic system for collecting, storing, and transporting waste to processing sites. It is also important for each industrial process to be stable and handle to produce large amounts of a uniform product; process specifications also need to consider the variability in raw material, which is extremely important when the substrate is waste (high possibility of inhomogeneity). What is also important, is that biorefinery technology should provide easy and inexpensive storage and transport of gases like H2; new technology like proton-exchange-membrane (PEM) is still not cost-competitive to the alternative steam-methane-reformation (SMR) process that uses natural gas (Takors et al., 2018). Additionally, the competitiveness of biorefinery plants could be based on the economic benefits of recovering CO2 as a by-product of the processes carried out. For this purpose, it is necessary to analyze the possibilities of implementing various methods, depending on the composition of the substrates, e.g., waste or synthesis gas.
An interesting direction is also the integration of aerobic treatment of organic waste with biorefinery processes. The expanding range of possible routes to use biological CO as a valuable resource in reference to recent reports on the production of this gas from the composting process (Stegenta et al., 2019a; Stegenta et al., 2019b) raises a new, important question: is it possible to control and enhance the biological CO production from aerobic processes for the biorefinery industry and/or H2 production? Observations made for green waste (Kurola et al., 2010), their mixture with manure (Hellebrand and Kalk, 2001), organic waste (Haarstad et al., 2006), the municipal waste ((Phillip et al., 2011; Stegenta et al., 2018) and studies on the dual nature of CO production biotic and abiotic, (Stegenta-Dąbrowska et al., 2019) indicate that the composted mass may contain bacteria responsible for metabolizing CO, capable of producing the enzyme carbon monoxide dehydrogenase (CODH). If this hypothesis was confirmed, it would significantly increase the potential sources of CO to be used in the further development of biorefineries. Therefore, laboratory-scale studies are needed to understand in detail the process and biological factors influencing CO production during composting.
Additionally, due to the described earlier problems associated with CO high affinity to metalloenzymes, there is a concentration barrier for this compound in the liquid phase that limits the growth and activity of microorganisms. Therefore, it is essential to develop strain engineering using tools developed on the organism model, which will allow not only to prepare the bacteria to work in the harsh anaerobic environment of the process with gaseous substrates but also to obtain new products from the same substrates. The support may be predictive models based on omics data and the use of bioinformatics tools that enable learning about the rules and variables affecting metabolic processes, as well as their control and optimization. Moreover, one of the biggest problems with the efficiency of biorefinery processes, mass transfer limitations, is being gradually addressed by the latest technological advances such as the use of high mass-transfer bioreactors and anaerobic biofoundries. However, further efforts are needed to develop technologies on a larger scale, which is linked to the need to finance pilot operations.
Conclusion
As shown in this review article, such an inconspicuous molecule as CO plays an essential role in each of the elements of the environment, directly and indirectly influencing the processes taking place. Moreover, its absence would disrupt natural changes in nature and affect plants and the organisms’ functioning—both animals and humans.
Ignored in many aspects of pro-environmental and economic activities, CO is becoming noticed by an increasing number of researchers, activists, and industry representatives, gaining more and more importance, e.g., in medicine, veterinary medicine, and the chemical industry and energy industries. The initial neglect of CO as a harmful gas over many years of research conducted on plants, animals, and humans has turned into an approach consisting of minimizing the effects of CO and the pathways of its effective use and achievement of intended goals. Similarly, the first observations and research conducted on CO fate in the atmosphere, soils, and water bodies became the basis for the currently extended analyzes of the ways of metabolizing CO in a biological way using microorganisms, which are crucial for the development of the biorefinery industry. The first laboratory analyses, as well as experiments carried out in pilot plants, subsequently led to the transfer of CO as a substrate to commercial plants, which proves that despite its toxic nature, this compound is a valuable material for biological processes.
Because of that, CO application in biorefinery can be a part of the circular economy. However, the technically and economically successful implementation of such processes has been revealed to be very challenging and requires continuous progress. Like every industrial process, using CO in biorefinery should be economically efficient, which is connected with the possibility of stable production of large amounts of a standardized product. Additionally, process specifications need to consider the variability in raw waste material. Therefore, further studies on the processes’ kinetics in fully controlled and well-mixed laboratory-scale stirred-tank bioreactors are needed to provide the data allowing for the modeling of the biorefinery concept on an industrial scale.
Author Contributions
KS: Conceptualization, Data curation, Funding acquisition, Resources, Writing–original draft. SS-D: Conceptualization, Data curation, Resources, Writing–original draft. GL: Conceptualization, Writing–review and editing. JK: Conceptualization, Supervision, Writing–review and editing. AB: Conceptualization, Data curation, Funding acquisition, Resources, Supervision, Writing–review and editing.
Funding
The APC is co-financed by Wroclaw University of Environmental and Life Sciences co-financed by the European Social Fund under the Operational Program Knowledge Education Development, under Contract No. POWR.03.05.00-00-Z062/18 of June 4, 2019.
Conflict of Interest
The authors declare that the research was conducted in the absence of any commercial or financial relationships that could be construed as a potential conflict of interest.
Publisher’s Note
All claims expressed in this article are solely those of the authors and do not necessarily represent those of their affiliated organizations, or those of the publisher, the editors and the reviewers. Any product that may be evaluated in this article, or claim that may be made by its manufacturer, is not guaranteed or endorsed by the publisher.
Acknowledgments
The presented article was prepared as part of the activity of the leading research team—Waste and Biomass Valorization Group (WBVG).
References
Adach, W., Błaszczyk, M., and Olas, B. (2020). Carbon Monoxide and its Donors - Chemical and Biological Properties. Chemico-Biological Interactions 318, 108973. doi:10.1016/j.cbi.2020.108973
Alfano, M., and Cavazza, C. (2018). The Biologically Mediated Water-Gas Shift Reaction: Structure, Function and Biosynthesis of Monofunctional [NiFe]-Carbon Monoxide Dehydrogenases. Sustain. Energ. Fuels 2, 1653–1670. doi:10.1039/C8SE00085A
Amos, W. A. (2004). Biological Water-Gas Shift Conversion of Carbon Monoxide to Hydrogen: Milestone Completion Report. National Renewable Energy Lab., Golden, CO(US) Available at: https://www.osti.gov/biblio/15006928 (Accessed December 30, 2020).
Andreae, M. O., Artaxo, P., Beck, V., Bela, M., Freitas, S., Gerbig, C., et al. (2012). Carbon Monoxide and Related Trace Gases and Aerosols over the Amazon Basin during the Wet and Dry Seasons. Atmos. Chem. Phys. 12, 6041–6065. doi:10.5194/acp-12-6041-2012
Annau, Z. (1975). “The Comparative Effects of Hypoxic and Carbon Monoxide Hypoxia on Behavior,” in Behavioral Toxicology Environmental Science Research. Editors B. Weiss, and V. G. Laties (Boston, MA: Springer US), 105–127. doi:10.1007/978-1-4684-2859-9_5
Ayoub Meo, S., Adnan Abukhalaf, A., Sami, W., and Hoang, T. D. (2021). Effect of Environmental Pollution PM2.5, Carbon Monoxide, and Ozone on the Incidence and Mortality Due to SARS-CoV-2 Infection in London, United Kingdom. J. King Saud Univ. - Sci. 33, 101373. doi:10.1016/j.jksus.2021.101373
Back, K. C., and Dominguez, A. M. (1968). Psychopharmacology of Carbon Monoxide under Ambient and Altitude conditionsAMRL-TR-68-175. AMRL TR, 81–92.
