- 1Instituto de Ciencias, Benemérita Universidad Autónoma de Puebla, Puebla, Mexico
- 2Facultad de Ingeniería Química, Benemérita Universidad Autónoma de Puebla, Puebla, Mexico
Antibiotics used in humans and farmed animals are an essential source of water and soil contamination. Ampicillin is a micropollutant commonly found in water, sludge, food, flora, and fauna. However, the methods used for its detection in environmental samples are often complicated and expensive. Therefore, developing more straightforward strategies to detect well-known target antibiotics is necessary. In this context, enzyme-based detection methods have been demonstrated to be selective, sensitive, rapid, and relatively simple. In this study, a fluorescent byproduct from the ampicillin oxidation using Chloroperoxidase (CPO) enzyme was used as a pointer compound to determine ampicillin concentration in environmental water samples. We oxidized 80% ampicillin for 1h, producing a fluorescent compound with m/z 274.2517. A response surface methodology (RSM) based on a central composite design (CCD) was used to evaluate and optimize the effects of hydrogen peroxide, enzyme concentration, and time as independent variables on the maximum fluorescence signal as the response function. The methodology proposes to build a calibration curve that relates the initial concentration of ampicillin with fluorescence intensity after the reaction with CPO, which helps detect ampicillin in the concentration range from 0.035 to 40 μM, with a limit of detection of 0.026 μM. The application of the method to fortified environmental water samples allowed percentages of recovery from 86 to 140%. The formation of the fluorescent compound was not affected by the presence of salts commonly found in wastewater; however, it was affected by other antibiotics. The proposed methodology was tested in the context of water from water bodies, urban, and WWTP effluents.
Introduction
Antibiotics are the third best-selling medicines worldwide. Between 2000 and 2010, antibiotic drug consumption increased by 36% (from 54 billion standard units to 73 billion standard units); Brazil, Russia, India, China, and South Africa had a significant increase in this change (van Boeckel et al., 2014; Senadheera et al., 2020). A total of 50%–75% of antibiotics consumed worldwide are, used in veterinary medicine and animal husbandry (Steinfeld et al., 2009) Globally, antibiotic consumption in livestock reached 63,151 tons in 2010, and an increase of 67%–200% is projected by 2030 (Ibrahim et al., 2020; Senadheera et al., 2020) According to a recently reported spatial modeling study, the global antibiotic consumption rate of 9.8 defined daily doses (DDD) per 1,000 population per day in 2000, increased by 46% from 9.8% in 2018 (14.3 DDD). The model incorporated antibiotic usage and consumption data and used geostatistical modeling techniques to estimate the antibiotic consumption of 204 countries from 2000 to 2018 (Browne et al., 2021). Penicillin, macrolides, and fluoroquinolones are the most commonly used in humans, while tetracyclines, penicillin, and sulfonamides are the most widely used drugs in animals (Ma et al., 2021). Drug residues contaminate water, soil, flora, and fauna because of the low drug absorption both in humans and animals, the use of animal excreta as fertilizer, the poor final disposal of expired antibiotics in homes and hospitals, the WWTP’s low biodegradability, and the high environmental mobility of antibiotics (Lv et al., 2019; Mejías et al., 2021).
Antibiotic-resistant bacteria are the main consequence of antibiotic contamination, which is a current health problem whose prognosis for the future is a worldwide concern (Uddin et al., 2021; Haenni et al., 2022). Therefore, the proper detection of antibiotic residues in the environment is a very active area of current research. Liquid or gas chromatography coupled with mass detectors is the analysis par excellence due to its accuracy, precision, versatility, and sensitivity (di Rocco et al., 2017; Ghosh et al., 2021; Lakew et al., 2022). However, due to the high cost of analysis, the need for highly trained personnel, and the costly infrastructure associated with it, together with a large number of samples to be analyzed, it has been necessary to develop alternative methods and devices for their detection (Mehlhorn et al., 2018; Yue et al., 2021; Gawrońska et al., 2022).
Ampicillin is a micropollutant commonly found in water, sludge, food, flora, and fauna (Kumar et al., 2019; Peris-Vicente et al., 2022). In recent years several methods and devices have been reported for detecting ampicillin in water or food, with successful results in terms of sensitivity, accuracy, and application in environmental mixtures (Soledad-Rodríguez et al., 2017; Sharma et al., 2019; Sta Ana et al., 2021). However, most reported methods and devices are still technically complex and, primarily based on nanomaterials or composites that require specialized production methods, highly controlled working conditions, or sophisticated equipment (Luo et al., 2017; Shrivas et al., 2017; Modh et al., 2018; Shu and Tang, 2020).
Therefore, there is a need for alternative and simple strategies for ampicillin detection, that maintain performance parameters such as accuracy, precision, and sensitivity to obtain reliable results that are the basis of an environmental diagnosis. Nowadays, several studies have focused on methods based on enzyme activity to analyze the presence of pollutants in the environment (Ejeian et al., 2018; Pachapur et al., 2019; Sarkar et al., 2019; Reynoso et al., 2022; Zhai et al., 2022). Given the nature of enzymes, enzyme-based detection methods are selective, sensitive, rapid, and relatively simple to apply. Enzymes have been used to detect organophosphorus pesticides, phenolic compounds, amine compounds, and pharmaceuticals. Among the reported enzymes, Chloroperoxidase (CPO) is a versatile biocatalyst for multipollutant determination due to its wide substrate variability (Rebollar-Pérez et al., 2016; Morsi et al., 2020; Lin, 2021). In addition, CPO’s kinetics and reaction mechanism are known, and its three-dimensional structure is well defined, which may facilitate the eventual elaboration of detection devices.
In this study, a biocatalytic method was developed for detecting ampicillin in environmental water samples using CPO; the procedure was shown to be in the sensitivity range of other reported techniques, accurate, and potentially applicable to the analysis of numerous samples over short times.
Materials and methods
Chemicals
Ampicillin, sulfamethoxazole, tetracycline, and amoxicillin were purchased from Sigma-Aldrich (St. Louis, MO, United States). Chloroperoxidase (CPO) from Caldaromyces fumago was purchased from Alltaenzymes (Edmonton, AB, Canada) with an RZ of 1.4 and specific activity of 22,000 min−1 for the halogenation of monochlorodimedone. Buffer salts, potassium chloride, and hydrogen peroxide were purchased from J.T. Baker (Phillipsburg, NJ, United States).
