- Stetson University Biology Department, DeLand, FL, United States
Freshwater springs and other groundwater-dependent ecosystems represent important natural resources in landscapes, providing consistent, high-quality water to both freshwater and terrestrial organisms. However, spring and GDE conservation does not appear to be a prominent feature on the US conservation radar. Therefore, this study evaluated the distribution and size of springs in the US with the national water dataset: the US Geological Survey National Water Information System. Using all available measurements of spring discharge and the sampling dates for all springs with data in the dataset (10,279 springs), I compared the number and sizes of springs between states and, where time series were available, determined whether the springs were maintaining historic discharges. I evaluated data quality using the date of the last sampling, number of times sampled, and the length of time sampled for each spring. Finally, I searched the literature for spring endemic species and recorded the states in which they occurred. Within the database, springs were most abundant in western states, but average discharges were largest for states in the southeast and for Idaho and Alaska. Very large springs occurred in some western states, but the multitudes of tiny springs reduced the average discharges. The data were poorly resolved as many of the springs had been sampled only once, often 40–50 years ago. Time series were available only for 126 springs and half of these springs exhibited declines in discharge. Endemic species were mentioned in the literature for 24 states, particularly those in the lower half of the country, and so loss of spring integrity would threaten biodiversity in many states. Due to the poor resolution of the data, broad conclusions about the integrity of these important freshwater systems are difficult to impossible to make for most states using the national dataset. Therefore, I call for a concerted national effort to more broadly evaluate spring and GDE resources. Springs and GDEs are likely to become even more important in the future as climate changes and their roles as freshwater refuges, temperature buffers, and bellwethers become even more important.
Introduction
The spatial extent of the United States makes it one of the largest countries in the world. Conservation on such a scale represents a massive undertaking. As a result, conservation goals must be prioritized, since it is difficult to intensively manage all natural resources simultaneously. Research and policy can help to set those priorities. In the 1980s, the US Fish and Wildlife Service undertook an evaluation of wetland losses from the 1700s to their present, which culminated in a report to Congress in 1990 (Dahl, 1990). This report generated interest in wetland conservation and led to the “No Net Loss” policy for wetland conservation. Whether this policy has produced its desired goal has been hotly contested with some authors suggesting that wetland conservation claims have been overstated, either in terms of acres of wetlands conserved or the ability of those conserved wetlands to preserve their function (e.g., .Turner et al., 2001; Bendor, 2009). However, having an imperfect policy for natural resource protection, which can be refined over time, is better than unmanaged landscape conversion. This policy also led to research into wetland management approaches such wetland mitigation and mitigation banking (e.g., Bendor, 2009; Mayer and Lopez, 2011; Levrel et al., 2017). The ability of these approaches for preservation of natural or appropriately functioning wetlands also has been vehemently debated.
In addition to wetlands, there are other examples of natural resources that have received national scrutiny and protection, such as some old growth forests or landscapes protected under the umbrella of national parks. However, many other natural resources have either no explicit protection or only variable state protection. Freshwater springs and other groundwater-dependent ecosystems (GDEs) provide an example of a type of natural resource that is: 1) important ecologically (Cantonati et al., 2012; Davis et al., 2017) and 2) underprotected in the US (Howard and Merrifield, 2010; Junghans et al., 2016; Rohde et al., 2017; Cantonati et al., 2020; Stevens et al., 2020). Freshwater springs and GDEs provide unique freshwater resources to every landscape in which they occur (Barquín and Scarsbrook, 2007; Cantonati et al., 2020). In arid areas, they may represent the only reliable freshwater for both aquatic and terrestrial organisms (Shepard, 1993; Sada et al., 2005; Stevens and Meretsky, 2008; Davis et al., 2017; Cartwright et al., 2020), but in all types of habitats, they may provide a temperature buffer for freshwater organisms. In warm climates and seasons, they may provide a permanent water source and cool water refuge (Davis et al., 2013; Caldwell et al., 2020; Cartwright et al., 2020), whereas in colder climates and seasons, they may provide access to warm water refuges and perhaps water that is not frozen (Ilmonen, 2008; Caldwell et al., 2020). Furthermore, freshwater springs and GDEs receive their water from aquifers that are recharged by water that percolates through the soil; this percolation process changes the water chemistry of spring water relative to the surrounding surface water (Barquín and Scarsbrook, 2007; Springer and Stevens, 2009; Junghans et al., 2016). As a result of the percolation process, the chemistry of the water supplying springs and GDEs typically reflects the chemistry of the local groundwater rather than that of the surface water and may possess fewer pollutants than surface water, although the spring water chemistry may change quickly after issuing from underground (Barquín and Scarsbrook, 2007; Cantonati et al., 2012; Junghans et al., 2016). Because springs and GDEs represent leakage from underground aquifers, they typically occur disparately in the landscape (Shepard, 1993; Davis et al., 2017). This disconnect between individual springs/GDEs can be particularly extreme in arid areas, producing a level of isolation that has led to a large number of endemic species that reside only in springs and GDEs, sometimes in one system only (Cantonati et al., 2012; Davis et al., 2013; Davis et al., 2017; Cantonati et al., 2020; Cartwright et al., 2020).
Springs and GDEs also represent a window into the nation’s groundwater resources (Howard and Merrifield, 2010; Giardina, 2011), on which the entire nation, both the human and non-human, depends either directly or indirectly. Many states use groundwater for drinking water and others use groundwater for irrigation (Alley, 2006). At the same time, there are ecosystems all over the world that depend on access to groundwater for survival (Rohde et al., 2017). Despite the importance of these groundwater-dependent ecosystems (of which springs are one example), there is no national policy protecting them (Howard and Merrifield, 2010; Rohde et al., 2017), although the USGS collects data on spring discharges and, in some cases, water quality. Many springs have been made the focal point of state parks (e.g., Silver Spring State Park, FL; Mammoth Spring State Park, AR; Mammoth Cave National Park, KY; Bennett Spring State Park, MO; Thousand Springs State Park, ID) or federal reserves (e.g., Crystal River National Wildlife Refuge, FL; Ash Meadows National Wildlife Refuge, NV; Fish Springs National Wildlife Refuge, UT) and it is likely that these springs receive greater scrutiny and protection than the host of other springs and GDEs that occur in landscapes across the country. A number of researchers are working towards a more complete picture of spring classification, distribution, density, and ecosystem integrity (Springer and Stevens, 2009; Howard and Merrifield, 2010; Junghans et al., 2016; Stevens et al., 2020); for example, the online database from the University of Northern Arizona, Springs Online, contains physical, chemical, and biological data on thousands of springs throughout the southwestern US and a growing number of springs outside this region. Despite this concerted effort, many of the spring studies are be done in-house by state and federal scientists, staff at the parks and refuges themselves, or by nearby academic researchers, producing a scattershot picture of the nation’s spring and GDE resources. The data produced by agencies, parks, refuges, and academics may or may not be used for local management and may or may not be published, reducing the clarity of the picture of spring and GDE resource integrity on a national level. However, from studies that have been published, we know that springs and GDEs are threatened in at least some areas. For example, many of Florida’s springs have experienced declines in discharge and water quality over the last 50 years (Barquín and Scarsbrook, 2007; Knight and Clarke, 2014; Work 2020), as have springs in west Texas (Nicot et al., 2022). The geologic landscape that produces springs and other GDEs differs across the country (Florea and Vacher, 2006), so the integrity of springs and GDEs throughout the country also likely differs.
