- 1Department of Environmental Studies for Advanced Society, Graduate School of Environmental Studies, Tohoku University, Sendai, Japan
- 2Geotechnical and Geosphere Research Group, Saitama University, Saitama, Japan
Enhanced weathering of industrial Ca-rich silicate byproducts in croplands is potentially profitable for large-scale atmospheric CO2 removal; during the weathering process, CO2 dissolves to form HCO3− and CO32− in alkaline soil pore water, which eventually flows into the ocean. However, the effectiveness of such systems is still in doubt, owing to the unrealistic models used for prediction and the insufficient consideration of the dynamic influences of soils on fluid chemistry. We determined the effectiveness of such systems for atmospheric CO2 removal, along with their characteristics, through a set of batch- and flow-through-type laboratory experiments, using andosol and decomposed granite soil as agricultural and non-agricultural soils, respectively, and Portland cement, steelmaking slag, and coal fly ash as industrial byproducts. The results of the batch-type experiments demonstrated that agricultural soils were suitable for CO2 removal, owing to their moderately high pH and Ca concentrations in pore water that prevented intensive calcium carbonate precipitation. The flow-through experiments demonstrated that a higher Ca-content byproduct can have a large atmospheric CO2 removal capacity. However, the magnitude of CO2 removal and its time-dependent behavior were difficult to predict because they were not in conjunction with the changes in the average pH value. This indicated that the diffusive transport of CO2 from the atmosphere-soil interface to deeper soils was more complex than expected. Maximizing CO2 removal requires a better understanding of the diffusive transport of CO2 through gas-filled pore spaces, created by unsteady-state air–water two-phase flow, due to intermittent rainfall.
1 Introduction
Based on the Paris Agreement, the United Nations Intergovernmental Panel on Climate Change (IPCC) aims to limit anthropogenic global warming; for this, it is imperative to consider large-scale atmospheric carbon dioxide (CO2) removal (Hansen et al., 2017; Rockström et al., 2017; IPCC 2018; Bach et al., 2019). The atmospheric CO2 removal technologies recently developed focus on the use of soil and vegetation for atmospheric CO2 removal (Green et al., 2019; Humphrey et al., 2021; Terrer et al., 2021; Zeng et al., 2022). In these reports, enhanced weathering of calcium (Ca) and/or magnesium (Mg) silicate rocks/minerals applied in croplands has shown potential for atmospheric CO2 removal. Such enhanced weathering also has the possible co-benefits of improved food and soil security and reduced ocean acidification (Kantola et al., 2017; Zhang G. et al., 2018; Beerling et al., 2018, 2020; Bach et al., 2019; Goll et al., 2021).
A recent study by Beerling et al. (2020) suggested the possibility of large-scale profitable atmospheric CO2 removal, through the enhanced weathering of industrial Ca/Mg-rich silicate byproducts in croplands. Industrial silicate byproducts, including steelmaking slag, waste cement, and coal fly ash (Lam et al., 2000; Jorat et al., 2020; Abdel-Gawwad et al., 2021; Klemettinen et al., 2021), have been used in the agricultural sector to supplement minerals to the soil (Das et al., 2019). Based on a one-dimensional (1D) vertical reactive transport model simulation, Beerling et al. (2020) estimated that an average global atmospheric CO2 removal of 0.5–2 Gt/year may be achieved by applying enhanced weathering in various countries, such as China, India, Brazil, and the United States of America (United States), with the costs being approximately USD 80–180 per ton of CO2. In this CO2 removal process, CO2 is captured as HCO3− and CO32- in alkaline soil pore water, resulting from the fast dissolution of fine-grained Ca/Mg-rich silicates that eventually flow into the ocean. For instance, calcium silicate (CaSiO3) dissolves in soil pore water, which can be expressed as the following equation:
In this reaction, protons (H+) are consumed, facilitating the dissolution of atmospheric CO2 into pore water. This reaction can be expressed as follows:
However, in this atmospheric CO2 removal process, carbonate minerals, such as CaCO3, may be generated under conditions of high pH (i.e., low solubility of carbonate minerals in water) and high concentrations of Ca2+/Mg2+ and CO32-, as shown in Eq. 3:
Ca- and Mg-rich silicate materials have another role in CO2 removal; they serve as conditioners for Ca- and Mg-depleted soils, respectively. Therefore, intensive formation of carbonate minerals is not desirable for croplands, because they reduce the readily available Ca/Mg for plants. Additionally, this may result in the dissolution of carbonate minerals, consequently resulting in the release of CO2 into the atmosphere; notably, this may occur unless the pH of the pore water is high enough to limit carbonate dissolution.
Croplands have been considered as good candidates for the application of large-scale profitable atmospheric CO2 removal technologies (Beerling et al., 2020). However, the existing studies do not determine the properties of croplands that contribute to atmospheric CO2 removal and the CO2 removal pathways and mechanisms in croplands. Moreover, the dynamic influences of cropland soils on fluid chemistry, such as the kinetics of pH buffering and cation exchange, have been rarely considered (Russell et al., 2006; Nelson and Su 2010; Torrent et al., 2015; Wuenscher et al., 2015; Baquy et al., 2018; Dai et al., 2018). The steady-state single-phase (water) flow in a soil, with a static distribution of gas-phase CO2 concentration, is not realistic, as water inputs to croplands by rainfall intermittently cause an unsteady-state air–water two-phase flow in soils that have a dynamic distribution of gas-phase CO2 concentration.
Notably, it is unclear whether agricultural soils can quickly achieve moderately high pH and effective Ca and Mg concentrations in soil pore water, required to prevent the intensive precipitation of carbonate minerals, even when highly reactive Ca/Mg-rich silicate materials react with the pore water. It is also unclear whether the CO2 removal behavior can be predicted based only on the average changes in the chemistry of the pore water, while neglecting the spatially variable distribution of the gas phase, due to the unsteady-state air–water two-phase flow. In this study, we aimed to clarify these points through a set of batch- and flow-through-type laboratory experiments on atmospheric CO2 removal, using powders of Portland cement, steelmaking slag, and coal fly ash (as representatives of industrial Ca-rich silicate materials) and andosol and decomposed granite (DG) soil (as representatives of agricultural and non-agricultural soils). Batch-type experiments conducted using both agricultural and non-agricultural soils revealed the effectiveness of applying silicate materials, particularly to croplands. Flow-through-type experiments conducted using agricultural soil, through which vertical water flow occurred intermittently, were used to investigate the characteristics of atmospheric CO2 removal.
