- MOE Key Laboratory of Groundwater Circulation and Environmental Evolution, China University of Geosciences Beijing, Beijing, China
This paper reviews progresses in the interactions between goethite and organic matter (OM) and their environmental effects in recent decades. The interactions mainly include the effect of organic matter on the surface properties and the crystallization of goethite, molecular changes of OM caused by goethite, and their interaction mechanisms, which can be depicted by the commonly used Langmuir model, the charge distribution multi-site complexation model (CD-MUSIC model), ligand charge distribution model (LCD model), and natural organic matter charge distribution model (NOM-CD model). The influencing factors of the interactions are summarized with emphasis on the external, including pH, ionic strength, carbon dioxide (CO2), and the internal, including the structure of OM and iron species. The goethite—OM complexes caused by the interactions will affect migration and transformation of conventional heavy metals and emerging antibiotics. The complexes, as the carrier of carbon and iron, are also the critical parts of the carbon and iron cycles, which are associated with climate change. This review provides a basis for future mechanism studies of formation, transformation, and effects of goethite—OM complexes (particulate OM or carbon-contained minerals) in different environmental systems at a molecular level.
1 Introduction
Iron is the fourth most abundant element in nature and widely distributed in the lithosphere. Iron is easy to combine with other elements to form iron minerals, so there is little natural pure iron on the earth, and it often exists in the form of oxidized iron. There are 12 known iron oxides which are grouped into iron hydroxide [Fex(OH)y] and iron oxide (FexOy) in nature. The former includes goethite [α-FeO(OH)], lepidocrocite [γ-FeO(OH)], limonite [FeO(OH)·nH2O], schwertmannite [Fe8O8(OH)6SO4], hexagonite [FeO(OH)], ferrite (Fe2+), garnet [K(AlSi2)O6)], and patina [Cu2(OH)2CO3]; the latter includes hematite (Fe2O3), magnetite (Fe3O4), maghemite (γ-Fe2O3), and ferrous oxide (FeO). Hematite, goethite, and magnetite are relatively stable and mostly exist as end-state products, whereas ferrihydrite, lepidocrocite, maghemite, schwertmannite, and patina are unstable in nature and mostly exist as intermediates. Goethite is one of the most common iron hydroxide minerals in the earth’s surface system. In general, goethite can be formed by the differentiation of olivine or pyrite through complex physical, chemical, and biological processes, which can be described by the following chemical equations (Schwertmann and Cornell, 2000):
Organic matter (OM) is a collection of organic compounds originating from organisms in the critical zones of the earth, showing diverse chemical compositions and structural characteristics, and exhibits various environmental behaviors and ecological effects in the hydrosphere, pedosphere, lithosphere, and their transition zones (Kadar et al., 2011; Maeng et al., 2011). OM is divided into fresh, semi-decomposed, and humified fractions according to the degree of decomposition. Fresh OM refers to plant and animal residues that have just entered the soil, still maintain the original anatomical characteristics of the organism, and have not been decomposed by microbes. Semi-decomposed OM refers to the residues partially decomposed by microorganisms. It has lost its anatomical characteristics and is mostly dark and brown. Humified OM, also known as humic substances (HS), is the gray-black colloidal material formed by the transformation of fresh OM through enzymes, generally accounting for more than 85% of the total soil OM (Wang et al., 2017). HS can be further subdivided into humic acid (HA), fulvic acid (FA), and humin (HM) according to their solubility in acid and alkali. HA is a hardly acid-dissolved macromolecular organic acid with various functional groups, which have physiochemical behaviors, including absorption, complexation, and exchange (Christensen, 1992; Montalvo et al., 2018). FA is a short carbon chain molecular structure extracted from HS with a low molecular weight, which can dissolve in either acid or alkali (Wang et al., 2019). HM is the insoluble fraction after the OM contained in the soil is treated with a diluted mixture of sodium hydroxide and sodium pyrophosphate; it cannot dissolve in neither acid nor alkali (Chiou et al., 2000). Since HM contains few functional groups and is less active, the HS mentioned in this review mainly comprises HA and FA. Besides the natural OM, a large amount of artificial OM, including phthalates, aromatic hydrocarbons, and antibiotics, is discharged into the environment with the increase of anthropogenic activities. Antibiotics are organic substances naturally synthesized by microorganisms through secondary metabolism or artificially synthesized from industry, which are mostly discussed in the environment as synthetic antibiotics, including drugs, herbicides, and agricultural feed.
Iron minerals and OM are distributed as complexes in the natural environment, and it is of great importance to study their interaction processes and environmental effects. Over the past few decades, goethite has been shown to act as an adsorbent to trap OM from soil and transport them to aquatic systems, affecting the global carbon cycle (Sollins et al., 2009; Gabriel et al., 2018). In addition, goethite is a natural catalyst for the catalytic oxidation of OM in soil or wastewater (Lu, 2000; Lai et al., 2021), which will affect the migration and transformation of pollutants in soil and aquatic environments. The interactions between goethite and OM are affected by many factors, including content and chemical composition of OM, pH, and ion species (Kleber et al., 2015). And the influence of various factors on the combination of goethite and OM can be determined through a series of batch experiments. Based on experimental data, the main interaction process like adsorption between goethite and OM can be depicted by the theoretic or empirical mathematic models (Ni et al., 2017).
The interactions between natural OM (NOM) and iron minerals have been intensively studied over the past decades. The earliest studies began in 1981, when Tipping studied the interactions between HS and aqueous iron oxide (α-FeOOH, α-Fe2O3, amorphous Fe-gel), and analyzed it by adsorption isotherms, showing that iron oxide strongly interacted with the surface negative charge of HA (Tipping, 1981). Subsequently, researchers began to consider the complex molecular dynamics of HA dispersed in soil and water environment, including aggregation and dissolution phenomenon, hydrophobicity and hydrophilicity, structural stability, complexing mechanism, etc. At the same time, they began to study the effects of iron minerals and organic matter complexes on heavy metal ions and carbon cycling in soil (Chiou et al., 2000; Vindedahl et al., 2016; Bao et al., 2021; Di Iorio et al., 2022). Some previous reviews focused on the surface properties of goethite alone (Liu et al., 2014), the role of dissolved organic matter (DOM) on the transformation of pollutants on the mineral surface (Polubesova and Chefetz, 2013), the interactions between mineral and OM as well as the immobilization of toxic elements and hazardous substances and carbon sequestration at the molecular level (Vindedahl et al., 2016; Otero-Fariña et al., 2017; Wang et al., 2017; Di Iorio et al., 2022), and the differences between the two modes of adsorption and co-precipitation of OM with goethite and the fixation and reactivation of metals by the complexes (Bao et al., 2021). Most reviews in the past paid attention to the interaction processes and mechanisms between iron and OM, but few reviewed the influence factors of the interactions, especially the factors of goethite crystallization affected by OM. Hence, this review summarized the surface properties and crystallization process of goethite changed by OM and the ability of goethite to adsorb OM as well as their influencing factors. The potential effects of complexes formed by goethite and OM on heavy metalions, emerging antibiotics, and carbon and iron cycles were carefully documented. This review is of great scientific significance for reviewing and understanding the influencing factors and environmental effects of the interactions between goethite and OM (Figure 1).
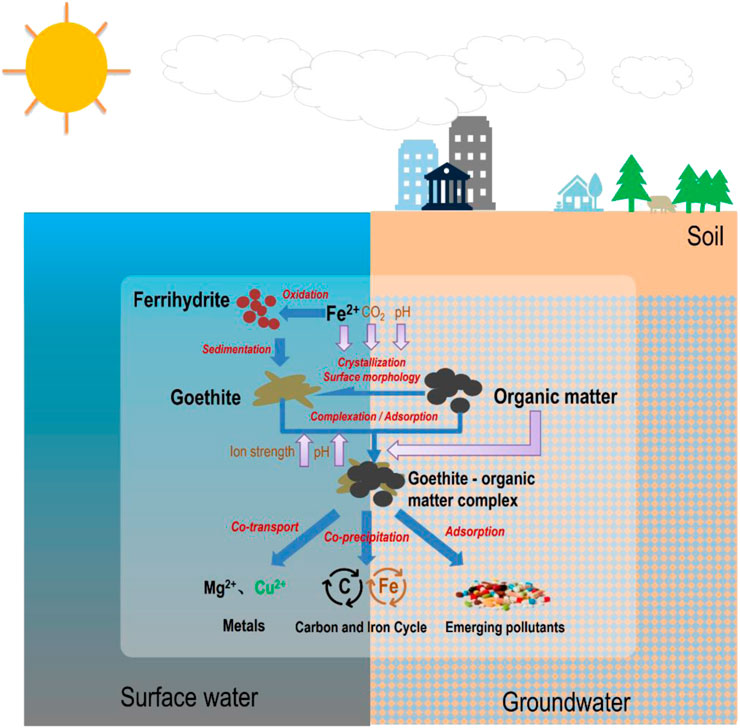
FIGURE 1. Influencing factors and environmental effects of interactions between goethite and OM. The crystallization process and surface properties of goethite are responsible for its ability to adsorb organic matter, and the interactions are controlled by external factors such as pH, CO2, ionic strength, and internal factors of their concentrations and properties. The interactions between goethite and OM have a critical environmental impact on the behavior of metal migration, adsorption of emerging contaminants (antibiotics), and carbon and iron cycles in the aquatic and soil environment.
2 Mutual effects between goethite and organic matter and their influencing factors
2.1 Effects of organic matter on goethite
2.1.1 Surface properties and crystallization of goethite affected by organic matter
Generally, goethite formed by natural differentiation has poor crystallization and is rich in defects and impurities, which make the good surface activity of goethite. Most goethite crystals are needle-like or columnar, formed by FeO3(OH)3 octahedrons connected into octahedral pairs with common edges, and grow as double chains along the C-axis. Each Fe atom in goethite is coordinated with six O atoms around it, and each Fe-O bond has a 0.5 positive charge. Each O atom coordinates with three Fe atoms to form the Fe3O0.5 coordination group. The two Fe3O0.5 coordination sites above and below the channel are connected by hydrogen bonds, and H+ neutralized the charge carried by them, so the crystal is electrically neutral. The structure of goethite is shown in Figure 2. At the liquid-solid interface, acidic groups in NOM, including carboxylic acid and phenolics, can combine with a hydroxyl group on the surface of solid goethite, thus forming the organic-mineral complex. Goethite combined with OM can change its surface properties. Otero-Fariña et al. found that the isoelectric point (IEP) shifted to lower pH values with increasing carbon content on the surface of goethite with HA coating compared to pure goethite (Otero-Fariña et al., 2017). The presence of NOM caused the presence of negatively charged groups on the surface of goethite and increased the negative potential near the liquid/solid interface (Saito et al., 2003). Electrostatic interaction between the HA and goethite would result in the organic molecules occupying the surface sites of the goethite, leading to a decrease in the surface area of goethite (Mikutta et al., 2006). Amini et al. (2020) and Antelo et al. (2007) also came to the similar conclusion: the presence of HA near the surface of goethite introduces carboxyl and phenolic groups and increased the negative potential at the interface, also affects the porosity of goethite. Kaiser et al. (2007) demonstrated that the adsorption of OM on minerals significant decreased N2 accessible micro- and mesopore volume of minerals, and the strongest reduction in microporous volume on the surface of goethite occurred when the OM content was low, and the variation in microporous volume gradually plateaued with OM. The stronger response of micropores to increased loading of adsorbed OM compared to mesopores suggested that OM was preferentially adsorbed in the micropores, but more likely at the openings of the micropores, thereby clogging and making N2 inaccessible.