The alarm call for springs and other GDEs, which began decades ago (e.g., Shepard, 1993), has only continued to sound and has gotten louder and more global as time has passed (Sada, 2001; Barquín and Scarsbrook, 2007; Kodrick-Brown and Brown, 2007; Stevens and Meretsky, 2008; Cantonati et al., 2012; Davis et al., 2017; Cantonati et al., 2020; Cartwright et al., 2020; Stevens et al., 2020). Given the increasing threat to springs and other GDEs globally and the incomplete nature of the current picture of these resources in the US, the purpose of this study was fourfold. First, I evaluated the distribution of springs in the US, as represented in the USGS database, to determine where the highest densities of springs are and where the largest springs occur. Second, I evaluated the quality of those data in terms of the frequency and temporal proximity of sampling (i.e., how recently sampled) to determine whether springs are being evaluated regularly. Third, I evaluated trends in spring discharge over time when sufficient time series were available to determine whether the integrity of their discharges were being maintained. Finally, I evaluated the spatial distribution of identified spring endemic species in the literature to determine where biodiversity may be most vulnerable to spring losses. I used these four analyses to evaluate whether the US has collected and is collecting sufficient data to manage, conserve, and/or preserve its springs, groundwater-dependent ecosystems, and the species that occur within them.
Methods
To evaluate the quantity and quality of US spring discharge data, I collected discharge data for springs represented in the USGS National Water Information System (NWIS) database. I collected these data from the NWIS mapper by opening the data files for every spring with discharge data for each state in the US. The NWIS database contains location data for tens of thousands of springs, but many of these files have no associate discharge data. Therefore, I reduced the search to the springs that were coded as having “measurements”, although this filtering process may have resulted in some data loss. I collected all discharge data for each spring. However, this process produced data that were very biased because some springs had only one data point and others had hundreds or thousands. Therefore, I randomly selected one data point from the dataset for each spring that had multiple data points using the “randbetween” function in MS Excel. This function produced a random number between 1 and the maximum number of data points in the dataset. I used this random number to select the data point to represent the discharge for the spring. I repeated this process for each spring that had a dataset of more than one point. Then I calculated the mean and standard error of the discharges in each state to estimate the magnitude and variability in spring discharge for each state.
To provide the USGS NWIS discharge data with some context, I collected data from two additional sources. First, I collected historical information on Texas springs. Brune (1980) visited hundreds of springs in 182 of the 254 counties of Texas and recorded a variety of archeological information, land use history, and any known changes in flow for each spring. I recorded the total number of springs and the number that he described as having lost flow for each of these counties. Second, to provide biological context for the importance of the discharge data, I scoured the literature for references to endemic species in US springs. I recorded the name and state of origin for each species that I found and then summed the number of endemic species for each state.
Statistical analysis
To address my first study goal, I compared whether the number of springs differed between states by comparing the counts for each state to the global mean number of springs across the US with a chi-square test in RStudio (R Core Team, 2022). To evaluate whether spring discharge differed between states, I compared the states’ average spring discharges with a Kruskall-Wallis test followed by Wilcoxon multiple comparisons in JMP (JMP®, 2012). I created maps showing the number of springs, the average discharge, and the standard error of the means for each state using “maps” (Becker et al., 2022) and “ggplot2” in RStudio (Wickham, 2016).
To address my second study goal, I first evaluated when the spring had last been sampled and calculated an average last sample date for the state. Then I calculated an index of data quality using the time since each spring had been last sampled, the number of data points in the data set for each spring, and the number of years that each spring had been sampled:
I compared the index of data quality with the average and maximum discharges in a state with regression using “lm” in RStudio (R Core Team, 2022).
To address my third study goal, I evaluated whether there were trends in spring discharges using Mann Kendall’s tau using “Kendall” in RStudio (McLeod, 2022) for all of the springs that had time series data that represented a span of 20 or more years, had at least 10 data points in this series, and had been sampled in the 2000s. The discharge of many of the springs in the dataset were highly variable, including periods of little or no flow for some springs, so I categorized this variability using the discharge variability ratio, or DVR (Stevens et al., 2020, after Meinzer 1923):
Discharge mean, where DVR = 1 represented constant flow, 1 > DVR < 10 represented moderately variable flow, DVR > 10 represented highly variable flow, and undefined DVR represented ephemeral flow.
For Texas, I correlated the percent of drying springs in the 1970s to the tau coefficient of the time series for the springs in each county described by Brune (1980) using Spearman rank correlation in SPSS (IBM Corp, 2019). I made a map with color shading to indicate the percent of springs of each county that were drying in the 1970s using “maps” (Becker et al., 2022) and “ggplot2” in RStudio (Wickham, 2016)and overlaid graphs of the time series from USGS NWIS.
Finally, to address my fourth study goal, I determined whether there was a relationship between the number of endemic species and the number of springs in a state with Spearman rank correlation in SPSS (IBM Corp, 2019). I plotted this relationship using “maps” (Becker et al., 2022) and “ggplot2” in RStudio (Wickham, 2016).
Results
The number of springs for which there were “measurement” data in the NWIS dataset varied from 1 to 1,764 (Supplementary Table S1) and differed for each state (p = 2.2 × 10−16). The states with the lowest numbers of springs were in the upper Midwest (Ohio west to Minnesota) and the states with the highest numbers of springs were in western states (Montana south to Arizona and Texas). The database contained spring discharge data for all but eight of its states. However, these eight states (Maine, Vermont, Connecticut, Michigan, Indiana, South Carolina, Mississippi, and Louisiana) were surrounded by states that possessed data for spring discharges (Figure 1A). The discharge of springs within states differed greatly, in some cases from no flow to very high discharge, but even so, the average spring discharge differed between states (p < .0001, Figure 1B; Supplementary Table S2). The largest average discharges were in the south (Missouri south and east through Florida), Idaho, and Alaska; these areas also possessed the highest average variability in spring discharge (Figure 1C). In all but 13 states, there were springs with no recorded discharge, although states with the highest mean discharges had few to none of these springs (Figure 2).