2 Materials and methods
2.1 Soils and Ca-rich silicate materials
In this study, andosol and DG soils, which form naturally and are commercially available in Japan, were used as representatives of agricultural and non-agricultural soils, respectively (Figure 1). Andosol, known as Kuroboku soil in Japan, mainly consists of volcanic ash and humus. It is distributed over ∼31% of Japan’s land area, and covers ∼47% of the country’s crop fields. Table 1 lists the major element concentrations and total organic carbon (TOC) of the soils used in this study.
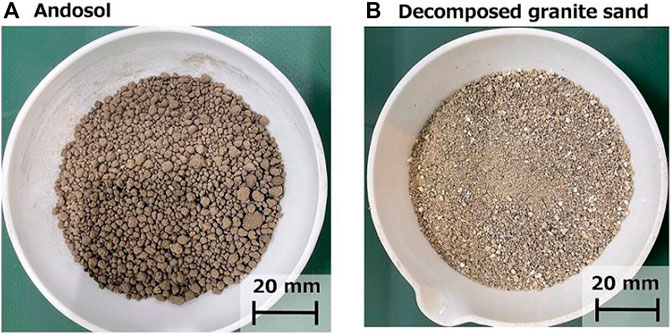
FIGURE 1. Photos of (A) andosol and (B) decomposed granite soil sample before sieving; sieving was carried out to adjust the particle size for the experiment.
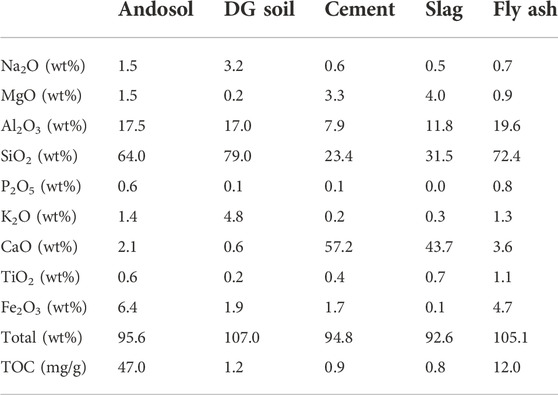
TABLE 1. Major element concentrations and total organic carbon (TOC) in the andosol, decomposed granite (DG) soil, Portland cement, steelmaking slag, and coal fly ash samples used in this study.
The elemental concentration and TOC of the soils and Ca-rich silicate materials were determined using an energy dispersive X-ray fluorescence analyzer (EDX-720, Shimadzu Corporation, Kyoto, Japan) and an organic elemental analyzer (vario MACRO cube, Elementar, Germany). In general, both andosol and DG soils are particularly rich in Si and Al. However, andosols have higher TOC concentrations, as the soil contains substantial amounts of humus, indicating high pH buffering and cation exchange abilities, which contribute to atmospheric CO2 removal, without intensive CaCO3 precipitation. X-ray diffraction (XRD) analysis was conducted to analyze the soils and Ca-rich silicate materials using an X-ray diffractometer (MiniFlex, Rigaku Corporation, Japan) with Cu-Kα radiation, from 10° to 70° (with a step size of 2θ), at 40 kV and 15 mA. The XRD patterns shown in Figure 2 reveal that the dominant crystal phases are albite (NaAlSi3O8), gibbsite (Al(OH)3), and quartz (SiO2) for andosol and albite and quartz for DG soil, indicating no or negligible amounts of carbonate minerals in the two types of soils. Both soils were air-dried, and particles of sizes <2 mm, segregated by sieving, were selected for the experiments. Figure 3 portrays the particle size distributions for both soils.
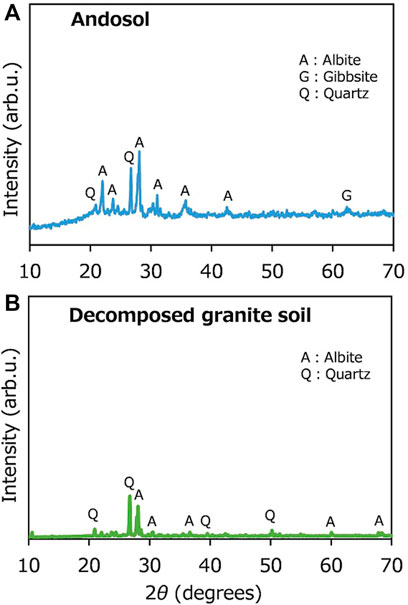
FIGURE 2. X-ray diffraction patterns observed in the experiment for (A) andosol and (B) decomposed granite soil.
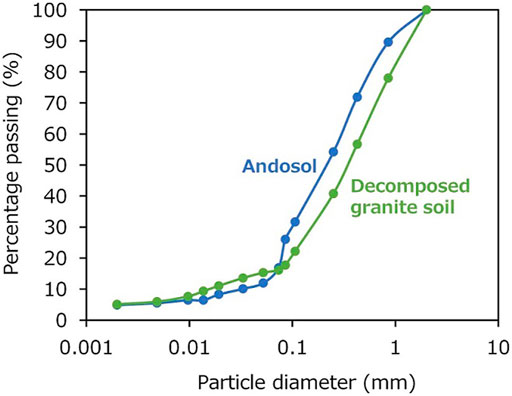
FIGURE 3. Particle size distribution curves for andosol and decomposed granite soil after sieving, to adjust the particle size of the soil samples for the experiment.
In this experimental study, Portland cement, steelmaking slag, and coal fly ash were used as representative Ca-rich industrial byproducts (Figure 4). These materials were air-dried and used in the experiments without particle size adjustment.
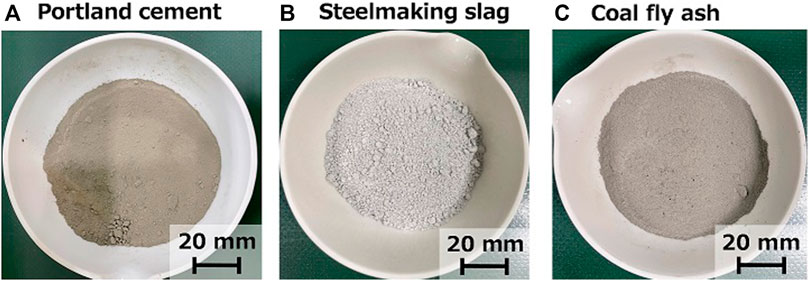
FIGURE 4. Photos of (A) Portland cement, (B) steelmaking slag, and (C) coal fly ash powders used in this study.
