- Faculty of Agriculture and Environmental Sciences, University of Rostock, Rostock, Germany
Drained peatlands have been rewetted for restoration in Europe and North America for about 25 years. However, information on spatial variability of soil chemical and biochemical properties in long-term drained and restored peatlands is insufficient to design appropriate research methods and soil sampling protocols for monitoring biogeochemical processes. The study aimed to examine the influence of long-term drainage and rewetting of peatlands on smallscale spatial variability of the soil chemical properties and enzyme activities. We collected 400 soil samples from the 0–15 cm and 15–30 cm soil depths of a drained and a corresponding rewetted peatland. The number of grid cells was 100 for each of the drained and the rewetted peatland, and the size of each grid cell was 3 m × 3 m. We analyzed 17 soil parameters from the surfaces and 14 from the subsurface of both sites. The variability (range, SD, and CV) of all the soil properties was higher in the drained peatland than in the restored peatlands except for the soil pH. The geostatistical analysis revealed only the soil pH, acid phosphatase, β-glucosidase, and arylsulfatase activities disclosed the strong spatial dependency at the ≤5 m semivariance range in the drained peatland. However, more than 80% of the soil properties showed a strong spatial dependence within the 4–20 m semivariance ranges in the restored peatland. The strong spatial dependencies of all the soil properties in the long-term restored peatland conclusively call for the spatial soil sampling and geostatistical data analysis methods to capture substantial spatial variability that has important implications in degraded peatland restoration.
Introduction
Different literature showed that 50%–75% of peatlands had been drained for various land use purposes worldwide since 1900 (Clarkson et al., 2013; Bess et al., 2014; Lamers et al., 2015; Chimner et al., 2017). As a result, peatland drainage has contributed to losing ecosystems services such as regulating water quality and supply, carbon storage, biodiversity, recreation, knowledge archives, cultural heritage, flood-water regulation, sediments, and nutrients trapping (Clarkson et al., 2013; Tomscha et al., 2021). Restoring of degraded and rewetting of drained peatlands have been practiced for the last 25 years in North America and Europe, where 85% of the 4 million km2 global peatlands are currently located (Lamers et al., 2015; Chimner et al., 2017; Andersen et al., 2017; Peters and von Unger, 2017). The initial phase of peatland restoration is different from region to region based on the land-use systems contributing to peatland degradation such as mining, road constructions, erosion, afforestation, timber production, agriculture, and peat cutting (Bess et al., 2014; Chimner et al., 2017). The standard peatland restoration techniques are drain blocking, restoring cutaway peat, transplanting peat-forming plants on bare peat, constructing dams from different materials, and reprofiling (Cooper and MacDonald, 2000; Rochefort et al., 2003; Cobbaert et al., 2004; Lamers et al., 2015; Chimner et al., 2017). Since the significant land uses contributing to drainage and degradation of peatlands in most European countries have been agriculture (Lamers et al., 2015; Andersen et al., 2017), the large-scale peatland restoration has been practiced by drain-blocking (Armstrong et al., 2009; Urzainki et al., 2020).
Several studies have investigated the impacts of rewetting drained and degraded peatlands on freshwater eutrophication (Zak et al., 2008; Forsmann and Kjaergaard, 2014), greenhouse gas emissions (Hahn et al., 2015; Kandel et al., 2019), ecological successions (Haapalehto et al., 2017; Renou-Wilson et al., 2019), nutrient cycles (Wang and Moore, 2014; Schillereff et al., 2016; Berger et al., 2017; Nieminen et al., 2017; Dietrich and MacKenzie, 2018), soil chemistry (Heller and Zeitz, 2012; Duval and Radu, 2018; Negassa et al., 2019a), and enzymes activities (Song et al., 2019; Zhao et al., 2019). These previous studies were conducted on peatlands after one to 3 years of rewetting, and the spatial soil sampling method was not used.
High soil spatial variability can influence plant species recolonization, carbon sequestration, and eutrophication during the restoration of degraded peatlands (Larkin, 2016). Therefore, sustainable peatland management practices require detailed soil properties information that influences biogeochemical processes. Knowledge about the spatial distribution of soil properties could also help to understand the influence of long-term drainage and rewetting peatlands on various ecosystem services and functions (Arrouays et al., 2014).)
Drainage and rewetting peatlands can affect surface micro-elevations, biogeochemical processes, and distribution of vegetation types (Richert et al., 2000; Limpens et al., 2008). Such influences are eminent in agriculturally used fens that potentially can result in spatial heterogeneity of soil properties. Drainage and rewetting result in subsidence and expansion of peatlands, respectively, and that could influence spatial variability of soil chemical and biochemical properties differently in such contrasting management practices (Camporese et al., 2006; Nijp et al., 2019; Jurasinski et al., 2020). Although drainage and cultivation can homogenize surface and subsurface soil horizons, rewetting has a high potential to increase the heterogeneity of surface soils because of groundwater dynamics, sedimentation depositions from the surrounding landscapes, and occasional flooding by nearby freshwater resources (Leifeld et al., 2011; Ahmad et al., 2020a; Ahmad et al., 2020b). As a result, rewetting can enhance existing variations in microclimates that influence important biogeochemical processes such as increasing anoxic conditions, decreasing greenhouse emissions (Girkin et al., 2019; slowing plant nutrient cycles (Dietrich and MacKenzie, 2018), changing microbial community structure (Groß-Schmölders et al., 2021), and biochemical properties (Negassa et al., 2019b). Long-term rewetting is also one of the strategies to restoring degraded peatlands by reestablishing peat-forming communities that also change biogeochemical processes compared to drained peatlands used for crop and feed productions (Zerbe et al., 2013).
Several studies have been conducted to understand wetland biogeochemistry, and methods have been developed to study biogeochemistry processes in wetland ecosystems as compiled in different books (Reddy and DeLaune, 2008; DeLaune et al., 2013; Schlesinger and Bernhardt, 2020). In these cited four books, the wetlands biogeochemistry and research methods are described in detail; however, spatial variability of the different soil properties in various wetland types has not been addressed except the soil sampling methods in wetland ecosystems (Casado et al., 2013; Osborne and DeLaune, 2013). Although more than 100 papers have been published on mineral soil spatial variability annually (Wang et al., 2021), only a few studies have been reported on peatland and wetland soils for the last 2 decades. These studies include the effect of long-term peatland rewetting on spatial variability of hydrophysical soil properties (Ahmad et al., 2020a) and denitrification in short-term (three to six yrs) rewetted, drained, and constructed peatlands (Bruland et al., 2006), and soil pH, soil organic matter (SOM), and total phosphorus (P) in natural and anthropogenic impacted wetlands (Cohen et al., 2008). Similarly, studies also investigated the spatial variability of greenhouse gas emissions (Girkin et al., 2019) and P in a south Florida wetland (Grunwald et al., 2004). More than 913 km2 of drained peatlands have been rewetted in Western Europe between 1993 and 2015 (Andersen et al., 2017); however, only the results of a study is available on the spatial variability of soil chemical and biochemical properties in the long-term rewetted peatlands (Negassa et al., 2019b). Thus, understanding the influence of long-term drainage and rewetting on spatial variability of soil chemical and biochemical properties could help design appropriate soil sampling methods for monitoring biogeochemical processes in restored peatlands.
There are classic (designed-based) and geostatistical (model-based) soil sampling methods that can be used based on the spatial variability of the soil properties under consideration (Webster and Oliver, 2007; Casado et al., 2013; Lawrence et al., 2020). The classic soil sampling method involves subsampling, and compositing into a single sample could overestimate or underestimate the effect of soil management practices on a value of soil property, provided that the spatial variability of a soil property is strongly dependent. Thus, the classic soil sampling method could be suitable when the soil properties are spatially independent. On the other hand, geostatistical soil sampling can increase precision in determining soil properties compared to classic soil sampling because of the spatial correlation values (Hergert et al., 1995). Furthermore, geostatistical analyses quantify spatial correlations between observations to reproduce spatial processes’ behavior across the entire domain of interest (Montero et al., 2015). Geostatistical studies also provide estimates of semivariogram sill and range that could provide information about sample size and the minimum distance required for soil sampling intervals to be considered spatially independent, respectively (Loescher et al., 2014). Suppose the heterogeneity of the soil properties is spatially independent; the classic randomization sampling method with fewer composite samples can be sufficient compared to the number of soil samples required for spatially dependent soil parameters (Lawrence et al., 2020). Thus, knowing the spatial structure and variability of soil properties is essential to use appropriate soil sampling methods for monitoring soil chemical and biochemical properties in drained and rewetted peatlands to monitor the restoration success of degraded peatlands (Kibblewhite et al., 2012).
Advancement in geostatistical and soil mapping tools for precision agriculture, environmental management has increased the study of spatial heterogeneity of soil properties (Trangmar et al., 1985; Si et al., 2007). The approach helps to determine whether the heterogeneity of soil properties is changing randomly or systematically from a sampling point to the next for better experimental design, sampling methods, statistical analysis, and interpretation (Biswas and Si, 2011; Shit et al., 2016; Lawrence et al., 2020). The conventional soil sampling method and standard statistical analysis, such as analysis of variance, assume soil samples are spatially independent. However, soil properties are not always spatially independent. The geospatial analysis can identify whether the heterogeneity of soil properties changes randomly or systematically among the sampling points. Almost all previous studies investigated the impact of drainage and rewetting peatlands on soil chemical and biochemical properties with the classic design-based soil sampling method. Applying the designed-based soil sampling methods for spatially dependent soil properties could provide misleading results that can negatively influence the restoration of degraded peatlands by various stakeholders. Thus, this study aimed to examine the smallscale spatial variability of selected soil chemical and biochemical properties in long-term drained and rewetted peatlands of northeastern Germany. We hypothesize that soil chemical and biochemical properties are spatially dependent in long-term rewetted fen peatland than in the long-term drained fen peatland. The rewetted peatland has been influenced by patchy and diverse vegetations, microrelief, groundwater, and surface water that together may contribute to systematically change soil chemical and biochemical properties from the point of sampling to the next compared to the uniformly managed grassland of the drained peatland.
Materials and Methods
Study Sites and Soil Sampling
The drained and rewetted peatlands are located in the Trebel and Recknitz valleys of the Federal State of Mecklenburg-Western Pomerania, northeastern Germany (Figure 1A). Jurasinski et al. (2020) and Weil et al. (2020) gave detailed information on historical land use, elevation, and vegetation types. Hydrological reconstructions started in the 1960s in the Recknitz and Trebel valleys to intensify the use of the peatlands for grassland, silage, and hay production. The georeference (N, E) and altitude of the drained peatland are 54.1316, 12.6289, and 2.25 m, and that of the rewetted peatland is 54.1011, 12.7395, and 1.25 m. In the early 1990s, a peatland in the Trebel valley was rewetted by the EU-LIFE program that has been put under nature conservation. However, the drained peatland was not part of the rewetted peatland that had been used for pasture production when the site was sampled for the present study. The drained peatland was covered by uniform grassland, mainly consisting of Ranunculus repens and Deschampsia cespitosa with some Holcus lanatus and Poa trivialis. The rewetted peatland was covered by Carex acutiformis, Epilobium hirsutum, Deschampsia caespitosa, Carex acutiformis, Juncus subnodulosus, and Phalaris arundinacea. The humification degree of both sites’ peats indicated highly decomposed fen peat (Negassa et al., 2019a). According to the International Union of Soil Science Working Group World Reference for Soil Resource, the major soil units of both study sites were Sapric Histosols.
