- 1College of Water Resources and Architectural Engineering, Tarim University, Alaer, China
- 2College of Agriculture, Tarim University, Alaer, China
- 3State Key Laboratory of Frozen Soil Engineering, Northwest Institute of Eco-Environment and Resources, Chinese Academy of Sciences, Lanzhou, China
- 4Key Laboratory of Environment Remediation and Ecological Health, Ministry of Education, College of Environmental and Resources Sciences, Zhejiang University, Hangzhou, China
- 5Department of Environmental Sciences, University of Narowal, Narowal, Pakistan
Mining operations accelerate ecological damage in alpine mountain locations by contributing to soil erosion and nutrient loss in the freeze-thaw (FT) climate. However, limited studies have been conducted to reduce the soil erosion and nutrient loss in FT climate. This study’s goal was to determine how biochar effected soil erosion in the restored soil of the alpine mining region under FT circumstances. Rainfall simulation and FT cycles (FTCs; three and 5) were used to investigate the effects of 500 and 1,000 kg hm−2 biochar application rates (BARs) on runoff, soil loss, and runoff loss of ammonia nitrogen (AN), nitrate nitrogen (NN), total phosphorus (TP), and dissolved phosphorus (DP). Soil residual AN, NN, and DP concentrations after FTCs were also evaluated. Biochar application significantly reduced the runoff, soil loss rate, AN and NN loss rates, but increased the runoff NN concentration and decreased the total AN and NN loss under FTCs condition. Reductions in AN (37.2%–52.2%) and NN (14.3%–27.1%) runoff loss can be differentially attributed to the adsorption effect of biochar and decrease in total runoff. The runoff P concentrations, rates, and magnitudes for soils subjected to FTCs significantly decreased with biochar addition, owing to the particulate P loss decrease in soil and increased adsorption effect of soil DP. Biochar addition increased soil residual AN, NN, and DP concentrations. However, the increase in FTCs weakened the inhibitory effects of biochar on soil erosion. This study suggested the important role of biochar application for the recovery of low-nutrient eroded soils in alpine mining areas.
Introduction
Continuous land degradation has been recognized as a global crisis (Wei et al., 2019), especially in the Tianshan mountain region in Xinjiang, China (Li et al., 2020). To date, 138 kinds of mineral resources have been discovered in the Tianshan Mountains from which approximately 175.27 Mt of iron ore has been mined (Statistic Bureau of Xinjiang, 2013). However, continuous mining causes glacier base rock damage, produces a large number of tailings, and threatens the soil ecosystem. Moreover, soil erosion in the alpine areas is worsened by influencing factors, such as global warming (Li et al., 2020). So far, Xinjiang still has a high soil erosion intensity index, mainly in the key mineral resources development zone (Chen, 2013), resulting in increased damage areas and a range of environmental issues. Therefore, there is an urgent need to protect the local ecological environment. For the treatment of slag, the most conventional treatment method is to conduct landfill depending on the terrain. In Tianshan area, mining companies usually pile slag into ore dams of different heights through certain engineering measures, and then cover them with 20 cm soil layer before planting grass, which usually leads to loose soil structure (Li et al., 2022). Thus, it is important to consider the effect of freeze-thaw (FT) on soil erosion, soil nutrient migration, and remediation technologies in an environment subjected to FT cycles (FTCs).
In regions with seasonal freezing and thawing, FTCs have a significant impact on soil properties including soil porosity and aggregate stability (Liu et al., 2017; Fu et al., 2018; Wei et al., 2019). For instance, it has been reported that FTCs reduced soil strength and increased mean soil detachment capacity by 36.5% (Liu et al., 2017). Meanwhile, FT reduces the stability of soil aggregates (Sahin et al., 2008; Xie et al., 2015; Fu et al., 2018) due to the formation of large pores in soils with high moisture content, which consequently increased the hydraulic conductivity and decreased the soil water holding capacity (WHC) (Rima & Beier 2021; Wei et al., 2019). The main reason is that FTCs destroy the primary structure of soil and change the arrangement and combination of soil particles (Zhang et al., 2016), thus, the soil pore size increases with the freezing and expansion of water, thereby causing a reduction in soil strength and soil stability (Oztas and Fayetorbay, 2003; Joseph and Henry, 2008). FTCs may further promote the release of organic acids, resulting in a decrease in soil pH (Edwards and Cresser 1992). Feng et al. (2007) found that the free lipid content in organic matter decreased significantly, while the lignin content was relatively stable under FTCs. Männistö et al. (2009) showed that the migration of organic matter increased with an increase in soil moisture content under FTCs, resulting in its loss risk. FTCs may also cause the migration and transformation of soil nitrogen (N) and phosphorus (P) (Joseph and Henry, 2008; Jiang et al., 2018). Some studies have found that soil inorganic N content decreases first and then increases with the frequency of carbon exchange (Urakawa et al., 2014; Fu et al., 2019a). As the number of FTCs increased, the degree of soil N mineralization gradually declined (Urakawa et al., 2014; Fu et al., 2019a). However, most of these studies only focused on forest and agricultural soils and did not consider the impact of the rainfall process on soil and nutrient loss under FTCs. Additionally, there is a lack of knowledge regarding soil and water losses in alpine meadow mine-restoration areas.
It is extremely necessary to reclaim the soil in mining areas to mitigate the effects of severe environmental conditions such as FT. At present, soil reclamation using amendments is a low-cost and environmentally friendly method to improve damaged and polluted lands (El Rasafi et al., 2021). Soil amendments usually have sufficient remediation effects on both acidic and alkaline soils (Ji et al., 2019; El Rasafi et al., 2021), and thus exhibit broad application prospects. Biochar is an insoluble and stable solid substance produced by the pyrolysis of biological residues under high-temperature and anoxic conditions (Laird et al., 2010; Yao et al., 2012). Biochar as a soil amendment that can improve physicochemical and biological properties, and has been widely applied in various areas, attracting significant attention (Li F. Y. et al., 2019). Studies have showed that biochar can reduce soil bulk density, increase soil total porosity, affect soil aggregates, improve soil structure (Yao et al., 2012; Tammeorg et al., 2014; Zhang Q. et al., 2015), and increase soil WHC (Fu et al., 2019a; Wang et al., 2019). Furthermore, biochar is beneficial for the microbial activity and soil fertility (Li F. Y. et al., 2019). In addition, biochar has a large specific surface area and strong adsorbability (Gai et al., 2014; Liu et al., 2018; Li F. Y. et al., 2019) which can binds heavy metal ions and has a strong adsorption ability for phosphate (PO43+), Ammonium (NH4+), and nitrate (NO3−) ions (Li F. Y. et al., 2019). Biochar addition has been shown to reduce soil nutrient loss (Nyambo et al., 2018), effectively block the migration or change the occurrence forms of heavy metals, and reduce their environmental risk (Li Y. et al., 2021). Most previous studies on the effects of biochar application on soil erosion were conducted in non-FT periods. For example, Hseu et al. (2014) found that biochar addition reduced soil erosion by 35%–90% after a 168-day artificially simulated rainfall test. However, few studies have investigated the effect of biochar addition on soil erosion and nutrient loss under FT conditions, and studies concerning alpine mine restoration areas have not been reported. In addition, if and how FT affects environmental reclamation with biochar amendment in these areas is still unclear.
The soil in the mine restoration area usually has a loose structure and a limited quantity of stable aggregates, which hinder soil and water conservation. Restorative agents should be added to reduce soil erosion and promote rapid vegetation restoration in alpine mining areas (Li et al., 2022). Many studies have reported that FT may lead to an increase in soil erosion (Liu et al., 2017; Jiang et al., 2018), and examined biochar has positive effect on soil erosion and nutrient loss in agricultural soils (Li et al., 2021). Thus, in this study, soil samples were collected from the alpine mine restoration area, and the effect of biochar addition on runoff, soil sediment, and nutrient loss was investigated. Artificial rainfall simulation and FT tests were performed under different FTCs and biochar application rates (BARs). The main hypotheses in this study were as follows: 1) FT will accelerate the irreversible loss of water, soil, and nutrients in the alpine mine restoration soil, which will increase with the increment of FTCs. 2) biochar addition is beneficial in reducing soil erosion, and this remediation effect will be enhanced with an increase in BARs.
Materials and methods
Study area and soil sampling
The soil samples were collected from the restoration region of the X-Lake Iron Mine (85°3′40″N, 43°18′50″E) located in the central part of the West Tianshan Mountain (Figure 1), which is a middle-high mountain area (2, 573–3,641 m above sea level (a.s.l)) with an average altitude of >3,500 m a.s.l. The annual ore mining output in this area exceeded 3 Mt by 2020. The mining area has a temperate continental climate, experiencing both rain and snowfall, and featuring moderate to deep (>1 m) seasonally frozen soil (Li F. Y. et al., 2020; Li et al., 2022). The average annual rainfall of the area is 576 mm, mainly occurring from June to August, with an average relative humidity of 43% (Li F. Y. et al., 2020). The maximum temperature in summer is approximately 16°C, and the minimum temperature in winter is approximately −30°C. There is a large difference between day and night temperatures, and part of the snow cover melts during the summer, causing seasonal floods.