Biocatalytic oxidation of ampicillin
Ampicillin was enzymatically oxidized in a reaction mixture containing 10–50 µM ampicillin, 1 mM H2O2, 20 mM KCl, and 0.1 µM CPO in phosphate buffer pH 3.0, 60 mM. Reaction progress was analyzed by steady-state fluorescence (Cary Eclipse fluorescence spectrometer from Varian, equipped with a Xe lamp and Czerny-Turner 0.125 monochromators) with an excitation wavelength of 330 nm, followed by the light emission of the reaction products at 450 nm. The assays were completed within 1 h under the tested conditions.
Optimization of reaction conditions
A central composite design (CCD) with three independent variables and three levels—enzyme concentration (0.05, 0.075, and 0.1 µM), reaction time (0.5, 0.75, and 1 h), and H2O2 concentration (0.5, 1.0, and 1.5 mM)— was applied to obtain the highest fluorescence intensity indicative of the greater production of byproducts. Five central and six axial points were included in the design. A total of 35 experiments were conducted according to the CCD. The experimental results were analyzed through a response surface methodology (RSM), using Design-Expert software (trial version 13.0.11.0, United States). The response was related to the selected variable via a mathematical model, and optimization was performed to obtain the highest response values. Optimum values with desirability one were recorded for the variables.
Identification of enzymatic transformation products
Aliquots were collected at different time intervals during the enzymatic oxidation of ampicillin and filtered through 0.2 µm nylon membranes. Then, aliquots were analyzed by chromatography using an LC/DAD/MS instrument (Chromatograph Series 1,260 from Agilent Technologies, Santa Clara, CA, United States) to determine ampicillin concentration over time and to observe the byproducts formed. Separation was performed on an Agilent Eclipse Plus C18 column (4.6 × 100 mm, 3.5 µm). The mobile phases consisted of water (A) and acetonitrile (B), both with 0.1% (v/v) formic acid. The following elution program was employed: 90%A for the first 2 min; changed linearly to 40% (2–12 min); then, again, 90% A from 12 to 12.5 min. Finally, the column was re-equilibrated for 2.5 min with these initial conditions for a total run time of 15 min. The detection by DAD was at 215 and 330 nm. For the mass detector (ESI-Q-TOF-MS 6520 detector, Agilent), ESI source parameters were as follows: positive and negative ionization mode, fragmentor voltage: 175 V, capillary voltage: 3,500 V, gas temperature: 350°C, N2 flow: 11 L min−1, nebulizer pressure: 60 psi.
Chromatographic fractions were collected according to the chromatographic peaks’ retention time, and analyzed by fluorescence spectrometry to identify products with an emission spectrum equivalent to that observed in the biocatalytic oxidation step.
Selectivity tests in the presence of interfering compounds
To identify possible individual chemical interferents, the method was tested in the presence of several salts at the maximum concentrations found in the environmental water samples (KH2PO4 [0.029 g L−1], NaH2PO4 [0.029 g L−1], NaNO3 [0.81 g L−1], NH4NO3 [0.081 g L−1] and CaCl2 [0.195 g L−1]. On the other hand, the assay was also performed in the presence of amoxicillin, tetracycline, sulfamethoxazole, and their mixture with a 30 µM concentration for each. The ampicillin oxidation assay was performed as described in the previous section using 30 µM ampicillin.
Assays on environmental samples
Three different ampicillin-enriched water samples were tested to evaluate possible environmental interferences of the sample matrix on ampicillin detection. First, a detection assay was performed on five samples of water spiked with 5 and 10 µM ampicillin. Samples from the treatment plant’s secondary effluent, lagoon water, drinking water, groundwater, and river water were used. The samples had previously been filtered through nylon membranes of 0.45 µm and stored at 4°C until use. The determined parameters in water samples are summarized in Supplementary Table S1. The enzymatic assay was performed as described in the previous section.
Results and discussion
Ampicillin biocatalysis with chloroperoxidase
CPO was able to oxidize 70% of the ampicillin in the first 20 min. After that, the reaction rate slowed, reaching 80% conversion in 1 h. Compared to other CPO substrates, the oxidation of ampicillin was lower; for example, the oxidation of tetracycline and sulfamethoxazole reached 70% conversion in 4 min (García-Zamora et al., 2018). Fitting the reaction kinetics to a pseudo-first order equation yielded a reaction rate constant of 0.103 min−1 (Supplementary Figure S1); which is similar to that reported for the oxidation of the flame retardant tetrabromobisphenol by CPO (García-Zamora et al., 2019). In addition, some of the oxidation rate or conversion is within the range of physicochemical oxidation, although physicochemical methods have a characteristic that can lead to the mineralization of the compound (Frontistis et al., 2018; Silva et al., 2019; Montoya-Rodríguez et al., 2020).
Ampicillin and its enzymatic oxidation products showed similar electronic absorption spectra (and, in addition, low light absorption, Supplementary Figure S2); in contrast, the oxidized products exhibit fluorescence, which allows differentiation from the basal one, which has negligible fluorescence at the excitation wavelength (Figure 1). The emission spectrum presents a maximum at 460 nm. After 2 h of the biocatalytic process, fluorescent byproducts were observed. Given the reaction conditions and the mechanism reported for CPO, the reaction products are likely to be chlorinated derivatives of ampicillin, although non-chlorinated compounds of lower molecular weight could also be produced in subsequent chemical reactions (García-Zamora et al., 2018; Wang K. et al., 2019; Undiano et al., 2021). Through HPLC-MS, the mass of some oxidation products was observed, and their molecular formula was proposed with a difference of mass/charge Δm/z ≤ 8.02 ppm (Table 1). As can be seen, most oxidation products have a higher molecular mass than ampicillin, which is not surprising because the peroxidase oxidation mechanism follows a free radical mechanism, which can lead to polymerization of some reaction products (Ortiz de Montellano, 2010; Dunford, 2016). The product responsible for fluorescence was identified with m/z 274.2517 and the formula C14H31N3O2, suggesting a fragmentation of the ampicillin molecule upon oxidation. The oxidation kinetics of ampicillin coincides with the appearance of the fluorescent product, which was monitored by mass spectrometry, and fluorescence (Supplementary Figure S3 and Figure 1), which suggests that it is the first oxidation product from the enzymatic reaction. None of the other reaction products identified showed fluorescence under the tested conditions. Hence, it was considered as a pointer compound to indirectly determine the concentration of ampicillin, measuring the fluorescence of samples after the enzymatic reaction, as will be described later.