The crystallization of goethite is a complex mineralizing process, and the mechanism of crystal nucleation and growth is not fully disclosed, Wang et al. examined the nucleation and growth processes of the crystalline grains by the transmission electron micrographs during the reaction (Wang et al., 1998). Experiments showed that nucleation of α-FeOOH crystals occurred both on the surface of Fe (OH)2 grains and in the solution, depending on the local concentration of α-FeOOH. According to experiments, the early oxidation rate played an important role in the crystal growth process. Most of the crystal nuclei were formed during the initial oxidation phase. With the increase of oxidation rate, the size and aspect ratio of the final product decreased. Therefore, when the early oxidation rate was relatively faster, many small seed crystals could be formed, whereas a large seed crystal would be formed when the early oxidation rate was relatively slower. In addition, temperature and synthesis conditions may affect the crystallinity of goethite. The presence of OM also affected the crystallization of goethite (Philippe et al., 1985; Prélot et al., 2003). Kodama and Schnitzer (1977) investigated the effect of many aliphatic carboxylic acids and hydroxy carboxylic acids with low molecular weight on the crystallization of hematite or gothite in newly precipitated hydrated ferrites. The results showed that citric, tartaric, and malic acids inhibited crystallization, but formic, acetic, oxalic, and malonic acids did not. The common feature of these inhibitory acids is that they are hydroxy-carboxylic acid (polyacid base) compounds. Xing et al. (2020) showed that HA could induce the transformation of minerals from siderite to goethite in the natural environment. The transition from siderite to goethite in the presence of HA could be divided into three stages. Initially, the released ferrous ions were chelated by HA to form Fe(II)-HA complexes. Then, Fe(II) was oxidized and crystallized to form ferrihydrite. Finally, ferrihydrite in the form of monocrystal rearranged on the surface of goethite crystal and fused with the crystal structure of goethite. Bond breaking between acid and Fe atoms occurred during crystal growth, followed by the expulsion of HA, which could then chelate with fresh Fe(II). Shi et al. (2021) further analyzed the redox properties of persistent free radicals (PFR) and reactive oxygen species (ROS) of HS with different molecular weights and their role in the reduction of goethite, which might change the crystal properties. During the reaction with goethite, PFR and phenolic hydroxyl groups were the main electron donors for the reduction of goethite by HA, while ROS inhibited the reduction of goethite. HA with higher molecular weight had a higher reductive effect on goethite.
2.1.2 Influencing factors
The OM has an inhibitory effect on the crystallization of amorphous ferric hydroxide. The strong adsorption of organic anions by amorphous ferric hydroxide may prevent their rapid crystallization (Schwertmann, 1966). This section summarizes three critical factors that influence the crystallization of gothite by OM, including pH, CO2, and Fe species.
2.1.2.1 pH
As early as 1966, Schwertmann (1966) concluded that the alkaline-extracted OM might have an inhibitory effect on the crystallization of amorphous ferric hydroxide. As widely acceptable knowledge, the hydrolysis of Fe3+ in the formation process of goethite would lead to a decrease in pH, which facilitate the transformation of iron minerals into goethite (Bruyn and De Bruyn, 1976; Yapp, 1991). Both the experimental and modelling research indicated that crystallization of goethite from amorphous ferric hydroxide preferentially occurs at pH ≤ 5 (Schwertmann and Murad, 1983; Yapp, 2001). Thus, we conclude that lower pH might decrease the solubility of OM, which weakens the inhibitory effect of crystallization of goethite by OM.
2.1.2.2 CO2
In general, CO2 affects the crystallization process of goethite mainly through the formation of a complex between carbonate and goethite. Due to the presence of CO2, there is a small amount of iron carbonate [Fe(CO3)OH] on the surface of goethite (Yapp, 1987). It is generally believed that CO2 has three sources, including CO2 from the atmosphere, oxidation of OM, and dissolution of calcite (Yapp, 2001; Tabor N et al., 2004; Fritz and Yapp, 2018). The sources might combine CO2 into goethite crystal. Presumably, the carbonates form an inner sphere surface complex at bonding sites within the structural grooves along the c-crystal axis and are subsequently trapped during particle growth along the a and b crystal axes. Thus, The C-doped goethite indirectly affected by oxidation of OM indicated that oxidation products of OM can also control the properties and purities of goethite crystal.
2.1.2.3 Iron species
At the redox interface, Fe(II) is oxidized to Fe(III), and Fe(III) can precipitate to form hydrous iron ore, which has low crystallinity and is unstable and can continue to be converted to goethite or hematite. During the process, The rate of electron transfer from HS to Fe(III) was strongly influenced by the properties of Fe(III) compounds (Bauer and Kappler, 2009). The reduced HS can reduce not only dissolved or poorly crystalline Fe(III) compounds but also highly crystalline Fe(III) minerals, but the reduction rate is slower for highly crystalline minerals like goethite. With respect to the oxidation process of Fe(II) to Fe(III), only 10% of ferrihydrite can be converted to goethite when there is no other divalent cation or other NOM (Lu et al., 2020). As summarized in Section 2.1.1, HA can chelate with the released ferrous ions to form Fe(II)-HA complexes, which accelerate the oxidation of Fe(II) by DOM in water and form monocrystals (ferrihydrite), which in turn induce the formation of goethite (Xing et al., 2020). Thus, the electron exchange process between aqueous Fe(II) and structural Fe(III), which underlies the formation of goethite crystals, is controlled by OM.
2.2 Effects of goethite on organic matter
2.2.1 Molecular properties of organic matter affected by goethite
The goethite can also affect the molecular properties of OM, including the specific ultraviolet absorbance (SUVA), molecular weight (MW), and ratio of absorbance at 250 and 365 nm (E2/E3), where SUVA is positively correlated with the aromaticity of NOM. Qin et al. (2015) investigated the fractionation of HS after adsorption onto goethite by column and batch experiments, and the results showed that the MW of HA decreased with increasing reaction time and was smaller than its initial value. E2/E3 and SUVA did not change significantly with reaction time and were smaller and higher than their initial values, respectively. Therefore, it can be concluded that HA fractions with higher aromaticity were preferentially adsorbed on the goethite. A similar study by Xing and Xing (2008) showed that E2/E3 of HA decreased with the adsorption process, indicating that goethite preferentially adsorbs more aromatic, hydrophobic, and lower-MW fractions of HA when it interacts with HA. However, in studies by Xu et al. (2022) and Wang et al. (2019), the results indicated that goethite preferentially adsorbs the higher MW fraction of HA, which was inconsistency with Kang et al. The inconsistent conclusions reached may be due to a lack of comprehensive understanding of the preferential adsorption of NOM by goethite. Thus, more researches of molecular properties of OM affected by goethite are needed in the future.
2.2.2 Influencing factors
2.2.2.1 pH
The study of Wang et al. (2019) showed that SUVA was more susceptible to pH at low concentrations of OM. Although E2/E3 of HA is almost independent of pH, E4/E6 is more influenced by pH. And MW of HA after adsorption by goethite depended on pH. Safiur Rahman et al. (2013) explained the role of pH in the effect of goethite adsorption on the MW distribution of DOM. In detail, in the solution where needle ferrite was present together with DOM, the weight-average of DOM molecular weights fraction (Da) remaining in the solution decreased with decreasing pH, and the decrease in the weight-average of DOM molecular weight fraction was more pronounced above pH 6.5. This may be due to the protonation of the surface hydroxyl groups of Fe in acidic media (pH < 6.5), which makes the surface hydroxyl groups more exchangeable and therefore contributes to the pH-dependent adsorption of the higher molecular weight fraction of organic ligands.
2.2.2.2 Ionic strength
The surface properties of OM after adsorption on goethite are influenced by the ionic strength in the solution. Gerzabek et al. (2022) proposed a supramolecular view that the screening of HA charge by electrolyte ions or the supramolecular nature of HA promoted different degrees of fractionation or aggregation of HA fractions. It then caused fractionation of HA molecules, and the average molar mass of adsorbed HA increased with ionic strength when the fractionation is fixed. Xu et al. (2022) calculated the effect of ionic strength on fractionation by modeling the decreasing negative value of the Donnan potential in solution as the ionic strength increased, which would favor the association process.
3 Interactions between goethite and organic matter
3.1 Potential mechanisms
The potential interaction mechanisms of OM by goethite can be divided into six categories, including anion exchange (electrostatic interaction), ligand exchange, hydrophobic interaction, entropy effect, van der Waals force, and cation bridging as shown in Figure 3 and Table 1 (Gu et al., 1994): (1) electrostatic interaction is controlled by coulomb force, which is resulted from the negatively charged OM and the positively charged goethite at slightly acidic or acidic pH (Guhra et al., 2019). (2) Ligand exchange mainly involves the formation of complexes by coordination reaction between acid functional groups of OM, including carboxyl and phenolic hydroxyl groups and hydroxyl groups on goethite (Albuquerque et al., 2022). (3) Hydrophobic interaction is caused by some non-polar components in OM, which exhibit hydrophobicity, leading to their alienation from water molecules and their adsorption on the surface of goethite (Chen et al., 2019). (4) Entropy effect means that the complexation or adsorption between OM and goethite is driven by physical adsorption, which is conducive to entropy change (Ghosh et al., 2017). (5) The van der Waals force’s contribution to the adsorption is generally not strong, but only shows a relatively important role in a strong ionic strength system. In the case of strong ionic strength, OM is compressed, causing thinning of the electric double layer, and decreasing of polymer with electrostatic repulsion. Thus, OM is easier to affiliate the surface of goethite. The performance of hydrogen bonding is also very important (Olsson et al., 2011). (6) Cationic bridging refers to the formation of a bridge between the negatively charged goethite and the anionic or polar groups of OM by hypervalent cations (Olu-Owolabi et al., 2010).
