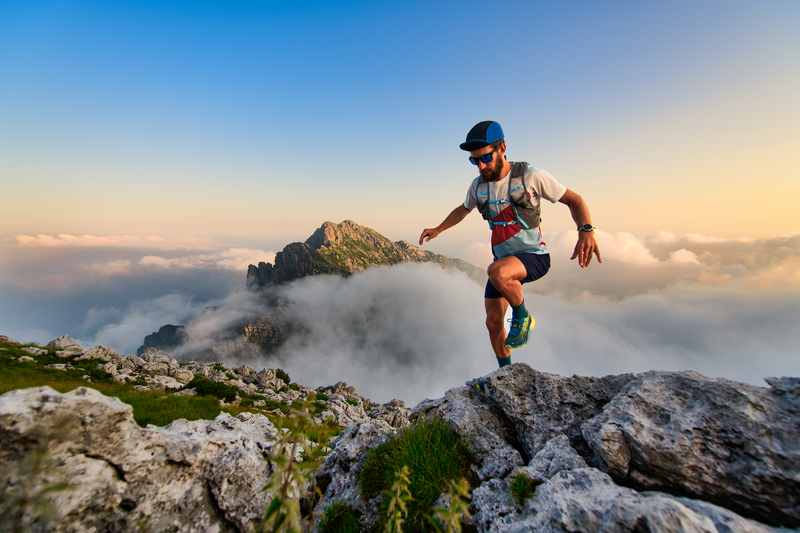
95% of researchers rate our articles as excellent or good
Learn more about the work of our research integrity team to safeguard the quality of each article we publish.
Find out more
ORIGINAL RESEARCH article
Front. Environ. Sci. , 07 April 2022
Sec. Freshwater Science
Volume 10 - 2022 | https://doi.org/10.3389/fenvs.2022.806340
Terrestrial leaf-litter (LL) inputs impose great bottom-up effects on freshwater ecosystems by fueling detritus-based food webs, affecting macroinvertebrate and microbial communities, and influencing ecosystem functioning. However, increasing intensive anthropogenic activities including the inputs of herbicide glyphosate disturb the breakdown of LL in streams. In this study, an anthropogenic carrion subsidy (chicken meat) and glyphosate (a stressor) were used to investigate their individual and combined effects on LL breakdown in urban streams and forest streams in China. We found that: 1) carrion subsidy decreased LL breakdown rate in both urban and forest streams and increased total and predator richness in forest streams, the reduced LL breakdown rates may be attributed to the foraging shift of macroinvertebrates from LL to carrion subsidy; 2) glyphosate depressed LL breakdown rate in forest but not in urban streams, the reduced LL breakdown rate may be caused by the negative effects on microbes; 3) forest streams showed significantly higher LL breakdown rates in both coarse and fine mesh bags than urban streams which were induced by the high dissolved oxygen (DO) and collector-gatherer richness. Our results provide evidence that LL breakdown in streams is sensitive to inputs of anthropogenic carrion subsidy and glyphosate through the impacts on macroinvertebrates and microbes, respectively. Furthermore, this study underscores the importance of local macroinvertebrate and microbial communities when assessing the responses of stream ecosystem functioning and macroinvertebrate communities to multiple stressors, as the individual and combined effects of stressors can be site-specific in streams with different physical characteristics and biological communities.
Terrestrial ecosystems transport large quantities of leaf-litter to streams and rivers fueling detritus-based food webs and influencing ecosystem structure and function in freshwaters (Wallace et al., 1997; Zhang et al., 2004; Marks, 2019). As the decomposition of leaf-litter is mainly driven by macroinvertebrates and microbes (Graça, 2001), any factors that can directly and/or indirectly influence these two factors may affect leaf-litter breakdown in streams (Gessner and Chauvet, 2002; Mollá et al., 2017; Boyero et al., 2021). The presence of other subsidies especially those with higher nutrient values than leaf-litter may influence LL decomposition in streams. Carrion subsidy ranging from insects to large mammals is another widespread terrestrial subsidy which is transported to freshwaters due to “arthropod rain”, migrations, and improper deposition of dead livestock (Chan, et al., 2007, 2008; Zhang and Richardson, 2011; Lamberti et al., 2020). Most of previous carrion subsidy studies focused on salmon carcasses, however, the ecological consequences of other carrion subsidies on stream ecosystems are still unclear (Lamberti et al., 2020). Some studies investigated the decomposition or the impacts of non-salmon and non-insect carcasses subsidies on streams, such as mouses (Huntsman et al., 2011), pig (Haefner et al., 2004; Barrios and Wolff, 2011), wildebeest (Subalusky et al., 2018), and bison (Wenger et al., 2019). The studies focused on the salmon carcasses have found that the input of carcasses can affect individuals and communities of macroinvertebrates and microbes (Chaloner et al., 2007; Verspoor et al., 2011). A considerable number of macroinvertebrates including caddisflies, mayflies, chironomids, and chloroperlids may benefit (e.g., increased growth rate and larger population biomass) from carrion subsidy via various pathways such as direct consumption of carrion tissue, grazing on microbes colonized on decaying carrion, shredding on enhanced quality of leaf-litter, feeding on small particle organic matter detached from decomposing carrion, and by preying on other invertebrates attracted by carrion (Minakawa et al., 2002; Fenoglio et al., 2005; Kiffney et al., 2018). Decaying carrion subsidy also provide nutrient-rich food resources for microbes including both fungi and bacteria, because macronutrients (e.g., N and P), micronutrients (e.g., Ca and K), fats, and proteins leached from carcasses can stimulate the growth of microbes (Wipfli et al., 1998; Fenoglio et al., 2010; Currier et al., 2020). Therefore, we may anticipate two contrasting effects of carcasses on leaf-litter breakdown: 1) slower its breakdown rate due to macroinvertebrates switched their diet from leaf-litter to carrion subsidy (Zhang et al., 2003); and 2) stimulated microbial activities may enhance leaf-litter breakdown rate (Claeson et al., 2006). Consequently, the overall effects of carrion subsidy on leaf-litter decomposition may depend on the relative importance of carrion subsidy on macroinvertebrates and microbes.
Apart from the inputs of carrion subsidies, streams and rivers are threatened by many other anthropogenic stressors such as pesticides (including insecticides, herbicides, and fungicides) due to the fast development of modern agriculture (Kreuger, 1998; Malmqvist and Rundle, 2002; Smedbol et al., 2018). Glyphosate is the most widely used herbicide worldwide among all the pesticides (Annett et al., 2014). The detection of glyphosate in freshwater ecosystems (e.g., streams, rivers, and lakes) have been frequently reported worldwide (Feng et al., 1990; Okada et al., 2020). Glyphosate pollution in freshwaters impacts water quality and influences communities of microbes and macroinvertebrates (Pesce et al., 2009; Magbanua et al., 2013b). Such impacts can further adversely affect leaf-litter decomposition in streams through the decreased species richness (Relyea, 2005), reduced population density (e.g., Chironomidae and Vivipariae), and changed biomass (Rzymski et al., 2013) of macroinvertebrates and microbes. What remains unclear is that thus far, no consistent agreement on the effects of low and/or environmental concentration of glyphosate on freshwater ecosystems. On the one hand, some researchers have suggested that such ecological effects in freshwaters are minor (Giesy et al., 2000). For example, Magbanua et al. (2013a) found that the addition of glyphosate (0–370 μg/L) into outdoor streamside mesocosms only affected algal community evenness. By contrast, others have found significant negative effects on the aquatic organisms such as biofilm communities (Bricheux et al., 2013). Therefore, it is unclear whether glyphosate could affect leaf-litter breakdown in streams when the concentration was low or at environmental relevant (i.e., the range of concentrations reported in most natural waterbodies) level.
