- 1Research Unit of Sustainable Chemistry, Faculty of Technology, Oulu University, Oulu, Finland
- 2School of Engineering, Kajaani University of Applied Sciences, Kajaani, Finland
In this study, a technical scheme of an ammonium recovery process from diluted municipal or industrial wastewaters was developed, and the main operational parameters of adsorption/desorption and air-stripping/acid-scrubbing or membrane units were examined. The proposed approach combines the removal of ammonium nitrogen by an ion-exchange mechanism on metakaolin-based geopolymers (MKGPs) followed by adsorbent regeneration. A regeneration agent was purified by the air-stripping technique or membrane technology. A ready-to-use market-grade fertilizer or industrial-grade ammonia water could be obtained as the final product. The properties and regeneration ability of MKGP, prepared from activated kaolinite clay, were compared with new geopolymer adsorbents based on papermill sludge (FS MKGP). Adsorption fixed-bed column experiments with continuously circulated regeneration solution purified by air-stripping or the membrane approach were conducted to determine the limits of the regeneration solution’s application. Sodium and potassium salts were tested as regeneration agents, and the influence of regeneration solution composition on ammonium removal and recovery rates was investigated. Based on a breakthrough curve analysis, the removal rate of ammonium N by FS MKGP was found to be 3.2 times higher than that by MKGP for actual wastewater samples. Moreover, there were substantial differences in the regeneration regime between the two adsorbents. For the air-stripping technique, a liquid-phase temperature of 45°C was minimal and enough for efficient ammonia transfer to the gaseous phase. For the membrane technique, a feed-phase temperature of 40°C was enough for removing ammonia from the regeneration solution, while no heating of a receiving phase was required.
1 Introduction
Nowadays, the main process of ammonia production is the Haber–Bosch scheme. The process relies on fossil fuels, predominantly natural gas, which is used to produce hydrogen via the steam reforming of methane. While steam reforming consumes more than 80% of the required energy, ammonia synthesis is also an energy-intensive process because high temperatures (up to 500°C) and pressures (up to 300 bar) are required to conduct a catalytic reaction. In the context of the ongoing energy crisis and challenges to the accessibility of fossil fuels, ammonia is emerging as a strategic commodity.
Roughly 2% of the world’s total energy is consumed in ammonia production, and the process substantially contributes to the world’s CO2 emissions (International Energy Agency, 2007; Liu et al., 2020; Ammonia: zero-carbon fertiliser, fuel, and energy store, 2020). Approximately one-fifth part of all the produced ammonia is used for industrial purposes (manufacturing of plastics and organic compounds, fibers, explosives, etc.), while the larger part is converted to solid or liquid fertilizers.
During the last decade, several technologies have been developed for nutrient recovery from municipal and industrial wastewaters. Owing to chemical and physical features and finite raw sources, it is more attractive to develop P-recovery technologies. Thus, the technology readiness level (TRL) for P-recovery approaches is higher than that of nitrogen recovery and reuse techniques. However, still much attention has been paid to develop new approaches and options for cost-effective phosphorous removal and recovery, and such endeavors have been supported at the international level recently, e.g., the Phos4YOU project (Phos4YOU, 2020) (PHOSphorus Recovery from waste water FOR YOUr Life) and European Sustainable Phosphorus Platform (ESPP), 2020. Piloting or full-scale application levels (with TRL no less than 7–8) have mostly reached technologies that treated reject waters after sludge anaerobic digestion, separated urine schemes, or P-recovery schemes from sludge ashes. In contrast, captured N is renewable in nature; however, the Haber–Bosch process is highly energy extensive and has a substantial carbon footprint. Thus, we believe that approaches to recover and reuse captured N will attract increasing interest in the near future.
Widely, only the removal (not the recovery) of ammonium N by deaerating, aerobic treatment, or ANAMMOX process is applied at municipal and industrial sites. Several review articles discussing ammonium removal and recovery technologies at the pilot-scale level (at TRL 6 or 7) have appeared in the literature (Mehta et al., 2015; Sengupta et al., 2015; Monfet et al., 2018; Ye et al., 2018). Dong et al. studied an N recovery pilot-scale system composed of a stripper, an absorber, and a crystallizer. This scheme treats anaerobic digestate to recover N as the ammonium phosphate form, a valuable fertilizer. The recovery efficiency was calculated to be 72% of the input N mass (Dong et al., 2020). Costamagna et. al. studied a pilot-scale system coupling thin film evaporator technology for anaerobic digestion. The recovery was reported to be 4.1 g N–NH4 per kg of sludge (19.3 g N–NH4 per kg of total solids), recovered in the form of ammonium sulfate (Costamagna et al., 2020). The membrane technology is another alternative to the air-stripping approach for ammonia recovery. Direct application of membrane technology for complex matrix, however, has some limitations, such as high-energy consumption, sensitivity of the membrane contactor to the content of suspended solids, or scaling up problems. As merits in comparison to other physical water treatment processes such as air-stripping or evaporation/distillation approaches, extremely high selectivity toward ammonium is the main advantage. Ulbricht et al. (2013) reported ammonium recovery from wastewaters with a high ammonium content (>400 mg/L). The consumption of chemicals was indicated as the largest expense in this process (Hasanoğlu et al., 2010) because the dissolved ammonium ions must be converted to free ammonia gas by the pH adjustment of the feed phase to remove ammonia from a wastewater stream. Thus, direct application to low-laden streams containing ammonium N is not economically feasible due to the operating cost.
Other valuable fertilizers belong to the class of struvite (M2+NH4(K)PO4∙6H2O, where M2+ is a bivalent metal). Struvite, mostly magnesium and ammonium phosphate, can be obtained by the precipitation of ammonium and phosphate under alkaline conditions with the addition of various magnesium salts (Nelson et al., 2003). It is a valuable option because it can be used as a fertilizer without further treatment (Rahman et al., 2014). Wei et al. demonstrated a pilot-scale solution for the recovery of N and P from separated human urine in struvite form. The recovery efficiency for ammonium was 85% (Wei et al., 2018). It is worth mentioning that recovering nutrients via struvite precipitation was mostly considered a primary technology for P recovery, while N present in excess requires additional treatment procedures to reach safe discharge limits.
All these reported approaches involve wastewaters with high nutrient contents (1–6 g N/L), while the concentration of ammonium N in dilute solutions often does not exceed 0.1 g/L. That makes the application of well-known physical methods not feasible from an economic point of view because of substantial operational costs. Liberti et al. (1981) studied a pilot-scale and a full-scale plant that aimed to recover N and P from diluted wastewaters using ion-exchange (IE) technology. After the removal of elements using IE resins, they were collected as separate eluates and recovered further as struvite (Liberti et al., 1979, 1981). Later, based on these principles, a process named RemNut® was described by Liberti et. al., who considered various possible schemes for simultaneous nutrient recovery. One possibility, as shown by the authors, is to recover nutrients such as P, N, and K using IE membranes and subsequent precipitation with a calculated production performance of up to 285 t struvite/year (Liberti et al., 2001).