Badr, O., and Probert, S. D. (1994). Carbonmonoxide Concentration in the Earth's Atmosphere. Appl. Energ. 49, 99–143. doi:10.1016/0306-2619(94)90035-3
Badr, O., and Probert, S. D. (1995). Sinks and Environmental Impacts for Atmospheric Carbon Monoxide. Appl. Energ. 50, 339–372. doi:10.1016/0306-2619(95)98803-A
Bakwin, P. S., Tans, P. P., and Novelli, P. C. (1994). Carbon Monoxide Budget in the Northern Hemisphere. Geophys. Res. Lett. 21, 433–436. doi:10.1029/94GL00006
Barbosa, S. G., Peixoto, L., Alves, J. I., and Alves, M. M. (2021). Bioelectrochemical Systems (BESs) towards Conversion of Carbon Monoxide/syngas: A Mini-Review. Renew. Sustain. Energ. Rev. 135, 110358. doi:10.1016/j.rser.2020.110358
Bartholomew, G. W., and Alexander, M. (1979). Microbial Metabolism of Carbon Monoxide in Culture and in Soil. Appl. Environ. Microbiol. 37, 932–937. doi:10.1128/aem.37.5.932-937.1979
Bartholomew, G. W., and Alexander, M. (1981). Soil as a Sink for Atmospheric Carbon Monoxide. Science 212, 1389–1391. doi:10.1126/science.212.4501.1389
Bassett, D., and Fisher, A. (1976). Metabolic Response to Carbon Monoxide by Isolated Rat Lungs. Am. J. Physiology-Legacy Content 230, 658–663. doi:10.1152/ajplegacy.1976.230.3.658
Bates, T. S., Kelly, K. C., Johnson, J. E., and Gammon, R. H. (1995). Regional and Seasonal Variations in the Flux of Oceanic Carbon Monoxide to the Atmosphere. J. Geophys. Res. 100, 23093–23101. doi:10.1029/95JD02737
Bédard, C., and Knowles, R. (1989). Physiology, Biochemistry, and Specific Inhibitors of CH4, NH4+, and CO Oxidation by Methanotrophs and Nitrifiers. Microbiol. Rev. 53, 68–84. doi:10.1128/mr.53.1.68-84.1989
Bérard, E., Bongard, V., Dallongeville, J., Arveiler, D., Amouyel, P., Wagner, A., et al. (2015). Expired-air Carbon Monoxide as a Predictor of 16-year Risk of All-Cause, Cardiovascular and Cancer Mortality. Prev. Med. 81, 195–201. doi:10.1016/j.ypmed.2015.09.001
Berent, A. C., Todd, J., Sergeeff, J., and Powell, L. L. (2005). Carbon Monoxide Toxicity: a Case Series. J. Vet. Emerg. Crit. Care 15, 128–135. doi:10.1111/j.1476-4431.2005.00140.x
Bergamaschi, P., Hein, R., Brenninkmeijer, C. A. M., and Crutzen, P. J. (2000). Inverse Modeling of the Global CO Cycle: 2. Inversion of13C/12C and18O/16O Isotope Ratios. J. Geophys. Res. 105, 1929–1945. doi:10.1029/1999JD900819
Bilban, M., Haschemi, A., Wegiel, B., Chin, B. Y., Wagner, O., and Otterbein, L. E. (2008). Heme Oxygenase and Carbon Monoxide Initiate Homeostatic Signaling. J. Mol. Med. 86, 267–279. doi:10.1007/s00109-007-0276-0
Blomquist, B. W., Fairall, C. W., Huebert, B. J., and Wilson, S. T. (2012). Direct Measurement of the Oceanic Carbon Monoxide Flux by Eddy Correlation. Atmos. Meas. Tech. 5, 3069–3075. doi:10.5194/amt-5-3069-2012
Bruhn, D., Albert, K. R., Mikkelsen, T. N., and Ambus, P. (2013). UV-induced Carbon Monoxide Emission from Living Vegetation. Biogeosciences 10, 7877–7882. doi:10.5194/bg-10-7877-2013
Bukur, D. B., Todic, B., and Elbashir, N. (2016). Role of Water-Gas-Shift Reaction in Fischer-Tropsch Synthesis on Iron Catalysts: A Review. Catal. Today 275, 66–75. doi:10.1016/j.cattod.2015.11.005
Burrell, G. A., and Seibert, F. M. (1914). Experiments with Small Animals and Carbon Monoxide. J. Ind. Eng. Chem. 6, 241–244. doi:10.1021/ie50063a027
Butler, J. H., Jones, R. D., Garber, J. H., and Gordon, L. I. (1987). Seasonal Distributions and Turnover of Reduced Trace Gases and Hydroxylamine in Yaquina Bay, Oregon. Geochimica et Cosmochimica Acta 51, 697–706. doi:10.1016/0016-7037(87)90080-9
Cao, Z.-Y., Xuan, W., Liu, Z.-Y., Li, X.-N., Zhao, N., Xu, P., et al. (2007b). Carbon Monoxide Promotes Lateral Root Formation in Rapeseed. J. Integr. Plant Biol. 49, 1070–1079. doi:10.1111/j.1672-9072.2007.00482.x
Cao, Z., Huang, B., Wang, Q., Xuan, W., Ling, T., Zhang, B., et al. (2007a). Involvement of Carbon Monoxide Produced by Heme Oxygenase in ABA-Induced Stomatal Closure in Vicia faba and its Proposed Signal Transduction Pathway. CHINESE SCI. BULL. 52, 2365–2373. doi:10.1007/s11434-007-0358-y
Chapman, J. T., Otterbein, L. E., Elias, J. A., and Choi, A. M. (2001). Carbon Monoxide Attenuates Aeroallergen-Induced Inflammation in Mice. Am. J. Physiol. Lung Cell. Mol. Physiol. 281, L209–L216. doi:10.1152/ajplung.2001.281.1.L209
Chappelle, E. W. (1962). Carbon Monoxide Oxidation by Algae. Biochim. Biophys. Acta 62, 45–62. doi:10.1016/0006-3002(62)90491-2
Choi, O., Kim, T., Woo, H. M., and Um, Y. (2014). Electricity-driven Metabolic Shift through Direct Electron Uptake by Electroactive Heterotroph Clostridiumpasteurianum. Sci. Rep. 4, 6961. doi:10.1038/srep06961
Choi, O., and Sang, B.-I. (2016). Extracellular Electron Transfer from Cathode to Microbes: Application for Biofuel Production. Biotechnol. Biofuels 9, 11. doi:10.1186/s13068-016-0426-0
Claassens, N. J., Cotton, C. A. R., Kopljar, D., and Bar-Even, A. (2019). Making Quantitative Sense of Electromicrobial Production. Nat. Catal. 2, 437–447. doi:10.1038/s41929-019-0272-0
Clomburg, J. M., Crumbley, A. M., and Gonzalez, R. (2017). Industrial Biomanufacturing: The Future of Chemical Production. Science 355. doi:10.1126/science.aag0804
Coburn, R., Wallace, H., and Abboud, R. (1971). Redistribution of Body Carbon Monoxide after Hemorrhage. Am. J. Physiology-Legacy Content 220, 868–873. doi:10.1152/ajplegacy.1971.220.4.868
Conrad, R., Schütz, H., and Seiler, W. (1988). Emission of Carbon Monoxide from Submerged rice fields into the Atmosphere. Atmos. Environ. (1967) 22, 821–823. doi:10.1016/0004-6981(88)90021-2
Conrad, R., and Seiler, W. (1982a). Arid Soils as a Source of Atmospheric Carbon Monoxide. Geophys. Res. Lett. 9, 1353–1356. doi:10.1029/GL009i012p01353
Conrad, R., Seiler, W., Bunse, G., and Giehl, H. (1982). Carbon Monoxide in Seawater (Atlantic Ocean). J. Geophys. Res. 87, 8839–8852. doi:10.1029/JC087iC11p08839
Conrad, R., and Seiler, W. (1985a). Characteristics of Abiological Carbon Monoxide Formation from Soil Organic Matter, Humic Acids, and Phenolic Compounds. Environ. Sci. Technol. 19, 1165–1169. doi:10.1021/es00142a004
Conrad, R., and Seiler, W. (1979). Field Measurements of Hydrogen Evolution by Nitrogen-Fixing Legumes. Soil Biology + Biochemistry (UK). Available at: https://agris.fao.org/agris-search/search.do?recordID=XE8001911 [Accessed June 25, 2020].doi:10.1016/0038-0717(79)90041-5
Conrad, R., and Seiler, W. (1985b). Influence of Temperature, Moisture, and Organic Carbon on the Flux of H2and CO between Soil and Atmosphere: Field Studies in Subtropical Regions. J. Geophys. Res. 90, 5699–5709. doi:10.1029/JD090iD03p05699
Conrad, R., and Seiler, W. (1985c). Influence of Temperature, Moisture, and Organic Carbon on the Flux of H2and CO between Soil and Atmosphere: Field Studies in Subtropical Regions. J. Geophys. Res. 90, 5699–5709. doi:10.1029/jd090id03p05699
Conrad, R., and Seiler, W. (1980a). Photooxidative Production and Microbial Consumption of Carbon Monoxide in Seawater. FEMS Microbiol. Lett. 9, 61–64. doi:10.1111/j.1574-6968.1980.tb05606.x
Conrad, R., and Seiler, W. (1980b). Role of Microorganisms in the Consumption and Production of Atmospheric Carbon Monoxide by Soil. Appl. Environ. Microbiol. 40, 437–445. doi:10.1128/aem.40.3.437-445.1980
Conrad, R., and Seiler, W. (1982b). Utilization of Traces of Carbon Monoxide by Aerobic Oligotrophic Microorganisms in Ocean, lake and Soil. Arch. Microbiol. 132, 41–46. doi:10.1007/BF00690815
Conrad, R. (1996). Soil Microorganisms as Controllers of Atmospheric Trace Gases (H2, CO, CH4, OCS, N2O, and NO). Microbiol. Rev. 60, 609–640. doi:10.1128/mr.60.4.609-640.1996
Correa-Aragunde, N., Graziano, M., and Lamattina, L. (2004). Nitric Oxide Plays a central Role in Determining Lateral Root Development in Tomato. Planta 218, 900–905. doi:10.1007/s00425-003-1172-7
Crutzen, P. J., and Gidel, L. T. (1983). A Two-Dimensional Photochemical Model of the Atmosphere: 2. The Tropospheric Budgets of the Anthropogenic Chlorocarbons CO, CH4, CH3Cl and the Effect of Various NOxsources on Tropospheric Ozone. J. Geophys. Res. 88, 6641–6661. doi:10.1029/JC088iC11p06641
Cui, W., Qi, F., Zhang, Y., Cao, H., Zhang, J., Wang, R., et al. (2015). Methane-rich Water Induces Cucumber Adventitious Rooting through Heme Oxygenase1/carbon Monoxide and Ca2+ Pathways. Plant Cel Rep 34, 435–445. doi:10.1007/s00299-014-1723-3
Cui, X., Su, H.-Y., Chen, R., Yu, L., Dong, J., Ma, C., et al. (2019). Room-temperature Electrochemical Water-Gas Shift Reaction for High Purity Hydrogen Production. Nat. Commun. 10, 86. doi:10.1038/s41467-018-07937-w
Dai, Y., Wensink, P. C., and Abeles, R. H. (1999). One Protein, Two Enzymes. J. Biol. Chem. 274, 1193–1195. doi:10.1074/jbc.274.3.1193
Dang, J., Wang, N., and Atiyeh, H. K. (2021). Review of Dissolved CO and H2 Measurement Methods for Syngas Fermentation. Sensors 21, 2165. doi:10.3390/s21062165
DeBias, D. A., Banerjee, C. M., Birkhead, N. C., Greene, C. H., Scott, S. D., and Harrer, W. V. (1976). Effects of Carbon Monoxide Inhalation on Ventricular Fibrillation. Arch. Environ. Health Int. J. 31, 42–46. doi:10.1080/00039896.1976.10667188
Dekker, J., and Hargrove, M. (2002). Weedy Adaptation in Setaria Spp. V. Effects of Gaseous Environment on Giant Foxtail (Setaria Faberii) (Poaceae) Seed Germination. Am. J. Bot. 89, 410–416. doi:10.3732/ajb.89.3.410
Devarapalli, M., and Atiyeh, H. K. (2015). A Review of Conversion Processes for Bioethanol Production with a Focus on Syngas Fermentation. Biofuel Res. J. 2, 268–280. doi:10.18331/BRJ2015.2.3.5
Diender, M., Stams, A. J. M., and Sousa, D. Z. (2015). Pathways and Bioenergetics of Anaerobic Carbon Monoxide Fermentation. Front. Microbiol. 6. doi:10.3389/fmicb.2015.01275
Drake, H. L., and Daniel, S. L. (2004). Physiology of the Thermophilic Acetogen Moorella Thermoacetica. Res. Microbiol. 155, 869–883. doi:10.1016/j.resmic.2004.10.002
Drennan, C. L., Heo, J., Sintchak, M. D., Schreiter, E., and Ludden, P. W. (2001). Life on Carbon Monoxide: X-ray Structure of Rhodospirillum Rubrum Ni-Fe-S Carbon Monoxide Dehydrogenase. Proc. Natl. Acad. Sci. 98, 11973–11978. doi:10.1073/pnas.211429998
Duggin, J. A., and Cataldo, D. A. (1985). The Rapid Oxidation of Atmospheric CO to CO2 by Soils. Soil Biol. Biochem. 17, 469–474. doi:10.1016/0038-0717(85)90011-2
Dulak, J., and Józkowicz, A. (2003). Carbon Monoxide -- a "new" Gaseous Modulator of Gene Expression. Acta Biochim. Pol. 50, 31035001031–31035001047. doi:10.18388/abp.2003_3712
Duncan, B. N., Logan, J. A., Bey, I., Megretskaia, I. A., Yantosca, R. M., Novelli, P. C., et al. (2007). Global Budget of CO, 1988-1997: Source Estimates and Validation with a Global Model. J. Geophys. Res. 112. doi:10.1029/2007JD008459
Ehrich, W. E., Bellet, S., and Lewey, F. H. (1944). Cardiac Changes from CO Poisoning. Am. J. Med. Sci. 208, 511–523.