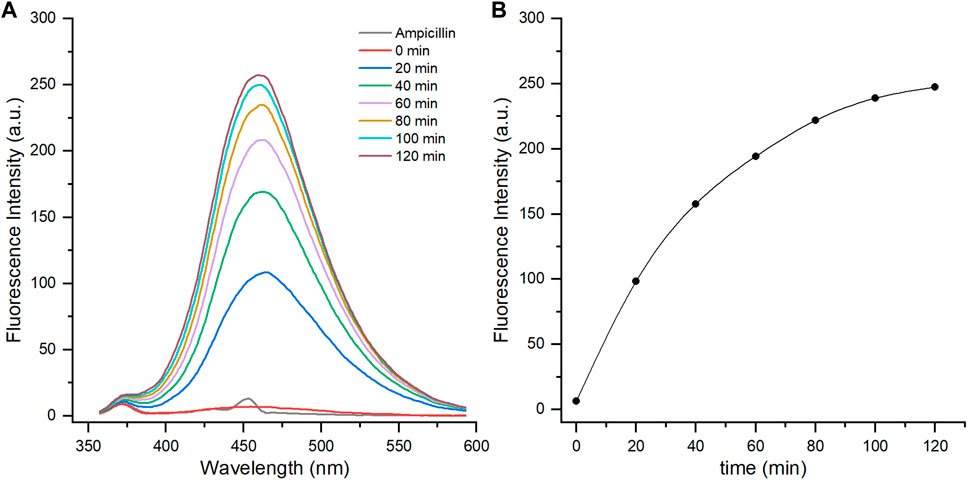
FIGURE 1. (A) Fluorescence emission spectra of a byproduct of an ampicillin biocatalysis by CPO and (B) their appearance kinetics.
Optimization of the detection method by a fluorescent byproduct of biocatalysis
The detection method proposed in this study is based on the formation of a fluorescent byproduct of the oxidation reaction of ampicillin with CPO. The fluorescence response can be influenced by many experimental parameters that should be optimized to obtain the best performance. CCD was selected because it is a design that includes linear, quadratic, and interaction terms and allows for greater level numbers without performing experiments using every combination of factor levels. The enzyme concentration, reaction time, and hydrogen peroxide concentration were optimized among catalytic factors. These three parameters are significant factors in biocatalysis with CPO (Rebollar-Pérez et al., 2016). Since the fluorescent product is one among several, it is crucial to determine the right amount of enzyme and reaction time for its maximum production; moreover, the amount of peroxide must be optimized to prevent the enzyme from becoming inactive and generating less product. Table 2 presents the results of the applied CCD. As can be seen, signal intensities vary from 28.9 to 145 AU. These experimental results were fitted to a second-order polynomial equation, resulting in the following mathematical expression:
The results of the analysis of variance (ANOVA) of the model are shown in Supplementary Table S2. The model and every term, including interaction time*CPO were statistically significant at the 5% level. Furthermore, the coefficient of determination (R2) obtained was 0.96, indicating that the equation adequately represents the relationship between fluorescence intensity and the independent variables.
It was possible to build contour graphs using this model. Figure 2 shows the response surface plot of the significant interaction found in the model. As can be seen, to achieve higher intensity values, it is necessary to increase the time and apply low enzyme concentrations. This last result would seem contradictory given that higher enzyme concentrations increase the transformation of ampicillin. However, a high concentration of the enzyme can trigger a subsequent modification of the fluorescent compound based on the detection at 450 nm, which decreases the fluorescence signal.
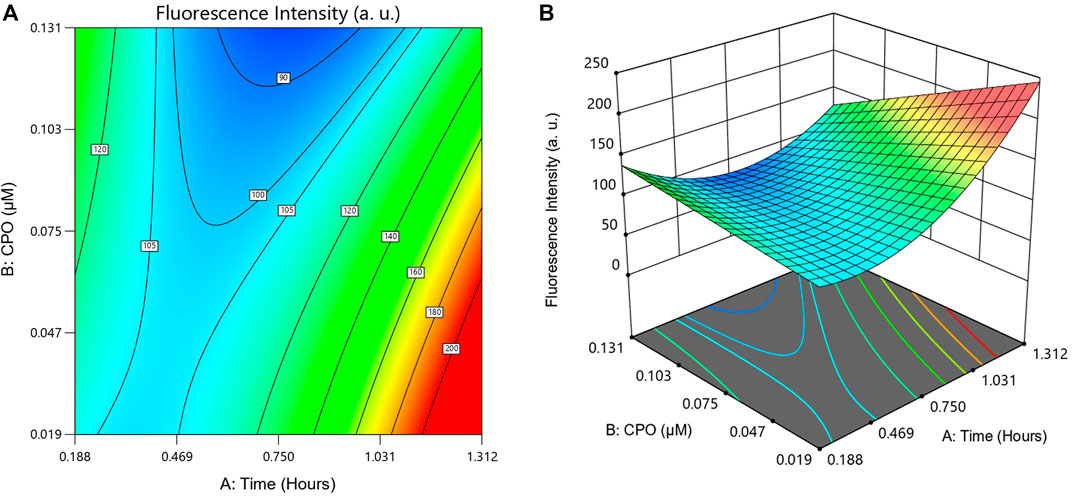
FIGURE 2. (A) Contour plot and (B) response surface plot of the interaction among CPO concentration and time reaction at 1.7 mM H2O2 in relation to fluorescence intensity of ampicillin byproduct.
Response optimization was performed to determine the values of each independent variable that produced maximum fluorescence. Overall desirability is an objective function ranging from 0 (if the optimal values are outside the selected ranges) to 1 (if the goal for the response is reached). Then, optimal values with desirability 1 were 1.1 h, 0.043 µM CPO, and 1.7 mM H2O2, resulting in the model’s theoretical intensity of 167.87 AU. A confirmatory experiment was performed with the predicted optimum conditions, and the experimental fluorescence intensity obtained was 163.788 ± 2.18 AU, validating the fluorescent compound’s production equation to detect ampicillin.
