- 1Institut für Biologie, Freie Universität Berlin, Berlin, Germany
- 2Berlin-Brandenburg Institute of Advanced Biodiversity Research (BBIB), Berlin, Germany
Conventional agriculture has relied extensively on the use of fungicides to prevent or control crop diseases. However, some fungicides, particularly broad-spectrum fungicides, not only eliminate target pathogens but also non-target and beneficial soil microbes. This scenario is not only limited to agricultural soil, but this may also potentially occur when neighboring environments are contaminated by fungicides through spray drift. Although concentrations may be sub-lethal, the chemicals may accumulate in the soil when used continuously resulting in more toxic effects. In this study, the effect on the colony extension rate of 31 filamentous soil saprobic fungi, initially isolated from a protected grassland ecosystem, were analyzed under fungicide treatment. These isolates were considered naive (no deliberate exposure), hence presumed to have not developed resistance. Two currently used fungicides with different modes of action were added to Potato Dextrose Agar at varying concentrations. Results showed a wide range of tolerance and sensitivity to isopyrazam and prothioconazole. Fungi belonging to the phylum Basidiomycota were most negatively affected by both fungicides. Phylum Mucoromycota were the most tolerant to prothioconazole while isolates belonging to phylum Ascomycota differed in their responses to both fungicides. Negative effects on the growth rate were more pronounced at higher concentrations except for a few isolates that were inhibited at 1 mg·L−1. A slightly positive effect was also observed in three of the isolates under fungicide treatment. Lastly, the negative impact of fungicides was not associated with the growth strategy of the fungi, whether fast growing or slow growing, rather it is isolate-specific and phylogenetically conserved. The results of this study demonstrate that co-occurring fungi differ in their sensitivity to fungicides even without prior exposure. This difference in sensitivity among co-occurring fungi may result in shifts in community composition of the soil fungal community to the detriment of the more sensitive isolates.
Introduction
Fungi, together with bacteria dominate soil microbial biomass (Fierer, 2017; He et al., 2020). They are found in virtually all types of soil ecosystems such as grasslands, forests, and agriculture. They are important in organic matter decomposition and nutrient cycling, biocontrol and regulation, and supporting ecosystem functions (Frac et al., 2018). Even though several species are associated with disease, many fungi serve as indicators of soil health (Ding et al., 2022).
Soil fungal biodiversity is altered by several factors including other organisms, soil properties (e.g., pH, salinity, water holding capacity), and chemical amendments like fertilizers and pesticides (Frąc et al., 2018; Jiang et al., 2021). Pesticides are widely accepted plant protection products commonly applied to maximize crop yield by preventing or controlling plant diseases (Bünemann et al., 2006; Wiik and Rosenqvist, 2010; Turkington et al., 2012; Caldwell et al., 2017). Farmers rely on intensive farming practices to increase productivity and profitability by minimizing crop loss. These include the use of fungicides to combat fungal-associated plant diseases (Wegulo et al., 2011; Fones et al., 2020). Despite public concerns on the impact of these chemicals to health and environment, pesticide sales remain constant within the 2011–2018 period, with fungicides and bactericides as the most sold group (Eurostat, 2020).
Prothioconazole and isopyrazam are foliar fungicides currently used in agriculture (Fungicide Resistance Action Committee, 2022). Prothioconazole is a broad-spectrum systemic fungicide belonging to the chemical class triazolinthione (United States Environmental Protection Agency, 2007). It is most commonly used to control diseases of cereals (Edwards and Godley, 2010; Sanssené et al., 2011) and other crops (Lonergan et al., 2015; Miller, Standish and Quesada-Ocampo, 2020). As a sterol 14α-demethylase inhibitor (DMI), it inhibits the formation of ergosterol, a major component of fungal cell membrane, thereby stopping fungal growth (Revie et al., 2018). Isopyrazam is a carboxamide that is also used against diseases of various crops (Pérez-Vicente, 2012; Ajigboye et al., 2014). It inhibits the enzyme succinate dehydrogenase (SDHI) which is crucial in the tricarboxylic cycle (TCA) associated with fungal respiration (Santísima-Trinidad et al., 2018).
Undeniably, fungicides have been useful in minimizing crop loss and increasing plant yield via the control and prevention of plant diseases. However, other non-target species may also be affected by the prolonged use and overuse of fungicides. Accumulation of foliar fungicides that enter into the soil may affect the microbiota that play an important role in decomposition, nutrient cycling, or production of important metabolites for plant growth (Karlsson et al., 2014; Baćmaga et al., 2016; Manoharan et al., 2017; Pánková et al., 2018; Santísima-Trinidad et al., 2018; Baćmaga et al., 2019; Baćmaga et al., 2020; Carpio et al., 2020; Meena et al., 2020; Roman et al., 2021; Edlinger et al., 2022; Noel et al., 2022; Sim et al., 2022; Yao et al., 2022). Some of these organisms are even found to have antagonistic activities against pathogens that could rather be tapped to maximize the effect of existing fungicide application strategies (Tagawa et al., 2010; Patkowska et al., 2019; Chen et al., 2021). Like agricultural ecosystems, grasslands also benefit from soil microorganisms to maintain ecosystem functions or services. The structure of microbial communities in grassland ecosystems is affected by animal activities like grazing and human activities including long-term application of fertilizers (Cassman et al., 2016; Huhe et al., 2017; Liao et al., 2021). In turn, microbial composition, the presence of plant pathogens and plant-fungal interactions shape plant diversity and biomass production (Huhe et al., 2017). Land-use conversion of grasslands to agriculture have inadvertently exposed surrounding natural ecosystems to fungicides and other chemicals. Even nature conservation areas adjacent to agricultural fields are not exempted from this. Pesticide residues including fungicides are found on insect bodies collected from nature reserves all over Germany, including Oderhänge Mallnow where the fungi used in this study were collected (Brühl et al., 2021). Indirect contamination of neighboring habitats may also be possible via spray drift or the transmission of pesticide droplets through air to non-target sites (Van Den Berg et al., 1999; United States Environmental Protection Agency, 2007, OCSPP, 2015).
Grassland studies involving fungi mostly focused on their biodiversity and role in soil health (Yang T et al., 2017; Yang Y et al., 2017; Navrátilová et al., 2019; Ceulemans et al., 2019; Kang et al., 2020; Wu H et al., 2021; Yu and Dijkstra, 2020; Yu et al., 2021; Zhang et al., 2021). So far, only a few studies have focused on the effect of fungicides on the grassland soil fungal community (Frank et al., 2003; Daniel et al., 2015; Sułowicz et al., 2016; Sułowicz and Piotrowska-Seget, 2016; Pánková et al., 2018) and even fewer on grasslands within nature reserves. In the study of Brühl et al. (2021) mentioned above, it clearly showed that pesticide contamination poses a risk on non-target organisms inside a protected habitat adjacent to areas where conventional agriculture is being practiced. In the present study, soil fungi previously isolated from a protected grassland environment were used to evaluate the potential effect of fungicides on naive (i.e., not deliberately exposed to fungicide) fungal isolates. These isolates come from three fungal phyla, namely Ascomycota, Mucoromycota and Basidiomycota. Their tolerance against two currently used fungicides in agriculture was determined at different concentrations. Although these fungicides were not among the reported residues detected in insect bodies living in the grassland area where these fungi were isolated (Brühl et al., 2021), traces of exposure via spray drift from nearby farms cannot be excluded. This set of fungi (referred to as Rillig Lab Core Set, RLCS) was previously evaluated for different trait characteristics and growth strategies (Andrade-Linares et al., 2016; Veresoglou et al., 2018; Lehmann et al., 2019; Camenzind et al., 2020). These well-characterized isolates were used to test the following hypotheses: 1) the application of the fungicides prothioconazole and isopyrazam will negatively affect growth of fungal isolates from a natural grassland, with stronger detrimental effects at higher fungicide concentration, 2) the isolates will respond differently against the two fungicides having different modes of actions, 3) slow-growing fungi will have better tolerance to fungicides than fast-growing ones, and 4) responses are phylogenetically conserved.
Materials and methods
Fungal isolates
Thirty-one fungal strains were previously isolated from a natural semi-arid grassland situated inside a natural reserve in Mallnow Lebus, Brandenburg, Germany (52°27.778′N, 14°29.349′E), as described in Andrade-Linares et al. (2016). These comprised species from Ascomycota, Basidiomycota and Mucoromycota (Supplementary Table S1).