Einzig, S., Nicoloff, D. M., and Lucas Jr., R. V. (1980). Myocardial Perfusion Abnormalities in Carbon Monoxide Poisoned Dogs. Can. J. Physiol. Pharmacol. 58, 396–405. doi:10.1139/y80-067
Engel, M., Holtmann, D., Ulber, R., and Tippkötter, N. (2019). Increased Biobutanol Production by Mediator‐Less Electro‐Fermentation. Biotechnol. J. 14, 1800514. doi:10.1002/biot.201800514
Erickson, D. J. (1989). Ocean to Atmosphere Carbon Monoxide Flux: Global Inventory and Climate Implications. Glob. Biogeochem. Cycles 3, 305–314. doi:10.1029/GB003i004p00305
Erickson, D. J., and Taylor, J. A. (1992). 3-D Tropospheric CO Modeling: The Possible Influence of the Ocean. Geophys. Res. Lett. 19, 1955–1958. doi:10.1029/92GL01475
Erickson, H. H., and Buckhold, D. K. (1972). Coronary Blood Flow and Myocardial Function during Exposure to 100 Ppm Carbon Monoxide. Physiologist 15.
Evans, W. F. J., and Puckrin, E. (1995). An Observation of the Greenhouse Radiation Associated with Carbon Monoxide. Geophys. Res. Lett. 22, 925–928. doi:10.1029/95GL00606
Fackler, N., Heijstra, B. D., Rasor, B. J., Brown, H., Martin, J., Ni, Z., et al. (2021). Stepping on the Gas to a Circular Economy: Accelerating Development of Carbon-Negative Chemical Production from Gas Fermentation. Annu. Rev. Chem. Biomol. Eng. 12, 439–470. doi:10.1146/annurev-chembioeng-120120-021122
Fast, A. G., and Papoutsakis, E. T. (2012). Stoichiometric and Energetic Analyses of Non-photosynthetic CO2-fixation Pathways to Support Synthetic Biology Strategies for Production of Fuels and Chemicals. Curr. Opin. Chem. Eng. 1, 380–395. doi:10.1016/j.coche.2012.07.005
Fein, A., Grossman, R. F., Jones, J. G., Hoeffel, J., and McKay, D. (1980). Carbon Monoxide Effect on Alveolar Epithelial Permeability. CHEST 78, 726–731. doi:10.1378/chest.78.5.726
Ferenci, T. (1974). Carbon Monoxide-Stimulated Respiration in Methane-Utilizing Bacteria. FEBS Lett. 41, 94–98. doi:10.1016/0014-5793(74)80962-2
Fisher, A. B., Hyde, R. W., Baue, A. E., Reif, J. S., and Kelly, D. F. (1969). Effect of Carbon Monoxide on Function and Structure of the Lung. J. Appl. Physiol. 26, 4–12. doi:10.1152/jappl.1969.26.1.4
Fricker, S. P. (1999). Nitric Oxide Scavengers as a Therapeutic Approach to Nitric Oxide Mediated Disease. Expert Opin. Investig. Drugs 8, 1209–1222. doi:10.1517/13543784.8.8.1209
Fukui, Y., Akane, A., Takahashi, S., Matsubara, K., and Maseda, C. (1987). Derivative Spectrophotometric Studies on Cytotoxic Effects of Carbon Monoxide. Forensic Sci. Int. 33, 75–82. doi:10.1016/0379-0738(87)90142-3
Gaubert, B., Worden, H. M., Arellano, A. F. J., Emmons, L. K., Tilmes, S., Barré, J., et al. (2017). Chemical Feedback from Decreasing Carbon Monoxide Emissions. Geophys. Res. Lett. 44, 9985–9995. doi:10.1002/2017GL074987
Goldberg, H. D., and Chappell, M. N. (1967). Behavioral Measure of Effect of Carbon Monoxide on Rats. Arch. Environ. Health Int. J. 14, 671–677. doi:10.1080/00039896.1967.10664817
Gothert, M., Hock, P., Malorny, G., and Seiler, H. J. (1972). Mechanism of Hyperglycemia during Acute Carbon Monoxide Poisoning, 274. Naunyn-Schmiedeberg’s Archives of Pharmacology.
Göthert, M., Lutz, F., and Malorny, G. (1970). Carbon Monoxide Partial Pressure in Tissue of Different Animals. Environ. Res. 3, 303–309. doi:10.1016/0013-9351(70)90023-X
Guo, K., Kong, W. W., and Yang, Z. M. (2009). Carbon Monoxide Promotes Root Hair Development in Tomato. Plant Cel Environ 32, 1033–1045. doi:10.1111/j.1365-3040.2009.01986.x
Guo, K., Xia, K., and Yang, Z.-M. (2008). Regulation of Tomato Lateral Root Development by Carbon Monoxide and Involvement in Auxin and Nitric Oxide. J. Exp. Bot. 59, 3443–3452. doi:10.1093/jxb/ern194
Haarstad, K., Bergersen, O., and Sørheim, R. (2006). Occurrence of Carbon Monoxide during Organic Waste Degradation. J. Air Waste Manage. Assoc. 56, 575–580. doi:10.1080/10473289.2006.10464470
Halebian, P., Barie, P., Robinson, N., and Shires, G. T. (1984). Effects of Carbon Monoxide on Pulmonary Fluid Accumulation. Curr. Surg. 41, 369–371.
Hameed, S., Cess, R. D., and Hogan, J. S. (1980). Response of the Global Climate to Changes in Atmospheric Chemical Composition Due to Fossil Fuel Burning. J. Geophys. Res. 85, 7537–7545. doi:10.1029/JC085iC12p07537
Han, Y., Xuan, W., Yu, T., Fang, W.-B., Lou, T.-L., Gao, Y., et al. (2007). Exogenous Hematin Alleviates Mercury-Induced Oxidative Damage in the Roots ofMedicago Sativa. J. Integr. Plant Biol. 49, 1703–1713. doi:10.1111/j.1744-7909.2007.00592.x
Han, Y., Zhang, J., Chen, X., Gao, Z., Xuan, W., Xu, S., et al. (2008). Carbon Monoxide Alleviates Cadmium‐induced Oxidative Damage by Modulating Glutathione Metabolism in the Roots of Medicago Sativa. New Phytol. 177, 155–166. doi:10.1111/j.1469-8137.2007.02251.x
Heichel, G. H. (1973). Removal of Carbon Monoxide by Field and Forest Soils. J. Environ. Qual. 2, 419–423. doi:10.2134/jeq1973.00472425000200040001x
Hellebrand, H. J., and Kalk, W.-D. (2001). Emission of Carbon Monoxide during Composting of Dung and green Waste. Nutrient Cycling in Agroecosystems 60, 79–82. doi:10.1023/A:1012666929651
Henstra, A. M., Sipma, J., Rinzema, A., and Stams, A. J. (2007). Microbiology of Synthesis Gas Fermentation for Biofuel Production. Curr. Opin. Biotechnol. 18, 200–206. doi:10.1016/j.copbio.2007.03.008
Hino, S., and Tauchi, H. (1987). Production of Carbon Monoxide from Aromatic Amino Acids by Morganella morganii. Arch. Microbiol. 148, 167–171. doi:10.1007/BF00414807
Huang, B.-K., Xu, S., Xuan, W., Li, M., Cao, Z.-Y., Liu, K.-L., et al. (2006). Carbon Monoxide Alleviates Salt-Induced Oxidative Damage in Wheat Seedling Leaves. J. Integr. Plant Biol. 48, 249–254. doi:10.1111/j.1744-7909.2006.00220.x
Hubley, J. H., Mitton, J. R., and Wilkinson, J. F. (1974). The Oxidation of Carbon Monoxide by Methane-Oxidizing Bacteria. Arch. Microbiol. 95, 365–368. doi:10.1007/BF02451778
Hugod, C. (1981). Myocardial Morphology in Rabbits Exposed to Various Gas-phase Constituents of Tobacco Smoke an Ultrastructural Study. Atherosclerosis 40, 181–190. doi:10.1016/0021-9150(81)90037-x
Hussain, A., Bruant, G., Mehta, P., Raghavan, V., Tartakovsky, B., and Guiot, S. R. (2014). Population Analysis of Mesophilic Microbial Fuel Cells Fed with Carbon Monoxide. Appl. Biochem. Biotechnol. 172, 713–726. doi:10.1007/s12010-013-0556-9
Hussain, A., Guiot, S. R., Mehta, P., Raghavan, V., and Tartakovsky, B. (2011a). Electricity Generation from Carbon Monoxide and Syngas in a Microbial Fuel Cell. Appl. Microbiol. Biotechnol. 90, 827–836. doi:10.1007/s00253-011-3188-4
Hussain, A., Mehta, P., Raghavan, V., Wang, H., Guiot, S. R., and Tartakovsky, B. (2012). The Performance of a Thermophilic Microbial Fuel Cell Fed with Synthesis Gas. Enzyme Microb. Tech. 51, 163–170. doi:10.1016/j.enzmictec.2012.05.008
Hussain, A., Tartakovsky, B., Guiot, S. R., and Raghavan, V. (2011b). Use of Silicone Membranes to Enhance Gas Transfer during Microbial Fuel Cell Operation on Carbon Monoxide. Bioresour. Tech. 102, 10898–10906. doi:10.1016/j.biortech.2011.09.057
Infantes, A., Kugel, M., Raffelt, K., and Neumann, A. (2020). Side-by-Side Comparison of Clean and Biomass-Derived, Impurity-Containing Syngas as Substrate for Acetogenic Fermentation with Clostridium Ljungdahlii. Fermentation 6, 84. doi:10.3390/fermentation6030084
Ingersoll, R. B., Inman, R. E., and Fisher, W. R. (1974). Soil's Potential as a Sink for Atmospheric Carbon Monoxide. Tellus 26, 151–159. doi:10.1111/j.2153-3490.1974.tb01961.x10.3402/tellusa.v26i1-2.9746
Inman, R. E., Ingersoll, R. B., and Levy, E. A. (1971). Soil: A Natural Sink for Carbon Monoxide. Science 172, 1229–1231. doi:10.1126/science.172.3989.1229
Ismail, K. S. K., Najafpour, G., Younesi, H., Mohamed, A. R., and Kamaruddin, A. H. (2008). Biological Hydrogen Production from CO: Bioreactor Performance. Biochem. Eng. J. 39, 468–477. doi:10.1016/j.bej.2007.11.003
Jackson, C. B., and Drobatz, K. (2002). Neurologic Dysfunction Associated with Smoke Exposure in Dogs. J. Vet. Emerg. Crit. Care 12.