In addition, it is not sufficient for only knowing the individual effects of subsidy or stressor on stream ecosystems, because streams usually face multiple stressors that interact each other (Magbanua et al., 2013b; Piggott et al., 2015; Burdon et al., 2020). This is important because streams in agricultural and urban settings where glyphosate was detected at high frequency were affected by multiple stressors such as high nutrient concentration, high water temperature, and the presence of other pesticides (Kolpin et al., 2006; Magbanua et al., 2010; Okada et al., 2020). These multiple stresses may have additive effect (no interaction) or non-additive (synergistic or antagonistic) effects on aquatic organisms (Magbanua et al., 2013a; Carles and Artigas, 2020), which can make difficulty to predict the impacts of their interactions on leaf-litter decomposition. In fact, some studies failed to detect any effects of glyphosate on leaf-litter decomposition in freshwaters and the authors suggested that the factors such as high background nutrient (e.g., N) concentration may have compensated the negative effects (Magbanua et al., 2010; Talk et al., 2016).
Differences in surrounding environment, such as urban and forest setting, can influence the processing rate of leaf litter due to the divergent biotic and abiotic attributes of these streams (Molinero et al., 1996; de Mello Cionek et al., 2021). Changes in the water quality, benthic habitats, and macroinvertebrate and microbial communities associated with urbanization may have both positive and negative effects on leaf-litter decomposition. For example, catchments with medium levels (30–40%) of impervious area had the highest biomass of fungal community, corresponding to the fastest leaf-litter breakdown rates (Chadwick et al., 2006). Whereas, the decreased macroinvertebrate richness, especially the reduced or even absent shredders in urban streams, resulted in decreased leaf-litter breakdown rates (Iñiguez-Armijos et al., 2016). These findings suggested that higher microbial activities, higher nutrient concentrations, and high abundance of grazer snails in urban streams may enhance the leaf-litter decomposition (Imberger et al., 2008; Wiederkehr et al., 2020). Therefore, it remains unclear whether urban streams have higher or lower leaf-litter breakdown rate than forest streams. Differences in macroinvertebrate and microbial communities between highly human-impacted streams and forest streams can also affect their responses to other stressors, with streams that harbor a greater proportion of tolerant species being more insensitive to stressors (Baumgartner and Robinson, 2015). For example, stream fungal communities in agricultural watershed were dominated by tolerant species and they barely responded to fungicide exposure, while fungicide inhibited fungal diversity and functioning in forest streams where most fungi were stress-sensitive species (Gardeström et al., 2016). Therefore, it is important to take local into consideration when investigating the individual and combined effects of stressors on stream ecosystems.
In this study, we assessed the individual and combined effects of glyphosate and chicken meat, as an anthropogenic stressor and carrion subsidy, respectively, on leaf-litter breakdown rate in forest and urban streams. We hypothesized that: 1) carrion subsidy would induce the diet shift of macroinvertebrates reducing leaf-litter breakdown rate, and glyphosate would negatively influence microbes, leading to a lower leaf-litter breakdown rate; 2) forest streams harboring higher abundance and richness of detritivores and better water quality would have faster leaf-litter breakdown rate than urban streams; and 3) the higher proportion of tolerant species in urban streams than in forest streams would affect the responses of macroinvertebrate communities and leaf-litter breakdown rate to carrion subsidy and glyphosate, with the impacts of the two factors being stronger in forest streams than in urban streams.
This study was conducted in five streams in Suzhou (population size: 10.68 million; area: 8,488 km2), Jiangsu Province, and four streams in Huangshan (population size: 1.38 million; area: 9,807 km2), Anhui Province, China (Figure 1). Physical characteristics of the nine streams were described previously (Xiang, 2019). In short, the five streams in Suzhou were featured by planted riparian trees (e.g., Salix and Cinnamomum), concrete stream banks, channelized watercourses, and silt and mud benthic substrate (Supplementary Table S1). By contrast, the four streams in Huangshan were characterized by highly diverse riparian vegetation (e.g., Pterocarya stenoptera and Bambusoideae), natural stream banks (e.g., stones), natural meandering watercourse, and diverse benthic substrates (mainly gravels, cobbles, and boulders). One exception was a stream which had similar substrate to that of urban streams because it located in the downstream of Huangshan District. Therefore, the nine streams were classified into two groups (i.e., forest and urban) based on the physical characteristics and surrounding environment. Three streams in Huangshan that located in Nature Reserve or surrounded by natural habitats with low human impacts were classified as forest streams, and the others were urban streams because they located in the city or town. Background water quality parameters (water temperature, dissolved oxygen (DO), pH, conductivity, ammonium-N, and nitrate-N) were measured using multiparameter water quality meter (YSI Pro Plus) and the physical characteristics (e.g., coordinate, elevation, flow velocity, and substrate) were recorded on the day leaf-litter (LL) bags were deployed. Water quality was measured at four locations where the four treatments of LL bags were deployed in each stream. Flow velocity was not measured in the five streams of Suzhou city because they were not wadable streams and all of them had velocity <0.10 m/s (estimated by using float method).
FIGURE 1. Locations of the nine streams in Suzhou (upper left) and Huangshan (lower left). Red and blue circles represent urban and forest streams, respectively.
Freshly shed Cinnamomum camphora leaves were collected daily near the campus of Xi’an-Jiaotong Liverpool University (31.27 N, 120.74 E) between June and July 2016. C. camphora is an evergreen tree species which is widely distributed and planted in Southern and Southwestern China due to the ability to absorb greenhouse gases (e.g., CO2), resistance to pests, and elegant dense canopy. Leaves with no visual damage were selected out, had attachments (e.g., small particles) gently removed and were then oven dried (60°C, 48 h) for further use. Each fine and coarse (mesh size: 0.1 and 8.0 mm) mesh bag contained 1.33 ± 0.01 g and 7.67 ± 0.02 g of dry mass leaves, respectively. The difference in LL quantity between coarse and fine mesh bags was due to the limit amount of LL. However, this difference may have negligible effects on the microbial and total decomposition, and macroinvertebrate colonization (Tiegs et al., 2008).
We had four treatments for the LL bags, i.e., control (only LL), carrion subsidy (LL and chicken meat), glyphosate (LL + glyphosate), and both carrion subsidy and glyphosate (LL + chicken meat + glyphosate). For treatments with carrion subsidy, ∼15 and ∼30 g fresh chicken meat (without bone) was put in each fine and coarse mesh litter bag, correspondingly. The final loading rates of carrion subsidy in the experimental reach (20 m between each treatment) varied between 1.0 and 2.5 g/m2, which falls in the lower range reported in other studies (Yanai and Kochi, 2005; Cram et al., 2011; Quinn et al., 2018). For glyphosate treatments, agar was used to “immobilize” glyphosate and make it diffuse over the experimental period as described by Shaw and Mibbayad (2016). This method can guarantee a non-linear continuous exposure of glyphosate with a large pulse (>10%) being released in the first 2 days followed by decreasing concentration (Shaw and Mibbayad, 2016). Detailed information on the glyphosate treatment can be found in Xiang et al. (2020). In short, the glyphosate (20 mg/L) was mixed with 2% agar, and the agar-glyphosate solutions were poured into PVC pipes (1.5 cm in diameter, 8 cm in length). Each glyphosate treatment LL bag contained one PVC pipe which equal to approximately 85 μg glyphosate. We did not replace nor add PVC pipes during the experimental period. The final exposed glyphosate concentration fell within the range of reported concentrations (0–710 μg/L) in natural waterbodies with similar habitats or in the same area as this study (Lu et al., 2015; Yang, 2015).