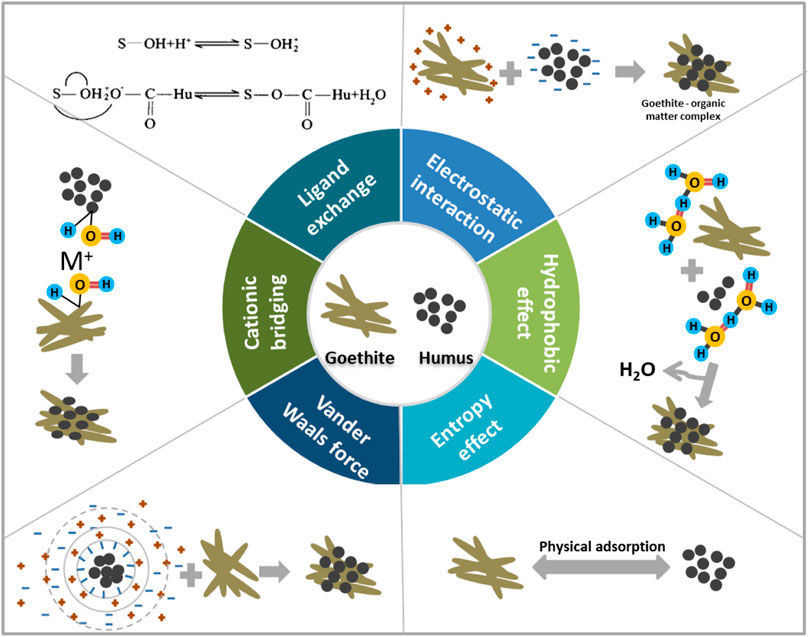
FIGURE 3. Potential interaction mechanisms of OM with goethite. They mainly include anion exchange (electrostatic interaction), ligand exchange, hydrophobic interaction, entropy effect, van der Waals force, and cation bridging.
3.2 Major influencing factors
The interactions of OM with goethite usually depends on the pH and ionic strength the of solution as well the as compositions and structure of OM, which have been carefully documented through various experiments and models (Table 1).
3.2.1 pH
In general, when the pH is slightly lower than PZC, it is conducive to the increase of the adsorption amount of organic matter by goethite. This is because the surface of goethite exhibits a positive surface charge at low pH, and most OM contains at least one acid group. The adsorption between goethite and OM mainly depends on electrostatic interaction. For example, there was no interaction between the goethite surface and organic anions when the pH was higher than the PZC of goethite, and the adsorption increased gradually with the decrease of pH. Consistent conclusions were also drawn in the adsorption of organic acids (Evanko and Dzombak, 1998), glucose (Olsson et al., 2011), diclofenac (Zhao et al., 2017), HA (Kang and Xing, 2007; Saito et al., 2003), and FA (Filius et al., 2000). In addition, the inner sphere OM—goethite complexes are easier to form at lower pH, whereas the relative solubility of outer sphere OM—goethite complexes increases with pH (Persson and Axe, 2005). It is explained that OM adsorption on goethite is mainly by ligand exchange under acid conditions, and the adsorption effect is relatively stronger. Under alkaline pH conditions adsorption is mainly controlled by hydrophobic bond and van der Waals force, and the adsorption effect is relatively weaker (Jiang, 2011).
3.2.2 Ionic strength
OM and iron oxide might exist in the form of colloid particles in the soil, sediment, and water. The electric double-layer diffuse (EDLD) model of the colloid has been widely accepted and approved. The EDLD is described as follows: when a charged colloidal particle is dispersed in an electrolyte solution, opposite charge ions in the solution will approach the surface due to electrostatic attraction, and the thermal movement of ions causes them to diffuse away from the surface. Ions with the same charge in solution show the opposite trend of movement. When the electrostatic attraction is balanced with the thermal motion, a diffusion layer mainly composed of counter-charged ions is formed on the particle surface. The increase of ionic strength will reduce the thickness of the diffusion double electric layer on the colloidal surface (Senesi and Loffredo, 1998). Therefore, some background ions in the soil and water environment will affect the interaction process (adsorption) of OM with gothite. The strong influencing ions on adsorption include Ca2+, K+, Na+, Cl−, and NO3−. Ramos found that specific metal cations would change the dispersion of organic ligands (Ramos, 1996). Ca2+ is more conducive to flocculation of OM than K+ because the chemisorption of polyvalent cations or organic ligands change the surface charge and reduce the amount of surface charge. In a study of the influence of ionic strength on the adsorption process of monocarboxylate by goethite (Norén and Persson, 2007), the maximum adsorption of acetate, benzoate, and cyclohexane carboxylate decreased with the background electrolyte (NaCl). This is because the surface complex is mainly stabilized by electrostatic force, which is affected by NaCl. The ionic strength could also change the conformation of HA, which causes the adsorption of HA on goethite to decrease with the ionic strength (Antelo et al., 2007). In addition, different adsorption responses of HA and FA by goethite to the ionic strength are also observed because of their compositions and structure (Weng et al., 2007), which will be discussed in the following section.
3.2.3 Compositions and structure of organic matter
The compositions and structure of OM also affect its interaction with goethite as shown in Table 1. According to the adsorption of simple organic acid by goethite (Filius et al., 1997), most of them formed inner sphere complex through complexation between carboxyl function in OM and goethite except citric acid, which interacted with goethite in the form of a ternary complex at the low pH. With respect to organic acids containing benzene rings, the position and number of phenolic substituents and carboxyl substituents would affect the adsorption (Evanko and Dzombak, 1998). With respect to the adsorption of monocarboxylic acid and monophenol by goethite, the hydrogen bond was the main interaction (Yu et al., 2019). The HS fraction with higher molecular weight and lower aromatic degree would preferentially be adsorbed on the surface of goethite (Xing and Xing, 2008; Tunega et al., 2009; Qin et al., 2015). It is explained that surface charge and aggregation of goethite were affected by different OMs (Dultz et al., 2018). When the surface charge of goethite changed from positive to negative, the continuous adsorption of OM on goethite caused a sharp decline of surface charge to more negative. The acidity effect of OM on the surface charge is the main factor controlling the aggregation of goethite as well as the particle concentration of goethite in the suspension.
3.3 Mathematic models
Based on the experimental research, researchers established a series of models to further depict the interaction mechanism between goethite and OM. The surface complexation model is a model to calculate the interfacial adsorption reaction, which is based on the complexation between the reaction site on the adsorbent surface and the adsorbent and considers the effect of charge change. The commonly used advanced surface complexation models include the charge distribution multi-site complexation model (CD-MUSIC model), ligand charge distribution model (LCD model), and natural organic matter charge distribution model (NOM-CD model) (Figure 4). Those surface models are based on the Langmuir model, which treats the adsorbate as a single homogeneous component bound to a surface with sites, ignoring chemical heterogeneity and polydispersity (Wang and Guo, 2020; Xu et al., 2022).
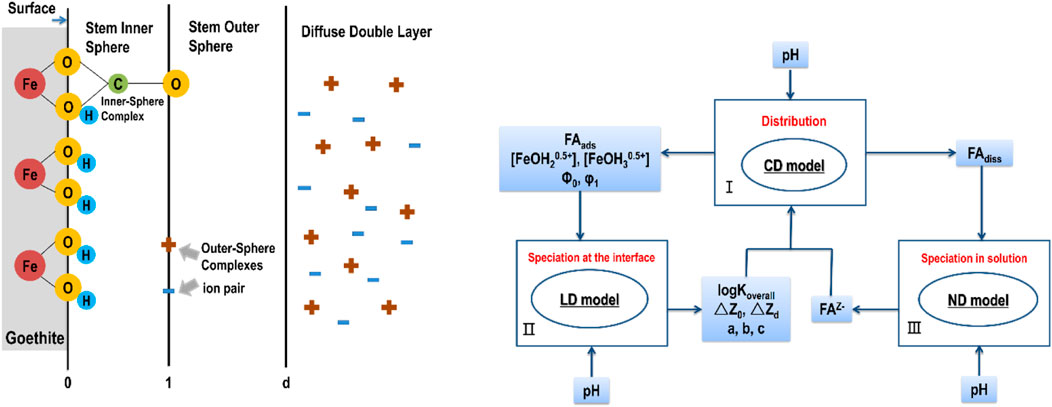
FIGURE 4. Conceptual models of the LCD. (I) The CD-MUSIC model calculates the distribution of organic molecules in solid and solution phases. (II) The LD model calculates the average adsorption mode of adsorbed organic molecules, which determines the input parameters of the CD-MUSIC model. (III) The amount of dissolved FA is converted to the reference material FAz−, which is used as the input of the CD-MUSIC model. The affinity constants (logKoverall), charge distributions (δZ0, δZ1), stoichiometric coefficients (a, b, c), and FA activity in solution (FAz−) were input parameters of the CD-MUSIC model. LogKoverall, δZ0, δZD and the values of a, b, c were also the output of the morphological calculations of the adsorbed FA molecules. The figure is modified according to (Mangold, 2013) and (Filius et al., 2003).
3.3.1 Langmuir model
The Langmuir model is a monolayer adsorption theory proposed by Langmuir in 1916 (Langmuir, 1917), which is based on the following four assumptions: (1) there are adsorption sites exist on the adsorbent surface, and the adsorbent molecules can only be adsorbed on the sites in a single layer; (2) the adsorption sites are homogeneous in the thermodynamic and kinetic sense (uniform surface properties of the adsorbent) and the heat of adsorption is independent of the surface coverage; (3) there are no interactions between adsorbed molecules and no lateral interactions; (4) the adsorption-desorption process is in a kinetic equilibrium (Wang and Guo, 2020). Although Langmuir model describes the equilibrium conditions for monolayer homogeneous adsorption, for most of the adsorption processes the adsorbent material is homogeneous on a macroscopic scale or in the presence of continuous stirring, so the model is also suitable for describing the adsorption process of goethite with OM.
As mentioned before, there are six main mechanisms of interaction between goethite and OM, and Langmuir adsorption model can be used to explain which mechanism plays the main role. The study by Kaiser, (2003) showed that the adsorption isotherms of OM on goethite conformed to the Langmuir equation, and the hydrophobic component was more strongly adsorbed than the hydrophilic component. Moreover, the main mechanism of interaction between OM and goethite under depended on pH conditions, i.e., the ligand exchange was mainly dominated under acidic conditions, whereas the synergistic effect of water-repellent bonds and van der Waals forces dominated under alkaline conditions. Zhou et al. (2001) investigated the interaction of goethite with FA using Langmuir’s fitting curve and found that more high-density reactive sites goethite interacted with OM through ligand exchange and electrostatic gravitational force between goethite and NOM was weak at low pH. The study by Shin et al. (1999) showed that the interaction mechanism between small molecule OM and goethite was mainly ligand exchange and electrostatic interaction by fitting the Langmuir model, whereas the interaction mechanism between OM with larger MW and goethite was mainly hydrophobic, mainly because OM with larger MW had less acidic functional groups and higher aliphatic content and was less aromatic than OM with smaller MW (Liu et al., 2010).