Before collecting the soil samples, plots with the size of 30 m × 30 m (900 m2) with similar topography and vegetation were divided into 100 small grid cells of 3 m × 3 m size for each site with string (Figures 1B,C). The litter from the center of each grid cell was removed using a spade and marked. The georeferenced data were taken using a geographical positioning system from each marked point before sampling. The soil samples were collected from the center of each grid cell using a peat sampler (Eijkelkamp Soil & Water, Giesbeek, Netherlands). The number of soil samples collected from each peatland was 200 (100 from the surfaces and 100 from subsurface subsurfaces) in summer 2018. We included subsurface horizons sampling since soluble and solid soil materials can transfer between the surface and subsurface horizons, affecting the spatial variability of soil properties. The spatial pattern of micro-elevations of the drained and rewetted peatland sampling points were calculated (Figure 2).
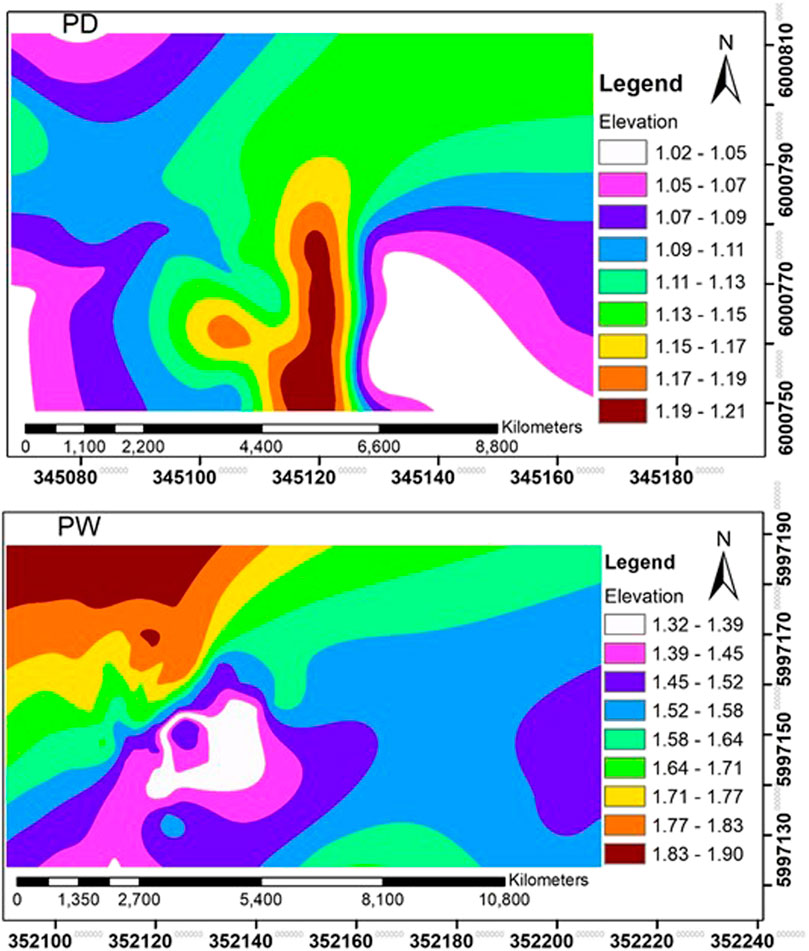
FIGURE 2. Spatial patterns of elevations of the drained (PD) and rewetted (PW) peatlands of the soil sampling points elevation: m.
Analysis of Soil Chemical Properties and Enzyme Activities
The 0.01 M CaCl2 solution was used to determine soil pH at a soil-to-solution ratio of 1: 2.5 at room temperature (VDLUFA, 2016). We estimated the soil organic matter (SOM) concentration by the loss-on-ignition method, where the oven-dried soil samples were ignited at 550°C for 4 hours (Nelson and Sommers, 1996). The total P, potassium (K), calcium (Ca), magnesium (Mg), iron (Fe), manganese (Mn), zinc (Zn), and aluminum (Al) concentrations were measured by the inductively coupled plasma-optical emission spectroscopy (ICP-OES) (PerkinElmer Optima 8300, Waltham, MA, USA) after microwave-assisted acid digestion (US EPA, 2007). We used the double lactate (DL) method to extract the plant-available P, K, and Mg (VDLUFA, 2012) and the detailed DL extraction procedures already published elsewhere (Negassa et al., 2020). The concentrations of the plant available nutrients were determined by the ICP-OES method. The soil enzyme activities involved in the C, P, and S cycles were determined from the surface horizons of the drained and rewetted peatlands. The soil enzyme activities such as the acid phosphatase were measured using the photometric incubation methods of Tabatabai and Bremner (1969) for analyses of the acid phosphomonoesterase activity (acid phosphatase), of Eivazi and Tabatabai (1988) for analysis of β-glucosidase activity, and of Tabatabai and Bremner (1970) for the analysis of arylsulfatase activity. In all these photometric tests, the color intensity of p-nitrophenol released from a pre-given enzyme-specific substrate during incubation at 37°C, and the solution was measured within an hour. Overall, we analyzed 17 and 14 soil properties from the surface and subsurface horizons of the drained and rewetted peatlands. We did not determine enzyme activities from the subsurface horizons of drained and rewetted peatlands since the enzyme activities lacked spatial variability in our previous study (Negassa et al., 2019b).
Statistical Analysis
We ran the descriptive statistics of 17 soil properties using the SAS 9.4 software. The mean, median, minimum, maximum, standard deviation (SD), and coefficient of variation (CV) were computed for each soil property. We also ran the principal component analysis (PCA) by PROC FACTOR in SAS software for the soil parameters. The PCA was computed for the total elements, available plant nutrients, and enzyme activities. Geostatistical software GS+TM version 7 (Gamma Design Software, Plainwell, Michigan, USA) was used to analyze the spatial dependence of the individual soil properties considered in the present study and define the semivariograms. Detailed information about the semivariogram and interpretation was provided elsewhere (Berry, 2005; Webster and Oliver, 2007). In brief, we computed the semivariogram by fitting values of the different soil properties to the semivariogram models. These models are linear, exponential, spherical, and Gaussian models and the best fit models to the values of each soil property were selected based on the lowest value of the residual sum of square (RSS). These models comprise nugget, sill, and range except for the linear model, which has only nugget. The nugget to sill ratio for the linear model is 1.0 since the nugget is equal to sill. Accordingly, when the nugget to sill ratio of a soil parameter is: >0.75 spatially independent, from 0.25 to 0.75 moderately dependent, and <0.25 strongly spatially dependent (Cambardella et al., 1994).
Results
Descriptive Statistics of Soil Chemical Properties and Enzyme Activities
The summary of the descriptive statistics of soil pH and SOM concentration are presented in Table 1.
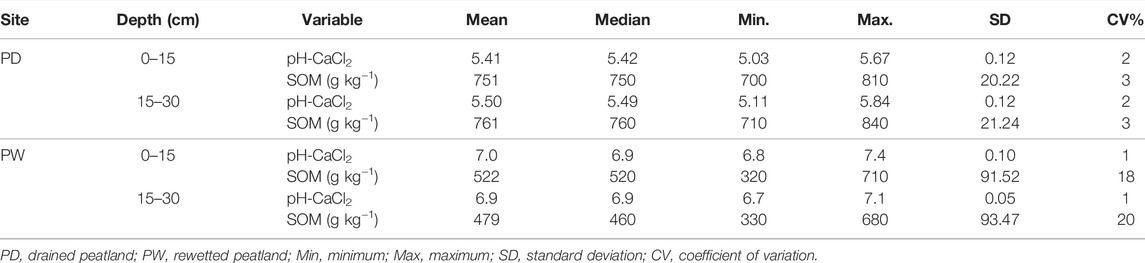
TABLE 1. The soil pH and organic matter concentrations in the surface and subsurface horizons of the drained and rewetted peatlands.
The soil pH and SOM concentration were higher at 0–15 cm than at 15–30 cm soil depths of the rewetted peatland, and the soil properties were slightly lower at the 0–15 cm soil depths than at the 15–30 cm of the drained peatland. The soil pH in the rewetted peatland was higher than in the drained peatland. However, the SOM concentration of the rewetted peatland was lower than in the drained peatland. The measure of the variability (range = Max-Min, standard deviation (SD), and coefficient of variation (CV)) of the soil pH and SOM was similar in both the surface and subsurface horizons of the drained peatland and soil pH of the rewetted peatland. However, the variability of the SOM concentration in both soil horizons of the rewetted peatland was substantially higher than in the drained peatland. Accordingly, the SOM range, SD, and CV in the rewetted peatland were higher than those of the drained peatland by 3, 4.6, and 6 times, respectively.
The summary of the descriptive statistics of the total P, K, Ca, Mg, Fe Mn, Zn, and Al concentrations in the surface and subsurface horizons of the drained and rewetted peatlands are presented in Table 2.
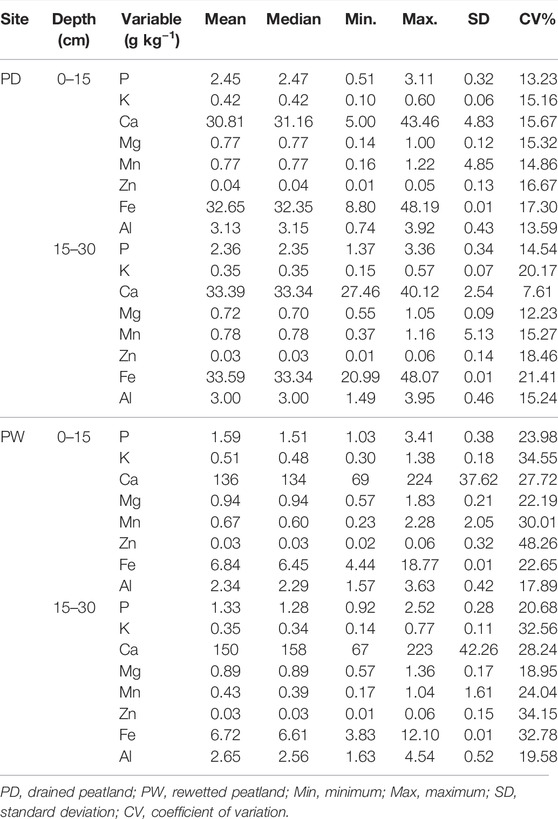
TABLE 2. The concentrations of total elements of in the surface and subsurface horizons of the drained and rewetted peatlands.
The total P, K, Ca, Mg, Fe Mn, Zn, and Al concentrations at the surface horizons of the drained and rewetted peatlands were similar to their subsurface horizons. The concentrations of these elements were also similar between the drained and rewetted peatlands except for the total Fe and Ca concentrations. However, the variability of most total elements was similar between the surface and subsurface horizons of the drained peatlands; however, the variability of most total elements in the surface horizon was higher than in the subsurface horizons of the rewetted peatland. Although the concentrations of the elements were more variable in both surface and subsurface of the rewetted peatlands, their variability was more pronounced in the surface horizons of the rewetted peatlands.