James, W. E., Tucker, C. E., and Grover, R. F. (1979). Cardiac Function in Goats Exposed to Carbon Monoxide. J. Appl. Physiol. 47, 429–434. doi:10.1152/jappl.1979.47.2.429
Johnson, J. E., and Bates, T. S. (1996). Sources and Sinks of Carbon Monoxide in the Mixed Layer of the Tropical South Pacific Ocean. Glob. Biogeochem. Cycles 10, 347–359. doi:10.1029/96GB00366
Jones, R. A., Strickland, J. A., Stunkard, J. A., and Siegel, J. (1971). Effects on Experimental Animals of Long-Term Inhalation Exposure to Carbon Monoxide. Toxicol. Appl. Pharmacol. 19, 46–53. doi:10.1016/0041-008X(71)90188-8
Jones, R. D., and Amador, J. A. (1993). Methane and Carbon Monoxide Production, Oxidation, and Turnover Times in the Caribbean Sea as Influenced by the Orinoco River. J. Geophys. Res. 98, 2353–2359. doi:10.1029/92JC02769
Jones, R. D. (1991). Carbon Monoxide and Methane Distribution and Consumption in the Photic Zone of the Sargasso Sea. Deep Sea Res. A. Oceanographic Res. Pap. 38, 625–635. doi:10.1016/0198-0149(91)90002-W
Jones, R. D., and Morita, R. Y. (1983). Carbon Monoxide Oxidation by Chemolithotrophic Ammonium Oxidizers. Can. J. Microbiol. 29, 1545–1551. doi:10.1139/m83-237
Jones, R., Morita, R., and Griffiths, R. (1984). Method for Estimating In Situ Chemo-Lithotrophic Ammonium Oxidation Using Carbon Monoxide Oxidation. Mar. Ecol. Prog. Ser. 17, 259–269. doi:10.3354/meps017259
Katori, M., Anselmo, D. M., Busuttil, R. W., and Kupiec-Weglinski, J. W. (2002). A Novel Strategy against Ischemia and Reperfusion Injury: Cytoprotection with Heme Oxygenase System. Transpl. Immunol. 9, 227–233. doi:10.1016/S0966-3274(02)00043-6
Katsumata, Y., Aoki, M., Oya, M., Yada, S., and Suzuki, O. (1980). Liver Damage in Rats during Acute Carbon Monoxide Poisoning. Forensic Sci. Int. 16, 119–123. doi:10.1016/0379-0738(80)90164-4
Katz, E. J., Bruce, J. G., and Petrie, B. D. (1980). “Salt and Mass Flux in the Atlantic Equatorial Undercurrent,” in Oceanography and Surface Layer Meteorology in the B/C Scale. Editors G. Siedler, J. D. Woods, and W. Düing (Pergamon), 137–160. doi:10.1016/B978-1-4832-8366-1.50026-1
Kent, M., Creevy, K. E., and Delahunta, A. (2010). Clinical and Neuropathological Findings of Acute Carbon Monoxide Toxicity in Chihuahuas Following Smoke Inhalation. J. Am. Anim. Hosp. Assoc. 46, 259–264. doi:10.5326/0460259
Khalil, M. a. K., and Rasmussen, R. A. (1984). Carbon Monoxide in the Earth's Atmosphere: Increasing Trend. Science 224, 54–56. doi:10.1126/science.224.4644.54
Kiessling, M., and Meyer, O. (1982). Profitable Oxidation of Carbon Monoxide or Hydrogen during Heterotrophic Growth ofPseudomonas Carboxydoflava. FEMS Microbiol. Lett. 13, 333–338. doi:10.1111/j.1574-6968.1982.tb08283.x
Kim, C., Kim, M. Y., Michie, I., Jeon, B.-H., Premier, G. C., Park, S., et al. (2017). Anodic Electro-Fermentation of 3-hydroxypropionic Acid from Glycerol by Recombinant Klebsiella pneumoniae L17 in a Bioelectrochemical System. Biotechnol. Biofuels 10, 199. doi:10.1186/s13068-017-0886-x
Kim, D., and Chang, I. S. (2009). Electricity Generation from Synthesis Gas by Microbial Processes: CO Fermentation and Microbial Fuel Cell Technology. Bioresour. Tech. 100, 4527–4530. doi:10.1016/j.biortech.2009.04.017
King, G. M. (1999a). Attributes of Atmospheric Carbon Monoxide Oxidation by Maine Forest Soils. Appl. Environ. Microbiol. 65, 5257–5264. doi:10.1128/AEM.65.12.5257-5264.1999
King, G. M. (1999b). Characteristics and Significance of Atmospheric Carbon Monoxide Consumption by Soils. Chemosphere - Glob. Change Sci. 1, 53–63. doi:10.1016/S1465-9972(99)00021-5
King, G. M., and Crosby, H. (2002). Impacts of Plant Roots on Soil CO Cycling and Soil-Atmosphere CO Exchange. Glob. Change Biol. 8, 1085–1093. doi:10.1046/j.1365-2486.2002.00545.x
King, G. M. (2000). Land Use Impacts on Atmospheric Carbon Monoxide Consumption by Soils. Glob. Biogeochem. Cycles 14, 1161–1172. doi:10.1029/2000GB001272
Kinoshita, H., Türkan, H., Vucinic, S., Naqvi, S., Bedair, R., Rezaee, R., et al. (2020). Carbon Monoxide Poisoning. Toxicol. Rep. 7, 169–173. doi:10.1016/j.toxrep.2020.01.005
Kleinert, H. D., Scales, J. L., and Weiss, H. R. (1980). Effects of Carbon Monoxide or Low Oxygen Gas Mixture Inhalation on Regional Oxygenation, Blood Flow, and Small Vessel Blood Content of the Rabbit Heart. Pflugers Arch. 383, 105–111. doi:10.1007/BF00581870
Kong, W. W., Zhang, L. P., Guo, K., Liu, Z. P., and Yang, Z. M. (2010). Carbon Monoxide Improves Adaptation of Arabidopsis to Iron Deficiency. Plant Biotechnol. J. 8, 88–99. doi:10.1111/j.1467-7652.2009.00469.x
Koob, G. F., Annau, Z., Rubin, R. J., and Montgomery, M. R. (1974). Effect of Hypoxic Hypoxia and Carbon Monoxide on Food Intake, Water Intake, and Body Weight in Two Strains of Rats. Life Sci. 14, 1511–1520. doi:10.1016/0024-3205(74)90162-3
Köpke, M., Held, C., Hujer, S., Liesegang, H., Wiezer, A., Wollherr, A., et al. (2010). Clostridium Ljungdahlii Represents a Microbial Production Platform Based on Syngas. Proc. Natl. Acad. Sci. 107, 13087–13092. doi:10.1073/pnas.1004716107
Köpke, M., and Simpson, S. D. (2020). Pollution to Products: Recycling of 'above Ground' Carbon by Gas Fermentation. Curr. Opin. Biotechnol. 65, 180–189. doi:10.1016/j.copbio.2020.02.017
Kracke, F., Virdis, B., Bernhardt, P. V., Rabaey, K., and Krömer, J. O. (2016). Redox Dependent Metabolic Shift in Clostridium Autoethanogenum by Extracellular Electron Supply. Biotechnol. Biofuels 9, 249. doi:10.1186/s13068-016-0663-2
Krall, A. R., and Tolbert, N. E. (1957). A Comparison of the Light Dependent Metabolism of Carbon Monoxide by Barley Leaves with that of Formaldehyde, Formate and Carbon Dioxide. Plant Physiol. 32, 321–326. doi:10.1104/pp.32.4.321
Kramkowski, K., Leszczynska, A., Mogielnicki, A., Chlopicki, S., Fedorowicz, A., Grochal, E., et al. (2012). Antithrombotic Properties of Water-Soluble Carbon Monoxide-Releasing Molecules. Atvb 32, 2149–2157. doi:10.1161/ATVBAHA.112.253989
Kuhlbusch, T. A. J., Zepp, R. G., Miller, W. L., and Burke, R. A. (1998). Carbon Monoxide Fluxes of Different Soil Layers in upland Canadian Boreal Forests. Tellus B: Chem. Phys. Meteorology 50, 353–365. doi:10.3402/tellusb.v50i4.16136
Kumar, G., Saratale, R. G., Kadier, A., Sivagurunathan, P., Zhen, G., Kim, S.-H., et al. (2017). A Review on Bio-Electrochemical Systems (BESs) for the Syngas and Value Added Biochemicals Production. Chemosphere 177, 84–92. doi:10.1016/j.chemosphere.2017.02.135
Kurola, J. M., Arnold, M., Kontro, M. H., Talves, M., and Romantschuk, M. (2010). Effect of Light Sphagnum Peat on Odour Formation in the Early Stages of Biowaste Composting. Waste Manage. 30, 779–786. doi:10.1016/j.wasman.2009.12.014
Lamontagne, R. A., Swinnerton, J. W., and Linnenbom, V. J. (1971). Nonequilibrium of Carbon Monoxide and Methane at the Air-Sea Interface. J. Geophys. Res. 76, 5117–5121. doi:10.1029/JC076i021p05117
Laties, V. G., and Merigan, W. H. (1979). Behavioral Effects of Carbon Monoxide on Animals and Man. Annu. Rev. Pharmacol. Toxicol. 19, 357–392. doi:10.1146/annurev.pa.19.040179.002041
Levine, J. S., Rinsland, C. P., and Tennille, G. M. (1985). The Photochemistry of Methane and Carbon Monoxide in the Troposphere in 1950 and 1985. Nature 318, 254–257. doi:10.1038/318254a0
Lewey, F. H., and Drabkin, D. L. (1944). Experimental Chronic Carbon Monoxide Poisoning of Dogs. Am. J. Med. Sci. 208, 502–511. doi:10.1097/00000441-194410000-00009
Liebl, K. H., and Seiler, W. (1976). “CO and H2 Destruction at the Soil Surface,” in Microbial Production and Utilization of Gases (E. Goltze KG), 215–229.