However, IE resins are not the only materials that could be used for preconcentration purposes. Recently, numerous studies have been reported on natural inorganic adsorbents for ammonium removal as lower-cost solutions (Gupta et al., 2009; Mehta et al., 2015; Sengupta et al., 2015). Rožić et al. investigated N removal using natural aluminosilicates (clay and zeolite) after alkaline and acid modification. Croatian zeolite clinoptilolite from the area of Donje Jesenje and Croatian bentonite clay from the Kutina area were used as natural filtration materials, achieving removal efficiencies of up to 61.1% wt (Rožić et al., 2000). Wen et al. (2010) obtained N removal efficiencies of up to 85% using natural and NaCl-pretreated zeolites with subsequent chemical precipitation and recovery of ammonium (Wen et al., 2010). Langwaldt (2008) studied the adsorption capacities of different clays in continuous and batch tests against particle size, pH, temperature, and potassium concentration, achieving adsorption capacities of 48.3 mg NH4+/g zeolite (Langwaldt, 2008). Natural and NaCl-pretreated Bulgarian clinoptilolite from the Beli Plast deposit were studied by Vassileva et al. under different adsorption conditions. The maximum adsorption capacity was 18.4 mg NH4+/g (Vassileva and Voikova, 2009). A thermodynamic process of ammonium IE on pretreated Chinese natural clinoptilolite was studied by Wang et al. (2008). Jha et al. studied sodium hydroxide pretreatment and wet ball milling as treatments for Japanese natural clinoptilolite, achieving a maximum NH4+ retention capacity of 25.2 mg/g (Jha and Hayashi, 2009). Mostly, adsorption capacities reported in the literature reflect the adsorbents’ characteristics under ideal modeling conditions in the absence of competing ions. Under actual conditions, the capacities of zeolites and clays could substantially differ from the model values.
Another demerit of natural materials is that their capacity and properties could vary depending on production site conditions. Geopolymers, a different class of aluminosilicates, have also been investigated as ammonium-removal materials (Luukkonen et al., 2019; Samarina and Takaluoma, 2019). Geopolymers are amorphous analogues of zeolites. They possess similar adsorption properties for different pollutants. Luukkonen et al. obtained adsorption capacities of up to 21.1 mg NH4+/g for metakaolin-based geopolymers (MKGP) (Luukkonen et al., 2016; Luukkonen et al., 2017). For geopolymers prepared with fiber sludge from papermill, the maximal capacity was 36 mg/g (Samarina and Takaluoma, 2019). The major difference between geopolymers and naturally occurring materials is the stability of the production process ensuring consistency of their performance qualities.
This study aimed to examine metakaolin-based geopolymer formulations and to design an efficient recovery scheme for the recovery of ammonium N from diluted wastewaters. Inorganic polymers obtained by alkaline activation of aluminosilicate precursor in the form of granules, and utilizing low-cost and/or abundant byproduct materials, commercial metakaolin, or metakaolin retrieved from papermill sludge, in water treatment were examined. The final evaluations of the ammonium N removal scheme were conducted on actual wastewater matrices, which distinguishes this research from most of the literature examples. A systematic investigation of regeneration in a cyclic regime was conducted for the first time. A hybrid wastewater treatment scheme for N removal and recovery has been proposed.
2 Materials and methods
In this section, a description of adsorbents, adsorbate, samples used, methods of analysis, and experiments conducted is presented.
2.1 Materials and wastewater samples
Ammonium chloride, sodium chloride, sodium hydroxide, sulfuric acid, phosphoric acid, sodium sulfate, potassium sulfate, and dipotassium phosphate were purchased from VWR Chemicals. Sodium silicate (ZEOPOL 25, 42%–46%, molar ratio SiO2:Na2O is 2.4–2.6) was purchased from JV Huber. Commercially available metakaolin was obtained from Aquaminerals Finland Oy. Ammonium chloride was dried at 105°C for 2 h prior to use. The papermill sludge was preliminarily dried at room temperature for 4 days. The sludge was calcined for 3 h at 750°C in order to convert the remaining kaolinite to metakaolin. The results of XRF analysis and surface characteristics of commercial and retrieved metakaolin are presented in Supplementary Tables S1, S2. Salts used for regeneration (sodium chloride, sodium sulfate, potassium sulfate, and dipotassium phosphate) were of technical grade and applied as received without further purification.
Synthetic solutions prepared with tap water and spiked with ammonium of the required concentration were used to define the optimal operation conditions for a fixed-bed adsorption layout, an air-stripping tower, and a membrane contactor. The range of ammonium concentration in the inlet stream for stripping experiments was 250–1,200 mg/L. Run-off mine water (local mine in the Lapland region) and wastewater treatment plant samples (effluent after secondary treatment, Kajaani Vesi) were collected into plastic tanks and preserved with sulfuric acid (1 ml per 1 L of the sample). The composition of wastewaters is shown in Table 1. Prior to the adsorption experiments, the required amount of wastewater sample was taken from the storage tank, the pH of the sample was adjusted with 5M NaOH to pH 6.5–7.2, and ammonium was spiked to a level of 40 mg/L.
2.2 Methods
An Eirich high-intensive mixer (model R05/T) was used to prepare the geopolymers in a granulated form. The chemical composition of the prepared geopolymers was determined using X-ray fluorescence spectroscopy (PanAnalytical Minipal 4, Omnian software). X-ray diffractograms of geopolymers were recorded using a powder X-ray diffractometer (XRD, a PANAlytical X´Pert PRO MPD diffractometer, Co Kα radiations generated at 40 kV and 40 mA, step width of 0.02°, Highscore software 3.0). The initial and residual concentration of ammonium was determined using an ammonium ion-selective electrode (Orion ThermoFS) according to EPA Method 350.3. The surface characteristics, namely, specific surface areas and pore volume of the initial materials and the prepared geopolymers, were determined from N adsorption isotherms measured at −196°C using a high-performance gas and vapor adsorption instrument (Micrometrics ASAP 2020). The specific surface area was calculated based on the Brunauer–Emmett–Teller (BET) isotherm. Pore size distributions were calculated from the desorption data using the Barrett–Joyner–Halenda (BJH) method.
2.3 Preparation of geopolymers
A full preparation procedure for bulk geopolymers is described in other studies (Luukkonen et al., 2017; Luukkonen et al., 2018; Samarina and Takaluoma, 2019). Based on the ammonium adsorption capacity of bulk geopolymer formulations and economic feasibility, the composition of alkaline activator 8M NaOH: Na2SiO3 = 1.2 was selected for a granulation process. A lower molarity alkaline solution (6M NaOH) resulted in a 12% decrease in the ammonium removal rate (Samarina and Takaluoma, 2019). With a lower ratio between alkaline and sodium silicate in the alkaline activator composition, the resulting granules were brittle, and unreacted metakaolin was visually observed. The reaction mass is overheated during granulation if alkaline with higher molarity (12–14M NaOH) was used, leading to an increase in the size of granules or, with further mixing, to the formation of a liquid slurry. As a general procedure, granulated geopolymers were formed by mixing commercial metakaolin or metakaolin retrieved from papermill sludge and the alkaline activator (8M NaOH: Na2SiO3 = 1.2) at a solid-to-liquid ratio (S/L) of 3:1. The solid and liquid components were loaded in a mixer simultaneously. The mixer rotating impeller was set at a speed of 80 rpm for 3 min and then 30 rpm for 12 min with visual control of the resulting granules. The obtained geopolymers were named MKGP and FS MKGP. The granules were left to consolidate for 3 days at ambient temperature. The main fraction was 1–4 mm and represented no less than 80% of the total mass of the prepared adsorbents. Then, the granules were sieved to obtain fractions with a particle size of 0.25–1 mm, 1–4 mm, and 4–8 mm.