2.2 Experimental system, procedures, and conditions
2.2.1 Bach type experiment for atmospheric CO2 removal
In the batch-type experiment, we used 600 ml of Milli-Q water (pH ∼7), with/without 120 g of soil, and/or 6 g of Ca-rich silicate material was put into a cylindrical polypropylene tank (wall thickness: 0.2 mm, outer diameter and height: 11.5 cm); all contents were mixed well, using a stainless-steel stirrer, at 25°C for 2 h, during which the pH of the suspension was measured every 10 min, using a pH meter (HM-31P, DKK-TOA Corporation, Japan). Table 2 lists the combinations of soil and Ca-rich silicate materials used in each experiment.
After 2 h of mixing, a part of the solution suspension was filtered through a 0.45-μm membrane to obtain the solution, and the concentrations of Al, Si, and Ca (which were high in the raw solid materials) were measured using an inductively coupled plasma optical emission spectrometer (ICP-OES) (Agilent 5,110, Agilent Scientific Instruments, United States). The concentrations of TOC and inorganic carbon (IC) were measured using a TOC analyzer (Sensing eye 332, Techno Morioka Co., Ltd, Yamagata, Japan). Higher TOC and IC values reflect higher concentrations of organic compounds eluted from the raw solid materials into the solution and the CO2 species absorbed from the atmosphere into the solution. Thermogravimetry (TG) in air was also conducted on the soils. Additionally, after the experiment, selected mixtures of soil and Ca-rich materials were dried at 80°C for 24 h, to confirm CaCO3 precipitation; for this, we used a differential thermogravimetric analyzer (Thermo plus EVO TG 8120, Rigaku Corporation, Tokyo, Japan), for a range of temperatures from room temperature (25°C) to 1,000°C, at a heating rate of 10°C/min (Wang et al., 2022). All experiments were only conducted for once, but the measurements were conducted for three times for ICP-OES, with a good reproducibility within a margin of error of 3%.
2.2.2 Flow-through type experiment for atmospheric CO2 removal
Two kinds of flow-through type experiments were conducted at 25°C, by sprinkling Milli-Q water on the andosol sample packed in a cylindrical container having a discharge port at its bottom, with the Ca-rich silicate material distributed uniformly on the soil surface (Figure 5). The cylindrical container was made of polypropylene and had a wall thickness of 0.5 cm, outer diameter of 12.0 cm, and outer height of 14.0 cm. We placed 600 g of commercially available natural quartz sand (particle diameter: 1.2–2.4 mm) at the bottom of the container, which served as a highly permeable layer of approximately 3 cm; the layer facilitated the sampling of different effluents for chemical analyses. Note that a filter paper was placed at the outlet port, so that the quartz sand remained inside the container. The highly permeable layer enabled the collection of all the water that passed through the soil within several minutes. Next, 600 g of andosol was placed above the quartz sand layer to form a ∼9-cm-thick soil layer. Milli-Q water (400 ml, pH ∼7) was sprinkled onto the soil surface to create an initial air–water two-phase condition, where the volumetric water saturation of the soil was approximately 50%.
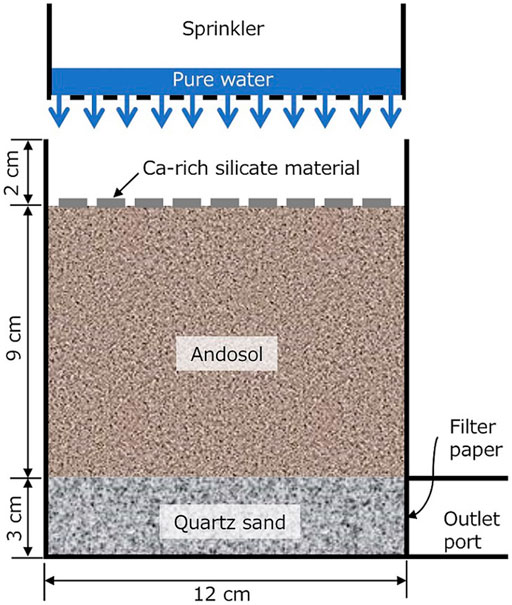
FIGURE 5. Schematic illustration of the flow-through type atmospheric CO2 removal experiment conducted in this study.
After making this initial condition, one of the flow-through-type experiments was conducted in a closed 40-cm cubic box. Milli-Q water (100 ml) was sprinkled onto the soil surface at a rate of ∼60 ml/s, with or without 30 g of Portland cement, steelmaking slag, or coal fly ash. After the water flow from the outlet port stopped, the atmospheric concentration of CO2 in the box was measured (with the outlet port plugged every 10 min for 600 min), using a portable gas monitor (GX-6000, Riken Keiki Co., Ltd. Japan), which was also placed in a closed box.
In contrast, after developing the initial condition, the other flow-through type experiment was conducted, by intermittently sprinkling Milli-Q water, without using the closed box. Milli-Q water (100 ml) was sprinkled twice per day (10:00 and 16:00) for 5 days. For every sprinkle, the outlet port of the container was unplugged to collect effluent. Each fluid sample was first, filtered using a membrane filter and then analyzed for pH, elemental concentration, TOC, and IC.
3 Results
3.1 Effectiveness of the model for atmospheric CO2 removal
Cement was first used to mix with andosol and DG soil in batch experiments. The composition of the cement is shown in Table 1. It was rich in Si, Ca, and Al, with the CaO concentration of 57.2 wt%. The XRD patterns shown in Figure 6 revealed that the dominant crystal phases were alite (Ca3SiO5), brownmillerite [Ca2(Al,Fe)2O5], and calcium silicon aluminum sulfur oxide [Ca2(Si,Al,S)O4]. There were no or negligible amounts of carbonate minerals in cement. Figure 7A portrays the pH changes with time in the batch-type experiments for the soil samples mixed with cement. In the experiments conducted using only water (Run 1), andosol (Run 2), and DG soil suspensions (Run 6), the pH initially decreased to ∼7 within 30 min and then was almost stable at pH 6.0 in Runs 1 and 2 and pH 6.5 in Run 6. In the experiment conducted using only Portland cement (Run 10), the pH was initially much higher (∼pH 12) and remained almost constant throughout the experiment. In contrast, in the experiment conducted using andosol and cement (Run 3), the pH was initially ∼11, which was lower than that in Run 10, and gradually decreased to approximately 10, demonstrating the quick and substantial pH buffering ability of andosol. The increased pH due to mixing cement into andosol may enhance the leaching of humic acids from soil, resulting in a high dissolved TOC concentration, as shown in Figure 7B (Runs 2 and 3). Notably, the concentration of IC (used as a proxy of the total concentrations of CO2 species) tended to be higher for the experiment with a high final pH, rather than being positively correlated to the pH (Figure 7A). The concentration of IC in the experiment conducted using andosol and cement (Run 3) was 3.1 times higher than that conducted using andosol alone (Run 2); for DG soil, adding cement only increased the IC in the solution by 1.7 (Runs 6 and 7).