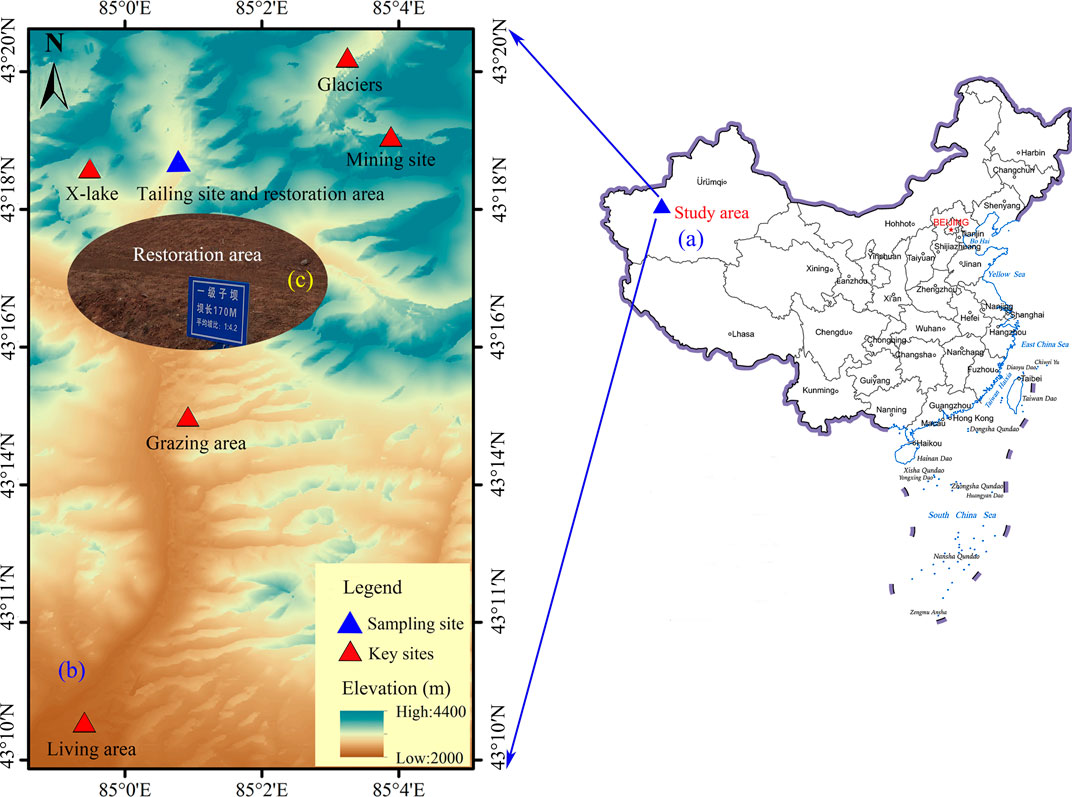
FIGURE 1. Location of the study area (A), sampling and important environmental sites (B), and restoration field (C) in the mining area in Xinjiang Uygur Autonomous Region, China.
The dominant vegetation types in the restoration field are Polygonum viviparum L., AstragalustibetanusBenth.exBunge, Thalictrum alpinum L., Carex stenocarpa Turcz. ex V. Krecz., Leontopodium leontopodioides (Willd.) Beauv, and Artemisia carvifolia (Li F. Y. et al., 2020; Li et al., 2022). Mining activities have caused extreme damage to this environment, especially in summer, when the temperature rises, and snow melts forming surface runoff. Meanwhile, abundant rainfall in summer superimpose the FT effect, resulting in increasingly serious soil erosion in the area, aggravating the loss of soil nutrients and leaching of heavy metals, thus causing environmental pollution (Yuan et al., 2021).
In September 2020, surface soil from depths of 0–20 cm in the restoration field (∼170,000 m2) of the mining region was randomly selected, and 20 sub-samples were collected and mixed thoroughly to obtain a standard sample weighing 200 kg. The soil in the mine restoration area was covered with slag and grass. The collected soil samples were brought back to the laboratory and placed in a cool and ventilated place for air drying without pollution. Then, debris (such as stones and plant residues) was removed from the dried samples, which were ground with a glass mortar, sieved through a 2-mm mesh sieve, transferred into zip-lock bags, and stored in a dry and dark place. The basic physical and chemical properties of the soil are listed in Table 1.
Experimental setup
The experiments were conducted in the environmental engineering laboratory of the engineering training building at Tarim University, China. We included three bars of 0 kg hm−2 (B0; control), 500 kg hm−2 (B1), and 1000 kg hm−2 (B2) according to previous research results in a black soil under FT condition (Li Y. et al., 2020), and three FTCs of 0 (FT0; control), 3 (FT3), and 5 (FT5) to investigate the effect of biochar application on soil and nutrient loss from the studied soil under FT conditions. The treatments were split into nine groups (FT0 × B0, FT0 × B1, FT0 × B2, FT3 × B0, FT3 × B2, FT3 × B2, FT5 × B0, FT5 × B1, and FT5 × B2) with three replications for each. The biochar used in this experiment was produced by heating cotton straw at 600°C for 4 h using a tube furnace (KPX-9162 MBE, China) under limited oxygen conditions. After air-cooling to 25°C, the products were washed using deionized water and subsequently oven-dried at 80°C for 12 h. The fine pulverized products were further separated by passing through a 0.154-mm sieve. The properties of the cotton straw biochar are listed in Table 1. The apparatus used in this study is illustrated in Supplementary Data S1 and Supplementary Figure S1.
Rainfall simulation and soil erosion test
After passing through a 5-mm sieve, the collected soil was mixed thoroughly with the cotton straw biochar based on the abovementioned application rates (B0, B1, and B3). The soil was evenly loaded into the soil test tank. The soil was then scraped and compacted adequately to obtain a homogeneous texture (Eltohamy et al., 2021) with a consistent bulk density of 1.1 g cm−3, which was the same as the original meadow soil. Once the soil moisture content was set to 20% (on a gravimetric basis), the soil surface was covered with a plastic film to prevent water evaporation.
The FTC’s test was carried out after a 1-week preculture. The FT events were designed as 12 h freezing at –20°C followed by 12 h thawing at 25°C based on the extreme FT conditions in the alpine area in Tianshan Mountains. After the FTCs, the rainfall simulation and soil erosion tests were carried out with three replications for each treatment. The horizontal projection area of the rainfall range was 0.5 m × 0.4 m. During the rainfall simulation, the water pressure was kept constant, the vertical distance between the dropper and the slope was 2.0 m, and the rain intensity was controlled by the flowmeter. The rainfall intensity was set at 48 mm h−1 based on the extreme case in the Tianshan mountains, the soil slope was fixed at 15°, and the continuous rainfall time was set to 60 min. During the rainfall experiment, the runoff samples of the soil flowing out of the lower end of the soil tank were collected in a beaker at the outlet of the collecting trough every 5 min. The quantity of each beaker was determined using a weighing method. The remaining water sample was left standing for 24 h. Then, the supernatant was transferred, a part of which was collected for subsequent analyses. The sediment at the bottom of the beaker was collected, and the sediment yield was measured after drying at 105°C for 24 h (Ejack and Whalen, 2021). After the FTCs experiments, three soil samples were selected randomly from each treatment to determine the residual nutrients in the slope soil.
Analytical methods
The runoff samples were filtered using a 0.45-μm membrane filter (Millipore, Billerica, MA, United States) for the determination of dissolved P (DP), and another aliquot was kept unfiltered to determine the total P (TP) concentrations. The filtered or unfiltered runoff sample (5 ml each) was mixed with 1 ml of a solution containing 150 mmol L−1 K2O8S2 and 180 mmol H2SO4 L−1 boiled at 121°C for 1 h (Pagel et al., 2008). They were then heated for 60 min in a water bath at 95°C after adding 0.7 ml of ascorbic acid solution (188 mmol L−1), and the concentration of TP and DP in runoff was determined by the colorimetric method (Murphy and Riley, 1962). Runoff samples were filtered through 0.45-μm cellulose nitrate membranes (Millipore, Billerica, MA, United States), and the concentrations of ammonia N (AN) were analyzed using the salicylate-hypochlorite method (Crooke and Simpson, 1971), while the concentrations of nitrate N (NN) were analyzed through the Griess-Ilosvay method (Keeney and Nelson, 1982) using ultraviolet spectrophotometry (UV-5500, Shanghai, China).
The ring knife method was used to determine the original soil bulk density and WHC (Zhu et al., 2018). Soil pH was measured using a pH meter (German WTW-MULTI 3630 IDS) with a soil-to-water ratio of 1:5 (w/v) after shaking for 30 min. Soil TP was determined by the molybdenum blue procedure following digestion with H2SO4–HClO4 at 300°C for 2 h (Murphy and Riley, 1962). The DP was determined by water extraction and molybdenum-antimony resistance spectrophotometry (Olsen and Sommers 1982). In brief, the soil was extracted with 2 mol L−1 KCl solution (solid: liquid ratio, 1:10). The extracted solution was filtered through a 0.45-μm cellulose nitrate membrane (Millipore, Billerica, MA, United States) and then analyzed for DP using the molybdenum blue method. The method of Keeney and Nelson (1982) was used for the colorimetric determination of soil AN and NN.