2.4 Adsorption–desorption column experiments in the cyclic mode
A fraction of 0.25–1 mm was used for preliminary batch tests with the collected wastewater. To this end, 5 g of each geopolymer was agitated with 200 ml of wastewater (overhead shaker) for 1 h.
To perform column experiments, plastic fixed-bed columns of 3.15 cm internal diameter and 11.5 cm height (volume 80 mL) were loaded with 40 g of the granulated geopolymer material (1–4 mm) and rinsed with tap water until the effluent pH was 7.8. The empty bed volume was 40 ml for both MKGP and FS MKGP adsorbents. The experiments in the continuous fixed-bed mode were performed by pumping synthetic, spiked run-off, or municipal wastewater samples at an upward constant flow rate.
The first series of experiments aimed at the optimization of the adsorption procedure and were conducted utilizing synthetic solutions. For that purpose, tap water was spiked with ammonium ions to a level of 40 mg/L. The impact of sample retention time in a column was investigated at various flow rates (0.6, 0.35, or 0.24 L/h) to compare possible matrix effects as empty bed contact time (EBCT). The spiked water was pumped through the column for 1 h at the set flow rate. The amount of ammonium was determined in a feed vessel before the absorption and in a receiving vessel after 1 h.
To obtain a fully saturated adsorbent prior to the regeneration experiments, tap water spiked with 140 mg/L of ammonium was pumped through the column at a flow rate of 1 L/h. Both potassium and sodium salts were used for regeneration experiments with at least five adsorption–desorption cycles. Each of the regeneration solutions was pumped at a flow rate of 0.24 L/h (at 10 min EBCT). The ammonium concentration in effluents was determined at regular time intervals, and the obtained breakthrough curves were used for the interpretation of results. Next, the regeneration solution was purified by one of the stripping methods to a residual amount of ammonium less than 0.05 mg N/L. The purified regeneration solution was used repeatedly for further adsorbent regeneration. Five regeneration–purification cycles were conducted to estimate the system sustainability and chemical consumption demand.
Figure 1 shows a schematic diagram of the experimental layout. The adsorption unit consisted of two adsorption columns, one of which was in the adsorption mode while the other was in the regeneration mode. This layout was designed to demonstrate the workability of the entire N recovery technology in a continuous mode.
2.5 Ammonium recovery via stripping techniques
2.5.1 Air-stripping experiments
Demonstration experiments were performed in a laboratory-scale air-stripping tower (Supplementary Figure S1) coupled sequentially with a scrubber for the absorption of ammonia as ammonium sulfate. The experimental setup consisted of a vertical acrylate stripping column (diameter 5.5 cm, height 35 cm) jacketed with a temperature-isolating material to minimize possible heat loss. The tower was filled with Raschig rings (6 mm, packing factor 1,600), and the empty bed volume was 0.5 L. An air compressor provided air to the system via a gas flow meter at a range of 1–6 L/min. A gas distributor was installed to ensure sufficient interfacial contact. In addition, a demister was installed at the top of the stripping column to prevent foam from entering the controlling valve or the absorption tank. Air was supplied through the packing material openings at the bottom of the tower as the ammonia-laden regeneration solution was pumped to the top of the packed tower using a peristaltic pump from a storage tank. The purified solution was pumped out from the bottom of the tower using a peristaltic pump back to the storage tank. The solution in the storage tank was preheated to the desired temperature using a hot plate. Thermosensors were installed to control the temperature in a tank for a liquid phase and supplied air. The sensors were connected to a temperature-contorting unit to track the temperature changes and to preset the designated temperatures.
The experiments were conducted under a variety of operational conditions, including the changes in pH, the composition of the liquid phase, the liquid flow rate, the airflow rate, and their temperatures. The air-stripping tower was loaded with a sample preheated to the designated temperatures (25, 30, 35, 40, or 45°C). Prior to sample loading, the sample was adjusted to different pH values (10.5, 11, and 12) using solid NaOH. Three prescribed air flow rates were set at 1, 4, and 6 L/min. The stripped ammonia was absorbed using 500 ml of sulfuric acid (10%). Samples (up to 5 ml) were taken periodically from the regeneration and adsorption tanks to measure the ammonium concentration and pH. To compensate for the sample loss, the same amount of liquids (regeneration solution or absorption solution without ammonium) was refilled into the system. All the experiments were carried out in duplicate, and averaged values have been reported.
2.5.2 Membrane-stripping experiments
A laboratory-scale demonstration using the transmembrane chemical absorption (TMCA) technique was carried out in parallel with air-stripping experiments to compare the feasibility of both approaches (Supplementary Figure S3). As for the air-stripping layout, the liquid phase obtained during adsorbent regeneration was purified in a Liqui-Cel® membrane contactor to recover the ammonium N as ammonium sulfate or phosphate. Operational conditions of the TMCA process, such as shellside and lumenside feed speed, temperature of the feed solution, and pH of the feed and receiving phases, were optimized to determine the maximal capacity of the setup. One contactor 2.5×8-inch Liqui-Cel® membrane was used to determine the optimal operational conditions that would guarantee high performance at the fastest recovery rate. Technical-grade sulfuric acid or phosphoric acid, up to 5%, was used as the lumenside stream. Prior to the pumping, a shellside stream was preheated to the designated temperatures (25°C, 30°C, or 40°C). Different pH values (10.5, 11, and 12) were tested for a feed stream to minimize the expenses on chemicals.
An experimental run was carried out under optimal operation conditions with the regeneration solution collected during a small pilot (side project) of MKGP at a local municipal wastewater treatment station. The initial concentration of the ammonium in the feed phase was 3030 ± 51 mg N/L (n = 3, p = 0.05), and the volume of the treated solution was 70 L. The pH of the feed and receiving phases was controlled permanently and adjusted by the addition of solid NaOH or concentrated H3PO4 to maintain a pH higher than 11 (shellside) or lower than 4 (lumenside) during the experimental run. The phosphoric acid (5%, 5.5 L) served as the receiving phase.
3 Results and discussion
3.1 Adsorption–regeneration in the cyclic mode
Preliminary batch adsorption tests with the granulated forms showed the ability of both geopolymer adsorbents to remove ammonium from low-laden ammonium streams with complex matrixes such as an effluent after secondary treatment (up to 50 mg NH4-N/L) or run-off mine wastewaters (up to 40 mg NH4-N/L). The FS MKGP had a better ammonium N removal rate in wastewaters with high concentrations of competing ions than the MKGP (Figure 2).
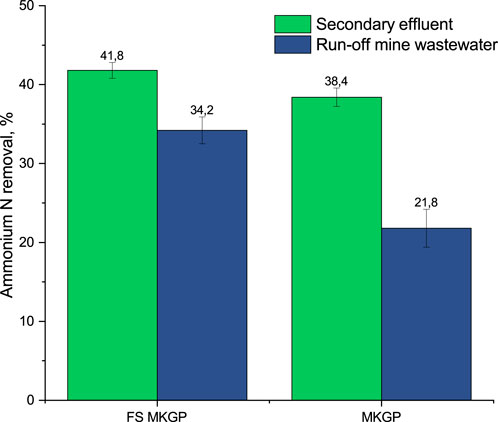
FIGURE 2. Removal rate of ammonium N from wastewaters by MKGP and FS MKGP in a batch mode: 5 g of adsorbent with particle size 0.25–1 mm, 1 h agitation time, pH 6.8 (n = 3, p = 0.95).
