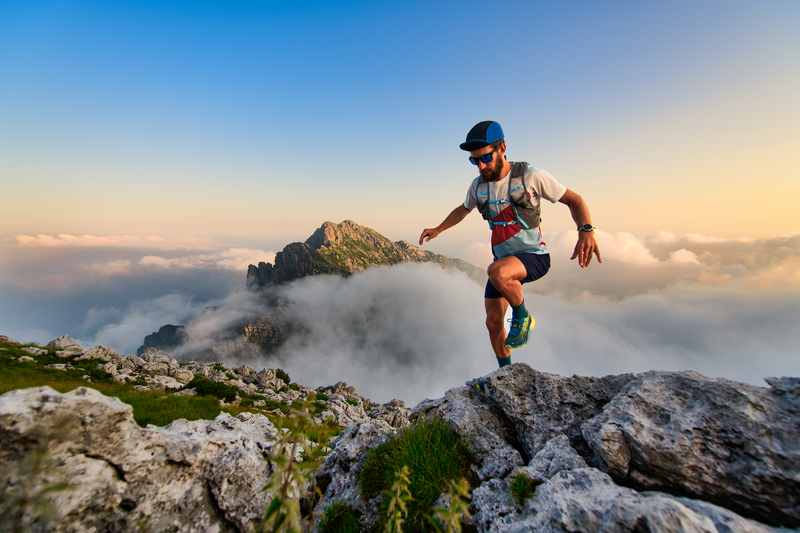
95% of researchers rate our articles as excellent or good
Learn more about the work of our research integrity team to safeguard the quality of each article we publish.
Find out more
ORIGINAL RESEARCH article
Front. Environ. Sci. , 26 September 2022
Sec. Environmental Informatics and Remote Sensing
Volume 10 - 2022 | https://doi.org/10.3389/fenvs.2022.1020028
This article is part of the Research Topic ICT Diffusion and Environmental Sustainability View all 13 articles
The present research was carried out to investigate the behavior of radon (222Rn) concentrations over the carbonate and evaporite sequences and to assess the related health hazards. A total of 50 points from three different stratigraphic units, namely, the Bahadurkhel Salt, Jatta Gypsum, and the Kohat Formation of the Eocene age, were analyzed for radon concentrations in the district of Karak, Khyber Pakhtunkhwa, Pakistan. Measurements for radon levels were made by using RAD7 of Durridge, United States. The highest average 222Rn concentration (16.5 Bq/L) was found in the limestone unit of the Kohat Formation of the Eocene age. However, the lowest radon levels were observed in the salt-bearing strata of the Bahadurkhel Salt of the Eocene age. The study revealed that the average radon concentration in all the lithologies varied in the order of RnLimestone > RnSalt > RnGypsum. The findings of the current research suggest that the study area is safe from radon-related health hazards.
222Rn, an invisible radioactive gas with a half-life of 3.82 days, is naturally occurring in all types of soils in measurable quantities (Goodwin et al., 2009). 222Rn is a 238U chain progeny decaying to 218Po and discharging a potentially harmful alpha particle. Radon and its progenies like 218Po, 214Po, and 214Bi have been pointed out to be the major sources of public exposure from natural radioactivity, contributing to almost 50% of the worldwide mean effective dose to the community (UNSCEAR, 2000; Somlai et al., 2007). Over 90% of the total radiation dose received due to radon exposure is contributed by two of the alpha-emitting daughters of 222Rn, i.e., 218Po and 214Po (Gillmore et al., 2001). After inhalation or ingestion, radon decays and releases energy, causing lung and stomach cancer (Khattak et al., 2011). Exposure to elevated radon concentrations via inhalation for a long time is the second major cause of lung cancer, with smoking being the first cause (The World Health Organization, 2005; Matiullah and Muhammad, 2017). This phenomenon of radon makes it a human health menace. The presence of radon, on the other hand, in the rocks, soil, and alluvial material can serve as a tool for exploring geological features and predicting earthquakes.
