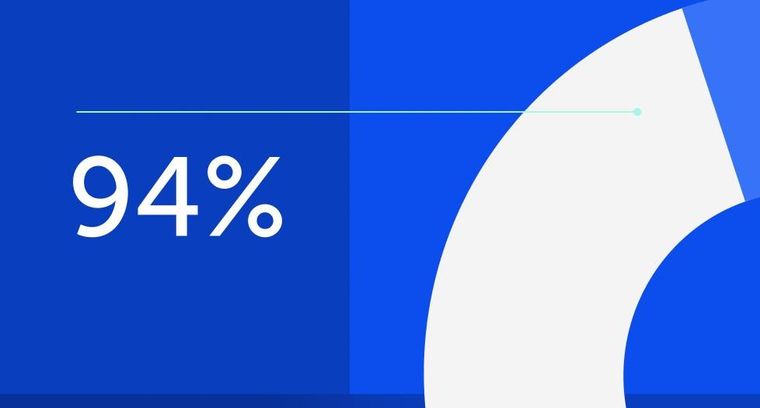
94% of researchers rate our articles as excellent or good
Learn more about the work of our research integrity team to safeguard the quality of each article we publish.
Find out more
ORIGINAL RESEARCH article
Front. Environ. Sci., 16 May 2022
Sec. Land Use Dynamics
Volume 10 - 2022 | https://doi.org/10.3389/fenvs.2022.687723
This article is part of the Research TopicLandscapes, Land-Use Change and Ecosystem Services in Colombia's Orinoquia Region: Recent Change and Future ProspectsView all 9 articles
The process of biological invasions resulting from the introduction of exotic species is one of the most critical components of global environmental change. Although many hypotheses try to explain the processes underlying biological invasions, changes in land use are essential drivers mediating the colonization of exotic species at the landscape level. We used potential species distribution models developed in Maxent and a database of nine functional traits associated with invasion success for 18 exotic species with a high risk of establishment and difficulty of control in the Orinoco region from Colombia. We found that 67% of the species differed from the centroid in the climatic niche when comparing native and invaded localities. Overall, the native distribution localities showed a more restricted dispersal in their climatic niche, and for most species, native distribution was found within the broad environmental gradients of the invaded localities. Additionally, we found high climatic suitability for all exotic species across all the biomes and ecosystems; however, transformed ecosystems showed exotic plant species’ higher potential functional richness. Our research allowed us to identify key geographical areas that are highly susceptible to invasions and prioritize species that need control in particular ecosystems and biomes. This work provides early warnings on the potential risks of invasion of exotic species, and it will improve the monitoring and management efforts in the Orinoco region of Colombia.
Biological invasion, resulting from the introduction of exotic species, is one of the most critical components of global environmental change (Sala et al., 2000), causing homogenization of biota (McKinney and Lockwood, 1999; Quian and Ricklefs, 2006) and strong impacts on ecosystem functioning (Strayer et al., 2006; Vásquez-Valderrama et al., 2020). Several studies have revealed that land-use change is a critical factor driving the colonization and establishment of exotic species (DeGasperis and Motzkin, 2007; Chytrý et al., 2008; Mosher et al., 2009). Land-use changes may promote invasions by reducing local species diversity (Simberloff et al., 2013). Diverse communities, like those present in natural ecosystems, are highly competitive and have near complete use of resources (space, light, or nutrients), resulting in greater resistance to invasions due to low resources availability for the establishment of the exotic species (Levine and D’Antonio, 1999; Kennedy et al., 2002). Another hypothesis postulates that disturbance is the main factor promoting biological invasions by eliminating strong native competitors who could not use limited resources adequately (Hobbs and Atkins, 1988; Burke and Grime, 1996). Additionally, land-use changes may also increase resource availability for exotic species, expand colonization opportunities (Davis et al., 2000), or create corridors that promote exotic species dispersion (With, 2002; Vilá and Ibañez, 2011). Despite many hypotheses explaining invasions, little is known about the potential invasion risk using distribution models and trait-based ecology following changes in land use.
Understanding the potential distribution of exotic species and the underlying biological mechanisms is essential to guiding appropriate control and management strategies of these species in non-native habitats. The ecological niche modeling (ENM) uses associations between geolocalized species records and environmental variables of the species to calculate the suitability of the habitat known to be occupied by the species or the habitat that can be potentially occupied by the species (Peterson, 2003; Phillips et al., 2006). The ENM has been widely used to predict the extent of plant invasion (e.g., Peterson, 2003; Thuiller et al., 2005; Atwater et al., 2018; Briscoe Runquist et al., 2019). It is widely accepted that the similarity in climate and environmental conditions between native and invaded habitats is essential for a successful invasion (Panetta and Mitchell, 1991; Thuiller et al., 2005). However, mixed evidence has been found regarding climatic niche shifts during an invasion. For instance, in a meta-analysis with 50 invasive species, just 15% of the species had more than 10% of their invaded distribution outside their native climatic niche (Petitpierre et al., 2012), and the transferability was close to 70% for 163 invasive plant species (Liu et al., 2020a). Conversely, several studies have reported changes in niche breadth and/or position (i.e., niche shift) between native and invaded areas (Broennimann et al., 2007; Pearman et al., 2008; Guisan et al., 2014; Atwater et al., 2018). These niche changes may result from changes in biotic and abiotic conditions in new colonized places (realized niche) or even shifts in the fundamental niche through genetic recombination, interspecific hybridization, or natural selection (Pearman et al., 2008; Guisan et al., 2014). Under this conflicting evidence, exploring climatic niche shifts is fundamental for including native and invaded localities in the ENM. Additionally, the information is essential for improving our predictions of the spatial distribution of exotic species as well as increasing our ability to forecast species responses to upcoming climate changes (Wiens et al., 2009).
It is now widely accepted that successful colonization and establishment of exotic plants depend on invaded habitat properties, and it is highly related to species’ functional traits (sensu invasiveness; Kempel et al., 2013). However, functional strategies promoting successful biological invasions seem habitat-dependent (Daneshgar and Jose, 2009; Bernhardt-Römermann et al., 2011). For instance, C4 grasses have an advantage in dry and open environments such as tropical savannas because their leaves fix more carbon than C3 plants in those environments, causing higher growth rates and efficiency in water use (Bond, 2008; Schmidt et al., 2011; Mora-Fernandez and Peñuela-Recio, 2013; Silvério et al., 2013). However, C3 woody species such as Leucaena leucocephala or Ricinus communis have been reported as successful colonizers in savannas due to their extensive ability to acclimate to contrasting shade levels (Benjamin et al., 1991; Martins et al., 2011). Additionally, they have human-assisted dispersal and soil seed banks waiting for the best conditions to germinate (USDA-NRCS, 2002; Martins et al., 2009). The facets of functional diversity summarize the complexity derived from the biotic communities under study and their functional traits (Mouchet et al., 2010). In this sense, potential functional richness maps of exotic species allow us to give early warning that go beyond the presence of the species in an ecosystem, focusing the monitoring and control on species that are particularly hazardous because of their functional traits.
Many studies exploring the relationship between land-use change and biological invasions have focused on temperate forests or grasslands (Quian and Ricklefs, 2006; DeGasperis and Motzkin, 2007; Chytrý et al., 2008; Mosher et al., 2009). Unique tropical ecosystems, such as savannas, have been poorly studied despite their high diversity and importance to local community livelihoods. The Orinoco region, located in Venezuela and Colombia, is a mosaic of different biomes and ecosystems, including savannas, forests alongside rivers, and tropical rainforest remnants, that have been exposed to high anthropogenic transformation for decades (Etter et al., 2010). For instance, in the early 1970s, exotic grass species were introduced for extensive livestock ranching, and between 1980 and 2000, forestry practices were implemented (Andrade et al., 2009; Romero-Ruiz et al., 2011). In the last two decades agro-industrial uses and forest plantations such as rice and African oil palm have been promoted by the national governments, substantially increasing their extension across the Orinoco landscape (Romero-Ruiz et al., 2011; Vargas et al., 2018). These agricultural practices have intensified the land-use transformation, creating a mosaic of natural and transformed lands that converts this region into an ideal model to understand the relationships between functional richness, land-use change, and the potential for biological invasion.
We explored the climatic niche variation between native and invaded localities for 18 exotic species in the Orinoco region in Colombia. Additionally, we identified which natural biomes and transformed ecosystems are potentially susceptible to invasion by exotic plants and how functional richness could vary under these new invasion scenarios. We chose nine plant functional traits related to the spread and establishment phases of invasion. The species were selected due to their high risk of establishment, the difficulty of control and their potential to spread at the landscape level, which may cause negative impacts on natural ecosystems (Cárdenas-López et al., 2010; Cárdenas-López et al., 2017). Specifically, we addressed the following questions: 1) How does the climatic niche of exotic species vary between native and invaded localities? 2) Which natural biomes and transformed ecosystems are most suitable for exotic plant species’ establishment? And 3) using the potential distribution of exotic species, how does functional richness vary among natural biomes and transformed ecosystems?