A calibration curve was then generated by applying these conditions at different concentrations of ampicillin (Figure 3, DI water). As can be seen, there is a good fit of the data to a straight line, with a correlation coefficient of 0.99; the line equation, helpful in determining LOD and LOQ is found in Figure 3. The obtained LOD and LOQ of the ampicillin concentration were 0.035 µM and 0.12 µM respectively, and the linear range was from 0.75 to 40 µM. These values are among the best obtained by methods based on a chemical transformation of ampicillin, as shown in Table 3 (analysis methods: 3, 13, 21, and 22); when compared to methods based on nanomaterials, the results are below that performance (analysis methods: 6, 10, 16, and 17 from Table 3). However, the simplicity of the technique confers certain implementation advantages; even so, the LOD value obtained here compares with others produced with more sophisticated methods and devices, such as those based on aptamers (analysis methods: 9, 11, 12, 14, and 15 from Table 3).
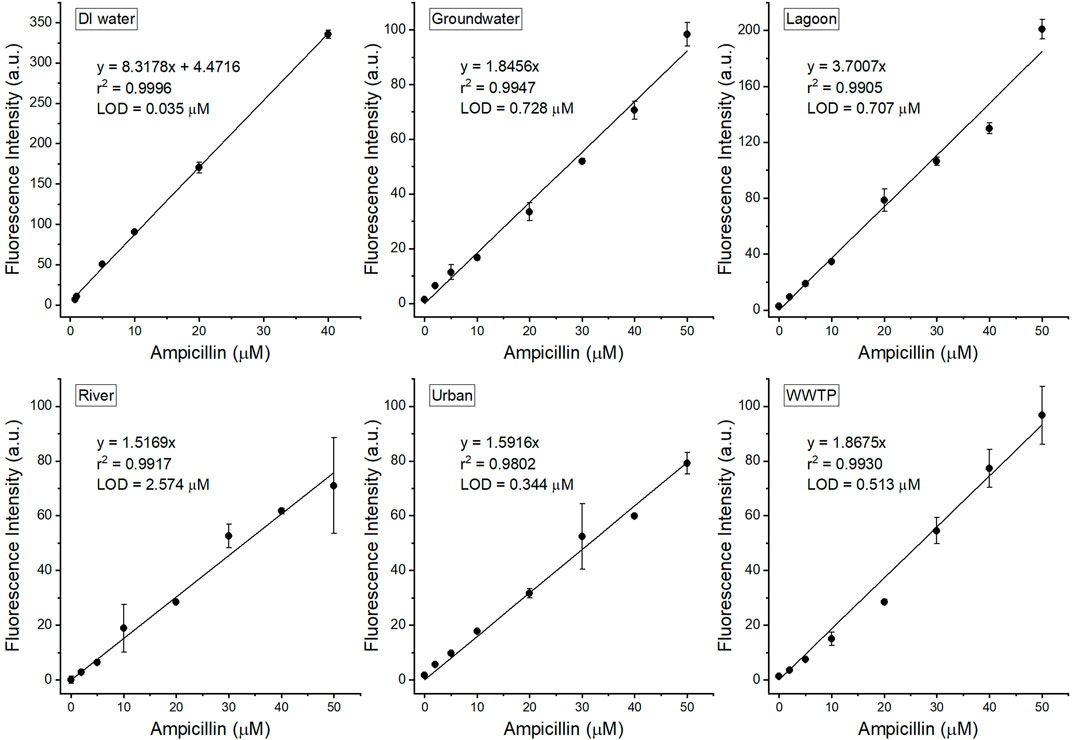
FIGURE 3. Calibration curves and LOD for the detection of ampicillin based on the fluorescence intensity of a byproduct of the biocatalytic degradation of ampicillin by CPO in different environmental water matrices.
The biocatalytic method is simple and does not require extensive training; however, as signal intensity depends on the initial ampicillin concentration, an additional amplification procedure is necessary to improve sensitivity.
Selectivity in the formation of the pointer compound
The selectivity of the assay was tested by determining the catalytic activity effect of CPO caused by salts and other antibiotics (Table 4) and, as a consequence, the formation of the pointer compound. All the salts tested showed less than 10% interference in the ampicillin assay, using the highest salt concentrations found in the environmental samples (see next section). However, in the presence of other antibiotics, catalytic activity was significantly affected because they are also substrates of the enzyme, and CPO could catalyze at the same time as the oxidation of more than one of them, affecting the transformation rate of ampicillin. It has been previously reported that CPO can transform sulfamethoxazole and tetracycline; the conversion of these two antibiotics by CPO was carried out in 4 min under similar conditions, so they are better substrates than ampicillin (García-Zamora et al., 2018). A similar result was reported for determining organophosphorus pesticides by CPO in the presence of some compounds (Rebollar-Pérez et al., 2016). Increasing the reaction time or amount of enzyme did not improve the reaction toward ampicillin oxidation. However, the pointer compound was still produced after a specific time, and the detection was not affected by the presence of other antibiotics. Neither sulfamethoxazole and tetracycline nor their reaction products present fluorescence under these conditions.
Analysis of environmental spiked water samples
The assay was applied to several environmental water samples to test the proposed assay’s applicability. Supplementary Table S1 shows the physicochemical characterization of water samples collected from different sites. The BOD5 values for water samples from the WWTP and the lagoon indicate that the water is contaminated; given the COD values, all water samples are heavily polluted. The vast difference in values between BOD5 and COD is due to the presence of non-biodegradable substances.
The recovery percentage was used to describe the method’s analytical performance and the assay’s precision. Recovery refers to the amount of analyte measured as a percentage of the amount of analyte initially added to a sample of the appropriate matrix, which contains no detectable level of the analyte or a detectable level. As seen in Table 5, the proposed method adequately predicts the concentration of ampicillin at 10 µM in the different water matrices, and the recovery percent ranges from 86 to 107%. However, interfering effects were observed at a lower-range concentration (5 µM ampicillin) with a recovery of 14,474% and a variation of 7%–23%. The method’s accuracy and precision depend on interferents simultaneously present in the matrix, which may have a summative or synergistic effect. To consider this effect, it is advisable to prepare the calibration curve in the environmental matrix in which the analysis will be performed. Figure 3 shows the calibration curves in each environmental matrix used; as can be seen, the fit presents an adequate coefficient of determination, and LOD and LOQ vary slightly, except in the lagoon water matrix, where we can see an effect on fluorescence intensity.