Radon emanates from rocks and easily migrates and enters the groundwater bodies that fracture (Arabi et al., 2016; Khan et al., 2022). Although radon can occur in almost all rock and soil types, the concentration differs with different sites and geological materials (Singh et al., 1999). The source and distribution of radon is mainly controlled by the geology (Appleton and Miles, 2005; Appleton and Miles, 2010). Particular rock types (not all) and unconsolidated deposits have relatively high radon emanation, for example, granites, phosphatic rocks with uranium enrichment, and organic shales. The chemical composition of the rocks and soils and the distribution of alluvium and till deposits are controlled by the geology of the region from which radon is being released. According to Gundersen et al. (1992) and Schumann (1993), different rocks and soils act as sources for radon gas. Amongst the most likely rock types that are causing radon emissions are carbonaceous shales, glauconitic sandstones, fluvial deposits, carbonate rocks (karst topography), chalk, phosphorites, tillites, granites, metamorphosed rocks with granitic composition, bauxite, different types of coal deposits, graphite schists, silica-rich volcanic rocks, fractured or faulted rocks, and some types of contact metamorphic rocks. The different rocks, e.g., quartzose sandstone, non-organic shales and siltstones, and silica-deficient igneous and metamorphic rocks, are least likely to cause radon emanations. Localized uranium deposition as hydrothermal vein deposits in igneous and metamorphic rocks and roll-front deposits in sedimentary rocks can cause exceptions to radon discharge (Gundersen, 1991).
The most important fundamental influence on soil 222Rn potential is the concentration of radionuclides in the rocks. In most cases, a high soil gas 222Rn concentration is developed over the rocks with high 226Ra concentrations (Schumann and Gundersen, 1996; Khan et al., 2022). Not only is the 238U or 226Ra concentration of a soil controlled by the type of bedrock but also the gas permeability of a soil, which determines the migration distance, depends on the bedrock. By comparing the average 238U concentration of rocks and soils indicate that enrichment of radionuclides can take place in soils due to weathering processes. This is particularly true for limestones; even if these rocks are poor in 226Ra, high 226Ra concentrations are observed within their soils (Wiegand, 2001). Limestones were intensely chemically weathered during the tropic conditions of the Tertiary period, absorbing a large fraction of the mobilized 226Ra by the thin layer of clay minerals, which is usually developed above limestone. The overall concentration of 238U in sediments from the limestone catchment is low (2.8–3.6 ppm). High radon emissions can occur on limestone because of the higher permeability and high specific surface area of these uranium minerals, allowing the efficient release of radon (Ball et al., 1991). Additionally, elevated migration rates are prompted by the high fracture and joint permeability of the limestone and the permeability of the overlying soils.
The term evaporite is generally used for rocks that are formed as a result of the process called evaporation. These rocks are also called crystalline rocks because they are formed by the linking or bonding of crystals with each other. The most common types of evaporites are salt (NaCl) and gypsum (CaSO4.2H2O). These rocks are made up of crystals of minerals that do not have uranium in them, so they are considered to be less radon-prone rocks (Baloch et al., 2012). If any intrusion occurs, it may increase the probability of radon emanation in evaporites, such as granitic intrusion (Scheib et al., 2013).
The transport of gases is influenced by the main component of soil regarded as diffusivity (Ball et al., 1991; Ehsan et al., 2018). The interstitial pore spaces of soil are the sites from which radon gas can emanate. A controlled measurement of radon can be carried out from the bedrock or soil as it diffuses to the surface before decaying. The two main constituents required to predict the radon hazard potential of an area include the soil radon concentration and soil gas permeability (Neznal et al., 2004). The elevated levels of radon gas identified in indoor air make it a dangerous agent for human health.
Different research practices have been carried out to assess the impacts of environmental issues on human health. According to the United Nations’ sustainable development goals, environmental conditions have direct and indirect impacts on human wellbeing. It is claimed that better opportunities for human wellbeing are produced by better ecological conditions, thus creating positive associations between good quality environmental conditions and human wellbeing (Sohail et al., 2019; Bhandari, 2022; Sohail et al., 2022). The present study is aimed to measure the soil gas radon concentration over three different lithological units, i.e., salt, gypsum, and limestone, with the active method of using an electronic RAD7 radon monitor to establish a database for soil gas radon levels and associated health risks as the literature survey shows that no attempt has been made in the area in this regard.