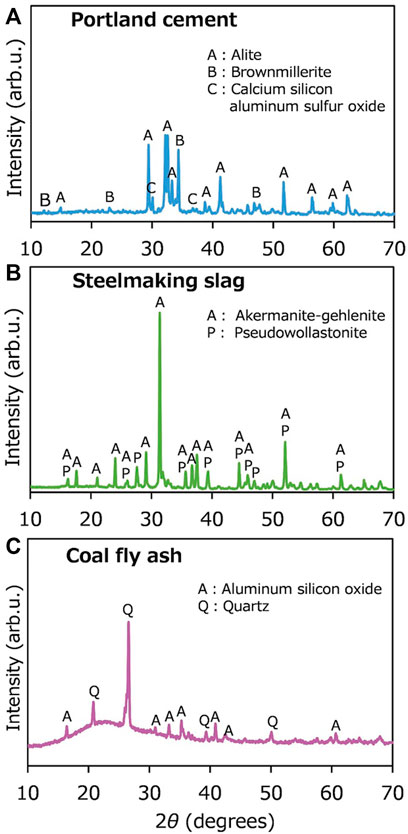
FIGURE 6. X-ray diffraction patterns observed for (A) Portland cement, (B) steelmaking slag, and (C) coal fly ash.
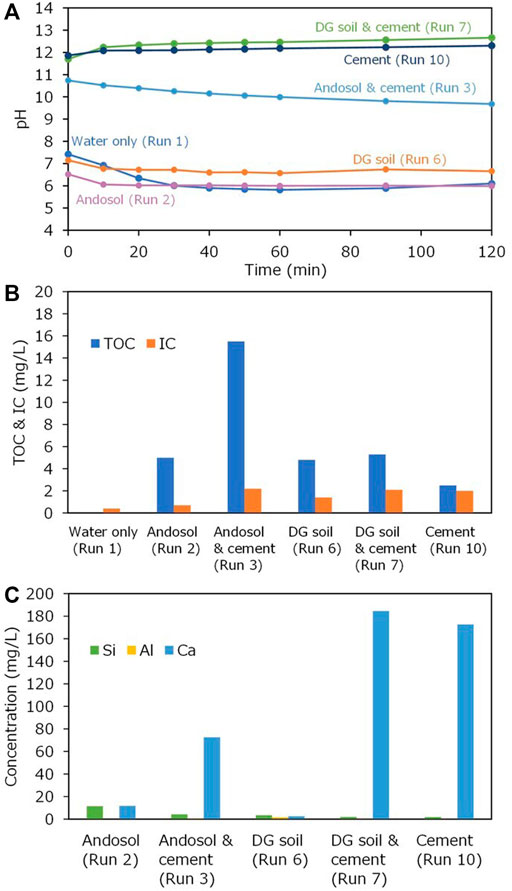
FIGURE 7. (A) Changes in pH with time during the batch-type experiments (Runs 1, 2, 3, 6, 7, and 6); (B) total organic carbon (TOC) and inorganic carbon (IC) after the batch-type experiments; and (C) Si, Al, and Ca concentrations for the liquid samples obtained after the experiments.
Figure 7C portrays the main elemental concentrations of the solution after the batch-type experiments. The Ca concentration in the experiment conducted using andosol and cement (Run 3) was much lower than in that conducted using cement alone (Run 10); this demonstrated the substantial cation exchange capacity of andosol, which was consistent with the results of Madeira et al. (2003). When DG soil was used, the addition of cement resulted in a higher concentration of Ca. It is implied that a large difference in the Ca concentration in the experiments conducted using andosol and DG soil with cement (Runs3 and 7) occurred in the early stage of the experiment, considering the large difference in the effect of cement addition on the IC concentrations in the experiments.
Figure 8A compares the TG curves for the solids collected from the experiments conducted using andosol with and without cement (Runs 2 and 3, respectively). For both samples, most weight loss occurred below 500°C, resulting in qualitatively similar TG curves. The less weight loss in the experiment conducted using andosol with cement (Run 3) may be attributed to the elution of more TOC, e.g., humic acids from andosol. In general, the decomposition of amorphous and crystalline calcium carbonates is known to occur at ∼600–800°C (Kimura and Koga, 2011; Schmidt et al., 2014; Zhang J. et al., 2018; Rao et al., 2019). Therefore, we could confirm that the precipitation of calcium carbonate did not occur intensively in the experiment conducted using andosol and cement (Run 3). In contrast, the TG and DTG curves for the solids collected from the experiment conducted using DG soil with cement (Run 7) portrayed an additional weight loss at 600–700°C, which was not observed in the experiment conducted using DG soil alone (Run 6) (Figure 8B). Therefore, we could verify that precipitation of calcium carbonate occurred in the experiment conducted using DG soil and cement (Run 7). The generation of calcium carbonates also explains the smaller increase in the IC, resulting from the addition of cement to the DG soil.
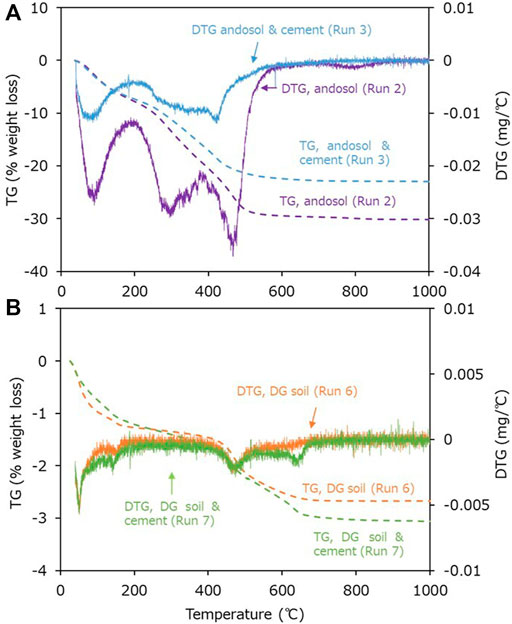
FIGURE 8. Thermogravimetry (TG) curves for the solid samples in the experiments using (A) andosol without and with Portland cement (Runs 2 and 3) and (B) decomposed granite (DG) soil without and with the cement (Runs 6 and 7).
