- 1College of the Environment, Zhejiang University of Technology, Hangzhou, China
- 2College of Petrochemical Engineering and Environment, Zhejiang Ocean University, Zhoushan, China
Biofiltration of hydrophobic and recalcitrant volatile organic compounds faces challenges, and the bioaerosols sourced from the biofiltration might cause secondary pollution. In this study, the combination of ultraviolet photodegradation and biotrickling filtration (UV-BTF) was designed to treat gaseous cyclohexane, and UV post-treatment (post-UV) was further utilized for the bioaerosol emissions management. Results showed that the combined UV-BTF permitted faster biofilm formation and had better removal efficiencies (REs) than the single biotrickling filter (BTF). The maximum elimination capacity (EC) of UV-BTF and single BTF was 4.4 and 1.32 g m−3 h−1, respectively. Carbon balance for the bioreactor showed that both BTF and UV-BTF could convert more than 50% of the initial cyclohexane into microbial biomass. High-throughput sequencing analysis showed that UV-BTF had a richer and more diverse microbial community compared with the single one. The post-UV had a good inactivation effect on the bioaerosols. Not solely the concentrations additionally the particle sizes of the bioaerosols from the post-UV became lower and smaller than those from the single BTF. Microbial analysis showed that UV had a greater impact on the species and quantity of both bacteria and fungi, but mainly on the number of bacteria. Such results suggested that UV could be used as the pretreatment for the enhancement of hydrophobic and recalcitrant VOCs removal in the subsequent biopurification, and also as the post-treatment for the inactivation of some harmful bioaerosols.
1 Introduction
Cyclohexane is commonly used as a solvent and production material in industry. Because of its highly volatility (saturated vapor pressure at 20°C is 12.7 kPa), it is easy to emit into the surroundings during industrial usage (Hwang and Sagadevan, 2014). It is a low-toxic organic substance but is mildly irritating to the skin, eyes, and respiratory tract (Campbell, 2011). At present, the common removal technologies for cyclohexane emission are mostly adsorption and photocatalysis (Andrade et al., 2020; Hao et al., 2021). With the characteristics of poor stability and high recycling cost (refer to adsorption), lower natural light utilization efficiency, and catalyst poisoning, these treatments have limited applications in industry.
The biopurification of VOCs has attracted an extensive attention due to its advantages of low cost, simple operation, green process, and harmless products (Estrada et al., 2011; Salamanca et al., 2021). Some literatures about the biological treatment of cyclohexane emissions have been reported. The first reported bacteria that had the biodegradability for cyclohexane was isolated by Tausz J (1919) in 1919, and more cyclohexane degrading bacteria have been reported recently. Salamanca and Engesser (2014) isolated two cyclohexane-degrading strains from the sludge sourced from the sewage treatment plant, studying their biodegradabilities and applying a BTF for the treatment of cyclohexane emission (Salamanca et al., 2017). BTF is widely used in the treatment of hydrophilic and biodegradable VOCs, but its removal for hydrophobic VOCs is poor, mainly due to the mass transfer problem caused by the low solubility of the gaseous compound. Several researchers used pretreatment to convert hydrophobic VOCs into easily soluble intermediates, which were easily mass transferred into liquid phase, and thus facilitated the subsequent biopurification operating normally (Wang et al., 2009b; Wei et al., 2010; Wu et al., 2018). UV photooxidation is a simple and common technology, and usually selected as the pretreatment before BTF for the conversion of hydrophobic and recalcitrant pollutants (Moussavi and Mohseni, 2007; Cheng et al., 2011b). Den et al. (2006) used ultraviolet light (UV) as the pretreatment to convert trichloroethylene and tetrachloroethylene into more water-soluble intermediates, and the combined system UV-BTF had better removal effects. Zhu et al. (2015) found that UV converted styrene into readily biodegradable compounds such as benzoate, phenol, and isopropanol, which were completely removed and mineralized in the subsequent biofilter. Although related bacteria with the ability to degrade cyclohexane have been reported, due to its highly hydrophobic and highly volatility, its removal in biofilter was poor. Therefore, it is a necessity for the utilization of the combined system UV-BTF treating cyclohexane emission.