The study area lies in the southern Kohat Plateau. The geology of the area has been studied in great detail by many workers (Meissner et al., 1974; Ahmad, 2003). In general, the rocks are characterized by multiple deformations resulting in superimposed folding and repeated faulting and thrusting. The stratigraphic units of the study area comprise the Bahadurkhel Salt (salt with intercalations of shale) and Jatta Gypsum (gypsum and clay intercalations) of the Eocene age, being the oldest exposed rocks in the study area. The salt and gypsum units are overlain by the Kuldana (red clay) and Kohat formations (limestone and shale) of the Eocene age, respectively (Figure 1). The complete rock series of the Siwalik Group (Miocene–Pleistocene age), comprising the Kamlial Formation (sandstone, siltstone and conglomeratic lenses), the Chinji Formation (shale and sandstone), the Nagri Formation (massive sandstone and shale), the Dhok Pathan Formation (friable sandstone), and the Soan Formation (pebbles, sandstone, and shale), in turn overlies the Eocene sequence in the area (Meissner et al., 1974). Karak District consists of huge deposits of evaporites, hydrocarbons, coal, and uranium (District Profile Karak, 2009).
FIGURE 1. Geological map of the study area (redrawn after Meissner et al. (1974).
During late Miocene, the Kohat Plateau had been subjected to the southward progression in deformation. The boundary in the northern part of the plateau is marked by the Main Boundary Thrust (MBT). This thrust brings the older rocks of the Kohat Range of Mesozoic age over the younger rocks of the Tertiary age of the Kohat Plateau (Yeats and Hussain, 1987). Toward the south, the Kohat Plateau is bounded by the Bannu Basin, while the south-eastern boundary is marked by the Surghar Range. The left lateral Kurram Fault is juxtaposing the Mesozoic age rocks with the Tertiary age rocks toward the west (Ahmad, 2003).
The area is tectonically disturbed and consists of major thrust faults: the Karak Thrust, Methakhel Thrust, Garori Thrust, Banda Khungarah Fault, and Nari Panos Fault. Along the Karak Thrust, Jatta Gypsum is thrown over the Chinji Formation, while the Garori and Methakhel thrusts emplace the Nagri Formation on top of the Chinji Formation, and similarly, both Banda Khungarah and Nari Panos faults are back-thrust and bring the older Eocene rocks on top of the Pliocene age rocks in the area (Khan, 2013; Khattak et al., 2014; Khattak et al., 2016; Khan et al., 2022) (Figure 1).
Radon measurements can be made in the field or samples can be taken and measured in laboratories where background contributions can be reduced by shielding the detector (e.g., by using lead) from ambient gamma radiation. Continuous and time-integrating measurements are generally made in situ, while instantaneous measurements can be made in the laboratory or in situ. In this study, the grab-sampling mode of Durridge RAD7 was used during the measurements of in situ radon. For collection of soil samples, a depth of 60 cm was attained at every measuring station.
A stainless steel soil gas probe supplied by Durridge Company (United States) was used to measure radon activities in soil gas. A 60 cm deep and 2 cm wide hole was made by inserting the probe with a hollow tube through the soil with the help of a hammer (Figure 2). Sometimes the depth of the hole was less than 50 cm due to poor development of soil above the parent rock or the pebbly nature of the soil that did not allow the rod to reach 60 cm depth. The probe was connected to RAD7 by pushing the plug-in hose connector into the probe to prevent any water entry into the detector. After the water trap, the air passed through a drierite desiccant tube (calcium sulfate, CaSO4), then to a filter, and finally to the RAD7 system. Before the counting process started, the hole was properly sealed in order to prevent the mixing of soil air with air from the atmosphere. Air was then drawn up the tube into RAD7 and circulated in a closed circuit for a period of 5–10 min, and then, four 5-min cycles are counted. A printout summary with the bar chart for the counted cycles (four), the average concentration of radon, and a collective spectrum is produced by RAD7 at the end. A total of 30 min is taken by the process of measurement in the grab method. Correction factors for radon decay must be applied if the measurements are made after an hour of sample collection. But during this study, all the measurements were taken at the location, which is why no correction factors have been applied (Khan et al., 2022).