Determination of minimum inhibitory concentration
To determine the concentration of the fungicides that will be used in the experiment, a preliminary test to establish the MIC was performed using the broth microdilution technique. This is a reference method that makes use of microdilution plates (e.g., 96-well plate) containing a series of two-fold dilutions of the antifungal drug, inoculated with the test organism and evaluated visually or spectrophotometrically (Pfaller et al., 2000). Minimum inhibitory concentration (MIC) is defined as the lowest concentration of an antimicrobial agent that causes a specified reduction in visible growth (CLSI, 2008). Eight species from the three fungal phyla were grown on PDA plates at 25°C for 7 days. To dislodge the spores, 5 ml of sterile distilled water with 0.1% Tween 80 (v/v) was pipetted over the mycelium and the surface was gently rubbed with a sterile glass rod. The liquid containing the spores was harvested and vortexed for 10 s to distribute the spores evenly. An aliquot of 2,000 µl was obtained from the collected suspension and centrifuged at 12,500 rpm for 5 min. Afterwards, 1,800 µl of the suspension was discarded and the remaining 200 µl were used to count the number of spores using a Neubauer counting chamber. Final spore concentration was adjusted to 4.0 × 104 (Balouiri, Sadiki and Ibnsouda, 2016). Stock solutions of the fungicides prothioconazole (Sigma-Aldrich) and isopyrazam (Sigma-Aldrich) were prepared using 100% dimethyl sulfoxide (BioChemica, Germany) and were further diluted to 2-fold of the final working concentration (30 mg·L−1). On the first well, 200 µl of the fungicide solution was pipetted. This served as the fungicide control (blank well). Aliquots of 100 µl Potato Dextrose Broth were dispensed on wells 2–12. A two-fold serial dilution was employed using a multichannel pipette from well 1–11 giving final concentrations ranging from 0.015 to 16 mg·L−1. From the prepared suspension of the test organisms, 100 µl was inoculated from wells 2–12. The last well served as growth control well containing only the test organism and PDB. Plates were incubated at 25°C for 48 h. Following incubation, the wells were visually inspected for growth and absorbance (OD) was measured at 570 nm using a microplate reader (Bio-RAD, United States). Prior to calculating the MIC, the optical densities (ODs) of the blank (uninoculated well) were first subtracted from the ODs of the inoculated wells. The percentage of growth were then calculated for the individual wells using the formula:
In vitro assessment of the effects of fungicides on the growth of the test fungi
A mycelial plug of 6-mm diameter of fungal mycelium was obtained from the margin of an actively growing culture and placed at the center of Potato Dextrose Agar supplemented with fungicides. The inoculated plates were then individually sealed with parafilm and incubated in upright position at 25°C for 7 days. Concentrations of 1, 3 and 5 mg·L−1 were chosen as treatment conditions based on the MIC tests and published literature (Hof, 2001). These concentrations were considered sub-lethal for most of the tested isolates, therefore allowing measurable effects within the experimental period instead of completely preventing fungal growth. Plates without fungicide were included as controls for each treatment. Three replicates were prepared for all the organisms and for each concentration (including the controls). Colony diameter was measured after 3, 5, and 7 days. Two perpendicular measurements were made for every plate and subsequently averaged to calculate the colony area. Colony extension rate (mm2·d−1) was computed following the equation:
Similar experiments were also conducted at temperatures of 30°C and 35°C (results are presented in Supplementary Materials, Supplementary Figure S5; Supplementary Table S6).
Data analysis
Colony extension rate was calculated as the response variable. Since the correlation of the colony extension rate to concentration mostly followed non-normal distributions and showed heteroskedasticity, generalized linear mixed models (GLMM) were used to analyze the effects of these treatment conditions using the function glmmPQL() in R (package MASS, Venables and Ripley, 2002). Concentration and fungicide types were used as fixed factors and species identity nested within phylum were used as random factors to analyze the collective response of all fungi to the two fungicides. For individual fungal isolates, replicate was considered a random factor. Gamma distribution with log link was used to deal with skewed but non-zero inflated data. In cases where skewness was due to many zeros (e.g., no growth), quasipoisson was used instead.
Effect sizes of fungicide concentrations were calculated for each isolate using the natural logarithm of the response ratios of the treatment mean and control mean, LRR = ln (
Finally, the phylogenetic signal of the treatment effects of the different concentrations were analyzed based on Blomberg’s K statistics (Blomberg et al., 2003; Keck et al., 2016) using the function phyloSignal (Keck et al., 2016). All statistical analysis were done using R version 4.0.5 (R Core Team, 2021).
Results
The broth microdilution assay provided a range of minimum concentration that inhibits the growth of the eight isolates from the three phyla (Table 1; Supplementary Figure S2). These MICs were used as the basis for the range of concentrations used to determine the effect of fungicides on the growth of soil fungi in terms of colony extension rate. The MIC50 of the representative isolates displayed the variability in tolerance and sensitivity among the isolates in this pre-test.
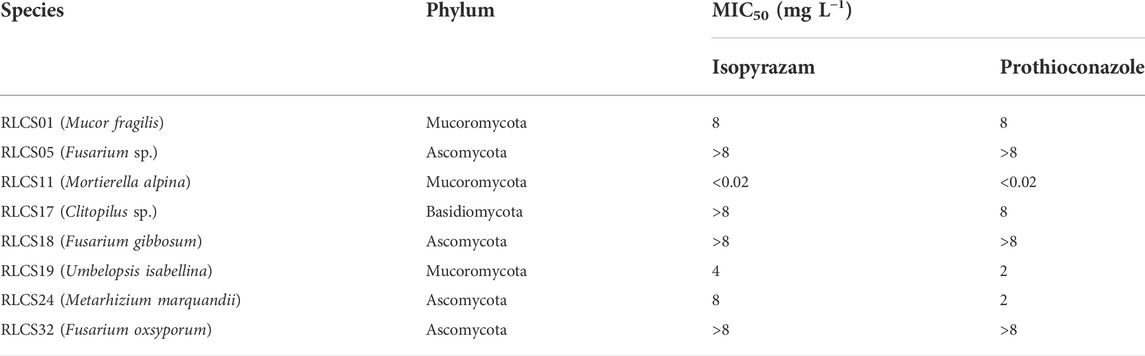
TABLE 1. Minimum inhibitory concentrations (MIC50) of the representative isolates belonging to three different phyla.
In the absence of fungicides, colony extension rate of the 31 soil fungi tested ranged from 7.2 to 1,341.07 mm2·day−1 with the fastest growing species belonging to phylum Mucoromycota (Supplementary Figure S3). Overall, the two fungicides did not differ (p = 0.82) in their effect on fungal colony extension rate (Figure 1).
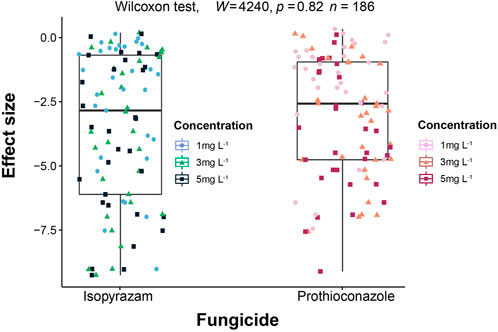
FIGURE 1. Comparison of the effect sizes on fungal colony extension rate between isopyrazam and prothioconazole. Effect sizes (ES) are calculated as the natural logarithm of the response ratios of the treatment and control mean values (LRR = ln (
However, when effects were compared at the phylum level under different fungicide concentrations, results revealed different responses to the two fungicides. Among the three phyla, the strongest negative effect of prothioconazole occurred for Basidiomycota (Figure 2). On the other hand, Mucoromycota were least negatively affected by this fungicide. At 1 mg·L−1, effect sizes on Mucoromycota and Ascomycota were not significantly different (p = 0.092, Figure 2; Supplementary Table S2). However, at higher concentrations, the effects differ significantly between these two phyla with greater negative effect on the latter (3 mg·L−1: p= <0.001 and 5 mg·L−1: p = <0.001, Supplementary Table S2). With isopyrazam, Basidiomycota remained the most negatively affected under all concentrations. The fungicide effect on phylum Mucoromycota and Ascomycota varied widely but there was no significant difference between these two phyla (1 mg·L−1: p = 0.834; 3 mg·L−1: p = 0.516 and 5 mg·L−1: p = 0.539, Supplementary Table S2).
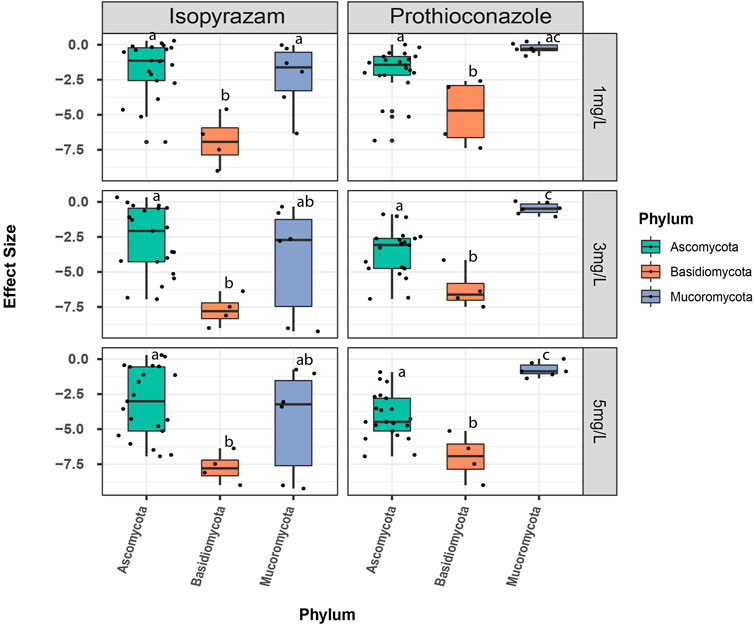
FIGURE 2. Comparison of effect sizes of the fungicide concentrations at the phylum level. Effect sizes (ES) are calculated as the natural logarithm of the response ratios of the treatment and control mean values (LRR = ln (
Looking into the individual isolates, the increasing concentration of fungicide elicited variable responses from the 31 fungi belonging to different classes and orders from these three fungal phyla. In most species tested, colony extension rate (CER) decreased with increasing fungicide concentration (Figure 3). Five isolates (RLCS09, RLCS16, RLCS23, RLCS28, and RCLCS29) were highly sensitive to isopyrazam, such that the addition of 1 mg·L−1 completely inhibited their growth. Similarly, 1 mg·L−1 of prothioconazole inhibited the growth of four isolates, namely, RLCS27, RLCS28, RLCS29, and RLCS31. In general, isolates belonging to Basidiomycota (RLCS09, RLCS16, RLCS17, and RLCS29) were the most sensitive to both fungicides. Their CER were reduced significantly at all concentrations. Fungi in Ascomycota also showed high sensitivity to the two fungicides with RLCS23 being the most sensitive. In the Ascomycota, isolates belonging to class Sordariomycetes (RLCS05, RLCS07, RLCS13, RLCS18, RLCS24, RLCS25, and RLCS32) were the most tolerant to isopyrazam together with RLCS12. Contrary to the predicted outcome, RLCS25 (Gliomastix sp.) had higher CER at 5 mg·L−1 of isopyrazam, resulting in a positive effect size. Similarly, CER of RLCS31 (Cyphellophora sp.) also slightly increased when treated with isopyrazam. Under prothioconazole treatment, fungi belonging to phylum Mucoromycota (RLCS01, RLCS02, RLCS03, RLCS11, RLCS15, and RLCS19) were the most tolerant whereas greatest reduction was detected for RLCS27, RLCS28, RLCS29, and RLCS31. Interestingly, isolate RLCS19 (Umbelopsis isabellina) even had higher CER at all concentrations of prothioconazole compared with the control (Figure 3).