BTF relies on the microbial activity to degrade gaseous pollutants, and the microorganisms on the packing materials are also prone to form microbial aerosols with the exhaust discharging from BTF (Ghosh et al., 2015; Pearson et al., 2015). Bioaerosols pose potential hazards and risks to human health due to the presence of some inhalable pathogenic microorganisms. Waste gas biological treatment facilities are not only the “sink” of microbial aerosols but also the “source” of microbial aerosols. The control and health risk assessment of microbial aerosols produced by bioreactors has attracted more and more attentions from researchers. Sun et al. (2018) studied the microbial aerosol characteristics in tail gas emissions of sulfur dioxide and ortho-xylene treated by biofilters. Results showed that the average concentration of bioaerosol released by biofiltration was 318 CFU m−3, and the bioaerosol contained some potential pathogenic bacteria. Hu et al. (2020) studied the effect of biofiltration on bioaerosol emissions, and the results showed that factors such as biomass, air intake velocity, and water content had significant effects on bioaerosol emissions. In recent years, various technologies, such as heat treatment, ultraviolet sterilization, microwave radiation, etc. have been developed to control the microbial aerosols, among which UV is the most commonly used inactivation method (Kujundzic et al., 2007; Wang et al., 2019b). Wang et al. (2019b) studied the relationship between UV irradiation dose and microbial inactivation efficiency, and in-depth analyzed the endotoxin changes during the bioaerosols inactivation process. Wang et al. (2009a) found that ozone produced by UV pretreatment, had an effective technique for reducing bioaerosol emissions from biofilters. However, most biofilter studies focused only on bioaerosol concentration, and rarely assessed the bioaerosol hazards from particle size and microbial community, especially the effects of inactivation technologies (e.g., UV) on various microbial populations belonging to the biological aerosols. Therefore, it is necessary to carry out the researches about microbial analysis. Such results will provide useful information for the accurate control of the concentration and harm of bioaerosol in the exhaust of bioreactor.
Previous studies showed that UV-BTF had an attenuating effect on bioaerosol concentrations in biofilters, and further consideration would be given to design a separate UV placed behind the combined device to enhance its inactivation effects. In this study, the performance of a UV-BTF to treat gaseous cyclohexane and the inactivation impact of UV on bioaerosol were evaluated. The effects of initial cyclohexane concentration, empty bed residence time (EBRTs), and inlet loading were investigated by the comparison with the single BTF. Investigation of carbon distribution in both systems provided valuable information on their own performance in cyclohexane removal and carbon conversion. High-throughput sequencing assessed the differences between microbial communities in the BTFs. In addition, data on bioaerosol concentration, particle size, and microbial diversity at the three sampling sites was evaluated to discuss the bioaerosol control effect by UV. Some relevant results will further expand the application of the coupling technology in industrial waste gas treatment and bioaerosol control.
2 Materials and methods
2.1 Experimental setup
The mixed flora used in this experiment was acclimated from the sludge of a sewage treatment plant in Hangzhou. Polyurethane foam (PUF) was selected as the packings, with the characteristics of good mechanical strength, high porosity, no toxic, and low cost. The required culture medium is shown in Supplementary Table S1.
BTF was constructed of two cylindrical glass sections with an internal diameter of 8.0 cm and an effective length of 80.0 cm. Figure 1 is the schematic diagram of BTF used in this study, which was mainly composed of four parts: a gas circuit, a recirculating nutrient solution, a UV irradiation equipment, and a filter. A certain proportion of cyclohexane and air was passed through the gas mixing tank into single BTF and combined UV-BTF systems, the gas velocity was controlled by a rotameter to adjust the proper EBRTs. The mineral salt nutrient solution was pumped and sprayed from the top of BTF through a circulating pump, forming a countercurrent with the exhaust gas. Both BTFs were equipped with two sampling ports including the inlet and outlet, each of which for the measurement of VOCs and CO2 concentrations. Besides, the biofilters had openings for microbial samples used for the analysis of microbial diversity.
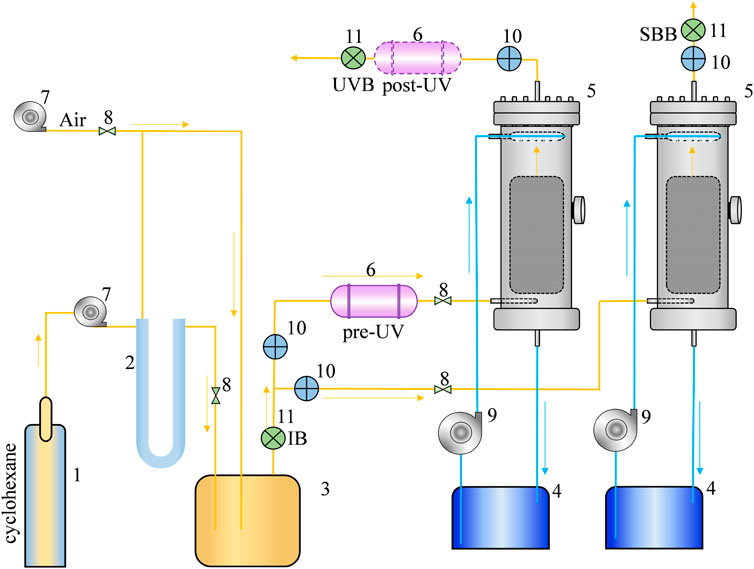
FIGURE 1. Schematic diagram of the bio-trickling filter (1. cyclohexane reagent; 2. U-tube; 3. air mixed tank; 4. nutrient tank; 5. BTF; 6. UV photoreactor; 7. air pump; 8. rotameter; 9. circulating pump; 10. sampling port; 11. bioaerosols sampling site).