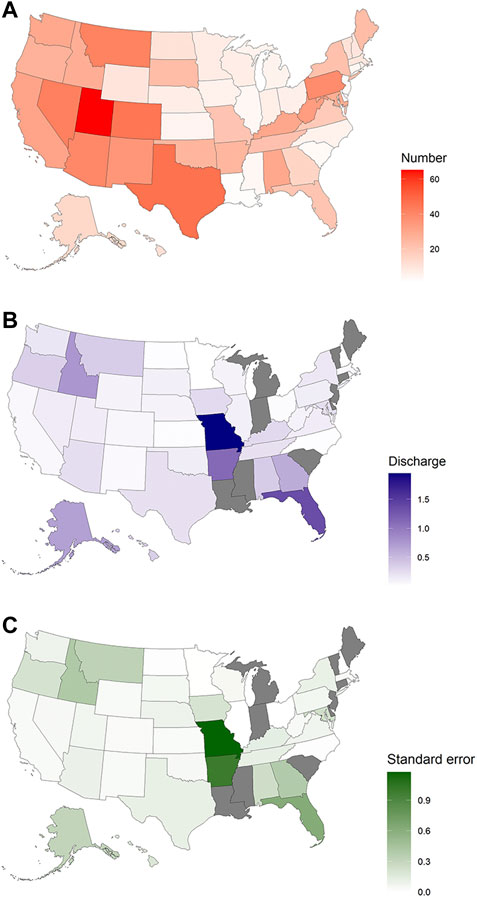
FIGURE 1. Average number of springs (A), average discharge (B), and average variability in discharge (C), as measured by standard error, for US states. Data for all measurements were square root transformed.
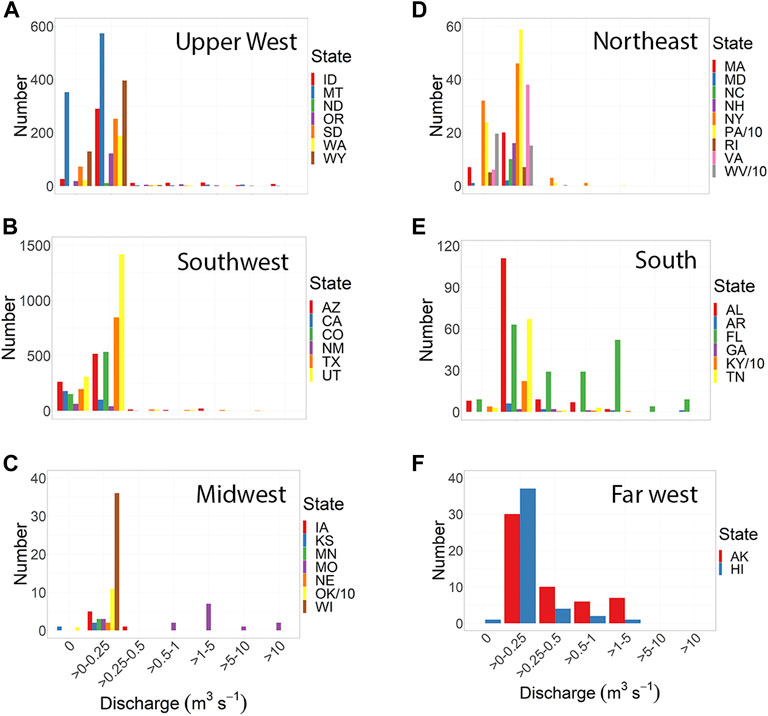
FIGURE 2. Size frequency distributions of springs ranging from not flowing to over 100 cfs in the 42 of 50 US states for which there were discharge data in the NWIS database. The states were somewhat arbitrarily divided by region: the upper west (A), southwest (B), midwest (C), northeast (D), south (E), and Alaska and Hawaii (F).
The quality of the spring discharge data generally was poor. Many thousands of springs had only been sampled once and often that sample was collected prior to 2000. However, most states had at least one spring that had been sampled more recently (Supplementary Table S1). The average window of time between the last spring sample and the present was 40–50 years ago for 24 of the 40 states that had data. Generally, the states with the highest mean discharge had the highest quality data (p = 6.2 × 10−16, Figure 3A), although there was no relationship between data integrity and maximum discharge for a state (p = .57, Figure 3B). Furthermore, for all but five states, the average period of time over which discharge data were recorded was less than 10 years (Supplementary Table S1). Only fifteen states had one or more springs that met the requirement for time series analysis: 1) At least 10 years of data, 2) Over a span of at least 20 years, and 3) with data collected in the 2000s. Only four of these states had more than a handful of these springs.
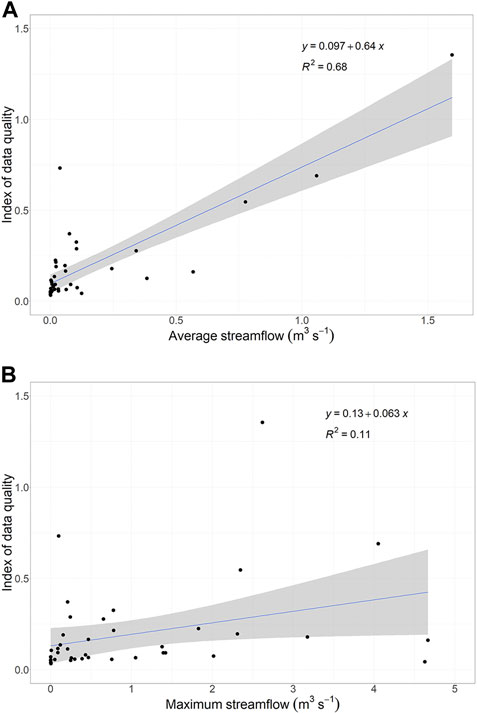
FIGURE 3. The relationship between the index of data integrity and the size of a state’s springs, as measured by average discharge (A) and the maximum discharge for the state (B). The index of data integrity was calculated as a function of the number of years since each spring was last sampled, the number of years that it was sampled, and the number of times that it was sampled within that time span. Grey shading represents the standard error.