Data processing and statistics
Microsoft Excel 2020, SPSS Statistics 22.0 (IBM Corp., Armonk, NY, United States), and Origin 2021b software (OriginLab Corp., Northampton, United States) were used for data analysis. Runoff parameters were calculated according to Li et al. (2022), which were also shown in supplementary data. A two-way analysis of variance was used to evaluate the FTCs and BARs and their interaction effects on runoff volume, soil sediment yield, and nutrient loss (AN, NN, TP, and DP). The nonlinear model function in SPSS was used to investigate the relationship between the loss rates of runoff and sediment versus the concentration of nutrients during runoff, significant levels were set at p < 0.05.
Results
Effect of biochar addition on runoff and soil loss
Remarkably, the runoff loss rate was characterized by a sharp increase in the initial 15-min rainfall period (Figure 2), followed by a stable loss rate during the remaining period (15–60 min). In addition, the runoff curve for each treatment followed the exponential equation (Figures 2A–C).
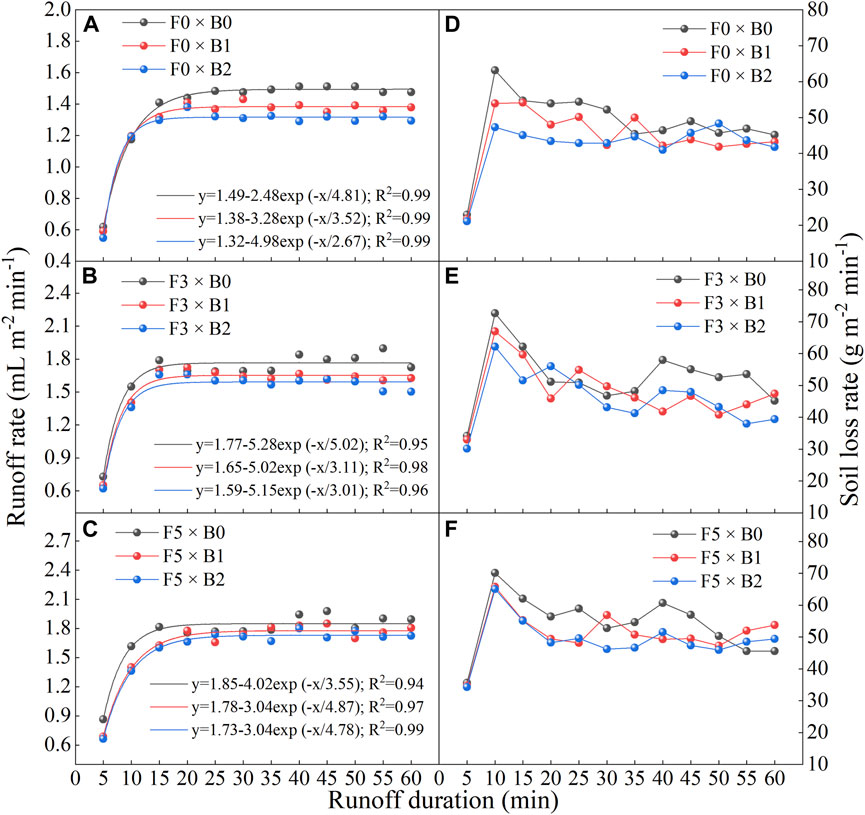
FIGURE 2. Influence of biochar application rates (B0: 0 kg hm−2, B1: 500 kg hm−2, and B2: 1,000 kg hm−2), on (A–C) runoff rate and (D–F) soil loss rate under the freeze-thaw conditions (F0: 0 cycle, F3: 3 cycles, and F5: 5 cycles).
No interactive effect was found between BARs and FTCs on total runoff (Supplementary Table S1); however, both had significant individual effects on it (p < 0.01). Irrespective of the BARs, the increase in FTCs caused accretion in the runoff rate. Higher total runoff yield was recorded under FT5 (994.34 ± 6.72 m3 hm−2) followed by FT3, compared to that under FT0 (Table 2). In contrast, biochar addition resulted in a notable reduction in the average (6.1%–10.1%) runoff rates compared to that in the control (B0), with a higher impact of B2 than B1 (Figures 2A–C). Similarly, biochar addition significantly reduced the accumulated runoff by approximately 6.0%–11.4% compared with that in B0 (Table 2, p < 0.05). Considering the effect of FTCs × BARs interactions, the highest runoff was observed for FT5 × B0 which was 1.26 times that of FT0 × B0 (Table 2). Compared to other interactions, only FT0 × B2 and FT0 × B1 interactions lowered the runoff rate by 10.2% and 6.1% relative to FT0 × B0, respectively.
There was no significant interaction effect of both BARs and FTCs on the soil loss rate (p > 0.05; Supplementary Table S1); however, both individually significantly affected the total soil loss (p < 0.01). Regardless of BARs effects, the soil loss rate increased with an increase in FTCs (Table 2). Higher accumulated soil loss was observed under FT5 followed by FT3, compared to that under FT0. In contrast, biochar application significantly inhibited soil loss (p < 0.01; Supplementary Table S1). Under FTCs conditions, biochar addition reduced the total soil loss rate by 5.7%–12.5% compared to that in B0 (Figures 2D–F). Despite this, there was no significant difference between B1 and B2 across all FTCs (p > 0.05).
The FT0 × B2 reduced the soil loss, on average, by 12.4% compared to that of FT0 × B0 (Table 2). Other interactions that caused a reduction in the soil loss followed the order: FT0 × B1 (7.9%) > FT3 × B2 (4.8%) > FT3 × B1 (0.5%), respectively, relative to FT0 × B0.
Effects of biochar on nitrogen loss
The effects of both FTCs and BAR treatments on AN and NN loss showed similar trends (Figure 3) to those of soil loss rates. Each treatment showed a high initial AN loss rate and a very high initial NN loss rate (Supplementary Figures S2A–F). Notably, the initial increase in the AN loss rate under both FT5 and FT3 conditions was greater than that under FT0; after 15 min, the gap declined with time (Figures 3A–C). However, the initial loss rate of AN was very low, subsequently peaked at 10 min, and then decreased slowly. Under the same FTCs, the initial concentration of NN in slope runoff increased with BARs, but was lower than that in the non-biochar application group after 10 min (Supplementary Figures S2D–F). The decrease in the NN loss rate in the slope soil with biochar application (i.e., B1 and B2) was much higher than that without biochar application (B0).
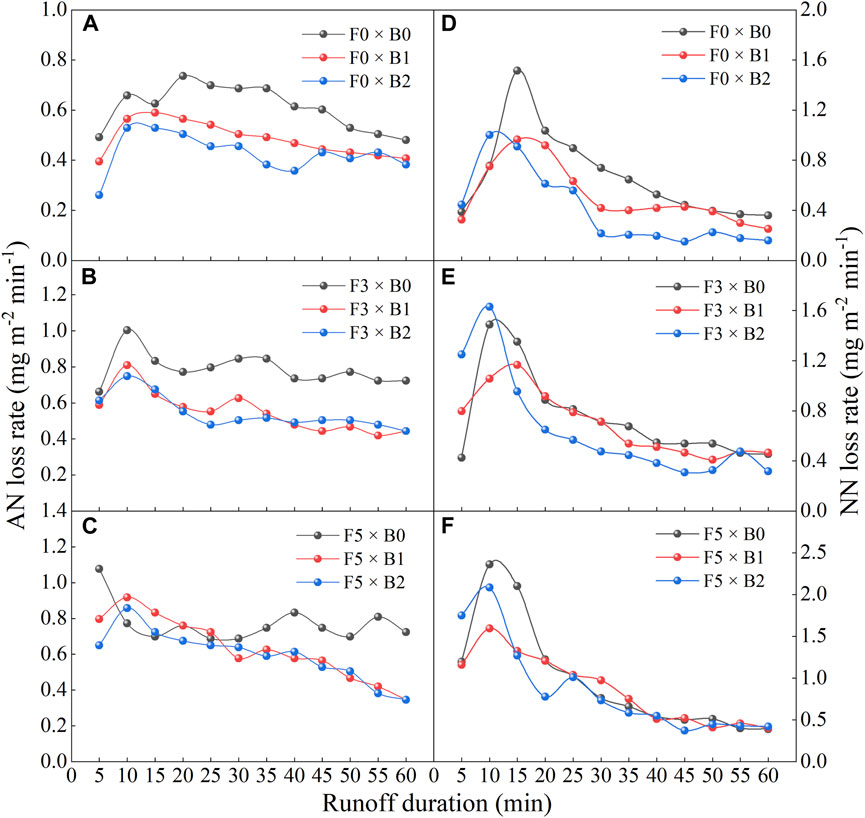
FIGURE 3. Influence of biochar application rates (B0: 0 kg hm−2, B1: 500 kg hm−2, and B2: 1000 kg hm−2) on the loss rates of (A–C) ammonia nitrogen (AN) and (D–F) nitrate nitrogen (NN) under the freeze-thaw conditions (F0: 0 cycles, F3: 3 cycles, and F5: 5 cycles).
The average NN runoff concentration of the B1 and B2 treatments under the same FTCs conditions increased by 1.5%–23.9% compared to that with B0 treatment (Supplementary Figures S2A–C). However, the addition of biochar significantly reduced the NN loss rate. The average NN runoff rate in each treatment with biochar application under the same FTCs decreased by 6.6%–35.1%, respectively, compared to that without biochar application. The average AN runoff rate in each treatment with BARs under the same FTCs decreased by 17.7%–31.1%, respectively, compared with B0. Meanwhile, we found that there was no significant linear correlation between runoff rate and loss concentration and the AN and NN runoff rate (p > 0.05), and no significant correlation was found between initial (first 5 min) soil loss rate and the AN and NN loss concentration and rate. However, after a 5-min rainfall duration, AN and NN loss concentrations and rates were significantly correlated with soil loss rate (Figure 5).