3.3.2 Complexation model
The CD-MUSIC model is compose of the charge distribution (CD) model and the multi-site complexation (MUSIC) model, based on the crystalline morphology and surface chemistry of minerals, with experimental data on adsorption as the main part, combined with information on the surface structure obtained in spectroscopy (Hiemstra and Riemsdijk, 1996). The CD model is a description of the inner circle complex formed by adsorbed ions on the surface of metal oxides. The MUSIC model is mainly used to describe the charge properties of metal oxides, which assumes that the metal oxides are electrically neutral inside and charged on the surface. The surface sites containing O atoms can be classified as single coordination groups (FeOH0.5−), double coordination groups (Fe2OH0), and triple coordination groups (Fe3O0.5−) according to the number of coordination Fe atoms around the O atoms of goethite. When the MUSIC model is used to simulate the predicted surface charge properties of goethite, the double coordination group (Fe2OH0) is unresponsive to protons at the pH range of 2–12 and is regarded as an inert group, so the charging reactions of goethite are described by the protonation reactions of single coordination groups (FeOH0.5−) and triple coordination groups (Fe3O0.5−) (Hiemstra and Riemsdijk, 1996; Boily et al., 2001).
The CD-MUSIC model can well fit the adsorption of various groups on the oxide surface based on only a small amount of experimental data. Researchers often use this model to model the adsorption process between goethite and OM. For example, Filius et al. (1997) used this model to determine the adsorption of lactate, oxalate, malonate, phthalate, and citrate by goethite as a function of concentration, pH, and ionic strength. Iglesias et al. (2010) used the CD-MUSIC model to study the adsorption of pesticide 4-chloro-2-methylphenoxyacetic acid (MCPA) on the surface of goethite and humic acid-coated goethite, and concluded that the adsorption of MCPA depended on the concentration of electrolyte, and the adsorption amount increased with the decrease of ionic strength. Xiong et al. (2015) used the CD-MUSIC model to depict the effects of soil FA and HA on the binding of Pb to goethite. The results showed that the adsorption of HS on goethite strongly depended on pH value and was promoted by Pb binding. Qin et al. used this model to simulate the effect of NOM with different properties on the adsorption of antibiotics (levofloxacin, LEV) to minerals. The simulation results showed that aromatic moieties of OM played a critical role in Pb absorption by NOM-coated goethite (Qin et al., 2018).
3.3.3 Ligand charge distribution model
In 2001, Filius et al. (2001) proposed an advanced modeling approach for OM-mineral interactions, which was called the ligand charge distribution (LCD) model. The LCD model makes up drawback that the information produced by infrared spectroscopy is about the local environment of individual active groups of organic acids, rather than the adsorption mode of the whole organic molecules. In the LCD model, the non-ideal competitive adsorption (NICA) model is used to describe the chemical binding of ions and surface sites to reactive ligands on HS in solution and adsorbed phases. The NICA model calculates the statistical distribution of humic ligands among the various possible chemical states that the ligands can adopt (i.e., protonation, deprotonation, complexation with surface sites, etc.). The CD-MUSIC model was used to calculate the binding and electrostatic effects of small ions to oxides and the adsorption of HS on the surface of goethite. As mentioned above, goethite surface has many types of sites. Assuming that the basic charge is caused by the protonation and deprotonation of oxygen on the single (−FeOH0.5−) and tri (−Fe3O0.5−) coordinated surfaces, the following reaction equations can be obtained as follows (Boily et al., 2001):
LCD models is used to describe the adsorption of various carboxylic acids by goethite. The original LCD model is only limited to carboxylic acid groups. Because FA contains both carboxylic and hydroxyl groups, Filius et al. extended the original LCD model to consider the complete form of the hydroxyl groups of the adsorbed FA molecules (Filius et al., 2003). The LCD model can simultaneously describe the concentration, pH, and salt dependence of adsorption with at least three adjustable parameters. In addition, the model can accurately predict proton co-adsorption to adapt to a wider range of conditions. The previous LCD model only tested the pH and salt concentration dependence of ferric oxide adsorption by simple organic acids and FA and did not test the FA-goethite system in the presence of multivalent cations. Therefore, Weng et al. (2005) used the LCD model in the ternary system (Ca-FA-goethite), and the model calculation showed that the interaction between Ca and FA on the surface of goethite was mainly due to electrostatic effect. In previous versions of the LCD, particle adsorption was calculated using the bulk reaction form. In this form, the concentration of completely dissociated HA molecules was required, but the calculation was incorrect in the previous LCD. In order to compensate for this deficiency, Weng et al. introduced ADsorption and AdaPTation (ADAPT) model into the LCD model (Weng et al., 2006). This new module replaced the integral reaction form of the previous LCD and defined the equilibrium conditions for the mass phase distribution. The results showed that the LCD model could fully describe the effects of pH, ionic strength, and load on the adsorption of FA, and the chemical complexation between FA and goethite was the main driving force of adsorption, whereas the electrostatic repulsion between particles and surface was the main limiting factor for further adsorption. Weng et al. (2007) continued to use the improved LCD model to study the adsorption of HA on goethite. The calculation results of the model showed that, compared with FA, the adsorption of HA was stronger and more dependent on pH value and ionic strength. The number of reactive groups per HA particle was greater than that FA particles, leading to the stronger adsorption of HA.
3.3.4 Natural organic matter charge distribution model
In the above discussion, the interaction between FA or HA and goethite can be described by LCD model. In principle, the model can relate water molecule concentration to NOM surface loading if the molecular conformation is known. But in soil, it is difficult to relate the adsorbed fraction of interacting organic molecules to the solution concentration of operationally defined NOM molecules. Therefore, it has been suggested to use virtual surface components in situ surface spectroscopy and complexation models (SCM) (Hiemstra et al., 2010). In the LCD model, NOM adsorbed on metal oxides is mainly attributed to the interaction between the carboxyl and phenolic hydroxyl groups and metal oxides, whereas in the NOM-CD model, NOM is regarded as a virtual group HNOM−1. This virtual group can form three surface complexes with the single coordination group (FeOH0.5−) on the surface of goethite, namely, the inner ring complex, the outer ring complex, and the protonated inner ring complex. Hiemstra et al. (2013) used this model to study the interaction between natural or pyrogenic HA and phosphate on the surface of goethite, and the results showed that pH-dependent oxyanion competition of the FA-goethite can be described. Otero-Fariña et al. (2017) studied the effect of NOM on the binding of arsenate and copper on goethite. The results showed that the increase of carbon content would lead to the reduction of arsenate adsorption and the enhancement of Cu adsorption on organic-mineral composite. Calculations using the NOM-CD model make it easy to predict the behavior of these ternary systems and are in good agreement with the spectral information available for copper and arsenate binding. Deng et al. (2019) studied the main characteristics that response to interaction between NOM and phosphorus/arsenic at the goethite-water interface, and the results showed that NOM affected the mobility of phosphate and arsenate at acidic pH, whereas NOM had a relatively strong influence on the mobility of arsenate at neutral pH. The competitive interaction between NOM and oxyanion at the gothite—water interface was mainly determined by the differences of carboxyl group site density, carboxyl protonation constant, and spatial distribution of adsorbed NOM. The spatial distribution of NOM adsorbed on goethite surface was related to the particle size, surface load, aromaticity, and site density of carboxyl groups in NOM. NOM-CD modeling is relatively simple when it comes to simulate the interactions between oxyanion and various NOM at the goethite-water interface, whereas LCD modeling can better differentiate the effects of different factors and mechanisms.
4 Potential environmental effects of goethite—organic matter interaction
Goethite and OM are important components in soil, sediment, and water, and they participate many environmental processes (Figure 5). Goethite and OM are combined in two forms: adsorption and co-precipitation states. In the adsorption state, HS and other OM are wrapped on the surface of goethite. Due to the variable charge of goethite, negatively charged OM is easily adsorbed onto the mineral surface to form a complex. In the co-precipitation state, humic molecules are involved in the formation process of goethite, which can selectively retain the aromatics (Karlsson and Persson, 2012; Arroyave et al., 2016). The interaction between goethite and organic matter will affect the adsorption of metal ions, thus affecting their migration and bioavailability in water and soil, whereas the adsorption of OM by goethite will also affect the degradation of pollutants in the environment (Stewart et al., 2013; Cheng et al., 2020), and their interaction will also affect the global carbon and iron cycles as well as bury of carbon and iron (Stewart et al., 2013; Sodano et al., 2017).
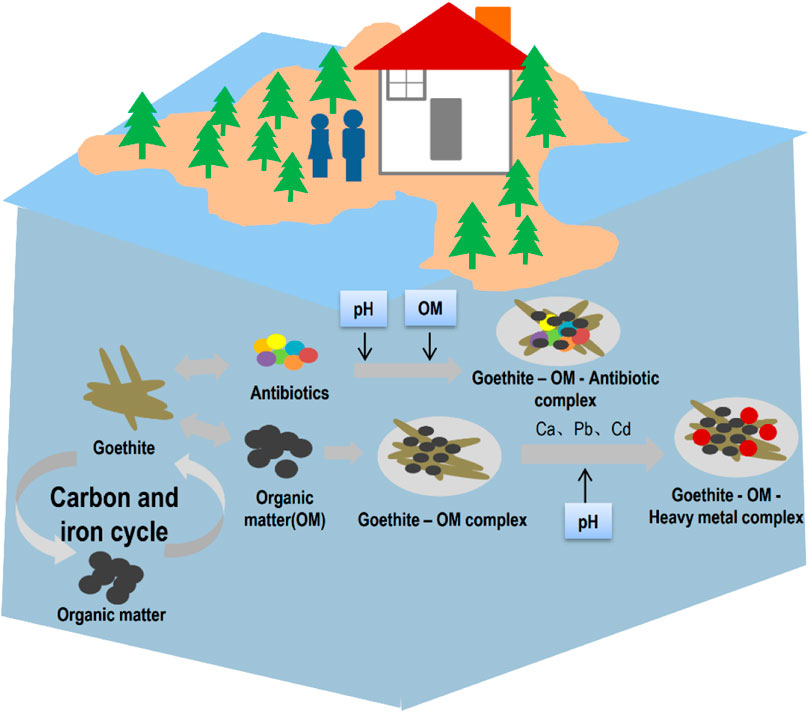
FIGURE 5. Potential environmental effects of goethite-OM complex. The complex of goethite and OM has a variety of potential environmental effects, including complexation with metal ions to immobilize metal pollutants in soil and binding to emerging contaminants (antibiotics) to change their mobility. The combination of goethite and organic matter can change the carbon and iron cycles.