Among the 126 springs for which time series were analyzed, maintenance of discharge was highly variable and half (63) of springs exhibited no significant trends in discharge over time (Table 1; Supplementary Table S3). For the springs that did exhibit a change in discharge, 49 springs exhibited decreases and 14 springs exhibited increases in discharge. However, the patterns in discharge trend varied by state and Florida and Idaho possessed the most complete time series. For Florida, 44% of the 50 springs analyzed exhibited trends and all but one of these trends were negative. For Idaho, all but one of the springs analyzed exhibited a decline in discharge over time (Table 1). Only five springs in Nevada and seven springs in Texas exhibited trends in discharge, and they were split between positive and negative trends. In Texas, the springs that exhibited negative trends were in western Texas counties that also had been described as drying in the 1970s (r = -.80, p = .001, Figure 4). The two springs that occurred in counties with few drying springs in the 1970s are located in central Texas and showed no change (Hueco Springs) or an increase in discharge (Barton Springs) in the current time series.
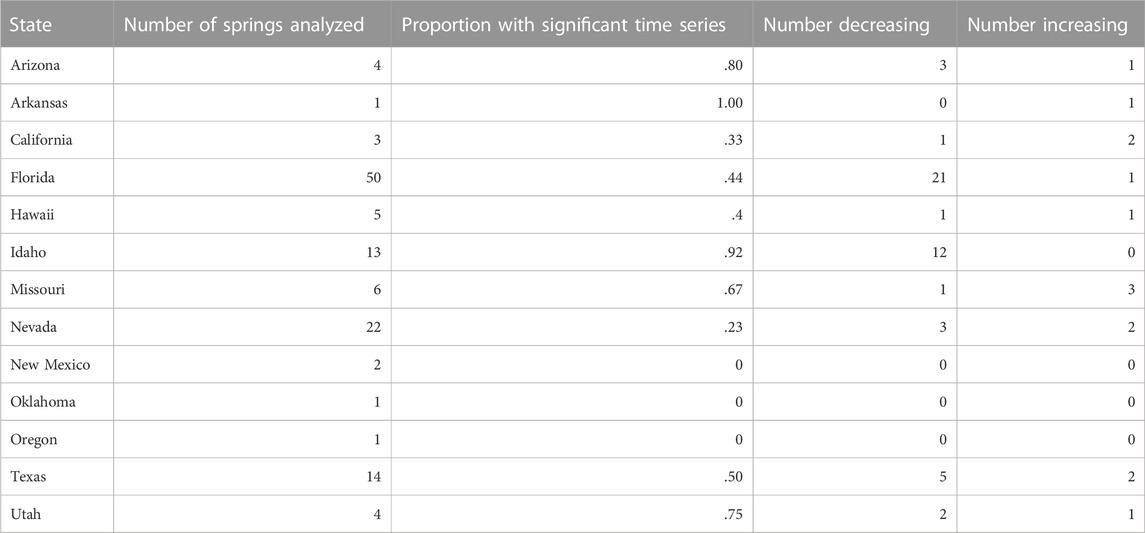
TABLE 1. Numbers of springs that were analyzed in each state, including the number of springs that showed significant increasing and decreasing trends.
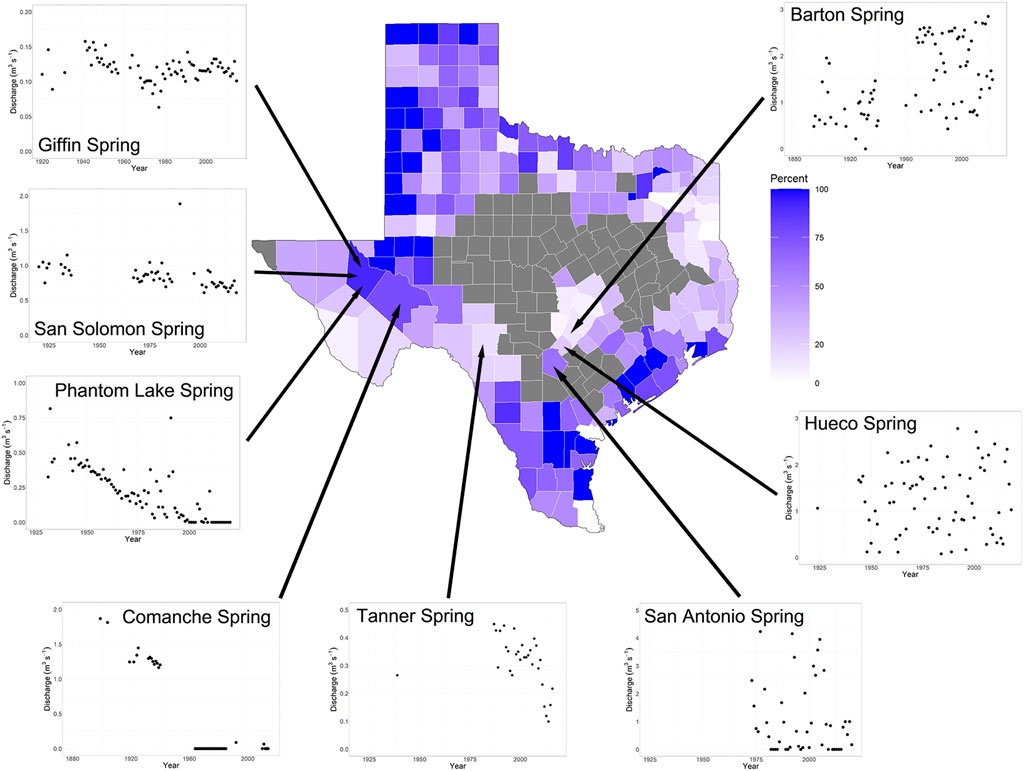
FIGURE 4. Trends in spring discharge for Texas. The map depicts trends in springs in Texas counties described by Brune (1980). He evaluated springs in roughly half of the counties in Texas and described the status of these springs in the 1970s. The counties that he described are color coded for the proportion of springs in the county that he described as drying, ranging from white (no springs in the county drying) to dark purple (all springs in the county drying). Grey counties were not described in Brune (1980). The graphs surrounding the map represent USGS NWIS time series for springs in a subset of the counties described by Brune (1980).
Endemic spring species were represented in the literature for 28 of the 50 states (Figure 5A). This literature search produced 453 individual species, ranging from salamanders to flatworms. The largest number of endemic species were mentioned in the literature describing Texas and Nevada springs. However, there were references to endemic species for states throughout much of the country, with the exception of the upper Midwest and the extreme Northeast. This pattern mimicked the distribution of springs; the number of endemic species was roughly correlated with the number of springs in a state (p = .0018, Figure 5B).