Leaf-litter bags were deployed in late July of 2016 in Suzhou and Huangshan. In short, LL bags were deployed from upstream to downstream in a fixed sequence: treatments of controls, with subsidy only, with both carrion subsidy and glyphosate, and with glyphosate only in each stream. Each treatment of LL bags was at least 20 m far away from each other. We tied five LL bags of the same treatment to one fishing line and LL bags were at least 20 cm apart. Five LL bags were then deployed to the streambed and were fastened to one steel bar that was hammered into the streambed to >30 cm depth. Five LL bags of each treatment of both mesh sizes were retrieved after deploying for 15 and 30 days. Each LL bag was placed in a plastic zipper bag and immediately stored in a 4°C cool box. Leaf-litter bags were brought back to the laboratory within 1 day and then stored at 4°C.
Invertebrates were picked out (for coarse mesh bags) and preserved in 70% ethanol for further identification, and the remaining leaf materials were gently washed to remove sediments within 48–72 h. Then, LL were oven dried (48–72 h, 60°C) to constant weight for the calculation of LL breakdown rates. Invertebrates were identified to the lowest possible level (most were at genus level). Functional feeding groups of macroinvertebrates were determined according to Mandaville (2002). As scrapers and shredders were the main driver of macroinvertebrate-mediated LL decomposition, and the abundance and richness of shredders were relatively low in this study, we pooled the data of scrapers and shredders as detritivores in this study. Total abundance, total richness, Shannon-Wiener diversity index (hereafter Shannon-Wiener), Simpson diversity index (hereafter Simpson), Chironomidae abundance, and abundance and richness of collector-gatherer, predator, and detritivore were also calculated for each LL bag.
The exponential decay model was used to calculate LL breakdown rate (k, d−1) as following: Wt = WI*e–kt, where Wt and WI are the final and initial dry mass of LL, respectively, and t is incubation time (day) (Bärlocher, 2020). The effect size of our treatments for each response variables (LL breakdown rate and macroinvertebrate communities) were calculated thus: effect size = (treatment value−mean control value)/mean control value (Cornejo et al., 2021; Rubio-Ríos et al., 2021).
Leaf-litter breakdown rates were compared among treatments using general linear model (GLMM) with mesh size as within-subject factor, glyphosate, and carrion subsidy as between-subject factors (fixed factors), and harvest date as covariate. The GLMM was conducted for both urban and forest streams. As we lost many samples in Suzhou area because LL bags were pulled out of streams by the residents, data collected from S1 and S3 at first sampling date were removed, and we only kept data of S4 at second sampling date in the model. Differences in macroinvertebrate communities (abundance, richness, and diversity indices) were also analyzed by using GLMM, with sampling date as within-subject factor, glyphosate, and carrion subsidy as between-subject factors. We also checked the differences of effect sizes of each response variables among treatments and between urban streams and forest streams by conducting two-way ANOVA (analysis of variance). Water quality characteristics were compared between urban and forest streams by using one-way ANOVA with stream type as a fixed factor. Differences in macroinvertebrate communities (abundance, richness, and diversity indices) in coarse mesh bags between urban and forest streams were also compared by conducting t-test. Finally, multiple linear regression analysis was conducted to test the correlations between LL breakdown rates and macroinvertebrate communities and water quality characteristics. Before conducting the analysis, linear correlations were done to exclude the variables that did not have linear relationships with LL breakdown rates and collinearity was checked for R values larger than 0.7. Before conducting all analyses, the normality of residuals of all data were checked, and when data deviated from normality they were transformed (e.g., log). All data were analyzed by using SPSS 26.0.
All measured water quality characteristics differed between urban streams and forest streams (Supplementary Figure S1). Urban streams had 4.2°C higher mean water temperature than forest streams. Dissolved oxygen (DO) in forest streams was 8.78 mg/L which was 2.55 times of that in urban streams. By contrast, conductivity, ammonium-N, and nitrate-N in urban streams were 5.47, 5.59, and 2.02 times of that in forest streams. In addition, the urban stream in Huangshan had similar pH and water temperature with the forest streams in Huangshan.
Leaf-litter (LL) breakdown rates in coarse mesh bags (0.024 d−1) were significantly higher than in fine mesh bags (0.022 d−1) when all data were combined. However, the differences of LL breakdown rates between coarse and fine mesh bags only appeared in forest streams (Table 1). Leaf-litter breakdown rates in forest streams (0.029 d−1) were 61.1% higher than in urban streams (0.018 d−1, p < 0.001, Figure 2). The addition of carrion subsidy reduced LL breakdown rates by 16.1 and 10.5% in forest and urban streams, respectively (Table 1). By contrast, glyphosate decreased LL breakdown rates by 10.0% in forest streams but had no effect in urban streams. The effects of carrion subsidy and glyphosate on LL breakdown rates interacted with mesh size (Table 1). The negative effects of carrion subsidy were greater in coarse than in fine mesh bags in both forest and urban streams. The addition of glyphosate negatively decreased the LL breakdown rate in fine mesh bags but had no effects in coarse mesh bags in forest streams. There was no interaction between mesh size and glyphosate on LL breakdown rates in urban streams. No interaction between carrion subsidy and glyphosate were found on LL breakdown rates in neither forest nor urban streams. The effect size of our treatments on LL breakdown rates only differed in coarse mesh bags, with the effect size for glyphosate was higher than for carrion subsidy and the addition of both glyphosate and carrion subsidy (Supplementary Figure S2).
TABLE 1. Results of general linear model comparing leaf litter breakdown rates (day−1) in coarse and fine mesh bags across treatments of glyphosate (G) and carrion subsidy (S). Mesh size (MS) was “within-subject” factor (fixed), glyphosate and carrion subsidy were “between-subject” factors (fixed), and sampling date (T) were treated as covariate. p values <0.05 are shown in bold.
FIGURE 2. Violin plot of leaf-litter breakdown rate (k, day−1) in urban and forest streams. The box plots summarize the distribution of all values for each type of stream. Box plots indicated interquartile ranges (box part), 25th and 75th percentiles (lower and upper error bars), and mean values (red circles in the box).
None of the macroinvertebrate community attributes (e.g., abundance, richness, and diversity indices) were affected by glyphosate in neither forest nor urban streams (Table 2). The addition of carrion subsidy increased total and predator richness in forest streams by 2.3 and 19.9%, respectively. However, none of the measured macroinvertebrate indices were affected by the addition of carrion subsidy in urban streams (Table 2). Six out of the 11 measured macroinvertebrate indices were affected by the sampling date in both forest and urban streams (Table 2). In forest streams, Shannon-Wiener, Simpson, total richness, and abundance and richness of detritivores were higher while Chironomidae abundance was lower at the second sampling date (30 days) than at the first sampling date (15 days). In urban streams, Shannon-Wiener, Simpson, total richness, predator abundance, and detritivore richness were higher while total abundance was lower at the second sampling date (30 days) than at the first sampling date (15 days). In addition, no interactions between carrion subsidy and glyphosate were found on macroinvertebrate communities in neither forest nor urban streams. However, there were some interactions between sampling date and our treatments (carrion subsidy and glyphosate) on several macroinvertebrate indices in urban streams. Furthermore, the effect sizes of our treatments only differed between urban and forest streams for predator and detritivore richness, with the effect sizes were higher in forest than in urban streams (Supplementary Figure S3). Forest streams had higher abundance and richness for total, collector-gatherer, predator, and detritivore than urban streams (Supplementary Figure S4).
TABLE 2. Results of general linear model comparing macroinvertebrate communities (H, Shannon-Wiener diversity index; Sim, Simpson diversity index; total (T_A) and Chironomidae abundance (Ch_A); T_R, total richness; and abundance and richness of collector-gatherer (c-g), predator (pre), and detritivore) at first (15 days) and second (30 days) sampling date across treatments of glyphosate (G) and carrion subsidy (S). Sampling date (T) was “within-subject” factor (fixed), glyphosate and carrion subsidy were “between-subject” factors (fixed). ns not significant, *p < 0.05, **p < 0.01, ***p < 0.001.