3.1.1 Optimization of ammonium N removal by fixed-bed column adsorption
The main goal of using adsorption prior to the recovery of ammonium via stripping techniques is to increase the ammonium concentration in the feed stream to facilitate the mass transfer rate. The lower the ammonium concentration in the feed phase of air-stripping or membrane shellside, the longer the time of treatment will be. To define the main characteristics of a developed preconcentration technology, the effects of common parameters such as EBCT and adsorbent layer on adsorption and composition of the regeneration solution were systematically investigated. The EBCT varied from 2 to 10 min at an adsorbent layer of 10 cm (fully filled column) for both the adsorbents. The removal rates were at least 90% (Supplementary Figure S4) for EBCTs from 4 min (flow rate 0.6 L/h) to 10 min (flow rate 0.24 L/h), while a further decrease of EBCT to 2 min (flow rate 1.2 L/h) decreased the removal rate by third for both the adsorbents. The decrease in the removal rate at the shortest EBCT is likely attributed to the adsorption kinetics, which is governed by the diffusion of the adsorbate to the adsorbent surface and in the adsorbent pores. According to the batch tests for the powder forms (Luukkonen et al., 2018; Samarina and Takaluoma, 2019), the adsorption of ammonium by both adsorbents in the first stage proceeds rapidly. The main part of ammonium was absorbed during the first hour on the adsorbent surface carrying a negative charge. To reach full saturation, a longer time was required, probably due to the slower diffusion of ammonium ions into the pores of the adsorbent. At flow rates higher than 0.6 L/h and a relatively low initial ammonium concentration in a continuous mode, a substantial amount of ammonium was eluted from the column due to insufficient contact time.
The optimum EBCT for the investigation of the adsorbent layer effect was established as 4 min (with a flow rate of 0.6 L/h). The effect of the adsorbent layer was investigated for layers 5 (half filling), 7.5, and 10 cm (full filling). To keep the EBCT at a constant level, in the first two cases, gaps at the top of the columns were filled with an inert material (lightweight expanded clay aggregate, LECA) with the same granule size. Ammonium uptake by adsorbents in a fixed-bed column mainly depends upon the height of the adsorbent bed in the column. Higher layers of adsorbents resulted in a higher removal rate, which, in turn, led to higher concentration in a regeneration solution. The exhaustion time (Ct/C0 = 0.95) increased with an increase in the adsorbent bed height (Figure 3) owing to the availability of more adsorption sites at a higher loading mass and an increased contact time between the adsorbate and adsorbents. The slope of the breakthrough curves slightly decreased with an increase in the height of the adsorbent bed, which may correspond to a wider mass transfer zone (Patel, 2019).
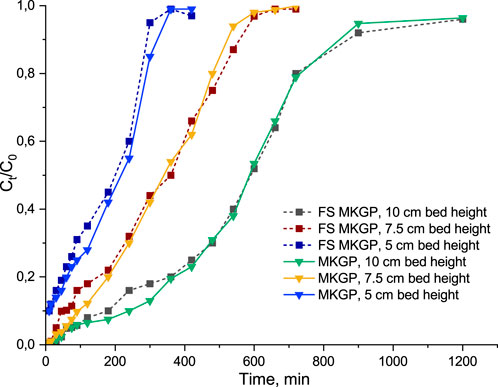
FIGURE 3. Breakthrough curves at different adsorbent bed heights for ammonium adsorption onto MKGP and FS MKGP: initial ammonium concentration 40 mg/L, EBCT 4 min.
To better understand the geopolymer performance with complex matrixes, the actual samples of wastewaters, effluent from WWTP after the secondary treatment and local mine run-off water, were used for fixed-bed column adsorption experiments. It was found that MKGP had a removal ability equal to FS MKGP toward ammonium N when adsorption was conducted from effluents after the secondary treatment. The removal rates measured after an exhaustion point (C/C0 = 0.95, effluent volume 12 L) were 67% and 65% for FS MKGP and MKGP, respectively. However, FS MKGP showed a higher protective time of up to three times that of the column (C/C0 = 0.5) compared to MKGP when a local mine run-off wastewater was used as the matrix (Figure 4). The difference in the adsorption behavior can be attributed to the structure of the adsorption materials, more specifically to better selectivity of FS MKGP toward the macrocomponents coexisting with the target substance in the treated stream (Table 1). The XRD patterns of the prepared geopolymers (Supplementary Figure S2) revealed the existence of a zeolite phase (analcime, 12 wt%) in the FS MKGP structure, while the XRD diffractogram of MKGP showed no indication of any zeolite structure in it.
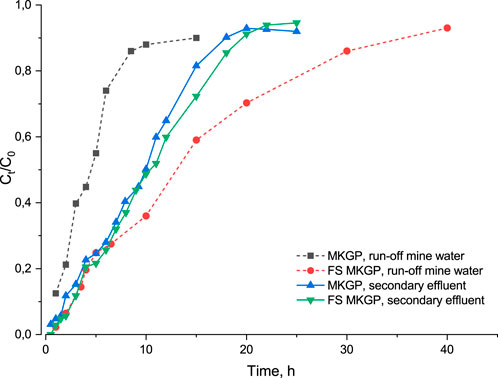
FIGURE 4. Breakthrough curves for ammonium adsorption from the actual wastewaters onto MKGP and FS MKGP: initial ammonium concentration 40 mg/L, EBCT 4 min.
3.1.2 Desorption of ammonium after fixed-bed column adsorption
To decrease the operational costs of the overall technology, the regeneration of adsorbents was considered. Sodium chloride and sodium sulfate, as well as potassium sulfate and potassium phosphate, were studied as potential regeneration solutions (with sodium/potassium ion concentration 1 M, pH 12). Although sodium salts are less expensive options, potassium salts were selected because of their potential to be used directly after desorption as liquid fertilizers. Each of the regeneration solutions was pumped at a flow rate of 0.24 L/h (at 10 min EBCT). Five adsorption–desorption cycles with synthetic solutions (140 mg/L NH4+ spiked in tap water) were conducted to evaluate if the removal abilities of adsorbents decreased substantially. After each desorption cycle, a new adsorption cycle was performed, and the removal rate was calculated. Regeneration of both adsorbents with sodium chloride or sodium sulfate resulted in 68%–79% ammonium recovery (1 L of regeneration solution, 1M Na+), while both potassium salts, chloride or sulfate, brought about 96%–100% ammonium recovery. Potassium phosphate was the least effective among all the regeneration solutions for both the adsorbents (only 31% regeneration). This may be due to the higher buffering capacity of the phosphate solution than that of chloride or sulfate potassium salts. The pH of the freshly prepared solution with 1M K+ was 8.6, while the pH of the rest potassium-containing solutions was no less than 12. It was also found that after regenerating FS MKGP with potassium salts, the removal efficiency towards ammonium at the next adsorption cycle reduced substantially, up to 55%. It is important to emphasize that the removal ability could be restored by filling the column with 5 M sodium chloride for 24 h. That result might possibly reflect a higher affinity of FS MKGP toward potassium ions due to the presence of zeolite in its structure.