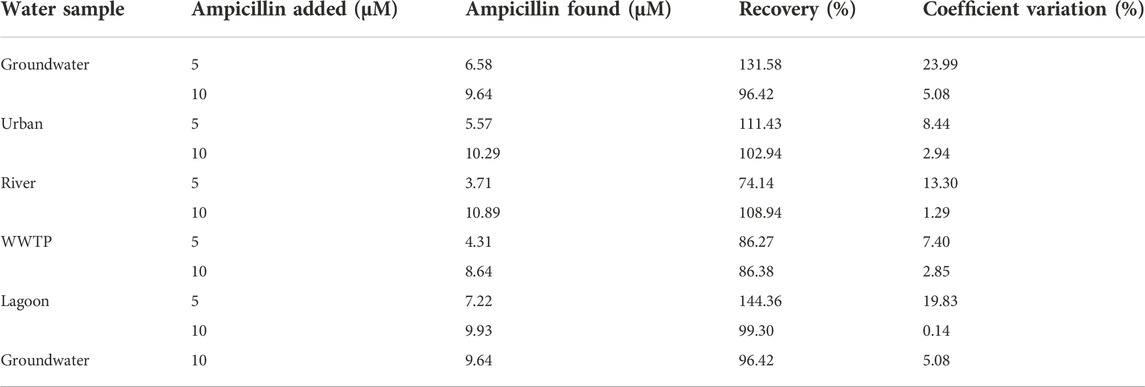
TABLE 5. Analysis of ampicillin in spiked environmental water samples by the proposed biocatalytic method.
Conclusion
The detection of ampicillin in aquatic environmental matrices is relevant given its occurrence in water bodies, urban, and WWTP effluents. The biocatalytic reaction of ampicillin with the CPO enzyme reached around 80% conversion in 1 h, producing a fluorescent compound useful for detecting ampicillin in water samples. The RSM-CCD method optimized enzyme concentration, hydrogen peroxide concentration, and reaction time for fluorescent product formation. The method’s sensitivity allowed ampicillin detection and quantification at the micromolar level (LOD and LOQ of 0.035–0.12 μM, respectively). Enzyme activity was not affected by the presence of salts commonly found in wastewater; however, it was affected by other antibiotics that are even better substrates than ampicillin. Optimizing biocatalysis conditions achieved 86%–140% recovery of spiked ampicillin from different environmental water samples.
Using a straightforward methodology, it was possible to determine ampicillin in different environmental water samples. Detection f compounds of environmental interest using enzymatic methods have a potential application given the mild reaction conditions and simple implementation steps; these methods are also fast and selective. However, there is a need to improve the sensitivity at nanomolar or picomolar levels; this could be achieved by pre-treating the sample to concentrate it thousands of times or amplifying the enzymatic reaction’s response signal.
Data availability statement
The original contributions presented in the study are included in the article/Supplementary Material, further inquiries can be directed to the corresponding author.
Author contributions
JG-Z, Biocatalytic oxidation, identification of reaction products; JA-A, selectivity, detection, and quantification limits; GR-P, Optimization of the detection method, FP-A, Statistical analysis, EG-D, methodology, writing-review, and editing, ET, Conceptualization, supervision, funding acquisition, writing-review, and editing.
Funding
The authors gratefully acknowledge the financial support of the Consejo Estatal de Ciencia y Tecnología de Puebla (CONCYTEP) and the Vicerrectoría de Investigación y Estudios de Posgrado (VIEP-BUAP). The authors are grateful for the financial support of CONACYT for JG-Z with the scholarship (769944).
Conflict of interest
The authors declare that the research was conducted in the absence of any commercial or financial relationships that could be construed as a potential conflict of interest.
Publisher’s note
All claims expressed in this article are solely those of the authors and do not necessarily represent those of their affiliated organizations, or those of the publisher, the editors and the reviewers. Any product that may be evaluated in this article, or claim that may be made by its manufacturer, is not guaranteed or endorsed by the publisher.
Supplementary material
The Supplementary Material for this article can be found online at: https://www.frontiersin.org/articles/10.3389/fenvs.2022.1040903/full#supplementary-material
References
Blidar, A., Feier, B., Tertis, M., Galatus, R., and Cristea, C. (2019). Electrochemical surface plasmon resonance (EC-SPR) aptasensor for ampicillin detection. Anal. Bioanal. Chem. 411, 1053–1065. doi:10.1007/s00216-018-1533-5
Browne, A. J., Chipeta, M. G., Haines-Woodhouse, G., Kumaran, E. P. A., Hamadani, B. H. K., Zaraa, S., et al. (2021). Global antibiotic consumption and usage in humans, 2000–18: A spatial modelling study. Lancet Planet Health 5, e893. doi:10.1016/S2542-5196(21)00280-1
Darweesh, S. A., Yaseen, H. M., Mahmood, R. M., Al-Khalisy, R. S., and Khalaf, H. S. (2020). Spectrophotometric determination of ampicillin with sulfanilic acid by oxidative coupling reaction. Indian J. Forensic Med. Toxicol. 14, 2016–2021. doi:10.37506/ijfmt.v14i4.11844
di Rocco, M., Moloney, M., O'Beirne, T., Earley, S., Berendsen, B., Furey, A., et al. (2017). Development and validation of a quantitative confirmatory method for 30 β-lactam antibiotics in bovine muscle using liquid chromatography coupled to tandem mass spectrometry. J. Chromatogr. A 1500, 121–135. doi:10.1016/j.chroma.2017.04.022
Dunford, H. B. (2016). “Chapter 5 heme peroxidase kinetics,” in The royal society of chemistry (London, UK, 99–112. doi:10.1039/9781782622628-00099doi:RSC Publishing
Ejeian, F., Etedali, P., Mansouri-Tehrani, H.-A., Soozanipour, A., Low, Z.-X., Asadnia, M., et al. (2018). Biosensors for wastewater monitoring: A review. Biosens. Bioelectron. 118, 66–79. doi:10.1016/j.bios.2018.07.019
Frontistis, Z., Mantzavinos, D., and Meriç, S. (2018). Degradation of antibiotic ampicillin on boron-doped diamond anode using the combined electrochemical oxidation - sodium persulfate process. J. Environ. Manage 223, 878–887. doi:10.1016/j.jenvman.2018.06.099
Fu, Y., Zhao, S., Wu, S., Huang, L., Xu, T., Xing, X., et al. (2020). A carbon dots-based fluorescent probe for turn-on sensing of ampicillin. Dyes Pigments 172, 107846. doi:10.1016/j.dyepig.2019.107846
Gao, J., Chen, Y., Ji, W., Gao, Z., and Zhang, J. (2019). Synthesis of a CdS-decorated Eu-MOF nanocomposite for the construction of a self-powered photoelectrochemical aptasensor. Analyst 144, 6617–6624. doi:10.1039/C9AN01606F