The macro-basin of the Colombian Orinoco covers approximately 35,616,167 hectares and comprises a mosaic of different types of landscapes due to its topographic and geologic diversity (Prüssmann et al., 2020). The region includes several ecosystems and biomes (Table 1), but primarily savannas with an elevation range from 200 to 1,200 m a.s.l. (Etter et al., 2010). Vegetation is adapted to soils with low nutrient content and little productive capacity since they are usually acidic with superficial lateritic shells. However, the vegetation surrounding streams and lakes is denser because of more recent sediments and elevated moisture in the soil. Forest-type vegetation is also observed to the west of the region, in the areas that border the foothills of the Andes Mountain range (Etter et al., 2010). The economy of this region is based mainly on petroleum, livestock (cattle ranching), African oil palm, and forestry monocultures (e.g., Pinus caribaea), all activities that introduced extensively exotic plant species to the landscape (Lozano et al., 2007; Etter et al., 2010; Romero-Ruiz et al., 2011; Vargas et al., 2018).
TABLE 1. Natural biomes and transformed ecosystems for the Orinoco’s Region and area in km2. Descriptions based on Etter (1998) and Etter et al. (2010), Etter et al. (2017).
From a list of 42 potentially invasive species of Colombia (Cárdenas-López et al., 2010; Giraldo-Cañas, 2011), 18 plant species whose presence has been confirmed in the Colombian Orinoco region were selected (Cárdenas-López et al., 2017). Species selection included 13 herbs (four C3 species and nine C4 grasses) and five woody species distributed among nine families (Supplementary Table S1).
Nine functional traits related to different plant strategies were obtained for each species (Supplementary Table S1). Growth form is a vegetative trait determined by canopy structure and height and may be associated with light competition and responses to climatic and land-use factors (Cornelissen et al., 2003). Spinescence plays a prominent role in anti-herbivore defense (Hanley et al., 2007). It could be essential to reduce heat or drought stress, a primary environmental filter in Orinoco ecosystems. Vegetative propagation is associated with the plant’s competitive vigor, ability to expand its distribution range, and capacity to persist after environmental disturbance (Klimes et al., 1997; Cornelissen et al., 2003). Dispersal syndrome and the presence of seed banks are related to dispersion and colonization success (Moravcová et al., 2015). Photosynthetic pathways and leaf shape have significant consequences for the leaf energy and water balance and CO2 assimilation rates under contrasting environments (Ripley et al., 2007; Ghannoum, 2009). Sexual reproduction is related to dependence on a pollinator, where dioecious species may be more vulnerable than monoecious and hermaphroditic species. Finally, allelopathy is associated with the negative effect of one plant on another one through the release of chemical compounds into the environment (Hierro and Callaway, 2003). Several studies have demonstrated the importance of these traits to invasion success, particularly in the spread and establishment stages (Callaway and Aschehoug, 2000; Pyšek and Richardson, 2007; van Kleunen et al., 2010).
Functional trait information was obtained from academic databases (ISI Web of Science, SCOPUS, ScienceDirect, and Google Scholar) using the species name and the name of each functional trait. Additionally, specialized botanical and invasive species databases were reviewed, such as the IUCN Global Invasive Species Database, JSTOR Global Plants, Invasive Species Compendium CABI.org, KEW (online World Grass Flora), Missouri Botanical Garden, HEAR (Hawaiian Ecosystem at Risk Project), CONABIO’s Mexican Invasive Species database (Invasive species fact-sheet), the Inter American Biodiversity Network (Invasive Species), TRY (Plant Trait Database), the flora do Brasil, the flora of North America, and some others.
We downloaded 111,924 occurrences (native and non-native distributions) from the Global Biodiversity Information Facility database (GBIF.org, 2020; http://www.gbif.org/) using the occ_search function of the rgbif package (Chamberlain et al., 2021) in R (R Core Team, 2019) on 27 October 2020, for the 18 invasive species with probable distribution in the Orinoco region from Colombia. We retained 15,409 records with longitude and latitude data, removing duplicates and other records with inconsistent geo-referencing (on the sea, zero- longitude and latitude; Chapman, 2005). To avoid model overfitting due to spatial autocorrelation and geographical sampling bias, occurrence data was thinned with a 50 km radius rule (Briscoe Runquist et al., 2019), using the R package spThin (Aiello-Lammens et al., 2015). Based on these criteria, the maximum number of localities used for modeling was 2,865, and the minimum number of localities was 54 (Supplementary Table S2).
We generated species distribution models using the Maxent package ver. 3.4.0 (Phillips et al., 2017) to identify suitable habitats for each exotic plant species. Maxent was run using the “dismo” (Hijmans et al., 2019) and ENMeval packages (Muscarella et al., 2014) in R version 3.5.3 (R Core Team, 2019). Explanatory variables were taken from the 19 bioclimatic layers, WorldClim ver. 2.1 (Fick and Hijmans, 2017, see https://www.worldclim.org/data/worldclim21.html, accessed 24 September 2020), at a resolution of 30 arc-seconds (∼1 km2). We included the complete set of variables due to the algorithm learning which ones are important via regularization (Phillips et al., 2006; Feng et al., 2019). Models were calibrated using explanatory variables and records without distinguishing between the species’ native and non-native ranges. We built the final models using the settings chosen as optimal for each species and all spatially filtered occurrence records. Finally, the optimal models were transferred to the study area in the Colombian Orinoco Region. A logistic output format was used to describe the probability of a species’ presence (Phillips and Dudík, 2008), which is a continuous habitat suitability range between 0 (unsuitable) and 1 (the most suitable). Details of the modeling protocol and maps of potential distribution per species can be consulted in Supplementary Tables S2, S3, S4.
We analyzed two diversity dimensions of the exotic plant community: species and functional richness. Functional diversity is usually split into three components: functional richness, functional evenness, and functional divergence. Functional richness is the most widely used approach for measuring and investigating functional diversity (Legras et al., 2018). Therefore, we selected functional richness (FD, Petchey and Gaston, 2002) to represent the functional diversity. FD based on functional richness measures the extent of trait complementarity among species through a continuous metric. Meanwhile, species richness indicates the number of species distributed in a location. Taxonomic and functional metrics were represented in maps at 1 km2 pixel resolution (Supplementary Tables S5, S6). To calculate species richness predictions, we stack the binary maps of the individual Species Distribution Models (SDM) as the total sum of the number of species in each pixel. To calculate the index of functional richness (FD) that measures the total length of the branches from all species pool on a functional dendrogram of species by traits (Petchey and Gaston, 2002), we ran the function “dist” to compute a pairwise similarity matrix of the species, based on the Jaccard-type functional (dis)similarity (Anderson and Willis, 2003; Chao et al., 2019). Then, we built a dendrogram using the unweighted pair group method with the arithmetic mean (UPGMA) clustering procedure via a hierarchical cluster implemented in the function “hclust” of the stats package to cluster the species based on similarities of their traits. We used the “proxy” and “stats” packages to calculate the distance matrix and the UPGMA, respectively (Meyer and Buchta, 2019). We calculated the FD map using the speciesRaster package (speciesRaster: Generation of SpeciesRaster Object and Calculation of morphological and phylogenetic metrics; available at https://github.com/ptitle/speciesRaster). The dendrogram was used to calculate the branch length of the species by pixel, considering that each of them represents a biotic community of exotic plant species. The species in each pixel are characterized by the continuous habitat suitability ranges of the species. The package uses four radius cells to define the moving window and measures turnover in which branches are weighted by the inverse of the species ranges (Cardoso et al., 2014). This procedure improves the diversity comparisons among communities (Gotelli and Colwell, 2001). Finally, the FD is expressed in a map that shows values ranging from 0 to 1, indicating high FD when it takes values near 1.
To determine the spatial distribution of taxonomic and functional richness between transformed and natural Colombian Orinoco ecosystems and biomes, we overlapped the species richness map and FD map over the general ecosystem map of Colombia (Etter, 1998; Etter et al., 2017). Sixteen categories of ecosystems and biomes were considered, eight of which were found at the level of natural biomes. Specifically for the transformed biome, we proceeded to discriminate it at the level of eight ecosystems (Table 1). We used the geographical unit of the biome to identify the main natural ecological areas in the Orinoco region (Etter et al., 2017) and it has already been used in other studies (Thuiller et al., 2005). For the anthropogenically transformed biome, we choose the ecosystem category since, at this level, the effect of exotic species is better recorded in perturbed areas (With, 2002; Vilá and Ibáñez, 2011). Then, we rasterized to 1 km2 each map unit and extracted the values for pixels of the taxonomic and functional richness maps for each of the 16 categories. Based on the distribution of 18 exotic plant species in the 242,978 pixels that comprise the Orinoco region of Colombia, we summed the presence data on each of the 16 biomes/ecosystems and calculated the distribution percentages. The analyses were performed using the raster and rgdal packages (Bivand et al., 2020; Hijmans, 2020) in R version 3.5.3 (R Core Team, 2019).