The BARs and number of FTCs showed a significant interaction (p < 0.05) with the total AN runoff loss (Supplementary Table S2), while there was no interaction effect on the total NN loss (Supplementary Table S2). However, both had significant independent effects on total NN loss (p < 0.01). Although the FTCs significantly increased the total AN and NN loss (p < 0.05), biochar addition significantly decreased the total loss (p < 0.05) (Table 3). On average, B2 and B1 significantly reduced the total AN loss in the runoff by 34.4% and 28.4%, respectively, compared with B0 (p < 0.05); however, there were no significant differences under FT conditions (Table 3). Moreover, FTCs resulted in a decrease in the concentration of soil residual AN, but the increase in BARs increased AN concentration in the soil (Figure 6A). The total NN loss in each treatment with biochar application under the same FTCs decreased by 14.3%–47.4% compared with that without biochar treatment. Freezing and thawing resulted in a decrease in the concentration of residual NN in the soil, but the increased application rate of biochar significantly increased NN concentration in the soil (p < 0.05, Figure 6A).
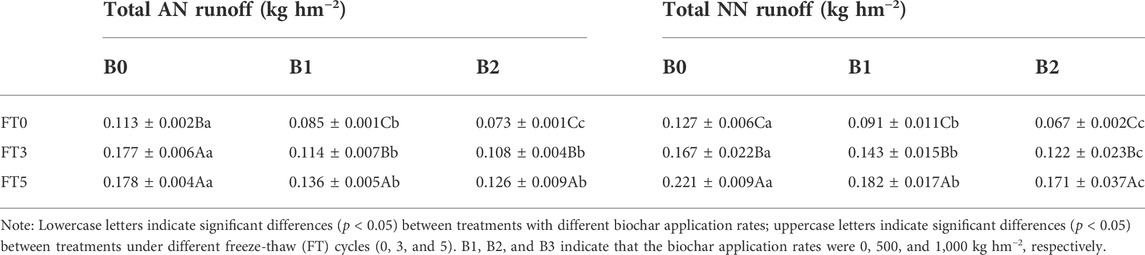
TABLE 3. Total loss of ammonia nitrogen (AN) and nitrate nitrogen (NN) under different biochar application rates.
Effects of biochar on phosphorus loss
The TP and DP concentrations of the BAR treatments in the FT0 environment showed a linear trend of gradual decrease with rainfall duration, and their initial concentrations were much lower than those under FTCs (Supplementary Figure S3). In general, the B1 and B2 treatments lowered the runoff TP and DP concentrations under the same FTCs by 6.7%–23.2% and 14.8%–64.4%, respectively, compared to that in B0. In addition, B1 and B2 effectively reduced TP and DP loss rates during the rainfall period (Figure 4). The average TP and DP runoff rates in each treatment with biochar application under the same FTCs were 12.0%–34.7% and 12.5%–68.8%, respectively, which were lower than those of B0. Unlike TP, which showed little change during the entire rainfall duration (Figures 4A–C), the DP loss rate gradually decreased with rainfall duration (Figures 4D–F). There was no significant correlation between runoff rate and TP and DP loss concentration and rate (p > 0.05), and there was no significant correlation between the initial soil loss rate and TP and DP loss concentration and rate. However, after 5 min of rainfall, TP and DP loss concentrations and rates were closely related to soil loss (Figure 5).
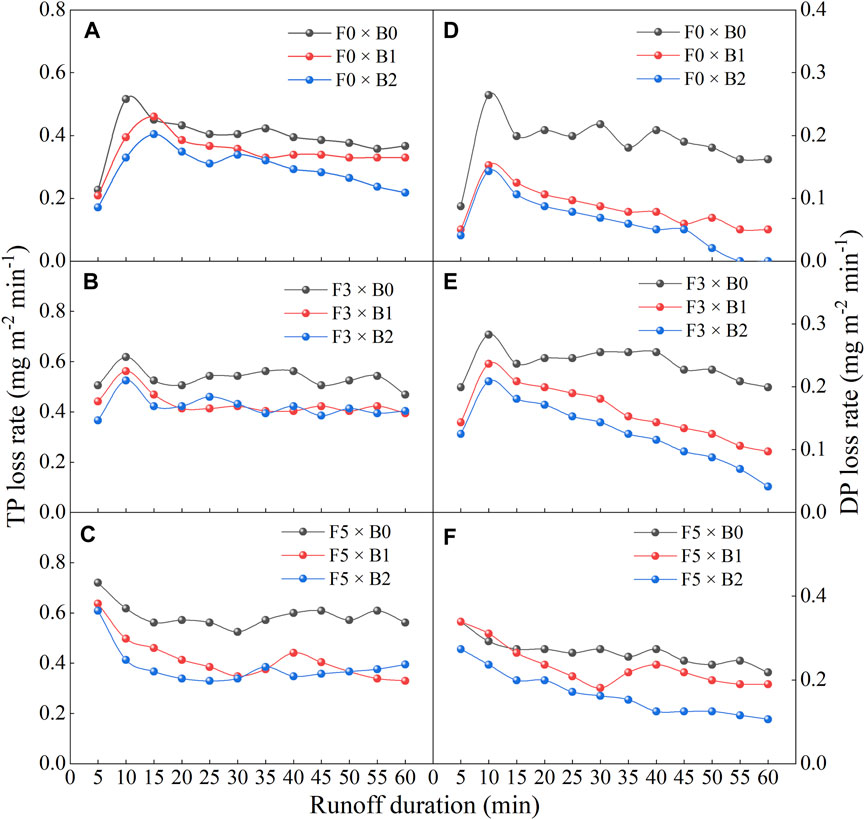
FIGURE 4. Influence of biochar application rates (B0: 0 kg hm−2, B1: 500 kg hm−2, and B2: 1000 kg hm−2) on the loss rates of total phosphorus (TP, (A–C)) and dissolved phosphorus (DP, (D–F)) under the freeze-thaw conditions (F0: 0 cycles, F3: 3 cycles, and F5: 5 cycles).
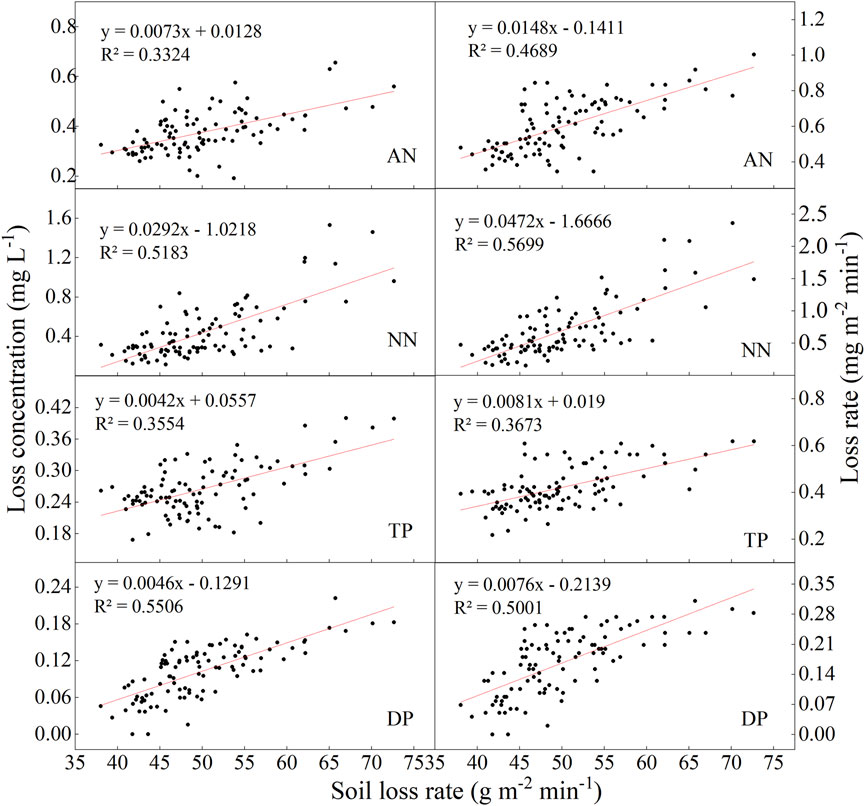
FIGURE 5. Correlation between soil loss and nutrient loss under the freeze-thaw conditions after 5 min rainfall duration. AN, ammonia nitrogen; NN, nitrate nitrogen; TP, total phosphorus; DP, dissolved phosphorus.
The BARs and FTCs had significant independent effects (p < 0.001; Supplementary Table S3) on TP and DP runoff losses. Irrespective of BAR treatments, FT3 and FT5 significantly increased TP and DP losses (p < 0.05; Table 4) compared to FT0. In addition, there were significant differences between the DP losses in FT3 and FT5 (p < 0.05). Unlike DP, there were no significant differences between the TP losses in FT3 and FT5 (Table 4). On the other hand, B1 and B2 significantly reduced TP and DP losses compared to B0 (Supplementary Table S3), but there were no significant differences between B1 and B2 in terms of TP loss.
