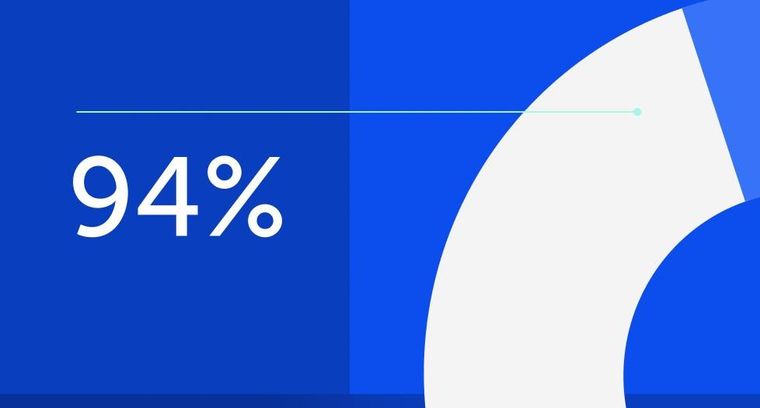
94% of researchers rate our articles as excellent or good
Learn more about the work of our research integrity team to safeguard the quality of each article we publish.
Find out more
ORIGINAL RESEARCH article
Front. Environ. Sci., 01 December 2022
Sec. Toxicology, Pollution and the Environment
Volume 10 - 2022 | https://doi.org/10.3389/fenvs.2022.1018739
This article is part of the Research TopicPollutants and Heat Treatment of Livestock Manure: Emission, Control, and Environmental Risk AssessmentView all 5 articles
The application of animal manures to cropland is an important nutrient recycling strategy in many parts of the world. Commonly, aggregated manure wastes contain chemical stressors including veterinary antimicrobials, heavy metals, and antimicrobial resistance genes (ARGs) that can stimulate the development and proliferation of antimicrobial resistance (AMR). While the presence of antimicrobials in manure is well-documented, the co-occurrence of other potentially impactful chemical stressors in swine manure remains underreported. This study quantifies and analyzes correlations between antimicrobials, metals, and certain ARGs present in manure samples from swine farms in Iowa, United States. Relationships between chemical stressors and different stages of swine production or feed composition are also investigated. Results revealed substantial levels of tetracyclines [up to 1,260 µg g−1 dry weight (d.w.) of manure for oxytetracycline] detected in all samples. Tiamulin, two ionophores (monensin and lasalocid), and one macrolide (tilmicosin) were detected at maximum class concentrations of 9.4, 0.547, and 0.472 µg g−1 d.w., respectively. The median relative abundances of ermB and tetM were 0.13 and 0.17 copies g−1 wet weight (w.w.) manure (normalized to 16S gene), respectively. Additionally, high levels of copper (Cu), iron (Fe), and zinc (Zn) were detected in all samples, with maximum concentrations of 887, 1,900, and 2,100 µg g−1 d.w., respectively. Notably, uranium (U) was detected in 11 samples, at concentrations up to 0.77 µg g−1. A global analysis of AMR-stressor relationships using Spearman’s rank correlation indicates Cu, and Ba are the most positively and significantly correlated with cytotoxic anhydrotetracycline (ATC) and/or anhydrochlortetracycline (ACTC) concentrations in all tested facilities (Cu-ATC: ρ = 0.67, p = 0.0093; Cu-ACTC: ρ = 0.75, p = 0.0022; Ba-ATC: ρ = 0.84, p = 0.0002). Interestingly, ermB and tetM genes were strongly, positively correlated to each other (ρ = 0.92, p < 0.0001), suggesting possible co-selection, despite the absence of correlation between ARGs and tetracycline concentrations. This study demonstrates the complexity of interactions between antimicrobials, metals, and ARGs in multiple manure storage pits prior to cropland application.
Antimicrobial resistance (AMR)-related deaths are increasing globally at an alarming rate, with an estimated 4.95 million AMR-linked deaths in 2019 alone (Antimicrobial Resistance Collaborators, 2022). As a result, it is critical to study the presence and mitigation of antimicrobial resistance gene (ARG) promoting agents released to the environment. Veterinary pharmaceuticals may be important ARG-promoting agents and are considered either medically-important or non-medically-important to humans (WHO, 2019). It has been suggested that both categories can have mutual impacts on human health, as current literature is inadequate to conclude otherwise (Wong, 2019). Although not used in humans, some non-medically-important antimicrobials, such as ionophores, may pose a threat to human health through cross-resistance or co-selection of AMR (Wong, 2019). Cross-resistance occurs when a single gene confers resistance to more than one agent due to structural similarities or common resistance mechanisms, while co-selection occurs where agents have genetically linked resistance genes and selection for one drug causes simultaneous selection for at least one other drug (Wong, 2019). Therefore, it is crucial to investigate the influences of both medically-important and non-medically important antimicrobials on AMR. Additionally, metals co-occurring with antimicrobials in agriculturally impacted soil and water have been linked to co-selection of ARGs (Wong, 2019). This can occur when metal concentrations exceed the minimum co-selective concentration (MCC), or the smallest metal concentration correlated with increased bacterial resistance to antimicrobials (Seiler and Berendonk, 2012).
Land application of manure is a major source of antimicrobial and metal release to agricultural environments (Chee-Sanford et al., 2009; Srivastava et al., 2017). Up to 90% of certain antimicrobials may be excreted unchanged, potentially retaining antimicrobial activity in soil receiving land-applied manure (Kumar et al., 2005). In 2020, more than 10.4 million kilograms of antimicrobials were sold in the United States (US) for agricultural use, including livestock disease control and growth promotion (USFDA, 2021). According to US Department of Agriculture (USDA) data on thirteen states, 93.5% of sites with more than 1,000 swine used antimicrobials in feed, 78.4% in water, and 92.4% administered antimicrobials by injection (USDA, 2019). Of the tested sites, 10.5% of nursery-age sites and 44.1% of grower/finisher sites administered antimicrobials in feed for growth promotion.
The introduction of antimicrobials to soil may stimulate the development and proliferation of AMR in bacteria exposed to these antimicrobials, which act as chemical stressors (Manyi-Loh et al., 2018). Similarly, certain metals necessary for cellular metabolic function are often added as mineral supplements to swine feed to promote growth and animal health (Hill et al., 2000; Medardus et al., 2014). Zinc (Zn) and copper (Cu) are often added to nursery swine diets to combat post-weaning diarrhea and stimulate growth (Hill et al., 2000; De Mille et al., 2022). However, an increased awareness of the negative impacts of excess metals on both the surrounding environment and AMR proliferation have raised concerns over the use of metals (ex. Cu, Zn) at high concentrations exceeding dietary requirements in livestock production (Hölzel et al., 2012; Dębski, 2016).
Our previous work revealed widespread occurrence of tetracycline antimicrobials and ARGs conferring resistance to tetracyclines in manure collected from dairy animals in three states in the United States (Hurst et al., 2019). However, no correlations were observed between the monitored tetracycline ARGs and tetracycline concentrations in manure, suggesting that other external pressures may induce expression of the observed tetracycline resistance. Importantly, a number of other studies report positive correlations between the concentrations of metals and/or antimicrobials, and ARG copies in swine manure (Zhang et al., 2015), swine lagoons and beef cattle storage ponds (Zhang et al., 2013), and manure-amended soil (Zhu et al., 2013). While the presence of antimicrobials in manure is well-documented (Congilosi and Aga, 2021), very little has been reported on the co-occurrence of other chemical stressors, such as potentially toxic metals, in swine manure that may stimulate proliferation and spread of AMR in exposed bacteria. The state of Iowa is currently the top swine producing state in the United States, with 23 million swine as of June 2022 (USDA, 2022). Therefore, this study seeks to: i) detect and quantify antimicrobials, potentially toxic metals, and select ARGs present in manure samples from swine farms in Iowa; and ii) explore possible relationships between antimicrobials, metals, and ARGs in swine manure from different stages of animal production and feed composition.