FIGURE 2. Measurement procedure for radon in soil (Liu et al., 2016).
Durridge calibrates all instruments to a set of four “master” instruments with a calibration precision of about 1%. The master instruments have been calibrated by way of inter-comparison with secondary standard radon chambers designed by the U.S. EPA. The estimated accuracy of the master instrument is to be within 4%, based on inter-comparison results. It is estimated that the overall calibration accuracy of RAD7 is better than 5%.
The evaporitic sequence of the study area consists of Bahadurkhel Salt and Jatta Gypsum of the Eocene age. The concentration of radon in Bahadurkhel Salt varies from 0.15 to 7.6 Bq/L with an average of (1.8 Bq/L) for 10 measuring points, and Jatta Gypsum yielded radon levels in the range of 0.2–3.4 Bq/L; the average concentration of radon for Jatta Gypsum for 20 sampling points is revealed (1.6 Bq/L (Figures 3, 4). The number of sampling points along Bahadurkhel Salt was limited to 10 because of the extent of the formation in the study area. The results of the present study clearly indicate that Bahadurkhel Salt has higher values of radon concentrations than Jatta Gypsum.
The limestone present in the study area belongs to the Kohat Formation of the Eocene age. The Kohat Formation comprises bioclastic limestone in the lower parts and grades into massive limestone toward the top. Throughout the limestone unit, variable radon concentrations were observed. The highest concentration of radon was measured in the lower unit of the formation. A total of 20 radon measurements were made along the Kohat Formation (Figure 5), and a wide range of radon concentrations was observed. The radon concentrations varied between a wide range of 0.44–16.5 Bq/L and an average concentration of 3.3 Bq/L (Figure 6).
Radon is mainly responsible for such public exposure to natural radiation (Cinelli et al., 2015). Different factors might be responsible for the soil-radon activity: 1) the parent radionuclide concentration in different rocks; 2) the volume-to-surface ratio of the soil and sub-soil clasts (the low-volume ratio to surface area causes low efficacy in the radon escape from the rock matrix); 3) the sub-surface rocks’ average bulk permeability and permeability type (secondary or primary); and 4) due to variance in the deep gas flux, the changes in the transport mechanism driven by advection (Singh et al., 2002).
Low radon levels, as expected, were observed in the areas where salt and gypsum occur, clearly indicating that these lithological units are made up of mineral crystals poor in uranium mineralization, so they are considered to be less radon-prone rocks (Baloch et al., 2012). If any intrusion occurs, it may increase the probability of radon concentrations in evaporites, such as granitic intrusion (Scheib et al., 2013). It can also be inferred that the salt-bearing Bahadurkhel Formation is yielding much concentrations of radon in comparison to the gypsum-dominated Jatta because secondary porosity is more common in salt, creating more intense pores for facilitating invasion.
Radon is considered to have originated from uranium-rich bedrocks (Esan et al., 2020). Elevated radon concentrations in limestone can be attributed to the generally fairly high concentration of uranium in the carbonates as compared to evaporites. The high surface area of uranium and the high permeability of the bedrock can result in the high concentrations of radon (Scheib et al., 2013). Uranium has a uniform distribution within the limestones, which is usually <10 ppm, and is often associated with the finely disintegrated organic matter of bioclastic limestones. This high surface area of uranium in limestones compared to granites allows the effective release of 222Rn into the air and water (Appleton and Ball, 1995). But the migration of radon is likely to be restricted by the carbonate cements by greatly reducing both the permeability and porosity of the matrix (Scheib et al., 2013).
Due to ubiquitous aeration of the soil, the radon concentration is variable from the soil to the atmosphere (Clavensjö and Åkerblom, 1994). The overall concentration of radon in all the three lithologies at different sampling locations in the studied area ranges between 0.15 and 16.5 Bq/L. Nevertheless, the variation in the average radon levels in all the three lithologies fluctuates between 1.8 Bq/L, 1.6 Bq/L, and 3.3 Bq/L for Bahadurkhel Salt, Jatta Gypsum, and Kohat Formation, respectively.