The feasibility of using steelmaking slag and coal fly ash was then investigated. As shown in Table 1, the CaO concentrations in slag and fly ash were 43.7 wt% and 3.6 wt%, respectively. The slag and fly ash samples were also rich in Si, Ca, and Al. The XRD patterns shown in Figure 6 revealed that the dominant crystal phases were åkermanite-gehlenite [Ca2(MgAl) ((Si,Al)2O7)] and pseudowollastonite (CaSiO3) for slag, and aluminum silicon oxide (xAl₂O₃·ySiO₂·zH₂O) and quartz for fly ash. Carbonate minerals were not found. The effects of cement, slag and fly ash on the pH changes, TOC and IC, and elemental concentrations in the batch-type atmospheric CO2 removal experiments conducted using andosol were compared in Figure 9 (Runs 2–5). The general trend in pH change was similar in all experiments conducted using Ca-rich silicate material, i.e., the pH portrayed a decrease in the initial stage and became stable with the reaction time. However, the pH was higher in the samples containing cement, slag, and fly ash (in this sequence). Additionally, the TOC concentration was higher at higher final pH, because of the more intensive elution of humic acids from andosol. The IC concentration was higher in the order of: samples containing slag, cement, flyash, and andosol alone (IC of 3.8, 2.2, 1.2, and 0.7 mg/L, respectively), demonstrating the effectiveness of the experiments for atmospheric CO2 removal, for all silicate materials tested in the present study. The inconsistency between the orders of the pH and IC values may be due to the relatively larger amount of calcium carbonate formation after the addition of cement, considering the much higher Ca concentrations of the sample (Figure 9C).
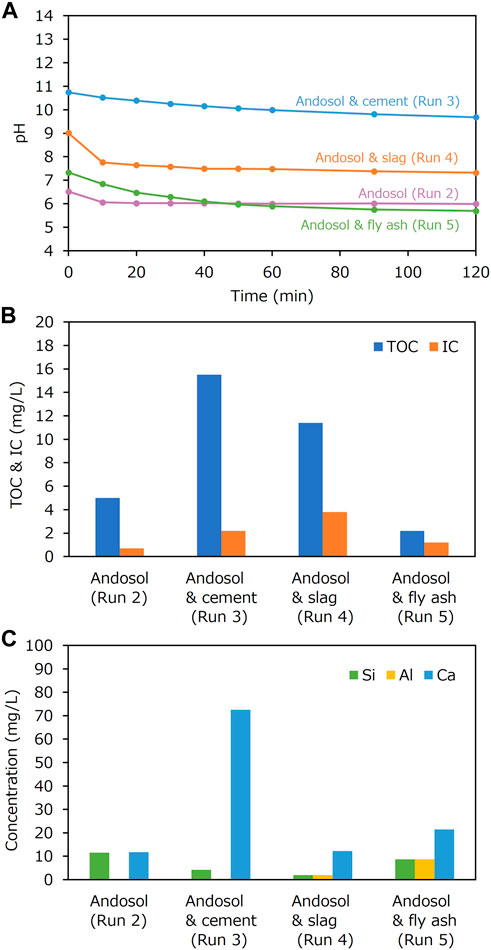
FIGURE 9. (A) Changes in pH with time during the batch-type experiments (Runs 2–5); (B) total organic carbon (TOC) and inorganic carbon (IC) concentrations and (C) Si, Al, and Ca concentrations in the liquid samples, after the experiments.
Based on the above discussion, we can verify that agricultural soil with high humic acids contents, such as andosol, can quickly achieve moderately high pH and Ca concentrations in soil pore water due to the cation exchange effects, thus preventing intensive precipitation of carbonate minerals, even when highly reactive Ca-rich silicate materials, such as cement, react with the pore water. Additionally, the silicate materials tested in this study can be applied in croplands to enhance the removal of atmospheric CO2.
3.2 Rate-limiting process observed in this study
Figure 10 portrays the temporal change in atmospheric CO2 concentration from its initial value within the closed box in the flow-through type experiments for different combinations of andosol and Ca-rich silicate material. The initial CO2 concentrations in the experiments conducted using andosol, without and with Portland cement, steel making slag, and coal fly ash were 460, 560, 460, and 500 ppm, respectively. In the experiment conducted using andosol alone, the decrease in the CO2 concentration ceased within 60 min, resulting in a small reduction of 20 ppm. In contrast, in the experiment conducted using andosol with Ca-rich silicate material, the CO2 concentration decreased continuously throughout the 600-min (10-h) experiment, achieving a larger reduction (>100 ppm). The change in the CO2 concentration was highly nonlinear for all conditions; most changes occurred within the first 360 min (6 h), achieving stability with time. Additionally, the CO2 concentration change reduction extent can be arranged in increasing order: cement, fly ash, and slag.
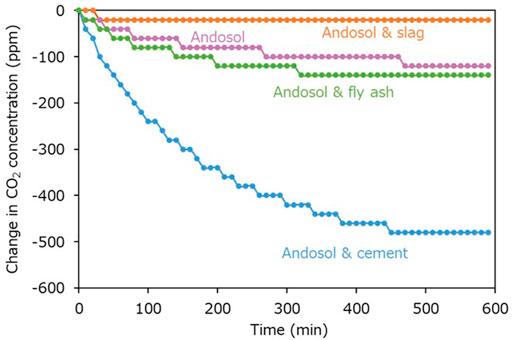
FIGURE 10. Changes in atmospheric CO2 concentration from its initial value in the flow-through type experiments. The changes in CO2 concentration were calculated by subtracting the initial CO2 concentration from the measured CO2 concentration during the reaction. The reduced CO2 was captured by the reaction system.
3.3 Behavior of atmospheric CO2 removal process
Figure 11 portrays the changes in the TOC, IC, and Ca and Si concentrations in and pH of the effluents in the flow-through type experiments conducted in an unclosed box (as a function of total water addition). Notably, water was added at 10:00 and 16:00, with the time interval being 6 h at the maximum. This means fresh water was added after the point when atmospheric CO2 removal by preexisting water almost ceased, based on the results of the previous section (Figure 11).