All water quality indices showed significant linear relationships with LL breakdown rates except for nitrate (Supplementary Figure S5). Leaf-litter breakdown rates also were correlated with all the measured macroinvertebrate community attributes (Supplementary Figure S5). Results of multiple linear regression analysis indicated that DO was the main water quality factor driving LL breakdown in streams regardless of mesh size, which explained 45.1 and 37.9% of the variations in LL breakdown in coarse and fine mesh bags, respectively (Table 3). An increase of 1.0 mg/L in DO result in an increase of 0.002 d−1 and 0.001 d−1 in LL breakdown rate in coarse and fine mesh bags, respectively (Figure 3A). Richness of collector-gatherers was the most influential factor which contributed to 39.1% of the variations in LL breakdown rates (Figure 3B).
TABLE 3. Results of multiple linear regression analyses of leaf litter breakdown rates in streams with water quality and macroinvertebrate communities. *p < 0.05, **p < 0.01, ***p < 0.001.
FIGURE 3. Variations in leaf-litter breakdown rate in relation to dissolved oxygen (A) and collector-gatherer richness (B). *p < 0.05, **p < 0.01, ***p < 0.001.
Carrion subsidy reduced leaf-litter (LL) breakdown rate, supports our first hypothesis. The interaction between carrion subsidy and mesh size showed that the effect of carrion subsidy on LL breakdown rate only appeared in coarse mesh bags in both forest and urban streams, which suggested that carrion subsidy function through the impacts on macroinvertebrates to influence LL breakdown. This result is similar to studies that reported no positive correlation between carrion subsidy and microbes (Claeson et al., 2006; Cram et al., 2011), but it is contrary to other studies that showed carcass subsidy stimulated biofilm production through the release of nutrients (Currier et al., 2020). As we did not measure the biomass nor the microbial community structures, we could not explain why we did not find any effects of carrion subsidy on LL breakdown rates in fine mesh bags. However, one possible explanation may be attributed to the relatively short duration of this study (i.e., 1 month) because the indirect uptake of carrion-derived nutrients by microbes may be delayed by around 2 months (Claeson et al., 2006). An alternative explanation for this lack of effect on carrion subsidy is that the loading rates applied (∼1.0–2.5 g/m2) were low and is far less than the loading rate (∼1.0–2.0 kg/m2) for peak responses of primary producers (Cram et al., 2011), thereby limiting the supply of nutrients for the growth of microbes (Cram et al., 2011). Further studies should be conducted to test our two hypothesized explanations.
In forest streams, carrion subsidy increased total and predator richness, and the effect size of carrion subsidy on the abundance and richness of detritivores and predators were larger than zero indicating that abundance and richness of detritivores and predators were enhanced by the addition of carrion subsidy. If higher abundance and richness of detritivores corresponding to faster LL breakdown rate, we should have found positive effects of carrion subsidy on LL decomposition. However, we found that carrion subsidy decreased LL breakdown rates in forest streams. Therefore, we suggested that carrion subsidy induced temporal diet shift of detritivores and predators from LL and other macroinvertebrates to carrion subsidy to slow down LL breakdown rate (Bretherton et al., 2011). Because macroinvertebrate communities in our forest streams were dominated by aquatic insects such as Chironomidae, Heptageniidae, Ephemerellidae, and Elmidae (Xiang et al., 2020), and these species can rapidly colonize and directly consume carrion subsidy (Fenoglio et al., 2010). However, we were not sure why carrion subsidy decreased LL breakdown rates in urban streams because none of the measured macroinvertebrate indices were affected by carrion subsidy. One possible explanation may be that carrion subsidy enhanced the abundance of Erpobdellidae in urban streams (Xiang et al., 2020), whose predatory pressure might have depressed the abundance and richness of other macroinvertebrates, with further consequence on the inhibited LL breakdown rates. Further studies should be conducted to test the mechanisms of carrion subsidy on LL breakdown rates in urban streams.
Glyphosate significantly decreased LL breakdown, which support our first hypothesis. Numerous studies have found significant impacts of pesticides on LL breakdown rate in streams and rivers (Wallace et al., 1982; Schäfer et al., 2012). Pesticides can affect water quality, communities of microbes (e.g., bacteria and fungi) and macroinvertebrates, which all influence LL breakdown. For example, glyphosate increased P concentration (Vera et al., 2010), induced oxidative stress on caddisfly (Hydropsyche exocellata) (Puértolas et al., 2010), and stimulated the abundance of some bacterial species (e.g., Chloroflexi, Bacteroidetes, and Planctomycetes) that can use glyphosate as P source (Carles and Artigas, 2020). As we found no significant effect of glyphosate on macroinvertebrate communities and LL breakdown rate was decreased by the addition of glyphosate only in fine mesh bags. These results imply that glyphosate may have no or low effects on macroinvertebrates while it could impose negative effects on microbes to reduce LL breakdown. Many studies have found that the addition of glyphosate in freshwaters adversely affected microbes by reducing biomass of biofilms (Shaw and Mibbayad, 2016) and altering microbial community composition and diversity (Pesce et al., 2009). For example, Vera et al. (2010) found delayed colonization of periphyton and mortality of algae especially diatoms by the addition of glyphosate in a 42-days outdoor mesocosms experiment. In addition, glyphosate may interacted with other factors (e.g. P concentration and other pesticides) to affect microbial communities (Carles and Artigas, 2020) which should be taken into consideration for future studies. As the application concentration of glyphosate was low in this study, our results and many other studies suggested that the safety threshold set for the protection of aquatic organisms in many countries (e.g., 800 μg/L in Canada) are still be toxic to a number of aquatic organisms including plants, microbes and macroinvertebrates (Crowe et al., 2011; Smedbol et al., 2018), or the proposed safety threshold in guidelines may still impose negative effects on aquatic organisms (e.g., fish) due to the interaction with other multiple stressors (Kelly et al., 2010).
We found significantly higher LL breakdown rates in forest streams than in urban streams in both coarse and fine mesh bags which support our second hypothesis. Differences in the macroinvertebrate communities, physical habitats (e.g., sediment composition), and water quality (e.g., DO) between urban and forest streams may be responsible for these differences (Molinero et al., 1996; Iñiguez-Armijos et al., 2016). Because we found higher macroinvertebrate richness and diversity especially for detritivore abundance and richness, as well as better water quality (e.g., higher DO and lower ammonium-N concentration), in forest streams than urban streams. Richness and abundance of macroinvertebrates in urban streams were usually lower than those in forest streams, especially for shredders, the main contributor to macroinvertebrate-mediated LL breakdown (Iñiguez-Armijos et al., 2016; Tagliaferro et al., 2020). Even though, we found higher abundance of grazing snails (e.g., Viviparidae and Planorbidae) in urban than in forest streams, these snails did not compensate for the loss of detritivore insects for macroinvertebrate-mediated LL decomposition. Probably because most of the snails were found in one single urban stream (S4). The low detritivore richness and abundance in urban streams further resulted in no significant differences in LL breakdown rate between coarse and fine mesh bags. Our result also indicates that macroinvertebrate richness especially collector-gatherer richness is more important than abundance for LL breakdown when shredder abundance and diversity were low (Rosemond et al., 1998; Menéndez et al., 2001; Chadwick et al., 2006). Because most aquatic insects are opportunistic feeders and collector-gatherer may replace the role of shredders in LL decomposition when shredders are scarce (Anderson and Sedell, 1979).