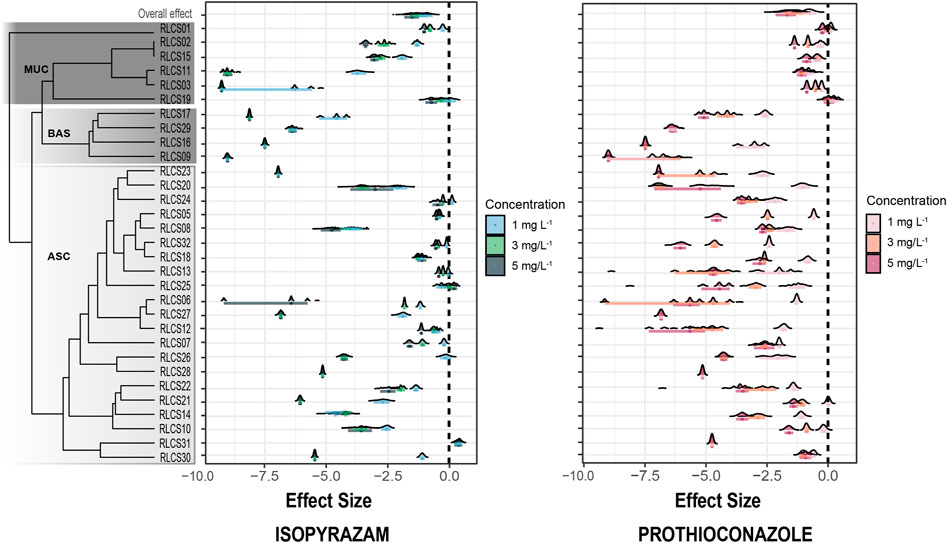
FIGURE 3. Effect sizes of fungicide treatment on the colony extension rate (CER) of individual isolates. Effect sizes (ES) are calculated as the natural logarithm of the response ratios of the treatment and control mean values (LRR = ln (
To evaluate whether the effect of fungicide was more pronounced for fast-growing fungi than for slow-growing isolates, the relationship between the fungicide effect size and the colony extension rate under control conditions was investigated. A Kendall test revealed a weak, non-significant correlation between the growth rate and the treatment effects (Figure 4). The same relationship was observed when phylogenetic dependence was considered (Isopyrazam at 1 mg·L−1: R2 = 0.057, p = 0.1036; at 3 mg·L−1: R2 = 0.052, p = 0.1141; at 5 mg·L−1: R2 = −0.029, p = 0.722; and Prothioconazole at 1 mg·L−1: R2 = 0.1021, p = 0.045; 3 mg·L−1: R2 = −0.005, p = 0.365; 5 mg·L−1: R2 = 0.095, p = 0.051, Supplementary Table S3).
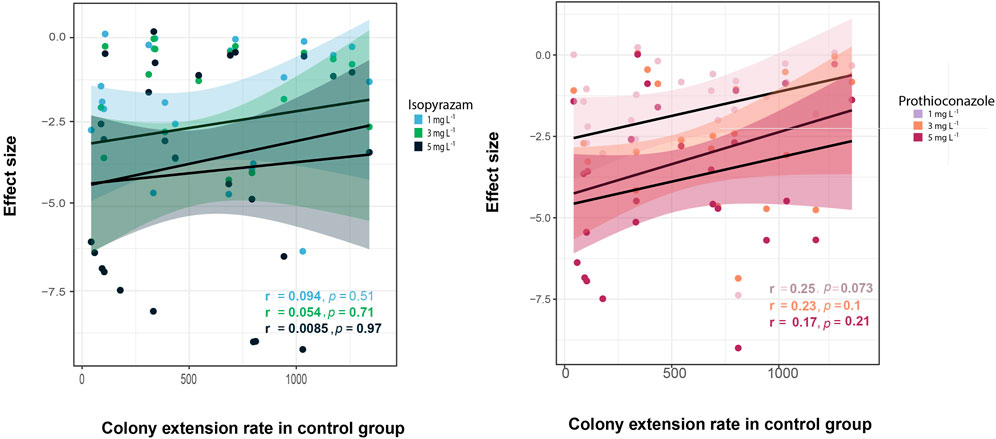
FIGURE 4. Correlation between the effects sizes of the fungicides and the colony extension rate (mm2·day−1) in the control group. Dots represent effect sizes for individual isolates under certain concentration of isopyrazam (blue) and prothioconazole (light red). Increasing fungicide concentration is depicted by increasing color intensity. Linear regression (black lines) and confidence interval (colored band) in every treatment are presented. Correlation coefficients (r) and significance level (p < 0.05) are included in each panel.
Effect sizes of fungicide treatments on colony extension rate were also assessed for phylogenetic signal using Blomberg’s K statistics (Supplementary Table S4). Moderate phylogenetic signals were detected on the effect sizes on colony extension rate of isopyrazam under all concentration treatments (1 mg·L−1: K = 0.474, p = 0.016; 3 mg·L−1: K = 0.465, p = 0.014; 5 mg·L−1: K = 0.512, p = 0.003) as well as at lower concentration of prothioconazole (1 mg·L−1: K = 0.349, p = 0.087). At higher concentrations of prothioconazole, high phylogenetic signals were detected (3 mg·L−1: K = 0.902, p = 0.001; 5 mg·L−1: K = 0.925, p = 0.001).
In the parallel experiment using 30°C and 35°C, prothioconazole and isopyrazam did not differ in their over-all effect sizes despite the difference in their modes of action (Supplementary Figure S4). However, there was a remarkable decrease in colony extension rate at higher temperature, with the greatest reduction seen at 35°C even without the addition of fungicides. The reduction was further exacerbated by the addition of fungicides in both temperature conditions (Supplementary Table S6). These results provide insight on how increasing temperature in the environment might aggravate the impact of fungicides on non-target soil organisms.
Additional observations
Although not quantified, it was also observed that mycelium pigmentation changed in the presence of fungicides as the case of isolates RLCS05, RLCS06, RLCS10, and RLCS12 (Supplementary Figure S4). Mycelia color of these isolates became lighter when grown on either prothioconazole or isopyrazam. In particular, isolates RLCS06 and RLCS12 form visible asci on their mycelium (Camenzind et al., 2022) but the application of fungicides could have suppressed the formation of ascospores resulting in reduced coloration.
Discussion
Differential susceptibility to the fungicides
Despite being isolated from the same grassland ecosystem and presumed to not have been particularly exposed to fungicides previously, these saprobic, non-target soil fungi have a wide range of tolerance and sensitivity to fungicides. The lowest concentration that inhibits 50% of growth (MIC50) based on the reduction in turbidity (OD reading), ranged from <0.02 to >8.0 mg·L−1. This differential susceptibility implies that exposure to fungicides could potentially affect diversity and structure of microbial communities in the soil. Although at low concentrations, some pesticides may persist in soil for years (Chiaia-Hernandez et al., 2017). Repeated fungicide application may lead to accumulation in soil subsequently affecting beneficial soil organisms (Lewandowska and Walorczyk, 2010; Pelosi et al., 2021). In a study conducted in European agricultural topsoil, as low as 0.1 mg·kg−1 to more than 2.0 mg·kg−1 of different pesticides were detected either as a single residue or a mixture of several pesticides, of which broad-spectrum fungicides were among the most frequently found (Silva et al., 2019). These concentrations are comparable to the experimental concentrations used in this study.
Implication of differential sensitivity on potential soil-borne pathogens
Although isolated from grassland ecosystem, some of these fungi are potential plant pathogens such as RLCS01 - Mucor fragilis (Ghuffar et al., 2018; Wu X et al., 2021; Khan and Javaid, 2022), RLCS05, RCLCS08, RLCS13, RLCS18, and RLCS32—Fusarium sp. (Michielse and Rep, 2009; Nag et al., 2022), RLCS07—Truncatella angustata (Arzanlou et al., 2013; Wenneker et al., 2016; Sokolova et al., 2022), RLCS10—Alternaria sp. (RLCS10, De Mers, 2022; Htun et al., 2022; Martinko et al., 2022; Xu et al., 2022), RLCS22—Paraphoma chrysanthemicola (Wang et al., 2022), and RLCS23—Paramyrothecium sp. (Haudenshield et al., 2018; Pinruan et al., 2022; Withee et al., 2022),. These isolates were more tolerant to the sub-lethal concentrations of either prothioconazole or isopyrazam used in this study. Thus, differential sensitivity among isolates implies that growth and proliferation of potential pathogens that are more tolerant to the fungicides may be favored over the less tolerant ones.