In Table 1 a comparison of the soil gas radon concentration in the salt, gypsum, and limestone lithologies from different countries and the present investigation by using passive and active techniques has been presented. The yielded results show that the radon concentrations determined in the salt lithology of both Romania and Pakistan are lower than the reported values in the present investigation. However, the radon concentrations observed in the gypsum and limestone lithologies in India, Portugal, England, and Scotland are higher than the reported values in this study.
TABLE 1. Comparison of radon concentrations in salt, gypsum, and limestone under investigation with those in other countries.
Descriptive statistics of the soil gas radon concentrations obtained in the study area are presented in Table 2. Soil gas radon concentration values obtained across all the sampling locations ranged between 0.15 Bq/L and 16.5 Bq/L with a mean of 1.8 Bq/L. However, in the soil overlying the three lithologies, the concentration values varied from 0.15 to 7.6 Bq/L, 0.2 to 3.4 Bq/L, and 0.44 to 16.5 Bq/L with mean values of 1.8 Bq/L, 1.6 Bq/L, and 3.3 Bq/L for salt, gypsum, and limestone, respectively. The distributions of the soil gas radon concentration throughout the study area and across the three lithologies of the study area are positively skewed. A skewness value of 3.87 was obtained for the measured soil gas radon concentration across the study area, whereas across the three lithologies, skewness values of 1.99, 0.35, and 3.27 were obtained for salt, gypsum, and limestone, respectively.
The data have been analyzed using the box and whisker plot, as shown in Figure 7. The limestone box plot shows the four major quartiles, of which Q4 is the major quartile. The median lies on the value of 2.385. One value is found as an outlier in the data on sample 1. The evaporites show a narrow range of values as compared to the limestone, with a median value of 1.27 and major coverage by the Q4 values. One outlier was also found in this analysis for salt lithology.
On the basis of the intercomparison among these plots, it is clearly revealed that the highest variations of values are found in the limestone, whereas evaporites have lesser variations.
The radon concentrations over the carbonate and evaporite sequence of the study area indicate that the health hazards related to radon and its progenies are within safe limits. Radiological health hazards such as the presence of CO2, SO2, CO, and other radioactive elements posing health concerns are not considered in this study. Proper regulatory measures such as ventilation and regular dosimetry of inhabitants must be adopted in order to minimize the health hazards related to radon and its progenies and other radioactive pollutants. It is also recommended that extensive research should be carried out in the quarries and mines where the gypsum and salt are extracted because the dust in these quarries and mine atmosphere normally contains radioactive aerosols that can adhere to the lungs and respiratory tract and can damage the bronchial tissues and cause lung cancer (Qureshi et al., 2000).
• The present study revealed that the radon concentration in the observed lithologies varies in the order of: RnLimestone > RnSalt > Rn Gypsum. The radon concentration in limestone is about two times greater than that of salt and gypsum.
• The soil gas radon concentration in the study area exhibits wide variation, ranging between 0.15 and 16.5 Bq/L, in comparison with salt, limestone, and gypsum.
• The elevated radon potential of the limestone in comparison to the evaporite sequence in the study area can be related to the high joint and fracture permeability of the limestone and to the amount of uranium present in carbonates.
• The area under study is fairly safe from the health hazards related to radon gas and its progenies. Other radioactive pollutants and gaseous elements posing health risks may be present in the area and need to be monitored and minimized.
• The present findings will act as baseline data for further investigation in the region regarding radon levels in soil gas, particularly in the carbonate and evaporite sequences.
The raw data supporting the conclusion of this article will be made available by the authors, without undue reservation.
All authors listed have made a substantial, direct, and intellectual contribution to the work and approved it for publication.
The authors are thankful to the National Centre of Excellence in Geology, University of Peshawar, for providing the laboratory facilities for sample analysis. This study was extracted from the PhD thesis of MK (first author), which was submitted to the Higher Education Commission (HEC), Pakistan repository, for awarding Ph.D. degree.
The authors declare that the research was conducted in the absence of any commercial or financial relationships that could be construed as a potential conflict of interest.
All claims expressed in this article are solely those of the authors and do not necessarily represent those of their affiliated organizations, or those of the publisher, the editors, and the reviewers. Any product that may be evaluated in this article, or claim that may be made by its manufacturer, is not guaranteed or endorsed by the publisher.