The PCA of SOM and the total elements concentrations of the surface and subsurface soil horizons also further confirmed the differences in variability between the soil horizons and the drained and rewetted peatlands (Figure 3). The PCA clearly showed these parameters clustered together in the drained peatland than in the rewetted peatland, particularly at the surface horizons. The PC1 and PC2 accounted for 53 and 16% of the total variance, respectively. Overall, among the parameters considered in this PCA, each of the total P, Fe, SOM, Ca, Fe, and Mn contributed >0.6 loadings to the PC1, whereas each of the total K, Mg, and Zn contributed to >0.6 loading to the PC2.
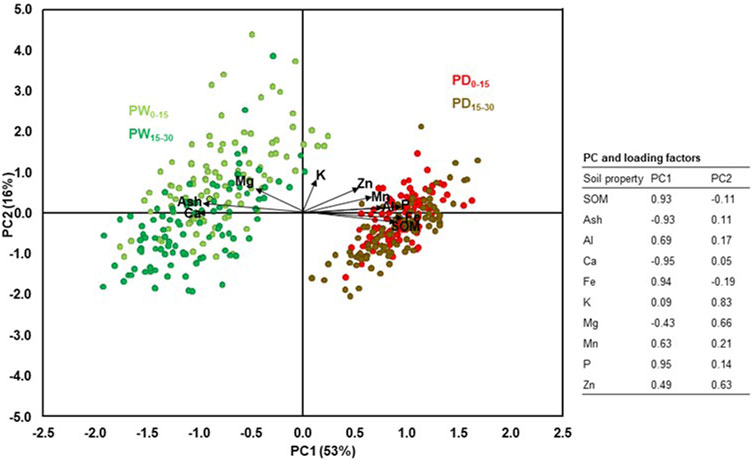
FIGURE 3. PCA scatter plot of SOM and concentrations of total elements in surface and subsurface horizons of the drained and rewetted peatlands. PD0-15: surface horizons of drained peatland, PD15-30: subsurface horizons of the drained peatland, PW0-15: surface horizons of rewetted peatland, PW15-30: subsurface horizons of the rewetted peatland.
The plant-available P, K, and Mg concentrations were similar between the surface and subsurface horizons of the drained peatland; however, these plant nutrient concentrations differed between the depths of the rewetted peatland (Table 3). Although the plant-available P concentration of the rewetted and drained peatlands was similar at the surface, the plant-available P in the surface of the rewetted peatland was higher than in its subsurface by four times. The plant-available K and Mg concentrations in both depths of the drained peatland were also similar. However, the plant-available Mg in the depths of the drained peatland was higher than that of the rewetted peatland. In addition, the plant-available K at the surface of the rewetted peatland was higher than in its subsurface by four times. The variability of plant-available P, K, and Mg concentrations was similar between the surface and subsurface horizons of the drained peatland; however, the plant nutrients were more variable in the surface than the subsurface horizons of the rewetted peatland. Variability of the plant nutrients in the drained peatland was similar to that of the subsurface horizon of the rewetted peatland. Overall, the variability of the plant nutrients in the surface horizons of the rewetted peatlands was higher than in its subsurface horizon and both depths of the drained peatland. The CVs of the plant nutrients in the drained and rewetted peatlands ranged from 20 to 28% and 25%–43%, respectively.
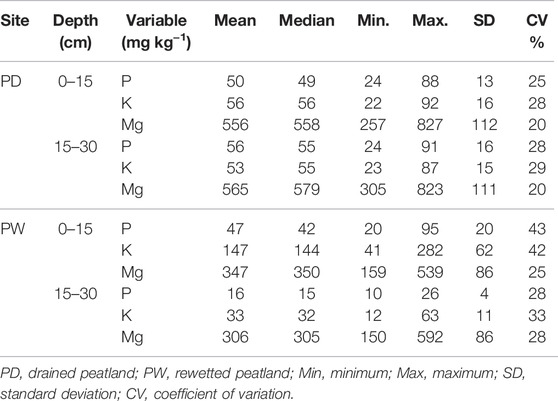
TABLE 3. Plant available phosphorus, potassium, and magnesium in the surface and subsurface horizons of the drained and rewetted peatlands.
The acid phosphatase, β-glucosidase, and arylsulfatase activities differed between the contrasting management practices (Table 4). The phosphatase activity in the rewetted peatland was lower than in the drained peatland, whereas the β-glucosidase and arylsulfatase activities were higher in the rewetted than in the drained peatland by two and three times, respectively. Nevertheless, the influence of the drainage and rewetting peatlands on the variability of the enzyme activities was not consistent. The phosphatase activity was less variable in the drained peatland than in the rewetted peatland, whereas the variability of the β-glucosidase and arylsulfatase activities were higher in the drained than in the rewetted peatland. Regardless of differences in enzyme activities and variability between the drained and rewetted peatlands, the variability of the enzyme activities in both drained and rewetted peatlands was high, and the CVs ranged from 21 to 39%. Overall, the variability of most soil properties in the surface and subsurface horizons of the drained peatlands were similar and less variable than the soil properties of the rewetted peatland. In addition, the variability of almost all soil properties in the surface horizon was higher than in the subsurface horizons of the rewetted peatland.
The PCA of the plant-available nutrients and enzyme activities of the drained and rewetted peatlands (Figure 4) also confirmed the variability of the plant nutrients and enzyme activity (Tables 3, 4). Like their variability, the distributions of the plant nutrients and enzyme activities were slightly more dispersed in the rewetted than in the drained peatlands. The PC1 accounted for 48% of the total variance, whereas the PC2 accounted for 22%.
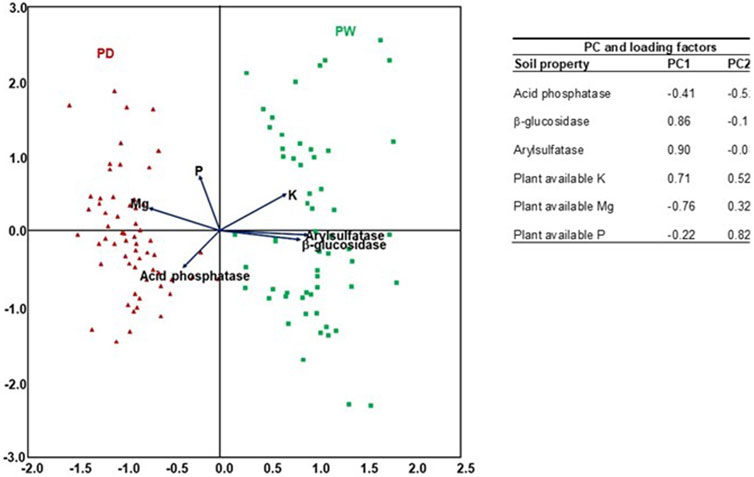
FIGURE 4. PCA scatter plot of plant available nutrient concentration and enzyme activity in the surface horizons of the drained and rewetted peatlands. PD: drained peatland, PW: rewetted peatland.
Spatial Variability of Soil Chemical Properties and Enzyme Activities
The result of geostatistical analysis of the soil pH and SOM of the drained and rewetted peatlands is presented in Table 5. The results revealed that the soil pH was strongly spatially dependent, whereas the SOM was spatially independent in the drained peatland. On the other hand, both soil parameters were strongly spatially dependent in the rewetted peatland. The spatial dependence appeared within the 4–6 m semivariogram range for the soil pH in both drained and rewetted peatlands, whereas the SOM was spatially dependent at both horizons of the rewetted peatland within 19 and 22 m semivariogram ranges, respectively.
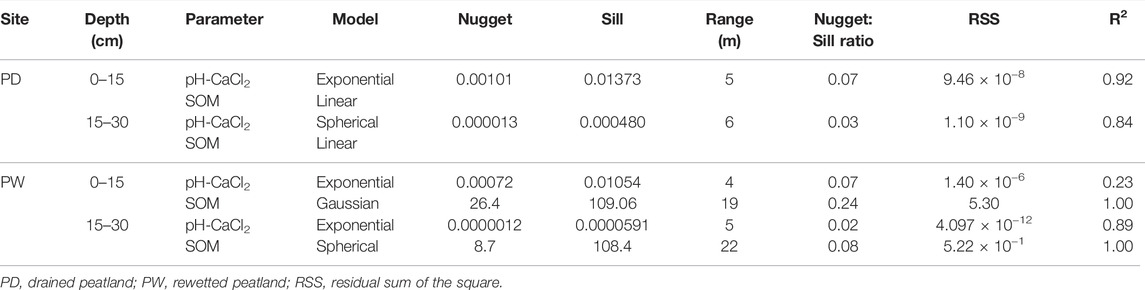
TABLE 5. Spatial dependence of soil pH and organic matter in the surface and subsurface horizons of the rewetted and drained peatlands.
The total elements (K, P, Ca, Mn, Mg, Zn, Fe, and Al) in the drained peatland were spatially independent (data not shown). However, all the elements were spatially dependent in the rewetted peatland (Table 6). Most elements exhibited strongly and moderately spatial dependencies in the surface and subsurface soil horizons of the rewetted peatland, respectively. The semivariogram ranges of most total elements in the surface and subsurface soil horizons of the rewetted peatland ranged from 5 to 10 m and 16–29 m, respectively.
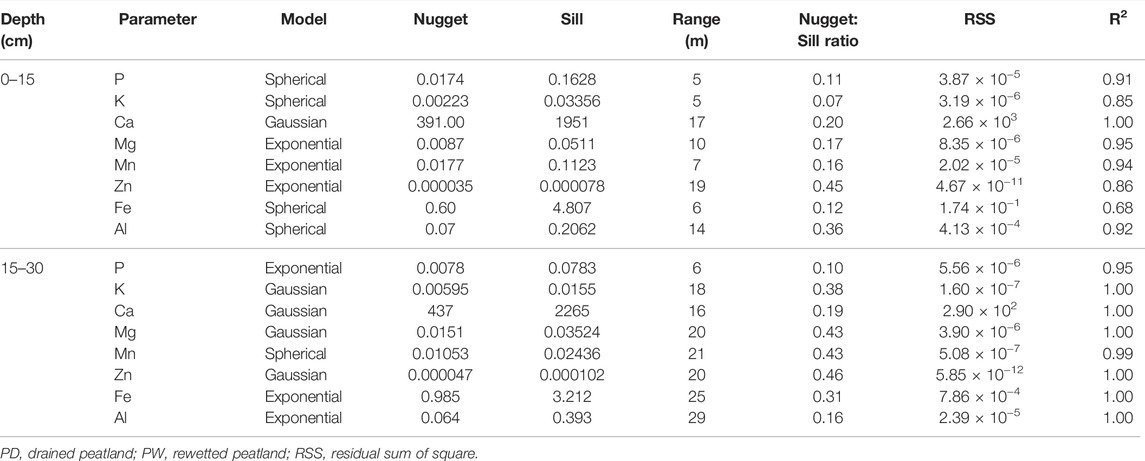
TABLE 6. Spatial dependence of total concentrations of selected elements in the surface and subsurface horizons of the rewetted peatland.
The plant-available P and Mg were spatially independent in the surface and subsurface horizons of the drained peatland; however, the plant-available K in the drained peatland and the three plant-available nutrients in the rewetted peatland were spatially dependent in the rewetted peatlands (Table 7). Similar to the total elements (Table 6), the spatial dependencies of the plant available nutrients were strong and moderate in the surface and subsurface horizons of the rewetted peatland, respectively. The semivariogram ranges were 4–12 m and 19–103 m for the strong and moderate spatial dependencies of the plant-available nutrients, respectively.