Isolate-specific responses on sub-lethal fungicide concentrations
In a similar study involving different azole derivatives, fungicide efficiency also varied against azole-sensitive fungal strains ranging from 0.075 to 8 mg·L−1 (Hof, 2001). As hypothesized, the increasing fungicide concentrations led to a decrease in colony extension rate in most of the isolates. However, in a few instances, isolates had a slightly increased growth rate (CER) when grown on fungicide-amended PDA such as the case of RLCS25, RLCS31 in isopyrazam and RLCS19 in prothioconazole. One possible explanation for this response is the use of sub-lethal concentrations of fungicides. Stimulatory effects of sub-lethal concentrations of fungicides have been reported by either increasing mycelial growth (Garzón et al., 2011; Zhou et al., 2014) or virulence (Audenaert et al., 2010; Cong et al., 2019) of fungal pathogens. Sub-lethal doses of prothioconazole also induced H2O2 production, which in turn trigger the production of mycotoxin (Audenaert et al., 2010).
Isolates of the phylum Mucoromycota showed remarkable tolerance to prothioconazole, a fungicide that inhibits ergosterol synthesis in the cell membrane of fungi. This observed tolerance can be intrinsic to these isolates due to the lack of ergosterol in most of them (Weete et al., 2010). However, in the case of Mucorales, despite ergosterol being the major sterol in its membrane (Weete et al., 2010; Müller et al., 2018), high tolerance to the azole fungicide was observed as in the case of RLCS01 (Mucor fragilis). As previously reported in some clinically important Mucoromycetes, azoles such as isavuconazole, voriconazole, itraconazole, and posaconazole are also inactive against most tested species (Alastruey-Izquierdo et al., 2009; Caramalho et al., 2017; Borman et al., 2021). Mucor sp. In particular had >16 mg·L−1 MIC90 for all azoles mentioned (Borman et al., 2021). This response implies other mechanisms are in place in these isolates to evade fungicide effects without necessarily developing fungicide resistance. One of which is altering the ergosterol biosynthetic pathways to convert intermediate products to ergosterol preventing severe damage to the membrane (Müller et al., 2018). For example, Müller and his team (2018) reported that the Mucorales species Rhizopus arrhizus has an increased actual amount of ergosterol relative to the biomass when exposed to sub-lethal concentration of posaconazole. They noted an increase in eburicol, another ergosterol precursor aside from lanosterol during the treatment. This led to an increased total amount of ergosterol, preventing detrimental damage to the membrane, and consequently overcoming fungicide effects.
Fungi in the Basidiomycota were the most sensitive to both isopyrazam and prothioconazole. The isolates, all in the class Agaricomycetes, are known to possess ergosterol as the major sterol in their membrane (Weete et al., 2010; McLaughlin et al., 2015). Basidiomycetes are essential drivers of carbon cycling in grassland ecosystem (Floudas et al., 2020; Lodato et al., 2021). Their lignolytic ability are responsible for the degradation of grass lignins, an important input in grasslands. Lignin decomposition consequently impact the quality and quantity of organic matter in the soil (Gilmullina et al., 2021) as well as the soil structure by enhancing soil aggregate formation and stability (Caesar-TonThat and Cochran, 2000; Lehmann et al., 2020). Hence, the high sensitivity of Basidiomycota to both fungicides may negatively impact these soil processes due to the eventual decline of these important terrestrial decomposers. Finally, Ascomycota had varying susceptibility to prothioconazole most likely due to the varying biochemical characteristics including the major sterol produced by the fungi belonging to this group (Weete et al., 2010). Some isolates were also observed to have tolerance to isopyrazam. Succinate dehydrogenase inhibitors (SDHIs) like isopyrazam block the conversion of succinate to fumarate in the tricarboxylic acid cycle (TCA), thereby inhibiting respiration (Sierotzki and Scalliet, 2013). Although not tested in this study, several mechanisms of avoiding the toxic effects of fungicides are possible (Deising, Reimann and Pascholati, 2008). These mechanisms include efflux transporters that pump the fungicide molecules to the extracellular space, modifying plasma membranes to reduce permeability, synthesizing enzymes that degrade the fungicide or utilization of alternative metabolic pathways (Del Sorbo, et al., 2000; Löffler et al., 2000; Schnabel and Jones, 2001; Steinfeld et al., 2001; Deising, Reimann and Pascholati, 2008).
This isolate-specific responses to fungicide treatment did not support our hypothesis that slow-growing fungi are more tolerant to stress (Maynard et al., 2019), hence will be less affected by the fungicides than the fast-growing isolates. Our results rather showed a weak correlation between the growth strategy and treatment effect. Therefore, the effect magnitude of the fungicide treatment is not dependent solely on the colony extension rate. Analysis using Blomberg’s K statistics revealed varying levels of phylogenetic signals across the different treatment conditions. Moderate signals were detected on low concentration (1 mg·L−1) of prothioconazole and all concentrations of isopyrazam. Higher concentrations of prothioconazole (3 and 5 mg·L−1), on the other hand, presented higher phylogenetic signals indicating that the treatment effect of this fungicide is phylogenetically conserved. This can be explained by the cell membrane composition of the isolates wherein some groups possess ergosterol while the others do not. On the other hand, isopyrazam targets the enzyme essential for respiration common to all fungi.
Other non-target effects of fungicides
Also in the study, other non-target effects of fungicides were observed on some of the isolates. Changes in pigmentation was observed in some isolates (RLCS05, RLCS06, RLCS10, and RLCS12) treated with either isopyrazam or prothioconazole. Spore production and asci formation by RLCS06 and RLCS12 (Chaetomium angustispirale) may have been reduced upon fungicide exposure similar to several reported studies (Buck et al., 2011; Celar and Kos, 2016). On the contrary, Han et al. (2022) found that sub-lethal concentrations of two 14a-demethylase inhibitor fungicides promoted the spore production of the fungus Colletotrichum spp. This two-way effect of fungicide on growth and reproduction of fungi emphasized the need for critical evaluation of fungicide prior to usage. Aside from potential reduction in spores, species of Chaetomium, Fusarium and Alternaria were also reported to produce the dark pigment, melanin (Anitha and Murugesan, 2008; Hu et al., 2012; Fernandes et al., 2016; Frandsen et al., 2016). Melanin provides protection against environmental stresses such as temperature, UV light and chemicals (Narsing Rao et al., 2017). Although not further explored in this study, the fungicides may have inhibited the synthesis of this pigment resulting in their noticeable decrease. Such an effect was also observed for several isolates of Penicillium, Aspergillus and other fungal species treated with fungicides due to the inhibition of 1,8-dihydroxynaphthalene (DHN)-melanin pathway (Wheeler and Kuch, 1995; Hamada et al., 2014). Several fungicides like tricyclazole, pyroquilon, phthalide, and carpropamid are used in agriculture due to their activity against melanin biosynthesis (Motoyama and Yamaguchi, 2003; Adeniyi et al., 2021; Fungicide Resistance Action Committee, 2022). Interestingly, in another study by Golubeva et al. (2020) on the same set of fungi, RLCS05 and RLCS12 were found to have darker mycelium when treated with copper suggesting that different environmental stressors produce different effects on the mycelial pigment. These results suggest that the change in pigmentation may be a signature non-target effects of chemical stressors, in this case, fungicide on some fungal species. With the limited studies currently available, these important responses are often overlooked in the study of the impact of fungicides on soil microbiota.
Conclusion
In conclusion, the result of this study highlights the non-target impact of fungicides on soil fungi isolated from a grassland community inside a nature conservation area. The variable responses to two currently used agricultural fungicides are likely to underpin possible shifts in fungal community structure in the field. Due to innate sensitivity to specific fungicides, important soil fungi involved in decomposition and nutrient recycling may be eliminated completely or outcompeted by the more tolerant strains. In contrast, potential plant pathogens that showed high tolerance to the fungicides could possibly dominate the community. It is also important to emphasize the observed increase in growth rate of some isolates under fungicide treatment. Further investigation on the mechanism involved in the positive effect size of fungicide is necessary to understand whether stimulatory effects of sub-lethal concentrations of fungicides is truly at play in this scenario. It is also interesting to investigate the role of the evolution of volatile compounds in the presence of fungicides and how this affects interactions among microbes in the community. As studies on temperature effects on the development of antimicrobial resistance of pathogens are increasing, it is also important not to overlook the impact of fungicides on non-target soil microbiota in future climate scenarios. Our controlled exposure study demonstrates the existence of differential effects of fungicides on non-target organisms, in this case soil fungi, that are essential for maintaining soil health.
Data availability statement
Original datasets are available in a publicly accessible repository: The original contributions presented in the study are publicly available. This data can be found here: https://doi.org/10.6084/m9.figshare.20473161.v2.
Author contributions
JC, TC, and MR designed the study. JC carried out the experiments, analyzed the data and wrote the first draft of the paper. TC assisted in analyzing the data. All authors revised the manuscript.
Funding
We acknowledge support by the Open Access Publication Initiative of Freie Universität Berlin. This work was supported by the European Research Council (ERC; Advanced Grant Number 694368). JC acknowledges support from the German Academic Exchange Service (DAAD).
Conflict of interest
The authors declare that the research was conducted in the absence of any commercial or financial relationships that could be construed as a potential conflict of interest.
