- 1College of Food and Pharmaceutical Sciences, Ningbo University, Ningbo, Zhejiang, China
- 2Lijiang Cheng Hai Bao Er Biological Development Co., Ltd., Lijiang, Yunnan, China
- 3Key Laboratory of Marine Biotechnology of Zhejiang Province, Ningbo University, Ningbo, Zhejiang, China
- 4Center for Biorefining, and Department of Bioproducts and Biosystems Engineering, University of Minnesota-Twin Cities, Saint Paul, MN, United States
Soil is the basis of agricultural production, and the quality of soil directly affects crop quality and yield. Microalgae can carry out photosynthesis, carbon and nitrogen fixation, and produce large amounts of valuable biomass coupled with wastewater treatment. Also, microalgae can produce plant hormones and other high-value products, which can promote plant growth, improve soil fertility, soil ecological health, and control crop diseases. This research reviews the characteristics of microalgae in improving soil health, discusses the situation of microalgae in controlling soil pollutants, elaborates on the technical application of microalgae in alleviating soil problems, and proposes potential applications of microalgae in ecological environment. Also, resource utilization of multifunctional microalgae is discussed, to provide a theoretical basis for the application of microalgae in soil improvement.
Introduction
Soil is the basic substance of the earth’s ecosystem, and an indispensable resource for economic and social development. Soil health is an important part of ecosystem health. As we know, the health soil can grow various products that benefit human health, it can improve the quality of water and the atmosphere, and has a certain degree of anti-pollution capability (Jun et al., 2018). Soil pollution is a ‘centralized’, ‘complex’ and ‘explosive’ problem, and would reduce the soil quality and productivity of cultivated land and thus directly endangers food safety, ecological security, and human health (Nematollahi et al., 2020). It also pollutes groundwater through water conservancy, which seriously damages the stability of soil ecosystems (Perez-Lucas et al., 2019).
Algae include macroalgae and microalgae, while microalgae are a class of low-grade organisms, small in size, simple in structure, but with high photosynthetic efficiency. Microalgae are widely distributed in different habitats, such as soil, oceans, rivers, lakes, and even extreme environments (high salinity, low pH, high temperature, etc.) (Jun et al., 2016; Little et al., 2021). About 40,000 different species of microalgae exist on earth and account for about 20%–30% of the unknown algae in the world (Dahiya, 2015). Microalgae include eukaryotes such as diatoms and green algae, and prokaryotes such as cyanobacteria, which offer the following advantages as soil biofertilizer, owing to their specific characteristics: 1) They can prevent nutrient loss by slowly releasing nitrogen, phosphorus, and potassium to meet the growth needs of plants (Coppens et al., 2016; Sasaki et al., 2020). 2) They also contain trace elements and substances that promote plant growth, such as plant hormones, vitamins, carotenoids, amino acids, and antifungal substances (Silva et al., 2019). These substances can promote crop growth and improve soil fertility. 3) Microalgae reproduce quickly and can be cultivated on a large scale, and their biomass can be directly used for soil inoculation without polluting the surrounding environment. They can also reclaim damaged soil, such as desertified soil and saline-alkali land (Wu et al., 2022).
In recent years, the overuse of fossil fuels has greatly increased energy consumption, as well as the adverse impact of industrial waste on the environment. Apart from industrial waste, agricultural waste is also a major cause of water pollution. Compared with other physical and chemical treatment methods, microalgae biotechnology is a promising method with important potential, to address the energy crisis and wastewater treatment (Randrianarison and Ashraf, 2017; Wollmann et al., 2019).
Moreover, carbon neutrality has become a consensus goal in the global response to climate change, but many challenges to achieve this goal still exist. The novel microalgae can use solar energy to efficiently fix CO2 through photosynthesis; they can convert and fix organic carbon through heterotrophic assimilation and convert them into biofuels, biomaterials, and biofertilizers to replace the use of fossil fuels, plastics, and fertilizers. In agricultural production, different pollutants from wastewater including pesticides, fertilizers, and rural domestic garbage, which contaminates the soil because of the lack of fixed pollution emission points. The use of microalgae could purify different types of wastewaters, and harvest microalgal biomass as a fertilizer (Wuang et al., 2016; Das et al., 2019), and greatly reduce production costs (Tang et al., 2020). Moreover, it can improve soil quality through its own growth, promoting microbial decomposition, improving the transformation and circulation of soil materials, enhancing soil fertility, and providing more usable materials for crops.
Therefore, this review focuses on three aspects: 1) microalgae cultivation for the improvement of soil ecological health, 2) using microalgae to control diseases and soil pollutants and reduce soil crop diseases, and 3) employing microalgae for the wastewater treatment and their resource utilization. This review is to provide a scientific basis for improving soil problems in agriculture, and to provide reference for the resource application of wastewater treatment with microalgae.
Microalgae cultivation improves soil ecological health
Farmland are susceptible to the loss of soil fertility due to large-scale intensive farming, which may lead to soil degradation of cultivatable land. Amongst other soil microbiota, the novel microalgae alone account for about 27% of the total biomass in agricultural land (Abinandan et al., 2019). Moreover, cyanobacteria are more suitable as soil biological indicators for land use and have the potential to improve soil health and fertility (Chamizo et al., 2018) (Table 1). Green algae and cyanobacteria produce abundant organic matter during climate change (Grzesik and Romanowska, 2015), increase soil organic carbon by releasing exopolysaccharides (EPSs) during algal cell decomposition, and become a readily available form of carbon, required for the growth of soil microbiota (Tiwari et al., 2019).
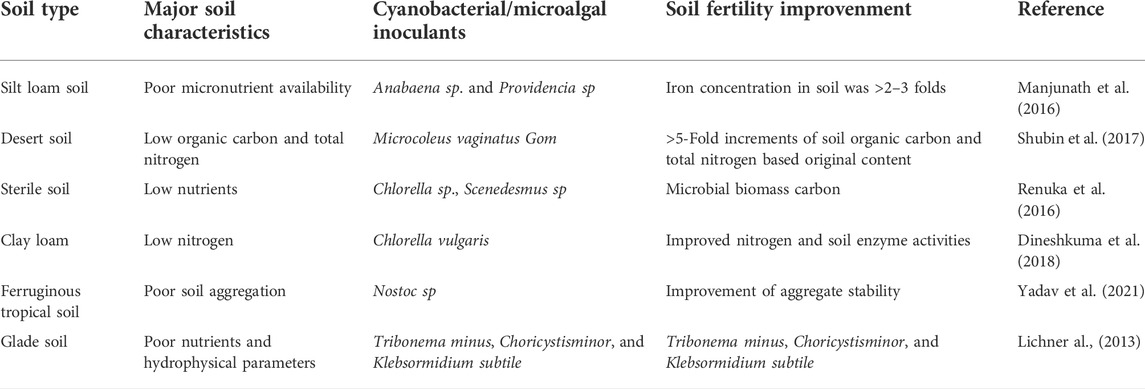
TABLE 1. Improvement of soil health and fertility with different microalgae. Adapted with the permission from Taylor & Francis Online (Abinanda et al., 2019).
Microalgae improve soil physicochemical properties
Soil microorganisms, including bacteria, fungi, viruses and microalgae, that distribute in different soil layers and are invisible to the naked eye. They promote the decomposition of soil organic matter and the transformation of nutrients through oxidation, nitrification, and nitrogen fixation, and have remarkable impacts on soil formation, material circulation, and fertility evolution. In addition to nitrogen and carbon fixation, microalgae can secrete a variety of active substances, such as carotenoids, proteins, fatty acids, plant hormones, extracellular polysaccharides (EPSs), vitamins and antibiotics. Plant hormones are a class of small molecules that act as chemical messengers to coordinate the activities of agricultural crops and higher plant cells (Raza et al., 2019). They play important roles in plant growth and development, while EPSs can provide nutrients for soil microorganisms, increase soil organic carbon and thus affect soil properties (Redmile-Gordon et al., 2020).