To estimate the climatic niche shift, distance-based tests for homogeneity of multivariate dispersions (PERMDISP routine; Anderson, 2006) were conducted per species to compare the distances from native and invaded localities to their group centroid (Supplementary Table S7). For each biological record, values for the 19 bioclimatic variables were obtained from WorldClim ver. 2.1 at the resolution of 30 arc-seconds (∼1 km2) (Fick and Hijmans, 2017, see https://www.worldclim.org/data/worldclim21.html, accessed 24 September 2020). For each species, the values of the bioclimatic variables were standardized, and a matrix of Euclidean distances between biological records was constructed. Niche shift directionality was estimated by measuring changes in the niche centroid, which is the center of mass of the climate niche between native vs. invaded localities (Atwater et al., 2018). The PERMDISP uses the F statistic to compare the distances from biotic records among native and invaded locations to their centroid to obtain p-values by using 9,999 permutations of the least-squares residuals (Clarke and Gorley, 2015). Based on the Euclidean distances of climatic niche, we ran principal coordinate analyses (PCoA, Gower, 1966) to visualize the variation in species occurrences differentiating between native and invaded localities (Supplementary Table S8).
To determine which transformed ecosystems and natural biomes are more susceptible to invasion by exotic plant species and how functional richness varies among them, 100 pixels were randomly selected from each biome and ecosystem, and a one-way ANOVA was performed. When significant effects were found, the differences were identified using a Bonferroni test. These analyses were performed in InfoStat software (Di Rienzo et al., 2020). Additionally, a heat map was built showing exotic species’ floristic composition per natural biome and transformed ecosystem. Specifically, for the floristic composition, the distribution percentage values per species for each ecosystem were previously transformed to the square root from which a Bray Curtis similarity matrix was calculated. The heat map represents the association of the exotic plant species concerning the natural biomes and transformed ecosystems based on a color gradient ranging from white (no association) to black (high association) (Somerfield and Clarke, 2013). The analyses were performed in the PRIMER 7.0.13 and PERMANOVA add-on program (Clarke and Gorley, 2015).
We found remarkable differences between native vs. introduced climatic niches for most species (Supplementary Tables S7, S8). Centroid shifts were significant for twelve of the 18 exotic species (67% of species), and exotic species tended to move towards wetter places, although this varied enormously among species (Supplementary Tables S7, S8). The native distribution localities showed a more restricted dispersal in their climatic niche, and for all species, native distribution was found within the broad environmental gradients of the invaded localities. For six species, breadfruit (Artocarpus altilis), African palm (Elaeis guineensis), white butterfly ginger lily (Hedychium coronarium), jaragua grass (Hyparrhenia rufa), itch grass (Rottboellia cochinchinensis), and Mexican sunflower (Tithonia diversifolia), there were no significant differences between native and invaded localities.
We did not find significant differences in potential exotic species richness between natural biomes and transformed ecosystems (F = 2.2 E-4; p = 0.988); however, we found high variation among them (F = 83.35; p < 0.0001) (Figure 1). Transformed ecosystems such as rice fields, livestock areas, and coffee fields are predicted to be suitable for most exotic species (Figure 1). Still, surprisingly, the suitability of rice fields did not vary from natural ecosystems such as Orinoco helobiomes or tropical forests (Figure 1). The rural areas with low to medium anthropic intervention (20–50% of the original ecosystem) showed the lowest suitability for exotic species, followed by Amazonian natural biomes such as litobiomes, orobiomes, peinobiomes, and helobiomes (Figure 1). Regarding functional richness, transformed ecosystems are predicted to be potentially invaded by species with more contrasting functional traits than natural biomes (F = 27.22; p < 0.0001), showing higher functional richness in transformed ecosystems. However, we found a high variation among transformed ecosystems and natural biomes (F = 54.98; p < 0.0001) (Figure 2). Most transformed ecosystems such as rural areas and mixed agroecosystems (Mix_agr and Ent_agr) as soybeans, sorghum and cotton are predicted to have a higher functional richness of exotic species (Figure 2); however, Andean orobiomes did not differ from rural areas (Rur20-50) (Figure 2). Amazonian peinobiomes are predicted to be colonized by exotic species functionally similar (Figure 2). The heat map showed that species such as A. altilis, bitter gourd (Momordica charantia), most grasses, and surprisingly, the aquatic species H. coronarium are expected to colonize all ecosystems and biomes (Figure 3). Unexpectedly, grass species such as bermuda grass (Cynodon dactylon), and signal grasses (Urochloa brizantha and U. decumbens), had a low probability of colonizing most ecosystems and biomes (Figure 3).
FIGURE 1. Boxplots showing taxonomic richness among biomes/ecosystems and land-use type (natural vs. transformed) of exotic species in the Orinoco region. Different letters indicate significant differences based on the Bonferroni test. For biomes/ecosystems see Table 1. Red: transformed ecosystems and green: natural biomes.
FIGURE 2. Boxplots showing functional richness among biomes/ecosystems and land-use type (natural vs. transformed) of exotic species in the Orinoco region. Different letters indicate significant differences based on the Bonferroni test. For biomes/ecosystems see Table 1. Red: transformed ecosystems and green: natural biomes.
FIGURE 3. Heat map of Whittaker (1952) association index of 18 exotic plant species concerning 16 natural biomes and transformed ecosystems in the Orinoco region.
Integrating species distribution models with climatic niche shifts between native and invasive localities allowed us to identify which species have more probability of invading natural biomes and transformed ecosystems in the Colombian Orinoco region. Specifically, we found that natural biomes and transformed ecosystems had the same invasion risk of exotic species, but the latter ones had a higher functional richness of invasive species. Potential functional richness, evidenced in a spatially explicit manner (Supplementary Table S6; https://data.mendeley.com/datasets/s9bdytdt2f/2), allowed us to identify potential sites in which exotic species with contrasting functional traits may exclude native species through resource-intensive foraging with ecosystem functioning consequences. Our results should be seen as an early warning for the potential risk of dispersion and colonization of exotic species in other areas where they are not yet present.
Climatic niche shifts in exotic species may result primarily from unfilling (Petitpierre et al., 2012), as it takes time for introduced species to colonize all suitable niche spaces (Jiménez-Valverde et al., 2011). However, for most exotic species of our study, the invaded range was larger than the native range. This niche expansion may result from different biotic interactions in the introduced range (Shea and Chesson, 2002) or evolutionary changes in the fundamental niche through hybridization, genetic recombination, or other processes (Pearman et al., 2008; Guisan et al., 2014). The rapid evolution of invasive species has been reported as a strategy for adapting to new environmental pressures in the invaded range (Kooyers and Olsen, 2012). Nevertheless, it is essential to recognize that erroneous estimations of the native range caused by sampling bias or local extinctions can cause a “false expansion” (Atwater et al., 2018). Therefore, several authors argued that only increased occupancy of environmental space in the exotic range also available in the native range should be interpreted as a niche shift (Liu et al., 2020b). Conversely, the absence of the differences in the climatic niche between native and invaded ranges for five of our studied species may be related to climatic preadaptation to recipient environments (Petitpierre et al., 2012). For instance, this could be the case for species from tropical Africa, such as A. altilis, or H. rufa. However, as most of these species were recently introduced to the Orinoco region, some of them may evolve with time, modifying climatic niches between native and invaded ranges (Liu et al., 2020b). In our study, Caribbean pine (P. caribaea), elephant grass (Cenchrus purpureus), H. coronarium, castor bean (R. communis), and common bamboo (Bambusa vulgaris) showed the most remarkable differences in mean deviations from the centroid between native and invaded localities (Supplementary Table S8). The capacity of these species for colonizing and establishing in climatically different places from their native localities is a wake-up call to prioritize the management and control of their populations in the Orinoco region.