Ahmad, S. (2003). “A comparative study of structural styles in the Kohat Plateau,” (NW Himalayas NWFP Pakistan: National Centre of Excellence in Geology, University of Peshawar.). Unpublished Ph.D. thesis.
Appleton, J. D., and Ball, T. K. (1995). “Radon and background radioactivity from natural sources: Characteristics, extent and the relevance to planning and development in great britain,”. Technical Report WP/95/2 (Nottinghamshire: British Geological Survey).
Appleton, J. D., and Miles, J. C. H. (2010). A statistical evaluation of the geogenic controls on indoor radon concentrations and radon risk. J. Environ. Radioact. 101 (10), 799–803. doi:10.1016/j.jenvrad.2009.06.002
Appleton, J. D., and Miles, J. C. H. (2005). “Radon in wales,” in Urban geology of wales. Editors D. Nicol, and M. G. Bassett (Cardiff United Kingdom: National Museum of Wales Geological Series).
Arabi, A. S., Futua, , Dewu, B. B. M., Kwaya, M. Y., Kurowska, E., Muhammad, A. M., et al. (2016). NORM, radon emanation kinetics and analysis of rocks associated radiological hazards. Environ. Earth Sci. 75, 689. doi:10.1007/s12665-016-5488-6
Ball, B. C., Scott, A., and Parker, J. P. (1991). Field N2O, CO2 and CH4 fluxes in relation to tillage, compaction and soil quality in Scotland. Soil Tillage Res. 53, 29–39. doi:10.1016/s0167-1987(99)00074-4
Baloch, M. A., Qureshi, A. A., Waheed, A., Ali, M., Ali, N., Tufail, M., et al. (2012). A study on natural radioactivity in khewra salt mines, Pakistan. J. Radiat. Res. 53, 411–421. doi:10.1269/jrr.11162
Bhandari, P. (2022). “Impacts on environment and on human health,” in Responsible consumption and production (Cham: Springer International Publishing), 349–357.
Calin, M. R., and Calin, M. A. (2010). Evaluation of the radon concentration in ocna dej salt mine, Romania. J. Radioanal. Nucl. Chem. 286, 169–173. doi:10.1007/s10967-010-0648-8
Cinelli, G., Tositti, L., Capaccioni, B., Brattich, E., and Mostacci, D. (2015). Soil gas radon assessment and development of a radon risk map in Bolsena, Central Italy. Environ. Geochem. Health 37 (2), 305–319. doi:10.1007/s10653-014-9649-9
Clavensjö, B., and Åkerblom, G. (1994). The radon book. Stockholm: The Swedish Council for Building Research.
District Profile Karak (2009). Small & medium enterprises development authority ministry of industries and production. Pakistan: Government of Pakistan, 1–11. Available at: http://www.karak.financekpp.gov.pk/index.php?option=com_content&view=article&id=53&Itemid=63.
Ehsan, M., Gu, H., Akhtar, M. M., Abbasi, S. S., and Ehsan, U. (2018). A geological study of reservoir formations and exploratory well depths statistical analysis in Sindh Province, Southern Lower Indus Basin, Pakistan. Kuwait jour. Sci. 45 (2), 84–93.
Esan, D. T., Sridhar, M. K. C., Obed, R., Ajiboye, Y., Afolabi, O., Olubodun, B., et al. (2020). Determination of residential soil gas radon risk indices over the lithological units of a Southwestern Nigeria University. Sci. Rep. 10 (1), 7368–7410. doi:10.1038/s41598-020-64217-8
Gillmore, G. K., Phillips, P., Denman, A., Sperrin, M., and Pearce, G. (2001). Radon levels in abandoned metalliferous mines, Devon, Southwest England. Ecotoxicol. Environ. Saf. 49, 281–292. doi:10.1006/eesa.2001.2062
Goodwin, T. A., Ford, K. L., Friske, P. W. B., and McIsaac, E. M. (2009). Radon soil gas in nova scotia. Canada: Nova Scotia Department of Natural Resources, 25–34.