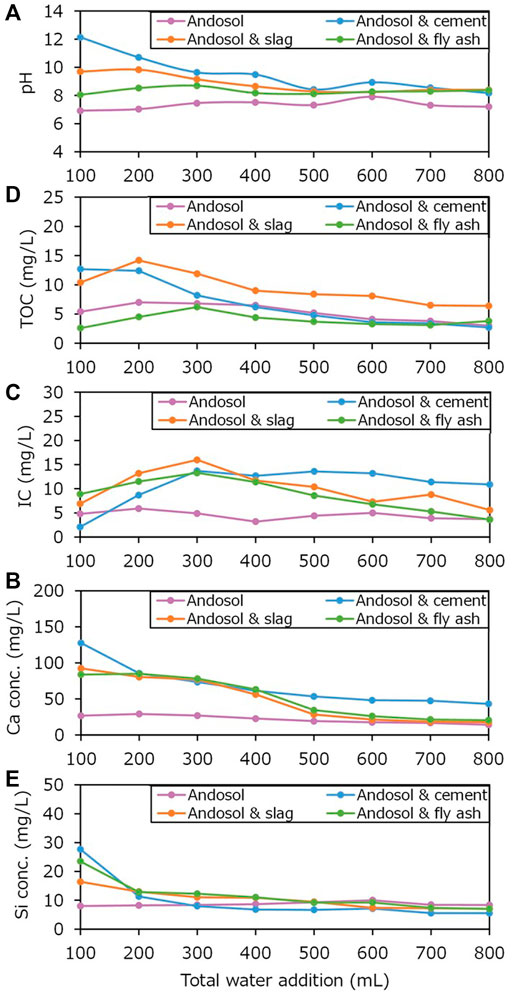
FIGURE 11. Changes in (A) pH of and (B) total organic carbon (TOC), (C) inorganic carbon (IC), and (D) Ca and (E) Si concentrations in the effluents in the flow-through type experiments, without the closed box, as a function of total water addition.
The pH values of the effluents obtained from the experiments conducted using andosol mixed with Portland cement, steelmaking slag, and coal fly ash were generally higher than those conducted using andosol alone (Figure 11A), wherein the pH tended to be larger (in the order of cement, slag, and fly ash), which was consistent with the results of the batch-type experiments (Runs 2–5). The IC in the experiment conducted using andosol alone portrayed a consistent trend, generally smaller than that observed in the experiments conducted using andosol and silicate materials (Figure 11B). In the experiments conducted using andosol and silicate materials, the IC first increased, until 300 ml of total water was added, followed by a decrease. Moreover, the order of magnitude of the IC was not constant throughout the experiment. There was no clear relationship between the changes in the pH and IC of the effluents. Nevertheless, the sum of the product of each IC value and the added water amount (0.1 L) differed (cement: ca. 8.6 mg, slag: ca. 8.0 mg, fly ash: ca. 6.9 mg). Therefore, the total CO2 removal tended to be larger for a higher Ca content (or higher pH). The changes in TOC also had no clear relationship with the changes in pH (Figure 11C). In contrast, the Ca and Si concentrations showed a general decreasing trend, and were thus related to the pH; this reflected the consumption of Ca-rich materials, with increasing total water addition, due to dissolution.
It was not difficult to understand the reason for the overall changes in the pH and the Ca and Si concentrations of the effluents, all of which were caused primarily by the reaction between water and Ca-rich silicate materials distributed uniformly on the soil surface. In contrast, it was quite difficult to understand the reason for the overall changes in the IC and TOC, which were primarily caused by the reactions between water and air and water and soil grains within pore spaces, despite the fact that the IC and TOC concentrations tended to be larger at higher pH, as demonstrated in the batch-type experiments (Figure 9). Empirically, the flow of water in the experiments conducted using slag and fly ash decreased with increasing water (that was added to promote dissolution), compared to that in the experiment conducted using cement, in which higher IC values were observed in the later stages of the experiment. This implies the importance of the spatially variable distribution of the gas phase and the corresponding distribution of the liquid phase, owing to the intermittently occurring air–water two-phase flows in soils.
4 Discussion
The batch experimental results in this study infer that the use of Ca-rich silicate byproducts facilitates the capture of atmospheric CO2 in both the andosol and DG soil systems, due to the pH increase caused by silicates dissolution. However, in andosol, due to the high humic acid content in the pore water, the Ca2+ released from cement was immediately trapped through the cations exchange effect. The exchange of Ca2+ with H+ in humic acid contributes to the released of H+ into solution, restoring the pH of the solution to neutral and avoiding the precipitation of CaCO3, as evidenced by TG measurement. The released of humic acid from andosol soil under alkaline condition, as confirmed by TOC and TG measurements, may also enhance the migration of Ca. Conversely, in DG soil with less humic acid, the Ca2+ released from cement reacted with dissolved CO2 to generate carbonates.
In the flow-through type experiments, the trend of CO2 concentration change shown in Figure 10 indicated that atmospheric CO2 was removed by the dissolution in the soil pore water, with an elevated pH that resulted from the dissolution of Ca-rich silicate material. Based on the results of the batch-type experiments, a large pH increase occurred immediately after the initiation of the contact between water and the Ca-rich materials, and the continuous decrease in the CO2 concentration demonstrated that the gas-phase diffusive transport of CO2 (from the atmosphere into the air-filled pore spaces) in the soil samples was the rate-limiting process of atmospheric CO2 removal. Additionally, the nonlinear changes in the CO2 concentration for all conditions indicated that the diffusive transport of CO2 occurred in an unsteady state.
This study also demonstrated that the absorption behavior of atmospheric CO2 by soil pore water cannot be predicted based only on the average changes in the chemistry of the pore water (such as pH), while neglecting the spatially variable distribution of the gas phase and the corresponding distribution of the liquid phase, owing to the unsteady-state air–water two-phase flow. As shown in Section 3.2, the unsteady-state gas-phase diffusive transport of CO2 from the atmosphere into the air-filled pore spaces in soils is a rate-limiting process of atmospheric CO2 removal. To maximize CO2 removal in croplands via the enhanced weathering of Ca-rich industrial byproducts, it is necessary to better understand the gas-phase diffusive transport behavior of CO2 from the atmosphere to the soil and through the soil; notably, this process is affected by the size and spatial distributions of gas-filled pore spaces in the soil, developed by unsteady-state air–water two-phase flow, due to intermittently occurring rainfall, all of which were not considered in the previous study (Beerling et al., 2020).
Furthermore, in the natural environment, the compositions of terrestrial rain could be complicated and vary significantly from place to place because the regional geology and human activities can greatly affect the types of particulates that get added to the atmosphere. The presence of sources of gaseous acids (SO3, NO2) and bases (NH3) may contribute to Ca-rich silicate byproducts dissolution and affect atmospheric CO2 dissolution, while the presence of metal ions may occupy the position in pore water of agriculture soil for Ca capture. Finally, before applying the system, the potential risks from the use of industrial byproducts, which may contain potentially toxic elements, to human and agriculture should be clarified. All the above factors may influence the experimental results and should be further investigated for practical application of the system.