Liew, F., Martin, M. E., Tappel, R. C., Heijstra, B. D., Mihalcea, C., and Köpke, M. (2016). Gas Fermentation-A Flexible Platform for Commercial Scale Production of Low-Carbon-Fuels and Chemicals from Waste and Renewable Feedstocks. Front. Microbiol. 7, 694. doi:10.3389/fmicb.2016.00694
Lin, Y., Zhang, W., Qi, F., Cui, W., Xie, Y., and Shen, W. (2014). Hydrogen-rich Water Regulates Cucumber Adventitious Root Development in a Heme Oxygenase-1/carbon Monoxide-dependent Manner. J. Plant Physiol. 171, 1–8. doi:10.1016/j.jplph.2013.08.009
Ling, T., Zhang, B., Cui, W., Wu, M., Lin, J., Zhou, W., et al. (2009). Carbon Monoxide Mitigates Salt-Induced Inhibition of Root Growth and Suppresses Programmed Cell Death in Wheat Primary Roots by Inhibiting Superoxide Anion Overproduction. Plant Sci. 177, 331–340. doi:10.1016/j.plantsci.2009.06.004
Linnenbom, V. J., Swinnerton, J. W., and Lamontagne, R. A. (1973). The Ocean as a Source for Atmospheric Carbon Monoxide. J. Geophys. Res. 78, 5333–5340. 1896-1977. doi:10.1029/JC078i024p05333
Liu, K., Xu, S., Xuan, W., Ling, T., Cao, Z., Huang, B., et al. (2007). Carbon Monoxide Counteracts the Inhibition of Seed Germination and Alleviates Oxidative Damage Caused by Salt Stress in Oryza Sativa. Plant Sci. 172, 544–555. doi:10.1016/j.plantsci.2006.11.007
Liu, X.-M., Chapman, G. B., Peyton, K. J., Schafer, A. I., and Durante, W. (2003). Antiapoptotic Action of Carbon Monoxide on Cultured Vascular Smooth Muscle Cells. Exp. Biol. Med. (Maywood) 228, 572–575. doi:10.1177/15353702-0322805-30
Liu, Y., Xu, S., Ling, T., Xu, L., and Shen, W. (2010). Heme Oxygenase/carbon Monoxide System Participates in Regulating Wheat Seed Germination under Osmotic Stress Involving the Nitric Oxide Pathway. J. Plant Physiol. 167, 1371–1379. doi:10.1016/j.jplph.2010.05.021
Logan, B. E., Call, D., Cheng, S., Hamelers, H. V. M., Sleutels, T. H. J. A., Jeremiasse, A. W., et al. (2008). Microbial Electrolysis Cells for High Yield Hydrogen Gas Production from Organic Matter. Environ. Sci. Technol. 42, 8630–8640. doi:10.1021/es801553z
Logan, J. A., Prather, M. J., Wofsy, S. C., and McElroy, M. B. (1981). Tropospheric Chemistry: A Global Perspective. J. Geophys. Res. 86, 7210–7254. doi:10.1029/JC086iC08p07210
Lovley, D. R. (2011). Powering Microbes with Electricity: Direct Electron Transfer from Electrodes to Microbes. Environ. Microbiol. Rep. 3, 27–35. doi:10.1111/j.1758-2229.2010.00211.x
Mahajan, S., and Jagtap, S. (2020). Metal-oxide Semiconductors for Carbon Monoxide (CO) Gas Sensing: A Review. Appl. Mater. Today 18, 100483. doi:10.1016/j.apmt.2019.100483
Maines, M. D. (1997). The Heme Oxygenase System: a Regulator of Second Messenger Gases. Annu. Rev. Pharmacol. Toxicol. 37, 517–554. doi:10.1146/annurev.pharmtox.37.1.517
Malorny, G. (1972). Survey of the Effect of Carbon Monoxide on Man. Staub Reinhalt. Luft (Engl. Ed. 32, 1–15.
Mandal, S., Ruggles, R., Leonardi, G., Goodfellow, F., Bradley, N., and Murray, V. (2011). Developing Best Practice Response to Carbon Monoxide Incidents: a Toolkit for Health protection Frontline Staff. Public Health 125, 148–156. doi:10.1016/j.puhe.2010.11.011
Mariani, C. L. (2003). Full Recovery Following Delayed Neurologic Signs after Smoke Inhalation in a Dog. J. Veter Emer Crit. 13, 235–239. doi:10.1111/j.1534-6935.2003.00101.x
Mehta, P., Hussain, A., Tartakovsky, B., Neburchilov, V., Raghavan, V., Wang, H., et al. (2010). Electricity Generation from Carbon Monoxide in a Single Chamber Microbial Fuel Cell. Enzyme Microb. Tech. 46, 450–455. doi:10.1016/j.enzmictec.2010.02.010
Meng, D. K., Chen, J., and Yang, Z. M. (2011). Enhancement of Tolerance of Indian Mustard (Brassica Juncea) to Mercury by Carbon Monoxide. J. Hazard. Mater. 186, 1823–1829. doi:10.1016/j.jhazmat.2010.12.062
Meo, S. A., Abukhalaf, A. A., Alomar, A. A., Alessa, O. M., Sami, W., and Klonoff, D. C. (2021). Effect of Environmental Pollutants PM-2.5, Carbon Monoxide, and Ozone on the Incidence and Mortality of SARS-COV-2 Infection in Ten Wildfire Affected Counties in California. Sci. Total Environ. 757, 143948. doi:10.1016/j.scitotenv.2020.143948
Meyer, O., and Schlegel, H. G. (1983). Biology of Aerobic Carbon Monoxide-Oxidizing Bacteria. Annu. Rev. Microbiol. 37, 277–310. doi:10.1146/annurev.mi.37.100183.001425
Miyahara, S., and Takahashi, H. (1971). Biological CO Evolution. J. Biochem. 69, 231–233. doi:10.1093/oxfordjournals.jbchem.a129450
Monson, R. K., and Holland, E. A. (2001). Biospheric Trace Gas Fluxes and Their Control over Tropospheric Chemistry. Annu. Rev. Ecol. Syst. 32, 547–576. doi:10.1146/annurev.ecolsys.32.081501.114136
Mopper, K., Zhou, X., Kieber, R. J., Kieber, D. J., Sikorski, R. J., and Jones, R. D. (1991). Photochemical Degradation of Dissolved Organic Carbon and its Impact on the Oceanic Carbon Cycle. Nature 353, 60–62. doi:10.1038/353060a0
Mopper, K., and Kieber, D. J. (2000). “Marine Photochemistry and its Impact on Carbon Cycling,” in The Effects of UV Radiation in the Marine Environment Cambridge Environmental Chemistry Series. Editors M. Vernet, S. Demers, and S. De Mora (Cambridge: Cambridge University Press), 101–129. doi:10.1017/CBO9780511535444.005
Moscoviz, R., Trably, E., and Bernet, N. (2018). Electro-fermentation Triggering Population Selection in Mixed-Culture Glycerol Fermentation. Microb. Biotechnol. 11, 74–83. doi:10.1111/1751-7915.12747
Moxley, J. M., and Smith, K. A. (1998a). Carbon Monoxide Production and Emission by Some Scottish Soils. Tellus B 50, 151–162. doi:10.1034/j.1600-0889.1998.t01-1-00003.x
Moxley, J. M., and Smith, K. A. (1998b). Factors Affecting Utilisation of Atmospheric CO by Soils. Soil Biol. Biochem. 30, 65–79. doi:10.1016/S0038-0717(97)00095-3
Muramoto, T., Tsurui, N., Terry, M. J., Yokota, A., and Kohchi, T. (2002). Expression and Biochemical Properties of a Ferredoxin-dependent Heme Oxygenase Required for Phytochrome Chromophore Synthesis. Plant Physiol. 130, 1958–1966. doi:10.1104/pp.008128
Musselman, N. P., Groff, W. A., Yevich, P. O., Wilinski, F. T., Weeks, M. S., and Oberst, F. W. (1960). Continuous Exposure of Laboratory Animals to Low Concentration of Carbon Monoxide. J. Occup. Environ. Med. 2, 409. doi:10.1097/00043764-196008000-00026
Nakao, A., Choi, A. M. K., and Murase, N. (2006). Protective Effect of Carbon Monoxide in Transplantation. J. Cell. Mol. Med. 10, 650–671. doi:10.1111/j.1582-4934.2006.tb00426.x
Neburchilov, V., Mehta, P., Hussain, A., Wang, H., Guiot, S. R., and Tartakovsky, B. (2011). Microbial Fuel Cell Operation on Carbon Monoxide: Cathode Catalyst Selection. Int. J. Hydrogen Energ. 36, 11929–11935. doi:10.1016/j.ijhydene.2011.06.076
Nevin, K. P., Hensley, S. A., Franks, A. E., Summers, Z. M., Ou, J., Woodard, T. L., et al. (2011). Electrosynthesis of Organic Compounds from Carbon Dioxide Is Catalyzed by a Diversity of Acetogenic Microorganisms. Applied and Environmental Microbiology. Available at: https://journals.asm.org/doi/abs/10.1128/AEM.02642-10 [Accessed January 9, 2022].doi:10.1128/aem.02642-10
Niden, A. H., and Schulz, H. (1965). The Ultrastructural Effects of Carbon Monoxide Inhalation on the Rat Lung. Virchows Arch. Path Anat. 339, 283–292. doi:10.1007/BF00959687
Olas, B. (2014). Carbon Monoxide Is Not Always a Poison Gas for Human Organism: Physiological and Pharmacological Features of CO. Chemico-Biological Interactions 222, 37–43. doi:10.1016/j.cbi.2014.08.005
Oliveira, A. P., Bornstein, R. D., and Soares, J. (2003). Annual and Diurnal Wind Patterns in the City of São Paulo. Water Air Soil Pollut. Focus 3, 3–15. doi:10.1023/A:1026090103764
Otterbein, L. E. (2002). Carbon Monoxide: Innovative Anti-inflammatory Properties of an Age-Old Gas Molecule. Antioxid. Redox Signaling 4, 309–319. doi:10.1089/152308602753666361
Ou Yang, C.-F., Lin, Y.-C., Lin, N.-H., Lee, C.-T., Sheu, G.-R., Kam, S.-H., et al. (2009). Inter-comparison of Three Instruments for Measuring Regional Background Carbon Monoxide. Atmos. Environ. 43, 6449–6453. doi:10.1016/j.atmosenv.2009.09.026
Ozaki, K. S., Kimura, S., and Murase, N. (2012). Use of Carbon Monoxide in Minimizing Ischemia/reperfusion Injury in Transplantation. Transplant. Rev. 26, 125–139. doi:10.1016/j.trre.2011.01.004
Pankow, D., and Ponsold, W. (1978). Effect of Single and Repeated Carbon Monoxide Intoxications on Urinary Catecholamine Excretion in Rats. Acta Biol. Med. Ger. 37, 1589–1593.