Primary analytical standards for lasalocid (LAS), monensin (MON), salinomycin (SAL), narasin (NAR), maduramycin (MAD), nonactin (NON, internal standard), nigericin (NIG, surrogate), sulfamethazine (SMZ), sulfadimethoxine (SDM), sulfameter (SMT), sulfamethiazole (SMI), sulfamerazine (SMR), sulfachloropyridazine (SCP), sulfadiazine (SPD), sulfamethoxazole (SMX), tetracycline (TC), oxytetracycline (OTC), demeclocycline (DMC, internal standard), minocycline (MIN, surrogate), azithromycin (AZI), clarithromycin (CLA), erythromycin (ERY), roxithromycin (ROX), tilmicosin (TIL), and tylosin (TYL) were purchased from Sigma Aldrich (St. Louis, MO, United States). Spiramycin (SPI; as a mixture of spiramycin II, and III), and sulfathiazole (STZ) were purchased from ICN Biomedicals, Inc. (Costa Mesa, CA, United States). Chlortetracycline (CTC), 4-epichlortetracycline (ECTC), anhydrochlortetracycline (ACTC), and anhydrotetracycline (ATC) were purchased from Acros Organics (now Fisher Scientific, Waltham, MA, United States). 4-Epitetracycline (ETC) was purchased from Spectrum Chemical Mfg. Corp. (New Brunswick, NJ, United States) Tiamulin fumarate (TIA) was purchased from Sigma Aldrich (St. Louis, MO, United States). Standards of Phenyl–13C6–sulfamethazine (13C6-SMZ), D4–sulfamethoxazole (D4-SMX), 13CD3-anhydroerythromycin (13CD3-AERY), and D10–carbamazepine (D10-CBZ) were purchased from Cambridge Isotope Laboratories, Inc. (Tewksbury, MA, United States). Surrogate standard D10-tiamulin (D10-TIA) was purchased from Toronto Research Chemicals (Toronto, ON, Canada). Liquid chromatography mass spectrometry (LC-MS) grade methanol (MeOH) and acetonitrile (ACN) were purchased from EMD Millipore (Darmstadt, Germany). High performance liquid chromatography (HPLC) grade ethyl acetate (EtOAc) was purchase from VWR Chemicals BDH® (Radnor, PA, United States). Disodium ethylenediamine tetraacetate (EDTA) and sodium hydroxide (NaOH) were purchased from Fisher Chemical (Fairlawn, NJ, United States). Citric acid monohydrate and anhydrous dibasic sodium phosphate (Na2HPO4) were purchased from Sigma Aldrich (St. Louis, MO, United States). Phosphoric acid, formic acid (88%), and Ammonium Acetate were of ACS grade and obtained through J.T. Baker (Phillipsburg, NJ, United States). The multi-element inductively-coupled plasma mass spectrometry (ICP-MS) certified reference standard (Model 82026-108) was purchased from VWR Chemicals BDH® ARISTAR® (Radnor, PA, United States). The rhodium (Rd) ICP-MS internal standard was purchased from Sigma Aldrich (St. Louis, MO, United States). TraceMetal™ Grade nitric acid (67%–70%) and hydrogen peroxide, 30% (Certified ACS grade), was purchased from Fisher Chemical (Waltham, MA, United States). Water (18.2 MΩ-cm) used throughout all experiments was purified using a Barnstead NANOpure™ Diamond system (Waltham, MA, United States).
Swine manure samples were collected in summer 2020 from manure lagoons at swine farms in Iowa, United States. The locations of the farms where the manure samples were collected are not disclosed in this study to avoid privacy infringement. However, the farms were independently owned and geographically independent from each other. All manure samples collected were from swine farms representative of those typical in Iowa. 14 manure samples were collected from three stages of production: nine wean-finish (WF), four grow-finish (GF), and one gilt development (GD); and from farms using two types of feed compositions. The two feed rations were generally composed of corn, soybean, and distillers dried grains with solubles (DDGS), mixed in different proportions. Additionally, two facility ventilation practices, curtain sided and hybrid curtain/tunnel fan, were utilized at the studied farms. Ventilation practices varied with feed composition among farms. Data on stage of production and feed/facility practices were collected to provide further understanding for correlation statistics.
Manure samples were prepared using solid phase extraction (SPE) and analyzed for thirty antimicrobials including five ionophores, seven tetracyclines, nine sulfonamides, eight macrolides, and one pleuromutilin, using liquid chromatography-tandem mass spectrometry (LC-MS/MS). One tetracycline (tetM) and one macrolide (ermB) gene were chosen to be analyzed based on their high occurrence in swine manure from previous literature (Whitehead and Cotta, 2013; Miller et al., 2020), and were quantified by quantitative polymerase chain reaction (qPCR) (Bio-Rad Laboratories, Inc.; Hercules, CA, United States). Genes conferring resistance to aminoglycosides (AMG: str, aadD, and aadA2), beta-lactams (BLA: blaPSE, and blaOXA-10), chloramphenicol (CAP: cmlA5, and cmlA1), fluroquinolones (FQ: floR), lincosamides (LIN: lnuC, and lnuA), sulfonamides (SUL: sul2, and sul1), tetracyclines (TET: tetX, tetW, tetT, tetO, tetM, tetL, tetA, and tet36), macrolides (MAC: ermT, ermQ, ermF, ermC, ermB, erm36, and erm35), and four mobile genetic elements (MGE: intl3, intl2, intl1F165-clinical, and intl1-a-marko) were monitored for presence by high-throughput qPCR (Biomark, Inc.; Boise, ID, United States). Finally, manure samples were digested and analyzed by ICP-MS for ten metals including barium (Ba), cadmium (Cd), cobalt (Co), copper (Cu), iron (Fe), manganese (Mn), lead (Pb), strontium (Sr), uranium (U), and zinc (Zn).
Approximately 100 mg of lyophilized, and homogenized manure were added to a 15-ml polypropylene centrifuge tube, spiked with 200 µl of NIG surrogate (200 ng ml−1), allowed to equilibrate for 30 min, and extracted using the method described in Hurst et al. (2018) without modification. Prior to LC-MS/MS analysis, samples were spiked with 50 ng ml−1 internal standard (NON) and split into 2× 200 µl aliquots for quantification by single-point standard addition; one aliquot was spiked with 20 µl of 500 ng ml−1 ionophore native mix in mobile phase (50 ng ml−1 in vial), and the other aliquot was volume adjusted with 20 µl mobile phase diluent.