Publisher’s note
All claims expressed in this article are solely those of the authors and do not necessarily represent those of their affiliated organizations, or those of the publisher, the editors and the reviewers. Any product that may be evaluated in this article, or claim that may be made by its manufacturer, is not guaranteed or endorsed by the publisher.
Supplementary material
The Supplementary Material for this article can be found online at: https://www.frontiersin.org/articles/10.3389/fenvs.2022.1020465/full#supplementary-material
References
Adeniyi, D. O., Kunwar, D., Dongo, L. N., Animasaun, D. A., and Aravind, T. (2021). “New-Generation fungicides for sustainable production and disease suppression,” in Emerging trends in plant pathology. (Singapore: Springer Nature Singapore Pte Ltd. 2021), 249–256.
Ajigboye, O. O., Murchie, E., and Ray, R. V. (2014). Foliar application of isopyrazam and epoxiconazole improves photosystem II efficiency, biomass and yield in winter wheat. Pestic. Biochem. Physiol. 114 (1), 52–60. doi:10.1016/J.PESTBP.2014.07.003
Alastruey-Izquierdo, A., Castelli, M. V., Cuesta, I., Monzon, A., Cuenca-Estrella, M., and Rodriguez-Tudela, J. L. (2009). Activity of posaconazole and other antifungal agents against mucorales strains identified by sequencing of internal transcribed spacers. Antimicrob. Agents Chemother. 53 (4), 1686–1689. doi:10.1128/AAC.01467-08
Andrade-Linares, D. R., Veresoglou, S. D., and Rillig, M. C. (2016). Temperature priming and memory in soil filamentous fungi. Fungal Ecol. 21, 10–15. doi:10.1016/j.funeco.2016.02.002
Anitha, R., and Murugesan, K. (2008). Melanin production in Alternaria helianthi. Archives Phytopathology And Plant Prot. 41 (5), 360–364. doi:10.1080/03235400600796695
Arzanlou, M., Narmani, A., Moshari, S., Khodaei, S., and Babai-Ahari, A. (2013). Truncatella angustata associated with grapevine trunk disease in Northern Iran. Archives Phytopathology Plant Prot. 46, 1168–1181. doi:10.1080/03235408.2012.761417
Audenaert, K., Callewaert, E., Höfte, M., De Saeger, S., and Haesaert, G. (2010). Hydrogen peroxide induced by the fungicide prothioconazole triggers deoxynivalenol (DON) production by Fusarium graminearum. BMC Microbiol. 10, 112–114. doi:10.1186/1471-2180-10-112
Baćmaga, M., Wyszkowska, J., and Kucharski, J. (2020). Response of soil microorganisms and enzymes to the foliar application of Helicur 250 EW fungicide on Horderum vulgare L. Chemosphere 242, 125163. doi:10.1016/j.chemosphere.2019.125163
Baćmaga, M., Wyszkowska, J., and Kucharski, J. (2019). The biochemical activity of soil contaminated with fungicides. J. Environ. Sci. Health Part B 54 (4), 252–262. doi:10.1080/03601234.2018.1553908
Baćmaga, M., Wyszkowska, J., and Kucharski, J. (2016). The effect of the Falcon 460 EC fungicide on soil microbial communities, enzyme activities and plant growth. Ecotoxicology 25, 1575–1587. doi:10.1007/s10646-016-1713-z
Balouiri, M., Sadiki, M., and Ibnsouda, S. K. (2016). Methods for in vitro evaluating antimicrobial activity: A review. J. Pharm. Anal. 6 (2), 71–79. doi:10.1016/j.jpha.2015.11.005
Blomberg, S. P., Garland, T., and Ives, A. (2003). Testing for phylogenetic signal in comparative data: Behavioral traits are more labile. Evolution 57 (4), 717–745. doi:10.1111/j.0014-3820.2003.tb00285.x
Borman, A. M., Fraser, M., Patterson, Z., Palmer, M. D., and Johnson, E. M. (2021). In vitro antifungal drug resistance profiles of clinically relevant members of the mucorales (Mucoromycota) especially with the newer triazoles. J. Fungi (Basel). 7, 271. doi:10.3390/jof7040271
Brühl, C. A., Bakanov, N., Köthe, S., Eichler, L., Sorg, M., Hörren, T., et al. (2021). Direct pesticide exposure of insects in nature conservation areas in Germany. Sci. Rep. 11, 24144. doi:10.1038/s41598-021-03366-w
Buck, J. W., Wise, K., and Dong, W. (2011). Effect of postsymptom application of fungicides on urediniospore production by Puccinia triticina on wheat and P. hemerocallidis on daylily. Plant Dis. 95 (3), 325–330. doi:10.1094/pdis-09-10-0646
Bünemann, E. K., Schwenke, G. D., and Van Zwieten, L. (2006). Impact of agricultural inputs on soil organisms - a review. Soil Res. 44 (4), 379–406. doi:10.1071/SR05125
Caesar-TonThat, T. C., and Cochran, V. (2000). Soil aggregate stabilization by a saprophytic lignin-decomposing basidiomycete fungus I. Microbiological aspects. Biol. Fertil. Soils 32, 374–380. doi:10.1007/s003740000263
Caldwell, C. D., MacDonald, D., Jiang, Y., Cheema, M. A., and Li, J. (2017). Effect of fungicide combinations for FHB control on disease incidence, grain yield and quality of winter wheat, spring wheat and barley. Can. J. Plant Sci. 97 (6), 1036–1045. doi:10.1139/cjps-2017-0001
Camenzind, T., Lehmann, A., Ahland, J., Rumpel, S., and Rillig, M. C. (2020). Trait-based approaches reveal fungal adaptations to nutrient-limiting conditions. Environ. Microbiol. 22 (8), 3548–3560. doi:10.1111/1462-2920.15132
Camenzind, T., Weimershaus, P., Lehmann, A., Aguilar-Trigueros, C., and Rillig, M. C. (2022). Soil fungi invest into asexual sporulation under resource scarcity, but trait spaces of individual isolates are unique. Environ. Microbiol. 24 (7), 2962–2978. doi:10.1111/1462-2920.16012
Caramalho, R., Tyndall, J. D. A., Monk, B. C., Larentis, T., Lass-Flörl, C., and Lackner, M. (2017). Intrinsic short-tailed azole resistance in mucormycetes is due to an evolutionary conserved aminoacid substitution of the lanosterol 14α-demethylase. Sci. Rep. 7, 15898. doi:10.1038/S41598-017-16123-9
Carpio, M. J., Garcia-Delgado, C., Marin-Benito, J. M., Sanchez-Martin, M. J., and Rodriguez-Cruz, M. S. (2020). Soil microbial community changes in a field treatment with chlorotoluron, flufenacet and diflufenican and two organic amendments. Agronomy 10 (8), 1166. doi:10.3390/agronomy10081166
Cassman, N. A., Leite, M. F. A., Pan, Y., de Hollander, M., van Veen, J. A., and Kuramae, E. E. (2016). Plant and soil fungal but not soil bacterial communities are linked in long-term fertilized grassland. Sci. Rep. 6, 23680–23711. doi:10.1038/srep23680
Celar, F. A., and Kos, K. (2016). Effects of selected herbicides and fungicides on growth, sporulation and conidial germination of entomopathogenic fungus Beauveria bassiana. Pest Manag. Sci. 72 (11), 2110–2117. doi:10.1002/PS.4240
Ceulemans, T., Van Geel, M., Jacquemyn, H., Boeraeve, M., Plue, J., Saar, L., et al. (2019). Arbuscular mycorrhizal fungi in European grasslands under nutrient pollution. Glob. Ecol. Biogeogr. 28 (12), 1796–1805. doi:10.1111/geb.12994
Chen, J., Zhou, L., Din, I. U., Arafat, Y., Li, Q., Wang, J., et al. (2021). Antagonistic activity of Trichoderma spp. against Fusarium oxysporum in rhizosphere of Radix pseudostellariae triggers the expression of host defense genes and improves its growth under long-term monoculture system. Front. Microbiol. 12, 579920. doi:10.3389/fmicb.2021.579920
Chiaia-Hernandez, A. C., Keller, A., Wächter, D., Steinlin, C., Camenzuli, L., Hollender, J., et al. (2017). Long-term persistence of pesticides and TPs in archived agricultural soil samples and comparison with pesticide application. Environ. Sci. Technol. 51 (18), 10642–10651. doi:10.1021/acs.est.7b02529
Clinical and Laboratory Standards Institute.M38-A2 Reference method for broth dilution antifungal susceptibility testing of filamentous fungi; approved standard-second edition (2008). Available at: www.clsi.org. [Accessed: 25 May 2022].