On the other hand, microalgae can enhance the stability of soil aggregates with the increment of organic matter, nitrogen, phosphorus and pH (6.5–8.5) in the soil (Redmile-Gordon et al., 2020). Stable soil aggregates are an important factor in maintaining soil fertility, which provide suitable pores for plant growth and nutrients absorption. A rank soil aggregate structure can increase soil oxygen content and water holding capacity. It was reported that organic soil aggregates mainly formed of algal filaments and EPSs after cyanobacteria were inoculated into the soil for 6 weeks (Malam Issa et al., 2007). Yilmaz et al. studied the effect of different microalgal biofertilizer on the stability of soil aggregates, and found that the retention of the available water and plant root growth could be improved by inoculating Chlorella sp. alone or in combination with vermiculite (Yilmaz and Sönmez, 2017). Previous research reported that alkali-hydrolyzed nitrogen, soil organic matter, available phosphorus and potassium, increased considerably after watering cucumber, tomato, pepper and radiant cowpea with different concentrations of microalgae liquid fertilizer, and the enzyme activity in soil such as protease, catalase, etc. also increased in varying degrees (Alobwede et al., 2019; Deepika and Mubarakali, 2020).
Microalgae, including prokaryotes and eukaryotes have a range of unique and valuable properties to cope with challenging agricultural scenarios (Rizwan et al., 2018; Kato and Hasunuma, 2021). Microalgae are beneficial for soil nutrient cycling, as they can improve nutrient utilization and produce biologically active substances (Ekinci et al., 2019; Ronga et al., 2019); while some other microalgae produce biofuels or other high value chemicals, such as EPSs, which improve soil structure and soil quality (Renuka et al., 2018).
Microalgae improve soil microbial community structure
Microalgae as the potential organic carbon have a wide range of agricultural uses (Renuka et al., 2018). The depletion of soil organic carbon leads to a decrease in soil fertility and is an important factor in farmland degradation (Stavi and Lal, 2015). Microalgae convert carbon (including organic carbon) into sugars through photosynthesis, which also affect soil microbial community structure, and thus improve soil aggregation and stability (Marks et al., 2019). Moreover, the multifunctional microalgae, especially for the cyanobacteria, can secrete plant hormones and active substances to further control pathogens and pests (Garcia-Gonzalez and Sommerfeld, 2016; Chanda et al., 2019). The biomass accumulated by the microalgae, can be converted into nutrients that can be used by other plants (Coppens et al., 2016; Alobwede et al., 2019).
The use of active microalgae-derived biofertilizers can improve the microbial ecosystem of the soil, plant growth, nutrient use efficiency, simultaneous tolerance to abiotic stress and reduce the utilize of chemical fertilizers (Ronga et al., 2019; Yoder and Davis, 2020). During the growth of some microalgae species, the contents of intracellular and extracellular polysaccharides increase considerably, which can fix more carbon in the soil; therefore, increase the dissolved organic carbon (DOC) and total carbon (TC) (Jiajun et al., 2019). Whether microalga-derived biostimulants are used alone or mixed with traditional biological fertilizers, they can promote the activity of soil nitrogenase (Cai et al., 2017). The synergetic effects of microalgal biomass on soil and plants would provide a basis for the application of microalgae in crop production (Alvarez et al., 2021) (Figure 1).
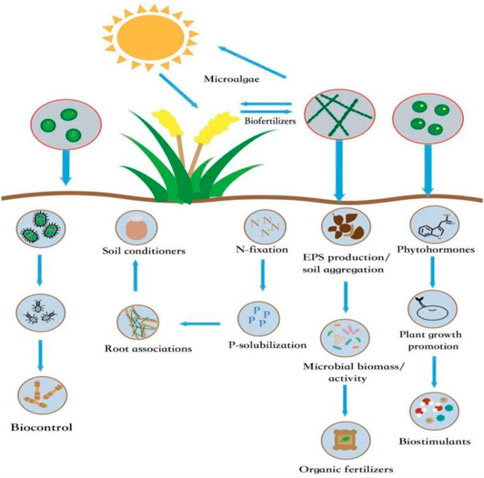
FIGURE 1. The beneficial mechanism of microalgae on soil and plants improvement. Permission from ELSEVIER (Alvarez et al., 2021).
Microalgae control specific pollutants that can cause plant diseases in soil
In agricultural production, biochemical pesticides are selected to control pests through non-toxic mechanisms, but high concentrations of pesticides or fertilizers could poison weeds and cause various growth abnormalities, such as root growth inhibition and carbon assimilation. Degradation of the microtubule system and membrane directly contributes to weed death (Mfarrej and rara, 2019). Therefore, the development of microbial pesticides that use organic matter, such as algae, bacteria or fungi has become necessary. Cyanobacteria are regarded as one of the main biological agents for the management of soil diseases and the control of pathogenic fungi in plants (Stavi and Lal, 2015). They produce bioactive compounds called secondary metabolites (Mager and Thomas, 2011), antifungal and antimicrobial activities are toxic to nematodes (Marks et al., 2019). Various species and genera of microalgae are potential producers of secondary metabolites owing to their biological control and insecticidal properties. Chemical compounds found in microalgae that have potential as biopesticides are listed in Table 2 (Costa et al., 2018).
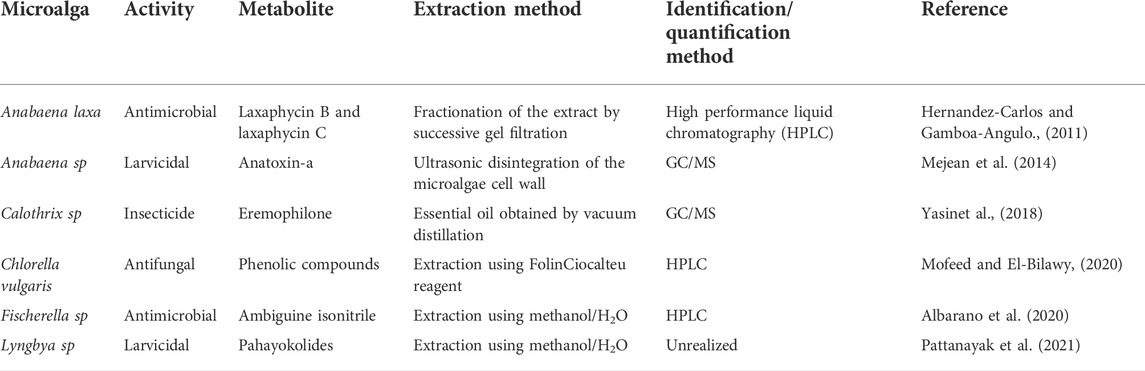
TABLE 2. Chemical compounds found in microalgae have potential as biopesticides. Adapted with the permission from Taylor & Francis Online (Costa et al., 2018).
Heavy metals
Heavy metals, such as chromium (Cr), arsenic (As), cadmium (Cd), mercury (Hg) and lead (Pb), are carcinogenic, and toxic even in trace amounts. They persist in the food chain, leading to bioaccumulation and thus posing a threat to human health and environmental ecology (Leong and Chang, 2020). Using microalgae in the remediation of heavy metals has become an emerging trend owing to their abundant resources, low price, high removal efficiency and eco-friendliness. Compared to higher plants, fast growth, simple and non-toxic process are also the main advantages for microalgae (Leong and Chang., 2020). Table 3 shows the ability of microalgae for the removal of heavy metals from aqueous solutions.