4.1 Effects of goethite-organic matter complexes on migration and transformation of heavy metal
The functional groups in OM, especially carboxyl and phenolic hydroxyl groups, have strong interactions with the surface groups of metal oxides, which can change the migration and transformation behaviors of ions on metal oxides. As shown in Table 2, the binding of HA to goethite alters the charge and adsorption capacity of mineral particles. Compared with the system without HA, the binding capacity of goethite-HA complex to Ca2+ (Weng et al., 2005), Pb2+ (Orsetti et al., 2006), Cd2+ (Liu et al., 2019), Cu2+ (Saito et al., 2005; Jonsson et al., 2006; Weng et al., 2008) and Hg2+ (Bäckström et al., 2003) ions is increased, but the adsorption process is affected by pH, which has different effects on the metal ions. Moreover, the presence of OM will affect the morphology, fluidity, and adsorption behavior of metal ions, thus affecting the migration and transformation of heavy metal ions between soil and water. For example, in view of the effect of FA on the adsorption/desorption of Pu4+ on goethite under two conditions, researchers have proposed two possible mechanisms. One mechanism is that the formation of metal-OM complex may directly change the adsorption behavior of metal ions on the mineral surface (Buda et al., 2008). The other mechanism is that OM in solution controls the redox reactions of metals and thus indirectly affects the adsorption characteristics of metal ions (Banik et al., 2007). Further studies by Tinnacher et al. (2015) showed that the presence of FA could control the oxidation state of Pu in the 4-valent solution and make it more mobile. Moreover, Pu-FA-goethite ternary complex could enhance the transport of colloid-promoted Pu. Guo et al. (2022) found that the presence of FA significantly increased the adsorption of Pb2+ by goethite, and the free Pb2+ in goethite liquid phase decreased with the FA concentration. Three possibilities were given, Firstly, the adsorption of FA reduced the positive surface charge of goethite and increased its negative charge, so the adsorption of Pb2+ was enhanced due to the enhanced electrostatic attraction between Fe oxide and Pb2+. Secondly, FA with many functional groups on the surface was adsorbed on the Fe/Al oxide surface, which provided more binding sites for Pb2+ adsorption. Finally, FA complexed Pb2+ to form negatively charged groups, which were linked to the goethite surface by ion bridging, further enriching metal cations on the Fe oxide surface. Qu et al. (2022) also observed that the presence of HA significantly changed the morphology of Cd and Fe during the transformation of iron (hydrogen) oxides. With the increase of HA, small and irregular particles were formed, indicating the stabilizing effect of HA on iron (hydrogen) oxide nanoparticles. Owing to the rich functional groups, HA promoted the surface adsorption and fixation of Cd ions onto mineral surface from the suspension.
4.2 Coupling effects of goethite and organic matter on migration of emerging organic pollutants
With the development of economy and the continuous improvement of people’s living standards, pharmaceutical and personal care products (PPCPs) are widely used in people’s daily life, agricultural activities, and animal husbandry. These emerging organic pollutants are discharged into the environment, causing potential damage to the ecological environment and human health. Antibiotics are the most representative PPCPs, which can be divided into five groups, including sulfonamides, tetracyclines, quinolones, macrolides, and β-lacamides, according to the different functional groups and properties. At present, the adsorption of quinolones and tetracyclines on goethite is of great concern (Table 3). In the study of the effects of HA on the adsorption of levofloxacin (LEV) by goethite (Qin et al., 2016), the presence of HA increased the adsorption of LEV by goethite, because HA improved the adsorption affinity and maximum binding capacity. The hydrogen bonding and cation complexation may not be important in the complexation of LEV with HA. But, hydrophobic interactions and π-π interactions are considered to be the main mechanisms for the complexation of LEV with OM (Qin et al., 2018). In another study, the contribution of HA to ofloxacin adsorption was more than 80% of the total adsorption amount (Peng et al., 2015), which was also observed for the effect of HA on the adsorption of ciprofloxacin and tetracycline on goethite (Zhao et al., 2011; Tan et al., 2015). In summary, the interaction mechanism of antibiotic adsorption by the coupling effects of goethite and organic matter is mainly the competition between OM and antibiotic for the adsorption potential on goethite and the electrostatic interaction between goethite and antibiotic.
4.3 Effects of goethite-organic matter interaction on carbon and iron cycles
The interaction between OM and minerals is one of the critical processes of global bury of carbon. OM can promote the dissolution of iron oxides to increase the utilization rate of iron, and at the same time, iron oxides can protect OM from decomposition through adsorption and co-precipitation processes (Kleber et al., 2021). The interaction between iron oxide and OM includes adsorption and co-precipitation. It is widely recognized that iron oxide retains carbon through surface adsorption, but the carbon retention of OM through co-precipitation was still relatively unknown. Due to the fluctuation of pH value, oxygen content, or redox potential of the water system, soluble Fe (II) may be chemically oxidized to an insoluble Fe (III) precipitate. If OM exists in Fe(II) solution at the same time, Fe(III) and OM will co-precipitate in an abiotic way (Mikutta et al., 2014). Sodano et al. (2017) proved that co-precipitate was also an important bury process of carbon, especially in hydrogenic soil. The experimental results showed that in the presence of OM derived from straw, co-precipitation during Fe2+ oxidation and hydrolysis resulted in a higher retention of adsorbed carbon on the surface, where a large amount of OM was contained in the highly aggregated Fe-OM association. Yu et al. (2021) further demonstrated that co-precipitated HA played an important role in accelerating Fe(III)/Fe(II) cycle and degradation efficiency. Luo et al. (2022) found that co-precipitation of HA and phosphate with Fe(III) was enhanced as the pH decreased or the initial Fe(III) concentration increased. The co-precipitation of DOM and Fe (III) is ubiquitous at the redox interface. Recent studies have noted that the visible light response of semiconductor minerals and the resulting photocatalytic capacity have been proved to be the main participants of the earth surface geochemical processes (Lu et al., 2019). Iron oxides are semiconductors with good response to visible light. Under visible light irradiation, the cumulative concentration of Fe2+ (aq) at the DOM-goethite interface was significantly increased, and DOM was also oxidized (Yang et al., 2022). ROS is generated at the DOM-goethite interface under visible light irradiation. Fe2+ (aq) produced in the photocatalytic process of goethite can be re-oxidized or precipitated with inorganic carbon, resulting in the formation of secondary Fe minerals on the surface of goethite. The complex interaction among OM, iron minerals, and ROS plays an important role in the geochemical behavior of iron and carbon cycling in soil (Jickells et al., 2005; Song et al., 2022), which would affect the climate change.
5 Summary and prospect
This paper mainly reviews the interaction between goethite and OM. Firstly, the mutual effects between goethite and OM, including the effect of OM on the surface properties and crystallization of goethite, molecular changes of OM by goethite, as well as their influencing factors. Secondly, OM—goethite interactions mechanisms, influencing factors, and mathematic models were summarized. This paper introduces four theoretic and empiric models, including Langmuir model, CD-MUSIC model, LCD model, and NOM-CD model. Finally, this review mainly introduces the effects of the complex of goethite and OM, especially HS, on the migration and transformation of heavy metal ions and antibiotic OM as well as carbon and iron cycles caused by goethite—OM interactions.
From this review, we found that many researchers have conducted a series of studies on the interactions between goethite and OM and their environmental effects, which provides a reference for exploring how OM affects the reactivity of goethite and the environmental behaviours of goethite - OM complex. However, the molecular pathway that NOM influences the crystallization potential and reactivity of Fe need more in-depth studies. In addition, the redox condition, compositions of OM in the solid-liquid interface from surface water, vadose zone, and aquifer are different. In the surface water or soil, fresh OM and abundant oxygen facilitate producing ferrihydrite with poor crystallization from iron oxide. Whereas, refractory OM and hypoxia facilitate formation of goethite in deep soil or aquifer. However, goethite also appears in the surface environment. To explain this phenomenon, it is necessary to conduct future mechanism studies of formation, transformation, effects of goethite—OM complexes (particulate OM or carbon-contained minerals) in different aqutic environmental systems at a molecular level.
Data availability statement
The original contributions presented in the study are included in the article/supplementary material, further inquiries can be directed to the corresponding author.
Author contributions
Conceptualization, WH; writing–original draft preparation, SY; writing–review and editing, WH, BY, XCh, XCa, and SY; supervision, WH; funding acquisition, WH. All authors agree to be accountable for the content of the work.
Funding
Funding for this study was supported by the National Natural Science Foundation of China (NSFC) (42177201), the Fundamental Research Funds for the Central Universities (265QZ2021004), and the Natural Science Foundation of Beijing Municipality (8202042).
Acknowledgments
We acknowledge Prof. Fei Liu’s for the suggestion of this manuscript.
Conflict of interest
The authors declare that the research was conducted in the absence of any commercial or financial relationships that could be construed as a potential conflict of interest.
Publisher’s note
All claims expressed in this article are solely those of the authors and do not necessarily represent those of their affiliated organizations, or those of the publisher, the editors and the reviewers. Any product that may be evaluated in this article, or claim that may be made by its manufacturer, is not guaranteed or endorsed by the publisher.