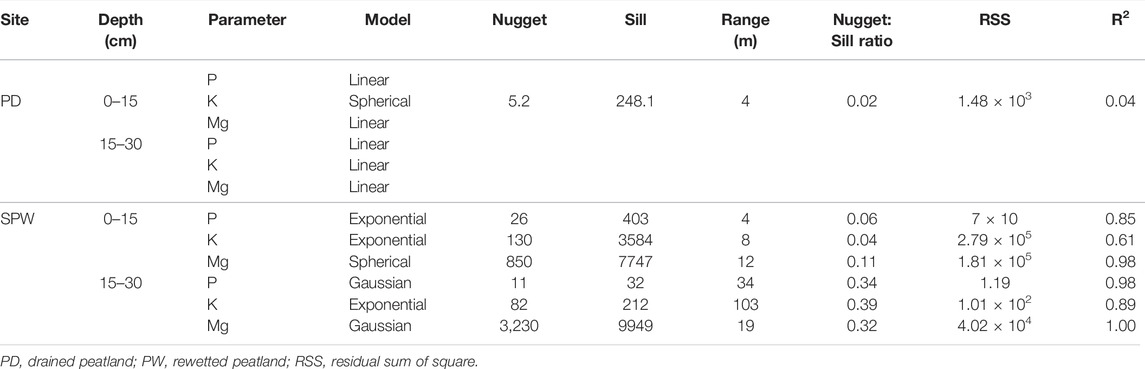
TABLE 7. Spatial dependence of plant available nutrients in the surface and subsurface horizons of the rewetted and drained peatlands.
Similar to their variability (Table 4), the spatial variability of the enzyme activities of the drained and rewetted peatlands were slightly different (Table 8). Accordingly, the three enzyme activities, phosphatase, β-glucosidase, and arylsulfatase were strongly spatially dependent in the drained and rewetted peatlands. However, the semivariogram ranges of the drained and rewetted peatlands were slightly different for the enzyme activities that were 5 m for the drained peatland and ranged from 5 to 9 m for the rewetted peatland.
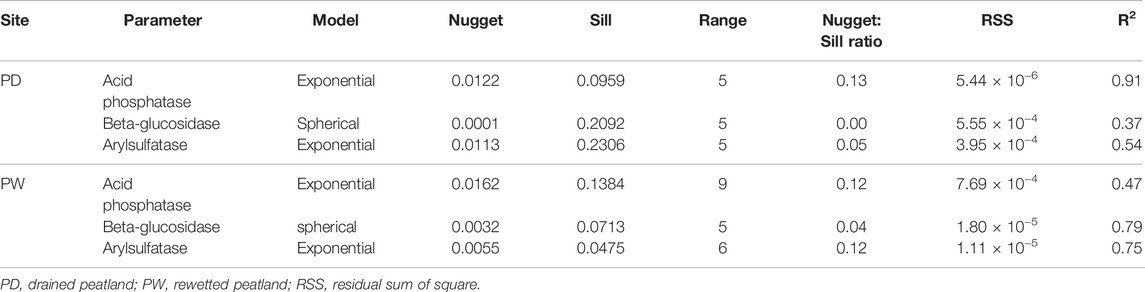
TABLE 8. Spatial dependence of soil enzyme activity in the surface and subsurface horizons of the rewetted and drained peatlands.
Discussion
Descriptive Statistics of Soil Properties
The higher soil pH in the surface horizons of the rewetted peatland (Table 1) can be explained by transported and settled inorganic sediment containing more Ca-containing minerals like calcite than the SOM-dominated drained peatland (Table 2). The higher Ca concentration in the rewetted than in the drained peatlands concurred with the higher soil pH, while the higher SOM concentration lowered the soil pH in the drained peatland (Tables 1, 2). The availability of fresh Ca-containing mineral sediments transported and deposited during the rewetting may also contribute to the higher soil pH (Slessarev et al., 2016). Previous studies also reported that rewetting wetland increased soil pH, while drainage decreased soil pH because of reduction and oxidation of S compounds and the predominance of pH-dependent charges in organic soils (Burton et al., 2011; Lundin et al., 2017). The lower SOM concentration of the rewetted peatland than the drained peatland (Table 1) can be attributed to the occasional deposition of the inorganic sediment materials from the surrounding landscape by erosion and nearby Trebel River by flooding. This is a typical phenomenon in minerotrophic fen peatlands (Leifeld et al., 2011). The rewetted peatland’s topographic position facilitates the deposition of inorganic sediments by soil erosion and flooding for the last 20 years, decreasing the relative proportion of SOM. The inorganic sediments also can facilitate aeration and increase electron acceptors, enhancing peat decomposition and increasing the proportion of the mineral fraction (Grønlund et al., 2008; Sutton-Grier et al., 2011). There is no question: rewetting increases peat accumulation, thereby SOM, but when the inorganic sediments deposition is greater than the peat accumulation, it masks the rewetting role in peat accumulation. In contrast, plant-derived phenolics and aromatic compounds could prevent substantial SOM loss in the drained peatland (Hodgkins et al., 2018) that had no chance to receive inorganic sediment materials since network drainage ditches stopped flooding from the surrounding landscapes.
The high total P concentration in the drained peatland (Table 2) could be related to the anthropogenic P loading. The drained peatland has received P fertilizer for hay production for the last two decades compared to the rewetted peatlands (Jurasinski et al., 2020). Nevertheless, the total P concentrations in both drained and rewetted peatlands were higher than the total P concentration of peatlands that anthropogenic factors have not influenced, ranging from 0.03 to 0.5 g kg−1 (Richardson and Reddy, 2013). The differences in total Fe concentration between the rewetted and the drained peatland (Table 2) could be associated with the differences in their redox conditions, soil pH, and deposition of inorganic sediments (Reddy and DeLaune, 2008). According to the total Fe concentration, the rewetted and drained peatlands can be categorized as Fe poor and Fe rich peatlands, respectively (Emsens et al., 2016). Although Fe-rich peatlands are regarded as beneficial for phosphate and sulfate sorption (Geurts et al., 2008), the result of another study claimed that high total Fe concentration (>14 g kg−1) could enhance SOM mineralization in rewetted peatlands (Emsens et al., 2016). These fragmented findings call for systematically designed long-term experiments since responses of some soil chemical properties to management practices are transitory.
Exceptionally high Ca concentration in the rewetted peatland (Table 2) unequivocally disclosed that Ca-bearing inorganic sediments had been imported from the surrounding landscape and overland flows (Chesworth, 2008). The drained and rewetted peatlands of the present study sites formed after the last glacial period in meltwater channels left by the retreating ice where rising sea levels during the Littorina transgression flooded the valleys and started depositing sediments, peat, and other detritus (Jurasinski et al., 2020); however, there were no influences of sediments and erosion from the surrounding landscape on the drained peatland because of the presence of deep ditch for decades. As a result, the total Ca concentration in the drained peatland was comparable with the one reported from the drained forest peatlands of Estonia (Kõlli et al., 2010). Although the differences in total Mg concentration between the drained and rewetted peatland were negligible in the present study, all factors influencing total Ca concentration in soils, such as leaching, mineral type, and plant uptake, affect the Mg status in soils (Merhaut, 2007).
The low K concentration in both drained and rewetted peatlands is explained by K leaching from partially decomposed peat since K is not incorporated into plants’ organic compounds (Weil and Brady, 2017). Both K and Mg were slightly higher in the rewetted than the drained peatlands, which is explained by the deposition of inorganic sediment in the former with surface water used for rewetting. The total Mn, Al, and Zn concentrations (Table 2) were in a range that was reported from mineral soils (Fageria et al., 2002; Storey, 2007; Reddy and DeLaune, 2008). Their lack of anthropogenic loadings can describe the similarity of the concentrations of these elements in the drained and rewetted peatlands.
The concentration of plant-available P in the drained and rewetted peatlands (Table 3) was higher than that of European fen peatlands that mostly ranged from 10 to 28 mg kg−1 in the surface and subsurface horizons (Schlichting et al., 2002). The concentration of plant available P in both soil depths of the drained and rewetted peatlands ranged from suboptimal to optimal concentrations according to soil P fertility classification for grassland use at peat soils in Northern Germany (Ministerium für Landwirtschaft und Naturschutz Mecklenburg-Vorpommern, 1998; Taube et al., 2015; VDLUFA, 2018). Similarly, the plant-available Mg concentration was higher than the optimum Mg concentration required for plant growth (Gerendás and Führs, 2013). However, the plant-available K indicates the deficiency range (Kuchenbuch and Buczko, 2011) except for the surface horizon of the rewetted peatland. In most horizons, the low plant-available K can be attributed to labile K most likely removed by leaching and plant uptake and temporal storage in the vegetation.
The phosphatase activity was similar (Table 4) to the one reported from the peat samples of the Trebel Valley about 20 years ago (Baum et al., 2003) and higher than in the rewetted peatland of Warnow Valley near Rostock/Germany (Negassa et al., 2019b). However, the β-glucosidase and the arylsulfatase activities in the present study were lower than those reported from boreal peatlands and Everglades wetland soils, respectively (Wright and Reddy, 2001; Song et al., 2019). This might be caused by the very dry vegetation period in the year of sampling (2018), with about half of the precipitation compared to the long average value at the sampling site. The differences in the enzyme activities among the various studies and sites can furthermore be associated with variations in soil pH, labile organic compounds, plant nutrients, current vegetation cover, and SOM (Penton and Newman, 2008; Reddy and DeLaune, 2008; Choi et al., 2009; Klose et al., 2011; Menon et al., 2013; Luo et al., 2017).
The lowest variability of the soil pH in the surface and subsurface horizons of the drained and rewetted peatlands (Table 1) indicated its slow changes among the soil sampling points of each management practice. The similarity in the variability of soil pH and SOM in the surface and subsurface horizons of the drained peatland indicated the peatland management practice similarly influenced both soil horizons. The higher variability of the SOM, total elements, and the plant-available nutrients in the rewetted peatland than the drained peatland (Tables 1–3) illustrated rewetting peatlands increased soil heterogeneity as revealed by the higher CVs of these soil parameters in the rewetted peatlands. The main contributing factors to the heterogeneity of soil properties in the rewetted peatlands were occasional deposition of the inorganic sediment materials from the surrounding landscape by erosion and flooding by the nearby Trebel river. Rewetting also enhances the recolonization of different patchy plant communities, affecting soil chemical and biochemical properties (Andersen et al., 2017). Such patchy vegetation can also trap sediments entering the rewetted peatland from the surrounding landscapes and flooding from the nearby rivers that increased microelevation of the rewetted peatland compared to the drained peatland (Figure 2). The higher and more dense vegetation in the rewetted peatland along with lesser or almost no disturbance by agricultural machinery provided shelter to sounders of boars that further disturb the near surface soil layers in search of food.
The distribution of SOM and total elements (Figure 3), plant-available nutrients, and enzyme activities (Figure 4), as revealed by the PCA, agreed with their variability (Tables 1–4), unequivocally indicated most of the soil properties in the drained peatland were less heterogeneous than that of the rewetted peatland. The continuous disturbance and fertilization for hay and pasture production contributed to less variability of the soil properties in the drained peatland than the soil properties of the rewetted peatland that has been under nature conservation for about 20 years (Jurasinski et al., 2020). The less soil variability in the drained peatland than in the rewetted peatland could also be associated with groundwater dynamics (Ahmad et al., 2020b).