2.2 Reactor operation and analysis methods
16 L PU packing (8 L per tower on average) in mineral salt nutrient solution (sludge volume: nutrient solution volume = 1:3, in which nutrient solution was 10 L) was left to stand for 48 h and then the packing was put into BTF. Discharged 2 L of nutrient solution every day, and supplemented the same amount of nutrient solution. The pH of the nutrient solution was maintained at 7.0, and the temperature of the nutrient solution was maintained at 30°C.
The pre-experiment determined the inlet concentration to be 50, 100, and 200 mg m−3, the EBRT of BTF was determined to be 100, 168, and 200 s, and the UV residence time was determined to be 10 s. The biofilter operation was divided into four periods: a start-up and three steady-state operation periods with different EBRTs. The combined UV-BTF and the single BTF systems operated for about 80 days under the same conditions (Supplementary Table S2; Supplementary Table S3). The control conditions in the start-up period were as follows: the inlet concentration was 100 mg m−3, the nutrient solution spraying volume was 30 L h−1, and the gas flow rate was 0.25 m3 h−1. In the steady-state operation periods, under the conditions of different inlet concentrations and residence times illustrated before, the effect of process parameters on the pollutant removal by the single BTF and combined UV-BTF were investigated. REs in the start-up and steady-state operation periods, as well as the elimination capacity (EC), and CO2 production of the single BTF and combined system were compared to assess their removal characteristics. The intermediates of UV photolysis in the combined UV-BTF system were analyzed. Further, the carbon distribution of the two systems was analyzed from the perspective of carbon balance, which was mainly analyzed into four parts: carbon in cyclohexane, carbon in CO2, carbon converted into cellular biomass, and carbon dissolved in the nutrient solution.
The concentration of cyclohexane in the gas phase was detected by a Fury 9790 II gas chromatograph equipped with a flame ionization detector (FID). Carbon dioxide concentration was detected by a Fore 9790 II gas chromatograph equipped with a Thermal Conductivity Detector (TCD). Total organic carbon (TOC) was measured using Thermo Fisher Vario TOC. The intermediates were analyzed by Agilent 7890A GC/MS. The ozone concentrations of the system were detected by the Shenzhen Pulitong Ozone detector. The above analysis methods and equipment are detailed in Supplementary Table S4.
In the present study, the volume change due to chemical reactions was negligible; thus, the cyclohexane RE was defined as follows:
EC (gm−3 h−1) was defined as the cyclohexane mass removed per unit volume of medium per unit time.
Mineralization efficiency (%) was defined as the proportion of carbon in the effluent CO2 to the carbon mass in the entry cyclohexane.
In these above formulas, Cin and Cout are the initial and final cyclohexane concentrations (mg m−3), respectively. Q is the gas flow rate (m3 h−1), and V is the flow rate (L min−1).
2.3 Bioaerosol sampling and analysis methods
In order to study the effect of biofilter operating conditions and post-UV on combined UV-BTF tail gas bioaerosol, three bioaerosol sampling points were set up (Figure 1) and named as: Inlet Bioaerosols (IB), the single BTF Bioaerosols (SBB), and post-UV Bioaerosols (UVB). The sampling time was 10 min, and the sampling flow rate was 28.3 L min−1. The sampling instrument was Anderson six-stage microbial sampler from Changzhou YiTong Analytical Instrument Manufacturing Co., Ltd. Bioaerosols were divided into six stages according to the size distribution, 0.65–1.1 µm, 1.1–2.1 µm, 2.1–3.3 µm, 3.3–4.7 µm, 4.7–7.0 µm, and >7.0 µm (Andersen, 1958). The above-mentioned particulate matter represents the deposition sites in the respiratory system. Collected bacterial samples were incubated at 30°C for 48 h, and fungal samples were incubated at 37°C for 120 h.
Colony count concentrations were corrected by the Positive-hole correction method. The calculation formula is as follows:
N is the total number of colonies on the Petri dish, CFU; Q is the flow rate of the sampling pump, 28.3 L min−1; T is the sampling time, 10 min.
Bioaerosol particle size distribution which is expressed as the percentage of colonies at all levels to the total colonies, the calculation formula is as follows:
The number of microbial particles at each level (%) = the number of colonies at this level/the total number of colonies × 100%.
The count median diameter (CMD) refers to the particle size of bioaerosol particles when the number of particles distributed above and below the particle size accounts for 50% of a certain value. Calculate according to the following formula (Finlay and Darquenne, 2020):
2.4 Microbial diversity analysis
Microbial and aerosol samples for UV-BTF and the single BTF systems were analyzed. Select the genus whose relative abundance accounts for more than 2% of all samples, retain the relative abundance, and analyze the combined UV-BTF system respectively relative abundance of species.