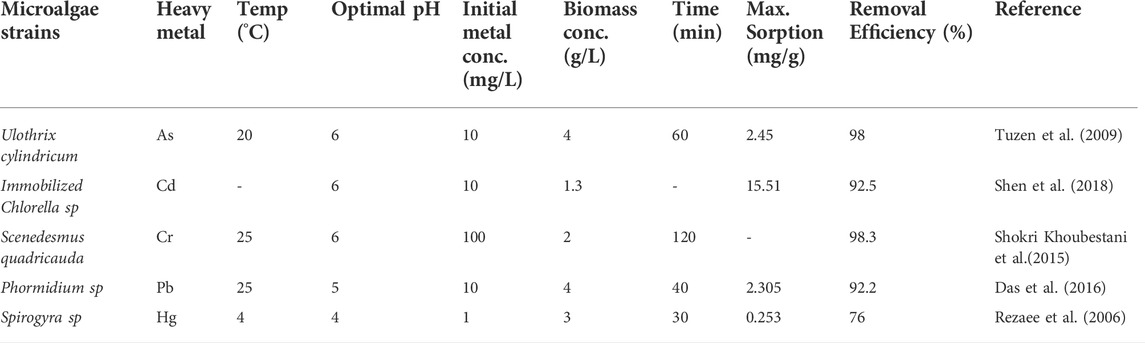
TABLE 3. The ability of microagae for the removal of heavy metals from aqueous solutions (Leong and Chang, 2020).
The adsorption process of microalgae not only releases free radicals against heavy metals, but also synthesizes antioxidants such as glutathione reductase, superoxide dismutase (SOD), peroxidase, ascorbate peroxidase, catalase, etc. (Upadhyay et al., 2016). As endogenous antioxidants, GSH and ASC play key roles in reducing both free radicals and reactive oxygen species (ROS) (Devars et al., 2000). Microalgae secrete high levels of ASC, which acts as a hydroxide-philic reducing buffer and is responsible for protecting cellular components from oxidative threats. ASC exerts a protective effect on microalgae by regulating the activity of ascorbic acid glutathione (ASC-GSH) pathway and metalloenzymes while maintaining the balance of ROS elimination and generation. Furthermore, high levels of GSH can protect microalgal cells by scavenging free radicals, providing tolerance, promoting PCs and ASC synthesis, and restoring substrates for other antioxidants (Gomez-Jacinto et al., 2015).
In detail, heavy metal removal with microalgae through two-stage action: biosorption and bioaccumulation (Figure 2). Biosorption is the fast passive adsorption outside the cell, while bioaccumulation is an active and slow transport process. Microalgal cell wall components include organic proteins, polysaccharides, lipids as well as cellular macromolecules such as peptides and exopolysaccharides, with various functional groups to bind heavy metals (Priatni et al., 2018). Moreover, laminar, carboxyl, monomeric alcohol and deodorizing sulfates of macromolecular clusters from microalgae can also be formed to attract cations and anions of different heavy metals (Pradhan et al., 2019; Leong and Chang., 2020).
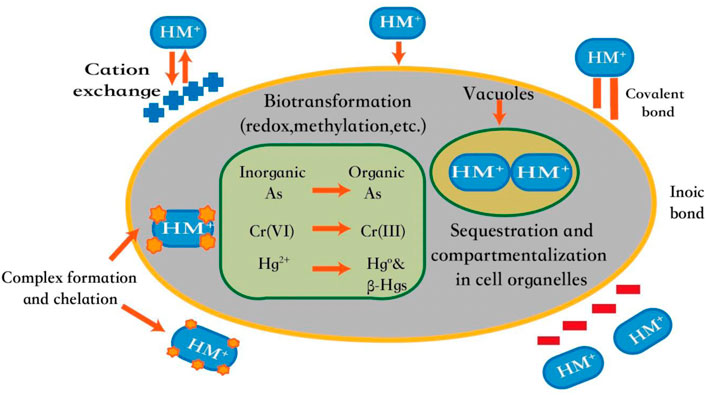
FIGURE 2. Mechanism of Removal of Heavy Metals by Microalgae. Adapted with the permission from ELSEVIER (Leong and Chang, 2020).
Organic pesticides
It was found that the organophosphorus pesticide malathion can inhibit the growth of Aspergillus oryzae and Nostoc muscorum in paddy fields; however, the nitrogen-fixing cyanobacteria could degrade malathion and utilize them as a source of phosphorus (Ibrahim et al., 2014). Amongst them, Candida grisea has the highest biodegradability (91%). Hangjun et al. found that Anabaena PD-1 isolated from rice fields could tolerate polychlorinated biphenyls (PCBs) (Hangjun et al., 2015), and low-chlorinated PCBs are more easily degraded by the algae than their highly chlorinated counterparts. Meta-trichlorodiphenyls, tetrachlorobiphenyls and para-trichlorodiphenyls are more susceptible to dechlorination degradation than their ortho-chlorinated counterparts. In addition, the algae can also degrade dioxin-like PCBs. After 25 days of treatment, the degradation rates of 12 dioxin-like PCB compounds ranged from 37.4% to 68.4%, indicating that the algae can be used for the in-situ bioremediation of PCB-contaminated paddy soil. Tiwari et al. found that Scytonema sp. BHUS-5 could remove and degrade methyl parathion and use the phosphorus after degradation (Tiwari et al., 2017). Abdel-Aty et al. found that Anabaena sphaerica has the ability to remove diurons, and when the initial diuron concentration was 40 mg/L and the pH was 3, adding 1 g/L Anabaena sp. could remove diuron with the rate of 80% in 80 min (Abdel-Aty et al., 2017).
Antibiotics
Antibiotics are the chemical substances produced during the metabolism of microorganisms that can inhibit the growth and activity of other microorganisms at low concentrations (Sherpa et al., 2015). Antibiotics are widely used in aquaculture, soil and crop planting (Chuah et al., 2016). It was reported that antibiotics have shown a powerful effect in inhibiting the reproduction, sterilization and bacteriostasis of aquatic bacteria (Lijian et al., 2020). It is accustomed to add different types of antibiotics to the algal fluid to achieve sterilization or bacteriostasis. Different antibiotic treatments can also be used in different growth stages of microalgae. Haematococcus pluvialis was sterilized with 8 different antibiotics and the antibacterial effect of adding griseofulvin in the early stage was the best, but the antibacterial effect of adding penicillin in the middle and late stage was more obvious (Kiki et al., 2020).
However, the applications of antibiotics are to inhibit the growth of bacteria in the process of microalgal culture, but the use of antibiotics also has an impact on the growth rate, algal cell density, and chlorophyll content (Dantas et al., 2019; Shan et al., 2020). The effect of antibiotics on algae is dual; they may be used as a poison to inhibit the growth of algae, it can also induce toxic irritant effects at specific concentrations; so as to activate protease and induce gene expression, etc., and further to promote the growth of microalgae.
Microalgae remove antibiotics in contaminated soil mainly through biosorption, bioaccumulation and biodegradation, and then achieve the purpose of degradation, thereby restoring soil fertility. Aseptic microalgal culture is very important in its genetic, biochemical, physiological, and taxonomic studies (Ananthi et al., 2018). It shows an effect in sterilization, as antibiotics inhibit the growth and reproduction of bacteria (Xiaoyu et al., 2022). Microalgae are more resistant to antibiotics than bacteria; hence, obtaining sterile algae by selectively using one or more antibiotics for aseptic treatment (Sandhya and Vijayan, 2019).
Microplastics
The problem of microplastic pollution in the soil has attracted global attention. Many reports have been conducted on microplastics, especially on the effect of the water and soil environment. As primary producers, microalgae play an important role in aquatic ecosystems and can maintain the balance of aquatic ecosystems (Nickelsen, 2017; Yokota et al., 2017). Previous studies have found that microplastics can act as substrates for the growth of Raphidocelis subcapitata and promote the growth of the algae (Canniff and Hoang, 2018). Microalgae are sensitive to toxic substances and all changes in microalgae will ultimately affect the structure and function of aquatic ecosystems (Guo et al., 2016; Cai et al., 2017).