The high variability of the three enzyme activities in both drained and rewetted peatlands (Table 4) indicated their sensitivity to slight changes in soil pH, labile organic compounds, plant nutrients, current vegetation cover, and SOM at the smallscale (Penton and Newman, 2008; Reddy and DeLaune, 2008; Choi et al., 2009; Klose et al., 2011; Menon et al., 2013; Luo et al., 2017). The positive and negative correlations among the plant nutrients and enzyme activities (Figure 4) revealed drainage and rewetting have opposite effects on plant nutrient cycling. Overall, the similar variability of the soil properties between the surface and subsurface horizons of the drained peatland (Tables 1–3) can be explained drainage uniformly influenced the soil properties in both horizons. However, the impact of rewetting on the variability of the soil properties was more pronounced on the surface horizon than on the subsurface horizons.
Spatial Variability of Soil Properties
The results of the descriptive and geostatistical analyses mostly agreed with our initial research hypothesis where rewetting increased the variability (Tables 1–4) and the spatial dependency of most soil properties (Tables 5–8). The lack of spatial dependency of the SOM in the drained peatland can be attributed to the homogeneity of the site at the soil sampling scale of the present study. Homogenization of the surface horizon of the drained peatland was the standard management practice by frequent tillage and new pasture planting since the 1960s (Jurasinski et al., 2020; Weil et al., 2020). The strong spatial variability of soil pH in the surface and subsurface horizons of the drained and rewetted peatland indicated the strong spatial dependence is independent of the lowest variability of soil pH as revealed by the measure of variability (Table 1).
The strong spatial dependence of soil pH and SOM in the rewetted peatland of the present study (Table 5) agreed with the results reported from the long-term rewetted peatland of the Warnow valley of northern Germany (Negassa et al., 2019b) and soil organic carbon (SOC) for Lesotho’s diverse wetlands (Nkheloane et al., 2012). Although there is no information on spatial variability of SOM at large scale sampling intervals in peatlands, studies conducted on mineral soils revealed that SOC showed a strong spatial dependence in agriculturally impacted mineral soils within the semivariogram range of 100 m (Cambardella et al., 1994; Cambardella and Karlen, 1999). Another study on mineral soil also reported 85 m semivariogram range for SOC in agricultural lands (Peukert et al., 2012). Overall, a patchy vegetation cover at small plots, soil transportation, groundwater dynamics, and inorganic sediment deposition, locally exceeding peat accumulation may contribute to the strong spatial dependency of the soil pH and SOM in the rewetted peatland. In addition, the microrelief created during the rewetting processes likely also influenced the spatial variability of the SOM concentration and redox potential in the rewetted peatland (Figure 2).
The lack of spatial dependence of the total element concentrations in drained peatland (data not shown) can be attributed to peatland variability, often changing randomly when subjected to a set of internal (biogeochemical processes) and external (drainage) stressors (Brooks et al., 2005). The major contributing factors to the strong and moderate spatial dependence of the total elements in the rewetted peatland (Table 6) can be attributed to lateral and vertical movements of soil materials systematically during rewetting and drying cycles in wet winter and dry summer seasons, respectively. In this respect, the more pronounced spatial dependence at the uppermost soil horizons indicates a significant influence of surface-near processes such as flooding and sediment input from the nearby river, established plant species from seedlings, or transported rhizomes (Bruland and Richardson, 2005; Bruland et al., 2006). These near-surface processes perhaps contributed to establishing patchy grass species such as Deschampsia caespitosa, Carex acutiformis, Juncus subnodulosus, and Phalaris arundinacea in rewetted fen peatland compared to only one predominant grass species (Deschampsia cespitosa) in the drained peatland. Other factors like swelling/shrinking and rooting may somehow alter the previous difference from surface processes (Camporese et al., 2006; Nijp et al., 2019). These together could explain why the strong and moderate spatial dependency at the surface and subsurface horizons of the rewetted fen peatland, respectively.
Like the other soil properties, the lack of spatial dependence in the plant-available P and Mg in both soil depths of the drained peatland (Table 7) is explained by the long-term drainage, occasional tillage, and fertilization. These agronomic practices most probably randomly changed the soil properties. The drained peatland was also covered with the same grass species for pasture improvement, which plausibly explains the independence of many soil properties compared to the rewetted peatland covered with diverse patchy vegetations (Jurasinski et al., 2020). The strong spatial dependence of the plant-available nutrients in the rewetted peatland (Table 7) agreed with the results reported from the long-term rewetted peatland (Negassa et al., 2019b). Factors that influenced soil pH, SOM, and total element concentrations such as patchy vegetation, sediment input by flooding and overland flow, and physical peat alteration also influenced plant-available nutrients in the rewetted peatland. For example, micro-elevation can create a potential hotspot for faster peat degradation than the lower micro-elevation, where water stands and favors peat accumulation (Ahmad et al., 2020a). The soil water characteristics, hydraulic conductivity, total porosity, groundwater dynamics can also influence peat accumulation, degradation rates, and matter transportation (Ahmad et al., 2020b; Wang et al., 2021).
Hotspots of the extracellular enzyme and root activity at microsites (Spohn and Kuzyakov, 2014) perhaps contributed to the strong spatial dependences of the three enzyme activities within the 5–9 m semivariogram ranges in both drained and rewetted peatlands (Table 8). The strong spatial variability of the enzyme activities in the present study also agreed with a similar study conducted on the long-term rewetted peatland, where the strong spatial dependence appeared within the 5 m semivariogram range (Negassa et al., 2019b). The similarity in spatial variability in the acid phosphatase, β-glucosidase, and arylsulfatase activities in both drained and rewetted peatland can be attributed to their similarity in soil water content during the soil sampling. The soil water content also governs microbial biomasses, individual microbial community structure, redox potential, soil pH, substrate quality, and other physicochemical properties that influence soil enzyme activity (Dick and Kandeler, 2004; Baldrian, 2014). The spatial heterogeneity of enzyme activities was high, even at scales smaller than a few square centimeters (Baldrian, 2014). The strong spatial dependency of soil enzyme activities in the drained and rewetted peatlands in the present study (Table 1) can also be explained by similarity in their substrate quality and soil water content at sampling (Straková et al., 2011).
Implication for Monitoring Peatland Restoration
Rewetting drained and degraded peatlands for restoring biodiversity, carbon sequestration, water purification, and alleviating ecologically undesired biogeochemical processes have been understood for decades. As a result, peatland restoration has been one of the crucial programs for environmental protection in Europe and North America for the last two decades (Chimner et al., 2017; Leifeld and Menichetti, 2018; Renou-Wilson et al., 2019). The influence of peatland restoration on soil biogeochemical processes from the inception to the long-term has been investigated without considering the spatial variability of soil properties. Considering, mainly, soil properties determined from the heterogeneous and mosaic of restored peatlands as random factors could result in either under or overestimation of the soil properties that can be used as indicators of peat restoration. In the present study, all the 17 soil properties from the surface soil horizons and 14 soil properties of the subsurface horizons of the rewetted peatland and soil pH and enzyme activities of the drained peatlands exhibited strong spatial dependence within a specific semivariance range for each soil property in a studied plot of 900 m2 of each site (Tables 5–8). Using the designed soil sampling and data analysis methods for such strongly spatially dependent soil properties does not represent the impact of peatland restoration on soil properties because dependent soil variables are considered independent. For instance, in designed based soil sampling and data analysis methods, the mean of strongly spatially dependent acid phosphatase was 2,112 and 1,575 µg PNp g−1 soil h−1 in drained and rewetted peatlands (Table 4), respectively. However, their values ranged from 870 to 4,100 µg PNp g−1 and 413 to 3,278 µg PNp g−1 for the drained and rewetted peatlands, respectively, and reporting the mean of the soil enzyme activity with such substantial range does not indicate the actual impact of management practices for the studied plot. Such approaches contradict the spatial relationship of soil properties since the values of a soil property from the soil sampling points closer distances are more similar than those of the soil sampling points further apart (Webster and Oliver, 2007; Casado et al., 2013). Thus, the soil sampling and data analysis methods should consider such spatial relationships of soil properties among the values of the soil sampling points.
The model-based soil sampling and data analysis methods do not consider the means of a soil parameter of all the sampling points but the mean of a soil parameter obtained from the sampling points within the semivariance range. The values of a soil parameter obtained beyond the sampling points of the semivariance range are independent of those obtained within the semivariance range. Let’s consider the phosphatase activity again in the drained and rewetted peatlands; the semivariance ranges for the drained and rewetted peatlands were 5 and 9 m, respectively (Table 8). Only the mean of soil sampling points within the semivariance range can be averaged and interpolated on the map if required. In practical terms, the soil samples obtained within the radii of 5 and 9 m of the drained and rewetted peatlands can be composited, or the acid phosphatase activity can be averaged and mapped depending on the objective of the study, respectively. The same principles apply to the soil properties either moderately or strongly spatially dependent in the present study.
To illustrate graphically, we presented spatial heterogeneity of SOM in the drained and rewetted peatland (Figure 5). The SOM was spatially independent in the drained peatland while strongly spatially dependent in the rewetted peatland. The SOM in the drained peatland changed randomly since it had no sill and semivrance range. Under such circumstances, the appropriate soil sampling method to study SOM in the drained peatland is the classic design-based soil sampling. However, the SOM was spatially dependent in rewetted peatland; the SOM changed systematically within the semivariance range of 19 m where spatial soil sample method need to be employed. In this case, the value of SOM was similar within the semivariance range of 19 m. If the soil properties are spatially dependent, the soil sampling has to be done separately for every semivariance range. Under such circumstance, value of a soil property either systematically increases or decreases from an initial sampling point, and taking average values beyond the semivariance range could not represent the actual field condition.
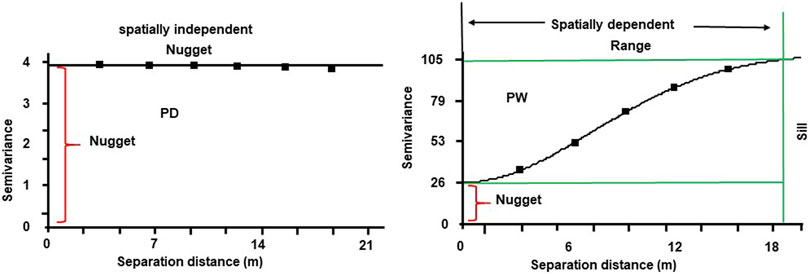
FIGURE 5. Graphical representation of spatial variability of soil organic matter at the surface horizons of the drained (PD) and rewetted (PW) peatlands.
Overall, the results of the present study and a previous study (Negassa et al., 2019b) showed that long-term rewetting increased the spatial variability of soil chemical and biochemical properties. As a result, any future study monitoring biogeochemical processes such as soil carbon sequestration, biochemical activities, microbial community structure, greenhouse gas emissions, and nutrient cycles in long-term restored peatlands should consider spatial soil sampling and geostatistical data analysis to capture the heterogeneity of the ecosystem. Applying the design-based soil sampling and data analysis methods in such heterogeneous experimental units could underestimate the benefits of rewetting drained and restoring degraded peatlands for ecosystem goods and services. Although both designed and model-based soil sampling and data analysis have their own merits and demerits (Casado et al., 2013), considering the relative advantage of the methods and policy implications of the research results are crucial for sustainable use of peatland ecosystems.