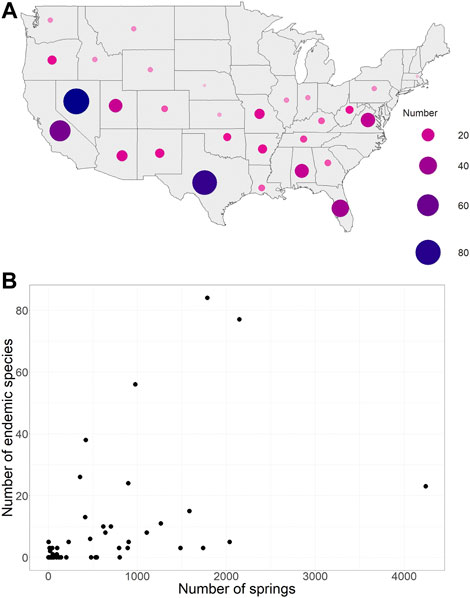
FIGURE 5. The distribution of species endemic to springs in the US (A), as represented in the literature, and the relationship between the number of endemic species and the average spring size for a state (B). The numbers range from 0 species to over 80 endemic species within a state.
Discussion
Springs are omnipresent in the US. Forty of the fifty states contained spring discharge data in the NWIS database. Even for the states with no springs recorded in NWIS, it is more than likely that, in reality, they contain springs that simply have not been sampled by USGS. Many of the US springs are small and many were not flowing at the time of sampling. However, even among the larger springs, many have not been sampled regularly or recently. Spring discharge data for Florida should have been among the best quality in the dataset as they included data from other sources collected during a previous study (Florida Water Management Districts and state parks, Work 2020), but the average date of last sample for Florida springs was 29 years ago, and many Florida spring datasets were spotty. As a result, conclusions about the integrity of these springs (i.e., whether they still flow and flow near historic levels) are difficult to make. What conclusions can be made?.
Spring number
Spring presence provides more information about real spring distribution patterns than does spring absence, given the uncertainty about sampling effort in different states. It is likely that there was a skew in sampling effort toward areas that have large basalt and limestone springs, like Idaho, Florida, Missouri, and Texas (Florea and Vacher, 2006). Despite the potential for uneven sampling, the NWIS database provides evidence that springs are very abundant in the west; there are thousands of small, and some quite large, springs scattered across many western states (Montana, Wyoming, Idaho, Nevada, Utah, Colorado, Arizona, and Texas). The high density of springs in the west is consistent with high densities estimated for the southwest by Stevens and Meretsky (2008); Junghans et al. (2016). The lower numbers of springs for New Mexico may be the result of lower sampling effort; given the large numbers of springs in the states surrounding New Mexico, it seems likely that this state also contains abundant springs and that its spring number is an undercount. Although most of the springs in these states are small, much of the west is quite arid and it is likely that these springs are very important ecologically (Shepard, 1993; Kodrick-Brown and Brown, 2007; Stevens and Meretsky, 2008; Davis et al., 2017; Cartwright et al., 2020). In some cases, and at some times, these springs may be the only surface water available to freshwater and terrestrial organisms (Shepard, 1993; Kodrick-Brown and Brown, 2007; Stevens and Meretsky, 2008; Davis et al., 2017; Cartwright et al., 2020).
Spring discharge
Among the thousands of springs in the NWIS database, most were small and discharged no more than .25 m3 s−1. The southeastern states had the largest mean spring discharges, although this large mean was due to greater proportions of large springs, or perhaps a skew in sampling effort to large springs, rather than possession of only large springs. It is highly likely that large springs were overrepresented in the database due to visibility, ease of sampling, or research priority. Still, many of the largest springs were in the southeast; Arkansas, Florida, and Missouri all possessed at least one spring with a discharge over 10 m3 s−1. Florida contained 9 such springs and while Missouri only contained 2, 67% of its springs discharged more than 1 m3 s−1. However, Idaho and Montanaalso possessed at least one of these very substantial springs and Oregon, Texas, and Utah possessed springs that discharged more than 5 m3 s−1. Therefore, although the dataset for Florida may contain the largest number of large springs and Florida undoubtedly has uniquely rich spring resources, large springs occur in many states of the US.
This variable distribution of springs is due to geologic variability across such a large country. The underlying geology of a region determines the magnitude and variability of spring discharge, with the effects of anthropogenic land and water use overlaid. Florea and Vacher (2006) studied the hydrographs of the basalt springs of Idaho and the large karstic limestone springs in Florida, Missouri, Kentucky, and Texas. These large springs in the five states studied differed in the variability of their discharge due to the permeability of the recharge area matrix, whether the rock that produced the aquifer had been recently deposited and exposed (eogenetic) or exposed after long ago burial (telogenetic), but all produced large discharges due to the conduit-forming nature of basalt and limestone. Of course, limestone and basalt are just two types of rock, highly variable in and of themselves, and the US is comprised of a variety of geologic formations with aquifers that vary in depth and permeability (Miller 1999). The regions with permeable limestone or basalt aquifers produced the largest springs in the US, but these areas contained more springs than just these large discharge systems. As a result, a large and permeable aquifer, such as one in a karst landscape, may be necessary to produce large springs, but that aquifer may produce a diversity of spring sizes (Stevens et al., 2020) and these springs may respond to changes in water availability differently (Weissinger et al., 2016; Cartwright and Johnson, 2018).
The factors that produce instability in spring discharge are likely similar among springs across a variety of landscapes. Drought certainly can affect discharge of springs, perhaps most significantly for aquifers that are tightly coupled to surface processes, such as highly fractured karst and volcanic aquifers (Smith and Hunt, 2010; Mancewicz et al., 2021). Climate change, which could produce drought-like conditions in many regions, could reduce spring discharges in already arid areas that may need springs most, leading to calls for conservation planning (Cartwright et al., 2020). Reductions in spring discharges during drought periods already have been observed in some areas of the world (Fiorillo and Guadagno, 2012; Jia et al., 2017). However, groundwater abstraction and loss of surface permeability in recharge zones can affect springs potentially anywhere. Cantonati et al. (2020) suggest that aquifer overdraft has already caused the loss of springs in landscapes all over the world. They, too, call for conservation planning to ward off these losses.