At present, plastics are made from chemicals derived from fossil fuels, particularly ethylene and propylene. However, lipids, proteins, and carbohydrates are important raw materials for bioplastic synthesis. Microalgae are rich in these substances; therefore, they become an important source of bioplastic raw materials. The main used in the industrial-scale production of bioplastics contain polylactic acid (PLA), polyhydroxybutyrates (PHBs), polyhydroxyalkanoates (PHAs), starch plastics, protein and cellulosic plastics (Laurens et al., 2017). Calothrix sp., Synechocystis sp., Nostoc sp., Oscillatoria sp., and Spirulina sp. are expected to be used for PHAs production, whereas photoautotrophic Scenedesmus sp. can be used for PLA production (Chaofan et al., 2019). Nutrient-limiting increase PHA and PHB production but without affecting microalgal growth (Kamravamanesh et al., 2018).
Microalgae biotechnology improves soil diseases
Microalgae biotechnology is based on the biology and engineering principles to study algal breeding, algal cell culture, and algal harvest and the treatment of water and nutrients. The established microalgae biotechnology involves carbon sequestration, emission reduction, large-scale cultivation, environmental remediation, bait and feed, functional or high value products (Alam et al., 2020). Moreover, the microalgae biotechnological potential in the maintenance of soil fertility and health are greatly useful and worth expecting.
Saline soil application
To date, about 99.13 million hm2 of saline-alkali land are present in China, especially in Gansu, Ningxia, Xinjiang and other provinces and regions in the northwest region. In detail, the soil affected by salinization in Gansu province is close to 30,000 hm2, especially in Hexi and Yanhuang irrigation areas. The area of soil salinization is increasing annually because of unreasonable irrigation. The annual loss of grains exceeds 100 million kg (Lili and Baoshan, 2021). Cyanobacteria play an important role in the improvement of saline soil. They can improve the contents of nutrients and contribute to the chelation of harmful sodium ions in the soil. The EPSs secreted by cyanobacteria have a tendency to chelate cations. EPSs have negatively charged groups, such as uric acid (Kumar et al., 2018), reduces the availability of Na+ in soil solution by binding to Na+. Nisha et al. isolated several salt- and drought-tolerant cyanobacteria from saline-alkali land and used cyanobacteria as biological fertilizer to improve saline soil, potassium, magnesium cation exchange capacity and soil water-holding energy and also decrease sodium ions and electrical conductivity. Saline-tolerant algae can survive in high-salinity environments and can be considered for application as biological bacterial fertilizers in saline-alkali soils.
In saline soils, the application of fungi Arbuscular mycorrhiza and green algae can increase pH, bacterial biomass carbon, root density, carbon mineralization, soil microbes and soil aggregate formation (Al-Maliki and Ebreesum, 2020). However, Gehringer et al. found that the inoculation of nitrogen-fixing cyanobacteria in high salinity soils can reduce wheat biomass and nutrient content, which may be due to the adsorption of nutrients by algal extracellular polymers, making them unable to be absorbed by plants (Gehringer et al., 2010). This finding indicates that nitrogen-fixing cyanobacteria may not be suitable for wheat in saline-alkali soil but are beneficial to soil fixation. Saline soils are usually low in nitrogen, carbon, and phosphorus; have high electrical conductivity and contain some toxic ions, which greatly limit plant growth (Munns et al., 2020).
Desertification soil application
Nitrogen-fixing cyanobacteria have been used in desertification soil control in the form of biological crusts in Northwest China and have achieved good results. However, the adaptation mechanism of nitrogen-fixing cyanobacteria to such extreme environmental conditions has not been fully elucidated. The researchers measured organic matter, soil texture, nitrogen, phosphorus, temperature, and solar irradiance in Brazil and found that Leptolyngbya sp. has better biocrust under drought conditions (De Lima et al., 2021). Wu et al. found that the shape, species and Colour composition of artificial algal crusts increased with the growth of crusts, and available nitrogen, phosphorus and soil moisturnd also increased (Wu et al., 2022). It shows that the artificial inoculation of biological crusts can improve soil fertility and micro-ecological environment of the topsoil. It was revealed that the tolerance of Lentinula boryana to drought stress could be expressed by the levels of intracellular proline, SOD and carotenoids (Prasanna et al., 2014).
Cyanobacteria act as an important protective role in biological soil crusts (BSCs) in arid and semi-arid regions; however, the cyanobacteria, green algae, and mosses are lesser concern in the wetter agricultural soils of eastern North America. Unlike the traditional BSCs growth pattern in semi-arid areas, the substrate growth in agricultural soils is frequent, short-lived and rapid (Xin and Bruns, 2019). Therefore, cyanobacteria, as renewable C and N resources and have a role in enhancing soil resilience, and have broad prospects in soil applications. Phylogenetic mapping showed that all typical members of the cyanobacterial circle were eutrophic and many of them were nitrogen-fixing bacteria. As assessed with qPCR, nitrogen fixation genes are actually several orders of magnitude more abundant than in bulk biocrust soils. In contrast, competition for CO2, low organic carbon concentrations, and light define a fraction of OTUs separated from cyanobacteria (Couradeau et al., 2019).
Nitrogen-fixing cyanobacteria may also promote desert plant growth and drought resistance. Some cyanobacteria can secrete active compounds which help in promotion of plant growth. Research showed that these active compounds have potential to reduce abiotic stress in crops (Gr et al., 2021). Moreover, cyanobacteria can improve soil quality and alleviate salinity stress and drought resistance with a wide application.
Microalgae wastewater treatment and soil improvement resource utilization
Traditional wastewater treatment has gone from backward and expensive. They simply transfer contaminants from the water, which also cause secondary pollution. Microalgal wastewater treatment is a biological process that removes pollutants while also fixing CO2, and the harvested microalgal biomass can be made into various high valuable products (Jacob et al., 2021). However, advanced methods are required to avoid the adverse effects of different pollutants when use of microalgal biomass based on the wastewater treatment for the improvement of soil (Wu et al., 2022). The characteristic pollutants in wastewater can affect the growth and metabolism of microalgae. Exogenous carbon can effectively increase the growth rate of microalgae and thus improve the removal effect of nitrogen and phosphorus, it also increases the cost of treatment and operation. Swine wastewater is rich in organic matter and afford an effective carbon for the growth of microalgae that can eliminate the cost of carbon supply and simultaneously reduce the organic matter. The researchers designed a comprehensive process for the treatment of digested manure wastewater for advanced treatment and resource recovery (Shubin et al., 2017). It is concluded that the activated carbon adsorption combined with microalgae purification can remove nutrients in wastewater with high efficiency. However, the resource utilization of microalgae after wastewater treatment can improve the economy. Some studies have shown that cyanobacteria polysaccharides in desert soil can play an important role in sand surface stability and soil nutrient retention (Xu et al., 2013). Water retention and structure protection abilities were considerably positively correlated with the content of total carbohydrates and macromolecular carbohydrates in the crust when watering the active microalgae. This indicates that the EPSs from microalgae can play a key role in soil remediation. Microalgae could accumulate 30%–80% of lipids, particularly triacylglycerols. Most algal strains, such as Chlorella, produce fatty acids in the range of C16–C18, whereas the carbon chain of biodiesel is concentrated in C14–C18; therefore, it is suitable for biodiesel production. Carbohydrates are one of the components of microalgal biomass and can be directly used to produce bioethanol. Studies have shown that algae grown in wastewater have the potential to accumulate carbohydrates; therefore, carbohydrate-rich biomass can be cultivated in soil to reduce the cost of microalgae production. In addition, many studies have shown that cultivating microalgae with agricultural wastewater can produce valuable compounds i.e., proteins, carbohydrates, pigments, vitamins, etc., which can be used to further produce cosmetics, food, feed, and/or medicine (Alvarez et al., 2021).