In conclusion, this study reported the impact of long-term peatland drainage and restoration on the spatial variability of the selected soil chemical and biochemical properties for the first time. The study results revealed that most soil properties in the surface and subsurface horizon of the long-term drainage lacked spatial dependence. In contrast, all the soil chemical and biochemical properties in the surface and subsurface horizons of the restored peatland were strongly spatially dependent. The lack of spatial dependence of most soil chemical properties in the drained peatland unequivocally discloses that the designed-based soil sampling and data analysis methods can be used for future research at the current sampling scale. However, the design-based soil sampling approach does not fit the rewetted peatland, where almost all the soil properties exhibited a strong spatial dependence at the 4–20 m semivariance ranges. Although spatial variability of soil properties is scale-dependent, we conclude that spatially resolved soil sampling and geostatistical data analysis are required to monitor biogeochemical processes in restored peatland at the scale of the present study for similar fen peatland, land-use history, and pedogenesis. Furthermore, it is likely though completely unknown if spatial variations in the fen peat soil properties could change annually. This is a very interesting and challenging research topic but would require large numbers of soil samples to be taken and analyzed.
Data Availability Statement
The datasets presented in this study can be found in online repositories. The names of the repository/repositories and accession number(s) can be found below: https://www.wetscapes.uni-rostock.de/datenportal/.
Author Contributions
WN: Conception, designed, and conducted the study, statistical data analysis and writing the manuscript; CB: Methodology, FB: Methodology; PL: Contributed to the conception, funding acquisition, and editing of the manuscript. All authors contributed to manuscript revision, read, and approved the submitted version.
Funding
This work was funded by the European Social Fund (ESF) and the Ministry of Education, Science, and Culture of Mecklenburg-Western Pomerania within the scope of the project WETSCAPES (ESF/14-BM-A55-0029/16-64160025).
Conflict of Interest
The authors declare that the research was conducted in the absence of any commercial or financial relationships that could be construed as a potential conflict of interest.
Publisher’s Note
All claims expressed in this article are solely those of the authors and do not necessarily represent those of their affiliated organizations, or those of the publisher, the editors and the reviewers. Any product that may be evaluated in this article, or claim that may be made by its manufacturer, is not guaranteed or endorsed by the publisher.
Acknowledgments
The authors were grateful for the technical assistance received from Elena Heilmann, Christoph Jahnke, Anika Zacher, and Nora Vitov, Soil Science Laboratory, and Wakjira Takala Dibaba, Hydrology and Applied Meteorology Department, University of Rostock.
References
Ahmad, S., Liu, H., Beyer, F., Kløve, B., and Lennartz, B. (2020a). Spatial Heterogeneity of Soil Properties in Relation to Microtopography in a Non-tidal Rewetted Coastal Mire. Mires and Peat 26, 1–18. doi:10.19189/MaP.2019.GDC.StA.1779
Ahmad, S., Liu, H., Günther, A., Couwenberg, J., and Lennartz, B. (2020b). Long-term Rewetting of Degraded Peatlands Restores Hydrological Buffer Function. Sci. Total Environ. 749, 141571. doi:10.1016/j.scitotenv.2020.141571
Andersen, R., Farrell, C., Graf, M., Muller, F., Calvar, E., Frankard, P., et al. (2017). An Overview of the Progress and Challenges of Peatland Restoration in Western Europe. Restor. Ecol. 25, 271–282. doi:10.1111/rec.12415
Armstrong, A., Holden, J., Kay, P., Foulger, M., Gledhill, S., McDonald, A. T., et al. (2009). Drain-blocking Techniques on Blanket Peat: A Framework for Best Practice. J. Environ. Manage. 90, 3512–3519. doi:10.1016/j.jenvman.2009.06.003
Arrouays, D., McKenzie, N., Hempel, J., de Forges, A. C. R., and McBratney, A. (2014). Global Soil Map: Basis of the Global Spatial Soil Information System. London, UK: CRC Press, Taylor & Francis Group.
Baldrian, P. (2014). Distribution of Extracellular Enzymes in Soils: Spatial Heterogeneity and Determining Factors at Various Scales. Soil Sci. Soc. America J. 78, 11–18. doi:10.2136/sssaj2013.04.0155dgs
Baum, C., Leinweber, P., and Schlichting, A. (2003). Effects of Chemical Conditions in Re-wetted Peats on Temporal Variation in Microbial Biomass and Acid Phosphatase Activity within the Growing Season. Appl. Soil Ecol. 22, 167–174. doi:10.1016/s0929-1393(02)00129-4
Berger, S., Gebauer, G., Blodau, C., and Knorr, K.-H. (2017). Peatlands in a Eutrophic World - Assessing the State of a Poor Fen-Bog Transition in Southern Ontario, Canada, after Long Term Nutrient Input and Altered Hydrological Conditions. Soil Biol. Biochem. 114, 131–144. doi:10.1016/j.soilbio.2017.07.011
Berry, J. K. (2005). Analyzing Geo-Spatial Resource Data: A Hands-On Case Study in Spatial Analysis and Data Mining. Denver, Colorado: University of Denver.
Bess, J. A., Chimner, R. A., and Kangas, L. C. (2014). Ditch Restoration in a Large Northern Michigan Fen: Vegetation Response and Basic Porewater Chemistry. Ecol. Restoration 32, 260–274. doi:10.3368/er.32.3.260
Biswas, A., and Si, B. C. (2011). “Scaling of Soil Physical Properties,” in Encyclopedia of Agrophysics, Encyclopedia of Earth Sciences Series. Editors J. Gliński, J. Horabik, and J. Lipiec. Dordrecht: Springer. doi:10.1007/978-90-481-3585-1
Brooks, R. P., Wardrop, D. H., Cole, C. A., and Campbell, D. A. (2005). Are We Purveyors of Wetland Homogeneity? Ecol. Eng. 24, 331–340. doi:10.1016/j.ecoleng.2004.07.009
Bruland, G. L., and Richardson, C. J. (2005). Spatial Variability of Soil Properties in Created, Restored, and Paired Natural Wetlands. Soil Sci. Soc. Am. J. 69, 273–284. doi:10.2136/sssaj2005.0273a
Bruland, G. L., Richardson, C. J., and Whalen, S. C. (2006). Spatial Variability of Denitrification Potential and Related Soil Properties in Created, Restored, and Paired Natural Wetlands. Wetlands 26, 1042–1056. doi:10.1672/0277-5212(2006)26[1042:svodpa]2.0.co;2
Burton, E. D., Bush, R. T., Johnston, S. G., Sullivan, L. A., and Keene, A. F. (2011). Sulfur Biogeochemical Cycling and Novel Fe-S Mineralization Pathways in a Tidally Re-flooded Wetland. Geochimica et Cosmochimica Acta 75, 3434–3451. doi:10.1016/j.gca.2011.03.020
Cambardella, C. A., and Karlen, D. L. (1999). Spatial Analysis of Soil Fertility Parameters. Precis. Agric. 1, 5–14. doi:10.1023/a:1009925919134
Cambardella, C. A., Moorman, T. B., Novak, J. M., Parkin, T. B., Karlen, D. L., Turco, R. F., et al. (1994). Field-scale Variability of Soil Properties in Central Iowa Soils. Soil Sci. Soc. America J. 58, 1501–1511. doi:10.2136/sssaj1994.03615995005800050033x
Camporese, M., Ferraris, S., Putti, M., Salandin, P., and Teatini, P. (2006). Hydrological Modeling in Swelling/shrinking Peat Soils. Water Resour. Res. 42, W06420. doi:10.1029/2005WR004495
Casado, M. R., Corstanje, R., Bellamy, P., and Marchant, B. (2013). “Issues of Sampling Design in Wetlands,” in Methods in Biogeochemistry of Wetlands. SSSA Book Series, no. 10. Editors R. D. DeLaune, K. R. Reddy, C. J. Richardson, and J. P. Megonigal (Madison, WI: Soil Science Society of America, 5585 Guilford Road), 1–19.
Chimner, R. A., Cooper, D. J., Wurster, F. C., and Rochefort, L. (2017). An Overview of Peatland Restoration in North America: where Are We after 25 years? Restor Ecol. 25, 283–292. doi:10.1111/rec.12434
Choi, J. H., Kang, H., and Park, S. S. (2009). Comparison of Enzyme Activities in Vegetated and Nonvegetated Sediments. J. Environ. Eng. 135, 299–305. doi:10.1061/(asce)ee.1943-7870.0000015
Clarkson, B. R., Ausseil, A. E., and Gerbeaux, P. (2013). “Wetland Ecosystem Services,” in Ecosystem Services in New Zealand – Conditions and Trends. Editor J. R. Dymond (Lincoln, New Zealand: Manaaki Whenua Press).
Cobbaert, D., Rochefort, L., and Price, J. S. (2004). Experimental Restoration of a Fen Plant Community after Peat Mining. Appl. Vegetation Sci. 7, 209–220. doi:10.1111/j.1654-109x.2004.tb00612.x
Cohen, M. J., Dunne, E. J., and Bruland, G. L. (2008). Spatial Variability of Soil Properties in cypress Domes Surrounded by Different Land Uses. Wetlands 28, 411–422. doi:10.1672/06-182.1
Cooper, D. J., and MacDonald, L. H. (2000). Restoring the Vegetation of Mined Peatlands in the Southern Rocky Mountains of Colorado, U.S.A. Restor Ecol. 8, 103–111. doi:10.1046/j.1526-100x.2000.80016.x
R. D. DeLaune, K. R. Reddy, C. J. Richardson, and J. P. Megonigal (Editors) (2013). Methods in Biogeochemistry of Wetlands. SSSA Book Series (Madison, WI: Soil Science Society of America), 10, 1004.
Dick, R. P., and Kandeler, E. (2004). “Enzymes in Soils,” in Encyclopedia of Soils in the Environment. Editor D. Hillel (London: Academic Press), 448–456.