As our results showed that most species had climatic niche shifts between native and invaded ranges (Supplementary Table S8), we built species distribution models including both native and invaded range records to represent, in the potential distributions, as much of the climatic niche as is represented in the set of biological records of the species. Our results about habitat suitability are based on the assumption that under no dispersal limitations are the climatic preferences of a species the primary driver of its distribution. Therefore, the species preferences may be used to predict the early stages of an invasion (Thuiller et al., 2005). Although the Orinoco region has a high climatic homogeneity, we found differences in the invasion risk among biomes and ecosystems. For instance, livestock agroecosystems, characterized by intense dry periods and high solar radiation and evaporation rates (Knapp, 1985; Forrestel et al., 2014), were highly susceptible to the invasion of plants such as African grasses and tree species such as E. guineensis and Pinus caribea (Figure 3). Natural Amazonian biomes such as orobiomes and litobiomes (characterized by montane areas with a temperate climate and rocky and sand formations, respectively) showed low susceptibility to the invasion of exotic species such as R. communis, and T. diversifolia, and grasses of the Urochloa genus (synonyms of Brachiaria brizantha and B. decumbens) (Figure 3). Despite contrasting invasion risks among ecosystems/biomes, the high climatic homogeneity makes the Orinoco region particularly vulnerable to establishing any exotic species that are already invading since no environmental filters control the dispersal and establishment of the species. This high vulnerability to invasion can be reflected in the wide potential distribution of at least 12 of the 18 species throughout the Orinoco region (Supplementary Table S5; https://data.mendeley.com/datasets/s9bdytdt2f/2). Additionally, most of these species are native to tropical areas (Cárdenas-López et al., 2017); therefore, their requirements may be supplied by the Orinoco regional environmental conditions. Under this scenario, our maps of potential distribution per biome and ecosystem are fundamental tools for prioritizing monitoring and control efforts that can help guard against further introductions and the initiation of new invasion forces (Thuiller et al., 2005).
It is essential to highlight that the successful establishment and actual distribution of exotic species depend on other local factors such as propagule density, soils, or biotic interactions (Willis and Whittaker, 2002). Several studies have shown a higher performance of exotic species under anthropogenic management regimes in transformed ecosystems (Wang et al., 2018). It could be related to eliminating strong native competitors that could not use limited resources adequately (Hobbs and Atkins, 1988; Burke and Grime, 1996). Likewise, sites with high potential functional richness show that invasive species could occupy the entire spectrum of resource use, displacing native species and altering ecosystem processes (Mason et al., 2005). African grasses, for instance, promote and are stimulated by fire. The large standing necromass left by these grasses at the end of the dry season facilitates the combustion and increases the intensity of fires (Williams and Baruch, 2000), modifying nutrient and carbon cycling and affecting biodiversity. This disturbance increases the net resources available that, summed to anthropogenic management (e.g., direct fertilization or other sources of pollution), would facilitate invasion (Davis et al., 2000), enabling exotic species establishment in transformed ecosystems that are frequently burned as part of management practices, such as rice fields and livestock areas (Mandal et al., 2004; Armenteras et al., 2021). Additionally, anthropogenic fire may exclude native fire-intolerant species, reducing woody plant cover (Armenteras et al., 2021) and impacting long-term non-management ecosystems. The disturbance and the massive expansion of exotic species plantations such as African palm (E. guineensis) and P. caribaea make all-natural biomes of Orinoco’s region potentially vulnerable to invasions of these species in the future (Lozano et al., 2007). Currently, E. guineensis is a commonly used crop for biodiesel production. Colombia is the primary producer of oil palm in Latin America (Erazo et al., 2020), with an annual area devoted to plantations increasing at a rate of 7,396.3 ha/year (Cordovez and Guhl, 2015). Unfortunately, anthropogenic disturbances at the landscape level or social dynamics (e.g., colonization of new areas by the development of road infrastructure and associated tourism) are rarely considered because accurate maps at an appropriate scale or field estimates are seldom available.
The establishment and actual distribution of exotic species is also related to their functional traits that determine species’ success at the different stages of invasion (introduction, establishment, and spread) under different environmental conditions (Kempel et al., 2013). It would be expected that species with contrasting functional traits have different probabilities for colonizing ecosystems and biomes. For instance, species with water dispersal may be easily transported through the freshwater flooding pulses that characterize Orinoco’s helobiomes promoting their spread from other ecosystems. Examples of these species are grasses such as Urochloa decumbens, Ischaemum rugosum, C. dactylon, and R. cochinchinensis. Additionally, the superior water and nutrient use efficiency, and the higher photosynthetic rates of C4 grasses in environments characterized by high solar radiation and soil temperatures and low soil fertility (Knapp, 1985; Forrestel et al., 2014; Atkinson et al., 2016) increase their establishment probability in Amazonian peinobiomes dominated by savannas, but also in transformed ecosystems. High dispersal abilities like producing and storing seeds or having animal and assisted dispersal syndromes are important traits to guarantee successful dispersion to new areas. Species such as E. guineensis, L. leucocephala, and P. caribeae do not depend on other individuals for reproduction (monoecious reproduction) and have animal and assisted dispersion, allowing them to expand their distribution ranges beyond plantations (Kuo, 2003). Native forests surrounded by African palm plantations show high palm regeneration and recruitment in Colombia (Lozano et al., 2007). Individuals of L. leucocephala frequently invade riverbanks, roadsides, and forest margins as well as cultivated lands and wastelands in Thailand (Marod et al., 2012). Additionally, L. leucocephala has fast growth rates and high drought stress tolerance (Luo et al., 2020), increasing its probability of establishment under any ecosystem/biome in the Orinoco region. Species such as L. leucocephala, H. coronarium, M. charantia, R. communis, and several grass species have allelopathic compounds inhibiting the growth of native species (Supplementary Table S1). For instance, L. leucocephala releases an amino acid (mimosine) that is poisonous to other plants (Matthews and Brand, 2004), inhibiting the growth and reproduction of native species (Wolfe and Van Bloem, 2012). U. decumbens allelopathic potential is reported in different organs and may remain throughout its lifetime including senescence (Barbosa et al., 2008), reducing the germination of native species (Barbosa et al., 2008). As exotic species have contrasting functional strategies, knowing the species’ life history and functional traits is another fundamental tool to prioritize the species that must be monitored and controlled. Therefore, building potential functional richness maps (Supplementary Table S6; https://data.mendeley.com/datasets/s9bdytdt2f/2) will allow field studies to focus on current processes of biological invasion and their relationship with the disturbances caused by the transformation of the landscape (sensu Huston, 2004; Gross et al., 2005).
Species potential distribution models are a powerful management and monitoring tool for exotic species (Jiménez-Valverde et al., 2011; Srivastava et al., 2019). Although several studies have shown their importance as unbiased first-step screening for early warning systems (Thuiller et al., 2005), it is essential to recognize that the species richness based on overlapping distribution maps is commonly higher than those inferred from species survey data that do not occur everywhere within their climatic range due to other local processes that are not considered in the correlative species’ niche modeling (Hurlbert and White, 2005; Jímenez-Valverde et al., 2011). It is crucial to control type 1 and 2 errors in predicting species distributions before proposing management and monitoring actions (Uden et al., 2015). Therefore, our species distribution models and richness maps should be considered early warning maps delineating probable occupancy of areas based on climatic suitability for a single species or groups of them (Thuiller et al., 2005; Jímenez-Valverde et al., 2011; Srivastava et al., 2019). In our study, this spatial overprediction in species’ distribution maps is a desirable property that reflects the nature of invasive species (Jímenez-Valverde et al., 2011) that can colonize both natural biomes and transformed ecosystems. Given that it is usually easier and more economical to prevent an introduction or spreading than to control an invasion (Pejchar and Mooney, 2009), distribution maps based on ecological niche models will be fundamental for guiding early detections and promoting rapid responses to invasion risks in the Orinoco region. As suggested by Thuiller et al. (2005), those areas identified as having a high probability of invasion due to their high climatic suitability for some exotic species should be monitored since eradication is costly and inefficient once the populations are established (Rejmánek and Pitcairn, 2002).
Since a national economic frontier is emerging throughout the Orinoco region (Andrade et al., 2009), it is crucial to understand how exotic plant species are distributed among different natural biomes and transformed ecosystems and which functional traits may promote their invasion processes. The main strength of our study is the identification of key geographical areas that are highly susceptible to invasions and the prioritization of species that need control in particular ecosystems and biomes. Our study may be helpful as an early warning to improve monitoring and management efforts for biological invasions in one of the most transformed regions in Colombia. The dilemma emerges regarding the economic benefits and negative impacts of intentionally introduced exotic species and their management (Pejchar and Mooney, 2009; Dickie et al., 2014). Considering our study, almost 50% of exotic species are of economic value as forage, agroforestry products, and biofuels, among other uses (Andrade et al., 2009; DNP, 2018). Currently, the big environmental challenge in the Orinoco region is to pursue cost-effective management within economic and ecological functional limits as a critical basis for stakeholders and decision-makers (Andrade et al., 2009). Therefore, the implementation of an integrative land-use and management planning should incorporate ecological regimes as well as natural and anthropogenic spatiotemporal dynamics that are of the utmost urgency and should be given priority (e.g., Lourival et al., 2011). In this sense, the exotic plants reported in this study should be monitored based on rigorous fieldwork to demonstrate by measuring hard functional traits (e.g., ecophysiological), the effects on communities of native species, and ecosystem processes and services. Future studies should consider the plasticity of functional traits measured in the field for exotic plant species in order to build mechanistic niche models (e.g., Chapman et al., 2017) and project them under global change scenarios (e.g., future changes in climate and land use). Finally, monitoring plans sharing information between sectors should consider the inclusion of exotic plant species lists as a potential risk in agricultural production databases as preliminary initiatives discussed by the UPRA (Unidad de Planificación Agropecuaria) of the Agricultural Ministry, as well as its articulation in the government’s interministerial agendas.