García-Zamora, J. L., León-Aguirre, K., Quiroz-Morales, R., Parra-Saldívar, R., Gómez-Patiño, M., Arrieta-Baez, D., et al. (2018). Chloroperoxidase-mediated halogenation of selected pharmaceutical micropollutants. Catalysts 8, 32. doi:10.3390/catal8010032
García-Zamora, J. L., Santacruz-Vázquez, V., Valera-Pérez, M. Á., Moreira, M. T., Cardenas-Chavez, D. L., Tapia-Salazar, M., et al. (2019). Oxidation of flame retardant tetrabromobisphenol a by a biocatalytic nanofiber of chloroperoxidase. Int. J. Environ. Res. Public Health 16. doi:10.3390/ijerph16244917
Gawrońska, M., Kowalik, M., and Makowski, M. (2022). Recent advances in medicinal chemistry of ampicillin: Derivatives, metal complexes, and sensing approaches. TrAC Trends Anal. Chem. 155, 116691. doi:10.1016/j.trac.2022.116691
Ghosh, B., Sengar, A., Ahamad, A., and Waris, R. F. (2021). “Pharmaceuticals and personal care products: Occurrence, detection, risk, and removal technologies in aquatic environment,” in Contamination of water (Amsterdam, Netherlands: Elsevier), 265–284. doi:10.1016/B978-0-12-824058-8.00021-9
Haenni, M., Dagot, C., Chesneau, O., Bibbal, D., Labanowski, J., Vialette, M., et al. (2022). Environmental contamination in a high-income country (France) by antibiotics, antibiotic-resistant bacteria, and antibiotic resistance genes: Status and possible causes. Environ. Int. 159, 107047. doi:10.1016/j.envint.2021.107047
Hamami, M., Bouaziz, M., Raouafi, N., Bendounan, A., and Korri-Youssoufi, H. (2021). MoS2/PPy nanocomposite as a transducer for electrochemical aptasensor of ampicillin in river water. Biosens. (Basel) 11, 311. doi:10.3390/bios11090311
Ibrahim, M., Ahmad, F., Yaqub, B., Ramzan, A., Imran, A., Afzaal, M., et al. (2020). “Current trends of antimicrobials used in food animals and aquaculture,” in Antibiotics and antimicrobial resistance genes in the environment (Amsterdam, Netherlands: Elsevier), 39–69. doi:10.1016/B978-0-12-818882-8.00004-8
Iranifam, M., and Kharameh, M. K. (2014). Determination of ampicillin sodium using the cupric oxide nanoparticles-luminol-H2O2 chemiluminescence reaction. Luminescence 29, 679–683. doi:10.1002/bio.2606
Kumar, M., Jaiswal, S., Sodhi, K. K., Shree, P., Singh, D. K., Agrawal, P. K., et al. (2019). Antibiotics bioremediation: Perspectives on its ecotoxicity and resistance. Environ. Int. 124, 448–461. doi:10.1016/j.envint.2018.12.065
Lakew, A., Assefa, T., Woldeyohannes, M., Megersa, N., and Chandravanshi, B. S. (2022). Development and validation of liquid chromatography method for simultaneous determination of multiclass seven antibiotic residues in chicken tissues. BMC Chem. 16, 5. doi:10.1186/s13065-022-00797-y
Li, T., Xu, X., Fu, S., Zhang, J., Zhang, K., Wang, S., et al. (2014). Structural elucidation of stress degradation products of ampicillin sodium by liquid chromatography/hybrid triple quadrupole linear ion trap mass spectrometry and liquid chromatography/hybrid quadrupole time-of-flight mass spectrometry. Rapid Commun. Mass Spectrom. 28, 1929–1936. doi:10.1002/rcm.6970
Li, Y., Tang, Y., Yao, H., and Fu, J. (2003). Determination of ampicillin and amoxycillin by flow injection chemiluminescence method based on their enhancing effects on the luminol-periodate reaction. Luminescence 18, 313–317. doi:10.1002/bio.741
Lin, H., Fang, F., Zang, J., Su, J., Tian, Q., Kumar Kankala, R., et al. (2020). A fluorescent sensor-assisted paper-based competitive lateral flow immunoassay for the rapid and sensitive detection of ampicillin in hospital wastewater. Micromachines (Basel) 11, 431. doi:10.3390/mi11040431
Lin, Y.-W. (2021). Biodegradation of aromatic pollutants by metalloenzymes: A structural-functional-environmental perspective. Coord. Chem. Rev. 434, 213774. doi:10.1016/j.ccr.2021.213774
Liu, X., Hu, M., Wang, M., Song, Y., Zhou, N., He, L., et al. (2019). Novel nanoarchitecture of Co-MOF-on-TPN-COF hybrid: Ultralowly sensitive bioplatform of electrochemical aptasensor toward ampicillin. Biosens. Bioelectron. 123, 59–68. doi:10.1016/j.bios.2018.09.089
Luo, Z., Wang, Y., Lu, X., Chen, J., Wei, F., Huang, Z., et al. (2017). Fluorescent aptasensor for antibiotic detection using magnetic bead composites coated with gold nanoparticles and a nicking enzyme. Anal. Chim. Acta 984, 177–184. doi:10.1016/j.aca.2017.06.037
Lv, J., Zhang, L., Chen, Y., Ye, B., Han, J., and Jin, N. (2019). Occurrence and distribution of pharmaceuticals in raw, finished, and drinking water from seven large river basins in China. J. Water Health 17, 477–489. doi:10.2166/wh.2019.250
Ma, F., Xu, S., Tang, Z., Li, Z., and Zhang, L. (2021). Use of antimicrobials in food animals and impact of transmission of antimicrobial resistance on humans. Biosaf. Health 3, 32–38. doi:10.1016/j.bsheal.2020.09.004
Manirul Haque, S. K. (2021). Optimized and validated spectrophotometric method for the determination of ampicillin in pharmaceutical formulations. Int. J. Pharm. Pharm. Sci., 34–38. doi:10.22159/ijpps.2021v13i2.40025
Mehlhorn, A., Rahimi, P., and Joseph, Y. (2018). Aptamer-based biosensors for antibiotic detection: A review. Biosens. (Basel) 8, 54. doi:10.3390/bios8020054
Mejías, C., Martín, J., Santos, J. L., Aparicio, I., and Alonso, E. (2021). Occurrence of pharmaceuticals and their metabolites in sewage sludge and soil: A review on their distribution and environmental risk assessment. Trends Environ. Anal. Chem. 30, e00125. doi:10.1016/j.teac.2021.e00125