Dietrich, S. T., and MacKenzie, M. D. (2018). Comparing Spatial Heterogeneity of Bioavailable Nutrients and Soil Respiration in Boreal Sites Recovering from Natural and Anthropogenic Disturbance. Front. Environ. Sci. 6, 126. doi:10.3389/fenvs.2018.00126
Duval, T. P., and Radu, D. D. (2018). Effect of Temperature and Soil Organic Matter Quality on Greenhouse-Gas Production from Temperate Poor and Rich Fen Soils. Ecol. Eng. 114, 66–75. doi:10.1016/j.ecoleng.2017.05.011
Eivazi, F., and Tabatabai, M. A. (1988). Glucosidases and Galactosidases in Soils. Soil Biol. Biochem. 20, 601–606. doi:10.1016/0038-0717(88)90141-1
Emsens, W.-J., Aggenbach, C. J. S., Schoutens, K., Smolders, A. J. P., Zak, D., and van Diggelen, R. (2016). Soil Iron Content as a Predictor of Carbon and Nutrient Mobilization in Rewetted Fens. PLoS ONE 11, e0153166. doi:10.1371/journal.pone.0153166
Fageria, N. K., Baligar, V. C., and Clark, R. B. (2002). Micronutrients in Crop Production. Adv. Agron. 77, 185–268. doi:10.1016/s0065-2113(02)77015-6
Forsmann, D. M., and Kjaergaard, C. (2014). Phosphorus Release from Anaerobic Peat Soils during Convective Discharge - Effect of Soil Fe:P Molar Ratio and Preferential Flow. Geoderma 223-225, 21–32. doi:10.1016/j.geoderma.2014.01.025
Gerendás, J., and Führs, H. (2013). The Significance of Magnesium for Crop Quality. Plant Soil 368, 101–128. doi:10.1007/s11104-012-1555-2
Geurts, J. J. M., Smolders, A. J. P., Verhoeven, J. T. A., Roelofs, J. G. M., and Lamers, L. P. M. (2008). Sediment Fe:PO4ratio as a Diagnostic and Prognostic Tool for the Restoration of Macrophyte Biodiversity in Fen Waters. Freshw. Biol. 53, 2101–2116. doi:10.1111/j.1365-2427.2008.02038.x
Girkin, N. T., Vane, C. H., Cooper, H. V., Moss-Hayes, V., Craigon, J., Turner, B. L., et al. (2019). Spatial Variability of Organic Matter Properties Determines Methane Fluxes in a Tropical Forested Peatland. Biogeochemistry 142, 231–245. doi:10.1007/s10533-018-0531-1
Grønlund, A., Hauge, A., Hovde, A., and Rasse, D. P. (2008). Carbon Loss Estimates from Cultivated Peat Soils in Norway: a Comparison of Three Methods. Nutr. Cycl. Agroecosyst. 81, 157–167. doi:10.1007/s10705-008-9171-5
Groß-Schmölders, M., Klein, K., Birkholz, A., Leifeld, J., and Alewell, C. (2021). Rewetting and Drainage of Nutrient-Poor Peatlands Indicated by Specific Bacterial Membrane Fatty Acids and a Repeated Sampling of Stable Isotopes (δ15N, δ13C). Front. Environ. Sci. 9, 730106. doi:10.3389/fenvs.2021.730106
Grunwald, S., Reddy, K. R., Newman, S., and DeBusk, W. F. (2004). Spatial Variability, Distribution and Uncertainty Assessment of Soil Phosphorus in a South Florida Wetland. Environmetrics 15, 811–825. doi:10.1002/env.668
Haapalehto, T., Juutinen, R., Kareksela, S., Kuitunen, M., Tahvanainen, T., Vuori, H., et al. (2017). Recovery of Plant Communities after Ecological Restoration of Forestry-Drained Peatlands. Ecol. Evol. 7, 7848–7858. doi:10.1002/ece3.3243
Hahn, J., Köhler, S., Glatzel, S., and Jurasinski, G. (2015). Methane Exchange in a Coastal Fen in the First Year after Flooding - a Systems Shift. PLoS ONE 10, e0140657. doi:10.1371/journal.pone.0140657
Heller, C., and Zeitz, J. (2012). Stability of Soil Organic Matter in Two Northeastern German Fen Soils: the Influence of Site and Soil Development. J. Soils Sediments 12, 1231–1240. doi:10.1007/s11368-012-0500-6
Hergert, G. W., Ferguson, R. B., Shapiro, C. A., Penas, E. J., and Anderson, F. B. (1995). “Classical Statistical and Geostatistical Analysis of Soil Nitrate-N Spatial Variability,” in Site-Specific Management for Agricultural Systems. Editors P. C. Robert, R. H. Rust, and W. E. Larson (Madisson, WI: ASA-CSSA-SSSA), 175–186.
Hodgkins, S. B., Richardson, C. J., Dommain, R., Wang, H., Glaser, P. H., Verbeke, B., et al. (2018). Tropical Peatland Carbon Storage Linked to Global Latitudinal Trends in Peat Recalcitrance. Nat. Commun. 9, 3640. doi:10.1038/s41467-018-06050-2
Jurasinski, G., Ahmad, S., Anadon‐Rosell, A., Berendt, J., Beyer, F., Bill, R., et al. (2020). From Understanding to Sustainable Use of Peatlands: The WETSCAPES Approach. Soil Syst. 4, 1–27. doi:10.3390/soilsystems4010014
Kandel, T. P., Karki, S., Elsgaard, L., and Lærke, P. E. (2019). Fertilizer-induced Fluxes Dominate Annual N2O Emissions from a Nitrogen-Rich Temperate Fen Rewetted for Paludiculture. Nutr. Cycl. Agroecosyst 115, 57–67. doi:10.1007/s10705-019-10012-5
Kibblewhite, M. G., Miko, L., and Montanarella, L. (2012). Legal Frameworks for Soil protection: Current Development and Technical Information Requirements. Curr. Opin. Environ. Sustainability 4, 573–577. doi:10.1016/j.cosust.2012.08.001
Klose, S., Bilen, S., Tabatabai, M. A., and Dick, W. A. (2011). “Sulfur Cycle Enzymes,” in Methods of Soil Enzymology. Editor R. P. Dick (Madison, WI: Soil Science Society of America), 125–159.
Kõlli, R., Asi, E., Apuhtin, V., Kauer, K., and Szajdak, L. W. (2010). Chemical Properties of Surface Peat on forest Land in Estonia. Mires Peat 6, 1–12.
Kuchenbuch, R. O., and Buczko, U. (2011). Re‐visiting Potassium‐ and Phosphate‐fertilizer Responses in Field Experiments and Soil‐test Interpretations by Means of Data Mining. Z. Pflanzenernähr. Bodenk. 174, 171–185. doi:10.1002/jpln.200900162
Lamers, L. P. M., Vile, M. A., Grootjans, A. P., Acreman, M. C., van Diggelen, R., Evans, M. G., et al. (2015). Ecological Restoration of Rich Fens in Europe and North America: from Trial and Error to an Evidence‐based Approach. Biol. Rev. 90, 182–203. doi:10.1111/brv.12102
Larkin, D. J. (2016). “Wetland Heterogeneity,” in The Wetland Book. Editors C. M. Finlayson, M. Everard, K. Irvine, R. J. McInnes, B. A. Middleton, A. A. van Damet al. (Dordrecht: Springer Science+Business Media). doi:10.1007/978-94-007-6172-8_52-6
Lawrence, P. G., Roper, W., Morris, T. F., and Guillard, K. (2020). Guiding Soil Sampling Strategies Using Classical and Spatial Statistics: A Review. Agron.j. 112, 493–510. doi:10.1002/agj2.20048
Leifeld, J., and Menichetti, L. (2018). The Underappreciated Potential of Peatlands in Global Climate Change Mitigation Strategies. Nat. Commun. 9, 1071. doi:10.1038/s41467-018-03406-6
Leifeld, J., Gubler, L., and Grünig, A. (2011). Organic Matter Losses from Temperate Ombrotrophic Peatlands: an Evaluation of the Ash Residue Method. Plant Soil 341, 349–361. doi:10.1007/s11104-010-0649-y
Limpens, J., Berendse, F., Blodau, C., Canadell, J. G., Freeman, C., Holden, J., et al. (2008). Peatlands and the Carbon Cycle: from Local Processes to Global Implications - a Synthesis. Biogeosciences 5, 1475–1491. doi:10.5194/bg-5-1475-2008
Loescher, H., Ayres, E., Duffy, P., Luo, H., and Brunke, M. (2014). Spatial Variation in Soil Properties Among North American Ecosystems and Guidelines for Sampling Designs. PLoS ONE 9, e83216. doi:10.1371/journal.pone.0083216
Lundin, L., Nilsson, T., Jordan, S., Lode, E., and Strömgren, M. (2017). Impacts of Rewetting on Peat, Hydrology and Water Chemical Composition over 15 Years in Two Finished Peat Extraction Areas in Sweden. Wetlands Ecol. Manage. 25, 405–419. doi:10.1007/s11273-016-9524-9
Luo, L., Meng, H., and Gu, J.-D. (2017). Microbial Extracellular Enzymes in Biogeochemical Cycling of Ecosystems. J. Environ. Manage. 197, 539–549. doi:10.1016/j.jenvman.2017.04.023
Menon, R., Jackson, C. R., and Holland, M. M. (2013). The Influence of Vegetation on Microbial Enzyme Activity and Bacterial Community Structure in Freshwater Constructed Wetland Sediments. Wetlands 33, 365–378. doi:10.1007/s13157-013-0394-0
Merhaut, D. J. (2007). “Magnesium,” in Handbook of Plant Nutrition. Editors A. V. Barker, and D. J. Pilbeam (New York: CRC Press, Taylor & Francis Group), 145–181.
Ministerium für Landwirtschaft und Naturschutz Mecklenburg-Vorpommern (1998). Düngung 1998. Hinweise und Richtwerte für die landwirtschaftliche Praxis. Schwerin: Leitfaden zur Umsetzung der Düngeverordnung, 136.
Montero, J. -M., Fernández-Avilés, G., and Mateu, J. (2015). Spatial and Spatio-Temporal Geostatistical Modeling and Kriging. West Sussex, United Kingdom: John Wiley & Sons.
Negassa, W., Acksel, A., Eckhardt, K.-U., Regier, T., and Leinweber, P. (2019a). Soil Organic Matter Characteristics in Drained and Rewetted Peatlands of Northern Germany: Chemical and Spectroscopic Analyses. Geoderma 353, 468–481. doi:10.1016/j.geoderma.2019.07.002
Negassa, W., Baum, C., Schlichting, A., Müller, J., and Leinweber, P. (2019b). Smallscale Spatial Variability of Soil Chemical and Biochemical Properties in a Rewetted Degraded Peatland. Front. Environ. Sci. 7, 1–15. doi:10.3389/fenvs.2019.00116
Negassa, W., Michalik, D., Klysubun, W., and Leinweber, P. (2020). Phosphorus Speciation in Long-Term Drained and Rewetted Peatlands of Northern Germany. Soil Sys 4, 1–20. doi:10.3390/soilsystems4010011
Nelson, D. W., and Sommers, L. E. (1996). “Total Carbon, Organic Carbon, and Organic Matter,” in Methods of Soil Analysis. Part 3. Chemical Methods. SSSA Book Ser. 5. Editor D. L. Sparks (Madison, WI: SSSA), 961–1010.
Nieminen, M., Sallantaus, T., Ukonmaanaho, L., Nieminen, T. M., and Sarkkola, S. (2017). Nitrogen and Phosphorus Concentrations in Discharge from Drained Peatland Forests Are Increasing. Sci. Total Environ. 609, 974–981. doi:10.1016/j.scitotenv.2017.07.210
Nijp, J. J., Metselaar, K., Limpens, J., Bartholomeus, H. M., Nilsson, M. B., Berendse, F., et al. (2019). High‐resolution Peat Volume Change in a Northern Peatland: Spatial Variability, Main Drivers, and Impact on Ecohydrology. Ecohydrology 12, e2114. doi:10.1002/eco.2114
Nkheloane, T., Olaleye, A. O., and Mating, R. (2012). Spatial Heterogeneity of Soil Physico-Chemical Properties in Contrasting Wetland Soils in Two Agro-Ecological Zones of Lesotho. Soil Res. 50, 579–589. doi:10.1071/sr12145
Osborne, T. Z., and DeLaune, R. D. (2013). “Soil and Sediment Sampling of Inundated Environments,” in Methods in Biogeochemistry of Wetlands. SSSA Book Series, no. 10. Editors. R. D. DeLaune, K. R. Reddy, C. J. Richardson, and J. P. Megonigal (Madison, WI: Soil Science Society of America, 5585 Guilford Road), 21–40.