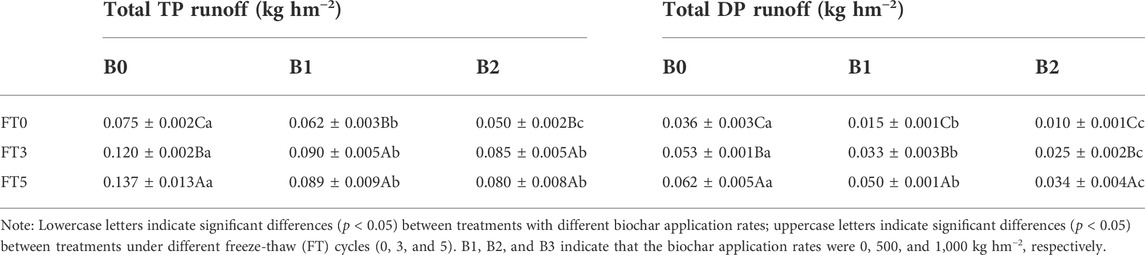
TABLE 4. Total phosphorus (TP) and dissolved phosphorus (DP) loss in slope with different biochar application rates.
Under non-FT conditions (F0), the TP loss caused by the B1 and B2 treatments decreased by 17.2% and 32.9%, respectively, compared with that in B0. However, under FTCs, the TP loss under biochar addition decreased, on average, by 32.8% compared with that in B0 (Table 4). The interaction of FTCs and BARs had little effect on soil TP, with no significant difference between B2 and B0 (Table 4). Under the same FTCs, B1 and B2 caused a decrease in total DP loss by 19.2%–58.3% and 45.2%–72.3%, respectively, compared to B0. In the absence of biochar addition (B0), the increment in FT cycles lowered the soil residual DP content. In contrast, with the increase in biochar application rate, the soil residual DP content increased (p < 0.05, Figure 6B).
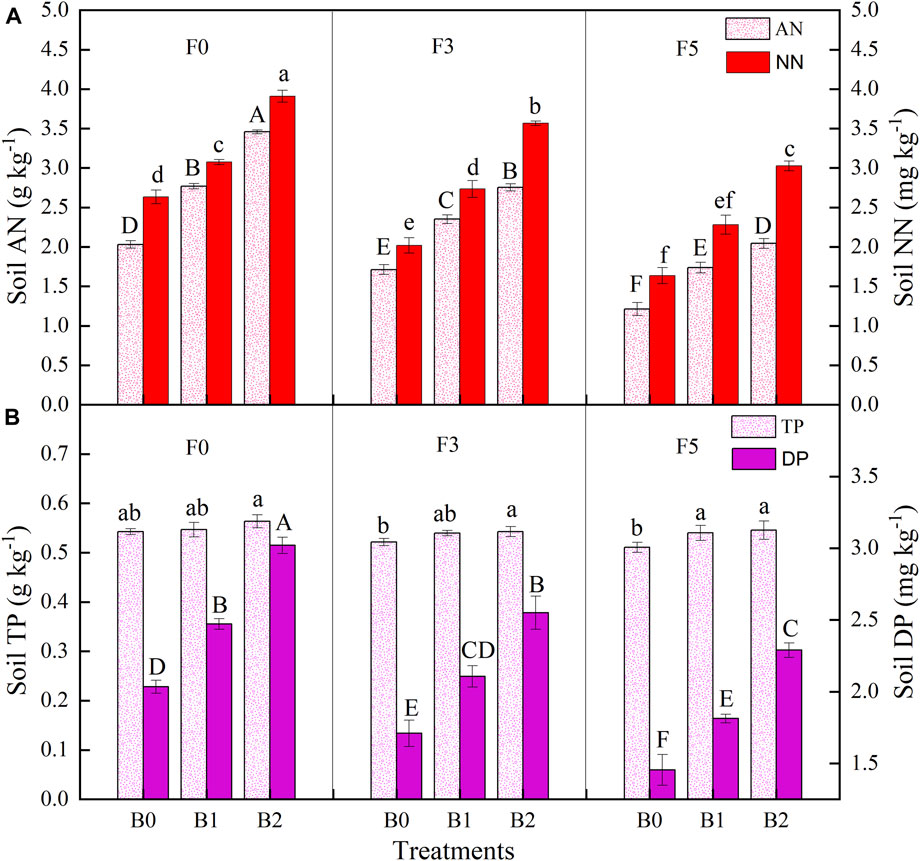
FIGURE 6. (A) soil residual ammonia nitrogen (AN) and nitrate nitrogen (NN) concentrations, (B) total phosphorus (TP) and dissolved phosphorus (DP) concentrations under different freeze-thaw cycles (F0: 0 cycles, F3: 3 cycles, and F5: 5 cycles) and biochar application rates (B0: 0 kg hm−2, B1: 500 kg hm−2, and B2: 1000 kg hm−2) at the end of the rainfall duration. Error bars denote standard deviations (n = 3). Different letters indicate significant differences (p < 0.05) between treatments under different freeze-thaw cycles and biochar application rates.
Discussion
Effects of biochar on runoff and soil loss under freezing and thawing
This study showed that biochar applications (i.e., 500 kg hm−2 and 1,000 kg hm−2) had a significant inhibitory effect on runoff rate and total runoff yield of the restored soil slope under FT conditions, which was similar to the results of a 2-year outdoor FT experiment of the agricultural soil under three artificial simulated rainfall tests (Li et al., 2021). Although some studies have demonstrated that excessive use of biochar may increase soil loss (Ahmadi et al. 2020; Li et al., 2020), this study found no significant difference in soil loss between the two application rates, which may be due to the lower application rates in this study. For example, a study on loess soil amended with biochar under simulated rainfall found that the addition of 1% or 3% biochar reduced soil loss rates, while the addition rate of 7% biochar increased the soil loss (Li et al., 2020). This is because the excessive application of biochar led to an imbalance between the soil liquid and gas phases, which negatively affected soil structure (Fu et al., 2019b). Another study showed that biochar addition decreased the total runoff by 2.4%–10.8% and significantly increased the total soil loss and interrill erodibility (Zhang et al., 2019). The differences between these results may be attributed to different soil types and physicochemical properties and the precursor, preparation temperature, and particle size of biochar (Kim et al., 2013; Głąb et al., 2016; Moragues-Saitua et al., 2017). For instance, adding biochar to clay loam soil can reduce soil bulk density and strength, delay the formation of soil seals, and reduce soil compactness, which may lower its resistance to erosive forces (Doan et al., 2015; Zhang et al., 2019). The inhibition effect of biochar on runoff rate is attributed to its ability to improve the soil permeability coefficient, leading to increased vertical water movement and reduced surface runoff (Abrol et al., 2016; Li et al., 2020). However, more studies have shown that biochar can reduce soil erodibility by increasing the quantity and stability of soil aggregates (Burrell et al., 2016; Li et al., 2019) which can retain more nutrients.
In general, biochar addition reduced the effect of FT on soil production and runoff. This may result in the improvement of soil micropore size, soil voids, and total porosity under the combination of biochar amendment and FTCs, which significantly increased the soil water retention capacity during the snow-melting period (Głąb et al., 2016; Fu et al., 2019b). Biochar application can also effectively reduce the turbulence degree of the flow on the rill of the slope (Li et al., 2021), which lead to reduce soil loss (Hseu et al., 2014). However, with an increase in the number of FTCs, the total runoff yield on the slope will still increase even with biochar application. This phenomenon may be because, during a low frequency of freezing and thawing (0–three FTCs), the soil cohesive force enhanced by biochar particles is greater than the damage of soil structure by FTCs, and has a good WHC in prophase. However, soil structure destruction intensified with an increase in the number of FTCs, which weakened the cohesive force between biochar and soil particles and reduced its inhibitory effect on soil loss (Wu et al., 2019).
Effects of biochar on nitrogen and phosphorus loss under freezing and thawing
After repeated freezing and thawing, the soil stored a large amount of pore water, resulting in a high initial concentration of accumulated soluble N and P, while the initial sediment loss rate was very low, and the N and P loss concentrations and rates were not significantly correlated with the soil loss rate at the initial stage (before 5 min). Therefore, the loss of N and P in the initial 5 min rainfall duration was mainly in soluble forms, and both showed a large loss rate and concentration. However, after 5 min, the concentration and rate of N and P loss were closely related to the soil loss rate (Figure 5), which indicates that the loss of N and P in the low-nutrient soil of the alpine mining region occurs mainly in particulate form after 5 min of rainfall. Nevertheless, the addition of biochar significantly reduced the N and P loss rate at the initial rainfall stage, thus significantly reducing the amount of N and P loss.
In this study the FT significantly increased N loss in the restored soil. Some studies have attributed the increased NH4+-N content to its from soil organic or inorganic colloids under FTCs (Freppaz et al., 2007; Fu et al., 2019a). Other studies have suggested that water in soil aggregates condenses into ice during FTCs and becomes fragmented after extrusion, which in turn affects the attachment ability of inorganic N to soil particles, thereby reducing the fixation effect of soil on inorganic N (Jackson-Blake et al., 2012; Gou et al., 2014). Herein, the application of biochar significantly inhibited the AN and NN loss on the slope under FT conditions, and the inhibition effect increased with an increase in the BARs. In addition, Zhou et al. (2017) showed that biochar addition in the field during the FT period reduced soil N loss; owing to the N adsorption ability of biochar. We also found that the inhibition effect of biochar was most significant under the non-FTC conditions, but the N retention capacity of biochar decreased with an increase in FTCs, which was consistent with the study by Wu et al. (2019), founded that the NN and AN retention capacity of biochar significantly decreased after the first round of FTCs. They attributed this behavior to the expansion of pore water, which increased the particle size of biochar in the FT process, decreased the surface area of biochar, and deteriorated the adsorption capacity (Wu et al., 2019). Therefore, the freezing and thawing processes weakened the N fixation effect of biochar to a certain extent.