The hypothesized higher microbial-mediated LL breakdown in forest than in urban streams was supported. If the differential in microbial-mediated LL breakdown rates between urban and forest streams were mainly resulted from the differences of nutrient (e.g., ammonium-N) concentrations. Then, LL breakdown rates in urban streams should be higher than those in forest streams because LL breakdown can be stimulated by an intermediate level of eutrophication (Woodward et al., 2012). However, we found a contrary result as LL breakdown rates were higher in forest streams than those in urban streams. Likewise, if LL breakdown rate increased with water temperature as found in other study (Ferreira and Chauvet, 2011), we should have find higher LL breakdown rates in urban streams than in forest streams. Whereas LL breakdown rates in the urban streams which had the highest water temperature were lower than in forest streams. These insignificant effects of nutrient concentrations and water temperature on LL breakdown rate were further evidenced by the results of multiple regression analysis which indicated that DO was responsible for the variation in LL breakdown rate. Forest streams had higher values of DO which was in accordance with faster LL breakdown rates, while the urban streams had lower DO and corresponding to slower LL breakdown rate. Our result support a newly published global-scale study that illustrated positive relationship between DO and LL breakdown rates in streams (Zhang et al., 2019). Taken together, these results may suggest that the positive effect of high nutrient and water temperature on microbial-mediated LL breakdown in urban streams may be overridden by the negative effects of low DO and the poor detritivore richness in these streams (Pascoal and Cássio, 2004).
The responses of LL breakdown rate and macroinvertebrates to carrion subsidy and glyphosate differed between urban and forest streams which support our third hypothesis, and was in accordance with other study (Gardeström et al., 2016). Specifically, the effects of carrion subsidy and glyphosate were stronger in forest than in urban streams. As discussed above, forest streams were dominated by many carrion-consuming insects such as Heptageniidae and Ephemerellidae, which can aggregate on the decomposing carrion subsidy. Whereas the presence of predatory Erpobdellidae attracted by carrion subsidy may decrease the abundance and richness of other macroinvertebrates in urban streams. In addition, the negative effect of glyphosate on LL decomposition only appeared in fine mesh bags in forest streams which support the view that forest streams are more sensitive to stressors because these streams harboring greater proportion of sensitive species (Baumgartner and Robinson, 2015). Global-scale studies also suggested that the response of LL decomposition to nutrient addition was stronger in oligotrophic than eutrophic streams (Woodward et al., 2012). Our results imply that in human-disturbed streams which have a high proportion of tolerant macroinvertebrate and microbial communities are likely to be less affected by stressors such as nutrient pollution (Feckler et al., 2018) while pristine streams may be especially sensitive to stressors.
In conclusion, we found that leaf-litter (LL) breakdown rates were reduced by carrion subsidy in coarse mesh bags in both forest and urban streams. The addition of carrion subsidy increased total and predator richness in forest streams but had no effects on macroinvertebrates in urban streams. Therefore, decreases in the LL breakdown rates were induced by the foraging shift of macroinvertebrates from LL to carrion subsidy in forest streams. Besides, the environmental concentration of glyphosate decreased LL breakdown rates in forest streams but not in urban streams. The interaction between glyphosate and mesh size further implies that glyphosate could negatively affect microbes, decreasing LL breakdown in forest streams. LL breakdown rates were significantly lower in urban streams than in forest streams caused by the differences in water quality and macroinvertebrate communities between the two types of streams. Specifically, we found low LL breakdown rates in urban streams due to the low dissolved oxygen concentration and macroinvertebrate richness. Our results suggest that both carrion subsidy and glyphosate would negatively affect stream ecosystem functioning through the impacts on macroinvertebrates and microbes, respectively. Moreover, forest streams are more sensitive and fragile to natural and anthropogenic stressors such as glyphosate than urban streams.
The original contributions presented in the study are included in the article/Supplementary Material, further inquiries can be directed to the corresponding author.
HX and YZ designed this project and conducted field works. HX analyzed the data and drafted the manuscript. HX and YZ revised the manuscript. DA and RS discussed the project with the group and commented the manuscript.
This work was supported by National Natural Science Foundation of China (Nos 32101310, 32071587), the fellowship of China Postdoctoral Science Foundation (2021M692728), the Development Fund Project of Xi’an Jiaotong-Liverpool University (RDF-15-01-50), Suzhou Water Affair Bureau Research Project (11320500014150085X), and the National Key Research and Development Program of China (Grant No. 2021YFE0200100; China-Portugal Belt and Road Cooperation Laboratory of Cultural Heritage Conservation Science).
The authors declare that the research was conducted in the absence of any commercial or financial relationships that could be construed as a potential conflict of interest.
All claims expressed in this article are solely those of the authors and do not necessarily represent those of their affiliated organizations, or those of the publisher, the editors and the reviewers. Any product that may be evaluated in this article, or claim that may be made by its manufacturer, is not guaranteed or endorsed by the publisher.
We thank editor JG and two reviewers (RR and KC) for their valuable comments and suggestions in improving the quality of this manuscript. We thank Xiaoyan Ni, Ting Zhang, Ying Hua, Xiang Li, Qingyang Lyu, Yuhang Zhang, Danny Hartono, Runze Yu, Wangmingyu Xia, and other XJTLU 2016 undergraduate students for their valuable help in the field and lab work. We would like to thank Xinhua Cao for his kind help during our field work in Huangshan. We thank Zhijie Wu for his help in making the sampling site map.
The Supplementary Material for this article can be found online at: https://www.frontiersin.org/articles/10.3389/fenvs.2022.806340/full#supplementary-material
Anderson, N. H., and Sedell, J. R. (1979). Detritus Processing by Macroinvertebrates in Stream Ecosystems. Annu. Rev. Entomol. 24, 351–377. doi:10.1146/annurev.en.24.010179.002031
Annett, R., Habibi, H. R., and Hontela, A. (2014). Impact of Glyphosate and Glyphosate-Based Herbicides on the Freshwater Environment. J. Appl. Toxicol. 34 (5), 458–479. doi:10.1002/jat.2997
Bärlocher, F. (2020). “Leaf Mass Loss Estimated by the Litter Bag Technique,” in Methods to Study Litter Decomposition: A Practical Guide. Editors F. Bärlocher, M. O. Gessner, and M. A. S. Graça (Cham: Springer International Publishing), 43–51. doi:10.1007/978-3-030-30515-4_6
Barrios, M., and Wolff, M. (2011). Initial Study of Arthropods Succession and Pig Carrion Decomposition in Two Freshwater Ecosystems in the Colombian Andes. Forensic Sci. Int. 212 (1), 164–172. doi:10.1016/j.forsciint.2011.06.008
Baumgartner, S. D., and Robinson, C. T. (2015). Land-use Legacy and the Differential Response of Stream Macroinvertebrates to Multiple Stressors Studied Usingin Situexperimental Mesocosms. Freshw. Biol. 60 (8), 1622–1634. doi:10.1111/fwb.12594
Boyero, L., López-Rojo, N., Tonin, A. M., Pérez, J., Correa-Araneda, F., Pearson, R. G., et al. (2021). Impacts of Detritivore Diversity Loss on Instream Decomposition Are Greatest in the Tropics. Nat. Commun. 12 (1), 3700. doi:10.1038/s41467-021-23930-2