Penney, D., and Maziarka, T. (1976). Effect of Acute Carbon Monoxide Poisoning on Serum Lactate Dehydrogenase and Creatine Phosphokinase. J. Toxicol. Environ. Health 1, 1017–1021. doi:10.1080/15287397609529404
Phillip, E. A., Clark, O. G., Londry, K., Yu, S., and Leonard, J. (2011). Emission of Carbon Monoxide during Composting of Municipal Solid Waste. Compost. Sci. Utilization 19, 170–177. doi:10.1080/1065657X.2011.10736996
Pierce, E., Xie, G., Barabote, R. D., Saunders, E., Han, C. S., Detter, J. C., et al. (2008). The Complete Genome Sequence of Moorella Thermoacetica (F. Clostridium Thermoaceticum). Environ. Microbiol. 10, 2550–2573. doi:10.1111/j.1462-2920.2008.01679.x
Pihlatie, M., Rannik, Ü., Haapanala, S., Peltola, O., Shurpali, N., Martikainen, P. J., et al. (2016). Seasonal and Diurnal Variation in CO Fluxes from an Agricultural Bioenergy Crop. Biogeosciences 13, 5471–5485. doi:10.5194/bg-13-5471-2016
Plevová, J., and Frantík, E. (1974). The Influence of Various Saturation Rates on Motor Performance of Rats Exposed to Carbon Monoxide. Act Nerv Super (Praha) 16, 101–102.
P. M. Maitlis, and A. D. Klerk (Editors) (2013). Greener Fischer-Tropsch Processes: For Fuels and Feedstocks. 1st edition (Weinheim: Wiley VCH).
Pos, W. H., Riemer, D. D., and Zika, R. G. (1998). Carbonyl Sulfide (OCS) and Carbon Monoxide (CO) in Natural Waters: Evidence of a Coupled Production Pathway. Mar. Chem. 62, 89–101. doi:10.1016/S0304-4203(98)00025-5
Potter, C. S., Klooster, S. A., and Chatfield, R. B. (1996). Consumption and Production of Carbon Monoxide in Soils: A Global Model Analysis of Spatial and Seasonal Variation. Chemosphere 33, 1175–1193. doi:10.1016/0045-6535(96)00254-8
Preziosi, T. J., Lindenberg, R., Levy, D., and Christenson, M. (1970). An Experimental Investigation in Animals of the Functional and Morphologic Effects of Single and Repeated Exposures to High and Low Concentrations of Carbon Monoxide. Ann. NY Acad. Sci. 174, 369–384. doi:10.1111/j.1749-6632.1970.tb49800.x
Rabaey, K., and Rozendal, R. A. (2010). Microbial Electrosynthesis - Revisiting the Electrical Route for Microbial Production. Nat. Rev. Microbiol. 8, 706–716. doi:10.1038/nrmicro2422
Rakitin, V. S., Elansky, N. F., Skorokhod, A. I., Dzhola, A. V., Rakitina, A. V., Shilkin, A. V., et al. (2021). Long-Term Tendencies of Carbon Monoxide in the Atmosphere of the Moscow Megapolis. Izv. Atmos. Ocean. Phys. 57, 116–125. doi:10.1134/S0001433821010102
Ramanathan, V., Cicerone, R. J., Singh, H. B., and Kiehl, J. T. (1985). Trace Gas Trends and Their Potential Role in Climate Change. J. Geophys. Res. 90, 5547–5566. doi:10.1029/JD090iD03p05547
Ramezani, M., Kashfipour, M. A., and Abolhasani, M. (2020). Minireview: Flow Chemistry Studies of High-Pressure Gas-Liquid Reactions with Carbon Monoxide and Hydrogen. J. Flow Chem. 10, 93–101. doi:10.1007/s41981-019-00059-4
Reddy, G. K., and Smirniotis, P. G. (2015). Water Gas Shift Reaction. Elsevier. doi:10.1016/C2013-0-09821-0
Rozante, J., Rozante, V., Souza Alvim, D., Ocimar Manzi, A., Barboza Chiquetto, J., Siqueira D’Amelio, M., et al. (2017). Variations of Carbon Monoxide Concentrations in the Megacity of São Paulo from 2000 to 2015 in Different Time Scales. Atmosphere 8, 81. doi:10.3390/atmos8050081
Ruth-Sahd, L. A., Zulkosky, K., and Fetter, M. E. (2011). Carbon Monoxide Poisoning. Dimensions Crit. Care Nurs. 30, 303–314. doi:10.1097/DCC.0b013e31822fb017
Sahara, H., Shimizu, A., Setoyama, K., Okumi, M., Oku, M., Samelson-Jones, E., et al. (2010). Carbon Monoxide Reduces Pulmonary Ischemia-Reperfusion Injury in Miniature Swine. J. Thorac. Cardiovasc. Surg. 139, 1594–1601. doi:10.1016/j.jtcvs.2009.09.016
Sanhueza, E., Cárdenas, L., Donoso, L., and Santana, M. (1994a). Effect of Plowing on CO2, CO, CH4, N2O, and NO Fluxes from Tropical savannah Soils. J. Geophys. Res. 99, 16429–16434. doi:10.1029/94JD00265
Sanhueza, E., Dong, Y., Scharffe, D., Lobert, J. M., and Crutzen, P. J. (1998). Carbon Monoxide Uptake by Temperate forest Soils: the Effects of Leaves and Humus Layers. Tellus B: Chem. Phys. Meteorology 50, 51–58. doi:10.3402/tellusb.v50i1.16021
Sanhueza, E., Donoso, L., Scharffe, D., and Crutzen, P. J. (1994b). Carbon Monoxide Fluxes from Natural, Managed, or Cultivated savannah Grasslands. J. Geophys. Res. 99, 16421–16427. doi:10.1029/93JD02918
Sansaniwal, S. K., Pal, K., Rosen, M. A., and Tyagi, S. K. (2017). Recent Advances in the Development of Biomass Gasification Technology: A Comprehensive Review. Renew. Sustain. Energ. Rev. 72, 363–384. doi:10.1016/j.rser.2017.01.038
Santoro, C., Arbizzani, C., Erable, B., and Ieropoulos, I. (2017). Microbial Fuel Cells: From Fundamentals to Applications. A Review. J. Power Sourc. 356, 225–244. doi:10.1016/j.jpowsour.2017.03.109
Savolainen, H., Kurppa, K., Tenhunen, R., and Kivistö, H. (1980). Biochemical Effects of Carbon Monoxide Poisoning in Rat Brain with Special Reference to Blood Carboxyhemoglobin and Cerebral Cytochrome Oxidase Activity. Neurosci. Lett. 19, 319–323. doi:10.1016/0304-3940(80)90281-5
Schade, G. W., and Crutzen, P. J. (1999). CO Emissions from Degrading Plant Matter (II). Tellus B: Chem. Phys. Meteorology 51, 909–918. doi:10.3402/tellusb.v51i5.16503
Schade, G. W., Hofmann, R.-M., and Crutzen, P. J. (1999). CO Emissions from Degrading Plant Matter. Tellus B 51, 889–908. doi:10.1034/j.1600-0889.1999.t01-4-00003.x
Scharffe, D., Hao, W. M., Donoso, L., Crutzen, P. J., and Sanhueza, E. (1990). Soil Fluxes and Atmospheric Concentration of CO and CH4in the Northern Part of the Guayana Shield, Venezuela. J. Geophys. Res. 95, 22475–22480. doi:10.1029/JD095iD13p22475
Schrenk, H. H., Patty, F. A., and Yant, W. P. (1932). Studies in Asphyxia: II. Blood Chemistry Changes Resulting from Comparatively Rapid Asphyxia by Atmospheres Deficient in Oxygen. Public Health Rep. 47, 136–146. 1896-1970. doi:10.2307/4580314
Seiler, W., Giehl, H., and Bunse, G. (1978). The Influence of Plants on Atmospheric Carbon Monoxide and Dinitrogen Oxide. PAGEOPH 116, 439–451. doi:10.1007/BF01636898
Seiler, W. (1974). The Cycle of Atmospheric CO. Tellus 26, 116–135. doi:10.1111/j.2153-3490.1974.tb01958.x10.3402/tellusa.v26i1-2.9743
Seiler, W. (1978). “The Influence of the Biosphere on the Atmospheric CO and H2 Cycles,” in Environmental Biogeochemistry and Geomicrobiology. Methods, Metals and Assessment (Ann Arbor Scientific Publishers), 773–810.