Modh, H., Scheper, T., and Walter, J.-G. (2018). Aptamer-modified magnetic beads in biosensing. Sensors 18, 1041. doi:10.3390/s18041041
Montoya-Rodríguez, D. M., Serna-Galvis, E. A., Ferraro, F., and Torres-Palma, R. A. (2020). Degradation of the emerging concern pollutant ampicillin in aqueous media by sonochemical advanced oxidation processes - parameters effect, removal of antimicrobial activity and pollutant treatment in hydrolyzed urine. J. Environ. Manage 261, 110224. doi:10.1016/j.jenvman.2020.110224
Morsi, R., Bilal, M., Iqbal, H. M. N., and Ashraf, S. S. (2020). Laccases and peroxidases: The smart, greener and futuristic biocatalytic tools to mitigate recalcitrant emerging pollutants. Sci. Total Environ. 714, 136572. doi:10.1016/j.scitotenv.2020.136572
Ortiz de Montellano, P. R. (2010). “Catalytic mechanisms of heme peroxidases,” in Biocatalysis based on heme peroxidases: Peroxidases as potential industrial biocatalysts. Editors E. Torres, and M. Ayala (Berlin, Heidelberg: Springer Berlin Heidelberg), 79–107. doi:10.1007/978-3-642-12627-7_5
Pachapur, P. K., Larios Martínez, A. D., Pulicharla, R., Pachapur, V. L., Brar, S. K., and Galvez-Cloutier, R. (2019). “Chapter 11 - advances in protein/enzyme-based biosensors for the detection of pesticide contaminants in the environment,” in Tools, techniques and protocols for monitoring environmental contaminants. Editors S. Kaur Brar, K. Hegde, and V. L. Pachapur (Amsterdam, Netherlands: Elsevier), 231–243. doi:10.1016/B978-0-12-814679-8.00011-X
Peris-Vicente, J., Peris-García, E., Albiol-Chiva, J., Durgbanshi, A., Ochoa-Aranda, E., Carda-Broch, S., et al. (2022). Liquid chromatography, a valuable tool in the determination of antibiotics in biological, food and environmental samples. Microchem. J. 177, 107309. doi:10.1016/j.microc.2022.107309
Rebollar-Pérez, G., Lima-Zambrano, F., Bairán, G., Rodríguez-Enríquez, A., Ornelas-Soto, N., Méndez, E., et al. (2016). Colorimetric assay for detection of organophosphorus pesticides by decrease of standard catalytic activity of chloroperoxidase. Environ. Eng. Sci. 33, 951–961. doi:10.1089/ees.2016.0018
Reynoso, E. C., Romero-Guido, C., Rebollar-Pérez, G., and Torres, E. (2022). “Chapter 16 - enzymatic biosensors for the detection of water pollutants,” in Nanomaterials for biocatalysis. Editors G. R. Castro, A. K. Nadda, T. A. Nguyen, X. Qi, and G. Yasin (Amsterdam, Netherlands: Elsevier), 463–511. doi:10.1016/B978-0-12-824436-4.00012-5
Sarkar, A., Sarkar, K. D., Amrutha, V., and Dutta, K. (2019). “Chapter 15 - an overview of enzyme-based biosensors for environmental monitoring,” in Tools, techniques and protocols for monitoring environmental contaminants. Editors S. Kaur Brar, K. Hegde, and V. L. Pachapur (Amsterdam, Netherlands: Elsevier), 307–329. doi:10.1016/B978-0-12-814679-8.00015-7
Senadheera, G. P. S. G., Sri Ranganathan, S., Fernando, G. H., and Fernandopulle, B. M. R. (2020). Antibacterial medicine consumption in private and state sector outpatient settings in colombo district, Sri Lanka. Pharm. J. Sri Lanka 9, 28. doi:10.4038/pjsl.v9i1.49
Sharma, D. K., Sood, S., and Raj, P. (2019). Spectrophotometric determination of amoxicillin, ampicillin, cefalexin and cefadroxil in pharmaceutical formulations, biological fluids and spiked water samples. Anal. Chem. Lett. 9, 345–361. doi:10.1080/22297928.2019.1644194
Shrivas, K., Sahu, J., Maji, P., and Sinha, D. (2017). Label-free selective detection of ampicillin drug in human urine samples using silver nanoparticles as a colorimetric sensing probe. New J. Chem. 41, 6685–6692. doi:10.1039/C7NJ00448F
Shu, J., and Tang, D. (2020). Recent advances in photoelectrochemical sensing: From engineered photoactive materials to sensing devices and detection modes. Anal. Chem. 92, 363–377. doi:10.1021/acs.analchem.9b04199
Silva, F. L., Sáez, C., Lanza, M. R. V., Cañizares, P., and Rodrigo, M. A. (2019). The role of mediated oxidation on the electro-irradiated treatment of amoxicillin and ampicillin polluted wastewater. Catalysts 9. doi:10.3390/catal9010009
Simmons, M. D., Miller, L. M., Sundström, M. O., and Johnson, S. (2020). Aptamer-based detection of ampicillin in urine samples. Antibiotics 9, 655. doi:10.3390/antibiotics9100655
Soledad-Rodríguez, B., Fernández-Hernando, P., Garcinuño-Martínez, R. M., and Durand-Alegría, J. S. (2017). Effective determination of ampicillin in cow milk using a molecularly imprinted polymer as sorbent for sample preconcentration. Food Chem. 224, 432–438. doi:10.1016/j.foodchem.2016.11.097
Song, K.-M., Jeong, E., Jeon, W., Cho, M., and Ban, C. (2012). Aptasensor for ampicillin using gold nanoparticle based dual fluorescence–colorimetric methods. Anal. Bioanal. Chem. 402, 2153–2161. doi:10.1007/s00216-011-5662-3
Sta Ana, K. M., Madriaga, J., and Espino, M. P. (2021). β-Lactam antibiotics and antibiotic resistance in Asian lakes and rivers: An overview of contamination, sources and detection methods. Environ. Pollut. 275, 116624. doi:10.1016/j.envpol.2021.116624
Steinfeld, H., Gerber, P. J., Wassenaar, T., Castel, V., Rosales, M., and de haan, C. (2009). Livestock’s long shadow: Environmental issues and options. https://www.fao.org/3/a0701e/a0701e.pdf.