Conclusion
Soil health is an important segment of ecological environment. However, soil pollution deteriorates the soil quality and productivity of cultivated land and thus directly endangers food safety and human health. Photosynthetic microalgae can improve soil physicochemical properties, soil microbial community structure, and recycling with their multiple active chemicals. Likewise, microalgae can control much pollutants of underlying factors for soil deterioration, such as heavy metals, pesticides, antibiotic, and microplastics, with their particular cell structure or functional group. Moreover, utilization of microalgal biomass after wastewater treatment has a sustainable prospect in saline soil and desertification soil application.
Author contributions
XS: Methodology, Data curation, Formal analysis, Investigation, Software, Visualization, Writing—original draft. YB and YF: Data curation, Formal analysis, Investigation, Writing—original draft. YT, CZ, and XY: Conceptualization, Writing—review & editing, Funding acquisition. RR and QX: Conceptualization, Funding acquisition, Project administration, Resources, Supervision, Writing—review & editing. PC: Conceptualization, Methodology, Data curation, Formal analysis, Investigation, Software, Visualization, Writing—original draft, Writing—review & editing, Funding acquisition.
Funding
This research was supported in part by grants from the National Natural Science Foundation of China (32170369), the Natural Science Foundation of Zhejiang Province (LZJWY22B070001), the Yunnan Key Laboratory of Microalgae (202105AG070013), University of Minnesota MnDrive Environment Program (MNE12), and University of Minnesota Center for Biorefining.
Conflict of interest
Authors YT and QX were employed by the company Lijiang Cheng Hai Bao Er Biological Development Co., Ltd.
The remaining authors declare that the research was conducted in the absence of any commercial or financial relationships that could be construed as a potential conflict of interest.
Publisher’s note
All claims expressed in this article are solely those of the authors and do not necessarily represent those of their affiliated organizations, or those of the publisher, the editors and the reviewers. Any product that may be evaluated in this article, or claim that may be made by its manufacturer, is not guaranteed or endorsed by the publisher.
References
Abdel-Aty, A. M., Ammar, N. S., Ghafar, H. H. A., and Ali, R. K. (2013). Biosorption of cadmium and lead from aqueous solution by fresh water alga Anabaena sphaerica biomass. J. Adv. Res. 4 (4), 367–374. doi:10.1016/j.jare.2012.07.004
Abinandan, S., Subashchandrabose, S. R., Venkateswarlu, K., and Megharaj, M. (2019). Soil microalgae and cyanobacteria: The biotechnological potential in the maintenance of soil fertility and health. Crit. Rev. Biotechnol. 39 (8), 981–998. doi:10.1080/07388551.2019.1654972
Al-Maliki, S., and Ebreesum, H. (2020). Changes in soil carbon mineralization, soil microbes, roots density and soil structure following the application of the arbuscular mycorrhizal fungi and green algae in the arid saline soil. Rhizosphere 14, 100203. doi:10.1016/j.rhisph.2020.100203
Alam, M. A., Jingliang, X., and Zhongming, W. (2020). Microalgae biotechnology for food, health and high value products. Singapore: Springer, 81–123.
Albarano, L., Esposito, R., Ruocco, N., and Costantini, M. (2020). Genome mining as new challenge in natural products discovery. Mar. Drugs 18 (4), 199. doi:10.3390/md18040199
Alobwede, E., Leake, J. R., and Pandhal, J. (2019). Circular economy fertilization: Testing micro and macro algal species as soil improvers and nutrient sources for crop production in greenhouse and field conditions. Geoderma 334, 113–123. doi:10.1016/j.geoderma.2018.07.049
Alvarez, A. L., Weyers, S. L., Goemann, H. M., Peyton, B. M., and Gardner, R. D. (2021). Microalgae, soil and plants: A critical review of microalgae as renewable resources for agriculture. Algal Res. 54, 102200. doi:10.1016/j.algal.2021.102200
Ananthi, V., Prakash, G. S., Rasu, K. M., Gangadevi, K., Boobalan, T., Raja, R., et al. (2018). Comparison of integrated sustainable biodiesel and antibacterial nano silver production by microalgal and yeast isolates. J. Photochem. Photobiol. B Biol. 186, 232–242. doi:10.1016/j.jphotobiol.2018.07.021
Cai, Z., Xiaohua, C., Jiangtao, W., and Liju, T. (2017). Toxic effects of microplastic on marine microalgae skeletonema costatum: Interactions between microplastic and algae. Environ. Pollut. 220, 1282–1288. doi:10.1016/j.envpol.2016.11.005
Canniff, P. M., and Hoang, T. C. (2018). Microplastic ingestion by Daphnia magna and its enhancement on algal growth. Sci. Total Environ. 633, 500–507. doi:10.1016/j.scitotenv.2018.03.176
Chamizo, S., Mugnai, G., Rossi, F., Certini, G., and De Philippis, R. (2018). Cyanobacteria inoculation improves soil stability and fertility on different textured soils: Gaining insights for applicability in soil restoration. Front. Environ. Sci. 6, 49. doi:10.3389/fenvs.2018.00049
Chanda, M. J., Merghoub, N., and El Arroussi, H. (2019). Microalgae polysaccharides: The new sustainable bioactive products for the development of plant bio-stimulants? World J. Microbiol. Biotechnol. 35, 177. doi:10.1007/s11274-019-2745-3
Chaofan, Z., Show, P. L., and Ho, S. H. (2019). Progress and perspective on algal plastics–a critical review. Bioresour. Technol. 289, 121700. doi:10.1016/j.biortech.2019.121700
Chuah, L. O., Effarizah, M. E., Goni, A. M., and Rusul, G. (2016). Antibiotic application and emergence of multiple antibiotic resistance (MAR) in global catfish aquaculture. Curr. Environ. Health Rep. 3 (2), 118–127. doi:10.1007/s40572-016-0091-2
Coppens, J., Grunert, O., Van Den Hende, S., Vanhoutte, I., Boon, N., Haesaert, G., et al. (2016). The use of microalgae as a high-value organic slow-release fertilizer results in tomatoes with increased carotenoid and sugar levels. J. Appl. Phycol. 28 (4), 2367–2377. doi:10.1007/s10811-015-0775-2