The original contributions presented in the study are included in the article/Supplementary Material, further inquiries can be directed to the corresponding authors. Raster maps of taxonomic and functional diversity, as well as species distribution models can be freely downloaded from the Mendeley Data repository at the link: https://data.mendeley.com/datasets/s9bdytdt2f/2.
BS-N, NU-C and MB conceived and designed the research. EN-U and CC-R performed species distribution models and taxonomic richness and functional diversity maps. NU-C performed the statistical analysis. BS-N wrote the first version of the manuscript and all authors contributed to revisions.
The authors declare that the research was conducted in the absence of any commercial or financial relationships that could be construed as a potential conflict of interest.
All claims expressed in this article are solely those of the authors and do not necessarily represent those of their affiliated organizations, or those of the publisher, the editors and the reviewers. Any product that may be evaluated in this article, or claim that may be made by its manufacturer, is not guaranteed or endorsed by the publisher.
We want to thank the land owners in Tauramena and San Martin municipalities, especially to Lalo Enciso from the Unamas Reserve who still believe in local biodiversity conservation. We are grateful to Diego Giraldo Cañas (Instituto de Ciencias Naturales -ICN- Universidad Nacional de Colombia) national expert in Poaceae and to Fernando Castro, a local botany expert. Juliana Cárdenas and Rosa Arrieta helped with species trait documentation and Maribel Vásquez collected species records from GBIF. Wilson Ramirez, Fernando Fernandez, Carolina Alcazar and Maria Angela Echeverry provided comments on an earlier version of this manuscript. We also thank two reviewers and the editor for comments that improved the manuscript. We are grateful to Dairon Cardenas-López (Instituto Amazónico de Investigaciones Científicas, SINCHI), an expert in botany and national invasive species, for all his technical advice and hope that this paper perpetuates the legacy of the research he carried out during his lifetime.
The Supplementary Material for this article can be found online at: https://www.frontiersin.org/articles/10.3389/fenvs.2022.687723/full#supplementary-material
Aiello-Lammens, M. E., Boria, R. A., Radosavljevic, A., Vilela, B., and Anderson, R. P. (2015). spThin: An R Package for Spatial Thinning of Species Occurrence Records for Use in Ecological Niche Models. Ecography 38, 541–545. doi:10.1111/ecog.01132
Anderson, M. J. (2006). Distance-based Tests for Homogeneity of Multivariate Dispersions. Biometrics 62, 245–253. doi:10.1111/j.1541-0420.2005.00440.x
Anderson, M. J., and Willis, T. J. (2003). Canonical Analysis of Principal Coordinates: a Useful Method of Constrained Ordination for Ecology. Ecology 84, 511–525. doi:10.1890/0012-9658(2003)084[0511:caopca]2.0.co;2
Andrade, G. I., Castro, L. G., Durán, A., Rodríguez, M., Rudas, G., Uribe, E., et al. (2009). La mejor Orinoquia que podemos construir. Elementos para la sostenibilidad ambiental del desarrollo Bogotá. Colombia: Universidad de los Andes Bogotá.
Armenteras, D., Meza, M. C., González, T. M., Oliveras, I., Balch, J. K., and Retana, J. (2021). Fire Threatens the Diversity and Structure of Tropical Gallery Forests. Ecosphere 12, e03347. doi:10.1002/ecs2.3347
Atkinson, R. R. L., Mockford, E. J., Bennett, C., Christin, P.-A., Spriggs, E. L., Freckleton, R. P., et al. (2016). C4 Photosynthesis Boosts Growth by Altering Physiology, Allocation and Size. Nat. Plants 2, 16038. doi:10.1038/nplants.2016.38
Atwater, D. Z., Ervine, C., and Barney, J. N. (2018). Climatic Niche Shifts Are Common in Introduced Plants. Nat. Ecol. Evol. 2, 34–43. doi:10.1038/s41559-017-0396-z
Barbosa, E. G., Pivello, V. R., and Meirelles, S. T. (2008). Allelopathic Evidence in Brachiaria Decumbens and its Potential to Invade the Brazilian Cerrados. Braz. Arch. Biol. Technol. 51, 625–631. doi:10.1590/s1516-89132008000400021
Benjamin, A. E., Newacheck, P. W., and Wolfe, H. (1991). Intergenerational Equity and Public Spending. Pediatrics 88, 75–83. doi:10.1542/peds.88.1.75
Bernhardt-Römermann, M., Gray, A., Vanbergen, A. J., Bergès, L., Bohner, A., Brooker, R. W., et al. (2011). Functional Traits and Local Environment Predict Vegetation Responses to Disturbance: A pan‐European Multi‐site experiment. J. Ecol. 99, 777–787. doi:10.1111/j.1365-2745.2011.01794.x
Bivand, R., Keitt, T., and Rowlingson, B. (2020). Rgdal: Bindings for the ‘Geospatial' Data Abstraction Library. R package version 1.5-12. Available at: https://CRAN.R-project.org/package=rgdal (Accessed January 1, 2020).
Bond, W. J. (2008). What Limits Trees in C4 Grasslands and Savannas? Annu. Rev. Ecol. Evol. Syst. 39, 641–659. doi:10.1146/annurev.ecolsys.39.110707.173411
Briscoe Runquist, R. D., Lake, T., Tiffin, P., and Moeller, D. A. (2019). Species Distribution Models throughout the Invasion History of Palmer Amaranth Predict Regions at Risk of Future Invasion and Reveal Challenges with Modeling Rapidly Shifting Geographic Ranges. Sci. Rep. 9, 2426–2512. doi:10.1038/s41598-018-38054-9
Broennimann, O., Treier, U. A., Müller-Schärer, H., Thuiller, W., Peterson, A. T., and Guisan, A. (2007). Evidence of Climatic Niche Shift during Biological Invasion. Ecol. Lett. 10, 701–709. doi:10.1111/j.1461-0248.2007.01060.x
Burke, M. J. W., and Grime, J. P. (1996). An Experimental Study of Plant Community Invasibility. Ecology 77, 776–790. doi:10.2307/2265501
Callaway, R. M., and Aschehoug, E. T. (2000). Invasive Plants versus Their New and Old Neighbors: a Mechanism for Exotic Invasion. Science 290, 521–523. doi:10.1126/science.290.5491.521
Cárdenas-López, D., Baptiste, M. P., and Castaño, N. (2017). Plantas exóticas con alto potencial de invasión en Colombia. Bogotá D.C: Instituto de Investigación de Recursos Biológicos Alexander von Humboldt.
Cárdenas-López, D., Castaño-Arboleda, N., and Cárdenas-Toro, J. (2010). “Análisis de riesgo y propuesta de categorización de especies introducidas para Colombia,” in Análisis de riesgo y propuesta de categorización. Editors M. P. Baptiste, N. Castaño, D. Cárdenas-López, F. de. P. Gutierrez, D. L. Gil, and C. A. Lasso (Bogotá: Bogotá, Instituto de Investigación de Recursos Biológicos Alexander von Humboldt), 52–71.
Cardoso, P., Rigal, F., Carvalho, J. C., Fortelius, M., Borges, P. A. V., Podani, J., et al. (2014). Partitioning Taxon, Phylogenetic and Functional Beta Diversity into Replacement and Richness Difference Components. J. Biogeogr. 41, 749–761. doi:10.1111/jbi.12239
Chamberlain, S., Barve, V., Mcglinn, D., Oldoni, D., Desmet, P., Geffert, L., et al. (2021). Rgbif: Interface to the Global Biodiversity Information Facility API. R package version 3.5.2. Available at: https://CRAN.R-project.org/package=rgbif.