Bretherton, W. D., Kominoski, J. S., Fischer, D. G., and LeRoy, C. J. (2011). Salmon Carcasses Alter Leaf Litter Species Diversity Effects on In-Stream Decomposition. Can. J. Fish. Aquat. Sci. 68 (8), 1495–1506. doi:10.1139/f2011-082
Bricheux, G., Le Moal, G., Hennequin, C., Coffe, G., Donnadieu, F., Portelli, C., et al. (2013). Characterization and Evolution of Natural Aquatic Biofilm Communities Exposed In Vitro to Herbicides. Ecotoxicology Environ. Saf. 88, 126–134. doi:10.1016/j.ecoenv.2012.11.003
Burdon, F. J., Bai, Y., Reyes, M., Tamminen, M., Staudacher, P., Mangold, S., et al. (2020). Stream Microbial Communities and Ecosystem Functioning Show Complex Responses to Multiple Stressors in Wastewater. Glob. Change Biol. 26 (11), 6363–6382. doi:10.1111/gcb.15302
Carles, L., and Artigas, J. (2020). Interaction between Glyphosate and Dissolved Phosphorus on Bacterial and Eukaryotic Communities from River Biofilms. Sci. Total Environ. 719, 137463. doi:10.1016/j.scitotenv.2020.137463
Chadwick, M. A., Dobberfuhl, D. R., Benke, A. C., Huryn, A. D., Suberkropp, K., and Thiele, J. E. (2006). Urbanization Affects Stream Ecosystem Function by Altering Hydrology, Chemistry, and Biotic Richness. Ecol. Appl. 16 (5), 1796–1807. doi:10.1890/1051-0761(2006)016[1796:uasefb]2.0.co;2
Chaloner, D. T., Lamberti, G. A., Cak, A. D., Blair, N. L., and Edwards, R. T. (2007). Inter-annual Variation in Responses of Water Chemistry and Epilithon to Pacific salmon Spawners in an Alaskan Stream. Freshw. Biol 52 (3), 478–490. doi:10.1111/j.1365-2427.2006.01715.x
Chan, E. K. W., Zhang, Y., and Dudgeon, D. (2008). Arthropod 'rain' into Tropical Streams: the Importance of Intact Riparian forest and Influences on Fish Diets. Mar. Freshw. Res. 59, 653–660. doi:10.1071/mf07191
Chan, E. K. W., Zhang, Y., and Dudgeon, D. (2007). Contribution of Adult Aquatic Insects to Riparian Prey Availability along Tropical forest Streams. Mar. Freshw. Res. 58, 725–732. doi:10.1071/mf07033
Claeson, S. M., Li, J. L., Compton, J. E., and Bisson, P. A. (2006). Response of Nutrients, Biofilm, and Benthic Insects to salmon Carcass Addition. Can. J. Fish. Aquat. Sci. 63 (6), 1230–1241. doi:10.1139/f06-029
Cornejo, A., Pérez, J., López-Rojo, N., García, G., Pérez, E., Guerra, A., et al. (2021). Litter Decomposition Can Be Reduced by Pesticide Effects on Detritivores and Decomposers: Implications for Tropical Stream Functioning. Environ. Pollut. 285, 117243. doi:10.1016/j.envpol.2021.117243
Cram, J. M., Kiffney, P. M., Klett, R., and Edmonds, R. L. (2011). Do fall Additions of salmon Carcasses Benefit Food Webs in Experimental Streams? Hydrobiologia 675 (1), 197–209. doi:10.1007/s10750-011-0819-9
Crowe, A. S., Leclerc, N., Struger, J., and Brown, S. (2011). Application of a Glyphosate-Based Herbicide to Phragmites Australis: Impact on Groundwater and Near-Shore lake Water at a beach on Georgian Bay. J. Great Lakes Res. 37 (4), 616–624. doi:10.1016/j.jglr.2011.08.001
Currier, C. M., Chaloner, D. T., Rüegg, J., Tiegs, S. D., D’Amore, D. V., and Lamberti, G. A. (2020). Beyond Nitrogen and Phosphorus Subsidies: Pacific salmon (Oncorhynchus spp.) as Potential Vectors of Micronutrients. Aquat. Sci. 82 (3), 50. doi:10.1007/s00027-020-00725-z
de Mello Cionek, V., Fogaça, F. N. O., Moulton, T. P., Pazianoto, L. H. R., Landgraf, G. O., and Benedito, E. (2021). Influence of Leaf Miners and Environmental Quality on Litter Breakdown in Tropical Headwater Streams. Hydrobiologia 848 (6), 1311–1331. doi:10.1007/s10750-021-04529-6
E. Shaw, L., Mibbayad, A., and Mibbayad, A. (2016). 2,4-D and Glyphosate Affect Aquatic Biofilm Accrual, Gross Primary Production, and Community Respiration. Aims Environ. Sci. 3 (4), 663–672. doi:10.3934/environsci.2016.4.663
Feckler, A., Goedkoop, W., Konschak, M., Bundschuh, R., Kenngott, K. G. J., Schulz, R., et al. (2018). History Matters: Heterotrophic Microbial Community Structure and Function Adapt to Multiple Stressors. Glob. Change Biol. 24 (2), E402–E415. doi:10.1111/gcb.13859
Feng, J. C., Thompson, D. G., and Reynolds, P. E. (1990). Fate of Glyphosate in a Canadian forest Watershed. 1. Aquatic Residues and Off-Target deposit Assessment. J. Agric. Food Chem. 38 (4), 1110–1118. doi:10.1021/jf00094a045
Fenoglio, S., Bo, T., Agosta, P., and Cucco, M. (2005). Mass Loss and Macroinvertebrate Colonisation of Fish Carcasses in Riffles and Pools of a NW Italian Stream. Hydrobiologia 532, 111–122. doi:10.1007/s10750-004-9451-2
Fenoglio, S., Bo, T., Cammarata, M., Malacarne, G., and Del Frate, G. (2010). Contribution of Macro- and Micro-consumers to the Decomposition of Fish Carcasses in Low-Order Streams: an Experimental Study. Hydrobiologia 637 (1), 219–228. doi:10.1007/s10750-009-9998-z
Ferreira, V., and Chauvet, E. (2011). Synergistic Effects of Water Temperature and Dissolved Nutrients on Litter Decomposition and Associated Fungi. Glob. Change Biol. 17 (1), 551–564. doi:10.1111/j.1365-2486.2010.02185.x
Gardeström, J., Ermold, M., Goedkoop, W., and McKie, B. G. (2016). Disturbance History Influences Stressor Impacts: Effects of a Fungicide and Nutrients on Microbial Diversity and Litter Decomposition. Freshw. Biol. 61 (12), 2171–2184. doi:10.1111/fwb.12698
Gessner, M. O., and Chauvet, E. (2002). A Case for Using Litter Breakdown to Assess Functional Stream Integrity. Ecol. Appl. 12 (2), 498–510. doi:10.1890/1051-0761(2002)012[0498:acfulb]2.0.co;2
Giesy, J. P., Dobson, S., and Solomon, K. R. (2000). “Ecotoxicological Risk Assessment for Roundup Herbicide,” in Reviews of Environmental Contamination and Toxicology: Continuation of Residue Reviews. Editor G. W. Ware (New York, NY: Springer New York), 35–120. doi:10.1007/978-1-4612-1156-3_2
Graça, M. A. S. (2001). The Role of Invertebrates on Leaf Litter Decomposition in Streams - a Review. Int. Rev. Hydrobiology 86 (4-5), 383–393. doi:10.1002/1522-2632(200107)86:4/5<383::aid-iroh383>3.0.co;2-d
Haefner, J. N., Wallace, J. R., and Merritt, R. W. (2004). Pig Decomposition in Lotic Aquatic Systems: the Potential Use of Algal Growth in Establishing a Postmortem Submersion Interval (PMSI). J. Forensic Sci. 49 (2), 330–336. doi:10.1520/jfs2003283
Huntsman, B. M., Venarsky, M. P., and Benstead, J. P. (2011). Relating Carrion Breakdown Rates to Ambient Resource Level and Community Structure in Four Cave Stream Ecosystems. J. North Am. Benthological Soc. 30 (4), 882–892. doi:10.1899/10-116.1
Imberger, S. J., Walsh, C. J., and Grace, M. R. (2008). More Microbial Activity, Not Abrasive Flow or Shredder Abundance, Accelerates Breakdown of Labile Leaf Litter in Urban Streams. J. North Am. Benthological Soc. 27 (3), 549–561. doi:10.1899/07-123.1
Iñiguez‐Armijos, C., Rausche, S., Cueva, A., Sánchez‐Rodríguez, A., Espinosa, C., and Breuer, L. (2016). Shifts in Leaf Litter Breakdown along a forest-pasture-urban Gradient in Andean Streams. Ecol. Evol. 6 (14), 4849–4865. doi:10.1002/ece3.2257
Kelly, D. W., Poulin, R., Tompkins, D. M., and Townsend, C. R. (2010). Synergistic Effects of Glyphosate Formulation and Parasite Infection on Fish Malformations and Survival. J. Appl. Ecol. 47 (2), 498–504. doi:10.1111/j.1365-2664.2010.01791.x
Kiffney, P. M., Naman, S. M., Cram, J. M., Liermann, M., and Burrows, D. G. (2018). Multiple Pathways of C and N Incorporation by Consumers across an Experimental Gradient of salmon Carcasses. Ecosphere 9 (4), e02197. doi:10.1002/ecs2.2197
Kolpin, D. W., Thurman, E. M., Lee, E. A., Meyer, M. T., Furlong, E. T., and Glassmeyer, S. T. (2006). Urban Contributions of Glyphosate and its Degradate AMPA to Streams in the United States. Sci. Total Environ. 354 (2), 191–197. doi:10.1016/j.scitotenv.2005.01.028
Kreuger, J. (1998). Pesticides in Stream Water within an Agricultural Catchment in Southern Sweden, 1990-1996. Sci. Total Environ. 216 (3), 227–251. doi:10.1016/S0048-9697(98)00155-7
Lamberti, G. A., Levesque, N. M., Brueseke, M. A., Chaloner, D. T., and Benbow, M. E. (2020). Editorial: Animal Mass Mortalities in Aquatic Ecosystems: How Common and Influential? Front. Ecol. Evol. 8 (343), 602225. doi:10.3389/fevo.2020.602225
Lu, S., Zhai, Z., Zhang, R., Liu, H., and Zhang, J. (2015). Primary Investigation on Glyphosate Contamination in Drinking Water and Source Water in Henan Province. J. Environ. Hyg. 5 (1), 55–57.