It was noted that the higher the concentration of ion-exchange ion (sodium, for instance) was present in the regeneration solutions, the faster, and with less volume of regeneration solution, a full regeneration could be accomplished. Higher sodium concentrations in the regeneration solution resulted in shorter times of desorption of adsorbed ammonium N (Supplementary Figure S5). Therefore, with a highly concentrated regeneration solution, most of the ammonium may be collected from the first portions of the regeneration solution, and dilution of the regeneration solution prior to ammonium N recovery can be avoided. According to the preliminary calculations of a cost treatment per kilo of N, the costs of chemicals used were a decisive factor in defining the costs of the whole ammonium N removal and recovery technology. Thus, the most cost-effective solution (5 M NaCl, pH 12) was selected for further adsorption experiments with actual samples and to test the air-stripping/scrubbing or membrane technique for ammonium N recovery.
To calculate enrichment factors, desorption of ammonium was conducted after full column saturation with the actual wastewaters. Ammonium N was desorbed by 1 L of 5M NaCl (pH 12) at a flow rate of 0.24 L/h. Enrichment factors with FS MKGP were up to 32 and 4.6 for the secondary effluent sample and industrial run-off water, respectively. In the case of MKGP, only an application for preconcentration of ammonium N from secondary effluents was feasible (enrichment factor 27) because the enrichment factor with industrial run-off water was 1.1.
3.2 Air-stripping experiments and ammonium recovery from real samples
Ammonium N preconcentrated by the regeneration solution was removed using the air-stripping technique on a tower filled with Raschig rings as the most cost-effective filler. In the first instance, an experiment on an air-stripping tower evaluated the possibility to remove ammonia at pH 12 and 20°C from a synthetic solution with 2,500 mg/L as ammonium because, according to the literature sources (Huang and Shang, 2006), this pH should be enough to convert up to 95% of ammonium into ammonia. The assumption was made that purification of the liquid phase containing ammonium could be done without any external heat supplement. However, only 36.8% of ammonia was removed after 24 h by an air flow at the maximum possible velocity (6 L/min), which was not enough for further use of regeneration solution in the proposed adsorption–desorption scheme for ammonium recovery. Thus, increasing the temperature of a treated regeneration solution was essential to obtain full ammonia removal from the regeneration solution. The liquid phase was preheated at five different temperatures in 5 ± 2°C increments. After 24h, 39.2, 59.1, 85.0, 98.1, and 98.9% of ammonium N were removed from the treated regeneration solution preheated to 25, 30, 35, 40, and 45°C (Figure 5).
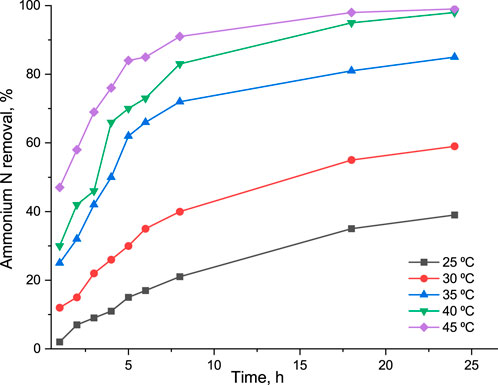
FIGURE 5. Removal rate of ammonium N from the regeneration solution (5M NaCl, pH 12) by air-stripping technique at different operational temperatures: initial ammonium concentration 2,500 mg/L, gas flow 6 L/min, liquid flow 0.25 L/h.
In this manner, the liquid phase preheated to 45°C ± 2°C was used at the second stage, and a conversion rate of 91% after 8 h was reached. It is noteworthy that higher ammonium removal rates from the liquid phase at lower temperatures via an air-stripping approach could be reached if a longer treatment time was used (Figure 5). However, it would lead to a higher energy demand and, as a consequence, to a higher operational cost of the treatment. Another parameter that had a drastic effect on the removal of ammonia from the regeneration solution was the velocity of the airflow. The conversion rate decreased from 69% to 28% with a decrease in air supply from a maximum of 6 L/min to 1 L/min. The temperature of the gas phase had no influence on the conversion rate, probably due to small scale. However, there are examples in the literature suggesting that the temperature of the gas phase may play a crucial role (Huang and Shang, 2006; Alam and Hossain, 2009; Lubensky et al., 2019). As the air-stripping-scrubbing system is designed as a close-loop system, the retention time in the stripping tower might have an influence on the ammonium conversion rate. Nevertheless, no difference was observed for retention times of 5 and 25 min after 24 h of the operation at 30°C, and the conversion rate did not exceed 62%.
After the regeneration solution was purified, e.g., the ammonium concentration was lower than 0.05 mg N/L; it was used over five times for the desorption procedure. The removal of ammonium from a fully saturated adsorption column with the purified regeneration solution was 92% ± 3% for MKGP and 91% ± 4% for FS MKGP within five adsorption–desorption cycles (Figure 6). That changed when the actual wastewater samples were used in adsorption–regeneration cycles. After the first cycle, a slight improvement in the removal rates was observed for both adsorbents. This is consistent with earlier results published by Luukkonen et al. (2016); Luukkonen et al. (2018) and could be explained by the substitution of calcium and magnesium balancing ions of the pristine geopolymers by sodium ions from the regeneration solution. As a consequence, it increased the number of adsorption sites for ammonium ions in the second cycle resulting in the higher removal rates. However, a downward trend of the removal rates was observed with each subsequent regeneration cycle for both adsorbents when run-off mine wastewaters were used for the column saturation. In the case of FS MKGP (Figure 6B), a decline in the removal of ammonium N on the successive cycles after regeneration by the purified regeneration solution was more noticeable. There could be at least two possible reasons for this behavior. Since the effect is more evident for FS MKGP, the adsorption capacity might have been reduced by the occupation of adsorption sites with competing ions, e.g., potassium, present in the sample. Potassium was removed only partially during the desorption cycle due to insufficient time for ion-exchange under the present conditions (Supplementary Table S2). Furthermore, these competing ions would be desorbed from the adsorbent surface during the regeneration cycle and accumulated in higher and higher concentrations in the regeneration solution in each subsequent regeneration–purification cycle. More detailed investigation on this issue should be conducted in the future for a better understanding of the regeneration process.
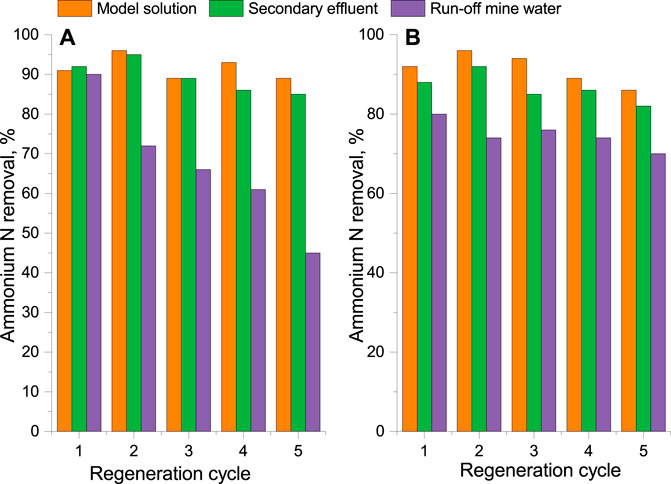
FIGURE 6. Removal rate of ammonium N from the model solution and the actual wastewaters within 5 adsorption–desorption cycles. (A) FS MKGP; (B) MKGP.