5 Conclusion
In previous studies, enhanced weathering of industrial Ca-rich silicate byproducts in croplands has been suggested for large-scale profitable atmospheric CO2 removal, through 1D vertical reactive transport model simulation (Beerling et al., 2020). However, considering the lack of realism of this model, in this study, we attempted to clarify the effectiveness and characteristics of such CO2 removal systems and illuminate the dynamic influences of soils on fluid chemistry, such as kinetics of pH buffering and cation exchange, through batch-type and flow-through type laboratory experiments.
Experimental results suggest that agricultural soils, such as andosol are suitable for CO2 removal as they provide moderately high pH and Ca concentrations in pore water, which can prevent intensive carbonate mineral precipitation. The IC concentration in the batch experiment conducted using andosol and cement was 3.1 times higher than that conducted using andosol alone. The flow-through experiments conducted in this study, using intermittent vertical water flow, indicated that greater atmospheric CO2 removal (>100 ppm) may be expected for materials having higher Ca content. However, the magnitude of CO2 removal and its time-dependent behavior are difficult to predict because they have no clear relation to the changes in the average pH value of soil pore water, probably due to the unexpectedly complex unsteady-state diffusive transport of CO2 from the atmosphere-soil interface to deeper soils; notably, this is a rate-limiting process. To maximize atmospheric CO2 removal in croplands via the enhanced weathering of industrial Ca-rich silicate byproducts, further studies are required to better understand the diffusive transport of CO2 through gas-filled pore spaces. Notably, the diffusive nature of the transport is created by the unsteady-state air–water two-phase flow resulting from intermittently occurring rainfall.
Data availability statement
The raw data supporting the conclusions of this article will be made available by the authors, without undue reservation.
Author contributions
KN and NW: conceptualization. RY and KN: data curation. RY and RS: formal analysis. KN, NW, and JW: funding acquisition. RY, KN, and NW: investigation. RY and KN: methodology. KN and NW: project administration. RY and KN: resources. RY and KN: software and writing-original draft. NW and JW: writing-reviewing and editing. All authors contributed to the article and approved the submitted version.
Funding
This study was partially supported by the Japan Society for the Promotion of Science (JSPS) through Grants-in-Aid for Scientific Research (B) (no. 22H02015), Challenging Research (Pioneering) (no. 21K18200), Early-Career Scientists (no. 21K14571), and Scientific Research (S) (no. 22H04932). This study was also supported by the Japan Science and Technology Agency (JST) and the Japan International Cooperation Agency (JICA) through JST/JICA Science and Technology Research Partnership for Sustainable Development (SATREPS) program (no. JPMJSA1703), by the Sumitomo Foundation through the Grant for Environmental Research Projects (no. 203137). The authors declare that this study received funding from Asahi Group Foundation, Ltd. through the Grant for Scientific Research Projects. This funder was not involved in the study design, collection, analysis, interpretation of data, the writing of this article, or the decision to submit it for publication.
Acknowledgments
We would like to thank Shinichi Yamasaki at the Graduate School of Environmental Studies, Tohoku University, for their assistance during the ICP-OES analyses.
Conflict of interest
The authors declare that the research was conducted in the absence of any commercial or financial relationships that could be construed as a potential conflict of interest.
Publisher’s note
All claims expressed in this article are solely those of the authors and do not necessarily represent those of their affiliated organizations, or those of the publisher, the editors and the reviewers. Any product that may be evaluated in this article, or claim that may be made by its manufacturer, is not guaranteed or endorsed by the publisher.
References
Abdel-Gawwad, H. A., Metwally, A. K., and Tawfik, T. A. (2021). Role of barium carbonate and barium silicate nanoparticles in the performance of cement mortar. J. Build. Eng. 44, 102721. doi:10.1016/j.jobe.2021.102721
Bach, L. T., Gill, S. J., Rickaby, R. E. M., Gore, S., and Renforth, P. (2019). CO2 removal with enhanced weathering and ocean alkalinity enhancement: Potential risks and co-benefits for marine pelagic ecosystems. Front. Clim. 1, 1–21. doi:10.3389/fclim.2019.00007
Baquy, M. A., Li, J-Y., Shi, R-Y., Kamran, M. A., and Xu, R-K. (2018). Higher cation exchange capacity determined lower critical soil pH and higher Al concentration for soybean. Environ. Sci. Pollut. Res. 25, 6980–6989. doi:10.1007/s11356-017-1014-y
Beerling, D. J., Kantzas, E. P., Lomas, M. R., Wade, P., Eufrasio, R. M., Renforth, P., et al. (2020). Potential for large-scale CO2 removal via enhanced rock weathering with croplands. Nature 583, 242–248. doi:10.1038/s41586-020-2448-9
Beerling, D. J., Leake, J. R., Long, S. P., Scholes, J. D., Ton, J., Nelson, P. N., et al. (2018). Farming with crops and rocks to address global climate, food and soil security. Nat. Plants 4, 138–147. doi:10.1038/s41477-018-0108-y
Dai, Y., Qiao, X., and Wang, X. (2018). Study on cation exchange capacity of agricultural soils. IOP Conf. Ser. Mat. Sci. Eng. 392, 042039. doi:10.1088/1757-899X/392/4/042039
Das, S., Kim, G. W., Hwang, H. Y., Verma, P. P., and Kim, P. J. (2019). Cropping with slag to address soil, environment, and food security. Front. Microbiol. 10, 1320. doi:10.3389/fmicb.2019.01320
Goll, D. S., Ciais, P., Amann, T., Buermann, W., Chang, J., Eker, S., et al. (2021). Potential CO2 removal from enhanced weathering by ecosystem responses to powdered rock. Nat. Geosci. 14, 545–549. doi:10.1038/s41561-021-00798-x
Green, J. K., Seneviratne, S. I., Berg, M., Findell, K. L., Hagemann, S., Lawrence, D. M., et al. (2019). Large influence of soil moisture on long-term terrestrial carbon uptake. Nature 565, 476–479. doi:10.1038/s41586-018-0848-x
Hansen, J., Sato, M., Kharecha, P., von Schuckmann, K., Beerling, D. J., Cao, J., et al. (2017). Young people’s burden: Requirement of negative CO2 emissions. Earth Syst. Dyn. 8, 577–616. doi:10.5194/esd-8-577-2017
Humphrey, V., Berg, A., Ciais, P., Gentine, P., Jung, M., Reichstein, M., et al. (2021). Soil moisture-atmosphere feedback dominates land carbon uptake variability. Nature 592, 65–69. doi:10.1038/s41586-021-03325-5
Intergovernmental Panel on Climate Change (IPCC) (2018). Global warming of 1.5 °C. Geneva, Switzerland: World Meteorological Organization.