Costa, O. Y., Raaijmakers, J. M., and Kuramae, E. E. (2018). Microbial extracellular polymeric substances: Ecological function and impact on soil aggregation. Front. Microbiol. 9, 1636. doi:10.3389/fmicb.2018.01636
Couradeau, E., Giraldo-Silva, A., De Martini, F., and Garcia-Pichel, F. (2019). Spatial segregation of the biological soil crust microbiome around its foundational cyanobacterium, Microcoleus vaginatus, and the formation of a nitrogen-fixing cyanosphere. Microbiome 7 (1), 55–12. doi:10.1186/s40168-019-0661-2
Dahiya, A. (2015). Algae biomass cultivation for advanced biofuel production. Bioenergy, 219–238. doi:10.1016/B978-0-12-407909-0.00014-6
Dantas, D. M. D. M., Oliveira, C. Y. B. D., Costa, R. M. P. B., Carneiro-da-Cunha, M. D. G., Gálvez, A. O., and Bezerra, R. D. S. (2019). Evaluation of antioxidant and antibacterial capacity of green microalgae Scenedesmus subspicatus. Food Sci. Technol. Int. 25 (4), 318–326. doi:10.1177/1082013218825024
Das, D., Chakraborty, S., Bhattacharjee, C., and Chowdhury, R. (2016). Biosorption of lead ions (Pb2+) from simulated wastewater using residual biomass of microalgae. Desalin. Water Treat. 57 (10), 4576–4586. doi:10.1080/19443994.2014.994105
Das, P., Quadir, M. A., Thaher, M. I., Alghasal, G. S. H. S., and Aljabri, H. M. S. J. (2019). Microalgal nutrients recycling from the primary effluent of municipal wastewater and use of the produced biomass as bio-fertilizer. Int. J. Environ. Sci. Technol. (Tehran). 16 (7), 3355–3364. doi:10.1007/s13762-018-1867-8
De Lima, N. M. M., Munoz-Rojas, M., Vazquez-Campos, X., and Branco, L. H. Z. (2021). Biocrust cyanobacterial composition, diversity, and environmental drivers in two contrasting climatic regions in Brazil. Geoderma 386, 114914. doi:10.1016/j.geoderma.2020.114914
Deepika, P., and MubarakAli, D. (2020). Production and assessment of microalgal liquid fertilizer for the enhanced growth of four crop plants. Biocatal. Agric. Biotechnol. 28, 101701. doi:10.1016/j.bcab.2020.101701
Devars, S., Aviles, C., Cervantes, C., and Moreno-Sanchez, R. (2000). Mercury uptake and removal by Euglena gracilis. Arch. Microbiol. 174 (3), 175–180. doi:10.1007/s002030000193
Dineshkumar, R., Kumaravel, R., Gopalsamy, J., Sikder, M. N. A., and Sampathkumar, P. (2018). Microalgae as bio-fertilizers for rice growth and seed yield productivity. Waste Biomass Valorization 9 (5), 793–800. doi:10.1007/s12649-017-9873-5
Ekinci, K., Erdal, I., Uysal, O., Uysal, F. O., Tunce, H., and Doğan, A. (2019). Anaerobic digestion of three microalgae biomasses and assessment of digestates as biofertilizer for plant growth. Environ. Prog. Sustain. Energy 38 (3), e13024. doi:10.1002/ep.13024
Garcia-Gonzalez, J., and Sommerfeld, M. (2016). Biofertilizer and biostimulant properties of the microalga Acutodesmus dimorphus. J. Appl. Phycol. 28 (2), 1051–1061. doi:10.1007/s10811-015-0625-2
Gehringer, M. M., Pengelly, J. J., Cuddy, W. S., Fieker, C., Forster, P. I., and Neilan, B. A. (2010). Host selection of symbiotic cyanobacteria in 31 species of the Australian cycad genus: Macrozamia (Zamiaceae). Mol. Plant. Microbe. Interact. 23 (6), 811–822. doi:10.1094/MPMI-23-6-0811
Gomez-Jacinto, V., Garcia-Barrera, T., Gomez-Ariza, J. L., Garbayo-Nores, I., and Vílchez-Lobato, C. (2015). Elucidation of the defence mechanism in microalgae Chlorella sorokiniana under mercury exposure. Identification of Hg-phytochelatins. Chem. Biol. Interact. 238, 82–90. doi:10.1016/j.cbi.2015.06.013
Gr, S., Yadav, R. K., Chatrath, A., Gerard, M., Tripathi, K., Govindsamy, V., et al. (2021). Perspectives on the potential application of cyanobacteria in the alleviation of drought and salinity stress in crop plants. J. Appl. Phycol. 33 (6), 3761–3778. doi:10.1007/s10811-021-02570-5
Grzesik, M., and Romanowska-Duda, Z. (2015). Ability of cyanobacteria and green algae to improve metabolic activity and development of willow plants. Pol. J. Environ. Stud. 24 (3), 1003–1012. doi:10.15244/pjoes/34667
Guo, J., Selby, K., and Boxall, A. B. (2016). Comparing the sensitivity of chlorophytes, cyanobacteria, and diatoms to major-use antibiotics. Environ. Toxicol. Chem. 35 (10), 2587–2596. doi:10.1002/etc.3430
Hangjun, Z., Xiaojun, J., Liping, L., and Wenfeng, W. (2015). Biodegradation of polychlorinated biphenyls (PCBs) by the novel identified cyanobacterium Anabaena PD-1. PLoS ONE 10 (7), e0131450. doi:10.1371/journal.pone.0131450
Hernandez-Carlos, B., and Gamboa-Angulo, M. M. (2011). Metabolites from freshwater aquatic microalgae and fungi as potential natural pesticides. Phytochem. Rev. 10, 261–286. doi:10.1007/s11101-010-9192-y
Ibrahim, W. M., Karam, M. A., El-Shahat, R. M., and Adway, A. A. (2014). Biodegradation and utilization of organophosphorus pesticide malathion by cyanobacteria. Biomed. Res. Int. 1-6, 392682. doi:10.1155/2014/392682
Jacob, A., Ashok, B., Alagumalai, A., Chyuan, O. H., and Le, P. T. K. (2021). Critical review on third generation micro algae biodiesel production and its feasibility as future bioenergy for IC engine applications. Energy Convers. Manag. 228, 113655. doi:10.1016/j.enconman.2020.113655
Jiajun, H., Hongcheng, G., Yiyun, X., Min-tian, G., Shiping, Z., Tsang, Y. F., et al. (2019). Using a mixture of microalgae, biochar, and organic manure to increase the capacity of soil to act as carbon sink. J. Soils Sediments 19 (11), 3718–3727. doi:10.1007/s11368-019-02337-z
Jun, C., Yan, W., Benemann, J. R., Xuecheng, Z., Hongjun, H., and Song, Q. (2016). Microalgal industry in China: Challenges and prospects. J. Appl. Phycol. 28 (2), 715–725. doi:10.1007/s10811-015-0720-4
Jun, W., Jian, L., Leiming, L., Xiuyun, M., and amd Yongming, L. (2018). Pollution, ecological-health risks, and sources of heavy metals in soil of the northeastern Qinghai-Tibet Plateau. Chemosphere 201, 234–242. doi:10.1016/j.chemosphere.2018.02.122
Kamravamanesh, D., Kovacs, T., Pflügl, S., Druzhinina, I., Kroll, P., Lackner, M., et al. (2018). Increased poly-β-hydroxybutyrate production from carbon dioxide in randomly mutated cells of cyanobacterial strain Synechocystis sp. PCC 6714: Mutant generation and characterization. Bioresour. Technol. 266, 34–44. doi:10.1016/j.biortech.2018.06.057