Approximately 100 mg of lyophilized, homogenized manure were added to a polypropylene centrifuge tube, spiked with 200 µl of surrogate standard mix (200 ng ml−1 final concentration for each of DMC, 13C6-SMZ, D10-TIA, 13CD3-AERY), and allowed to equilibrate in the dark for 30 min. Samples were then extracted using the method described in Wallace and Aga (2016) with slight modification. Following SPE elution, MeOH eluates were reduced to 200 µl under a stream of N2 gas and spiked with 25 µl internal standard mix (50 ng ml−1 of each D10-CBZ, D4-SMX, DMC in the final concentration), and reconstituted to 1 ml with 0.1% formic acid in water. Aliquots of each manure extract were taken to make 2x, 10x, and 50x dilutions to quantify analytes based on standard addition. This procedure is explained in more detail in Section 2.5 below. Undiluted and diluted extracts were split into 2× 200 µl aliquots for quantification by single point standard addition. One aliquot was spiked with 20 µl of 500 ng ml−1, 1 µg ml−1, or 2 µg ml−1 analyte mixtures in 0.1% formic acid in water, resulting in final concentrations of 50 ng ml−1, 100 ng ml−1, or 200 ng ml−1 in vial, respectively. Unspiked samples were volume normalized with 20 µl of 0.1% formic acid in water only. Samples were vortexed and then centrifuged for 5 min at 7,000 g prior to LC-MS/MS analysis.
The DNA from 0.25 g wet weight (w.w.) of manure samples were extracted with the MagAttract® PowerSoil® DNA EP Kit (Qiagen; Germantown, MD, United States) and an epMotion® 5075 automated robot (Eppendorf; Framingham, MA, United States). The eluted DNA was further cleaned with a Clean and Concentrator kit (Zymo Research; Irvine, CA, United States). The concentrations of DNA were measured with the Quant-iT™ dsDNA Assay Kit, high sensitivity (ThermoFisher Scientific Inc.; Waltham, MA, United States) and DNA samples were stored at −80°C until further analysis.
Ionophores were analyzed according to Hurst et al. (2018), but with runtime increased from 20 to 20.5 min to allow for complete elution of ionophores (Hurst et al., 2018). Sulfonamide, tetracycline, macrolide, and tiamulin antimicrobials were analyzed using an Agilent 6410 triple quadrupole mass spectrometer equipped with an Agilent 1100 HPLC system and electrospray ionization (ESI) source operated in positive mode (Palo Alto, CA, United States). Analytical separation of antimicrobials was achieved using a 10-µl injection volume on a Raptor™ C18 column (100 mm, 2.1 mm I.D, 2.7 µm particle size) (Restek Corp.; Belefonte, PA, United States), and flow rate of 0.4 ml min−1. The mobile phase consisted of (A) 0.1% formic acid in water and (B) 0.1% formic acid in ACN. The gradient profile consisted of 10% (B) ramped to 35% (B) over 4.5 min and 55% (B) over 2.5 min. This condition was held for 3 min before returning to the starting mobile phase of 10% (B). The total run time for each injection was 17 min. The analytical figures of merit for each analyte, including method limits of detection (LOD), can be found in the Supplementary Section S2.1 and Supplementary Table S1.
Lyophilized manure was digested for metal analysis according to US Environmental Protection Agency (USEPA) Method 3050B with minor modifications to ensure complete effervescence. Briefly, 0.2 g of lyophilized manure was added to a 50-ml digestion tube with reflux cap, and refluxed in 2.5 ml (1:1, v:v) water: Fuming nitric acid in a heating block for 15 min at 95°C. The tube was then removed from the heating block and cooled to ambient temperature. An additional 1.25-ml of nitric acid was then added into a tube and refluxed again for 30 min at 95°C. Samples were again cooled and a third 1.25 ml volume of nitric acid was added into a tube and refluxed at 95°C for 30 min. Next, samples were carefully digested in 0.5 ml of water and 0.75 ml of 30% hydrogen peroxide solution at 95°C until the peroxide reaction dissipated (effervescence ends). Tubes were removed from the heating block, cooled, and an additional 0.5 ml of 30% hydrogen peroxide solution was added before refluxing again at 95°C until effervescence ceased. This step was performed three times prior to a final 30-min reflux. Finally, acid digestates were diluted to 25 ml with water, spiked with 10 ng ml−1 internal standard 103Rd and filtered using a 0.45 µm PTFE (Environmental Express®; Charleston, SC, United States) filter before analysis by ICP-MS. Metals were quantified using a Thermo X-Series 2 ICP-MS (ThermoFisher Scientific Inc., Waltham, MA, United States) using Collision Cell Technology (CCT) mode for Fe, Co, Sr, Cd, and U to reduce polyatomic interferences), and standard mode for Mn, Cu, Zn, and Ba. Metals were quantified based on their respective external standard curves using the most abundant isotope, normalized to the internal standard 103Rd. Samples were analyzed undiluted, 10x diluted, and 50x diluted due to the wide range of metal concentrations present.
The following quality control/assurance criteria were established following the suggested guide in a publication by Angeles and Aga (2018) to ensure positive detections. The criteria requires: 1) an ion intensity ≥ 103 for all monitored m/z signals; 2) peaks for monitored fragment ions must have area ratios for quantitative:qualitative (Q:q) within 20%, 35%, 60%, and 40% of the expected ratios (Table 1) for ionophores, sulfonamides, tetracyclines, and macrolides, respectively, 3) peaks must elute within a ±0.5-min retention time (RT) window around the corresponding standard peak; and 4) the calculated concentration must be above the established LOD for the particular analyte (Angeles and Aga, 2018). Tolerance percentages for criteria 2 were determined by the range at which 95% of the spiked sample ratios were considered accepted ratios for each analyte class. To consider an analyte as positive detection, three out of the four criteria needs to be met.
TABLE 1. Optimized parameters for LC-MS/MS analysis of sulfonamides, tetracyclines, macrolides, and tiamulin (m/z values for precursor and fragment ions, fragmentor voltages, collision energies, and quantifying: qualifying ion ratios).
Here, we applied standard addition to quantify analytes due to the absence of commercially-available, stable isotope-labelled tetracycline standards, and to maintain a consistent quantification for all antimicrobial analytes. Standard addition quantification requires that the spiked concentrations be 50%–100% of the expected concentration in the sample (ThermoFisher Scientific, 2016). Preliminary analyses revealed variable, analyte-dependent concentrations. For example, the detected analytes of all antimicrobial classes analyzed within the same LC-MS/MS method, exhibited a concentration range of up to about 9,000 times the lowest detection within a single sample. Therefore, it was necessary to analyze both undiluted and diluted samples at three different standard addition levels to quantify analytes present at high and low concentrations. In brief, for each sample or diluted sample, a corresponding 200-µl aliquot was spiked with native analytes and analyzed in series with the unspiked aliquot for quantification. The undiluted and 2x diluted samples utilized 50 µg L−1 and 100 µg L−1, respectively, of standard-added concentrations, and 10x and 50x diluted samples utilized 200 µg L−1 standard-added concentrations to quantify for all analytes.
The ermB, tetM, and 16S rRNA gene copies were quantified in DNA samples by qPCR using standard curves specific to each gene ranging from 101 to 107 gene copies. The sample DNA was diluted 1:10 prior to quantification. There were three technical replicates included for each sample. We removed any outliers above 1.5 x the standard deviation of the three sample replicates. Absolute gene copies were based on the standard curve, and copies gram−1 of manure were back-calculated using the following equation:
Statistical tests to determine AMR-stressor relationships for the manure was performed using Spearman’s rank correlation in R. Feed ration compositions were coded -1 and 1 such that negative ρ values indicate correlation to diet 1 and positive ρ values indicate correlation to diet 2. Samples with non-detects were treated as 0 µg g−1 for correlation calculations to avoid data gaps during statistical analysis that could lead to spurious correlations.