Magbanua, F. S., Townsend, C. R., Blackwell, G. L., Phillips, N., and Matthaei, C. D. (2010). Responses of Stream Macroinvertebrates and Ecosystem Function to Conventional, Integrated and Organic Farming. J. Appl. Ecol. 47 (5), 1014–1025. doi:10.1111/j.1365-2664.2010.01859.x
Magbanua, F. S., Townsend, C. R., Hageman, K. J., Lange, K., Lear, G., Lewis, G. D., et al. (2013a). Understanding the Combined Influence of fine Sediment and Glyphosate Herbicide on Stream Periphyton Communities. Water Res. 47 (14), 5110–5120. doi:10.1016/j.watres.2013.05.046
Magbanua, F. S., Townsend, C. R., Hageman, K. J., and Matthaei, C. D. (2013b). Individual and Combined Effects of fine Sediment and the Herbicide Glyphosate on Benthic Macroinvertebrates and Stream Ecosystem Function. Freshw. Biol. 58 (8), 1729–1744. doi:10.1111/fwb.12163
Malmqvist, B., and Rundle, S. (2002). Threats to the Running Water Ecosystems of the World. Envir. Conserv. 29 (2), 134–153. doi:10.1017/S0376892902000097
Mandaville, S. (2002). Benthic Macroinvertebrates in Freshwaters: Taxa Tolerance Values, Metrics, and Protocols. Nova Scotia, A58: Soil &Water Conservation Society of Metro Halifax.
Marks, J. C. (2019). Revisiting the Fates of Dead Leaves that Fall into Streams. Annu. Rev. Ecol. Evol. Syst. 50 (1), 547–568. doi:10.1146/annurev-ecolsys-110218-024755
Menéndez, M., Martinez, M., Hernández, O., and Comín, F. A. (2001). Comparison of Leaf Decomposition in Two Mediterranean Rivers: A Large Eutrophic River and an Oligotrophic Stream (S Catalonia, NE Spain). Int. Rev. Hydrobiology 86 (4-5), 475–486. doi:10.1002/1522-2632(200107)86:4/5<475::aid-iroh475>3.0.co;2-5
Minakawa, N., Gara, R. I., and Honea, J. M. (2002). Increased Individual Growth Rate and Community Biomass of Stream Insects Associated with salmon Carcasses. J. North Am. Benthological Soc. 21 (4), 651–659. doi:10.2307/1468436
Molinero, J., Pozo, J., and Gonzalez, E. (1996). Litter Breakdown in Streams of the Agüera Catchment: Influence of Dissolved Nutrients and Land Use. Freshw. Biol. 36 (3), 745–756. doi:10.1046/j.1365-2427.1996.00125.x
Mollá, S., Casas, J. J., Menéndez, M., Basaguren, A., Casado, C., Descals, E., et al. (2017). Leaf-litter Breakdown as an Indicator of the Impacts by Flow Regulation in Headwater Streams: Responses across Climatic Regions. Ecol. Indicators 73, 11–22. doi:10.1016/j.ecolind.2016.09.005
Okada, E., Allinson, M., Barral, M. P., Clarke, B., and Allinson, G. (2020). Glyphosate and Aminomethylphosphonic Acid (AMPA) Are Commonly Found in Urban Streams and Wetlands of Melbourne, Australia. Water Res. 168, 115139. doi:10.1016/j.watres.2019.115139
Pascoal, C., and Cássio, F. (2004). Contribution of Fungi and Bacteria to Leaf Litter Decomposition in a Polluted River. Appl. Environ. Microbiol. 70 (9), 5266–5273. doi:10.1128/aem.70.9.5266-5273.2004
Pesce, S., Batisson, I., Bardot, C., Fajon, C., Portelli, C., Montuelle, B., et al. (2009). Response of spring and Summer Riverine Microbial Communities Following Glyphosate Exposure. Ecotoxicology Environ. Saf. 72 (7), 1905–1912. doi:10.1016/j.ecoenv.2009.07.004
Piggott, J. J., Townsend, C. R., and Matthaei, C. D. (2015). Reconceptualizing Synergism and Antagonism Among Multiple Stressors. Ecol. Evol. 5 (7), 1538–1547. doi:10.1002/ece3.1465
Puértolas, L., Damásio, J., Barata, C., Soares, A. M. V. M., and Prat, N. (2010). Evaluation of Side-Effects of Glyphosate Mediated Control of Giant Reed (Arundo donax) on the Structure and Function of a Nearby Mediterranean River Ecosystem. Environ. Res. 110 (6), 556–564. doi:10.1016/j.envres.2010.05.004
Quinn, T. P., Helfield, J. M., Austin, C. S., Hovel, R. A., and Bunn, A. G. (2018). A Multidecade experiment Shows that Fertilization by salmon Carcasses Enhanced Tree Growth in the Riparian Zone. Ecology 99 (11), 2433–2441. doi:10.1002/ecy.2453
Relyea, R. A. (2005). The Impact of Insecticides and Herbicides on the Biodiversity and Productivity of Aquatic Communities. Ecol. Appl. 15 (2), 618–627. doi:10.1890/03-5342
Rosemond, A. D., Pringle, C. M., and Ramírez, A. (1998). Macroconsumer Effects on Insect Detritivores and Detritus Processing in a Tropical Stream. Freshw. Biol. 39 (3), 515–523. doi:10.1046/j.1365-2427.1998.00301.x
Rubio-Ríos, J., Pérez, J., Salinas, M. J., Fenoy, E., López-Rojo, N., Boyero, L., et al. (2021). Key Plant Species and Detritivores Drive Diversity Effects on Instream Leaf Litter Decomposition More Than Functional Diversity: A Microcosm Study. Sci. Total Environ. 798, 149266. doi:10.1016/j.scitotenv.2021.149266
Rzymski, P., Klimaszyk, P., Kubacki, T., and Poniedziałek, B. (2013). The Effect of Glyphosate-Based Herbicide on Aquatic Organisms - a Case Study. Limnological Rev. 13 (4), 215–220. doi:10.2478/limre-2013-0024
Schäfer, R. B., von der Ohe, P. C., Rasmussen, J., Kefford, B. J., Beketov, M. A., Schulz, R., et al. (2012). Thresholds for the Effects of Pesticides on Invertebrate Communities and Leaf Breakdown in Stream Ecosystems. Environ. Sci. Technol. 46 (9), 5134–5142. doi:10.1021/es2039882
Smedbol, É., Gomes, M. P., Paquet, S., Labrecque, M., Lepage, L., Lucotte, M., et al. (2018). Effects of Low Concentrations of Glyphosate-Based Herbicide Factor 540 on an Agricultural Stream Freshwater Phytoplankton Community. Chemosphere 192, 133–141. doi:10.1016/j.chemosphere.2017.10.128
Subalusky, A. L., Dutton, C. L., Njoroge, L., Rosi, E. J., and Post, D. M. (2018). Organic Matter and Nutrient Inputs from Large Wildlife Influence Ecosystem Function in the Mara River, Africa. Ecology 99 (11), 2558–2574. doi:10.1002/ecy.2509