Tomassetti, M., Merola, G., Martini, E., Campanella, L., Sanzò, G., Favero, G., et al. (2017). Comparison between a direct-flow SPR immunosensor for ampicillin and a competitive conventional amperometric device: Analytical features and possible applications to real samples. Sensors 17, 819. doi:10.3390/s17040819
Uddin, T. M., Chakraborty, A. J., Khusro, A., Zidan, B. M. R. M., Mitra, S., Emran, T. bin, et al. (2021). Antibiotic resistance in microbes: History, mechanisms, therapeutic strategies and future prospects. J. Infect. Public Health 14, 1750–1766. doi:10.1016/j.jiph.2021.10.020
Undiano, E., Roman, R., Miranda-Molina, A., and Ayala, M. (2021). Halogenation of estrogens catalysed by a fungal chloroperoxidase. Nat. Prod. Res. 36, 1–5. doi:10.1080/14786419.2021.1925269
van Boeckel, T. P., Gandra, S., Ashok, A., Caudron, Q., Grenfell, B. T., Levin, S. A., et al. (2014). Global antibiotic consumption 2000 to 2010: An analysis of national pharmaceutical sales data. Lancet Infect. Dis. 14, 742–750. doi:10.1016/S1473-3099(14)70780-7
Wang, K., Huang, X., and Lin, K. (2019a). Multiple catalytic roles of chloroperoxidase in the transformation of phenol: Products and pathways. Ecotoxicol. Environ. Saf. 179, 96–103. doi:10.1016/j.ecoenv.2019.04.061
Wang, T., Yin, H., Zhang, Y., Wang, L., Du, Y., Zhuge, Y., et al. (2019b). Electrochemical aptasensor for ampicillin detection based on the protective effect of aptamer-antibiotic conjugate towards DpnII and Exo III digestion. Talanta 197, 42–48. doi:10.1016/j.talanta.2019.01.010
Xiang, Y., Yu, Z., Liu, D., Han, X., and Zhang, G. (2021). Study on the binding mode of aptamer to ampicillin and its electrochemical response behavior in two different reaction media. Anal. Bioanal. Chem. 413, 6877–6887. doi:10.1007/s00216-021-03646-4
Yan, T., Feng, Y., Ren, X., Li, J., Lu, Y., Sun, M., et al. (2021). Fabrication of CDs hybrid MIL-68(In) derived In2O3In2S3 hollow tubular heterojunction and their exceptional self-powered PEC aptasensing properties for ampicillin detecting. J. Materiomics 7, 721–727. doi:10.1016/j.jmat.2021.01.002
Yan, T., Zhang, X., Ren, X., Lu, Y., Li, J., Sun, M., et al. (2020). Fabrication of N-GQDs and AgBiS2 dual-sensitized ZIFs-derived hollow ZnxCo3xO4 dodecahedron for sensitive photoelectrochemical aptasensing of ampicillin. Sens. Actuators B Chem. 320, 128387. doi:10.1016/j.snb.2020.128387
Yu, Z., and Lai, R. Y. (2018). A reagentless and reusable electrochemical aptamer-based sensor for rapid detection of ampicillin in complex samples. Talanta 176, 619–624. doi:10.1016/j.talanta.2017.08.057
Yu, Z., Sutlief, A. L., and Lai, R. Y. (2018). Towards the development of a sensitive and selective electrochemical aptamer-based ampicillin sensor. Sens. Actuators B Chem. 258, 722–729. doi:10.1016/j.snb.2017.11.193
Yuan, R., Yan, Z., Shaga, A., and He, H. (2021). Design and fabrication of an electrochemical sensing platform based on a porous organic polymer for ultrasensitive ampicillin detection. Sens. Actuators B Chem. 327, 128949. doi:10.1016/j.snb.2020.128949
Yue, F., Li, F., Kong, Q., Guo, Y., and Sun, X. (2021). Recent advances in aptamer-based sensors for aminoglycoside antibiotics detection and their applications. Sci. Total Environ. 762, 143129. doi:10.1016/j.scitotenv.2020.143129
Zhai, R., Chen, G., Liu, G., Huang, X., Xu, X., Li, L., et al. (2022). Enzyme inhibition methods based on Au nanomaterials for rapid detection of organophosphorus pesticides in agricultural and environmental samples: A review. J. Adv. Res. 37, 61–74. doi:10.1016/j.jare.2021.08.008
Keywords: ampicillin, chloroperoxidase biocatalysis, detection method, emerging pollutant, fluorescence
Citation: García-Zamora JL, Alonso-Arenas J, Rebollar-Pérez G, Pacheco-Aguirre FM, García-Diaz E and Torres E (2022) Detection of ampicillin based on the fluorescence of a biocatalytic oxidation product. Front. Environ. Sci. 10:1040903. doi: 10.3389/fenvs.2022.1040903
Received: 09 September 2022; Accepted: 14 November 2022;
Published: 24 November 2022.
Edited by:
Shihai Deng, Xi’an Jiaotong University, ChinaReviewed by:
Binghan Xie, Harbin Institute of Technology, Weihai, ChinaBalendu Shekhar Giri, Indian Institute of Technology Guwahati, India
Copyright © 2022 García-Zamora, Alonso-Arenas, Rebollar-Pérez, Pacheco-Aguirre, García-Diaz and Torres. This is an open-access article distributed under the terms of the Creative Commons Attribution License (CC BY). The use, distribution or reproduction in other forums is permitted, provided the original author(s) and the copyright owner(s) are credited and that the original publication in this journal is cited, in accordance with accepted academic practice. No use, distribution or reproduction is permitted which does not comply with these terms.
*Correspondence: Eduardo Torres, ZWR1YXJkby50b3JyZXNAY29ycmVvLmJ1YXAubXg=