Out of 14 swine manure samples analyzed, 12 contained ionophores. However, only LAS and MON were detected, with concentrations ranging from 0.002 µg g−1 to 0.547 µg g−1 d.w. These results are notable because narasin is currently the only ionophore approved in the US for use in swine to promote growth (Rovira and Sturos, 2016). The presence of LAS and MON in manure may be attributed to their use as manure additives during storage to reduce foaming, rather than as animal medication (Berg, 2012). This is highly likely given that tiamulin combined with ionophores could be toxic to swine, and therefore should not be used together in feed (Rovira and Sturos, 2016).
Tetracyclines dominated the antimicrobial profiles of each sample, though each contained a unique combination of tetracycline analytes (Figure 1). In this study, OTC was detected in all samples with concentrations ranging from 2.39 to 1,260 µg g−1 d.w. Oxytetracycline is generally used in veterinary medicine as a broad spectrum antimicrobial for the treatment of gastrointestinal and respiratory diseases (Aktas and Yarsan, 2017). Oxytetracycline is typically administered via intravenous or intramuscular route, however, oral administration is more ideal when administering to large populations of food-producing animals (Martin-Jimenez et al., 1997). The supplementation of feed with sub-therapeutic concentrations of OTC has also been reported to promote swine growth (Soler et al., 2016). Most of the detections in this study fell within literature values of OTC in swine manure, which were reported from 0.21 to 354 µg g−1 d.w. (Martínez-Carballo et al., 2007; Chen et al., 2012). Additionally, the US Food and Drug Administration (FDA) approved dosage for swine is between 6.61 and 19.8 mg kg−1 body weight depending on treatment purpose (USFDA, 2004). Sample 5 is a notable exception with 1,260 µg g−1 d.w., which may be attributable to recent, wide-spread therapeutic OTC use in response to systemic infection.
FIGURE 1. (A) Antimicrobial and transformation product concentrations and (B) antimicrobial and transformation product profiles of 14 swine manure samples from Iowa farms, analyzed by liquid chromatography tandem mass spectrometry (LC-MS/MS). Antimicrobials: Anhydrochlortetracycline (ACTC), anhydrotetracycline (ATC), chlortetracycline (CTC), epichlortetracycline (ECTC), epitetracycline (ETC), oxytetracycline (OTC), tetracycline (TC), tiamulin (TIA), tilmicosin (TIL), lasalocid (LAS), and monensin (MON).
Chlortetracycline and ECTC were detected in about 75% of the samples at concentrations ranging from 0.75 to 37 µg g−1 d.w., while TC and ATC detections ranged from 0.25 to 9.7 µg g−1 d.w in 71%–93% of samples. ACTC and ETC were present in the lowest concentrations, ranging from 0.0204 to 1.79 µg g−1 d.w., but were present at concentrations above the LOD in 86%–93% of samples. Oxytetracycline was the most detected tetracycline with detections frequency decreasing according to the series: OTC > CTC > ECTC > TC > ATC > ACTC > ETC. This trend is in general agreement with the values reported by Qiao et al. (2012), who reported swine manure detections of CTC (9 µg g−1 d.w.) and OTC (2.5 µg g−1 d.w.) well above those of other tetracyclines (Qiao et al., 2012). The relatively high detection frequency and concentrations of tetracyclines were anticipated because tetracyclines represent the largest class of antimicrobials sold for use in swine by mass, with a total of 3,948,745 kg sold in the US for agricultural use in 2020 (USFDA, 2021).
Of the macrolide antimicrobials monitored, only TIL was detected, and it was present in only 4 manure samples. Concentrations did not exceed 0.472 µg g−1 d.w. for TIL. Despite the use of ERY in swine, ERY residues were not detected in any of the manure samples. In contrast, TIA was present in all samples at concentrations ranging from <LOD to 9.4 µg g−1 d.w. According to literature, the recommended dosage is 10 mg TIL and 12 mg TIA per kg of body weight per day, administered via animal feed (Perruchon et al., 2022).
Recent work by Hughes and Andersson (2012) indicates that sub-MIC levels of antimicrobials can lead to rapidly enriched resistance mutations and de novo ARG selection in impacted pathogens. The low-levels of antimicrobials promote selection of variants with reduced susceptibility to antimicrobial activity by allowing for continued microbial growth in the presence of the chemical stressor and opportunity for horizontal transfer. Although only a few significant statistical relationships were observed during this study, it is possible that the low antimicrobial levels are contributing to the proliferation of AMR in a complex, synergistic fashion that is not readily apparent by traditional statistical analyses. As a result, it is imperative to examine selection pressure holistically and not discount the potential impact of low-level antimicrobials on AMR development in swine manure and in soil that receives manure application.
Metals can be classified as essential, non-essential, less toxic, and highly toxic according to their importance to metabolic function (Kochare and Tamir, 2015). However, some of the essential metals such as Cu, Zn, Co, Cr, Mn, and Fe can be toxic if administered in excessive doses. For example, the recommended levels of supplementation for growing swine are 6 mg kg−1 body weight for Cu and 100 mg kg−1 body weight for Fe and Zn (NRC, 2012). Copper, Fe, and Zn were detected in all samples, with maximum concentrations of 887, 1,900, and 2,100 µg g−1 d.w., respectively. The concentrations of Cu and Zn observed in this study exceeded the MCCs of 11.8 µg g−1 and 22.8 µg g−1 in manure, respectively; these levels are consistent with other studies (Hölzel et al., 2012; Seiler and Berendonk, 2012). For example, Zhang et al. (2012) reported Cu concentrations between 77.62 and 1,521.43 mg kg−1 (d.w.), and Zn concentrations between 63.37 and 1,622.81 mg kg−1 (d.w.) in swine manures (Zhang et al., 2012). Additionally, Moral et al. (2008) reported values of 11–80 g Cu m−1, 87–168 g Fe m−1, and 75–533 g Zn m−1 in swine manure slurries (Moral et al., 2008). These elevated levels of Cu and Zn could be due to typical feed-mineral supplementation practices, driven by poor animal absorption (Ji et al., 2012).
Cobalt was present in one sample <0.55 µg kg−1 while Cd was not detected. Notably, the sample containing the highest total metal concentrations (sample 1, Figure 2), does not correspond with the sample containing the highest total antimicrobial concentrations (sample 5, Figure 1A). However, there is a moderate positive correlation between total metal concentrations and total antimicrobial concentrations (correlation coefficient, ρ = 0.64, p = 0.015). Iron concentrations were generally highest in WF swine, and Fe, Ba, and U concentrations were generally higher in swine fed with feed composition 1 (composed of corn, soybean, and DDGS, mixed in specific proportion).
FIGURE 2. Metal concentrations in swine manure samples from Iowa farms, measured by inductively-coupled plasma mass spectrometry (ICP-MS). Cobolt (59Co), strontium (88Sr), uranium (238U), iron (56Fe), barium (137Ba), zinc (66Zn), copper (65Cu), and manganese (55Mn).
Uranium (U) was included as a targeted metal in the ICP-MS analysis as a result of the detection of 238U during an initial survey analysis of digested manure. Observed U was present in all but two samples at concentrations ranging from 0.0048 to 0.77 µg g−1 (d.w.). U concentrations exceeding the USEPA drinking water maximum contaminant level (MCL) of 0.030 mg L−1 prompted further research into possible U contamination in the areas surrounding the sample locations (USEPA, 2004).