At the initial stage of runoff, a higher NN concentration was found in the experimental groups with biochar application (i.e., B1 and B2). The main reason is that cotton straw is rich in inorganic N and usually contains a large amount of NN, which migrates rapidly with runoff, leading to an increase in its initial runoff concentration (Nguyen et al., 2017). Soil AN with positive charges is easily adsorbed by soil colloids, whereas NN carries negative charges (Xiong et al., 2020; Yuan et al., 2020). As they are non-reactive ions, their interaction with soil particles is weak, and they are easily lost under the action of precipitation and runoff dissolution and leaching. The addition of biochar increases the availability of soil oxygen and promotes N mineralization under FTCs, which in turn increases the transformation of NH4+ to NO3−(Nelissen et al., 2012; Fu et al., 2019a). In the later period of rainfall, biochar has strong absorption of N in the soil due to its large specific surface area, therefore, it can effectively maintain N in the soil during runoff erosion, thus reducing N loss (Lehmann et al., 2006).
Under FT conditions, the addition of biochar significantly increased the mean NN concentration, but significantly reduced the NN loss rate compared with that in the control (B0). The existence of base functional groups, including chromenes, ketones, and pyrones, on biochar, can facilitate NN adsorption to biochar (Nguyen et al., 2017). This study indirectly indicates that FTCs weaken the absorption of NN by biochar, leading to the release of NN in biochar particles. Under FT conditions, the adsorption capacity of biochar worsens (Wu et al., 2019), and the effect of biochar on NN is not affected by the adsorption, but rather by reducing the runoff to mitigate nitrate loss.
Similar to inorganic N, freezing and thawing caused an increase in TP and DP concentrations in the initial runoff, which was mainly related to the damage of soil aggregates and the rapid release of adsorptive P from soil particles under FTCs (Han et al., 2019). Although DP in biochar may enhance soil P availability, increasing the risk of P loss (Li et al., 2019; Han et al., 2019), the application of biochar in this study effectively reduced the TP and DP loss in FT soil. In addition, the increase in the BARs promoted the inhibitory effect of biochar on P loss. In contrast, the restored alpine soil used in this experiment contained low P and other nutrients, and the P molecules could be quickly absorbed by soil particles after it was released from biochar. Moreover, biochar has a good adsorption capacity for DP, which can effectively reduce runoff loss under the FT conditions. This may be because biochar has a large number of functional groups and can effectively adsorb free phosphate ions in the soil, thus reducing runoff-scouring soil TP and DP loss (Bashagaluke et al., 2018; Xu et al., 2019). In addition, as mentioned above, biochar application improved soil structure and weakened soil erosion by rainwater, thus reducing the particulate P loss, which has been proven to be the main form of soil P loss in runoff (Zhang et al., 2015b; Li et al., 2021).
Under FTCs treatments, BARs had a significant influence on total DP loss, while the influence of BARs on total TP runoff loss was not significant, which is not consistent with the second hypothesis of this study. This is likely because, under the effect of freezing and thawing, the increase in biochar addition did not significantly reduce the soil yield, which may not lead to a decrease in particulate P loss. However, the proportion of DP to TP was lower than 50% (Table 4), indicating that a further increase in biochar application could not significantly reduce the TP loss under FTCs.
Compared with non-FTCs (F0) and non-biochar (B0) applied soils, biochar application resulted in an increase in total P concentration in the FT soils, but there was no significant difference in the concentration of residual TP between the two application rates. The low BARs and P content of biochar had limited influence on soil TP, indicating that the biochar reduced P loss mainly by reducing FT effects. In contrast, biochar application significantly increased DP residues in both FTCs and non-FTCs soils. This is due to the high DP content (156.4 mg kg−1) of biochar and the low initial soil DP concentration (3.27 mg kg−1). Therefore, the DP of biochar has a great influence on soil DP, which superimposes the weakening effect of biochar on P release under FT, thereby increasing soil DP residue. Therefore, biochar plays a paramount role in maintaining the soil available P content under FTCs. The addition of biochar can provide more nutrients for plants and effectively reduce the damage of FTCs to the soil in the alpine restoration area.
Environmental implication
The Tianshan Mountain region is the largest mountain system found in the arid area, which is an extremely fragile and complex alpine meadow ecosystem caused by the difference in rainfall intensity (Li F. Y. et al., 2020). However, nutrient input in this ecosystem mainly depends on soil mineral weathering and photosynthesis of meadow, and nutrient loss rate caused by soil erosion is much faster than its accumulation rate. This study found that once freeze-thaw occurs, the soil and nutrient loss rate will increase significantly. For mine restoration sites, relying on the natural growth of grass to restore the soil to its original state is undoubtedly a long-term process. Once rainfall and freeze-thaw action occur, this system will become extremely fragile, and an irreversible erosion process will happen. However, the results of laboratory experiments well prove that the application of biochar can not only import nutrients to the soil and improve soil structure but also significantly reduce the negative effects of freeze-thaw on soil erosion and nutrient loss. A large number of agricultural waste resources can be prepared into biochar as an amendment for soil restoration in alpine regions, which is of great significance for alpine ecological environment restoration. However, this study of biochar is still in its infancy, and the experiments related to soil erosion and biochar effects are mostly conducted under laboratory simulation conditions (Li et al., 2021). Therefore, studies or long-term field trials considering more factors including soil, terrain, climate, and land management are needed to evaluate the effects of biochar application on soil erosion and nutrient loss under freeze-thaw conditions.
Conclusion
Although soil N and P loss patterns were different under FTCs, our results suggests that biochar addition can significantly reduce the magnitude of runoff and sediment loss from soil, which in turn limits soil nutrient loss (e.g., N and P) in the alpine mine restoration area. However, the increase in FTCs weakened the inhibitory effect of biochar addition. The results of this study were obtained under laboratory simulation conditions, and the relevant results need to be verified in the actual alpine area. In addition, only one slope and rainfall intensity were used in this study, which may be completely different from the actual terrain and climate conditions. All these need to be considered in future studies. Nonetheless, this study provides a valuable reference for the rapid restoration of the ecological environment and the reduction of soil and water pollution and nutrient losses in the alpine mine restoration area.
Data availability statement
The original contributions presented in the study are included in the article/Supplementary Material, further inquiries can be directed to the corresponding author.
Author contributions
FL, DW, and GL contributed to the conception and design of the study. FL, XH, and YY, OD organized the database. FL performed the statistical analysis. FL and DW wrote the first draft of the manuscript. KE and SK revised the manuscript. All authors contributed to manuscript revision and read and approved the submitted version.
Funding
This research was supported by the Bingtuan Science and Technology Program (2021DB019), Youth and Middle-aged Scientific and Technological Innovation Leading Talents Program of the Corps (2022CB001-01), the National Natural Science Foundation of China (42275014; U1703244; 32060259) and Huazhong Agricultural University and Tarim University joint-fund (HNLH202003).
Conflict of interest
The authors declare that the research was conducted in the absence of any commercial or financial relationships that could be construed as a potential conflict of interest.
Publisher’s note
All claims expressed in this article are solely those of the authors and do not necessarily represent those of their affiliated organizations, or those of the publisher, the editors and the reviewers. Any product that may be evaluated in this article, or claim that may be made by its manufacturer, is not guaranteed or endorsed by the publisher.
Supplementary material
The Supplementary Material for this article can be found online at: https://www.frontiersin.org/articles/10.3389/fenvs.2022.1053843/full#supplementary-material
Abbreviations
FT, Freeze-thaw; FTCs, Freeze-thaw cycles; WHC, Soil water holding capacity; P, Phosphorus; N, Nitrogen; a.s.l, Above sea level; BARs, Biochar application rates; DP, Dissolved phosphorus; TP, Total phosphorus; AN, Ammonia nitrogen; NN, Nitrate nitrogen.