Microbial DNA was extracted using the E.Z.N.A. Soil DNA Kit (Omega Bio-tek, Norcross, GA, United States) according to manufacturer s protocols. The V3-V4 region of the bacteria 16S ribosomal RNA gene were amplified by PCR (95°C for 2 min, followed by 25 cycles at 95°C for 30 s, 55°C for 30 s, and 72°C for 30 s and a final extension at 72°C for 5 min) using primers 341F 5-barcode-CCTAYGGGRBGCASCAG-3′ and 806R 5′-GGACTACNNGGGTATCTAAT-3′, where barcode was an eight-base sequence unique to each sample. PCR reactions were performed in triplicate 20 μl mixture containing 4 μl of 5 × FastPfu Buffer, 2 μl of 2.5 mM dNTPs, 0.8 μl of each primer (5 μM), 0.4 μl of FastPfu Polymerase, and 10 ng of template DNA. Amplicons were extracted from 2% agarose gels and purified using the AxyPrepDNA Gel Extraction Kit (Axygen Biosciences, Union City, CA, United States) according to the manufacturer’s instructions and quantified using QuantiFluor™ -ST (Promega, United States). Purified amplicons were pooled in equimolar and paired-end sequenced (2,250 bp) on an Illumina MiSeq at Mingke Biotechnology (Hangzhou) Co., Ltd., Zhejiang province, China.
Reads obtained by Illumina Miseq sequencing were first spliced according to overlap, and sequence quality was controlled and filtered at the same time. After distinguishing the samples, OTU clustering analysis and species taxonomy analysis were performed. Based on the results of OTU clustering analysis, multiple diversity indices could be analyzed for OTUs. Based on taxonomic information, statistical analysis of community structure could be performed at various taxonomic levels.
2.5 Statistical analysis
Data analysis and graphing were performed using Excel 2016, Origin 2021, and R studio software.
3 Results and discussion
3.1 Reactor operating characteristics
For the single BTF (Figure 2A), at the startup period, when the concentration of cyclohexane was constant at 100 mg m−3, the REs rose slowly in the first 20 days, only 25.6%, which was considered to be the adaptation of microorganisms to cyclohexane, and the formation of biofilm was not yet complete; during 20–30 days, when the microorganisms grew in large numbers, REs increased and fluctuated with the changes in the activity of the microorganisms. After 30 days, the biofilm gradually stabilized, but REs were only about 40%. UV-BTF approach permitted faster biofilm formation than the single BTF (Figure 2B), and had a greater improvement in the overall RE. At the same inlet concentration, the REs had increased to 53% on 12 days, UV photodegradation produced less toxic biodegradable intermediates, which reduced the inlet cyclohexane loading and enabled rapid biofilm formation. From 12 to 32 days, a large number of microorganisms accumulated, and REs further increased to more than 95%. Moussavi and Mohseni (2007) indicated that UV pretreatment as a buffer could reduce the transient effects and durations of higher toluene and o-xylene loading, thereby improving the overall performance of BTF. Zhu et al. (2015) found that the pretreatment UV converted styrene into some easily biodegradable intermediates (benzoate, phenol, isopropyl alcohol, etc.), making styrene inlet load into the biofilter lower. As a result, the rapid formation of combined UV-BTF biofilms could be achieved.
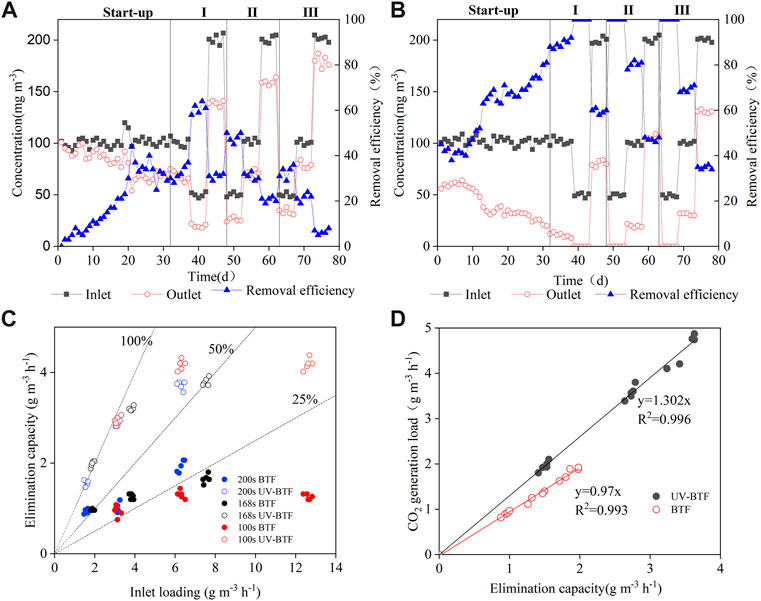
FIGURE 2. Overall performance of the single BTF (A) and the combined UV-BTF (B) during the start-up and stable operational periods. Performances (C) and CO2 productions (D) at different inlet loads and EBRTs during the whole operation.