Jorat, M. E., Goddard, M. A., Manning, P., Lau, H. K., Ngeow, S., Sochi, S. P., et al. (2020). Passive CO2 removal in urban soils: Evidence from brownfield sites. Sci. Total Environ. 703, 135573. doi:10.1016/j.scitotenv.2019.135573
Kantola, I. B., Masters, M. D., Beerling, D. J., Long, S. P., and DeLucia, E. H. (2017). Potential of global croplands and bioenergy crops for climate change mitigation through deployment for enhanced weathering. Biol. Lett. 13, 20160714. doi:10.1098/rsbl.2016.0714
Kimura, T., and Koga, N. (2011). Monohydrocalcite in comparison with hydrated amorphous calcium carbonate: Precipitation condition and thermal behavior. Cryst. Growth Des. 11, 3877–3884. doi:10.1021/cg200412h
Klemettinen, L., Avarmaa, K., Jokilaakso, A., and Taskinen, P. (2021). Iron activity measurements and spinel-slag equilibria in alumina-bearing iron silicate slags. J. Alloys Compd. 855, 157539. doi:10.1016/j.jallcom.2020.157539
Lam, L., Wong, Y. L., and Poon, C. S. (2000). Degree of hydration and gel/space ratio of high-volume fly ash/cement systems. Cem. Concr. Res. 30, 747–756. doi:10.1016/S0008-8846(00)00213-1
Madeira, M., Auxtero, E., and Sousa, E. (2003). Cation and anion exchange properties of Andisols from the Azores, Portugal, as determined by the compulsive exchange and the ammonium acetate methods. Geoderma 117 (3–4), 225–241. doi:10.1016/S0016-7061(03)00125-3
Nelson, P. N., and Su, N. (2010). Soil pH buffering capacity: A descriptive function and its application to some acidic tropical soils. Soil Res. 48, 201–207. doi:10.1071/SR09150
Rao, C., Guo, X., Li, M., Sun, X., Lian, X., Wang, H., et al. (2019). In vitro preparation and characterization of amorphous calcium carbonate nanoparticles for applications in curcumin delivery. J. Mat. Sci. 54, 11243–11253. doi:10.1007/s10853-019-03686-3
Rockström, J., Gaffney, O., Rogelj, J., Meinshausen, M., Nakicenovic, N., and Schellnhuber, H. J. (2017). A roadmap for rapid decarbonization. Science 355, 1269–1271. doi:10.1126/science.aah3443
Russell, A. E., Laird, D., and Mallarino, A. P. (2006). Nitrogen fertilization and cropping system impacts on soil quality in Midwestern mollisols. Soil Sci. Soc. Am. J. 70, 249–255. doi:10.2136/sssaj2005.0058
Schmidt, M. P., Ilott, A. J., Phillips, B. L., and Reeder, R. J. (2014). Structural changes upon dehydration of amorphous calcium carbonate. Cryst. Growth Des. 25, 938–951. doi:10.1021/cg401073n
Terrer, C., Phillips, R. P., Hungate, B. A., Rosende, J., Pett-Ridge, J., Craig, M. E., et al. (2021). A trade-off between plant and soil carbon storage under elevated CO2. Nature 591, 599–603. doi:10.1038/s41586-021-03306-8
Torrent, J., DelCampillo, M. C., and Barrón, V. (2015). Short communication: Predicting cation exchange capacity from hygroscopic moisture in agricultural soils of Western Europe. Span. J. Agric. Res. 13 (4), e11SC01. doi:10.5424/sjar/2015134-8212
Wang, J., Watanabe, N., Inomoto, K., Kamitakahara, M., Nakamura, K., Komai, T., et al. (2022). Sustainable process for enhanced CO2 mineralization of calcium silicates using a recycling agent under alkaline conditions. J. Environ. Chem. Eng. 10, 107055. doi:10.1016/j.jece.2021.107055
Wuenscher, R., Unterfrauner, H., Peticzka, R., and Zehetner, F. (2015). A comparison of 14 soil phosphorus extraction methods applied to 50 agricultural soils from Central Europe. Plant Soil Environ. 61, 86–96. doi:10.17221/932/2014-PSE
Zeng, S., Liu, Z., and Groves, C. (2022). Large-scale CO2 removal by enhanced carbonate weathering from changes in land-use practices. Earth. Sci. Rev. 225, 103915. doi:10.1016/j.earscirev.2021.103915
Zhang, G., Kang, J., Wang, T., and Zhu, C. (2018a). Review and outlook for agromineral research in agriculture and climate mitigation. Soil Res. 56, 113–122. doi:10.1071/SR17157
Keywords: atmospheric CO2 removal, cropland, enhanced weathering, industrial Ca-rich byproduct, sustainability
Citation: Yoshioka R, Nakamura K, Sekiai R, Wang J and Watanabe N (2022) Effectiveness and characteristics of atmospheric CO2 removal in croplands via enhanced weathering of industrial Ca-rich silicate byproducts. Front. Environ. Sci. 10:1068656. doi: 10.3389/fenvs.2022.1068656
Received: 13 October 2022; Accepted: 14 November 2022;
Published: 25 November 2022.
Edited by:
Yanqiu Zhang, Changzhou University, ChinaReviewed by:
Weiyu Shi, Southwest University, ChinaNataliya Yurkevich, Institute of Petroleum Geology and Geophysics (RAS), Russia
Tong Ouyang, Xiamen University, China
Copyright © 2022 Yoshioka, Nakamura, Sekiai, Wang and Watanabe. This is an open-access article distributed under the terms of the Creative Commons Attribution License (CC BY). The use, distribution or reproduction in other forums is permitted, provided the original author(s) and the copyright owner(s) are credited and that the original publication in this journal is cited, in accordance with accepted academic practice. No use, distribution or reproduction is permitted which does not comply with these terms.
*Correspondence: Kengo Nakamura, nakamurakengo@mail.saitama-u.ac.jp; Jiajie Wang, wang.jiajie.e4@tohoku.ac.jp; Noriaki Watanabe, noriaki.watanabe.e6@tohoku.ac.jp