Chao, A., Chiu, C. H., Villéger, S., Sun, I. F., Thorn, S., Lin, Y. C., et al. (2019). An Attribute‐diversity Approach to Functional Diversity, Functional Beta Diversity, and Related (Dis)similarity Measures. Ecol. Monogr. 89, e01343. doi:10.1002/ecm.1343
Chapman, D. S., Scalone, R., Štefanić, E., and Bullock, J. M. (2017). Mechanistic Species Distribution Modeling Reveals a Niche Shift during Invasion. Ecology 98, 1671–1680. doi:10.1002/ecy.1835
Chytrý, M., Jarošík, V., Pyšek, P., Hájek, O., Knollová, I., Tichý, L., et al. (2008). Separating Habitat Invasibility by Alien Plants from the Actual Level of Invasion. Ecology 89, 1541–1553. doi:10.1890/07-0682.1
Cordovez, J. M., and Guhl, F. (2015). The Impact of Landscape Transformation on the Reinfestation Rates of Rhodnius prolixus in the Orinoco Region, Colombia. Acta Tropica 151, 73–79. doi:10.1016/j.actatropica.2015.07.030
Cornelissen, J. H. C., Lavorel, S., Garnier, E., Díaz, S., Buchmann, N., Gurvich, D. E., et al. (2003). A Handbook of Protocols for Standardised and Easy Measurement of Plant Functional Traits Worldwide. Aust. J. Bot. 51, 335–380. doi:10.1071/bt02124
Daneshgar, P., and Jose, S. (2009). Role of Species Identity in Plant Invasions: Experimental Test Using Imperata Cylindrica. Biol. Invasions 11, 1431–1440. doi:10.1007/s10530-008-9351-x
Davis, M. A., Grime, J. P., and Thompson, K. (2000). Fluctuating Resources in Plant Communities: a General Theory of Invasibility. J. Ecol. 88, 528–534. doi:10.1046/j.1365-2745.2000.00473.x
DeGasperis, B. G., and Motzkin, G. (2007). Windows of Opportunity: Historical and Ecological Controls Onberberis Thunbergiiinvasions. Ecology 88, 3115–3125. doi:10.1890/06-2014.1
Di Rienzo, J. A., Casanoves, F., Balzarini, M. G., Gonzalez, L., Tablada, M., and Robledo, C. W. (2020). InfoStat Versión. Argentina: Centro de Transferencia InfoStat, FCA, Universidad Nacional de Córdoba. Available at: http://www.infostat.com.ar.
Dickie, I. A., Bennett, B. M., Burrows, L. E., Nuñez, M. A., Peltzer, D. A., Porté, A., et al. (2014). Conflicting Values: Ecosystem Services and Invasive Tree Management. Biol. Invasions 16, 705–719. doi:10.1007/s10530-013-0609-6
DNP (2018). Plan Nacional de Desarrollo 2018-2022. Pacto por Colombia, pacto por la equidad. XXIV. Pacto Región Llanos-Orinoquia: Conectar y potenciar la despensa sostenible de la región con el país y el mundo. 10p. Available at: https://colaboracion.dnp.gov.co/CDT/Prensa/PactoOrinoquiaPND2018-2022.pdf.
Erazo, D., González, C., Guhl, F., Umaña, J. D., Morales-Betancourt, J. A., and Cordovez, J. (2020). Rhodnius prolixus Colonization and Trypanosoma Cruzi Transmission in Oil palm (Elaeis Guineensis) Plantations in the Orinoco Basin, Colombia. Am. J. Trop. Med. Hyg. 103, 428–436. doi:10.4269/ajtmh.19-0331
Etter, A., Andrade, A., Saavedra, K., Amaya, P., and Arévalo, P. (2017). Estado de los Ecosistemas Colombianos: una aplicación de la metodología de la Lista Roja de Ecosistemas (Vers 2.0). Informe Final. Bogotá: Pontificia Universidad Javeriana y Conservación Internacional-Colombia.
Etter, A. (1998). Mapa General de Ecosistemas de Colombia Escala 1: 2´000.000. Bogotá: IAvH y PNUD. (ISBN 958-96529-1-3).
Etter, A., Sarmiento, A., and Romero, M. H. (2010). “Land Use Changes (1970-2020) and the Carbon Emissions in the Colombian Llanos,” in Ecosystem Function in Savannas. Measurement and Modeling at Landscape to Global Scales. Editors N. P. Hill, and M. J. Hanna (CRC Press), 383–402.
Feng, X., Park, D. S., Liang, Y., Pandey, R., and Papeş, M. (2019). Collinearity in Ecological Niche Modeling: Confusions and Challenges. Ecol. Evol. 9, 10365–10376. doi:10.1002/ece3.5555
Fick, S. E., and Hijmans, R. J. (2017). WorldClim 2: New 1‐km Spatial Resolution Climate Surfaces for Global Land Areas. Int. J. Climatol. 37, 4302–4315. doi:10.1002/joc.5086
Forrestel, E. J., Donoghue, M. J., and Smith, M. D. (2014). Convergent Phylogenetic and Functional Responses to Altered Fire Regimes in Mesic savanna Grasslands of North America and South Africa. New Phytol. 203, 1000–1011. doi:10.1111/nph.12846
Ghannoum, O. (2009). C4 Photosynthesis and Water Stress. Ann. Bot. 103, 635–644. doi:10.1093/aob/mcn093
Gotelli, N. J., and Colwell, R. K. (2001). Quantifying Biodiversity: Procedures and Pitfalls in the Measurement and Comparison of Species Richness. Ecol. Lett. 4, 379–391. doi:10.1046/j.1461-0248.2001.00230.x
Gower, J. C. (1966). Some Distance Properties of Latent Root and Vector Methods Used in Multivariate Analysis. Biometrika 53, 325–338. doi:10.1093/biomet/53.3-4.325
Gross, K. L., Mittelbach, G. G., Mittelbach, G. G., and Reynolds, H. L. (2005). Grassland Invasibility and Diversity: Responses to Nutrients, Seed Input, and Disturbance. Ecology 86, 476–486. doi:10.1890/04-0122
Guisan, A., Petitpierre, B., Broennimann, O., Daehler, C., and Kueffer, C. (2014). Unifying Niche Shift Studies: Insights from Biological Invasions. Trends Ecol. Evol. 29, 260–269. doi:10.1016/j.tree.2014.02.009
Hanley, M. E., Lamont, B. B., Fairbanks, M. M., and Rafferty, C. M. (2007). Plant Structural Traits and Their Role in Anti-herbivore Defence. Perspect. Plant Ecol. Evol. Syst. 8, 157–178. doi:10.1016/j.ppees.2007.01.001
Hierro, J. L., and Callaway, R. M. (2003). Allelopathy and Exotic Plant Invasion. Plant and soil 256, 29–39. doi:10.1023/a:1026208327014
Hijmans, R. J., Phillips, S., Leathwick, J., and Elith, J. (2019). Dismo: Species Distribution Modeling. version 1.3.3. Available at: https://CRAN.Rproject.org/package=dismo/(Accessed January 1, 2019).
Hijmans, R. J. (2020). Raster: Geographic Data Analysis and Modeling. R Package Version 3.3-13. Available at: https://CRAN.R-project.org/package=raster/(Accessed January 1, 2020).
Hobbs, R. J., and Atkins, L. (1988). Effect of Disturbance and Nutrient Addition on Native and Introduced Annuals in Plant Communities in the Western Australian Wheatbelt. Austral Ecol. 13, 171–179. doi:10.1111/j.1442-9993.1988.tb00966.x
Hurlbert, A. H., and White, E. P. (2005). Disparity between Range Map- and Survey-Based Analyses of Species Richness: Patterns, Processes and Implications. Ecol. Lett. 8, 319–327. doi:10.1111/j.1461-0248.2005.00726.x
Huston, M. A. (2004). Management Strategies for Plant Invasions: Manipulating Productivity, Disturbance, and Competition. Divers. Distrib 10, 167–178. doi:10.1111/j.1366-9516.2004.00083.x
Jiménez-Valverde, A., Peterson, A. T., Soberón, J., Overton, J. M., Aragón, P., and Lobo, J. M. (2011). Use of Niche Models in Invasive Species Risk Assessments. Biol. Invasions 13, 2785–2797. doi:10.1007/s10530-011-9963-4
Kempel, A., Chrobock, T., Fischer, M., Rohr, R. P., and van Kleunen, M. (2013). Determinants of Plant Establishment success in a Multispecies Introduction experiment with Native and Alien Species. Proc. Natl. Acad. Sci. U.S.A. 110, 12727–12732. doi:10.1073/pnas.1300481110
Kennedy, T. A., Naeem, S., Howe, K. M., Knops, J. M. H., Tilman, D., and Reich, P. (2002). Biodiversity as a Barrier to Ecological Invasion. Nature 417, 636–638. doi:10.1038/nature00776
Klimes, L., Klimesová, J., Hendriks, R., and Groenendael, J. V. (1997). “Clonal Plant Architecture: a Comparative Analysis of Form and Function,” in The Ecology and Evolution of Clonal Plants. Editor J. Groenendael (Leiden: Backhuys publishers).