References
Abrol, V., Ben-Hur, M., Verheijen, F. G. A., Keizer, J. J., Martins, M. A. S., Tenaw, H., et al. (2016). Biochar effects on soil water infiltration and erosion under seal formation conditions: Rainfall simulation experiment. J. Soils Sediments 16, 2709–2719. doi:10.1007/s11368-016-1448-8
Ahmadi, S. H., Ghasemi, H., and Sepaskhah, A. R. (2020). Rice husk biochar influences runoff features, soil loss, and hydrological behavior of a loamy soil in a series of successive simulated rainfall events. Catena 192, 104587. doi:10.1016/j.catena.2020.104587
Bashagaluke, J. B., Logah, V., Opoku, A., Sarkodie-Addo, J., and Quansah, C. (2018). Soil nutrient loss through erosion: Impact of different cropping systems and soil amendments in Ghana. PLoS One 13, e0208250. doi:10.1371/journal.pone.0208250
Burrell, L. D., Zehetner, F., Rampazzo, N., Wimmer, B., and Soja, G. (2016). Long-term effects of biochar on soil physical properties. Geoderma 282, 96–102. doi:10.1016/j.geoderma.2016.07.019
Chen, S. (2013). Analysis of soil loss present situation and variation trend in Xinjiang. Sci. Soil Water Conserv. 11, 93–97. doi:10.16843/j.sswc.2013.s1.020
Crooke, W. M., and Simpson, W. E. (1971). Determination of ammonium in Kjeldahl digests of crops by an automated procedure. J. Sci. Food Agric. 22, 9–10. doi:10.1002/jsfa.2740220104
Doan, T. T., Henry-Des-Tureaux, T., Rumpel, C., Janeau, J. L., and Jouquet, P. (2015). Impact of compost, vermicompost and biochar on soil fertility, maize yield and soil erosion in northern vietnam: A three year mesocosm experiment. Sci. Total Environ. 514, 147–154. doi:10.1016/j.scitotenv.2015.02.005
Edwards, A. C., and Cresser, M. S. (1992). Freezing and its effect on chemical and biological properties of soil, China 59–79. doi:10.1007/978-1-4612-2844-8_2
Ejack, L., and Whalen, J. K. (2021). Freeze-thaw cycles release nitrous oxide produced in frozen agricultural soils. Biol. Fertil. Soils 57, 389–398. doi:10.1007/s00374-020-01537-x
El Rasafi, T., Nouri, M., and Haddioui, A. (2021). Metals in mine wastes: Environmental pollution and soil remediation approaches–a review. Geosystem Eng., 32 1–16. doi:10.1080/12269328.2017.1400474
Eltohamy, K. M., Liu, C., Khan, S., Niyungeko, C., Jin, Y., Hosseini, S. H., et al. (2021). An internet-based smart irrigation approach for limiting phosphorus release from organic fertilizer-amended paddy soil. J. Clean. Prod. 293, 126254. doi:10.1016/j.jclepro.2021.126254
Feng, X., Nielsen, L. L., and Simpson, M. J. (2007). Responses of soil organic matter and microorganisms to freeze-thaw cycles. Soil Biol. Biochem. 39, 2027–2037. doi:10.1016/j.soilbio.2007.03.003
Freppaz, M., Williams, B. L., Edwards, A. C., Scalenghe, R., and Zanini, E. (2007). Simulating soil freeze/thaw cycles typical of winter alpine conditions: Implications for N and P availability. Appl. Soil Ecol. 35, 247–255. doi:10.1016/j.apsoil.2006.03.012
Fu, Q., Hou, R., Li, T., Wang, M., and Yan, J. (2018). The functions of soil water and heat transfer to the environment and associated response mechanisms under different snow cover conditions. Geoderma 325, 9–17. doi:10.1016/j.geoderma.2018.03.022
Fu, Q., Yan, J., Li, H., Li, T., Hou, R., Liu, D., et al. (2019a). Effects of biochar amendment on nitrogen mineralization in black soil with different moisture contents under freeze-thaw cycles. Geoderma 353, 459–467. doi:10.1016/j.geoderma.2019.07.027
Fu, Q., Zhao, H., Li, T. X., Hou, R., Liu, D., Ji, Y., et al. (2019b). Effects of biochar addition on soil hydraulic properties before and after freezing-thawing. Catena 176, 112–124. doi:10.1016/j.catena.2019.01.008
Gai, X., Wang, H., Liu, J., Zhai, L., Liu, S., Ren, T., et al. (2014). Effects of feedstock and pyrolysis temperature on biochar adsorption of ammonium and nitrate. PLoS One 9, e113888. doi:10.1371/journal.pone.0113888
Głąb, T., Palmowska, J., Zaleski, T., and Gondek, K. (2016). Effect of biochar application on soil hydrological properties and physical quality of sandy soil. Geoderma 281, 11–20. doi:10.1016/j.geoderma.2016.06.028
Gou, X., Wu, F., Yang, W., Tan, B., Xu, Z., Liu, J., et al. (2014). Effect of changes in seasonal freeze-thaw pattern on nitrogen loss from leaching in the alpine forest soil. Huanjing Kexue Xuebao/Acta Sci. Circumstantiae 34, 439–448. doi:10.13671/j.hjkxxb.2014.02.029
Han, Y., Chen, X., and Choi, B. (2019). Effect of freeze-thaw cycles on phosphorus fractions and their availability in biochar-amended mollisols of northeast China (Laboratory Experiment). Sustainability 11, 1006. doi:10.3390/su11041006
Hseu, Z. Y., Jien, S. H., Chien, W. H., and Liou, R. C. (2014). Impacts of biochar on physical properties and erosion potential of a mudstone slopeland soil. Sci. World J. 2014, 602197. doi:10.1155/2014/602197
Jackson-Blake, L., Helliwell, R. C., Britton, A. J., Gibbs, S., Coull, M. C., and Dawson, L. (2012). Controls on soil solution nitrogen along an altitudinal gradient in the Scottish uplands. Sci. Total Environ. 431, 100–108. doi:10.1016/j.scitotenv.2012.05.019
Ji, Z. Y., Zhou, J. X., Zhang, H., Guo, K. L., Liu, X., Jiang, H. M., et al. (2019). Effect of soil conditioners on the soil chemical properties and organic carbon pool of saline-sodic soil. J. Agro-Environment Sci. 38, 1759–1767. doi:10.11654/jaes.2019-0426
Jiang, N., Juan, Y., Tian, L., Chen, X., Sun, W., and Chen, L. (2018). Modification of the composition of dissolved nitrogen forms, nitrogen transformation processes, and diversity of bacterial communities by freeze–thaw events in temperate soils. Pedobiol. (Jena) 71, 41–49. doi:10.1016/j.pedobi.2018.08.004
Joseph, G., and Henry, H. A. L. (2008). Soil nitrogen leaching losses in response to freeze-thaw cycles and pulsed warming in a temperate old field. Soil Biol. Biochem. 40, 1947–1953. doi:10.1016/j.soilbio.2008.04.007
Keeney, D., and Nelson, D. (1982). “Nitrogen–inorganic forms,” in Methods of soil analysis, Part 2. Chemical and microbiological properties. Editors A. Page, R. Miller, and D. Keeney (Madison, WI: SSSA and ASA), 643–693.
Kim, W. K., Shim, T., Kim, Y. S., Hyun, S., Ryu, C., Park, Y. K., et al. (2013). Characterization of cadmium removal from aqueous solution by biochar produced from a giant Miscanthus at different pyrolytic temperatures. Bioresour. Technol. 138, 266–270. doi:10.1016/j.biortech.2013.03.186
Laird, D. A., Fleming, P., Davis, D. D., Horton, R., Wang, B., and Karlen, D. L. (2010). Impact of biochar amendments on the quality of a typical Midwestern agricultural soil. Geoderma 158, 443–449. doi:10.1016/j.geoderma.2010.05.013
Lehmann, J., Gaunt, J., and Rondon, M. (2006). Bio-char sequestration in terrestrial ecosystems - a review. Mitig. Adapt. Strateg. Glob. Chang. 11, 403–427. doi:10.1007/s11027-005-9006-5
Li, F., Jin, Y., He, S., Jin, J., Wang, Z., Khan, S., et al. (2021a). Use of polyacrylamide modified biochar coupled with organic and chemical fertilizers for reducing phosphorus loss under different cropping systems. Agric. Ecosyst. Environ. 310, 107306. doi:10.1016/j.agee.2021.107306
Li, F., Luo, R., You, Y., Hu, X., Qian, X., Zhang, P., et al. (2022). Alternate freezing and thawing enhanced the sediment and nutrient runoff loss in the restored soil of the alpine mining area. J. Mt. Sci. 19, 1823–1837. doi:10.1007/s11629-021-7143-2
Li, F. Y., Liang, X. Q., Niyungeko, C., Sun, T., Liu, F., and Arai, Y. (2019a). Effects of biochar amendments on soil phosphorus transformation in agricultural soils. Adv. Agron. 158, 131–172. doi:10.1016/bs.agron.2019.07.002
Li, F. Y., Yuan, C. Y., Yuan, Z. Q., You, Y. J., Hu, X. F., Wang, S., Li, G. Y., et al. (2020a). Bioavailable phosphorus distribution in alpine meadow soil is affected by topography in the Tian Shan Mountains. J. Mt. Sci. 17, 410–422. doi:10.1007/s11629-019-5705-3
Li, T., Yu, P., Liu, D., Fu, Q., Hou, R., Zhao, H., et al. (2021b). Effects of biochar on sediment transport and rill erosion after two consecutive years of seasonal freezing and thawing. Sustainability 13, 6984. doi:10.3390/su13136984
Li, Y., Feng, G., Tewolde, H., Yang, M., and Zhang, F. (2020b). Soil, biochar, and nitrogen loss to runoff from loess soil amended with biochar under simulated rainfall. J. Hydrol. X. 591, 125318. doi:10.1016/j.jhydrol.2020.125318
Li, Y., Yu, H., Liu, L., and Yu, H. (2021c). Application of co-pyrolysis biochar for the adsorption and immobilization of heavy metals in contaminated environmental substrates. J. Hazard. Mat. 420, 126655. doi:10.1016/j.jhazmat.2021.126655
Li, Y., Zhang, F., Yang, M., Zhang, J., and Xie, Y. (2019b). Impacts of biochar application rates and particle sizes on runoff and soil loss in small cultivated loess plots under simulated rainfall. Sci. Total Environ. 649, 1403–1413. doi:10.1016/j.scitotenv.2018.08.415
Liu, H., Yang, Y., Zhang, K., and Sun, C. (2017). Soil erosion as affected by freeze-thaw regime and initial soil moisture content. Soil Sci. Soc. Am. J. 81, 459–467. doi:10.2136/sssaj2016.08.0271
Liu, Y., Lonappan, L., Brar, S. K., and Yang, S. (2018). Impact of biochar amendment in agricultural soils on the sorption, desorption, and degradation of pesticides: A review. Sci. Total Environ. 645, 60–70. doi:10.1016/j.scitotenv.2018.07.099
Männistö, M. K., Tiirola, M., and Häggblom, M. M. (2009). Effect of freeze-thaw cycles on bacterial communities of Arctic tundra soil. Microb. Ecol. 58, 621–631. doi:10.1007/s00248-009-9516-x
Moragues-Saitua, L., Arias-González, A., and Gartzia-Bengoetxea, N. (2017). Effects of biochar and wood ash on soil hydraulic properties: A field experiment involving contrasting temperate soils. Geoderma 305, 144–152. doi:10.1016/j.geoderma.2017.05.041
Murphy, J., and Riley, J. P. (1962). A modified single solution method for the determination of phosphate in natural waters. Anal. Chim. Acta X. 27, 31–36. doi:10.1016/S0003-2670(00)88444-5
Nelissen, V., Rütting, T., Huygens, D., Staelens, J., Ruysschaert, G., and Boeckx, P. (2012). Maize biochars accelerate short-term soil nitrogen dynamics in a loamy sand soil. Soil Biol. Biochem. 55, 20–27. doi:10.1016/j.soilbio.2012.05.019
Nguyen, T. T. N., Xu, C. Y., Tahmasbian, I., Che, R., Xu, Z., Zhou, X., et al. (2017). Effects of biochar on soil available inorganic nitrogen: A review and meta-analysis. Geoderma 288, 79–96. doi:10.1016/j.geoderma.2016.11.004
Nyambo, P., Taeni, T., Chiduza, C., and Araya, T. (2018). Effects of maize residue biochar amendments on soil properties and soil loss on acidic hutton soil. Agronomy 8, 256. doi:10.3390/agronomy8110256
Olsen, S. R., and Sommers, L. E. (1982). “Phosphorus,” in Methods of soil analysis Part 2 chemical and microbiological properties. Editor A. L. Page (Madison: American Society of Agronomy, Soil Science Society of America), 403–430.