Kato, Y., and Hasunuma, T. (2021). Metabolic engineering for carotenoid production using eukaryotic microalgae and prokaryotic cyanobacteria. Adv. Exp. Med. Biol. 1261, 121–135. doi:10.1007/978-981-15-7360-6_10
Kiki, C., Rashid, A., Wang, Y., Li, Y., Zeng, Q., Yu, C. P., et al. (2020). Dissipation of antibiotics by microalgae: Kinetics, identification of transformation products and pathways. J. Hazard. Mat. 387, 121985. doi:10.1016/j.jhazmat.2019.121985
Kumar, D., Kastanek, P., and Adhikary, S. P. (2018). Exopolysaccharides from cyanobacteria and microalgae and their commercial application. Curr. Sci. 115 (2), 234–241. doi:10.18520/cs/v115/i2/234-241
Laurens, L. M., Markham, J., Templeton, D. W., Christensen, E. D., Van Wychen, S., Vadelius, E. W., et al. (2017). Development of algae biorefinery concepts for biofuels and bioproducts; a perspective on process-compatible products and their impact on cost-reduction. Energy Environ. Sci. 10 (8), 1716–1738. doi:10.1039/C7EE01306J
Leong, Y. K., and Chang, J. S. (2020). Bioremediation of heavy metals using microalgae: Recent advances and mechanisms. Bioresour. Technol. 303, 122886. doi:10.1016/j.biortech.2020.122886
Lichner, L., Hallett, P. D., Drongova, Z., Czachor, H., Kovacik, L., Mataix-Solera, J., et al. (2013). Algae influence the hydrophysical parameters of a sandy soil. Catena 108, 58–68. doi:10.1016/j.catena.2012.02.016
Lijian, L., Liang, W., Qin, X., Siyu, X., Wenting, L., Sen, L., et al. (2020). Use of microalgae based technology for the removal of antibiotics from wastewater: A review. Chemosphere 238, 124680. doi:10.1016/j.chemosphere.2019.124680
Lili, L., and Baoshan, W. (2021). Protection of halophytes and their uses for cultivation of saline-alkali soil in China. Biology 10 (5), 353. doi:10.3390/biology10050353
Little, S. M., Senhorinho, G. N., Saleh, M., Basiliko, N., and Scott, J. A. (2021). Antibacterial compounds in green microalgae from extreme environments: A review. Algae 36 (1), 61–72. doi:10.4490/ALGAE.2021.36.3.6
Mager, D. M., and Thomas, A. D. (2011). Extracellular polysaccharides from cyanobacterial soil crusts: A review of their role in dryland soil processes. J. Arid. Environ. 75 (2), 91–97. doi:10.1016/j.jaridenv.2010.10.001
Malam Issa, O., Défarge, C., Le Bissonnais, Y., Marin, B., Duval, O., Bruand, A., et al. (2007). Effects of the inoculation of cyanobacteria on the microstructure and the structural stability of a tropical soil. Plant Soil 290 (1), 209–219. doi:10.1007/s11104-006-9153-9
Manjunath, M., Kanchan, A., Ranjan, K., Venkatachalam, S., Prasanna, R., Ramakrishnan, B., et al. (2016). Beneficial cyanobacteria and eubacteria synergistically enhance bioavailability of soil nutrients and yield of okra. Heliyon 2 (2), e00066. doi:10.1016/j.heliyon.2016.e00066
Marks, E. A., Montero, O., and Rad, C. (2019). The biostimulating effects of viable microalgal cells applied to a calcareous soil: Increases in bacterial biomass, phosphorus scavenging, and precipitation of carbonates. Sci. Total Environ. 692, 784–790. doi:10.1016/j.scitotenv.2019.07.289
Mejean, A., Paci, G., Gautier, V., and Ploux, O. (2014). Biosynthesis of anatoxin-A and analogues (anatoxins) in cyanobacteria. Toxicon 91, 15–22. doi:10.1016/j.toxicon.2014.07.016
Mfarrej, M. F. B., and Rara, F. M. (2019). Competitive, sustainable natural pesticides. Acta Ecol. Sin. 39 (2), 145–151. doi:10.1016/j.chnaes.2018.08.005
Mofeed, J., and El-Bilawy, E. H. (2020). Toxicity and disruptive impacts of fenhexamid fungicide against the green alga, Chlorella vulgaris. Egypt. Acad. J. Biol. Sci. F. Toxicol. Pest Control 12 (1), 45–57. doi:10.21608/EAJBSF.2020.78133
Munns, R., Passioura, J. B., Colmer, T. D., and Byrt, C. S. (2020). Osmotic adjustment and energy limitations to plant growth in saline soil. New Phytol. 225 (3), 1091–1096. doi:10.1111/nph.15862
Nematollahi, M. J., Keshavarzi, B., Zaremoaiedi, F., Rajabzadeh, M. A., and Moore, F. (2020). Ecological-health risk assessment and bioavailability of potentially toxic elements (PTEs) in soil and plant around a copper smelter. Environ. Monit. Assess. 192 (10), 639–719. doi:10.1007/s10661-020-08589-4
Nickelsen, K. (2017). The organism strikes back: Chlorella algae and their impact on photosynthesis research, 1920s–1960s. Hist. Philos. Life Sci. 39 (2), 9–22. doi:10.1007/s40656-017-0137-2
Pattanayak, S., Das, S., and Biswal, G. (2021). Crop disease control through application of algal biopesticides: Alternate route map in organic farming. Biopestic. Org. Farming, 123–126. doi:10.1201/9781003027690-30
Perez-Lucas, G., Vela, N., El Aatik, A., and Navarro, S. (2019). Environmental risk of groundwater pollution by pesticide leaching through the soil profile. Pesticides. In ed L. Marcelo, and S. Sonia, Anthropogenic. Activities and the health of our the environment. 1–28. doi:10.5772/intechopen.82418
Pradhan, D., Sukla, L. B., Mishra, B. B., and Devi, N. (2019). Biosorption for removal of hexavalent chromium using microalgae Scenedesmus sp. J. Clean. Prod. 209, 617–629. doi:10.1016/j.jclepro.2018.10.288
Prasanna, R., Sood, A., Ratha, S. K., and Singh, P. K. (2014). Cyanobacteria as a green option for sustainable agriculture. Cyanobacteria 9, 145–166. doi:10.1002/9781118402238.ch9
Priatni, S., Ratnaningrum, D., Warya, S., and Audina, E. (2018). Phycobiliproteins production and heavy metals reduction ability of Porphyridium sp. IOP Conf. Ser. Earth Environ. Sci. 160, 012006. doi:10.1088/1755-1315/160/1/012006
Randrianarison, G., and Ashraf, M. A. (2017). Microalgae: A potential plant for energy production. Geol. Ecol. Landscapes 1 (2), 104–120. doi:10.1080/24749508.2017.1332853
Raza, A., Mehmood, S. S., Tabassum, J., and Batool, R. (2019). “Targeting plant hormones to develop abiotic stress resistance in wheat. Wheat. Production,” in Changing. Environments. (Singapore: Springer), 557–577.