Gundersen, L. C. S. (1991). “Radon in sheared metamorphic and igneous rocks,” in Geologic and geochemical field studies of radon in rocks, soils, and water. Editors L. C. S. Gundersen, and R. B. Wanty (U.S. Geological Survey Bulletin), 38–49.
Gundersen, L. C. S., Schumann, E. R., Otton, J. K., Dubief, R. F., Owen, D. E., and Dickenson, K. E. (1992). “Geology of radon in the United States,” in Geologic controls on radon gates. Editor L. C. S. Gundersen (United States: Geological Society America), 1–16.
Khan, M. A., Khattak, N. U., and Hanif, M. (2022). Radon emission along faults: A case study from district Karak, sub-himalayas, Pakistan. J. Radioanal. Nucl. Chem. 331, 1995–2003. doi:10.1007/s10967-022-08283-4
Khan, M. A. (2013). “Radon based geo-environmental investigation of Karak trough and its adjoining areas,” (District Karak, Khyber Pakhtunkhwa, Pakistan, Peshawar Pakistan: National Centre of Excellence in Geology University of Peshawar). M.S Thesis.
Khattak, N. U., Khan, M. A., Ali, N., Ahmed, F., and Shah, M. T. (2016). Recognition and characterization of a tectonically active Karak Thrust using radon measurement technique in the Southern Kohat Plateau, Pakistan. J. Himal. Earth Sci. 49 (2), 40–49.
Khattak, N. U., Khan, M. A., Shah, M. T., and Ali, N. (2014). Radon concentration in drinking water sources of the region adjacent to a tectonically active Karak Thrust, southern Kohat Plateau, Khyber Pakhtunkhwa, Pakistan. J. Radioanal. Nucl. Chem. 302 (1), 315–329. doi:10.1007/s10967-014-3257-0
Khattak, N. U., Khan, M. A., Shah, M. T., and Javed, M. W. (2011). Radon concentration in drinking water sources of the main campus of the university of peshawar and surrounding areas, khyber Pakhtunkhwa, Pakistan. J. Radioanal. Nucl. Chem. 290, 493–505. doi:10.1007/s10967-011-1297-2
Liu, H., Wang, N., Chu, X., Li, T., Zheng, L., Yan, S., et al. (2016). Mapping radon hazard areas using 238U measurements and geological units: A study in a high background radiation city of China. J. Radioanal. Nucl. Chem. 309, 1209–1215. doi:10.1007/s10967-016-4717-5
Matiullah, M., and Muhammad, W. (2017). Measurement of radon concentration levels in Pakistan: An overview. Indoor Built Environ. 26 (10), 1319–1334. doi:10.1177/1420326x16645849
Meissner, C. R., Master, J. M., Rashid, M. A., and Hussain, M. (1974). Stratigraphy of Kohat quadrangle Pakistan. United States: Geological Society of America professional paper, 89.
Mittal, S., Rani, A., and Mehra, R. (2016). Estimation of radon concentration in soil and groundwater samples of Northern Rajasthan, India. J. Radiat. Res. Appl. Sci. 9 (2), 125–130. doi:10.1016/j.jrras.2015.10.006
Neznal, M., Neznal, M., Matolin, I. B., Barnet, I., and Miksova, J. (2004). The new method for assessing the radon risk of building sites. Czech Geol. Surv. Spec. Pap. 16, 47–48.
Pereira, A. J. S. C., Neves, L. J. P. F., Costa, L. A. P. A., and Godinho, M. M. (1999). Soil gas radon potential in two urban areas of central Portugal. IL nuovo cimento 22C (3 4), 615–620.
Scheib, C., Appleton, J. D., Miles, J. C. H., Green, B. M. R., Barlow, T. S., and Jones, D. G. (2009). Geological controls on radon potential in Scotland. Scott. J. Geol. 45 (2), 147–160. doi:10.1144/0036-9276/01-401
Scheib, C., Appleton, J. D., Miles, J. C. H., and Hodgkinson, E. (2013). Geological controls on radon potential in England. Proc. Geologists'. Assoc. 124 (6), 910–928. doi:10.1016/j.pgeola.2013.03.004
Schumann, R. R. (1993). Geologic radon potential of EPA region (1-10): United States geological survey open file report. Washington, D.C: U.S. DEPARTMENT OF THE INTERIOR, 93–292.