A number of sites in Iowa reportedly handled and disposed of U and other radioactive materials in the 1950s–1960s. One Iowa disposal site was categorized as a class “b- significant threat to the environment—action required” site in 1991 by the Iowa Department of Natural Resources (IDNR) (IDNR, 2021a). The IDNR reports the site was used in the early 1950s for uncontrolled disposal of unknown quantities and types of radioactive materials. Additionally, purification and manufacture of high purity U in support of the US Department of Energy (USDOE) occurred on the site (Shirley, 1996). U shavings were similarly burned on the ground surface near the waste burial sites, requiring remediation in the 1980s. Groundwater and soil sampling conducted between the 1990s and 2008 report U levels as high as 7,500 µg L−1 (in groundwater) at this disposal site post-remediation; however, off-site migration has not been reported. Additionally, the army and Atomic Energy Commission (AEC) performed nuclear weapon assembly, storage, and disassembly at an ammunition plant located in Iowa between 1947 and 1975 (Fuortes, 2001; IDNR, 2021b). These activities lead to site contamination with depleted U. In 1990, the site was placed on the National Priorities List for Uncontrolled Hazardous Waste Sites by the USEPA (IDNR, 2021b).
As of 2021, two public drinking water systems in Iowa, serving a population of 2,037 reported U detections ranging from 0.015 to 0.030 mg L−1 (USEPA, 2004; USEPA, 2021). Despite the historic use and disposal of U near the sampling locations, it remains unclear whether the U detected in swine manure originated from ingestion of feed and water or leaching into the manure pit. Our results do not suggest enrichment, however, a significant, positive correlation between U and diet composition 1 suggest possible feed contamination related to crop production or grain formulation. Further work should be performed to determine whether swine farming enriches the U, or if it is merely an artifact of former regional use. Additionally, it is possible that positive U detection is a result of direct manure contact with soils in swine lagoons. Nevertheless, the detection of U presents a potential concern for animal and human health, and has the potential to stimulate the proliferation of AMR (USEPA, 2004; Zhou et al., 2022).
The ermB and tetM genes have been quantified in swine manure from storage pits in previous studies (Whitehead and Cotta, 2013; Miller et al., 2020). In this study, manure samples contained between 0.2 and 1.44 copies g−1 manure (w.w.) of the macrolide-encoding ARG ermB (normalized to the 16S rRNA copies). This range of relative abundance was higher than previously reported. For instance, in Nebraska stored manure the reported ermB relative abundance was 0.017 per 16S rRNA gene w.w., and an Iowa study measured 0.010 per 16S rRNA gene w.w. (Lopatto et al., 2019; Miller et al., 2020). A much wider range of tetM genes were present in manure, with the number of copies ranging between 0.05 and 5.36 copies g−1 w.w. relative to the 16S rRNA gene (Table 2). The tetM gene has the widest bacteria host range of all tetracycline genes and is considered highly transferable, being located on transposons and integrases (Roberts, 2005). A previous study reported tetM gene copies as three times more abundant than 16S genes in a swine manure storage pit in Iowa (Alt et al., 2021).
TABLE 2. Levels of antimicrobial resistance genes, antimicrobials, and metals detected in swine manure samples from manure lagoons at 14 swine farms in Iowa.
Notably, TET genes were present in all manure samples, with the highest number of different genes detections in samples 12 and 13 (Figure 3). Interestingly, samples 12 and 13 have the fourth and sixth lowest total tetracycline concentrations of the 14 samples, respectively. These results comport with our previous work, reporting no observable correlation between tetracyclines and the corresponding TET ARGs (Hurst et al., 2019). MAC genes were present in all but two samples, samples 5 and 7, neither of which had macrolide detections. The presence of macrolides alone is insufficient to account for the presence of the MAC resistance observed in the study, considering non-detection of macrolides in 67% of samples that contained MAC resistant genes. These results suggest that co-selection of TET and MAC genes by other antimicrobials and chemical stressors could be an important ARG proliferation mechanism in the studied swine systems. Only three samples contained SUL genes (3, 6, and 14), however, none of the manure samples showed any positive sulfonamide detections. The ionophore, tetracycline, macrolide, and tiamulin antimicrobials detected in these samples, and the overall high levels of resistance genes further suggest that co-selection or cross-resistance is an important mechanism of AMR spread in manure. The greatest number of different MGEs was detected in sample 14 (3 of the 4 MGEs included in the method), which also exhibited the second highest total metal content, while all three AMGs detected during this study were present in samples 6 and 14. Interestingly, the sample containing the lowest number of total ARGs had the highest total metal content and third highest total antimicrobial concentration (sample 1, Table 2), indicating the need for correlational analyses to help elucidate the AMR mechanisms and selection pressures more fully. The absence of positive relationship between potential selection agents and total ARG content suggests complex relationships between existing selection agents and potentially susceptible microbiota, or that additional, unmonitored agents are present in manure and contributing to ARG development and proliferation; support for these hypotheses require further investigation.
FIGURE 3. Antimicrobial resistance gene presence in 14 swine manure samples from Iowa farms, measured by quantitative polymerase chain reaction (qPCR). Quantified genes: Tetracycline gene (tetM), and macrolide gene (ermB). Gene classes: macrolide (MAC), aminoglycosides (AMG), sulfonamide (SUL), tetracycline (TET), and mobile genetic element (MGE).
There has been a notable historical absence of relationships between ARGs and antimicrobials in literature to date (Ji et al., 2012; Hurst et al., 2019; Komijani et al., 2021). However, several studies have reported strong correlations between ARGs and heavy metals in water (Komijani et al., 2021; Zou et al., 2021), animal manures (Ji et al., 2012; Liu et al., 2022), and soils (Ji et al., 2012; Knapp et al., 2017). Therefore, a global analysis of AMR-stressor relationships was performed for the manure sample set using Spearman’s rank correlation (Figure 4; Supplementary Section S4; Supplementary Tables S4, S5).
FIGURE 4. Spearman correlation matrix between antimicrobial [anhydrochlortetracycline (ACTC), anhydrotetracycline (ATC), chlortetracycline (CTC), epichlortetracycline (ECTC), epitetracycline (ETC), oxytetracycline (OTC), tetracycline (TC), tiamulin (TIA), lasalocid (LAS), and monensin (MON)] antimicrobial resistance genes (ARGs) [macrolide (ermB) and tetracycline (tetM)], ARG classes [macrolide (MAC), aminoglycosides (AMG), sulfonamide (SUL), tetracycline (TET), and mobile genetic element (MGE)], metals [manganese (Mn), copper (Cu), zinc (Zn), barium (Ba), iron (Fe), uranium (U), strontium (Sr), cobalt (Co)], diet, and stage of swine production (Stage). Correlation coefficients colored according to the value as depicted by scale, where a strong positive correlation is indicated by a dark blue box and a strong negative correlation is depicted by a dark red box. Size of squares corresponds to p-value, where correlations with p-value > 0.05 are considered insignificant and therefore left blank. Sulfonamides, spiramycin II and III (SPI2, SPI3), erythromycin (ERY), tylosin (TYL), and cobalt (Co) not included due to lack of detections in >1 sample, or concentrations < LOD.