Knapp, A. K. (1985). Effect of Fire and Drought on the Ecophysiology of Andropogon Gerardii and Panicum Virgatum in a Tallgrass Prairie. Ecology 66, 1309–1320. doi:10.2307/1939184
Kooyers, N. J., and Olsen, K. M. (2012). Rapid Evolution of an Adaptive Cyanogenesis Cline in Introduced North American white clover (Trifolium repens L.). Mol. Ecol. 21, 2455–2468. doi:10.1111/j.1365-294x.2012.05486.x
Kuo, Y. L. (2003). Ecological Characteristics of Three Invasive Plants (Leucaena Leucocephala, Mikania Micrantha, and Stachytarpheta Urticaefolia) in Southern Taiwan. Taiwan: Food & Fertilizer Technology Center.
Legras, G., Loiseau, N., and Gaertner, J.-C. (2018). Functional Richness: Overview of Indices and Underlying Concepts. Acta Oecologica 87, 34–44. doi:10.1016/j.actao.2018.02.007
Levine, J. M., and D'Antonio, C. M. (1999). Elton Revisited: A Review of Evidence Linking Diversity and Invasibility. Oikos 87, 15–26. doi:10.2307/3546992
Liu, C., Wolter, C., Xian, W., and Jeschke, J. M. (2020a). Species Distribution Models Have Limited Spatial Transferability for Invasive Species. Ecol. Lett. 23, 1682–1692. doi:10.1111/ele.13577
Liu, C., Wolter, C., Xian, W., and Jeschke, J. M. (2020b). Most Invasive Species Largely Conserve Their Climatic Niche. Proc. Natl. Acad. Sci. U.S.A. 117, 23643–23651. doi:10.1073/pnas.2004289117
Lourival, R., Drechsler, M., Watts, M. E., Game, E. T., and Possingham, H. P. (2011). Planning for Reserve Adequacy in Dynamic Landscapes; Maximizing Future Representation of Vegetation Communities under Flood Disturbance in the Pantanal Wetland. Divers. Distributions 17, 297–310. doi:10.1111/j.1472-4642.2010.00722.x
Lozano, J. S., Etter, A., and Sarmiento, A. (2007). Modelamiento espacial de la probabilidad de invasión de palma africana de aceite (Elaeis guineensis) a los bosques de galería del Piedemonte Llanero (Meta). Ambiente y Desarrollo 21, 79–92.
Luo, J., Cui, J., Pandey, S. P., Jiang, K., Tan, Z., He, Q., et al. (2020). Seasonally Distinctive Growth and Drought Stress Functional Traits Enable Leucaena Leucocephala to Successfully Invade a Chinese Tropical forest. Trop. Conservation Sci. 13, 1–7. doi:10.1177/1940082920949176
Mandal, K. G., Misra, A. K., Hati, K. M., Bandyopadhyay, K. K., Ghosh, P. K., and Mohanty, M. (2004). Rice Residue- Management Options and Effects on Soil Properties and Crop Productivity. Food Agric. Environ. 2, 224–231.
Marod, D., Duengkae, P., Kutintara, U., Sungkaew, S., Wachrinrat, C., Asanok, L., et al. (2012). The Influences of an Invasive Plant Species (Leucaena Leucocephala) on Tree Regeneration in Khao Phuluang Forest, Northeastern Thailand. Kasetsart J. Nat. Sci. 46, 39–50.
Martins, V. F., Guimarães, P. R., Haddad, C. R. B., and Semir, J. (2009). The Effect of Ants on the Seed Dispersal Cycle of the Typical Myrmecochorous Ricinus communis. Plant Ecol. 205, 213–222. doi:10.1007/s11258-009-9611-6
Martins, V. F., Haddad, C. R., and Semir, J. (2011). Responses of the Invasive Ricinus communis Seedlings to Competition and Light. New Zealand J. Bot. 49, 263–279. doi:10.1080/0028825x.2010.548069
Mason, N. W. H., Mouillot, D., Lee, W. G., and Wilson, J. B. (2005). Functional Richness, Functional Evenness and Functional Divergence: The Primary Components of Functional Diversity. Oikos 111, 112–118. doi:10.1111/j.0030-1299.2005.13886.x
Matthews, S., and Brand, K. (2004). Africa Invaded: The Growing Danger of Invasive Alien Species. Cape Town, South Africa: Global Invasive Species Programme, 79.
McKinney, M. L., and Lockwood, J. L. (1999). Biotic Homogenization: A Few Winners Replacing Many Losers in the Next Mass Extinction. Trends Ecol. Evol. 14, 450–453. doi:10.1016/S0169-5347(99)01679-1
Meyer, D., and Buchta, C. (2019). Package “Proxy”. R Package Version 0.4-25. Available at: https://cran.r-project.org/web/packages/proxy/index.html.
Mora-Fernández, C., and Peñuela-Recio, L. (2013). Salud ecosistémica de las sabanas inundables asociadas a la cuenca del río Pauto, Casanare, Colombia. Colombia: Yoluka ONG Pencil Work.
Moravcová, L., Pyšek, P., Jarošík, V., and Pergl, J. (2015). Getting the Right Traits: Reproductive and Dispersal Characteristics Predict the Invasiveness of Herbaceous Plant Species. Plos One 10, e0123634. doi:10.1371/journal.pone.0123634
Mosher, E. S., Silander, J. A., and Latimer, A. M. (2009). The Role of Land-Use History in Major Invasions by Woody Plant Species in the Northeastern North American Landscape. Biol. Invasions 11, 2317–2328. doi:10.1007/s10530-008-9418-8
Mouchet, M. A., Villéger, S., Mason, N. W. H., and Mouillot, D. (2010). Functional Diversity Measures: an Overview of Their Redundancy and Their Ability to Discriminate Community Assembly Rules. Funct. Ecol. 24, 867–876. doi:10.1111/j.1365-2435.2010.01695.x
Muscarella, R., Galante, P. J., Soley-Guardia, M., Boria, R. A., Kass, J. M., Uriarte, M., et al. (2014). ENMeval: An R Package for Conducting Spatially Independent Evaluations and Estimating Optimal Model Complexity forMaxentecological Niche Models. Methods Ecol. Evol. 5, 1198–1205. doi:10.1111/2041-210x.12261
Panetta, F. D., and Mitchell, N. D. (1991). Homoclime Analysis and the Prediction of Weediness. Weed Res. 31, 273–284. doi:10.1111/j.1365-3180.1991.tb01767.x
Pearman, P. B., Guisan, A., Broennimann, O., and Randin, C. F. (2008). Niche Dynamics in Space and Time. Trends Ecol. Evol. 23, 149–158. doi:10.1016/j.tree.2007.11.005
Pejchar, L., and Mooney, H. A. (2009). Invasive Species, Ecosystem Services and Human Well-Being. Trends Ecol. Evol. 24, 497–504. doi:10.1016/j.tree.2009.03.016
Petchey, O. L., and Gaston, K. J. (2002). Functional Diversity (FD), Species Richness and Community Composition. Ecol. Lett. 5, 402–411. doi:10.1046/j.1461-0248.2002.00339.x
Peterson, A. T. (2003). Predicting the Geography of Species' Invasions via Ecological Niche Modeling. Q. Rev. Biol. 78, 419–433. doi:10.1086/378926
Petitpierre, B., Kueffer, C., Broennimann, O., Randin, C., Daehler, C., and Guisan, A. (2012). Climatic Niche Shifts Are Rare Among Terrestrial Plant Invaders. Science 335, 1344–1348. doi:10.1126/science.1215933
Phillips, S. J., Anderson, R. P., Dudík, M., Schapire, R. E., and Blair, M. E. (2017). Opening the Black Box: an Open-Source Release of Maxent. Ecography 40, 887–893. doi:10.1111/ecog.03049
Phillips, S. J., Anderson, R. P., and Schapire, R. E. (2006). Maximum Entropy Modeling of Species Geographic Distributions. Ecol. Model. 190, 231–259. doi:10.1016/j.ecolmodel.2005.03.026
Phillips, S. J., and Dudík, M. (2008). Modeling of Species Distributions with Maxent: New Extensions and a Comprehensive Evaluation. Ecography 31, 161–175. doi:10.1111/j.0906-7590.2008.5203.x
Prüssmann, J., Rincón, S. A., Tavera, H. A., and Suárez, C. F. (2020). Estructura ecológica principal de la Orinoquia colombiana - Actualizaciónmetodológica mapa Sulu. Colombia: WWF, 88.
Pyšek, P., and Richardson, D. M. (2007). “Traits Associated with Invasiveness in Alien Plants: Where Do We Stand?,” in Biological Invasions, Ecological Studies 193. Editor W. Nentwig (Berlin & Heidelberg: Springer-Verlag), 97–126.