References
Albuquerque, C. Gd, Gavelaki, F., Melo, V. F., Motta, A. C. V., Zarbin, A. J. G., and Ferreira, C. M. (2022). Model of inner-sphere adsorption of oxyanions in goethite - why is phosphate adsorption more significant than that of sulfate? Rev. Bras. Cienc. do Solo 46, 46. doi:10.36783/18069657rbcs20210146
Amini, M., Antelo, J., Fiol, S., and Rahnemaie, R. (2020). Modeling the effects of humic acid and anoxic condition on phosphate adsorption onto goethite. Chemosphere 253, 126691. doi:10.1016/j.chemosphere.2020.126691
Antelo, J., Arce, F., Avena, M., López, S. F. R., Macías, a. F., and Macias, F. (2007). Adsorption of a soil humic acid at the surface of goethite and its competitive interaction with phosphate. Geoderma 138, 12–19. doi:10.1016/j.geoderma.2006.10.011
Arroyave, J. M., Waiman, C. C., Zanini, G. P., and Avena, M. J. (2016). Effect of humic acid on the adsorption/desorption behavior of glyphosate on goethite. Isotherms and kinetics. Chemosphere 145, 34–41. doi:10.1016/j.chemosphere.2015.11.082
Bäckström, M., Dario, M., Karlsson, S., and Allard, B. (2003). Effects of a fulvic acid on the adsorption of mercury and cadmium on goethite. Sci. Total Environ. 304 (1-3), 257–268. doi:10.1016/s0048-9697(02)00573-9
Banik, N. L., Buda, R. A., Bürger, S., Kratz, J. V., and Trautmann, N. (2007). Speciation and interactions of plutonium with humic substances and kaolinite in aquifer systems. J. Alloys Compd. 444, 522–525. doi:10.1016/j.jallcom.2006.12.048
Bao, Y., Bolan, N. S., Lai, J., Wang, Y., Jin, X., Kirkham, M. B., et al. (2021). Interactions between organic matter and Fe (hydr)oxides and their influences on immobilization and remobilization of metal(loid)s: A review. Crit. Rev. Environ. Sci. Technol. 52, 4016–4037. doi:10.1080/10643389.2021.1974766
Bauer, I., and Kappler, a. A. (2009). Rates and extent of reduction of Fe(III) compounds and O2 by humic substances. Environ. Sci. Technol. 43, 4902–4908. doi:10.1021/es900179s
Boily, J., Lützenkirchen, J., Balmès, O., Beattie, J., and Sjöberg, a. S. (2001). Modeling proton binding at the goethite (α-FeOOH)–water interface. Colloids Surfaces A Physicochem. Eng. Aspects 179 (1), 11–27. doi:10.1016/s0927-7757(00)00712-3
Bruyn, J. L., and De Bruyn, P. (1976). Hydrolysis-precipitation studies of iron solutions. I. Model for hydrolysis and precipitation from Fe(III) nitrate solutions. J. Colloid Interface Sci. 56 (3), 527–539. doi:10.1016/0021-9797(76)90119-3
Buda, R., Banik, N. L., Kratz, J. V., and Trautmann, N. (2008). Studies of the ternary systems humic substances – kaolinite – Pu(III) and Pu(IV). Radiochim. Acta 96 (9-11), 657–665. doi:10.1524/ract.2008.1550
Chen, H., Koopal, L. K., Xu, J., Wang, M., and Tan, W. (2019). Selective adsorption of soil humic acid on binary systems containing kaolinite and goethite: Assessment of sorbent interactions. Eur. J. Soil Sci., ejss.12803. doi:10.1111/ejss.12803
Cheng, W., Zhou, L., Marsac, R., Boily, J. F., and Hanna, K. (2020). Effects of organic matter-goethite interactions on reactive transport of nalidixic acid: Column study and modeling. Environ. Res. 191, 110187. doi:10.1016/j.envres.2020.110187
Chiou, Cary T., Kile, Daniel E., David, W., Rutherford, G. S., and Boyd, a. S. A. (2000). Sorption of selected organic compounds from water to a peat soil and its humic-acid and humin fractions potential sources of the sorption nonlinearity. Environ. Sci. Technol. 34 (7), 1254–1258. doi:10.1021/es990261c
Christensen, B. T. (1992). Physical fractionation of soil and organic matter in primary particle size and density separates. Adv. Soil 20.
Deng, Y., Weng, L., Li, Y., Ma, J., and Chen, Y. (2019). Understanding major NOM properties controlling its interactions with phosphorus and arsenic at goethite-water interface. Water Res. 157, 372–380. doi:10.1016/j.watres.2019.03.077
Di Iorio, E., Circelli, L., Angelico, R., Torrent, J., Tan, W., and Colombo, C. (2022). Environmental implications of interaction between humic substances and iron oxide nanoparticles: A review. Chemosphere 303, 135172. doi:10.1016/j.chemosphere.2022.135172
Dultz, S., Steinke, H., Mikutta, R., Woche, S. K., and Guggenberger, G. (2018). Impact of organic matter types on surface charge and aggregation of goethite. Colloids Surfaces A Physicochem. Eng. Aspects 554, 156–168. doi:10.1016/j.colsurfa.2018.06.040
Evanko, C. R., and Dzombak, a. D. A. (1998). Influence of structural features on sorption of NOM-analogue organic acids to goethite. Environ. Sci. Technol. 32 (19), 2846–2855. doi:10.1021/es980256t
Evanko, C. R., and Dzombak, D. A. (1999). Surface complexation modeling of organic acid sorption to goethite. J. Colloid Interface Sci. 214 (2), 189–206. doi:10.1006/jcis.1999.6184
Filius, J. D., Hiemstra, T., and Riemsdijk, W. H. V. (1997). Adsorption of small weak organic acids on goethite:modeling of mechanisms. J. Colloid Interface Sci. 195 (2), 368–380. doi:10.1006/jcis.1997.5152
Filius, J. D., Meeussen, J. C. L., Hiemstra, T., and van Riemsdijk, W. H. (2001). Modeling the binding of benzenecarboxylates by goethite: The ligand and charge distribution model. J. Colloid Interface Sci. 244 (1), 31–42. doi:10.1006/jcis.2001.7927
Filius, J. D., Meeussen, J. C. L., Lumsdon, D. G., Hiemstra, T., and van Riemsdijk, W. H. (2003). Modeling the binding of fulvic acid by goethite: The speciation of adsorbed FA molecules. Geochim. Cosmochim. Acta 67 (8), 1463–1474. doi:10.1016/s0016-7037(02)01042-6
Filius, Jeroen D., Lumsdon, David G., Meeussen, JohannesC. L., Hiemstra, Tjisse, Van W, H., and Riemsdijk, a. (2000). Adsorption of fulvic acid on goethite. Geochim. Cosmochim. Acta 64 (1), 51–60. doi:10.1016/s0016-7037(99)00176-3
Fritz, T. O., and Yapp, C. J. (2018). Miocene weathering environments in Western Australia—inferences from the abundance and 13C/12C of Fe(CO3)OH in CID goethite. Geochim. Cosmochim. Acta 227, 186–210. doi:10.1016/j.gca.2018.02.023
Gabriel, C. E., Kellman, L., and Prest, D. (2018). Examining mineral-associated soil organic matter pools through depth in harvested forest soil profiles. PLoS One 13 (11), e0206847. doi:10.1371/journal.pone.0206847
Gerzabek, M. H., Aquino, A. J. A., Balboa, Y. I. E., Galicia‐Andrés, E., Grančič, P., Oostenbrink, C., et al. (2022). A contribution of molecular modeling to supramolecular structures in soil organic matter #. J. Plant Nutr. Soil Sci. 185 (1), 44–59. doi:10.1002/jpln.202100360
Ghosh, M., Swain, K. K., and Verma, R. (2017). Interaction of niobium with iron-oxide colloids and the role of humic acid. J. Environ. Radioact. 178-179, 101–109. doi:10.1016/j.jenvrad.2017.08.003
Gu, B., Schmitt, J., Chen, Z., Liang, L., and McCarthy, J. F. (1994). Adsorption and desorption of natural organic matter on iron oxide mechanisms and models. Environ. Sci. Technol. 28 (1), 38–46. doi:10.1021/es00050a007
Gu, C., and Karthikeyan, K. G. (2008). Sorption of the antibiotic tetracycline to humic-mineral complexes. J. Environ. Qual. 37 (2), 704–711. doi:10.2134/jeq2007.0030
Guhra, T., Ritschel, T., and Totsche, K. U. (2019). Formation of mineral-mineral and organo-mineral composite building units from microaggregate-forming materials including microbially produced extracellular polymeric substances. Eur. J. Soil Sci. 70 (3), 604–615. doi:10.1111/ejss.12774
Guo, L. Y., He, X., Hong, Z. N., and Xu, R. K. (2022). Effect of the interaction of fulvic acid with Pb(II) on the distribution of Pb(II) between solid and liquid phases of four minerals. Environ. Sci. Pollut. Res. 29, 68680–68691. doi:10.1007/s11356-022-20315-w
Hiemstra, T., Antelo, J., van Rotterdam, A. M. D., and van Riemsdijk, W. H. (2010). Nanoparticles in natural systems II: The natural oxide fraction at interaction with natural organic matter and phosphate. Geochim. Cosmochim. Acta 74 (1), 59–69. doi:10.1016/j.gca.2009.10.019
Hiemstra, T., Mia, S., Duhaut, P. B., and Molleman, B. (2013). Natural and pyrogenic humic acids at goethite and natural oxide surfaces interacting with phosphate. Environ. Sci. Technol. 47 (16), 9182–9189. doi:10.1021/es400997n
Hiemstra, T., and Riemsdijk, W. (1996). A surface structural approach to ion adsorption:the charge distribution (CD) model. J. Colloid Interface Sci. 179 (2), 488–508. doi:10.1006/jcis.1996.0242
Iglesias, A., Lopez, R., Gondar, D., Antelo, J., Fiol, S., and Arce, F. (2010). Adsorption of MCPA on goethite and humic acid-coated goethite. Chemosphere 78 (11), 1403–1408. doi:10.1016/j.chemosphere.2009.12.063
Jiang, Q. (2011). Study on solution pH impacting adsorption mechanisms of goethite for dissolved organic matter. J. Wuhan Univ. Technol. 33 (10), 5.