Seiler, W., and Warneck, P. (1972). Decrease of the Carbon Monoxide Mixing Ratio at the Tropopause. J. Geophys. Res. 77, 3204–3214. 1896-1977. doi:10.1029/JC077i018p03204
Selvatico, D., Lanzini, A., and Santarelli, M. (2016). Low Temperature Fischer-Tropsch Fuels from Syngas: Kinetic Modeling and Process Simulation of Different Plant Configurations. Fuel 186, 544–560. doi:10.1016/j.fuel.2016.08.093
Shell, (2022). Pearl GTL. Available at: https://www.shell.com/about-us/major-projects/pearl-gtl.html [Accessed January 6, 2022].
Śmiałek, M., Sikorska, M., Korthals, J., Bicz, W., and Mossakowski, M. J. (1973). The Glycogen Content and its Topography and UDP Glucose: Glycogen α-4 Glucosyltransferase (EC 2.4.1.11). Activity in Rat Brain after Experimental Carbon Monoxide Intoxication. Acta Neuropathol. 24, 222–231. doi:10.1007/BF00687592
Smith, E., and Penrod, K. E. (1940). Blood Sugar, Insulin, and Dextrose Tolerance in Albino Rat Treated with Carbon Monoxide. Exp. Biol. Med. 45, 222–224. doi:10.3181/00379727-45-11634
Smith, K. A., Bremner, J. M., and Tabatabai, M. A. (1973). Sorption of Gaseous Atmospheric Pollutants by Soils. Soil Sci. 116, 313–319. doi:10.1097/00010694-197310000-00009
Sobhakumari, A., Poppenga, R. H., Pesavento, J. B., and Uzal, F. A. (2018). Pathology of Carbon Monoxide Poisoning in Two Cats. BMC Vet. Res. 14, 67. doi:10.1186/s12917-018-1385-4
Sokal, J., Majka, J., and Palus, J. (1986). Effect of Work Load on the Content of Carboxymyoglobin in the Heart and Skeletal Muscles of Rats Exposed to Carbon Monoxide. J. Hyg. Epidemiol. Microbiol. Immunol. 30, 57–62.
Sokal, J. A., Majka, J., and Palus, J. (1984). The Content of Carbon Monoxide in the Tissues of Rats Intoxicated with Carbon Monoxide in Various Conditions of Acute Exposure. Arch. Toxicol. 56, 106–108. doi:10.1007/BF00349080
Stegenta, S., Dębowski, M., Bukowski, P., Randerson, P. F., and Białowiec, A. (2018). The Influence of Perforation of Foil Reactors on Greenhouse Gas Emission Rates during Aerobic Biostabilization of the Undersize Fraction of Municipal Wastes. J. Environ. Manage. 207, 355–365. doi:10.1016/j.jenvman.2017.11.054
Stegenta, S., Sobieraj, K., Pilarski, G., Koziel, J. A., and Białowiec, A. (2019a). Analysis of the Spatial and Temporal Distribution of Process Gases within Municipal Biowaste Compost. Sustainability 11, 2340. doi:10.3390/su11082340
Stegenta, S., Sobieraj, K., Pilarski, G., Koziel, J., and Białowiec, A. (2019b). The Spatial and Temporal Distribution of Process Gases within the Biowaste Compost. Data 4, 37. doi:10.3390/data4010037
Stegenta-Dąbrowska, S., Drabczyński, G., Sobieraj, K., Koziel, J. A., and Białowiec, A. (2019). The Biotic and Abiotic Carbon Monoxide Formation during Aerobic Co-digestion of Dairy Cattle Manure with Green Waste and Sawdust. Front. Bioeng. Biotechnol. 7. doi:10.3389/fbioe.2019.00283
Stubbins, A., Uher, G., Kitidis, V., Law, C. S., Upstill-Goddard, R. C., and Woodward, E. M. S. (2006a). The Open-Ocean Source of Atmospheric Carbon Monoxide. Deep Sea Res. Part Topical Stud. Oceanography 53, 1685–1694. doi:10.1016/j.dsr2.2006.05.010
Stubbins, A., Uher, G., Law, C. S., Mopper, K., Robinson, C., and Upstill-Goddard, R. C. (2006b). Open-ocean Carbon Monoxide Photoproduction. Deep Sea Res. Part Topical Stud. Oceanography 53, 1695–1705. doi:10.1016/j.dsr2.2006.05.011
Stupfel, M., and Bouley, G. (1970). Physiological and Biochemical Effects on Rats and Mice Exposed to Small Concentrations of Carbon Monoxide for Long Periods. Ann. NY Acad. Sci. 174, 342–368. doi:10.1111/j.1749-6632.1970.tb49799.x
Sun, W., Kooijmans, L. M. J., Maseyk, K., Chen, H., Mammarella, I., Vesala, T., et al. (2018). Soil Fluxes of Carbonyl Sulfide (COS), Carbon Monoxide, and Carbon Dioxide in a Boreal forest in Southern Finland. Atmos. Chem. Phys. 18, 1363–1378. doi:10.5194/acp-18-1363-2018
Swinnerton, J. W., and Lamontagne, R. A. (1974). Carbon Monoxide in the South Pacific Ocean. Tellus 26, 136–142. doi:10.3402/tellusa.v26i1-2.9744
Swinnerton, J. W., Linnenbom, V. J., and Lamontagne, R. A. (1970). The Ocean: a Natural Source of Carbon Monoxide. Science 167, 984–986. doi:10.1126/science.167.3920.984
Takahashi, K. (1961). Cardiac Disturbances Due to CO Poisoning in Experimental Animals. Tohoku J. Exp. Med. 74, 211–223. doi:10.1620/tjem.74.211
Takors, R., Kopf, M., Mampel, J., Bluemke, W., Blombach, B., Eikmanns, B., et al. (2018). Using Gas Mixtures of CO, CO2and H2as Microbial Substrates: the Do's and Don'ts of Successful Technology Transfer from Laboratory to Production Scale. Microb. Biotechnol. 11, 606–625. doi:10.1111/1751-7915.13270
Tarr, M. A., Miller, W. L., and Zepp, R. G. (1995). Direct Carbon Monoxide Photoproduction from Plant Matter. J. Geophys. Res. 100, 11403–11413. doi:10.1029/94JD03324
Teichner, W. H. (1967). An Exploration of Some Behavioral Techniques for Toxicity Testing. J. Psychol. 65, 69–90. doi:10.1080/00223980.1967.10543823
Theodore, J., O’Donnell, R. D., and Back, K. C. (1971). Toxicological Evaluation of Carbon Monoxide in Humans and Other Mammalian Species. Air Force Aerospace Medical Research Lab Wright-Patterson Afb Oh Available at: https://apps.dtic.mil/sti/citations/AD0725203 [Accessed March 8, 2021].
Thom, S. R., Xu, Y. A., and Ischiropoulos, H. (1997). Vascular Endothelial Cells Generate Peroxynitrite in Response to Carbon Monoxide Exposure. Chem. Res. Toxicol. 10, 1023–1031. doi:10.1021/tx970041h
Thompson, A. M. (1992). The Oxidizing Capacity of the Earth's Atmosphere: Probable Past and Future Changes. Science 256, 1157–1165. doi:10.1126/science.256.5060.1157
Tolli, J. D., and Taylor, C. D. (2005). Biological CO Oxidation in the Sargasso Sea and in Vineyard Sound, Massachusetts. Limnol. Oceanogr. 50, 1205–1212. doi:10.4319/lo.2005.50.4.1205
Valentine, R. L., and Zepp, R. G. (1993). Formation of Carbon Monoxide from the Photodegradation of Terrestrial Dissolved Organic Carbon in Natural Waters. Environ. Sci. Technol. 27, 409–412. doi:10.1021/es00039a023
van Asperen, H., Warneke, T., Sabbatini, S., Nicolini, G., Papale, D., and Notholt, J. (2015). The Role of Photo- and thermal Degradation for CO2 and CO Fluxes in an Arid Ecosystem. Biogeosciences 12, 4161–4174. doi:10.5194/bg-12-4161-2015
Vanoli, E., De Ferrari, G. M., Stramba-Badiale, M., Farber, J. P., and Schwartz, P. J. (1989). Carbon Monoxide and Lethal Arrhythmias in Conscious Dogs with a Healed Myocardial Infarction. Am. Heart J. 117, 348–357. doi:10.1016/0002-8703(89)90778-3
Verma, A., Hirsch, D. J., Glatt, C. E., Ronnett, G. V., and Snyder, S. H. (1993). Carbon Monoxide: A Putative Neural Messenger. Science 259, 381–384. doi:10.1126/science.7678352
Voiland, A. (2015). Fourteen Years of Carbon Monoxide from MOPITT. NASA’s Earth Observatory. Available at: https://earthobservatory.nasa.gov/images/85967/fourteen-years-of-carbon-monoxide-from-mopitt [Accessed May 26, 2021].