Temporal trends in spring discharge could only be evaluated for 1% of the springs in the dataset due to lack of time-series data; however, for those springs that could be analyzed, discharge time-series patterns may be linked to geology and land and water use. West Texas spring discharge decline, for example, was attributed to groundwater abstraction over 40 years ago (Brune, 1980) and the trends continue today. South-central Texas springs, which emerge from a different aquifer and have been managed more intensively for discharge maintenance (Smith and Hunt, 2010), have maintained discharge at closer to historic levels. The discharges of most of the largest Florida springs (and many of the smaller springs) have declined in the recent decades (Knight and Clarke, 2014; Work 2020), particularly in areas of intensive agriculture and rapid population growth. Idaho springs also exhibited declines in discharge, but they present a special case of spring discharge decline. The Snake River springs, which erupt from the cliffs above the river or from canyons that lead into the river, occur at the southern end of the Snake River Plain and receive water from lateral flow through this plain. Historic flood irrigation of the Snake River Plain until the early 1950s increased groundwater availability and movement to the springs. Subsequent shifts to irrigation with groundwater reduced aquifer storage and later movement of aquifer water to the springs, resulting in reductions in spring flows (Kjelstrom, 1995; Zuidema et al., 2020). In contrast, the telogenetic springs of Missouri respond quickly to rainfall (Florea and Vacher, 2006) and have maintained their discharge. As a result of this complex interplay of geology, aquifer structure and conductivity, rainfall, and land and water use, more data would clearly help predict where problems are likely to occur.
Biodiversity
US springs contain a rich diversity of endemic species. Many endemic species occur in arid springs, as has been highlighted for decades (Shepard, 1993; Kodric-Brown and Brown, 2007; Hershler et al., 2014). In arid areas, connections between systems may be few and far between, resulting in less migration and more endemism in springs (Thomas et al., 1998; Seidel et al., 2009; Murphy et al., 2015). The literature on springs in the southwest included more endemic vertebrates than the literature from other regions; in the eastern US, vertebrates may be able to move between systems more readily in these wetter, more connected landscapes, leading to lower vertebrate endemism. However, the relative isolation of western springs not only affects vertebrates; the species lists in the arid southwestern springs included hundreds of endemic invertebrates: Hydrobiid snails, amphipods, isopods, flatworms, shrimp, etc. Given that many of the springs in the arid southwest are small, this endemic biodiversity, some of which may be as yet unidentified, may be particularly vulnerable to climate change and spring discharge declines. In the wetter eastern US, the endemic species were largely either cave specialists or tiny hydrobiid snails, which may be limited in their ability to move between springs even if they are connected in the landscape. The hydrobiid snails are so small (many <5 mm, Thompson 1985) that it may be difficult to move from spring to spring unaided, even within the same watershed. Many of the cave specialists also were small (e.g., amphipods and isopods), but they also may have been isolated for similar reasons as the animals in arid landscapes. Caves may occur disparately in the landscape and, for obligate cave dwellers, the streams or rivers that may connect caves may serve as barriers to dispersal. Whether the barriers are dry land or inhospitable connecting waterways, the end result appears to be similar: a large number of endemic species, and probably much higher than we realize, inhabit areas with a lot of springs.
Undoubtedly, this literature search missed publications that mentioned endemic species. However, it is likely that the patterns of endemic species’ distribution would be maintained with further searching. It seems very likely that there are many undiscovered species, some of which may occur in undiscovered springs, but it is also likely that there are species that have been missed in springs represented in the USGS database. Many of the species that occur in springs either are small (e.g., hydrobiid snails or amphipods) or difficult to sample (e.g., cave dwelling species). Some of these species require considerable skill and experience to identify or, alternately to establish as a new species (e.g., hydrobiid snails or amphipods, Seidel et al., 2009). As a result, a huge number of the endemic species have been identified by relatively few people, highlighting the persistent challenge in characterizing the US spring biodiversity.
Better records would produce better outcomes for spring protection
It is difficult to protect what is not understood well. Clearly, the national data on spring and other GDE discharges are incomplete, as has been suggested by other authors (Howard and Merrifield, 2010; Junghans et al., 2016), and many of the NWIS data are very old. It is highly likely that the data gaps are both spatial and temporal. If Florida data collection is comparable to the situation in other states, discharge data exist in a variety of institutions for springs and times not included in the NWIS database. A quick internet search of “Springs of…” (fill in state name) suggests that, at the very least, there are many springs that have not been recorded in the NWIS database and corresponding discharge data may or may not exist for these springs. Some organizations are collecting discharge data outside of government institutions; for example, the Springs Stewardship Institute in northern Arizona contains a database of spring physical, chemical, and biological data and the Florida Springs Institute has begun collecting citizen science data on a number of springs. However, storehouses like these are rare. As a result, most of the data are collected by USGS and stored in NWIS and so we have a spotty picture of spring number, size, and quality.
Springs are important freshwater systems in landscapes, providing consistent, high-quality water to freshwater and terrestrial ecosystems (Barquín and Scarsbrook, 2007), so coordinated information would help to protect what is still a relatively abundant resource. Many springs host endemic species, and even for those that do not, they provide temperature and chemical refuges relative to surface water in many landscapes (Barquín and Scarsbrook, 2007; Caldwell et al., 2020). Therefore, better protection for springs and other GDEs would help protect biodiversity. Spring biodiversity protection is critical now, as biodiversity is already being lost (Cantonati et al., 2020), and it is likely to become even more important in the future as the climate changes; the importance of some springs and GDEs are likely to increase as some arid areas become drier (Cartwright et al., 2020). Identifying springs and GDEs with the highest potential to serve as both the hydrologic oases that many now are, and the climate oases that they may be in the future, requires data on discharge stability, connectivity to other systems, and biodiversity (Cartwright et al., 2020). Finally, groundwater could become more important for humans as a resource in the future with climate change (Taylor et al., 2013). Many municipalities and farmlands rely on groundwater for drinking and irrigation water in the US and around the world (Alley, 2006; Cantonati et al., 2020), at times putting human use in direct competition with maintenance of the resource (Shepard, 1993; Kodrick-Brown and Brown, 2007; Howard and Merrifield, 2010; Davis et al., 2017). This competition may get more acute with climate change (Cantonati et al., 2020; Cartwright et al., 2020). Protecting springs and other GDEs could be considered a form of natural resource insurance. Therefore, I call for an evaluation of springs and other GDE resources comparable to what was done for wetlands in the 1980s. While the results of this evaluation and the resulting “No Net Loss” policy have not produced perfect protection for wetlands, I argue that the situation would be worse without the evaluation and policy changes. Without a similar national evaluation of springs, beyond what I was able to accomplish here, spring conservation will continue to be spotty and underfunded, up to the inspiration of the state.