From Figure 2C, when the EBRTs were 200 s and the inlet concentration was increased from 50 to 200 mg m−3, the REs of the single BTF decreased from 60% to 30%, and the maximum EC was 2.1 g m−3 h−1. For UV-BTF system, the RE decreased from 100% to 60%, and the maximum EC was 4.4 g m−3 h−1. When the EBRTs were 100 s and the inlet load increased to 12 g m−3 h−1, the maximum EC of the single BTF was 1.32 g m−3 h−1, while the maximum EC of combined UV-BTF was 4.4 g m−3 h−1. UV-BTF had a higher EC, and when the inlet load increased, the EC was relatively stable, showing better resistance to shock loading. According to the preliminary experiments about UV photodegradation of cyclohexane (Supplementary Figure S1), some intermediates with better water solubility and weaker biological toxicity were detected, such as n-butanol, cyclohexanone, and 2-methylcyclopentanone. The production of these intermediates not only enabled the continuous growth of biofilms by virtue of more abundant carbon sources, but also worked as the solvent enhancing the mass transfer of cyclohexane from gas to liquid. Previous studies (Rene et al., 2009) showed that the presence of hydrophilic compounds (alcohols, aldehydes, and ketones) in BTF enhanced the absorption and removal of hydrophobic VOCs. The destruction of photodegradable intermediates in BTF was caused by a combination of hydrolysis, adsorption and microbial degradation. On the one hand, the production of these intermediates improved the mass transfer effect (Cheng et al., 2020) and reduced the inlet load of cyclohexane; on the other hand, a variety of biodegradable compounds enriched the carbon source required by microorganisms and promoted the occurrence of the whole process of microbial degradation (Den et al., 2006). Therefore, the combined UV-BTF system had higher REs and EC than the single BTF. With the shorter EBRTs, the combined UV-BTF and the single BTF showed varying degrees of reduction in REs, and the reduction in removal capacity might be due to the metabolic sluggishness caused by insufficient contact time of pollutants with biofilms (Kennes et al., 2009). Salamanca et al. (2017) used a biofilter packed with PUF to treat cyclohexane, with REs and EC of 80%–99% and 5.4–38 g m−3 h−1, respectively. Compared with previous studies on the treatment of hydrophobic VOCs by BTF, the removal performance of present study was similar to that reported by Wu et al. (2016), the performance of which was Res of 40%–100% and EC < 8.39 g m−3 h−1 under EBRT of 200–400 s. Although the performance was slightly lower than that of some common VOCs, but much higher than that of chlorinated VOCs such as trichloroethylene (Quan et al., 2017).
It is ideal to convert VOCs into harmless product CO2, so the production of CO2 was further used to evaluate the performance of BTF. In theory, the linear relationship between the generation of CO2 and the removal of cyclohexane was y = 3.143x. In the present study, the linear relationships of the single and combined BTF were y = 0.97x and y = 1.302x, respectively, indicating that the mineralization efficiencies were 30.9% and 41.4%. The mineralization efficiency of both were low, and there was a certain gap between the theoretical and actual value. Nonetheless, as EC increased, the CO2 production efficiency was quite constant. It can be seen from Figure 2D that the mineralization efficiency of combined UV-BTF system was higher than the single BTF. The reason for this result might be that UV pretreatment could convert cyclohexane to some compounds, which the microorganisms could easily use them for their growths (Yu et al., 2014). In order to further clarify the fate of carbon in the reactor, an analysis of carbon balance was carried out in Section 3.2.
3.2 Carbon balance analysis for rector
Under the function of microorganisms inhabiting in single and combined BTF, the carbon contained by the initial cyclohexane was mainly converted into three parts: carbon contained by CO2 (remarked as C-CO2); carbon contained by residual cyclohexane in the outlet gas phase (C-C6H12); carbon contained by the growing microbial biomass (C-biomass); and total carbon dissolved in the circulating solution (TOC) (Li et al., 2019). When the residence time was 200 s, the mineralization efficiencies of the single BTF and UV-BTF systems in the present study were low. Therefore, the investigation of the fate of carbon other than CO2 was particularly important. Carbon distribution in different biofilters is shown in Table 1 below.