Redmile-Gordon, M., Gregory, A. S., White, R. P., and Watts, C. W. (2020). Soil organic carbon, extracellular polymeric substances (EPS), and soil structural stability as affected by previous and current land-use. Geoderma 363, 114143. doi:10.1016/j.geoderma.2019.114143
Renuka, N., Guldhe, A., Prasanna, R., Singh, P., and Bux, F. (2018). Microalgae as multi-functional options in modern agriculture: Current trends, prospects and challenges. Biotechnol. Adv. 36 (4), 1255–1273. doi:10.1016/j.biotechadv.2018.04.004
Rezaee, A., Ramavandi, B., Ganati, F., Ansari, M., and Solimanian, A. (2006). Biosorption of mercury by biomass of filamentous algae Spirogyra species. J. Biol. Sci. 6 (4), 695–700. doi:10.3923/jbs.2006.695.700
Rizwan, M., Mujtaba, G., Memon, S. A., Lee, K., and Rashid, N. (2018). Exploring the potential of microalgae for new biotechnology applications and beyond: A review. Renew. Sustain. Energy Rev. 92, 394–404. doi:10.1016/j.rser.2018.04.034
Ronga, D., Biazzi, E., Parati, K., Carminati, D., Carminati, E., and Tava, A. (2019). Microalgal biostimulants and biofertilisers in crop productions. Agronomy 9 (4), 192. doi:10.3390/agronomy9040192
Sandhya, S. V., and Vijayan, K. K. (2019). Symbiotic association among marine microalgae and bacterial flora: A study with special reference to commercially important isochrysis galbana culture. J. Appl. Phycol. 31 (4), 2259–2266. doi:10.1007/s10811-019-01772-2
Sasaki, M., Takagi, A., Ota, S., Kawano, S., Sasaki, D., and Asayama, M. (2020). Coproduction of lipids and extracellular polysaccharides from the novel green alga Parachlorella sp. BX1. 5 depending on cultivation conditions. Biotechnol. Rep. 25, e00392. doi:10.1016/j.btre.2019.e00392
Shan, C., Jiayuan, L., Wenbo, F., Mingzhe, Y., Zhang, W., Houtao, X., et al. (2020). Biochemical responses of the freshwater microalga Dictyosphaerium sp. upon exposure to three sulfonamides. J. Environ. Sci. 97, 141–148. doi:10.1016/j.jes.2020.05.018
Shen, Y., Zhu, W., Li, H., Ho, S. H., Chen, J., Xie, Y., et al. (2018). Enhancing cadmium bioremediation by a complex of water-hyacinth derived pellets immobilized with Chlorella sp. Bioresour. Technol. 257, 157–163. doi:10.1016/j.biortech.2018.02.060
Sherpa, R. T., Reese, C. J., and Aliabadi, H. M. (2015). Application of iChip to grow “uncultivable” microorganisms and its impact on antibiotic discovery. J. Pharm. Pharm. Sci. 18 (3), 303–315. doi:10.18433/j30894
Shokri Khoubestani, R., Mirghaffari, N., and Farhadian, O. (2015). Removal of three and hexavalent chromium from aqueous solutions using a microalgae biomass-derived biosorbent. Environ. Prog. Sustain. Energy 34 (4), 949–956. doi:10.1002/ep.12071
Shubin, L., Li, W., Haijian, Y., Delu, Z., and Chunxiang, H. (2017). A new biofilm based microalgal cultivation approach on shifting sand surface for desert cyanobacterium Microcoleus vaginatus. Bioresour. Technol. 238, 602–608. doi:10.1016/j.biortech.2017.04.058
Silva, G. H., Sueitt, A. P. E., Haimes, S., Tripidaki, A., van Zwieten, R., and Fernandes, T. V. (2019). Feasibility of closing nutrient cycles from black water by microalgae-based technology. Algal Res. 44, 101715. doi:10.1016/j.algal.2019.101715
Stavi, I., and Lal, R. (2015). Achieving zero net land degradation: Challenges and opportunities. J. Arid. Environ. 112, 44–51. doi:10.1016/j.jaridenv.2014.01.016
Tang, D. Y. Y., Khoo, K. S., Chew, K. W., Tao, Y., Ho, S. H., and Show, P. L. (2020). Potential utilization of bioproducts from microalgae for the quality enhancement of natural products. Bioresour. Technol. 304, 122997. doi:10.1016/j.biortech.2020.122997
Tiwari, B., Singh, S., Chakraborty, S., Verma, E., and Mishra, A. K. (2017). Sequential role of biosorption and biodegradation in rapid removal degradation and utilization of methyl parathion as a phosphate source by a new cyanobacterial isolate Scytonema sp. BHUS-5. Int. J. Phytoremediation 19 (10), 884–893. doi:10.1080/15226514.2017.1303807
Tiwari, O. N., Bhunia, B., Mondal, A., Gopikrishna, K., and Indrama, T. (2019). System metabolic engineering of exopolysaccharide-producing cyanobacteria in soil rehabilitation by inducing the formation of biological soil crusts: A review. J. Clean. Prod. 211, 70–82. doi:10.1016/j.jclepro.2018.11.188
Tuzen, M., Sari, A., Mendil, D., Uluozlu, O. D., Soylak, M., and Dogan, M. (2009). Characterization of biosorption process of As(III) on green algae Ulothrix cylindricum. J. Hazard. Mat. 165 (1), 566–572. doi:10.1016/j.jhazmat.2008.10.020
Upadhyay, A. K., Mandotra, S. K., Kumar, N., Singh, N. K., Singh, L., and Rai, U. N. (2016). Augmentation of arsenic enhances lipid yield and defense responses in alga Nannochloropsis sp. Bioresour. Technol. 221, 430–437. doi:10.1016/j.biortech.2016.09.061
Wollmann, F., Dietze, S., Ackermann, J. U., Bley, T., Walther, T., Steingroewer, J., et al. (2019). Microalgae wastewater treatment: Biological and technological approaches. Eng. Life Sci. 19 (12), 860–871. doi:10.1002/elsc.201900071
Wu, W., Ke, T., Zhou, X., Li, Q., Tao, Y., Zhang, Y., et al. (2022). Synergistic remediation of copper mine tailing sand by microalgae and fungi. Appl. Soil Ecol. 175, 104453. doi:10.1016/j.apsoil.2022.104453
Wuang, S. C., Khin, M. C., Chua, P. Q. D., and Luo, Y. D. (2016). Use of Spirulina biomass produced from treatment of aquaculture wastewater as agricultural fertilizers. Algal Res. 15, 59–64. doi:10.1016/j.algal.2016.02.009
Xiaoyu, W., Meiyin, W., Lei, Z., Yongdong, D., Yang, H., Chenda, Y., et al. (2022). ALA_PDT promotes ferroptosis-like death of Mycobacterium abscessus and antibiotic sterilization via oxidative stress. Antioxidants 11 (3), 546. doi:10.3390/antiox11030546
Xin, P., and Bruns, M. A. (2019). Development of a nitrogen-fixing cyanobacterial consortium for surface stabilization of agricultural soils. J. Appl. Phycol. 31 (2), 1047–1056. doi:10.1007/s10811-018-1597-9
Xu, Y., Rossi, F., Colica, G., Deng, S., De Philippis, R., and Chen, L. (2013). Use of cyanobacterial polysaccharides to promote shrub performances in desert soils: A potential approach for the restoration of desertified areas. Biol. Fertil. Soils 49 (2), 143–152. doi:10.1007/s00374-012-0707-0
Yadav, P., Gupta, R. K., Singh, R. P., Yadav, P. K., Patel, A. K., and Pandey, K. D. (2021). “Role of cyanobacteria in green remediation,” in Sustainable environmental clean-up (Amsterdam, Netherlands: Elsevier), 187–210. doi:10.1016/B978-0-12-823828-8.00009-8
Yasin, D., Fatma, T., Zafaryab, M., Ahmad, N., Aziz, N., and Rizvi, M. M. A. (2018). Exploring the bio-efficacies of methanolic extracts of Nostoc muscorum and Calothrix brevissima with their Characterization Using GC-MS. Nat. Prod. J. 8 (4), 305–316. doi:10.2174/2210315508666180807095636
Yilmaz, E., and Sönmez, M. (2017). The role of organic/bio–fertilizer amendment on aggregate stability and organic carbon content in different aggregate scales. Soil Tillage Res. 168, 118–124. doi:10.1016/j.still.2017.01.003
Yoder, N., and Davis, J. G. (2020). Organic fertilizer comparison on growth and nutrient content of three kale cultivars. HortTechnology 30 (2), 176–184. doi:10.21273/HORTTECH04483-19
Keywords: microalgae, soil fertility, characteristic pollutants, crop diseases, ecological environment protection
Citation: Song X, Bo Y, Feng Y, Tan Y, Zhou C, Yan X, Ruan R, Xu Q and Cheng P (2022) Potential applications for multifunctional microalgae in soil improvement. Front. Environ. Sci. 10:1035332. doi: 10.3389/fenvs.2022.1035332
Received: 02 September 2022; Accepted: 27 September 2022;
Published: 12 October 2022.
Edited by:
Xiaokai Zhang, Jiangnan University, ChinaReviewed by:
Ahmed Abdelhafez, The New Valley University, EgyptJianhua Fan, East China University of Science and Technology, China
Copyright © 2022 Song, Bo, Feng, Tan, Zhou, Yan, Ruan, Xu and Cheng. This is an open-access article distributed under the terms of the Creative Commons Attribution License (CC BY). The use, distribution or reproduction in other forums is permitted, provided the original author(s) and the copyright owner(s) are credited and that the original publication in this journal is cited, in accordance with accepted academic practice. No use, distribution or reproduction is permitted which does not comply with these terms.
*Correspondence: Qingshan Xu, bGpjaGJlckAxNjMuY29t; Pengfei Cheng, Y2hlbmdwZW5nZmVpQG5idS5lZHUuY24=