Data availability statement
The original contributions presented in the study are included in the article/Supplementary Material, further inquiries can be directed to the corresponding author.
Ethics statement
Ethical review and approval was not required for the animal study because I only used references from the literature. No animal was touched.
Author contributions
The author confirms being the sole contributor of this work and has approved it for publication.
Acknowledgments
I would like to thank Gary Garrett, Jeanette Howard, and Rob Van Kirk for helping me to think about the context of springs in different regions of the US. I would like to thank Tony Abbott for helping me to devise an index of data integrity. I would like to thank Missy Gibbs and Haleigh Ray for help editing the manuscript.
Conflict of interest
The authors declare that the research was conducted in the absence of any commercial or financial relationships that could be construed as a potential conflict of interest.
Publisher’s note
All claims expressed in this article are solely those of the authors and do not necessarily represent those of their affiliated organizations, or those of the publisher, the editors and the reviewers. Any product that may be evaluated in this article, or claim that may be made by its manufacturer, is not guaranteed or endorsed by the publisher.
Supplementary material
The Supplementary Material for this article can be found online at: https://www.frontiersin.org/articles/10.3389/fenvs.2022.1022424/full#supplementary-material
References
Alley, W. (2006). Tracking U.S. Groundwater: Reserves for the future? Environ. Sci. Policy Sustain. Dev. 48 (3), 10–25. doi:10.3200/envt.48.3.10-25
Becker, R. A., Wilks, A. R., Brownrigg, R., Minka, T., and Deckmyn, A. (2022). maps: Draw Geographical Maps. R package version 3.4.1. Available at: https://CRAN.R-project.org/package=maps.
Barquín, J., and Scarsbrook, M. (2007). Management and conservation strategies for coldwater springs. Aquatic Conservation Mar. Freshw. Ecosyst. 18 (5), 580–591. doi:10.1002/aqc.884
Bendor, T. (2009). A dynamic analysis of the wetland mitigation process and its effects on no net loss policy. Landsc. Urban Plan. 89, 17–27. doi:10.1016/j.landurbplan.2008.09.003
Caldwell, T. G., Wolaver, B. D., Bongiovanni, T., Pierre, J. P., Robertson, S., Abolt, C., et al. (2020). Spring discharge and thermal regime of a groundwater dependent ecosystem in an arid karst environment. J. Hydrology 587, 124947. doi:10.1016/j.jhydrol.2020.124947
Cantonati, M., Füreder, L., Gerecke, R., Jüttner, I., and Cox, E. J. (2012). Crenic habitats, hotspots for freshwater biodiversity conservation: Toward an understanding of their ecology. Freshw. Sci. 31, 463–480. doi:10.1899/11-111.1
Cantonati, M., Fensham, R. J., Stevens, L. E., Gerecke, R., Glazier, D. S., Goldscheider, N., et al. (2020). Urgent plea for global protection of springs. Conserv. Biol. 35 (1), 378–382. doi:10.1111/cobi.13576
Cartwright, J., and Johnson, H. M. (2018). Springs as hydrologic refugia in a changing climate? A remote-sensing approach. Ecosphere 9 (3), e02155. doi:10.1002/ecs2.2155
Cartwright, J. M., Dwire, K. A., Freed, Z., Hammer, S. J., McLaughlin, B., Misztal, L. W., et al. (2020). Oases of the future? Springs as potential hydrologic refugia in drying climates. Front. Ecol. Environ. 18 (5), 245–253. doi:10.1002/fee.2191
Dahl, T. E. (1990). Wetland losses in the United States 1780s to 1980s. Washington, D.C: U.S. Department of the Interior, Fish and Wildlife Service.
Davis, J. A., Kerezsy, A., and Nicol, S. (2017). Springs: Conserving perennial water is critical in arid landscapes. Biol. Conserv. 211, 30–35. doi:10.1016/j.biocon.2016.12.036
Davis, J., Pavlova, A., Thompson, R., and Sunnucks, P. (2013). Evolutionary refugia and ecological refuges: Key concepts for conserving Australian arid zone freshwater biodiversity under climate change. Glob. Change Biol. 19, 1970–1984. doi:10.1111/gcb.12203
Fiorillo, F., and Guadagno, F. M. (2012). Long karst spring discharge time series and droughts occurrence in Southern Italy. Environ. Earth Sci. 65, 2273–2283. doi:10.1007/s12665-011-1495-9
Florea, L. J., and Vacher, H. L. (2006). Springflow hydrographs: Eogenetic vs. telogenetic karst. Groundwater 44 (3), 352–361. doi:10.1111/j.1745-6584.2005.00158.x
Giardina, M. (2011). Challenges and strategies for spring ecosystem restoration in the Arid Southwest. Hydrol. Water Res. Ariz. Southwest 41, 5–10.
Hershler, R., Liu, H. P., and Howard, J. (2014). Springsnails: A new conservation focus in Western north America. BioScience 64, 693–700. doi:10.1093/biosci/biu100
Howard, J., and Merrifield, M. (2010). Mapping groundwater dependent ecosystems in California. PloS ONE 5 (6), e11249. doi:10.1371/journal.pone.0011249
Ilmonen, J. (2008). Crunoecia irrorata (Curtis) (Trichoptera: Lepidostomatidae) and conservation of boreal springs: Indications of clustering of red-listed species. Aquatic Conservation Mar. Freshw. Ecosyst. 18 (1), 6–18. doi:10.1002/aqc.808
Junghans, K., Springer, A. E., Stevens, L. E., and Ledbetter, J. D. (2016). Springs ecosystem distribution and density for improving stewardship. Freshw. Sci. 35 (4), 1330–1339. doi:10.1086/689182
Kjelstrom, L. C. (1995). Streamflow gains and losses in the Snake River and ground-water budgets for the Snake River plain, Idaho and eastern Oregon. Professional Paper 1408-C (Reston, VA: US Geological Survey).