Voituriez, B., and Herbland, A. (1979). The Use of the Salinity Maximum of the Equatorial Undercurrent for Estimating Nutrient Enrichment and Primary Production in the Gulf of Guinea. Deep Sea Res. Part A. Oceanographic Res. Pap. 26, 77–83. doi:10.1016/0198-0149(79)90087-6
von Oettingen, F. (1944). Carbon Monoxide : its Hazards and the Mechanism of its Action. Carbon Monoxide : its Hazards and the Mechanism of its Action. Available at: https://www.cabdirect.org/cabdirect/abstract/19462700490 [Accessed March 10, 2021].
Wainaina, S., Horváth, I. S., and Taherzadeh, M. J. (2018). Biochemicals from Food Waste and Recalcitrant Biomass via Syngas Fermentation: A Review. Bioresour. Tech. 248, 113–121. doi:10.1016/j.biortech.2017.06.075
Wang, M., and Liao, W. (2016). Carbon Monoxide as a Signaling Molecule in Plants. Front. Plant Sci. 7. doi:10.3389/fpls.2016.00572
Wang, R., Wang, Z., and Wu, L. (1997). Carbon Monoxide-Induced Vasorelaxation and the Underlying Mechanisms. Br. J. Pharmacol. 121, 927–934. doi:10.1038/sj.bjp.0701222
Wang, Y., Li, J., Wang, L., Lin, Y., Zhou, M., Yin, P., et al. (2021). The Impact of Carbon Monoxide on Years of Life Lost and Modified Effect by Individual- and City-Level Characteristics: Evidence from a Nationwide Time-Series Study in China. Ecotoxicology Environ. Saf. 210, 111884. doi:10.1016/j.ecoenv.2020.111884
Whalen, S. C., and Reeburgh, W. S. (2001). Carbon Monoxide Consumption in upland Boreal forest Soils. Soil Biol. Biochem. 33, 1329–1338. doi:10.1016/S0038-0717(01)00038-4
Wilks, S. S. (1959). Carbon Monoxide in green Plants. Science 129, 964–966. doi:10.1126/science.129.3354.964
Wilson, D. F., Swinnerton, J. W., and Lamontagne, R. A. (1970). Production of Carbon Monoxide and Gaseous Hydrocarbons in Seawater: Relation to Dissolved Organic Carbon. Science 168, 1577–1579. doi:10.1126/science.168.3939.1577
World Health Organization (2010). in Who Guidelines for Indoor Air Quality: Selected Pollutants (Copenhagen: WHO).
Wu, M., Ren, Q., Durkin, A. S., Daugherty, S. C., Brinkac, L. M., Dodson, R. J., et al. (2005). Life in Hot Carbon Monoxide: The Complete Genome Sequence of Carboxydothermus Hydrogenoformans Z-2901. Plos Genet. preprint, e65. doi:10.1371/journal.pgen.001006510.1371/journal.pgen.0010065.eor
Xie, H., Andrews, S. S., Martin, W. R., Miller, J., Ziolkowski, L., Taylor, C. D., et al. (2002). Validated Methods for Sampling and Headspace Analysis of Carbon Monoxide in Seawater. Mar. Chem. 77, 93–108. doi:10.1016/S0304-4203(01)00065-2
Xie, Y., Ling, T., Han, Y., Liu, K., Zheng, Q., Huang, L., et al. (2008). Carbon Monoxide Enhances Salt Tolerance by Nitric Oxide-Mediated Maintenance of Ion Homeostasis and Up-Regulation of Antioxidant Defence in Wheat Seedling Roots. Plant Cel Environ 31, 1864–1881. doi:10.1111/j.1365-3040.2008.01888.x
Xie, Y., Zhang, C., Lai, D., Sun, Y., Samma, M. K., Zhang, J., et al. (2014). Hydrogen Sulfide Delays GA-triggered Programmed Cell Death in Wheat Aleurone Layers by the Modulation of Glutathione Homeostasis and Heme Oxygenase-1 Expression. J. Plant Physiol. 171, 53–62. doi:10.1016/j.jplph.2013.09.018
Xu, J., Xuan, W., Huang, B., Zhou, Y., Ling, T., Xu, S., et al. (2006a). Carbon Monoxide-Induced Adventitious Rooting of Hypocotyl Cuttings from Mung Bean Seedling. CHINESE SCI. BULL. 51, 668–674. doi:10.1007/s11434-006-0668-5
Xu, S., Sa, Z.-S., Cao, Z.-Y., Xuan, W., Huang, B.-K., Ling, T.-F., et al. (2006b). Carbon Monoxide Alleviates Wheat Seed Germination Inhibition and Counteracts Lipid Peroxidation Mediated by Salinity. J. Integr. Plant Biol. 48, 1168–1176. doi:10.1111/j.1744-7909.2006.00337.x
Xuan, W., Huang, L., Li, M., Huang, B., Xu, S., Liu, H., et al. (2007). Induction of Growth Elongation in Wheat Root Segments by Heme Molecules: a Regulatory Role of Carbon Monoxide in Plants. Plant Growth Regul. 52, 41–51. doi:10.1007/s10725-007-9175-1
Xuan, W., Xu, S., Li, M., Han, B., Zhang, B., Zhang, J., et al. (2012). Nitric Oxide Is Involved in Hemin-Induced Cucumber Adventitious Rooting Process. J. Plant Physiol. 169, 1032–1039. doi:10.1016/j.jplph.2012.02.021
Xuan, W., Zhu, F.-Y., Xu, S., Huang, B.-K., Ling, T.-F., Qi, J.-Y., et al. (2008). The Heme Oxygenase/carbon Monoxide System Is Involved in the Auxin-Induced Cucumber Adventitious Rooting Process. Plant Physiol. 148, 881–893. doi:10.1104/pp.108.125567
Yannarelli, G. G., Noriega, G. O., Batlle, A., and Tomaro, M. L. (2006). Heme Oxygenase Up-Regulation in Ultraviolet-B Irradiated Soybean Plants Involves Reactive Oxygen Species. Planta 224, 1154–1162. doi:10.1007/s00425-006-0297-x
Yonemura, S., Kawashima, S., and Tsuruta, H. (2000). Carbon Monoxide, Hydrogen, and Methane Uptake by Soils in a Temperate Arable Field and a forest. J. Geophys. Res. 105, 14347–14362. doi:10.1029/1999JD901156
Zafiriou, O. C., Andrews, S. S., and Wang, W. (2003). Concordant Estimates of Oceanic Carbon Monoxide Source and Sink Processes in the Pacific Yield a Balanced Global "Blue-Water" CO Budget. Glob. Biogeochem. Cycles 17, 1015. doi:10.1029/2001GB001638
Zavarzin, G. A., and Nozhevnikova, A. N. (1977). Aerobic Carboxydobacteria. Microb. Ecol. 3, 305–326. doi:10.1007/BF02010738
Zellweger, C., Steinbacher, M., and Buchmann, B. (2012). Evaluation of Three New Laser Spectrometer Techniques for In-Situ Carbon Monoxide Measurements. Atmos. Meas. Tech. Discuss. 5, 4735–4769. doi:10.5194/amtd-5-4735-2012
Zepp, R. G., Miller, W. L., Tarr, M. A., Burke, R. A., and Stocks, B. J. (1997). Soil-atmosphere Fluxes of Carbon Monoxide during Early Stages of Postfire Succession in upland Canadian Boreal Forests. J. Geophys. Res. 102, 29301–29311. doi:10.1029/97JD01326
Zhang, C., Li, Y., Yuan, F., Hu, S., and He, P. (2012). Effects of Hematin and Carbon Monoxide on the Salinity Stress Responses of Cassia Obtusifolia L. Seeds and Seedlings. Plant Soil 359, 85–105. doi:10.1007/s11104-012-1194-7
Zhou, Y., Yu, W., Cao, J., and Gao, H. (2020). Harnessing Carbon Monoxide-Releasing Platforms for Cancer Therapy. Biomaterials 255, 120193. doi:10.1016/j.biomaterials.2020.120193
Zilli, C. G., Santa-Cruz, D. M., and Balestrasse, K. B. (2014). Heme Oxygenase-independent Endogenous Production of Carbon Monoxide by Soybean Plants Subjected to Salt Stress. Environ. Exp. Bot. 102, 11–16. doi:10.1016/j.envexpbot.2014.01.012
Zuo, Y., Guerrero, M. A., and Jones, R. D. (1998). Reassessment of the Ocean-To-Atmosphere Flux of Carbon Monoxide. Chem. Ecol. 14, 241–257. doi:10.1080/02757549808037606
Zuo, Y., and Hoigne, J. (1992). Formation of Hydrogen Peroxide and Depletion of Oxalic Acid in Atmospheric Water by Photolysis of Iron(III)-oxalato Complexes. Environ. Sci. Technol. 26, 1014–1022. doi:10.1021/es00029a022
Zuo, Y., and Jones, R. D. (1995). Formation of Carbon Monoxide by Photolysis of Dissolved marine Organic Material and its Significance in the Carbon Cycling of the Oceans. Naturwissenschaften 82, 472–474. doi:10.1007/BF01131598
Keywords: carbon monoxide, biorefinery, water-gas shift reaction, CO cycle, CO consumption, CO production
Citation: Sobieraj K, Stegenta-Dąbrowska S, Luo G, Koziel JA and Białowiec A (2022) Carbon Monoxide Fate in the Environment as an Inspiration For Biorefinery Industry: A Review. Front. Environ. Sci. 10:822463. doi: 10.3389/fenvs.2022.822463
Received: 26 November 2021; Accepted: 27 January 2022;
Published: 22 February 2022.
Edited by:
Nsikak U. Benson, Covenant University, NigeriaCopyright © 2022 Sobieraj, Stegenta-Dąbrowska, Luo, Koziel and Białowiec. This is an open-access article distributed under the terms of the Creative Commons Attribution License (CC BY). The use, distribution or reproduction in other forums is permitted, provided the original author(s) and the copyright owner(s) are credited and that the original publication in this journal is cited, in accordance with accepted academic practice. No use, distribution or reproduction is permitted which does not comply with these terms.
*Correspondence: Andrzej Białowiec, YW5kcnplai5iaWFsb3dpZWNAdXB3ci5lZHUucGw=