Jickells, T. D., An, Z. S., Andersen, K. K., Baker, A. R., Bergametti, G., Brooks, N., et al. (2005). Global iron connections between desert dust, ocean biogeochemistry, and climate. Science 308 (5718), 67–71. doi:10.1126/science.1105959
Jonsson, J., Sjoberg, S., and Lovgren, L. (2006). Adsorption of Cu(II) to schwertmannite and goethite in presence of dissolved organic matter. Water Res. 40 (5), 969–974. doi:10.1016/j.watres.2006.01.006
Kadar, E., Tarran, G. A., Jha, A. N., and Al-Subiai, S. N. (2011). Stabilization of engineered zero-valent nanoiron with Na-acrylic copolymer enhances spermiotoxicity. Environ. Sci. Technol. 45 (8), 3245–3251. doi:10.1021/es1029848
Kaiser, K., Mikutta, R., and Guggenberger, G. (2007). Increased stability of organic matter sorbed to ferrihydrite and goethite on aging. Soil Sci. Soc. Am. J. 71 (3), 711–719. doi:10.2136/sssaj2006.0189
Kaiser, K. (2003). Sorption of natural organic matter fractions to goethite (α-FeOOH): Effect of chemical composition as revealed by liquid-state 13C NMR and wet-chemical analysis. Org. Geochem. 34 (11), 1569–1579. doi:10.1016/s0146-6380(03)00120-7
Kang, S., and Xing, B. (2007). Humic acid fractionation upon sequential adsorption onto goethite. Langmuir 24 (6), 2525–2531. doi:10.1021/la702914q
Karlsson, T., and Persson, P. (2012). Complexes with aquatic organic matter suppress hydrolysis and precipitation of Fe(III). Chem. Geol. 322, 19–27. doi:10.1016/j.chemgeo.2012.06.003
Khasanova, A. B., Kalmykov, S. N., Perminova, I. V., and Clark, S. B. (2007). Neptunium redox behavior and sorption onto goethite and hematite in the presence of humic acids with different hydroquinone content. J. Alloys Compd. 444, 491–494. doi:10.1016/j.jallcom.2007.02.069
Kleber, M., Bourg, I. C., Coward, E. K., Hansel, C. M., Myneni, S. C. B., and Nunan, N. (2021). Dynamic interactions at the mineral–organic matter interface. Nat. Rev. Earth Environ. 2 (6), 402–421. doi:10.1038/s43017-021-00162-y
Kleber, M., Eusterhues, K., Keiluweit, M., Mikutta, C., Mikutta, R., and Nico, P. S. (2015). Mineral–organic associations: Formation, properties, and relevance in soil environments. Adv. Agron., 1–140. doi:10.1016/bs.agron.2014.10.005
Kodama, H., and Schnitzer, M. (1977). Effect of fulvic acid on the crystallization of Fe(III) oxides. Geoderma 19 (4), 279–291. doi:10.1016/0016-7061(77)90070-2
Lai, L., He, Y., Zhou, H., Huang, B., Yao, G., and Lai, B. (2021). Critical review of natural iron-based minerals used as heterogeneous catalysts in peroxide activation processes: Characteristics, applications and mechanisms. J. Hazard. Mat. 416, 125809. doi:10.1016/j.jhazmat.2021.125809
Langmuir, I. (1917). The constitution and fundamental properties of solids and liquids. J. Frankl. Inst. 183 (1), 102–105. doi:10.1016/s0016-0032(17)90938-x
Liu, H., Chen, T., and Frost, R. L. (2014). An overview of the role of goethite surfaces in the environment. Chemosphere 103, 1–11. doi:10.1016/j.chemosphere.2013.11.065
Liu, J., Wang, J., Chen, Y., Lippold, H., and Lippmann-Pipke, J. (2010). Comparative characterization of two natural humic acids in the Pearl River Basin, China and their environmental implications. J. Environ. Sci. 22 (11), 1695–1702. doi:10.1016/s1001-0742(09)60308-9
Liu, Q., Li, X., Tang, J., Zhou, Y., Lin, Q., Xiao, R., et al. (2019). Characterization of goethite-fulvic acid composites and their impact on the immobility of Pb/Cd in soil. Chemosphere 222, 556–563. doi:10.1016/j.chemosphere.2019.01.171
Lu, A., Li, Y., Ding, H., Xu, X., Li, Y., Ren, G., et al. (2019). Photoelectric conversion on Earth's surface via widespread Fe- and Mn-mineral coatings. Proc. Natl. Acad. Sci. U. S. A. 116 (20), 9741–9746. doi:10.1073/pnas.1902473116
Lu, M. (2000). Oxidation of chlorophenols with hydrogen peroxide in the presence of goethite. Chemosphere 40 (2), 125–130. doi:10.1016/s0045-6535(99)00213-1
Lu, Y., Hu, S., Liu, F., Liang, Y., and Shi, Z. (2020). Effects of humic acid and fulvic acid on the sequestration of copper and carbon during the iron oxide transformation. Chem. Eng. J. 383, 123194. doi:10.1016/j.cej.2019.123194
Luo, C., Wen, S., Lu, Y., Dai, J., and Du, Y. (2022). Coprecipitation of humic acid and phosphate with Fe(III) enhances the sequestration of carbon and phosphorus in sediments. Chem. Geol. 588, 120645. doi:10.1016/j.chemgeo.2021.120645
Maeng, S. K., Sharma, S. K., Lekkerkerker-Teunissen, K., and Amy, G. L. (2011). Occurrence and fate of bulk organic matter and pharmaceutically active compounds in managed aquifer recharge: A review. Water Res. 45 (10), 3015–3033. doi:10.1016/j.watres.2011.02.017
Mangold, J. E. (2013). Predicting ion adsorption onto the iron hydroxide goethite in single and multi-solute systems.
Mikutta, R., Kleber, M., Torn, M. S., and Jahn, R. (2006). Stabilization of soil organic matter: Association with minerals or chemical recalcitrance? Biogeochemistry 77 (1), 25–56. doi:10.1007/s10533-005-0712-6
Mikutta, R., Lorenz, D., Guggenberger, G., Haumaier, L., and Freund, A. (2014). Properties and reactivity of Fe-organic matter associations formed by coprecipitation versus adsorption: Clues from arsenate batch adsorption. Geochim. Cosmochim. Acta 144, 258–276. doi:10.1016/j.gca.2014.08.026
Montalvo, D., Vanderschueren, R., Fritzsche, A., Meckenstock, R. U., and Smolders, E. (2018). Efficient removal of arsenate from oxic contaminated water by colloidal humic acid-coated goethite: Batch and column experiments. J. Clean. Prod. 189, 510–518. doi:10.1016/j.jclepro.2018.04.055
Ni, L., Su, L., Li, S., Wang, P., Li, D., Ye, X., et al. (2017). The characterization of dissolved organic matter extracted from different sources and their influence on cadmium uptake by Microcystis aeruginosa. Environ. Toxicol. Chem. 36 (7), 1856–1863. doi:10.1002/etc.3728
Norén, K., and Persson, P. (2007). Adsorption of monocarboxylates at the water/goethite interface: The importance of hydrogen bonding. Geochim. Cosmochim. Acta 71 (23), 5717–5730. doi:10.1016/j.gca.2007.04.037
Olsson, R., Giesler, R., and Persson, P. (2011). Adsorption mechanisms of glucose in aqueous goethite suspensions. J. Colloid Interface Sci. 353 (1), 263–268. doi:10.1016/j.jcis.2010.09.023
Olu-Owolabi, B. I., Popoola, D. B., and Unuabonah, E. I. (2010). Removal of Cu2+ and Cd2+ from aqueous solution by bentonite clay modified with binary mixture of goethite and humic acid. Water Air Soil Pollut. 211 (1-4), 459–474. doi:10.1007/s11270-009-0315-2
Orsetti, S., Quiroga Mde, L., and Andrade, E. M. (2006). Binding of Pb(II) in the system humic acid/goethite at acidic pH. Chemosphere 65 (11), 2313–2321. doi:10.1016/j.chemosphere.2006.05.009
Otero-Fariña, A., Fiol, S., Arce, F., and Antelo, J. (2017). Effects of natural organic matter on the binding of arsenate and copper onto goethite. Chem. Geol. 459, 119–128. doi:10.1016/j.chemgeo.2017.04.012
Peng, H., Liang, N., Li, H., Chen, F., Zhang, D., Pan, B., et al. (2015). Contribution of coated humic acids calculated through their surface coverage on nano iron oxides for ofloxacin and norfloxacin sorption. Environ. Pollut. 204, 191–198. doi:10.1016/j.envpol.2015.04.029
Persson, P., and Axe, K. (2005). Adsorption of oxalate and malonate at the water-goethite interface: Molecular surface speciation from IR spectroscopy. Geochim. Cosmochim. Acta 69 (3), 541–552. doi:10.1016/j.gca.2004.07.009
Philippe, Schwertmann U., Cambier, P., and Murad, E. (1985). Properties of goethites of varying crystallinity. Clays Clay Minerals. 33. 369–378.
Polubesova, T., and Chefetz, B. (2013). DOM-affected transformation of contaminants on mineral surfaces: A review. Crit. Rev. Environ. Sci. Technol. 44 (3), 223–254. doi:10.1080/10643389.2012.710455
Prélot, B., Villiéras, F., Pelletier, M., Gérard, G., Gaboriaud, F., Ehrhardt, J-J., et al. (2003). Morphology and surface heterogeneities in synthetic goethites. J. Colloid Interface Sci. 261 (2), 244–254. doi:10.1016/s0021-9797(03)00058-4
Qin, X., Du, P., Chen, J., Liu, F., Wang, G., and Weng, L. (2018). Effects of natural organic matter with different properties on levofloxacin adsorption to goethite: Experiments and modeling. Chem. Eng. J. 345, 425–431. doi:10.1016/j.cej.2018.03.125
Qin, X., Hou, H., Zhao, L., Ma, J., Sun, Z., Xue, W., et al. (2016). Effect of humic acid on the adsorption of levofloxacin to goethite. Proceedings of the international conference on civil. Taipei, China: Architecture and Environmental Engineering.
Qin, X., Liu, F., Wang, G., Hou, H., Li, F., and Weng, L. (2015). Fractionation of humic acid upon adsorption to goethite: Batch and column studies. Chem. Eng. J. 269, 272–278. doi:10.1016/j.cej.2015.01.124
Qu, C., Chen, J., Mortimer, M., Wu, Y., Cai, P., and Huang, Q. (2022). Humic acids restrict the transformation and the stabilization of Cd by iron (hydr)oxides. J. Hazard. Mat. 430, 128365. doi:10.1016/j.jhazmat.2022.128365
Ramos, A. C. H. (1996). Goethite dispersibility in solutions of variable ionic strength and soluble organic matter content. Clays Clay Minerals 44 (2), 286–296. doi:10.1346/ccmn.1996.0440213
Safiur Rahman, M., Whalen, M., and Gagnon, G. A. (2013). Adsorption of dissolved organic matter (DOM) onto the synthetic iron pipe corrosion scales (goethite and magnetite): Effect of pH. Chem. Eng. J. 234, 149–157. doi:10.1016/j.cej.2013.08.077
Saito, T., Koopa, L. K., Whv, R, Nagasaki, S., and Tanaka, S. (2003). Adsorption of humic acid on goethite: Isotherms, charge adjustments, and potential profiles. Langmuir 20 (3), 689–700. doi:10.1021/la034806z
Saito, T., Koopal, L. K., Nagasaki, S., and Tanaka, S. (2005). Analysis of copper binding in the ternary system Cu2+/humic acid/goethite at neutral to acidic pH. Environ. Sci. Technol. 39 (13), 4886–4893. doi:10.1021/es0500308
Schwertmann, U., and Murad, E. (1983). Effect of pH on the formation of goethite and hematite from ferrihydrite. Clays Clay Minerals 31, 277–284. doi:10.1346/ccmn.1983.0310405
Schwertmann, U. (1966). Inhibitory effect of soil organic matter on the crystallization of amorphous ferric hydroxide. Nature 212, 645–646. doi:10.1038/212645b0
Schwertmann, U., and Cornell, R. M. (2000). Iron oxides in the laboratory: Preparation and characterization. Weinheim: Wiley-VCH.
Senesi, N., and Loffredo, E. (1998). "The chemistry of soil organic matter," in Soil physical chemistry. 2nd ed. Editors D. L. Sparks, N. Senesi, G. Uehara, R. J. Bartlett, W. P. Robarge, and U. Singh. (Boca Raton: CRC Press), 432.