Knight, R. L., and Clarke, R. A. (2014). Florida springs—A water-budget approach to estimating water availability. J. Earth Sci. Eng. 6, 59–72. doi:10.17265/2159-581X/2016.02.001
Kodrick-Brown, A., and Brown, J. H. (2007). Native fishes, exotic mammals, and the conservation of desert springs. Front. Ecol. Environ. 5 (10), 549–553. doi:10.1890/070002
Levrel, H., Scemama, P., and Vaissière, A-C. (2017). Should we be wary of mitigation banking? Evidence regarding the risks associated with this wetland offset arrangement in Florida. Ecol. Econ. 135, 136–149. doi:10.1016/j.ecolecon.2016.12.025
Mancewicz, L. K., Davisson, L., Wheelock, S. J., Burns, E. R., Poulson, S. R., and Tyler, S. W. (2021). Impacts of climate change on groundwater availability and spring flows: Observations from the highly productive medicine lake highlands/fall River springs aquifer system. J. Am. Water Resour. Assoc. 57 (6), 1021–1036. doi:10.1111/1752-1688.12976
Mayer, A. L., and Lopez, R. D. (2011). Use of remote sensing to support forest and wetlands policies in the USA. Remote Sens. 3, 1211–1233. doi:10.3390/rs3061211
McLeod, A. (2022). Kendall: Kendall rank correlation and Mann-Kendall trend test. R package version 2.2.1. Available at: https://CRAN.R-project.org/package=Kendall.
Meinzer, O. E. (1923). Outline of ground-water hydrology, with definitions. Water Supply Paper 494 (Washington, DC: U.S. Geological Survey).
Miller, J. A. (1999). Groundwater atlas of the United States: Introduction and national summary. Hydrologic Atlas 730-A (Reston, VA: US Geological Survey).
Murphy, N. P., Guzik, M. T., Cooper, S. J. B., and Austin, A. D. (2015). Desert spring refugia: Museums of diversity of evolutionary cradles? R. Swed. Acad. Sci. 44 (6), 693–701. doi:10.1111/zsc.12129
Nicot, J-P., Smyth, R. C., Darvari, R., and McKinney, S. T. (2022). New hydrogeochemical insights on a West Texas desert spring cluster: Trans-Pecos Balmorhea-Area Springs. Appl. Geochem. 142, 105331. doi:10.1016/j.apgeochem.2022.105331
R Core Team (2022). The R Stats package version 4.3.0. Available at: R-core@r-project.org.
Rohde, M. M., Froend, R., and Howard, J. (2017). A global synthesis of managing groundwater dependent ecosystems under sustainable groundwater policy. Groundwater 55 (3), 293–301. doi:10.1111/gwat.12511
Sada, D. W. (2001). A guide to managing, restoring, and conserving springs in the Western United States. Washington, DC: US Department of the Interior, Bureau of Land Management.
Sada, D. W., Fleishman, E., and Murphy, D. D. (2005). Associations among spring-dependent aquatic assemblages and environmental and land use gradients in a Mojave Desert mountain range. Divers. Distributions 11 (1), 91–99. doi:10.1111/j.1366-9516.2005.00131.x
Seidel, R. A., Lang, B. K., and Berg, D. J. (2009). Phylogeographic analysis reveals multiple cryptic species of amphipods (Crustacea: Amphipoda) in chihuahuan desert springs. Biol. Conserv. 142 (10), 2303–2313. doi:10.1016/j.biocon.2009.05.003
Shepard, W. (1993). Desert springs—Both rare and endangered. Aquatic Conservation Mar. Freshw. Ecosyst. 3 (4), 351–359. doi:10.1002/aqc.3270030409
Smith, B. A., and Hunt, B. B. (2010). A comparison of the 1950s drought of record and the 2009 drought, Barton Springs segment of the Edwards Aquifer, Central Texas. Gulf Coast Assoc. Geol. Soc. Trans. 60 611–622. doi:10.1130/2019.1215(07)
Springer, A. E., and Stevens, L. E. (2009). Spheres of discharge of springs. Hydrogeology J. 17, 83–93. doi:10.1007/s10040-008-0341-y
Stevens, L. E., Aly, A. A., Arpin, S. M., Apostolova, I., Ashley, G. M., Barba, P. Q., et al. (2022). “The ecological integrity of spring ecosystems: A global review,” in Imperiled: The encyclopedia of conservation. Editors D. A. DellaSala, and M. I. Goldstein (New York, NY: Elsevier Science), 2608.
Stevens, L. E., and Meretsky, V. J. (2008). Aridland springs of north America: Ecology and conservation. Tucson, AZ: The University of Arizona Press, 406.
Stevens, L. E., Schenk, E. R., and Springer, A. E. (2020). Springs ecosystem classification. Ecol. Appl. 31 (1), e02218. doi:10.1002/eap.2218
Taylor, R. G., Scanlon, B., Döll, P., Rodell, M., van Beek, R., Wada, Y., et al. (2013). Ground water and climate change. Nat. Clim. Change 3, 322–329. doi:10.1038/nclimate1744
Thomas, E. P., Blinn, D. W., and Keim, P. (1998). Do xeric landscapes increase genetic divergence in aquatic ecosystems? Freshw. Biol. 40 (4), 587–593. doi:10.1046/j.1365-2427.1998.00357.x
Thompson, F. G. (1985). The freshwater snails of Florida: A manual for identification. Gainesville, FL: University of Florida Press.
Turner, E. R., Redmond, A. M., and Zedler, J. B. (2001). Count it be acre of function—mitigation adds up to net loss of wetlands. NWN 23 (6), 5–16.
Weissinger, R., Philippi, T. E., and Thoma, D. (2016). Linking climate to changing discharge at springs in Arches National Park, Utah, USA. Ecosphere 7 (10), e01491. doi:10.1002/ecs2.1491
Work, K. (2020). Not just an arid landscape problem: Springflow declines in a region with high rainfall. Limnologica 82, 125766. doi:10.1016/j.limno.2020.125766
Keywords: spring discharge, time series, endemic species, hydrology, data quality
Citation: Work K (2023) The distribution, magnitude, and endemic species of US springs. Front. Environ. Sci. 10:1022424. doi: 10.3389/fenvs.2022.1022424
Received: 18 August 2022; Accepted: 27 December 2022;
Published: 16 January 2023.
Edited by:
Rosana Mazzoni, Rio de Janeiro State University, BrazilReviewed by:
Melissa M. Rohde, The Nature Conservancy, United StatesAbraham Springer, Northern Arizona University, United States
Copyright © 2023 Work. This is an open-access article distributed under the terms of the Creative Commons Attribution License (CC BY). The use, distribution or reproduction in other forums is permitted, provided the original author(s) and the copyright owner(s) are credited and that the original publication in this journal is cited, in accordance with accepted academic practice. No use, distribution or reproduction is permitted which does not comply with these terms.
*Correspondence: Kirsten Work, a3dvcmtAc3RldHNvbi5lZHU=