3.3 Ammonium recovery using a membrane contactor
Equally, the air-stripping and the transmembrane chemical absorption (TMCA) technique would not be economically feasible if applied directly for the treatment of diluted wastewater streams. Operation energy consumption and cost of chemicals for pH adjustment and treated water neutralization would level out merits recovery technology. However, the treatment of regeneration solution with preconcentrated ammonium N could be an attractive solution, because the pH of the solution is already at the required level, a high concentration of ammonia N ensures a high mass transfer rate, and the volume of liquid phase to be treated is reduced.
The membrane-based system for ammonium recovery consisted of an adsorption/desorption unit, a storage tank for regeneration solution, and a 3M Liqui-Cel® membrane contactor (Supplementary Figure S3). For preliminary experiments, 50 L of synthetic solution, 5M NaCl (pH 12) spiked with 1,000 mg/L as ammonium, was used to establish the optimal operational conditions. The speed of shellside and lumenside feed streams, working temperature, and pH of feed and receiving streams were evaluated.
At an equal speed of 100 L/h of both feed and receiving streams (5% sulfuric acid), full removal of ammonium N from the regeneration solution was reached at 22 h at 40°C. Lowering the receiving phase speed to 60 L/h with a feed flow of 100 L/h reduced the conversion time to10 h (40°C).
Technical-grade sulfuric acid or phosphoric acid was used as the lumenside stream. Phosphoric acid was selected because ammonium phosphate as the final product would have more market potential. Moreover, several approaches for the recovery of phosphorous from waste streams as phosphoric acid have been reported (Egle et al., 2018; Di Capua et al., 2022). Although ammonium nitrate could be an interesting option in terms of the final product, nitric acid was not used because of safety and legal issues. The working temperature was varied from 25°C to 40°C in order to find a balance between energy consumption and performance of the TMCA process. Generally, the conversion rate in phosphoric acid after 8 h was higher for all the working temperatures tested (Figure 7). However, the total time to the full removal of ammonium (concentration less than 0.2 mg N/L) from the feed phase was less for sulphuric acid. Thus, the full removal of ammonium from the regeneration solution at 40°C was reached after 12 and 18 h for treatment with sulphuric acid and phosphoric acid, respectively.
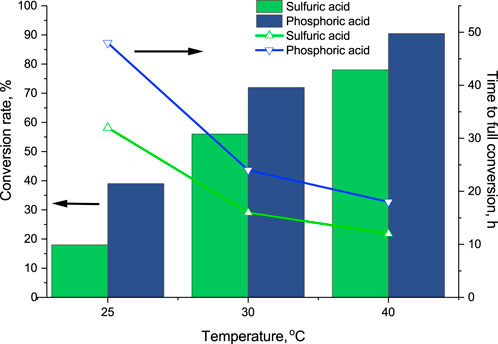
FIGURE 7. Influence of working temperature (8 h operational time) on ammonium conversion rate and time to full conversion (at 95% conversion rate). Shellside line: the regeneration solution, 5M NaCl, pH 12; lumenside line: 5% sulfuric or phosphoric acid.
The pH values on the shellside and lumenside of the membrane contactor are critical for the efficiency of the treatment. The shellside (feed phase) was less sensitive to this parameter and could be operated in the range of 10.5–12 pH without the loss of removal efficiency. The pH on lumenside, in turn, had more influence on the efficiency of the removal. The conversion rate decreased as the pH increased. Although a conversion rate of up to 89% was observed at a pH of more than 4.5, the time for full ammonium removal from the feed phase increased for 12–16 h. The following operational conditions were found to be acceptable and economically viable: 100 L/h shellside and 60 L/h lumenside feed streams, 40°C working temperature, pH ≥ 11. As the next step, experiments with regeneration solution (5 M NaCl, pH 12) obtained after the regeneration of MKGP containing ammonium adsorbed from municipal wastewater were carried out at the operational conditions listed earlier. The full removal of ammonium from the regeneration solution took 18 h. No scaling or clogging of the membrane contactor was observed. The pH of the resulting solution was 5.75, and the final amount of ammonium recovery was 198 g, i.e., a 93.6% conversion rate. Because a negligible amount of ammonium N was detected in the feed phase at the end of the process, the possible loss of ammonium (6.4%) can be explained by volatilization from the feed tank in the course of pH adjustment operations and sampling.
4 Future work and perspectives
The parameters established by this research were used to design (Figure 8 and Supplementary Figure S6) and construct a small-scale mobile unit for wastewater treatment (Supplementary Figure S7). Three adsorption columns were planned to ensure the wastewater treatment in continuous mode with a maximal treatment capacity of 1 m3/h. Two columns were reserved for adsorption, while the third column would be in regeneration mode. The TMCA technique was chosen as a simpler and more flexible approach, and a membrane contactor was installed (Supplementary Figure S8), providing the 0.5 m3/h treatment capacity for the purification of the regeneration solution. To further develop the presented technology, research at a pilot-scale on the industrial sites is required. To estimate the most economically feasible model through economic evaluation and to test the scalability of the process, larger-scale demonstration is essential. Multifaceted factors, including both economic and environmental factors, will likely be a strong driver for the removal and recovery of N and further valuable materials (P, K, and water). Precise estimation of treatment costs should be conducted to expand the product portfolio and help offset the treatment costs.
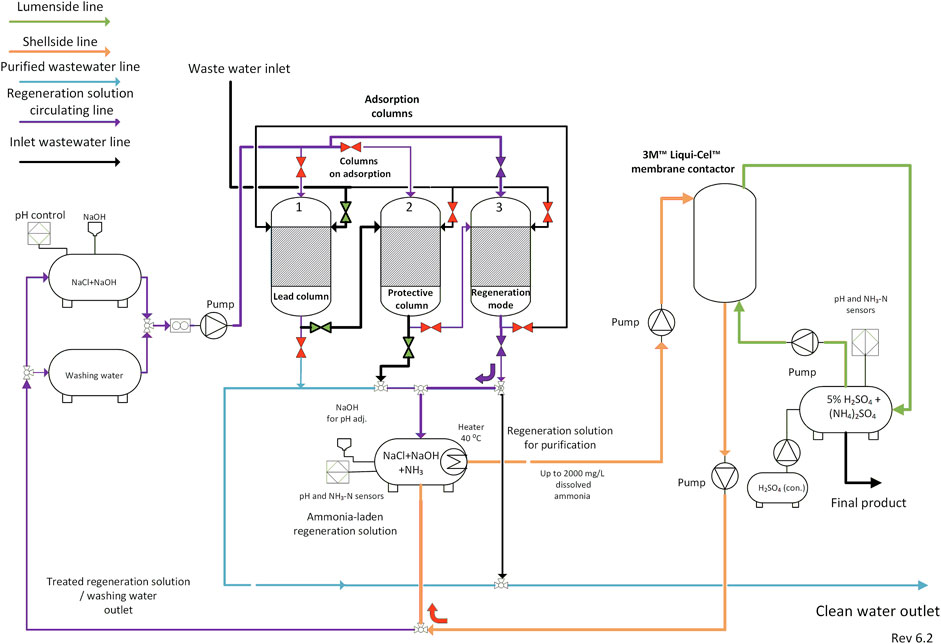
FIGURE 8. Principal schematic diagram of the proposed N recovery approach (transmembrane chemical absorption technique).