Oztas, T., and Fayetorbay, F. (2003). Effect of freezing and thawing processes on soil aggregate stability. Catena 52, 1–8. doi:10.1016/S0341-8162(02)00177-7
Pagel, H., Ilg, K., Siemens, J., and Kaupenjohann, M. (2008). Total phosphorus determination in colloid-containing soil solutions by enhanced persulfate digestion. Soil Sci. Soc. Am. J. 72, 786–790. doi:10.2136/sssaj2007.0178n
Sahin, U., Angin, I., and Kiziloglu, F. M. (2008). Effect of freezing and thawing processes on some physical properties of saline-sodic soils mixed with sewage sludge or fly ash. Soil Tillage Res. 99, 254–260. doi:10.1016/j.still.2008.03.001
Statistic Bureau of Xinjiang (2013). Xinjiang statistical yearbook. Beijing: China Statistical Publishing House.
Tammeorg, P., Parviainen, T., Nuutinen, V., Simojoki, A., Vaara, E., and Helenius, J. (2014). Effects of biochar on earthworms in arable soil: Avoidance test and field trial in boreal loamy sand. Agric. Ecosyst. Environ. 191, 150–157. doi:10.1016/j.agee.2014.02.023
Urakawa, R., Shibata, H., Kuroiwa, M., Inagaki, Y., Tateno, R., Hishi, T., et al. (2014). Effects of freeze-thaw cycles resulting from winter climate change on soil nitrogen cycling in ten temperate forest ecosystems throughout the Japanese archipelago. Soil Biol. Biochem. 74, 82–94. doi:10.1016/j.soilbio.2014.02.022
Wang, D., Li, C., Parikh, S. J., and Scow, K. M. (2019). Impact of biochar on water retention of two agricultural soils – a multi-scale analysis. Geoderma 340, 185–191. doi:10.1016/j.geoderma.2019.01.012
Wei, X., Huang, C., Wei, N., Zhao, H., He, Y., and Wu, X. (2019). The impact of freeze–thaw cycles and soil moisture content at freezing on runoff and soil loss. Land Degrad. Dev. 30, 3243–523. doi:10.1002/ldr.3243
Wu, Z., Cheng, H., Wang, J., Cheng, Q., Lu, L., and Sun, H. (2019). Effects of biochar addition rate and freeze-thaw cycles on soil nitrogen leaching in agricultural ditches. Huanjing Kexue Xuebao/Acta Sci. Circumstantiae 39, 1295–1302. doi:10.13671/j.hjkxxb.2018.0417
Xie, S. B., Jian-jun, Q., Yuan-ming, L., Zhi-wei, Z., and Xiang-tian, X. (2015). Effects of freeze-thaw cycles on soil mechanical and physical properties in the Qinghai-Tibet Plateau. J. Mt. Sci. 12, 999–1009. doi:10.1007/s11629-014-3384-7
Xiong, Z. Y., Wang, Z. F., Long, Y., Yan, D. C., Xu, G. X., and Gao, M. (2020). Response of nitrogen loss flux in purple soil sloping field to reduced fertilizer and combining straw. Huanjing Kexue/Environmental Sci. 41, 1930–1940. doi:10.13227/j.hjkx.201910002
Xu, M., Wu, J., Yang, G., Zhang, X., Peng, H., Yu, X., et al. (2019). Biochar addition to soil highly increases P retention and decreases the risk of phosphate contamination of waters. Environ. Chem. Lett. 17, 533–541. doi:10.1007/s10311-018-0802-z
Yao, Y., Gao, B., Zhang, M., Inyang, M., and Zimmerman, A. R. (2012). Effect of biochar amendment on sorption and leaching of nitrate, ammonium, and phosphate in a sandy soil. Chemosphere 89, 1467–1471. doi:10.1016/j.chemosphere.2012.06.002
Yuan, C., Li, F., Yuan, Z., Li, G., and Liang, X. (2021). Response of bacterial communities to mining activity in the alpine area of the Tianshan Mountain region, China. Environ. Sci. Pollut. Res. 28, 15806–15818. doi:10.1007/s11356-020-11744-6
Yuan, Z., Liao, Y., Zheng, M., Zhuo, M., Huang, B., Nie, X., et al. (2020). Relationships of nitrogen losses, phosphorus losses, and sediment under simulated rainfall conditions. J. Soil Water Conserv. 75, 231–241. doi:10.2489/JSWC.75.2.231
Zhang, F., Huang, C., Yang, M., Zhang, J., and Shi, W. (2019). Rainfall simulation experiments indicate that biochar addition enhances erosion of loess-derived soils. Land Degrad. Dev. 30, 2272–2286. doi:10.1002/ldr.3399
Zhang, Q., Du, Z. L., Lou, Y., and He, X. (2015a). A one-year short-term biochar application improved carbon accumulation in large macroaggregate fractions. Catena 127, 26–31. doi:10.1016/j.catena.2014.12.009
Zhang, S., Wang, L., Ma, F., Zhang, X., Li, Z., Li, S., et al. (2015). Can arbuscular mycorrhiza and fertilizer management reduce phosphorus runoff from paddy fields? J. Environ. Sci. 33, 211–218. doi:10.1016/j.jes.2015.01.016
Zhang, Z., Ma, W., Feng, W., Xiao, D., and Hou, X. (2016). Reconstruction of soil particle composition during freeze-thaw cycling: A review. Pedosphere 26, 167–179. doi:10.1016/S1002-0160(15)60033-9
Zhou, Y., Berruti, F., Greenhalf, C., Tian, X., and Henry, H. A. L. (2017). Increased retention of soil nitrogen over winter by biochar application: Implications of biochar pyrolysis temperature for plant nitrogen availability. Agric. Ecosyst. Environ. 236, 61–68. doi:10.1016/j.agee.2016.11.011
Keywords: freeze-thaw (FT), biochar, soil erosion, nitrogen loss, phosphorus loss
Citation: Li F, Wang D, You Y, Li G, Eltohamy KM, Khan S and Riaz L (2022) The application of biochar mitigated the negative effects of freeze-thaw on soil and nutrient loss in the restored soil of the alpine mining area. Front. Environ. Sci. 10:1053843. doi: 10.3389/fenvs.2022.1053843
Received: 26 September 2022; Accepted: 24 October 2022;
Published: 03 November 2022.
Edited by:
Dionisios Gasparatos, Agricultural University of Athens, GreeceReviewed by:
Ahmed H. El-Sappah, Zagazig University, EgyptAansa Rukya Saleem, Bahria University, Pakistan
Copyright © 2022 Li, Wang, You, Li, Eltohamy, Khan and Riaz. This is an open-access article distributed under the terms of the Creative Commons Attribution License (CC BY). The use, distribution or reproduction in other forums is permitted, provided the original author(s) and the copyright owner(s) are credited and that the original publication in this journal is cited, in accordance with accepted academic practice. No use, distribution or reproduction is permitted which does not comply with these terms.
*Correspondence: Desheng Wang, d2RzMTg1OEAxNjMuY29t