Cong, M., Zhang, B., Zhang, K., Guoqing, L., and Zhu, F. (2019). Stimulatory effects of sublethal doses of carbendazim on the virulence and sclerotial production of Botrytis cinerea. Plant Dis. 103 (9), 2385–2391. doi:10.1094/PDIS-01-19-0153-RE
Daniel, D. W., Smith, L. M., Belden, J. B., McMurry, S. T., and Swain, S. (2015). Effects of land-use change and fungicide application on soil respiration in playa wetlands and adjacent uplands of the U.S. High Plains. Sci. Total Environ. 514, 290–297. doi:10.1016/j.scitotenv.2015.01.066
De Mers, M. (2022). Alternaria alternata as endophyte and pathogen. Microbiology 168, 3. doi:10.1099/mic.0.001153
Deising, H. B., Reimann, S., and Pascholati, S. F. (2008). Mechanisms and significance of fungicide resistance. Braz. J. Microbiol. 39 (2), 286–295. doi:10.1590/s1517-83822008000200017
Del Sorbo, G., Schoonbeek, H. J., and De Waard, M. A. (2000). Fungal transporters involved in efflux of natural toxic compounds and fungicides. Fungal Genet. Biol. 30 (1), 1–15. doi:10.1006/fgbi.2000.1206
Ding, J., Travers, S. K., and Eldridge, D. J. (2022). Microbial communities are associated with indicators of soil surface condition across a continental gradient. Geoderma 405, 115439. doi:10.1016/j.geoderma.2021.115439
Edlinger, A., Garland, G., Hartman, K., Banerjee, S., Degrune, F., Garcia-Palacios, P., et al. (2022). Agricultural management and pesticide use reduce the functioning of beneficial plant symbionts. Nat. Ecol. Evol. 6, 1145–1154. doi:10.1038/s41559-022-01799-8
Edwards, S. G., and Godley, N. P. (2010). Reduction of Fusarium head blight and deoxynivalenol in wheat with early fungicide applications of prothioconazole. Food Addit. Contam. Part A 27 (5), 629–635. doi:10.1080/19440040903515942
Espinel-Ingroff, A., and Cantón, E. (2007). in ‘Antifungal susceptibility testing of filamentous fungi’ in antimicrobial susceptibility testing protocols. Editors R. Schwalbe, L. Steele-Moore, and A. C. Goodwin (Boca Raton: CRC Press), 209–241.
Eurostat.(2020). Sales of pesticides in the EU. Available at: https://ec.europa.eu/eurostat/web/products-eurostat-news/-/ddn-20200603-1 [Accessed 5 March 2022].
Fernandes, C., Prados-Rosales, R., Silva, B. M. A., Nakouzi-Naranjo, A., Zuzarte, M., Chatterjee, S., et al. (2016). ‘Activation of melanin synthesis in Alternaria infectoria by antifungal drugs’, Antimicrob. Agents Chemother. 60 (3), 1646–1655. doi:10.1128/AAC.02190-15
Fierer, N. (2017). Embracing the unknown: disentangling the complexities of the soil microbiome. Nat. Rev. Microbiol. 15 (10), 579–590. doi:10.1038/nrmicro.2017.87
Floudas, D., Bentzer, J., Ahrén, D., Johansson, T., Persson, P., and Tunlid, A. (2020). Uncovering the hidden diversity of litter-decomposition mechanisms in mushroom-forming fungi. ISME J. 14, 2046–2059. doi:10.1038/s41396-020-0667-6
Fones, H. N., Bebber, D. P., Chaloner, T. M., Kay, W. T., Steinberg, G., and Gurr, S. J. (2020). Threats to global food security from emerging fungal and oomycete crop pathogens. Nat. Food 1 (6), 332–342. doi:10.1038/s43016-020-0075-0
Frąc, M., Hannula, S. E., Belka, M., and Jędryczka, M. (2018). Fungal biodiversity and their role in soil health. Front. Microbiol. 9, 707. doi:10.3389/fmicb.2018.00707
Frandsen, R. J. N., Rasmussen, S. A., Knudsen, P. B., Uhlig, S., Petersen, D., Lysøe, E., et al. (2016). Black perithecial pigmentation in Fusarium species is due to the accumulation of 5-deoxybostrycoidin-based melanin. Sci. Rep. 6, 26206. doi:10.1038/srep26206
Frank, D. A., Gehring, C. A., Machut, L., and Phillips, M. (2003). Soil community composition and the regulation of grazed temperate grassland. Oecologia 137 (4), 603–609. doi:10.1007/S00442-003-1385-2
Fungicide Resistance Action Committee. (2022). ‘FRAC code list FRAC code list ©* Available at: https://www.frac.info/docs/default-source/publications/frac-code-list/frac-code-list-2022--final.pdf?sfvrsn=b6024e9a_2. [Accessed June 06, 2022].
Garzón, C. D., Molineros, J. E., Yánez, J. M., Flores, F. J., Jiménez-Gasco, M. D. M., and Woorman, G. W. (2011). Sublethal doses of mefenoxam enhance Pythium damping-off of Geranium. Plant Dis. 95 (10), 1233–1238. doi:10.1094/PDIS-09-10-0693
Ghuffar, S., Irshad, G., Aslam, M. F., Naz, F., Mehmood, N., Hamzah, A. M., et al. (2018). First report of Mucor fragilis causing bunch rot of grapes in Punjab, Pakistan. Plant Dis. 102 (9), 1858. doi:10.1094/PDIS-12-17-2032-PDN
Gilmullina, A., Rumpel, C., Klumpp, K., and Chabbi, A. (2021). Do grassland management practices affect soil lignin chemistry by changing the composition of plant-derived organic matter input? Plant Soil 469, 443–455. doi:10.1007/s11104-021-05174-7
Golubeva, P., Ryo, M., Muller, L. A. H., Ballhausen, M. B., Lehmann, A., Sosa-Hernandez, M. A., et al. (2020). Soil saprobic fungi differ in their response to gradually and abruptly delivered copper. Front. Microbiol. 11 (1195), 1195–1197. doi:10.3389/fmicb.2020.01195
Hamada, T., Asanagi, A., Satozawa, T., Araki, N., Banba, S., Higashimura, N., et al. (2014). Action mechanism of the novel rice blast fungicide tolprocarb distinct from that of conventional melanin biosynthesis inhibitors. J. Pestic. Sci. 39 (3), 152–158. doi:10.1584/jpestics.d14-033
Han, Y., Zeng, X., Guo, C., Zhang, Q., Chen, F., Ren, L., et al. (2022). Reproduction response of Colletotrichum fungi under the fungicide stress reveals new aspects of chemical control of fungal diseases. Microb. Biotechnol. 15 (2), 431–441. doi:10.1111/1751-7915.13754
Haudenshield, J. S., Miranda, C., and Hartman, G. L. (2018). First report of Paramyrothecium roridum causing myrothecium leaf spot on soybean in Africa. Plant Dis. 102 (12), 2638. doi:10.1094/PDIS-04-18-0624-PDN
He, L., Mazza Rodriguez, J. L., Soudzilovskaia, N. A., Barceló, M., Olsson, P. A., Song, C., et al. (2020). Global biogeography of fungal and bacterial biomass carbon in topsoil. Soil Biol. Biochem. 151, 108024. doi:10.1016/j.soilbio.2020.108024
Hof, H. (2001). Critical annotations to the use of azole antifungals for plant protection. Antimicrob. Agents Chemother. 45 (11), 2987–2990. doi:10.1128/AAC.45.11.2987-2990.2001
Htun, A. A., Liu, H. F., He, L., Xia, Z. Z., Aung, S. L. L., and Deng, J. X. (2022). New species and new record of Alternaria from onion leaf blight in Myanmar. Mycol. Prog. 21, 59–69. doi:10.1007/S11557-021-01765-x
Hu, Y., Hao, X., Lou, J., Zhang, P., Pan, J., and Zhu, X. (2012). A PKS gene, pks-1, is involved in chaetoglobosin biosynthesis, pigmentation and sporulation in Chaetomium globosum. Sci. China Life Sci. 55 (12), 1100–1108. doi:10.1007/S11427-012-4409-5
Huhe, Chen X., Hou, F., Wu, Y., and Cheng, Y. (2017). Bacterial and fungal community structures in Loess Plateau grasslands with different grazing intensities. Front. Microbiol. 8, 606. doi:10.3389/fmicb.2017.00606
Jiang, S., An, X., Shao, Y., Kang, Y., Chen, T., Mei, X., et al. (2021). Responses of arbuscular mycorrhizal fungi occurrence to organic fertilizer: A meta-analysis of field studies. Plant Soil 469, 89–105. doi:10.1007/s11104-021-05153-y
Kang, F., Yang, B., WujisigulengYang, X., Wang, L., Guo, J., et al. (2020). Arbuscular mycorrhizal fungi alleviate the negative effect of nitrogen deposition on ecosystem functions in meadow grassland. Land Degrad. Dev. 31 (6), 748–759. doi:10.1002/ldr.3491
Karlsson, I., Friberg, H., Steinberg, C., and Persson, P. (2014). Fungicide effects on fungal community composition in the wheat phyllosphere. PLoS ONE 9, e111786. doi:10.1371/journal.pone.0111786
Keck, F., Rimet, F., Bouchez, A., and Franc, A. (2016). phylosignal: an R package to measure, test, and explore the phylogenetic signal. Ecol. Evol. 6 (9), 2774–2780. doi:10.1002/ece3.2051
Khan, I. H., and Javaid, A. (2022). Mucor fragilis causing rot of Seychelles pole bean in Pakistan. Australas. Plant Pathol. 51 (3), 359–362. doi:10.1007/S13313-022-00859-8
Lajeunesse, M. J. (2015). Bias and correction for the log response ratio in ecological meta-analysis. Ecology 96 (8), 2056–2063. doi:10.1890/14-2402.1
Lehmann, A., Zheng, W., Ryo, M., Soutschek, K., Roy, J., Rongstock, R., et al. (2020). Fungal traits important for soil aggregation. Front. Microbiol. 10, 2904. doi:10.3389/fmicb.2019.02904
Lehmann, A., Zheng, W., Soutschek, K., Roy, J., Yurkov, A. M., and Rillig, M. C. (2019). Tradeoffs in hyphal traits determine mycelium architecture in saprobic fungi. Sci. Rep. 9, 141521–141529. doi:10.1038/s41598-019-50565-7
Lewandowska, A., and Walorczyk, S. (2010). Carbendazim residues in the soil and their bioavailability to plants in four successive harvests. Pol. J. Environ. Stud. 19 (4), 757–761.