In the gas phase, the residual cyclohexane in the single BTF and UV-BTF was 7.6% and 5.1% (Supplementary Figure S2), respectively, indicating that the UV-BTF system produced less second pollution to the environment. Although UV pretreatment generated more hydrophilic intermediates, less TOC and total inorganic carbon (TIC) contents of the liquid phase were detected in the case of UV-BTF. These intermediates were more hydrophilic and easier to be degraded by microorganisms without flowing out with the nutrient solution. They constituted a variety of carbon sources for the microorganisms’ growths in the UV-BTF system, reflecting a better overall removal performance. The carbon used for microbial biomass growth accounted for 51.3% and 58.9%, respectively. The biomass produced by UV-BTF was slightly lower than the single BTF, which was attributed to the effect of UV-generated ozone on microorganisms, and the ozone concentration in the present study is shown in Supplementary Figure S3. Yeung et al. (2021) showed that low doses of ozone can significantly improve the overall performance of biofilters by forming a stronger microbiome and improving clogging problems. Therefore, the amount of biomass alone was not enough to describe the role of microorganisms in the system, and information on the structure of the microbial community was also needed. In addition, the deviation between the inlet and outlet of carbon flow might be the soluble microbial products in the circulating solution, extracellular polymeric substances generated by bacteria, and so on (Jiang et al., 2010).
In the context of “dual carbon,” the efficient management of VOCs must take into account the reduction of carbon emissions. Previous studies on the removal of VOCs by UV-BTF had high mineralization efficiency. Jiang et al. (2016) showed that the mineralization efficiency of the single BTF and UV-BTF systems for chlorobenzene removal were 87.6% and 92.7%, respectively. Cheng et al. (2011a) found that the mineralization efficiency of α-pinene removal were 61.4% and 84.6%, respectively. Most studies about the biofiltration of VOCs showed that the proportions of C-CO2 by the bio-reactor was nearly >60% (Bester et al., 2011). Although CO2 might the proper endpoints in the past years, it was not popular endpoints in recent years. That meant it should minimize the production of CO2 while removing pollutants (Bordoloi and Gostomski, 2019). In the present study, the mineralization efficiency of the two BTF systems was low, more than 50% of carbon was converted into nutrients required for the growth and reproduction of microorganisms through assimilation, which had a certain carbon sequestration and emission reduction potential. Compared with the photocatalytic and thermocatalytic treatment of cyclohexane, the biological method had milder conditions and lower operating costs. The application of the biocatalytic route in recent years has improved the problems of high mineralization efficiency and poor reaction selectivity in biological methods. Salamanca et al. (2021) made an Acidovorax sp. mutant whole-cell biocatalyst by deleting the downstream enzymes of the bacteria, and 98% of cyclohexane was converted into omega hydroxycarboxylic acids with industrial value. The use of enzymes or whole cells to catalyze the conversion of cyclohexane and cyclohexanol to caprolactone has been extensively reported (Mallin et al., 2013; Staudt et al., 2013). Biological methods have potential in carbon emission reduction in the process of VOCs treatment. Reduce microbial respiration in different stages to increase the assimilated carbon sequestration process or selectively convert pollutants into valuable products; effectively utilize the chemical energy generated in the process of microbial degradation of VOCs, all of which will be important directions for biological removal of VOCs under the context of “dual carbon.”
3.3 The effect of UV post-treatment on bioaerosols in the tail gas
3.3.1 Bioaerosol emission concentration
The gas velocity determines the shear force between the biofilm and the gas flow, thereby affecting the emission of bioaerosols. From Figure 3A, the increase in gas velocity leads to an increase in the concentration of bioaerosols. At a gas velocity of 0.25 m3 h−1 (residence time is 200 s), the total bioaerosol concentration of the SBB and UVB was 578 CFU m−3 and 84 CFU m−3, which suggested that the post-UV had excellent inactivation effect on bioaerosol. When the gas velocity increased to 0.3 m3 h−1, the bioaerosol concentration of both increased, and the inactivation effect by UV decreased. The wavelength of the ultraviolet lamp used in this study was 185 nm, and its radiation dose was 8 J m−2 (10 s). The UV wavelength and exposure time in the study were similar to those of previous studies, but the lower UV intensity and radiation dose resulted in poor removal of aerosol microorganisms. Wang et al. (2019a) found that with the same UV exposure time (0–25 s), the E. coli inactivation efficiencies by different wavelengths were 185 nm > 254 nm > 365 nm, and they further proposed that 254 and 365 nm UV could act on microbial DNA fragments to cause their inactivation, but this damage could be repaired by itself; 185 nm UV inactivation was attributed to the reactive oxygen species by the photolysis. The study by King et al. (2011) showed that under a certain humidity, for 90% inactivation of the three bacteria B.atrophaeus, P. agglomerans and Y. ruckeri in the atmosphere, the required UV irradiation doses were 70.3, 73.3, and 18.3 J m−2, respectively. Studies have shown that UV inactivation of bioaerosols was related to the irradiation intensity and irradiation time of UV lamps (Ko et al., 2000). Therefore, it is necessary to increase the UV intensity to determine the appropriate irradiation dose. Ko et al. (2000) found that, unlike bioaerosols emitted in solids, bioaerosols produced in liquid phase were usually covered with a water film that resisted the inactivation of UV light. In the present study, the bioaerosol of the biofilter tail gas had a certain humidity, which might weaken the subsequent UV inactivation to a certain extent.