The following metals exhibited the most significant overall positive correlation with ATC and/or ACTC across the sample set: Cu (ATC: ρ = 0.67, p = 0.0093; ACTC: ρ = 0.75, p = 0.0022), and Ba (ATC: ρ = 0.84, p = 0.0002). TetM was not significantly correlated to any tetracycline antimicrobial concentrations. Interestingly, ermB and tetM genes are strongly, positively correlated to each other, which could suggest co-selection, despite the lack of individual ARG and tetracycline correlations. The number of different TET genes detected had strong negative correlations with ATC (ρ = −0.60, p = 0.024), which could be due high cytotoxicity of ATC relative to the native compound or a bactericidal mode of action different from the native compound (Halling-Sørensen et al., 2002), which likely inhibited bacterial growth and variant selection when present (Hughes and Andersson, 2012). The number of different SUL genes detected exhibited strong, positive correlation with TIL (ρ = 0.71, p = 0.0041), indicating possible co-selection between the macrolide and sulfonamide resistance genes. Moreover, the presence of TET and MAC were negatively correlated with Ba concentrations (TET: ρ = −0.60, p = 0.0242) (MAC: ρ = −0.73, p = 0.0033), suggesting that Ba exhibits limited selection pressure in the studied systems. The limited number of significant correlations observed in this study suggest the relationship between chemical stressors and ARG proliferation is extremely complex and may require studies under controlled conditions to fully understand the role of chemical stressors and the mechanisms of ARG development and spread in manure. Additionally, Lundström et al. (2016) reported low-level (1–10 µg L−1) tetracycline exposure in aquatic environments resulted in increased expression of both TET resistance genes and genes conferring resistance to different classes of antimicrobials, supporting the conclusion of co-selection of TET and MAC genes observed in this study (Lundström et al., 2016).
This study analyzed and compared levels of antimicrobials and metals to ARGs in swine manure, to determine any correlations between the levels of ARGs and the types of chemical stressors present in manure. No strong positive correlation between ARGs and the metals analyzed were observed. Instead, a weak correlation between antimicrobial concentrations and the presence of corresponding class of resistance genes was observed. For example, tetM gene concentrations were not correlated with tetracycline antimicrobials. Genes conferring resistance to specific classes of antimicrobials occurred at sites that did not contain those compounds, such as SUL gene presence in samples with only ionophore, tetracycline, macrolide, and pleuromutilin detections. Furthermore, results indicated tetM and ermB copies correlated significantly with each other. These findings suggest possible co-selection or cross-resistance, as indicated by (Lundström et al., 2016). It should be noted that some metal concentrations (Fe, Ba, and U) were positively correlated to diet 1, while Fe concentrations were positively correlated to an earlier stage of life (ex. WF). Future studies exploring correlations between AMR and other potential chemical stressors in manure are necessary to reveal other possible AMR-promoting agents. Additionally, the presence of U in 85% swine manure samples at levels up to 0.77 µg g−1 was unexpected and merits additional research to determine to what extent do chemical stressors other than antimicrobials contribute to AMR spread in agroecosystems.
Animal manure is an important source of nutrients and organic matter that can be used to improve soil health. However, untreated manure may be a continuous source of chemical stressors that increase selection pressure promoting AMR development and proliferation in receiving soils. The potential for AMR transmission from edible crops grown in soils fertilized with untreated manure, and the lack of understanding of the degree of human exposure to AMR generated through agriculture is a critical issue within the One Heath Framework. Results from this research provide new information that can be used to encourage better management practices that will lead to the reduction of AMR spread in agroecosystems.
The datasets presented in this study can be found in online repositories. The names of the repository/repositories and accession number(s) can be found below: https://datadryad.org/stash/dataset/doi:10.5061/dryad.v6wwpzh03.
JC: Conceptualization, methodology, investigation, writing—original draft. TN: Conceptualization, methodology, investigation, writing—original draft. AH: Conceptualization, funding acquisition, reviewing of manuscript. MS: Conceptualization, funding acquisition, reviewing of manuscript. JW: Conceptualization, methodology, writing—original draft. DA: Conceptualization, writing—original draft, funding acquisition.
This research was financially supported in part by the National Institute of Food and Agriculture, U.S. Department of Agriculture, under award number 2021-68015-33495.
The authors would like to acknowledge Jonathan Antle and Dr. Zia Ahmed for their contribution to RStudio script writing for statistical analyses.
The authors declare that the research was conducted in the absence of any commercial or financial relationships that could be construed as a potential conflict of interest.
All claims expressed in this article are solely those of the authors and do not necessarily represent those of their affiliated organizations, or those of the publisher, the editors and the reviewers. Any product that may be evaluated in this article, or claim that may be made by its manufacturer, is not guaranteed or endorsed by the publisher.
Any opinions, findings, conclusion, or recommendations expressed in this publication are those of the author(s) and do not necessarily reflect the view of the U.S. Department of Agriculture.
The Supplementary Material for this article can be found online at: https://www.frontiersin.org/articles/10.3389/fenvs.2022.1018739/full#supplementary-material
Aktas, İ., and Yarsan, E. (2017). Pharmacokinetics of conventional and long-acting oxytetracycline preparations in kilis goat. Front. Vet. Sci. 4, 229. doi:10.3389/fvets.2017.00229
Alt, L. M., Iverson, A. N., Soupir, M. L., Moorman, T. B., and Howe, A. (2021). Antibiotic resistance gene dissipation in soil microcosms amended with antibiotics and swine manure. J. Environ. Qual. 50, 911–922. doi:10.1002/jeq2.20240
Angeles, L. F., and Aga, D. S. (2018). Establishing analytical performance criteria for the global reconnaissance of antibiotics and other pharmaceutical residues in the aquatic environment using liquid chromatography-tandem mass spectrometry. J. Anal. Methods Chem, 1–9. doi:10.1155/2018/7019204
Berg, L. (2012). Manure pit foaming remains a perplexing problem. Available:https://www.nationalhogfarmer.com/environment/manure-pit-foaming-remains-perplexing-problem (Accessed 2022).
Chee-Sanford, J. C., Mackie, R. I., Koike, S., Krapac, I. G., Lin, Y.-F., Yannarell, A. C., et al. (2009). Fate and transport of antibiotic residues and antibiotic resistance genes following land application of manure waste. J. Environ. Qual. 38, 1086–1108. doi:10.2134/jeq2008.0128
Chen, Y., Zhang, H., Luo, Y., and Song, J. (2012). Occurrence and assessment of veterinary antibiotics in swine manures: A case study in east China. Chin. Sci. Bull. 57, 606–614. doi:10.1007/s11434-011-4830-3
Congilosi, J. L., and Aga, D. S. (2021). Review on the fate of antimicrobials, antimicrobial resistance genes, and other micropollutants in manure during enhanced anaerobic digestion and composting. J. Hazard. Mater. 405, 123634. doi:10.1016/j.jhazmat.2020.123634
De Mille, C. M., Burrough, E. R., Kerr, B. J., Schweer, W. P., and Gabler, N. K. (2022). Dietary pharmacological zinc and copper enhances voluntary feed intake of nursery pigs. Front. Anim. Sci. 3. doi:10.3389/fanim.2022.874284
Dębski, B. (2016). Supplementation of pigs diet with zinc and copper as alternative to conventional antimicrobials. Pol. J. Vet. Sci. 19, 917–924. doi:10.1515/pjvs-2016-0113
Fuortes, L. (2001). Iowa needs assessment-December 2001: Burlington atomic energy commission plant—former worker program. Available at: https://www.energy.gov/sites/default/files/2013/10/f3/iowa_needsassessment.pdf.