5 Conclusion
A laboratory-scale demonstration of a new hybrid ammonium N removal and recovery technology has been described. Possible variants of regeneration regimes and the composition of regeneration agents were studied to restore the exhausted granules after ammonium removal and to estimate the possible positive and negative scenarios of their further usability. A substantial difference in adsorbents’ behavior after adsorption was ascertained. The MKGP granules could be restored by sodium and potassium salts prior to the next regeneration cycle, whereas the FS MKGP granules lost their ability to adsorb ammonium N effectively after regeneration with potassium salts. Although potassium chloride and sulfate salts had better regeneration ability for both adsorbents, 5 M sodium chloride was chosen as the most suitable and cost-effective regeneration solution. Moreover, the solubility of sodium chloride enables an application of a highly concentrated regeneration solution, leading to a reduction in its volume and avoiding the dilution of a resulting concentrate. Potassium phosphate was found to be an unacceptable option due to low regeneration rate, only 31% of adsorbed ammonium N was collected in the regeneration solution. Thus, the enrichment of potassium phosphate with ammonium proved to be impractical for the direct preparation of liquid fertilizers as was planned initially. The actual water matrices had a great influence on successive regeneration. The positively charged ions contained in complex wastewater were adsorbed simultaneously with ammonium N due to limited selectivity, changing the composition of the regeneration solution and the ability to remove the target in successive saturation–regeneration cycles.
The use of the physical–chemical approach (air-stripping with sequential acid-scrubbing or transmembrane chemical absorption) coupled with the adsorption preconcentration technique allows to recover ammonium N at relatively low temperatures. Purification of the regenerative solution by air-stripping or membrane technology can be carried out in less than 16 h at 40°C with an ammonium N recovery of at least 90%. The recovery unit (a stripping tower or a membrane contactor) could be placed inside the wastewater treatment facilities, which would substantially decrease the energy consumption required for regeneration solution treatment. The adsorption/desorption unit could be operated at 4°C without any substantial loss of removal rate efficiency. The loop mode for both adsorption/desorption and ammonium recovery units makes the process flexible and scalable and provides a high recovery rate.
Data availability statement
The original contributions presented in the study are included in the article/Supplementary Material; further inquiries can be directed to the corresponding author.
Author contributions
TS: conceptualization, investigation, data curation, validation, methodology, and writing—original draft. LG: investigation, formal analysis, and writing—original draft. ET: conceptualization, methodology, and writing—reviewing. OL: data curation, validation, resources, and writing—reviewing. ST and JP: validation, supervision, and writing—reviewing. All authors have read and agreed to the published version of the manuscript.
Funding
This study was conducted as part of the WaterPro project (number A74635 EAKR, Keski-Pohjanmaan Liitto/Kainuun Liitto/Pohjois-Pohjanmaan liitto) and REMAC project (KA11000, Karelia CBC).
Acknowledgments
The authors wish to thank Jani Heikkinen for assisting with the supporting design and construction of laboratory demonstration experiments. Additionally, the authors are deeply grateful to 3M company for providing a non-commercial sample of membrane contactor for research.
Conflict of interest
The authors declare that the research was conducted in the absence of any commercial or financial relationships that could be construed as a potential conflict of interest.
Publisher’s note
All claims expressed in this article are solely those of the authors and do not necessarily represent those of their affiliated organizations, or those of the publisher, the editors, and the reviewers. Any product that may be evaluated in this article, or claim that may be made by its manufacturer, is not guaranteed or endorsed by the publisher.
Supplementary material
The Supplementary Material for this article can be found online at: https://www.frontiersin.org/articles/10.3389/fenvs.2022.1033677/full#supplementary-material
References
Alam, R., and Hossain, Md. D. (2009). Effect of packing materials and other parameters on the air stripping process for the removal of ammonia from the wastewater of natural gas fertilizer factory. J. Water Resour. Prot. 01, 210–215. doi:10.4236/jwarp.2009.13026
Ammonia: zero-carbon fertiliser, fuel and energy store (2020). Low carbon energy programme. Available at: https://royalsociety.org/topics-policy/projects/low-carbon-energy-programme/green-ammonia/(Accessed September 20, 2022).
Costamagna, P., Giordano, A., Lazzarini, Y., Delucchi, M., and Busca, G. (2020). Process of ammonia removal from anaerobic digestion and associated ammonium sulphate production: Pilot plant demonstration. J. Environ. Manag. 259, 109841. doi:10.1016/j.jenvman.2019.109841
Di Capua, F., de Sario, S., Ferraro, A., Petrella, A., Race, M., Pirozzi, F., et al. (2022). Phosphorous removal and recovery from urban wastewater: Current practices and new directions. Sci. Total Environ. 823, 153750. doi:10.1016/j.scitotenv.2022.153750
Dong, D., Choi, O. K., Lee, K., and Lee, J. W. (2020). Pilot-scale demonstration of nitrogen recovery in the form of ammonium phosphate (AP) from anaerobic digestate. Bioresour. Technol. 297, 122392. doi:10.1016/j.biortech.2019.122392
Egle, L., Zoboli, O., Amann, A., Krampe, J., Rechberger, H., and Zessner, M. (2018). “Comparison of technologies for phosphorus recovery – identification of an ideal solution?,” in Phosphorus: Polluter and resource of the future – removal and recovery from wastewater. Editor C. Schaum (International Water Association), London, UK, ISBN: 9781780408354 (Print) 455–486. doi:10.2166/9781780408361_457
European Sustainable Phosphorus Platform (ESPP) (2020). Phosphorus platform. Available at: https://www.phosphorusplatform.eu/platform/about-espp (Accessed August 13, 2020).