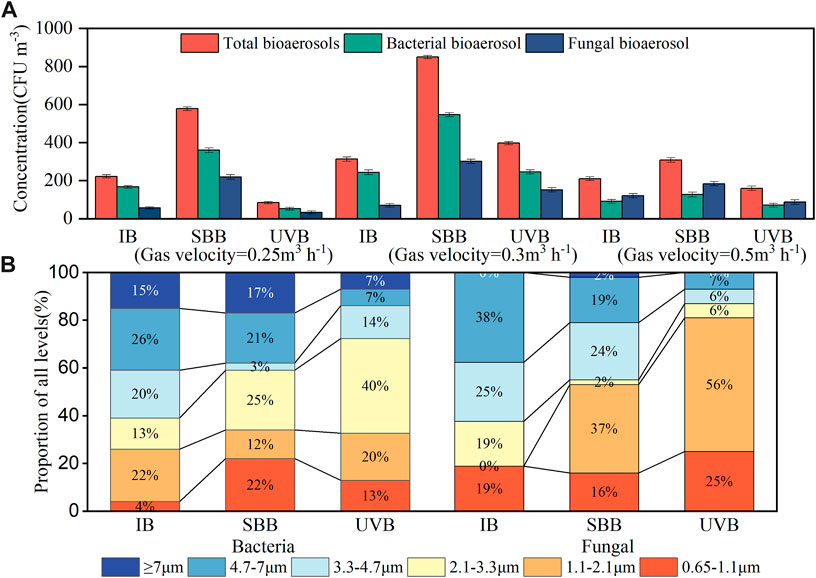
FIGURE 3. (A) Bioaerosol concentration at different gas velocities, (B) Particle size distribution of bacteria and fungi.
Figure 3A shows the mean concentrations of bacteria and fungi at different gas velocities detected at individual sampling sites. With the increasing in gas velocity, the bioaerosol concentrations of three sampling sites increased. When the gas velocity was 0.25 m3 h−1, the bioaerosol concentrations of bacterial and fungal in SBB site were 360 CFU m−3 and 219 CFU m−3. As the gas velocities increased gradually to 0.3 m3 h−1, the bacterial and fungal concentrations in SBB site increased to 547 CFU m−3 and 301 CFU m−3, respectively. Fungal and bacterial concentrations in UVB site also increased, from 53 CFU m−3 and 33 CFU m−3 to 246 CFU m−3 and 152 CFU m−3, respectively, UV still played an important role in inactivation. Airflow disturbance would have played an important role in the spread and transport of microorganisms in BTF-packed biofilms. Relevant studies (Lighthart and Shaffer, 1997) showed that wind speed was positively correlated with the concentration of microorganisms in the air, and the increase in wind speed caused the disturbances between the gas and liquid to spread the microorganisms in the liquid into the atmosphere (Montero et al., 2016). Wang et al. (2019b) found that, in sewage treatment plants, the increase in aeration rate increased the number and diversity of microorganisms. Higher gas velocity created strong shear forces on BTF biofilms, making it easy for microorganisms to spread into the gas phase. At a gas velocity of 0.50 m3 h−1, the bioaerosol concentrations of the three sampling sites were lower than those at the previous two gas velocities, and the proportion of fungi, unlike before, was greater than that of bacteria. When the gas velocity increased to a certain extent, although the bioaerosol was more likely to be brought out, the volume of the mixed gas also increased, so the dilution effect was dominant. Hu et al. (2020) showed that when the gas was increased to a certain flow rate, the bioaerosol emission concentration decreased due to dilution. In addition, the fungi were more hydrophobic and spore-producing, and thus accounted for a larger proportion of bioaerosols than bacteria (Spigno et al., 2003).
According to China’s “Indoor Air Quality Standards” (GB/T 18883-2002), the bioaerosol concentrations of the three sampling sites at each airspeed belonged to the “clean level” (the total bioaerosols <3,000 CFU m−3, bacteria <1,000 CFU m−3, fungi <500 CFU m−3). Hu et al. (2020) showed that the concentration of bioaerosols emitted from bioreactors ranges from 103 to 106 CFU m−3. Zhang et al. (2017) detected that the average annual bacterial concentration of airborne bioaerosols in the Beijing-Tianjin-Hebei region was 1,380–3020 CFU m−3 and fungi were 89–2270 CFU m−3. It can be seen that the concentration of bioaerosol discharged in this study was low, and at an approximate gas velocity, it was close to the concentration of the biochemical reaction tank for wastewater treatment reported by Wang et al. (2019b).