Halling-Sørensen, B., Sengeløv, G., and Tjørnelund, J. (2002). Toxicity of tetracyclines and tetracycline degradation products to environmentally relevant bacteria, including selected tetracycline-resistant bacteria. Arch. Environ. Contam. Toxicol. 42, 263–271. doi:10.1007/s00244-001-0017-2
Hill, G. M., Cromwell, G. L., Crenshaw, T. D., Dove, C. R., Ewan, R. C., Knabe, D. A., et al. (2000). Growth promotion effects and plasma changes from feeding high dietary concentrations of zinc and copper to weanling pigs (regional study). J. Animal Sci. 78, 1010–1016. doi:10.2527/2000.7841010x
Hölzel, C. S., Müller, C., Harms, K. S., Mikolajewski, S., Schäfer, S., Schwaiger, K., et al. (2012). Heavy metals in liquid pig manure in light of bacterial antimicrobial resistance. Environ. Res. 113, 21–27. doi:10.1016/j.envres.2012.01.002
Hughes, D., and Andersson, D. I. (2012). Selection of resistance at lethal and non-lethal antibiotic concentrations. Curr. Opin. Microbiol. 15, 555–560. doi:10.1016/j.mib.2012.07.005
Hurst, J. J., Oliver, J. P., Schueler, J., Gooch, C., Lansing, S., Crossette, E., et al. (2019). Trends in antimicrobial resistance genes in manure blend pits and long-term storage across dairy farms with comparisons to antimicrobial usage and residual concentrations. Environ. Sci. Technol. 53, 2405–2415. doi:10.1021/acs.est.8b05702
Hurst, J. J., Wallace, J. S., and Aga, D. S. (2018). Method development for the analysis of ionophore antimicrobials in dairy manure to assess removal within a membrane-based treatment system. Chemosphere 197, 271–279. doi:10.1016/j.chemosphere.2018.01.028
IDNR, (2021a). Ames laboratory, chemical disposal site (Ames, IA). Available at:tabid=717 https://www.iowadnr.gov/portals/idnr/uploads/consites/rames.pdf?amp.
IDNR, (2021b). United States of America (IAAAP). Available at:tabid=717 https://www.iowadnr.gov/portals/idnr/uploads/consites/rusaiaap.pdf?amp.
Antimicrobial Resistance Collaborators: Murray, C. J. L., Ikuta, K. S., Sharara, F., Swetschinski, L., Robles Aguilar, G., et al. (2022). Global burden of bacterial antimicrobial resistance in 2019: A systematic analysis. Lancet 399, 629–655. doi:10.1016/S0140-6736(21)02724-0
Ji, X., Shen, Q., Liu, F., Ma, J., Xu, G., Wang, Y., et al. (2012). Antibiotic resistance gene abundances associated with antibiotics and heavy metals in animal manures and agricultural soils adjacent to feedlots in Shanghai; China. J. Hazard. Mater. 235-236, 178–185. doi:10.1016/j.jhazmat.2012.07.040
Knapp, C. W., Callan, A. C., Aitken, B., Shearn, R., Koenders, A., and Hinwood, A. (2017). Relationship between antibiotic resistance genes and metals in residential soil samples from Western Australia. Environ. Sci. Pollut. Res. 24, 2484–2494. doi:10.1007/s11356-016-7997-y
Kochare, T., and Tamir, B. (2015). Assessment of dairy feeds for heavy metals. Am. Sci. Res. J. Eng. Technol. Sci. 11, 20–31. Available at: https://asrjetsjournal.org/index.php/American_Scientific_Journal/article/view/541.
Komijani, M., Shamabadi, N. S., Shahin, K., Eghbalpour, F., Tahsili, M. R., and Bahram, M. (2021). Heavy metal pollution promotes antibiotic resistance potential in the aquatic environment. Environ. Pollut. 274, 116569. doi:10.1016/j.envpol.2021.116569
Kumar, K., Gupta, C. S., Chander, Y., and Singh, A. K. (2005). Antibiotic use in agriculture and its impact on the terrestrial environment. Adv. Agron. 87, 1–54. doi:10.1016/S0065-2113(05)87001-4
Liu, C., Li, G., Qin, X., Xu, Y., Wang, J., Wu, G., et al. (2022). Profiles of antibiotic- and heavy metal-related resistance genes in animal manure revealed using a metagenomic analysis. Ecotoxicol. Environ. Saf. 239, 113655. doi:10.1016/j.ecoenv.2022.113655
Lopatto, E., Choi, J., Colina, A., Ma, L., Howe, A., and Hinsa-Leasure, S. (2019). Characterizing the soil microbiome and quantifying antibiotic resistance gene dynamics in agricultural soil following swine CAFO manure application. PLOS ONE 14, e0220770. doi:10.1371/journal.pone.0220770
Lundström, S. V., Östman, M., Bengtsson-Palme, J., Rutgersson, C., Thoudal, M., Sircar, T., et al. (2016). Minimal selective concentrations of tetracycline in complex aquatic bacterial biofilms. Sci. Total Environ. 553, 587–595. doi:10.1016/j.scitotenv.2016.02.103
Manyi-Loh, C., Mamphweli, S., Meyer, E., and Okoh, A. (2018). Antibiotic use in agriculture and its consequential resistance in environmental sources: Potential public health implications. Molecules 23, 795. doi:10.3390/molecules23040795
Martin-Jimenez, T., Craigmill, A. L., and Riviere, J. E. (1997). Extralabel use of oxytetracycline. J. Am. Vet. Med. Assoc. 211, 42–44. https://pubmed.ncbi.nlm.nih.gov/9215409/.
Martínez-Carballo, E., González-Barreiro, C., Scharf, S., and Gans, O. (2007). Environmental monitoring study of selected veterinary antibiotics in animal manure and soils in Austria. Environ. Pollut. 148, 570–579. doi:10.1016/j.envpol.2006.11.035
Medardus, J. J., Molla, B. Z., Nicol, M., Morrow, W. M., Rajala-Schultz, P. J., Kazwala, R., et al. (2014). In-feed use of heavy metal micronutrients in U.S. swine production systems and its role in persistence of multidrug-resistant salmonellae. Appl. Environ. Microbiol. 80, 2317–2325. doi:10.1128/AEM.04283-13
Miller, D. N., Jurgens, M. E., Durso, L. M., and Schmidt, A. M. (2020). Simulated winter incubation of soil with swine manure differentially affects multiple antimicrobial resistance elements. Front. Microbiol. 11, 611912. doi:10.3389/fmicb.2020.611912
Moral, R., Perez-Murcia, M. D., Perez-Espinosa, A., Moreno-Caselles, J., Paredes, C., and Rufete, B. (2008). Salinity, organic content, micronutrients and heavy metals in pig slurries from South-eastern Spain. Waste Manag. 28, 367–371. doi:10.1016/j.wasman.2007.01.009
NRC, (2012). Nutrient requirements of swine. Eleventh revised edition. Washington, DC: The National Academies Press. https://nap.nationalacademies.org/catalog/13298/nutrient-requirements-of-swine-eleventh-revised-edition.