Tagliaferro, M., Giorgi, A., Torremorell, A., and Albariño, R. (2020). Urbanisation Reduces Litter Breakdown Rates and Affects Benthic Invertebrate Structure in Pampean Streams. Internat Rev. Hydrobiol 105 (1-2), 33–43. doi:10.1002/iroh.201902000
Talk, A., Kublik, S., Uksa, M., Engel, M., Berghahn, R., Welzl, G., et al. (2016). Effects of Multiple but Low Pesticide Loads on Aquatic Fungal Communities Colonizing Leaf Litter. J. Environ. Sci. 46, 116–125. doi:10.1016/j.jes.2015.11.028
Tiegs, S. D., Peter, F. D., Robinson, C. T., Uehlinger, U., and Gessner, M. O. (2008). Leaf Decomposition and Invertebrate Colonization Responses to Manipulated Litter Quantity in Streams. J. North Am. Benthological Soc. 27 (2), 321–331. doi:10.1899/07-054.1
Vera, M. S., Lagomarsino, L., Sylvester, M., Pérez, G. L., Rodríguez, P., Mugni, H., et al. (2010). New Evidences of Roundup (Glyphosate Formulation) Impact on the Periphyton Community and the Water Quality of Freshwater Ecosystems. Ecotoxicology 19 (4), 710–721. doi:10.1007/s10646-009-0446-7
Verspoor, J. J., Braun, D. C., Stubbs, M. M., and Reynolds, J. D. (2011). Persistent Ecological Effects of a salmon-derived Nutrient Pulse on Stream Invertebrate Communities. Ecosphere 2 (2), art18. doi:10.1890/ES10-00011.1
Wallace, J. B., Eggert, S. L., Meyer, J. L., and Webster, J. R. (1997). Multiple Trophic Levels of a forest Stream Linked to Terrestrial Litter Inputs. Science 277 (5322), 102–104. doi:10.1126/science.277.5322.102
Wallace, J. B., Webster, J. R., and Cuffney, T. F. (1982). Stream Detritus Dynamics: Regulation by Invertebrate Consumers. Oecologia 53 (2), 197–200. doi:10.1007/BF00545663
Wenger, S. J., Subalusky, A. L., and Freeman, M. C. (2019). The Missing Dead: The Lost Role of Animal Remains in Nutrient Cycling in North American Rivers. Food Webs 18, e00106. doi:10.1016/j.fooweb.2018.e00106
Wiederkehr, F., Wilkinson, C. L., Zeng, Y., Yeo, D. C. J., Ewers, R. M., and O'Gorman, E. J. (2020). Urbanisation Affects Ecosystem Functioning More Than Structure in Tropical Streams. Biol. Conservation 249, 108634. doi:10.1016/j.biocon.2020.108634
Wipfli, M. S., Hudson, J., and Caouette, J. (1998). Influence of salmon Carcasses on Stream Productivity: Response of Biofilm and Benthic Macroinvertebrates in southeastern Alaska, U.S.A. Can. J. Fish. Aquat. Sci. 55 (6), 1503–1511. doi:10.1139/f98-031
Woodward, G., Gessner, M. O., Giller, P. S., Gulis, V., Hladyz, S., Lecerf, A., et al. (2012). Continental-scale Effects of Nutrient Pollution on Stream Ecosystem Functioning. Science 336 (6087), 1438–1440. doi:10.1126/science.1219534
Xiang, H. (2019). “The Impacts of Biotic and Abiotic Factors on Resource Subsidy Processes : Leaf Litter Breakdown in Freshwaters,” in PhD Electronic Thesis or Dissertation (University of Liverpool).
Xiang, H., Zhang, Y., Atkinson, D., and Sekar, R. (2020). Effects of Anthropogenic Subsidy and Glyphosate on Macroinvertebrates in Streams. Environ. Sci. Pollut. Res. 27 (17), 21939–21952. doi:10.1007/s11356-020-08505-w
Yanai, S., and Kochi, K. (2005). Effects of salmon Carcasses on Experimental Stream Ecosystems in Hokkaido, Japan. Ecol. Res. 20 (4), 471–480. doi:10.1007/s11284-005-0056-7
Yang, W. (2015). Study on Characteristics and Risk Assessment of Pesticide Non-point Pollution in the Typical Drinking Water of GuizhouMaster Thesis. Guizhou University.
Zhang, M., Cheng, X., Geng, Q., Shi, Z., Luo, Y., and Xu, X. (2019). Leaf Litter Traits Predominantly Control Litter Decomposition in Streams Worldwide. Glob. Ecol Biogeogr 28 (10), 1469–1486. doi:10.1111/geb.12966
Zhang, Y., Negishi, J. N., Richardson, J. S., and Kolodziejczyk, R. (2003). Impacts of marine-derived Nutrients on Stream Ecosystem Functioning. Proc. R. Soc. Lond. B 270 (1529), 2117–2123. doi:10.1098/rspb.2003.2478
Zhang, Y., and Richardson, J. S. (2011). Contrasting Effects of Cross-Ecosystem Subsidies and Predation on Benthic Invertebrates in Two Pacific Coastal Streams. Aquat. Sci. 73 (1), 53–62. doi:10.1007/s00027-010-0159-2
Keywords: ecosystem functioning, herbicide, multiple stressors, macroinvertebrate, land-water interaction
Citation: Xiang H, Zhang Y, Atkinson D and Sekar R (2022) Anthropogenic Carrion Subsidy and Herbicide Glyphosate Depressed Leaf-Litter Breakdown: Effects on Environmental Health in Streams. Front. Environ. Sci. 10:806340. doi: 10.3389/fenvs.2022.806340
Received: 25 November 2021; Accepted: 28 January 2022;
Published: 07 April 2022.
Edited by:
José Francisco Gonçalves Júnior, University of Brasilia, BrazilReviewed by:
Renan De Souza Rezende, Regional Community University of Chapecó, BrazilCopyright © 2022 Xiang, Zhang, Atkinson and Sekar. This is an open-access article distributed under the terms of the Creative Commons Attribution License (CC BY). The use, distribution or reproduction in other forums is permitted, provided the original author(s) and the copyright owner(s) are credited and that the original publication in this journal is cited, in accordance with accepted academic practice. No use, distribution or reproduction is permitted which does not comply with these terms.
*Correspondence: Yixin Zhang, eWl4aW4uemhhbmcyMDE5QHN1ZGEuZWR1LmNu
Disclaimer: All claims expressed in this article are solely those of the authors and do not necessarily represent those of their affiliated organizations, or those of the publisher, the editors and the reviewers. Any product that may be evaluated in this article or claim that may be made by its manufacturer is not guaranteed or endorsed by the publisher.
Research integrity at Frontiers
Learn more about the work of our research integrity team to safeguard the quality of each article we publish.