Gupta, V. K., Carrott, P. J. M., Carrott, M. M. L. R., and Suhas, (2009). Low-cost adsorbents: Growing approach to wastewater treatment—A review. Crit. Rev. Environ. Sci. Technol. 39, 783–842. doi:10.1080/10643380801977610
Hasanoğlu, A., Romero, J., Pérez, B., and Plaza, A. (2010). Ammonia removal from wastewater streams through membrane contactors: Experimental and theoretical analysis of operation parameters and configuration. Chem. Eng. J. 160, 530–537. doi:10.1016/j.cej.2010.03.064
Huang, J.-C., and Shang, C. (2006). “Air stripping,” in Advanced physicochemical treatment processes handbook of environmental engineering. Editors L. K. Wang, Y.-T. Hung, and N. K. Shammas (Totowa, NJ: Humana Press), 47–79. doi:10.1007/978-1-59745-029-4_2
International Energy Agency (2007). Paris: OECD Publishing. doi:10.1787/9789264030404-enTracking industrial energy efficiency and CO2 emissions
Jha, V. K., and Hayashi, S. (2009). Modification on natural clinoptilolite zeolite for its NH4+ retention capacity. J. Hazard. Mat. 169, 29–35. doi:10.1016/j.jhazmat.2009.03.052
Langwaldt, J. (2008). Ammonium removal from water by eight natural zeolites: A comparative study. Sep. Sci. Technol. 43, 2166–2182. doi:10.1080/01496390802063937
Liberti, L., Boari, G., and Passino, R. (1979). Phosphates and ammonia recovery from secondary effluents by selective ion exchange with production of a slow-release fertilizer. Water Res. 13, 65–73. doi:10.1016/0043-1354(79)90256-2
Liberti, L., Boari, G., Petruzzelli, D., and Passino, R. (1981). Nutrient removal and recovery from wastewater by ion exchange. Water Res. 15, 337–342. doi:10.1016/0043-1354(81)90038-5
Liberti, L., Petruzzelli, D., and Florio, L. D. (2001). REM NUT ion exchange plus struvite precipitation process. Environ. Technol. 22, 1313–1324. doi:10.1080/09593330409355443
Liu, X., Elgowainy, A., and Wang, M. (2020). Life cycle energy use and greenhouse gas emissions of ammonia production from renewable resources and industrial by-products. Green Chem. 22, 5751–5761. doi:10.1039/D0GC02301A
Lubensky, J., Ellersdorfer, M., and Stocker, K. (2019). Ammonium recovery from model solutions and sludge liquor with a combined ion exchange and air stripping process. J. Water Process Eng. 32, 100909. doi:10.1016/j.jwpe.2019.100909
Luukkonen, T., Heponiemi, A., Runtti, H., Pesonen, J., Yliniemi, J., and Lassi, U. (2019). Application of alkali-activated materials for water and wastewater treatment: A review. Rev. Environ. Sci. Biotechnol. 18, 271–297. doi:10.1007/s11157-019-09494-0
Luukkonen, T., Sarkkinen, M., Kemppainen, K., Rämö, J., and Lassi, U. (2016). Metakaolin geopolymer characterization and application for ammonium removal from model solutions and landfill leachate. Appl. Clay Sci. 119, 266–276. doi:10.1016/j.clay.2015.10.027
Luukkonen, T., Tolonen, E.-T., Runtti, H., Kemppainen, K., Perämäki, P., Rämö, J., et al. (2017). Optimization of the metakaolin geopolymer preparation for maximized ammonium adsorption capacity. J. Mat. Sci. 52, 9363–9376. doi:10.1007/s10853-017-1156-9
Luukkonen, T., Věžníková, K., Tolonen, E.-T., Runtti, H., Yliniemi, J., Hu, T., et al. (2018). Removal of ammonium from municipal wastewater with powdered and granulated metakaolin geopolymer. Environ. Technol. 39, 414–423. doi:10.1080/09593330.2017.1301572
Mehta, C. M., Khunjar, W. O., Nguyen, V., Tait, S., and Batstone, D. J. (2015). Technologies to recover nutrients from waste streams: A critical review. Crit. Rev. Environ. Sci. Technol. 45, 385–427. doi:10.1080/10643389.2013.866621
Monfet, E., Aubry, G., and Ramirez, A. A. (2018). Nutrient removal and recovery from digestate: A review of the technology. Biofuels 9, 247–262. doi:10.1080/17597269.2017.1336348
Nelson, N. O., Mikkelsen, R. L., and Hesterberg, D. L. (2003). Struvite precipitation in anaerobic swine lagoon liquid: Effect of pH and Mg:P ratio and determination of rate constant. Bioresour. Technol. 89, 229–236. doi:10.1016/S0960-8524(03)00076-2
Patel, H. (2019). Fixed-bed column adsorption study: A comprehensive review. Appl. Water Sci. 9, 45. doi:10.1007/s13201-019-0927-7
Phos4You (2020). PHOSphorus Recovery from waste water FOR YOUr Life. Available at: https://www.nweurope.eu/projects/project-search/phos4you-phosphorus-recovery-from-waste-water-for-your-life/ (Accessed August 13, 2020).
Rahman, Md. M., Salleh, M. A. Mohd., Rashid, U., Ahsan, A., Hossain, M. M., and Ra, C. S. (2014). Production of slow release crystal fertilizer from wastewaters through struvite crystallization – a review. Arabian J. Chem. 7, 139–155. doi:10.1016/j.arabjc.2013.10.007
Rožić, M., Cerjan-Stefanović, Š., Kurajica, S., Vančina, V., and Hodžić, E. (2000). Ammoniacal nitrogen removal from water by treatment with clays and zeolites. Water Res. 34, 3675–3681. doi:10.1016/S0043-1354(00)00113-5
Samarina, T., and Takaluoma, E. (2019). “Metakaolin-based geopolymers for removal of ammonium from municipal wastewater,” in Proceedings of the 5th world congress on new technologies. doi:10.11159/icepr19.195
Sengupta, S., Nawaz, T., and Beaudry, J. (2015). Nitrogen and phosphorus recovery from wastewater. Curr. Pollut. Rep. 1, 155–166. doi:10.1007/s40726-015-0013-1
Ulbricht, M., Schneider, J., Stasiak, M., and Sengupta, A. (2013). Ammonia recovery from industrial wastewater by TransMembraneChemiSorption. Chem. Ing. Tech. 85, 1259–1262. doi:10.1002/cite.201200237
Vassileva, P., and Voikova, D. (2009). Investigation on natural and pretreated Bulgarian clinoptilolite for ammonium ions removal from aqueous solutions. J. Hazard. Mat. 170, 948–953. doi:10.1016/j.jhazmat.2009.05.062
Wang, Y., Lin, F., and Pang, W. (2008). Ion exchange of ammonium in natural and synthesized zeolites. J. Hazard. Mat. 160, 371–375. doi:10.1016/j.jhazmat.2008.03.006
Wei, S. P., van Rossum, F., van de Pol, G. J., and Winkler, M.-K. H. (2018). Recovery of phosphorus and nitrogen from human urine by struvite precipitation, air stripping and acid scrubbing: A pilot study. Chemosphere 212, 1030–1037. doi:10.1016/j.chemosphere.2018.08.154
Wen, T., Zhang, X., Zhang, H. Q., and Liu, J. D. (2010). Ammonium removal from aqueous solutions by zeolite adsorption together with chemical precipitation. Water Sci. Technol. 61, 1941–1947. doi:10.2166/wst.2010.145
Keywords: metakaolin-based geopolymer, adsorption, ammonium nitrogen removal, nitrogen recovery and reuse, circular economy, transmembrane chemical absorption, membrane separation, air-stripping
Citation: Samarina T, Guagneli L, Takaluoma E, Tuomikoski S, Pesonen J and Laatikainen O (2022) Ammonium removal by metakaolin-based geopolymers from municipal and industrial wastewaters and its sequential recovery by stripping techniques. Front. Environ. Sci. 10:1033677. doi: 10.3389/fenvs.2022.1033677
Received: 31 August 2022; Accepted: 17 October 2022;
Published: 04 November 2022.
Edited by:
Kim Hung Mo, University of Malaya, MalaysiaReviewed by:
Tee How Tan, Tunku Abdul Rahman University, MalaysiaSalmabanu Luhar, Rochester Institute of Technology, United States
Copyright © 2022 Samarina, Guagneli, Takaluoma, Tuomikoski, Pesonen and Laatikainen. This is an open-access article distributed under the terms of the Creative Commons Attribution License (CC BY). The use, distribution or reproduction in other forums is permitted, provided the original author(s) and the copyright owner(s) are credited and that the original publication in this journal is cited, in accordance with accepted academic practice. No use, distribution or reproduction is permitted which does not comply with these terms.
*Correspondence: Tatiana Samarina, dGF0aWFuYS5zYW1hcmluYUBrYW1rLmZp