Perruchon, C., Katsivelou, E., Karas, P. A., Vassilakis, S., Lithourgidis, A. A., Kotsopoulos, T. A., et al. (2022). Following the route of veterinary antibiotics tiamulin and tilmicosin from livestock farms to agricultural soils. J. Hazard. Mater. 429, 128293. doi:10.1016/j.jhazmat.2022.128293
Qiao, M., Chen, W., Su, J., Zhang, B., and Zhang, C. (2012). Fate of tetracyclines in swine manure of three selected swine farms in China. J. Environ. Sci. 24, 1047–1052. doi:10.1016/S1001-0742(11)60890-5
Roberts, M. C. (2005). Update on acquired tetracycline resistance genes. FEMS Microbiol. Lett. 245, 195–203. doi:10.1016/j.femsle.2005.02.034
Rovira, A., and Sturos, M. (2016). Diagnosis of ionophore intoxications in pigs. Available: https://www.nationalhogfarmer.com/nutrition/diagnosis-ionophore-intoxications-pigs (Accessed 2022).
Seiler, C., and Berendonk, T. (2012). Heavy metal driven co-selection of antibiotic resistance in soil and water bodies impacted by agriculture and aquaculture. Front. Microbiol. 3, 399. doi:10.3389/fmicb.2012.00399
Shirley, R. S. (1996). The Ames laboratory chemical disposal site removal action: Source removal, processing, and disposal. Proceedings of the Spectrum '96: International conference on nuclear and hazardous waste management. Seattle, WA. Available at: https://www.osti.gov/biblio/474045.
Soler, L., Miller, I., Hummel, K., Razzazi-Fazeli, E., Jessen, F., Escribano, D., et al. (2016). Growth promotion in pigs by oxytetracycline coincides with down regulation of serum inflammatory parameters and of hibernation-associated protein HP-27. Electrophoresis 37, 1277–1286. doi:10.1002/elps.201500529
Srivastava, V., Sarkar, A., Singh, S., Singh, P., De Araujo, A. S. F., and Singh, R. P. (2017). Agroecological responses of heavy metal pollution with special emphasis on soil health and plant performances. Front. Environ. Sci. 5. doi:10.3389/fenvs.2017.00064
ThermoFisher Scientific (2016). Why and how to matrix spike: Document #ST-MATSPIKE-E-0416. https://assets.thermofisher.com/TFS-Assets/LPD/Product-Information/Why-How-Matrix-Spike-ST-MATSPIKE-EN.pdf.
USDA, (2022). Quarterly hogs and pigs. Washington, DC: NASS. June 2022. Available at: https://usda.library.cornell.edu/concern/publications/rj430453j?locale=en.
USDA, (2019). Antimicrobial use and stewardship on U.S. swine operations, 2017. Fort Collins, CO. USDA-APHIS-VS-CEAH-NAHMS. Available at: https://www.aphis.usda.gov/animal_health/nahms/downloads/amu-swine-operations.pdf.
USEPA, (2004). National primary drinking water regulations: Analytical method for uranium, 04-19333, 52176–52181. Available at: https://www.federalregister.gov/documents/2004/08/25/04-19333/national-primary-drinking-water-regulations-analytical-method-for-uranium.
USEPA, (2021). Iowa public health tracking portal: Uranium testing results in public water. https://tracking.idph.iowa.gov/Environment/Public-Drinking-Water/Uranium-Testing-Data.Iowa.gov
USFDA, (2004). Implantation or injectable dosage form new animal drugs; oxytetracycline. Fed. Reg., 69 (31), 878–879. Available at: https://www.federalregister.gov/documents/2004/06/08/04-12839/implantation-or-injectable-dosage-form-new-animal-drugs-oxytetracycline.
USFDA, (2021). 2020 Summary report on antimicrobials sold or distributed for use in food-producing animals Silver Spring, MD. Available at: https://www.fda.gov/media/154820/download.
Wallace, J. S., and Aga, D. S. (2016). Enhancing extraction and detection of veterinary antibiotics in solid and liquid fractions of manure. J. Environ. Qual. 45, 471–479. doi:10.2134/jeq2015.05.0246
Whitehead, T. R., and Cotta, M. A. (2013). Stored swine manure and swine faeces as reservoirs of antibiotic resistance genes. Lett. Appl. Microbiol. 56, 264–267. doi:10.1111/lam.12043
Who, (2019). Critically important antimicrobials for human medicine. Geneva: World Health Organization. https://apps.who.int/iris/bitstream/handle/10665/312266/9789241515528-eng.pdf.
Wong, A. (2019). Unknown risk on the farm: Does agricultural use of ionophores contribute to the burden of antimicrobial resistance? mSphere 4, e00433-19, 1–6. doi:10.1128/mSphere.00433-19
Zhang, F., Li, Y., Yang, M., and Li, W. (2012). Content of heavy metals in animal feeds and manures from farms of different scales in northeast China. Int. J. Environ. Res. Public Health 9, 2658–2668. doi:10.3390/ijerph9082658
Zhang, S., Gu, J., Wang, C., Wang, P., Jiao, S., He, Z., et al. (2015). Characterization of antibiotics and antibiotic resistance genes on an ecological farm system. J. Chem, 1–8. doi:10.1155/2015/526143
Zhang, Y., Zhang, C., Parker, D. B., Snow, D. D., Zhou, Z., and Li, X. (2013). Occurrence of antimicrobials and antimicrobial resistance genes in beef cattle storage ponds and swine treatment lagoons. Sci. Total Environ. 463-464, 631–638. doi:10.1016/j.scitotenv.2013.06.016
Zhou, S., Xiong, C., Su, Y., Wang, Y., Gao, Y., Tang, Z., et al. (2022). Antibiotic-resistant bacteria and antibiotic resistance genes in uranium mine: Distribution and influencing factors. Environ. Pollut. 304, 119158. doi:10.1016/j.envpol.2022.119158
Zhu, Y.-G., Johnson, T. A., Su, J.-Q., Qiao, M., Guo, G.-X., Stedtfeld, R. D., et al. (2013). Diverse and abundant antibiotic resistance genes in Chinese swine farms. Proc. Natl. Acad. Sci. U. S. A. 110, 3435–3440. doi:10.1073/pnas.1222743110
Keywords: swine manure, ARGs, uranium, antimicrobials, metals, co-selection
Citation: Congilosi JL, Wallace JS, Neher TP, Howe A, Soupir ML and Aga DS (2022) Co-occurrence of antimicrobials and metals as potential drivers of antimicrobial resistance in swine farms. Front. Environ. Sci. 10:1018739. doi: 10.3389/fenvs.2022.1018739
Received: 13 August 2022; Accepted: 01 November 2022;
Published: 01 December 2022.
Edited by:
Hua-Jun Huang, Jiangxi Agricultural University, ChinaReviewed by:
Mianzhi Wang, Yangzhou University, ChinaCopyright © 2022 Congilosi, Wallace, Neher, Howe, Soupir and Aga. This is an open-access article distributed under the terms of the Creative Commons Attribution License (CC BY). The use, distribution or reproduction in other forums is permitted, provided the original author(s) and the copyright owner(s) are credited and that the original publication in this journal is cited, in accordance with accepted academic practice. No use, distribution or reproduction is permitted which does not comply with these terms.
*Correspondence: Diana S. Aga, ZGlhbmFhZ2FAYnVmZmFsby5lZHU=
†These authors share first authorship
Disclaimer: All claims expressed in this article are solely those of the authors and do not necessarily represent those of their affiliated organizations, or those of the publisher, the editors and the reviewers. Any product that may be evaluated in this article or claim that may be made by its manufacturer is not guaranteed or endorsed by the publisher.
Research integrity at Frontiers
Learn more about the work of our research integrity team to safeguard the quality of each article we publish.