Penton, C. R., and Newman, S. (2008). Enzyme-based Resource Allocated Decomposition and Landscape Heterogeneity in the Florida Everglades. J. Environ. Qual. 37, 972–976. doi:10.2134/jeq2007.0248
Peters, J., and von Unger, M. (2017). Peatlands in the EU Regulatory EnvironmentSurvey with Case Studies on Poland and Estonia. Bonn, Germany: Federal Agency for Nature Conservation Bundesamt für Naturschutz.
Peukert, S., Bol, R., Roberts, W., Macleod, C. J. A., Murray, P. J., Dixon, E. R., et al. (2012). Understanding Spatial Variability of Soil Properties: a Key Step in Establishing Field- to Farm-Scale Agro-Ecosystem Experiments. Rapid Commun. Mass. Spectrom. 26, 2413–2421. doi:10.1002/rcm.6336
Reddy, K. R., and DeLaune, R. D. (2008). Biogeochemistry of Wetlands:-Science and Applications. New York: Tylor and Francis.
Renou-Wilson, F., Moser, G., Fallon, D., Farrell, C. A., Müller, C., and Wilson, D. (2019). Rewetting Degraded Peatlands for Climate and Biodiversity Benefits: Results from Two Raised Bogs. Ecol. Eng. 127, 547–560. doi:10.1016/j.ecoleng.2018.02.014
Richardson, C. J., and Reddy, K. R. (2013). “Methods for Soil Phosphorus Characterization and Analysis of Wetland Soils,” in Methods in Biogeochemistry of Wetlands. SSSA Book Series, no. 10. Editors. R. D. DeLaune, K. R. Reddy, C. J. Richardson, and J. P. Megonigal (Madison, WI: Soil Science Society of America), 603–638.
Richert, M., Dietrich, O., Koppisch, D., and Roth, S. (2000). The Influence of Rewetting on Vegetation Development and Decomposition in a Degraded Fen. Restor Ecol. 8, 186–195. doi:10.1046/j.1526-100x.2000.80026.x
Rochefort, L., Quinty, F., Campeau, S., Johnson, K., and Malterer, T. (2003). North American Approach to the Restoration of Sphagnum Dominated Peatlands. Wetl. Ecol. Manag. 11, 3–20. doi:10.1023/a:1022011027946
Schillereff, D. N., Boyle, J. F., Toberman, H., Adams, J. L., Bryant, C. L., Chiverrell, R. C., et al. (2016). Long-term Macronutrient Stoichiometry of UK Ombrotrophic Peatlands. Sci. Total Environ. 572, 1561–1572. doi:10.1016/j.scitotenv.2016.03.180
Schlesinger, W. H., and Bernhardt, E. S. (2020). Biogeochemistry: An Analysis of Global Change. London: Academic Press, 749.
Schlichting, A., Leinweber, P., Meissner, R., and Altermann, M. (2002). Sequentially Extracted Phosphorus Fractions in Peat-Derived Soils. J. Plant Nutr. Soil Sci. 165, 290–298. doi:10.1002/1522-2624(200206)165:3<290::aid-jpln290>3.0.co;2-a
Shit, P. K., Bhunia, G. S., and Maiti, R. (2016). Spatial Analysis of Soil Properties Using GIS Based Geostatistics Models. Model. Earth Syst. Environ. 2. doi:10.1007/s40808-016-0160-4
Si, B. C., Kachanoski, R. G., and Reynolds, W. D. (2007). “Analysis of Soil Variability,” in Soil Sampling and Methods of Analysis. Editor E. G. Gregorich (Boca Raton: CRC Press), 1163–1191.
Slessarev, E. W., Lin, Y., Bingham, N. L., Johnson, J. E., Dai, Y., Schimel, J. P., et al. (2016). Water Balance Creates a Threshold in Soil pH at the Global Scale. Nature 540, 567–569. doi:10.1038/nature20139
Song, Y., Song, C., Shi, F., Wang, M., Ren, J., Wang, X., et al. (2019). Linking Plant Community Composition with the Soil C Pool, N Availability and Enzyme Activity in Boreal Peatlands of Northeast China. Appl. Soil Ecol. 140, 144–154. doi:10.1016/j.apsoil.2019.04.019
Spohn, M., and Kuzyakov, Y. (2014). Spatial and Temporal Dynamics of Hotspots of Enzyme Activity in Soil as Affected by Living and Dead Roots-A Soil Zymography Analysis. Plant Soil 379, 67–77. doi:10.1007/s11104-014-2041-9
Storey, J. B. (2007). “Zinc,” in Handbook of Plant Nutrition. Editors A. V. Barker, and D. J. Pilbeam (New York: CRC Press, Taylor & Francis Group), 411–435.
Straková, P., Niemi, R. M., Freeman, C., Peltoniemi, K., Toberman, H., Heiskanen, I., et al. (2011). Litter Type Affects the Activity of Aerobic Decomposers in a Boreal Peatland More Than Site Nutrient and Water Table Regimes. Biogeosciences 8, 2741–2755. doi:10.5194/bgd-8-1879-2011
Sutton-Grier, A. E., Keller, J. K., Koch, R., Gilmour, C., and Megonigal, J. P. (2011). Electron Donors and Acceptors Influence Anaerobic Soil Organic Matter Mineralization in Tidal Marshes. Soil Biol. Biochem. 43, 1576–1583. doi:10.1016/j.soilbio.2011.04.008
Tabatabai, M. A., and Bremner, J. M. (1969). Use of P-Nitrophenyl Phosphate for Assay of Soil Phosphatase Activity. Soil Biol. Biochem. 1, 301–307. doi:10.1016/0038-0717(69)90012-1
Tabatabai, M. A., and Bremner, J. M. (1970). Factors Affecting Soil Arylsulfatase Activity. Soil Sci. Soc. America J. 34, 427–429. doi:10.2136/sssaj1970.03615995003400030023x
Taube, F., Appel, T., Ebertseder, T., Müller, T., Olfs, H.‐W., Nätscher, L., et al. (2015). Phosphordüngung nach Bodenuntersuchung Anpassung der Richtwerte für die Gehaltsklassen ist geboten und notwendig. Darmstadt: VDLUFA-Verlag.
Tomscha, S. A., Bentley, S., Platzer, E., Jackson, B., de Roiste, M., Hartley, S., et al. (2021). Multiple Methods Confirm Wetland Restoration Improves Ecosystem Services. Ecosyst. People 17, 25–40. doi:10.1080/26395916.2020.1863266
Trangmar, B. B., Yost, R . S., and Uehara, G. (1985). Application of Geostatistics to Spatial Studies of Soil Properties. Adv. Agron. 38, 45–94.
Urzainki, I., Laurén, A., Palviainen, M., Haahti, K., Budiman, A., Basuki, I., et al. (2020). Canal Blocking Optimization in Restoration of Drained Peatlands. Biogeosciences 17, 4769–4784. doi:10.5194/bg-17-4769-2020
US EPA (2007). Method 3051A (SW-846): Microwave Assisted Acid Digestion of Sediments, Sludges, and Oils. Washington, DC: United State Environmental Protection Agency. Revision 1.
VDLUFA (2012). “Methode A 6.2.1.1, Bestimmung von Phosphor und Kalium im Calcium-AcetatLactat-Auszug,” in das Handbuch der Landwirtschaftlichen Versuchs- und Untersuchungsmethodik (VDLUFA-Methodenbuch), Bd. I Die Untersuchung von Böden, 4. Auflage, 6. Teillieferung (Darmstadt: VDLUFA-Verlag).
VDLUFA (2016). “Bestimmung des pH-Wertes,” in das Handbuch der Landwirtschaftlichen Versuchs- und Untersuchungsmethodik (VDLUFA-Methodenbuch), Die Untersuchung von Böden, 1. Auflage, 7. Teillieferung (Darmstadt: VDLUFA-Verlag).
Wang, M., and Moore, T. R. (2014). Carbon, Nitrogen, Phosphorus, and Potassium Stoichiometry in an Ombrotrophic Peatland Reflects Plant Functional Type. Ecosystems 17, 673–684. doi:10.1007/s10021-014-9752-x
Wang, M., Liu, H., and Lennartz, B. (2021). Smallscale Spatial Variability of Hydro-Physical Properties of Natural and Degraded Peat Soils. Geoderma 399, 115123. doi:10.1016/j.geoderma.2021.115123
Webster, R., and Oliver, M. A. (2007). Geostatistics for Environmental Scientists. 2nd Ed. New York: Wiley, 315.
Weil, R. R., and Brady, N. C. (2017). The Nature and Properties of Soils. 15th ed. Essex, England: Pearson Education Limited.
Weil, M., Wang, H., Bengtsson, M., Köhn, D., Günther, A., Jurasinski, G., et al. (2020). Long-Term Rewetting of Three Formerly Drained Peatlands Drives Congruent Compositional Changes in Pro- and Eukaryotic Soil Microbiomes through Environmental Filtering. Microorganisms 8, 1–20. doi:10.3390/microorganisms8040550
Wright, A. L., and Reddy, K. R. (2001). Phosphorus Loading Effects on Extracellular Enzyme Activity in Everglades Wetland Soils. Soil Sci. Soc. Am. J. 65, 588–595. doi:10.2136/sssaj2001.652588x
Zak, D., Gelbrecht, J., Wagner, C., and Steinberg, C. E. W. (2008). Evaluation of Phosphorus Mobilization Potential in Rewetted Fens by an Improved Sequential Chemical Extraction Procedure. Eur. J. Soil Sci. 59, 1191–1201. doi:10.1111/j.1365-2389.2008.01081.x
Zerbe, S., Steffenhagen, P., Parakenings, K., Timmermann, T., Frick, A., Gelbrecht, J., et al. (2013). Ecosystem Service Restoration after 10 Years of Rewetting Peatlands in NE Germany. Environ. Manage. 51, 1194–1209. doi:10.1007/s00267-013-0048-2
Keywords: nugget to sill ratio, semivariance range, soil chemical properties, soil enzyme activities, spatial dependence
Citation: Negassa W, Baum C, Beyer F and Leinweber P (2022) Spatial Variability of Selected Soil Properties in Long-Term Drained and Restored Peatlands. Front. Environ. Sci. 10:804041. doi: 10.3389/fenvs.2022.804041
Received: 28 October 2021; Accepted: 25 March 2022;
Published: 14 April 2022.
Edited by:
Miguel Ferrer, Spanish National Research Council (CSIC), SpainReviewed by:
Colin P. R. McCarter, McMaster University, CanadaSaraswati Saraswati, University of Waterloo, Canada
Copyright © 2022 Negassa, Baum, Beyer and Leinweber. This is an open-access article distributed under the terms of the Creative Commons Attribution License (CC BY). The use, distribution or reproduction in other forums is permitted, provided the original author(s) and the copyright owner(s) are credited and that the original publication in this journal is cited, in accordance with accepted academic practice. No use, distribution or reproduction is permitted which does not comply with these terms.
*Correspondence: Wakene Negassa, d2ViYXNzYTk2OUBnbWFpbC5jb20=