Shi, Y., Zhang, C., Liu, J., Dai, Q., Jiang, Y., Xi, M., et al. (2021). Distribution of persistent free radicals in different molecular weight fractions from peat humic acids and their impact in reducing goethite. Sci. Total Environ. 797, 149173. doi:10.1016/j.scitotenv.2021.149173
Shin, H.-S., Monsallier, J. M., and Choppin, G. R. (1999). Spectroscopic and chemical characterizations of molecular size fractionated humic acid. Talanta 50 (3), 641–647. doi:10.1016/s0039-9140(99)00161-7
Sodano, M., Lerda, C., Nisticò, R., Martin, M., Magnacca, G., Celi, L., et al. (2017). Dissolved organic carbon retention by coprecipitation during the oxidation of ferrous iron. Geoderma 307, 19–29. doi:10.1016/j.geoderma.2017.07.022
Sollins, P., Kramer, M. G., Swanston, C., Lajtha, K., Filley, T., Aufdenkampe, A. K., et al. (2009). Sequential density fractionation across soils of contrasting mineralogy: Evidence for both microbial- and mineral-controlled soil organic matter stabilization. Biogeochemistry 96 (1-3), 209–231. doi:10.1007/s10533-009-9359-z
Stewart, A. G., Hudson-Edwards, K. A., and Dubbin, W. E. (2013). Mechanisms of goethite dissolution in the presence of desferrioxamine B and Suwannee River fulvic acid at pH 6.5. Geochim. Cosmochim. Acta 115, 1–14. doi:10.1016/j.gca.2013.04.006
Song, X., Wang, P., Van Zwieten, L., Bolan, N., Wang, H., Li, X., et al. (2022). Towards a better understanding of the role of Fe cycling in soil for carbon stabilization and degradation. Carbon Res. 1, 5. doi:10.1007/s44246-022-00008-2
Tabor, N. J., Yapp, C. J., and Montañez, I. P. (2004). Goethite, calcite, and organic matter from permian and triassic soils: Carbon isotopes and CO2 concentrations 1 1Associate editor. Geochim. Cosmochim. Ac. 68 (7), 1503–1517. doi:10.1016/S0016-7037(03)00497-6
Tan, Y., Guo, Y., Gu, X., and Gu, C. (2015). Effects of metal cations and fulvic acid on the adsorption of ciprofloxacin onto goethite. Environ. Sci. Pollut. Res. 22 (1), 609–617. doi:10.1007/s11356-014-3351-4
Tinnacher, R. M., Begg, J. D., Mason, H., Ranville, J., Powell, B. A., Wong, J. C., et al. (2015). Effect of fulvic acid surface coatings on plutonium sorption and desorption kinetics on goethite. Environ. Sci. Technol. 49 (5), 2776–2785. doi:10.1021/es505120s
Tipping, E. (1981). The adsorption of aquatic humic substances by iron oxides. Geochim. Cosmochim. Acta 45 (2), 191–199. doi:10.1016/0016-7037(81)90162-9
Tunega, D., Gerzabek, M. H., Haberhauer, G., Totsche, K. U., and Lischka, H. (2009). Model study on sorption of polycyclic aromatic hydrocarbons to goethite. J. Colloid Interface Sci. 330 (1), 244–249. doi:10.1016/j.jcis.2008.10.056
Vindedahl, A. M., Strehlau, J. H., Arnold, W. A., and Penn, R. L. (2016). Organic matter and iron oxide nanoparticles: Aggregation, interactions, and reactivity. Environ. Sci. Nano 3 (3), 494–505. doi:10.1039/c5en00215j
Wang, J., and Guo, X. (2020). Adsorption isotherm models: Classification, physical meaning, application and solving method. Chemosphere 258, 127279. doi:10.1016/j.chemosphere.2020.127279
Wang, L., Li, Y., Weng, L., Sun, Y., Ma, J., and Chen, Y. (2019). Using chromatographic and spectroscopic parameters to characterize preference and kinetics in the adsorption of humic and fulvic acid to goethite. Sci. Total Environ. 666, 766–777. doi:10.1016/j.scitotenv.2019.02.235
Wang, L., Ying, R., Shi, J., Long, T., and Lin, Y. (2017). Advancement in study on adsorption of organic matter on soil minerals and its mechanism. Acta Pedol. Sin. 54 (4), 805–818.
Wang, T., Jin, Y., Wang, Z., and Yu, a. Z. (1998). A study of the morphology of the goethite crystallization process. Chem. Eng. J. 69 (1), 1–5. doi:10.1016/s1385-8947(97)00104-6
Wei, Q., Zhang, Q., Chen, J., Jin, Y., Zhou, K., Chen, W., et al. (2022). Adsorption behavior and mechanism of tetracycline onto hematite: Effects of low-molecular-weight organic acids. Colloids Surfaces A Physicochem. Eng. Aspects 641, 128546. doi:10.1016/j.colsurfa.2022.128546
Weng, L., Van Riemsdijk, W. H., and Hiemstra, T. (2007). Adsorption of humic acids onto goethite: Effects of molar mass, pH and ionic strength. J. Colloid Interface Sci. 314 (1), 107–118. doi:10.1016/j.jcis.2007.05.039
Weng, L., Van Riemsdijk, W. H., and Hiemstra, T. (2008). Cu2+ and Ca2+adsorption to goethite in the presence of fulvic acids. Geochim. Cosmochim. Acta 72 (24), 5857–5870. doi:10.1016/j.gca.2008.09.015
Weng, L., Van Riemsdijk, W. H., Koopal, L. K., and Hiemstra, T. (2006). Ligand and Charge Distribution (LCD) model for the description of fulvic acid adsorption to goethite. J. Colloid Interface Sci. 302 (2), 442–457. doi:10.1016/j.jcis.2006.07.005
Weng, L. P., Koopal, L. K., Hiemstra, T., Meeussen, J. C. L., and Van Riemsdijk, W. H. (2005). Interactions of calcium and fulvic acid at the goethite-water interface. Geochim. Cosmochim. Acta 69 (2), 325–339. doi:10.1016/j.gca.2004.07.002
Xing, B., Graham, N., and Yu, W. (2020). Transformation of siderite to goethite by humic acid in the natural environment. Commun. Chem. 3 (1), 38. doi:10.1038/s42004-020-0284-3
Xing, S. K. B., and Xing, B. (2008). Humic acid fractionation upon sequential adsorption onto goethite. Langmuir 24 (6), 2525–2531. doi:10.1021/la702914q
Xiong, J., Koopal, L. K., Weng, L., Wang, M., and Tan, W. (2015). Effect of soil fulvic and humic acid on binding of Pb to goethite-water interface: Linear additivity and volume fractions of HS in the Stern layer. J. Colloid Interface Sci. 457, 121–130. doi:10.1016/j.jcis.2015.07.001
Xu, Y., Hiemstra, T., Tan, W., Bai, Y., and Weng, L. (2022). Key factors in the adsorption of natural organic matter to metal (hydr)oxides: Fractionation and conformational change. Chemosphere 308, 136129. doi:10.1016/j.chemosphere.2022.136129
Yang, S., Ge, X., Li, Y., Ding, H., Li, Y., Wang, C., et al. (2022). Light-induced coupling process of Fe redox cycling and natural dissolved organic matters oxidative decomposition at goethite surface: Key role of reactive oxidative species. Chem. Geol. 603, 120928. doi:10.1016/j.chemgeo.2022.120928
Yapp, C. J. (1987). A possible goethite-iron(III) carbonate solid solution and the determination of CO2 partial pressures in low-temperature geologic systems. Chem. Geol. 64 (3-4), 259–268. doi:10.1016/0009-2541(87)90006-4
Yapp, C. J. (2001). Mixing of CO2 in surficial environments as recorded by the concentration and δ13C values of the Fe(CO3)OH component in goethite. Geochim. Cosmochim. Acta 65 (22), 4115–4130. doi:10.1016/s0016-7037(01)00698-6
Yapp, C. J. (1991). Oxygen isotopes in an oolitic ironstone and the determination of goethite δ18O values by selective dissolution of impurities: The 5M NaOH method. Geochim. Cosmochim. Acta 55 (9), 2627–2634. doi:10.1016/0016-7037(91)90378-i
Yu, C., Devlin, J. F., and Bi, E. (2019). Bonding of monocarboxylic acids, monophenols and nonpolar compounds onto goethite. Chemosphere 214, 158–167. doi:10.1016/j.chemosphere.2018.09.080
Yu, H., Liu, G., Jin, R., and Zhou, J. (2021). Goethite-humic acid coprecipitate mediated Fenton-like degradation of sulfanilamide: The role of coprecipitated humic acid in accelerating Fe(III)/Fe(II) cycle and degradation efficiency. J. Hazard. Mat. 403, 124026. doi:10.1016/j.jhazmat.2020.124026
Zhang, H., Taujale, S., Huang, J., and Lee, G. J. (2015). Effects of NOM on oxidative reactivity of manganese dioxide in binary oxide mixtures with goethite or hematite. Langmuir 31 (9), 2790–2799. doi:10.1021/acs.langmuir.5b00101
Zhao, Y., Geng, J., Wang, X., Gu, X., and Gao, S. (2011). Adsorption of tetracycline onto goethite in the presence of metal cations and humic substances. J. Colloid Interface Sci. 361 (1), 247–251. doi:10.1016/j.jcis.2011.05.051
Zhao, Y., Liu, F., and Qin, X. (2017). Adsorption of diclofenac onto goethite: Adsorption kinetics and effects of pH. Chemosphere 180, 373–378. doi:10.1016/j.chemosphere.2017.04.007
Keywords: organic matter, geothite, adsorption model, influencing factors, potential effects
Citation: Yi S, Chen X, Cao X, Yi B and He W (2022) Influencing factors and environmental effects of interactions between goethite and organic matter: A critical review. Front. Environ. Sci. 10:1023277. doi: 10.3389/fenvs.2022.1023277
Received: 19 August 2022; Accepted: 10 October 2022;
Published: 25 October 2022.
Edited by:
Huacheng Xu, Nanjing Institute of Geography and Limnology (CAS), ChinaReviewed by:
Tong Yue, Central South University, ChinaChen Yang, South China University of Technology, China
Xiaopeng Qin, Chinese Research Academy of Environmental Sciences, China
Copyright © 2022 Yi, Chen, Cao, Yi and He. This is an open-access article distributed under the terms of the Creative Commons Attribution License (CC BY). The use, distribution or reproduction in other forums is permitted, provided the original author(s) and the copyright owner(s) are credited and that the original publication in this journal is cited, in accordance with accepted academic practice. No use, distribution or reproduction is permitted which does not comply with these terms.
*Correspondence: Wei He, d2VpLmhlQGN1Z2IuZWR1LmNu