Liao, L., Wang, X., Wang, J., Liu, G., and Zhang, C. (2021). Nitrogen fertilization increases fungal diversity and abundance of saprotrophs while reducing nitrogen fixation potential in a semiarid grassland. Plant Soil 465, 515–532. doi:10.1007/s11104-021-05012-w
Lodato, M. B., Boyette, J. S., Smilo, R. A., Jackson, C. R., Halvorson, H. M., and Kuehn, K. A. (2021). Functional importance and diversity of fungi during standing grass litter decomposition. Oecologia 195 (2), 499–512. doi:10.1007/s00442-020-04838-y
Löffler, J., Einsele, H., Hebart, H., Schumacher, U., Hrastnik, C., and Daum, G. (2000). Phospholipid and sterol analysis of plasma membranes of azole-resistant Candida albicans strains. FEMS Microbiol. Lett. 185 (1), 59–63. doi:10.1111/j.1574-6968.2000.tb09040.x
Lonergan, E., Pasche, J., Skoglund, L., and Burrows, M. (2015). Sensitivity of Ascochyta species infecting pea, lentil, and chickpea to boscalid, fluxapyroxad, and prothioconazole. Plant Dis. 99 (9), 1254–1260. doi:10.1094/pdis-06-14-0620-re
Manoharan, L., Rosenstock, N. P., Williams, A., and Hedlund, K. (2017). Agricultural management practices influence AMF diversity and community composition with cascading effects on plant productivity. Appl. Soil Ecol. 115, 53–59. doi:10.1016/j.apsoil.2017.03.012
Martinko, K., Ivanković, S., Lazarević, B., Đermić, E., and Đermić, D. (2022). Control of early blight fungus (Alternaria alternata) in tomato by boric and phenylboronic acid. Antibiotics 11 (3), 320. doi:10.3390/antibiotics11030320
Maynard, D. S., Bradford, M. A., Covey, K. R., Lindner, D., Glaeser, J., Talbert, D. A., et al. (2019). Consistent trade-offs in fungal trait expression across broad spatial scales. Nat. Microbiol. 4, 846–853. doi:10.1038/s41564-019-0361-5
McLaughlin, D. J., Arun Kumar, T. K., Blackwell, M., Letcher, P. M., and Roberson, R. (2015). “Subcellular structure and biochemical characters of fungal phylogeny,” in the Mycota: A comprehensive treatise on fungi as experimental systems for basic and applied research:VII systematics and evolution Part B. Editors D. J McLaughlin, and J. W. Spatafora (Berlin: Springer-Verlag Berlin Heidelberg), 229–258.
Meena, R. S., Kumar, S., Datta, R., Lal, R., Vijayakumar, V., Brtnicky, M., et al. (2020). Impact of agrochemicals on soil microbiota and management: A review. Land 9, 34. doi:10.3390/land9020034
Meletiadis, J., Mortensen, K. L., Verweij, P. E., Mouton, J. W., and Arendrup, M. C. (2017). Spectrophotometric reading of EUCAST antifungal susceptibility testing of Aspergillus fumigatus. Clin. Microbiol. Infect. 23 (2), 98–103. doi:10.1016/j.cmi.2016.10.017
Michielse, C. B., and Rep, M. (2009). Pathogen profile update: Fusarium oxysporum. Mol. Plant Pathol. 10 (3), 311–324. doi:10.1111/j.1364-3703.2009.00538.x
Miller, N. F., Standish, J. R., and Quesada-Ocampo, L. M. (2020). Sensitivity of Fusarium oxysporum f. sp. niveum to prothioconazole and pydiflumetofen in vitro and efficacy for fusarium wilt management in watermelon. Plant Health Prog. 13, 13–18. doi:10.1094/php-08-19-0056-rs
Motoyama, T., and Yamaguchi, I. (2003)“Fungicides, melanin biosynthesis inhibitors,” in Encyclopedia of Agrochemicals Vol. 2. Editors J. R. Plimmer, D. W. Gammon, and N. N. Ragsdale (Hoboken, New Jersey: John Wiley & Sons, Inc.), 584–592.
Müller, C., Neugebauer, T., Zill, P., Lass-Flörl, C., Bracher, F., and Binder, U. (2018). Sterol composition of clinically relevant mucorales and changes resulting from posaconazole treatment. Molecules 23, 1218. doi:10.3390/molecules23051218
Nag, P., Paul, S., Shriti, S., and Das, S. (2022). Defence response in plants and animals against a common fungal pathogen, Fusarium oxysporum. Curr. Res. Microb. Sci. 3, 100135. doi:10.1016/j.crmicr.2022.100135
Narsing Rao, M. P., Xiao, M., and Li, W. J. (2017). Fungal and bacterial pigments: Secondary metabolites with wide applications. Front. Microbiol. 8, 1113. doi:10.3389/FMICB.2017.01113
Navrátilová, D., Tláskalová, P., Kohout, P., Dřevojan, P., Fajmon, K., Chytrý, M., et al. (2019). Diversity of fungi and bacteria in species-rich grasslands increases with plant diversity in shoots but not in roots and soil. FEMS Microbiol. Ecol. 95 (1). doi:10.1093/femsec/fiy208
Noel, Z. A., Longley, R., Benucci, G. M. N., Trail, F., Chilvers, M. I., and Bonito, G. (2022). Non-target impacts of fungicide disturbance on phyllosphere yeasts in conventional and no-till management. ISME Commun. 2, 19. doi:10.1038/s43705-022-00103-w
Orme, D., Freckleton, R., Thomas, G., Petzoldt, T., Fritz, S., Isaac, N., et al. (2018). The caper package: Comparative analyses in phylogenetics and evolution in R in R package version 1.0.1. Available at: https://cran.r-project.org/package=caper (Accessed April 22, 2022).
Pánková, H., Dostálek, T., Vazačová, K., and Münzbergová, Z. (2018). Slow recovery of arbuscular mycorrhizal fungi and plant community after fungicide application: An eight-year experiment. J. Veg. Sci. 29 (4), 695–703. doi:10.1111/jvs.12656
Patkowska, E., Jamiołkowska, A., and Mielniczuk, E. (2019). Antagonistic fungi in the soil after Daucus carota L. cultivation. Plant Soil Environ. 65, 159–164. doi:10.17221/22/2019-pse
Pelosi, C., Bertrand, C., Daniele, G., Coeurdassier, M., Benoit, P., Nélieu, S., et al. (2021). Residues of currently used pesticides in soils and earthworms: A silent threat? Agric. Ecosyst. Environ. 305, 107167. doi:10.1016/j.agee.2020.107167
Pérez-Vicente, L. (2012). A holistic integrated management approach to control black sigatoka disease of banana caused by Mycosphaerella fijiensis. Technical Manual (TCP/SLC/3402). USA: Food and Agricultural Oragnization of the United Nations.
Pfaller, M. A., Messer, S. A., and Coffmann, S. (1995). Comparison of visual and spectrophotometric methods of MIC endpoint determinations by using broth microdilution methods to test five antifungal agents, including the new triazole D0870. J. Clin. Microbiol. 33 (5), 1094–1097. doi:10.1128/jcm.33.5.1094-1097.1995
Pfaller, M. A., Messer, S. A., Mills, K., and Bolmström, A. (2000). In vitro susceptibility testing of filamentous fungi: comparison of E-test and reference microdilution methods for determining itraconazole MICs. J. Clin. Microbiol. 38 (9), 3359–3361. doi:10.1128/jcm.38.9.3359-3361.2000
Pinruan, U., Unartngam, J., Unartngam, A., Piyaboon, O., Sommai, S., and Khamsuntorn, P. (2022). Paramyrothecium eichhorniae sp. nov., causing leaf blight disease of water hyacinth from Thailand. Mycobiology 50 (1), 12–19. doi:10.1080/12298093.2022.2027683
R Core Team (2021). R: A language and environment for statistical computing. Vienna, Austria: R Foundation for Statistical Computing. Available at: https://www.R-project.org/.