Schumann, R. R., and Gundersen, L. C. S. (1996). Geologic and climatic controls on the radon emanation coefficient. Environ. Int. 22 (1), 439–446. doi:10.1016/S0160-4120(96)00144-4
Singh, A. K., Sengupta, D., and Prasad, R. (1999). Radon exhalation rate and uranium estimation in rock samples from Bihar uranium and copper mines using the SSNTD technique. Appl. Radiat. Isot. 51, 107–113. doi:10.1016/s0969-8043(98)00152-3
Singh, S., Kumar, A., and Singh, B. (2002). Radon level in dwellings and its correlation with uranium and radi-um content in some areas of Himachal Pradesh, India. Environ. Int. 28 (1 2), 97–101. doi:10.1016/s0160-4120(02)00012-0
Sohail, M. T., Elkaeed, E. B., Irfan, M., Acevedo-Duque, Á., and Mustafa, S. (2022). Agricultural communities’ risk assessment and the effects of climate change: A pathway toward green productivity and sustainable development. Front. Environ. Sci. 10, 900193. doi:10.3389/fenvs.2022.948016
Sohail, M. T., Mahfooz, Y., Azam, K., Yen, Y., Genfu, L., and Fahad, S. (2019). Impacts of urbanization and land cover dynamics on underground water in Islamabad, Pakistan. Desalinat. Water Treat. 159, 402–411. doi:10.5004/dwt.2019.24156
Somlai, K., Tokonami, S., Ishikawa, T., Vancsura, P., Ga´spa´r, M., Jobba´gy, V., et al. (2007). 222Rn concentrations of water in the Balaton Highland and in the southern part of Hungary, and the assessment of the resulting dose. Radiat. Meas. 42, 491–495. doi:10.1016/j.radmeas.2006.11.005
The World Health Organization, (2005). Fact sheet No 291: Radon and cancer. Available at http://www.who.int/mediacentre/factsheets/fs291/en/index.html.
UNSCEAR (2000). United Nations scientific committee on the effects of atomic radiations. New York: The General Assembly with Scientific Annex.
Wiegand, J. (2001). A guideline for the evaluation of the soil radon potential based on geogenic and anthropogenic parameters. Environ. Geol. 40, 949–963. doi:10.1007/s002540100287
Keywords: radon, gypsum, salt, limestone, Eocene, Karak
Citation: Khan MA, Khattak NU, Hanif M, Al-Ansari N, Khan MB, Ehsan M and Elbeltagi A (2022) Health risks associated with radon concentrations in carbonate and evaporite sequences of the uranium-rich district Karak, Pakistan. Front. Environ. Sci. 10:1020028. doi: 10.3389/fenvs.2022.1020028
Received: 15 August 2022; Accepted: 06 September 2022;
Published: 26 September 2022.
Edited by:
Muhammad Tayyab Sohail, Xiangtan University, ChinaReviewed by:
Jar Ullah, China University of Geosciences, ChinaCopyright © 2022 Khan, Khattak, Hanif, Al-Ansari, Khan, Ehsan and Elbeltagi. This is an open-access article distributed under the terms of the Creative Commons Attribution License (CC BY). The use, distribution or reproduction in other forums is permitted, provided the original author(s) and the copyright owner(s) are credited and that the original publication in this journal is cited, in accordance with accepted academic practice. No use, distribution or reproduction is permitted which does not comply with these terms.
*Correspondence: Nadhir Al-Ansari, bmFkaGlyLmFsYW5zYXJpQGx0dS5zZQ==; Muhsan Ehsan, bXVoc2FuZWhzYW45OEBob3RtYWlsLmNvbQ==
Disclaimer: All claims expressed in this article are solely those of the authors and do not necessarily represent those of their affiliated organizations, or those of the publisher, the editors and the reviewers. Any product that may be evaluated in this article or claim that may be made by its manufacturer is not guaranteed or endorsed by the publisher.
Research integrity at Frontiers
Learn more about the work of our research integrity team to safeguard the quality of each article we publish.