Quian, H., and Ricklefs, R. E. (2006). The Role of Exotic Species in Homogenizing the North American flora. Ecol. Lett. 9, 1293–1298. doi:10.1111/j.1461-0248.2006.00982.x
R Core Team (2019). R: A Language and Environment for Statistical Computing. Vienna, Austria: R Foundation for Statistical Computing. ISBN 3-900051-07-0. Available at: https://www.R-project.org/(Accessed June 11, 2019).
Rejmánek, M., and Pitcairn, M. J. (2002). “When Is Eradication of Exotic Pest Plants a Realistic Goal,” in Proceedings of the International Conference on Eradication of Island Invasive Species (IUCN SSC Invasive Species Specialist Group, Gland), 249–253.
Ripley, B. S., Gilbert, M. E., Ibrahim, D. G., and Osborne, C. P. (2007). Drought Constraints on C4 Photosynthesis: Stomatal and Metabolic Limitations in C3 and C4 Subspecies of Alloteropsis Semialata. J. Exp. Bot. 58, 1351–1363. doi:10.1093/jxb/erl302
Romero-Ruiz, M. H., Flantua, S. G. A., Tansey, K., and Berrio, J. C. (2012). Landscape Transformations in Savannas of Northern South America: Land Use/Cover Changes Since 1987 in the Llanos Orientales of Colombia. Appl. Geogr. 32, 766–776. doi:10.1016/j.apgeog.2011.08.010
Sala, O. E., Stuart Chapin, F., Iii, J. J., Armesto, J. J., Berlow, E., Bloomfield, J., et al. (2000). Global Biodiversity Scenarios for the Year 2100. Science 287, 1770–1774. doi:10.1126/science.287.5459.1770
Schmidt, M., Thiombiano, A., Zizka, A., König, K., Brunken, U., and Zizka, G. (2011). Patterns of Plant Functional Traits in the Biogeography of West African Grasses (Poaceae). Afr. J. Ecol. 49, 490–500. doi:10.1111/j.1365-2028.2011.01283.x
Shea, K., and Chesson, P. (2002). Community Ecology Theory as a Framework for Biological Invasions. Trends Ecol. Evol. 17, 170–176. doi:10.1016/S0169-5347(02)02495-3
Silvério, D. V., Brando, P. M., Balch, J. K., Putz, F. E., Nepstad, D. C., Oliveira-Santos, C., et al. (2013). Testing the Amazon Savannization Hypothesis: Fire Effects on Invasion of a Neotropical Forest by Native Cerrado and Exotic Pasture Grasses. Phil. Trans. R. Soc. B 368, 20120427. doi:10.1098/rstb.2012.0427
Simberloff, D., Martin, J.-L., Genovesi, P., Maris, V., Wardle, D. A., Aronson, J., Courchamp, F., Galil, B., García-Berthou, E., Pascal, M., Pyšek, P., Sousa, R., Tabacchi, E., and Vilà, M. (2013). Impacts of Biological Invasions: What's What and the Way Forward. Trends Ecology Evolution 28, 58–66. doi:10.1016/j.tree.2012.07.013
Somerfield, P. J., and Clarke, K. R. (2013). Inverse Analysis in Non-Parametric Multivariate Analyses: Distinguishing Groups of Associated Species Which Covary Coherently Across Samples. J. Exp. Mar. Biol. Ecol. 449, 261–273. doi:10.1016/j.jembe.2013.10.002
Srivastava, V., Lafond, V., and Griess, V. C. (2019). Species Distribution Models (SDM): Applications, Benefits and Challenges in Invasive Species Management. CAB Rev. 14, 1–13. doi:10.1079/pavsnnr201914020
Strayer, D. L., Eviner, V. T., Jeschke, J. M., and Pace, M. L. (2006). Understanding the Long-Term Effects of Species Invasions. Trends Ecol. Evol. 21, 645–651. doi:10.1016/j.tree.2006.07.007
Thuiller, W., Richardson, D. M., Pyšek, P., Midgley, G. F., Hughes, G. O., and Rouget, M. (2005). Niche‐Based Modelling as a Tool for Predicting the Risk of Alien Plant Invasions at a Global Scale. Glob. Change Biol. 11, 2234–2250. doi:10.1111/j.1365-2486.2005.001018.x
Uden, D. R., Allen, C. R., Angeler, D. G., Corral, L., and Fricke, K. A. (2015). Adaptive Invasive Species Distribution Models: A Framework for Modeling Incipient Invasions. Biol. Invasions 17, 2831–2850. doi:10.1007/s10530-015-0914-3
van Kleunen, M., Weber, E., and Fischer, M. (2010). A Meta-Analysis of Trait Differences Between Invasive and Non-Invasive Plant Species. Ecol. Lett. 13, 235–245. doi:10.1111/j.1461-0248.2009.01418.x
Vargas, L., Willemen, L., and Hein, L. (2018). Linking Planetary Boundaries and Ecosystem Accounting, with an Illustration for the Colombian Orinoco River Basin. Reg. Environ. Change 18, 1521–1534. doi:10.1007/s10113-018-1282-1
Vásquez-Valderrama, M., González-M, R., López-Camacho, R., Baptiste, M. P., and Salgado-Negret, B. (2020). Impact of Invasive Species on Soil Hydraulic Properties: Importance of Functional Traits. Biol. Invasions 22 (6), 1849–1863. doi:10.1007/s10530-020-02222-8
Vilà, M., and Ibáñez, I. (2011). Plant Invasions in the Landscape. Landscape Ecol. 26, 461–472. doi:10.1007/s10980-011-9585-3
Wang, T., Hu, J., Wang, R., Liu, C., and Yu, D. (2018). Tolerance and Resistance Facilitate the Invasion Success of Alternanthera Philoxeroides in Disturbed Habitats: A Reconsideration of the Disturbance Hypothesis in the Light of Phenotypic Variation. Environ. Exp. Bot. 153, 135–142. doi:10.1016/j.envexpbot.2018.05.011
Whittaker, R. H. (1952). A Study of Summer Foliage Insect Communities in the Great Smoky Mountains. Ecol. Monogr. 22, 1–44. doi:10.2307/1948527
Wiens, J. A., Stralberg, D., Jongsomjit, D., Howell, C. A., and Snyder, M. A. (2009). Niches, Models, and Climate Change: Assessing the Assumptions and Uncertainties. Proc. Natl. Acad. Sci. U.S.A. 106, 19729–19736. doi:10.1073/pnas.0901639106
Williams, D. G., and Baruch, Z. (2000). African Grass Invasion in the Americas: Ecosystem Consequences and the Role of Ecophysiology. Biol. invasions 2, 123–140. doi:10.1023/a:1010040524588
Willis, K. J., and Whittaker, R. J. (2002). Species Diversity–Scale Matters. Science 295, 1245–1248. doi:10.1126/science.1067335
With, K. A. (2002). The Landscape Ecology of Invasive Spread. Conservation Biol. 16, 1192–1203. doi:10.1046/j.1523-1739.2002.01064.x
Keywords: exotic species, functional trait, transformed ecosystems, natural biomes, biological invasion
Citation: Salgado-Negret B, Urbina-Cardona N, Noguera-Urbano EA, Cruz-Rodríguez CA and Baptiste MP (2022) Predicting the Risk of Exotic Plant Invasions in the Orinoco Region: Importance of Distribution Models, Climatic Niche and Functional Richness. Front. Environ. Sci. 10:687723. doi: 10.3389/fenvs.2022.687723
Received: 29 March 2021; Accepted: 29 March 2022;
Published: 16 May 2022.
Edited by:
Glenn Hyman, Freelance Geographer, ColombiaReviewed by:
Silvia J. Alvarez, Wildlife Conservation Society, ColombiaCopyright © 2022 Salgado-Negret, Urbina-Cardona, Noguera-Urbano, Cruz-Rodríguez and Baptiste. This is an open-access article distributed under the terms of the Creative Commons Attribution License (CC BY). The use, distribution or reproduction in other forums is permitted, provided the original author(s) and the copyright owner(s) are credited and that the original publication in this journal is cited, in accordance with accepted academic practice. No use, distribution or reproduction is permitted which does not comply with these terms.
*Correspondence: Beatriz Salgado-Negret, YnNhbGdhZG9uQHVuYWwuZWR1LmNv; María Piedad Baptiste, bXBiYXB0aXN0ZUBodW1ib2xkdC5vcmcuY28=
Disclaimer: All claims expressed in this article are solely those of the authors and do not necessarily represent those of their affiliated organizations, or those of the publisher, the editors and the reviewers. Any product that may be evaluated in this article or claim that may be made by its manufacturer is not guaranteed or endorsed by the publisher.
Research integrity at Frontiers
Learn more about the work of our research integrity team to safeguard the quality of each article we publish.