Revie, N. M., Iyer, K. R., Robbins, N., and Cowen, L. E. (2018). Antifungal drug resistance: evolution, mechanisms and impact. Curr. Opin. Microbiol. 45, 70–76. doi:10.1016/j.mib.2018.02.005
Roman, D. L., Voiculescu, D. I., Filip, M., Ostafe, V., and Isvoran, A. (2021). Effects of triazole fungicides on soil microbiota and on the activities of enzymes found in soil: A review. Agriculture 11, 893. doi:10.3390/agriculture11090893
Sanssené, J., Selim, S., Roisin-Fichter, C., Djerroud, L., Deweer, C., and Halama, P. (2011). Protective and curative efficacy of prothioconazole against isolates of Mycosphaerella graminicola differing in their in vitro sensitivity to DMI fungicides. Pest Manag. Sci. 67 (9), 1134–1140. doi:10.1002/ps.2163
Santísima-Trinidad, A. B. L., Montiel-Rozas, M. d. M., Dieź-Rojo, M. Á., Pascual, A., and Ros, M. (2018). Impact of foliar fungicides on target and non-target soil microbial communities in cucumber crops. Ecotoxicol. Environ. Saf. 166, 78–85. doi:10.1016/j.ecoenv.2018.09.074
Schnabel, G., and Jones, A. L. (2001). The 14 alpha-demethylase (CYP51A1) gene is overexpressed in Venturia inaequalis strains resistant to myclobutanil. Phytopathology 91 (1), 102–110. doi:10.1094/phyto.2001.91.1.102
Sierotzki, H., and Scalliet, G. (2013). A review of current knowledge of resistance aspects for the next-generation succinate dehydrogenase inhibitor fungicides. Phytopathology 103 (9), 880–887. doi:10.1094/PHYTO-01-13-0009-RVW
Silva, V., Mol, H. G. J., Zomer, P., Tienstra, M., Ritsema, C. j., and Geissen, V. (2019). Pesticide residues in European agricultural soils – a hidden reality unfolded. Sci. Total Environ. 653, 1532–1545. doi:10.1016/j.scitotenv.2018.10.441
Sim, J. X. F., Doolette, C. L., Vasileiadis, S., Drigo, B., Wyrsch, E. R., Djordjevic, S. P., et al. (2022). Pesticide effects on nitrogen cycle related microbial functions and community composition. Sci. Total Environ. 807, 150734. doi:10.1016/j.scitotenv.2021.150734
Sokolova, O., Sivicka, I., Krivmane, B., and Kārkliņa, K. (2022). First report of Truncatella angustata causing leaf spot on oregano (Origanum vulgare) in Latvia. J. Phytopathol. (1986). 170 (3), 167–175. doi:10.1111/jph.13064
Steinfeld, U., Sierotzki, H., Parisi, S., Poirey, S., and Gisi, U. (2001). Sensitivity of mitochondrial respiration to different inhibitors in Venturia inaequalis. Pest Manage. Sci. 57, 787–796. doi:10.1002/ps.356
Sułowicz, S., Cycoń, M., and Piotrowska-Seget, Z. (2016). Ecotoxicology, 1047–1060. doi:10.1007/s10646-016-1661-7Non-target impact of fungicide tetraconazole on microbial communities in soils with different agricultural management
Sułowicz, S., and Piotrowska-Seget, Z. (2016). Response of microbial communities from an apple orchard and grassland soils to the first-time application of the fungicide tetraconazole. Ecotoxicol. Environ. Saf. 124, 193–201. doi:10.1016/j.ecoenv.2015.10.025
Tagawa, M., Tamaki, H., Manome, A., Koyama, O., and Kamagata, Y. (2010). Isolation and characterization of antagonistic fungi against potato scab pathogens from potato field soils. FEMS Microbiol. Lett. 305 (2), 136–142. doi:10.1111/j.1574-6968.2010.01928.x
Turkington, T. K., O'Donovan, J. T., Edney, M. J., Juskiw, P. E., McKenzie, R. H., Harker, K. N., et al. (2012). Effect of crop residue, nitrogen rate and fungicide application on malting barley productivity, quality, and foliar disease severity. Can. J. Plant Sci. 92 (3), 577–588. doi:10.4141/cjps2011-216
United States Environmental Protection Agency (2007). Introduction to pesticide drift. Available at: https://www.epa.gov/reducing-pesticide-drift/introduction-pesticide-drift [Accessed June 13, 2022].
United States Environmental Protection Agency (2007). Pesticide fact sheet for prothioconazole. Available at: https://www3.epa.gov/pesticides/chem_search/reg_actions/registration/fs_PC-113961_14-Mar-07.pdf. [Accessed March 22, 2021].
van den Berg, F., Kubiak, R., Benjey, W. G., Majewski, M. S., Yates, S. R., Reeves, G. L., et al. (1999). Emission of pesticides into the air. Water Air Soil Pollut. 115, 195–218. doi:10.1023/a:1005234329622
Venables, W. N., and Ripley, B. D. (2002). Modern applied statistics with S. New York, NY: Springer.
Veresoglou, S. D., Wang, D., Andrade-Linares, D. R., Hempel, S., and Rillig, M. C. (2018). Fungal decision to exploit or explore depends on growth rate. Microb. Ecol. 75, 289–292. doi:10.1007/s00248-017-1053-4
Wang, H., Kang, C., Wang, Y., Wang, S., Zhang, Y., Wang, T., et al. (2022). First report of Paraphoma chrysanthemicola causing crown and leaf rot of Atractylodes lancea in China. Plant Dis. 106, 2268. doi:10.1094/pdis-11-21-2408-pdn
Weete, J. D., Abril, M., and Blackwell, M. (2010). Phylogenetic distribution of fungal sterols. Plos One 5, e10899. doi:10.1371/journal.pone.0010899
Wegulo, S. N., Zwingman, M. V., Breathnac, J. A., and Baenziger, P. S. (2011). Economic returns from fungicide application to control foliar fungal diseases in winter wheat. Crop Prot. 30 (6), 685–692. doi:10.1016/j.cropro.2011.02.002
Wenneker, M., Pham, K. T. K., Boekhoudt, L. C., de Boer, F. A., van Leeuwen, P. J., Hollinger, T. C., et al. (2016). First report of Truncatella angustata causing postharvest rot on “topaz” apples in the Netherlands. Plant Dis. 101, 508. doi:10.1094/pdis-09-16-1374-pdn
Wheeler, M. H., and Kuch, M. A. (1995). The effects of tricyclazole, pyroquilon, phthalide, and related fungicides on the production of conidial wall pigments by Penicillium and Aspergillus species. Pestic. Biochem. Physiol. 52 (2), 125–136. doi:10.1006/pest.1995.1037
Wiik, L., and Rosenqvist, H. (2010). The economics of fungicide use in winter wheat in southern Sweden. Crop Prot. 29 (1), 11–19. doi:10.1016/j.cropro.2009.09.008
Withee, P., Haituk, S., Senwanna, C., Karunarathna, A., Tamakaew, N., Pakdeeniti, P., et al. (2022). Identification and pathogenicity of Paramyrothecium species associated with leaf spot disease in northern Thailand. Plants 11, 1445. doi:10.3390/plants11111445
Wu, H., Wu, J., Li, F., Zheng, L., Fan, J., and Lin, W. (2021). First report of mucor spp. causing root rot of radix pseudostellariae in guizhou province of China. Plant Dis. 105, 1200. doi:10.1094/pdis-07-20-1634-pdn
Wu, X., Yang, J., Ruan, H., Wang, S., Yang, Y., Naeem, I., et al. (2021). The diversity and co-occurrence network of soil bacterial and fungal communities and their implications for a new indicator of grassland degradation. Ecol. Indic. 129, 107989. doi:10.1016/j.ecolind.2021.107989
Xu, X., Zhang, L., Yang, X., Cao, H., Li, J., Cao, P., et al. (2022). Alternaria spp. associated with leaf blight of maize in heilongjiang province, China. Plant Dis. 106 (2), 572–584. doi:10.1094/PDIS-06-21-1151-RE
Yang, T., Adams, J. M., Shi, Y., He, J., Jing, X., Chen, L., et al. (2017). Soil fungal diversity in natural grasslands of the Tibetan plateau: associations with plant diversity and productivity. New Phytol. 215 (2), 756–765. doi:10.1111/nph.14606
Yang, Y., Dou, Y., Huang, Y., and An, S. (2017). Links between soil fungal diversity and plant and soil properties on the loess plateau. Front. Microbiol. 8, 2198. doi:10.3389/fmicb.2017.02198
Yao, X., Liu, Y., Liu, X., Qiao, Z., Sun, S., Li, X., et al. (2022). Effects of thifluzamide on soil fungal microbial ecology. J. Hazard. Mat. 431, 128626. doi:10.1016/j.jhazmat.2022.128626
Yu, X., and Dijkstra, F. A. (2020). Carbon and nitrogen dynamics affected by litter and nitrogen addition in a grassland soil: Role of fungi. Eur. J. Soil Biol. 100, 103211. doi:10.1016/j.ejsobi.2020.103211
Yu, Y., Zheng, L., Zhou, Y., Sang, W., Zhao, J., Liu, L., et al. (2021). Changes in soil microbial community structure and function following degradation in a temperate grassland. J. Plant Ecol. 14 (3), 384–397. doi:10.1093/jpe/rtaa102
Zhang, Y., Xie, Y., Ma, H., Zhang, J., Jing, L., Wang, Y., et al. (2021). The influence of climate warming and humidity on plant diversity and soil bacteria and fungi diversity in desert grassland. Plants 10, 2580. doi:10.3390/plants10122580
Keywords: differential sensitivity, fungicide, grassland, non-target fungi, soil filamentous fungi
Citation: dela Cruz JA, Camenzind T and Rillig MC (2022) Sub-lethal fungicide concentrations both reduce and stimulate the growth rate of non-target soil fungi from a natural grassland. Front. Environ. Sci. 10:1020465. doi: 10.3389/fenvs.2022.1020465
Received: 16 August 2022; Accepted: 14 September 2022;
Published: 03 October 2022.
Edited by:
Bilal Ahmed, Yeungnam University, South KoreaReviewed by:
Mohammad Tarique Zeyad, National Bureau of Agriculturally Important Microorganisms (ICAR), IndiaMurugan Kumar, National Bureau of Agriculturally Important Microorganisms (ICAR), India
Rokhana Faizah, Bogor Agricultural University, Indonesia
Copyright © 2022 dela Cruz, Camenzind and Rillig. This is an open-access article distributed under the terms of the Creative Commons Attribution License (CC BY). The use, distribution or reproduction in other forums is permitted, provided the original author(s) and the copyright owner(s) are credited and that the original publication in this journal is cited, in accordance with accepted academic practice. No use, distribution or reproduction is permitted which does not comply with these terms.
*Correspondence: Matthias C. Rillig, bWF0dGhpYXMucmlsbGlnQGZ1LWJlcmxpbi5kZQ==