3.3.2 Particle size distribution
The particle size distributions of bacteria and fungi in aerosols from the three sampling sites is shown in Figure 3B. UV radiation resulted in a greater overall reduction in particles >4.7 μm than particles <4.7 μm. After UV control, the proportion of 1.1–4.7 μm bioaerosol was relatively high. Combined with the previous studies (Li et al., 2021b), the bioaerosols with larger particle size were easily settled back into BTF systems after inactivation, while bioaerosols in particles <1.1 μm were more easily inactivated by UV. Studies showed that fine particles with a particle size <4.7 μm were more likely to deposit in the respiratory tract and alveoli, especially microbial aerosols that carried pathogenic bacteria and pollutants, which were more harmful to the human body.
Near 41% (IB) and 38% (SBB) bacteria were attached to particles larger than 4.7 μm; as for UVB, bacteria aerosols smaller than 4.7 μm accounted for nearly 86%, in which 2.1–3.3 μm dominated. Ferguson and Dumbrell (2017) reported that live bacterial bioaerosols existed in the form of single cells and cell aggregates, in which single cells attached to small particles (<3.3 μm), and cell aggregates attached to larger particles (>3.3 μm). In this study, due to the natural sedimentation of particles larger than 4.7 μm after inactivation, the proportion of bioaerosols of 3.3–4.7 μm in the outlet from UVB increased, and the single-cell bacterial aerosols of <3.3 μm were dominated in general. Li et al. (2021b) found that the overall reduction in coarse particles was greater than fine particles sourced from a rural wastewater treatment station.
Compared with bacteria, fungal aerosols accounted for more small and medium particle sizes, and there were almost no particles larger than 7 μm. The proportion of each site in the 4.7–7 μm particle size was IB > SBB > UVB; among the particle size <4.7 μm, the proportion of 1.1–2.1 μm particles increased, 0%, 37%, and 56% for IB, SBB and UVB, respectively. Other particle size distribution remained roughly unchanged at the three sampling sites. Fungal aerosols >4.7 μm accounted for 38% in IB site, which was close to the proportion of fungal aerosols by Li et al. (2021a). After post-UV inactivation, although the aerosol concentrations of fungi were reduced, their particle sizes shifted to smaller ones, which was probably mainly consisted of unicellular fungi or spores.
It can be seen from Supplementary Figure S4 that the difference CMD of bacteria and fungi at each sampling site was basically small, but the overall situation is IB > SBB > UVB, which was consistent with the results discussed above. The CMD of bacterial aerosols was larger than that of fungal aerosols. Such reasons might be that bacteria were mainly adsorbed on the surface of dust particles, while fungi existed in the air in the form of single spores.
3.4 Microbial diversity analysis
3.4.1 Comparison of microbial communities developed in biofilters
To investigate the effect of UV pretreatment on the microbial structure, the analysis of community diversity was performed on microbial inoculum (INO) and two BTFs before film hanging. The diversity index of microbial community in combined UV-BTF and the single BTF is shown in Supplementary Table S6. Such the comparison found that combined UV-BTF had more OTUs and exhibited higher microbial richness. As a result, combined UV-BTF system had the higher diversity (Shannon = 3.42, Simpson = 0.1076) than the single BTF (Shannon = 2.97, Simpson = 0.1291).
As shown in Figure 4, the genera such as Alicycliphilus in INO gradually disappeared after its inoculation into BTF, and other genera with relative abundance lower than 2% got good growth after inoculation, which may be the result of natural selection of superiority and inferiority under long-term stress of contaminants. The most dominant microorganisms in both BTFs were Pseudomonas, Ferruginibacter, Hydrogenophaga, Bacillus, and Acinetobacter. Pseudomonas accounted for 34.5%, Hydrogenophaga accounted for 7.9%, and Acinetobacter accounted for 3.7% in the single BTF; while Pseudomonas accounted for 41.2%, Hydrogenophaga accounted for 13.6%, Bacillus accounted for 12.1%, and Acinetobacter accounted for 4.1% in combined UV-BTF system. The increased proportion of these bacteria in UV-BTF and the single BTF might be related to the biodegradation of cyclohexane and some related compounds. Pseudomonas has been shown to be capable of effectively removing VOCs, and reported to convert cyclohexane to epsilon-caprolactone or 6-hydroxycaproic acid (Bretschneider et al., 2022). Acinetobacter was found to be the degrading bacteria of cyclohexanol and cyclohexanone (Cheng et al., 2000). The UV photodegradation product n-butanol was also reported to be degraded by Bacillus (Kuisiene et al., 2008). Figure 4 also shows that the species diversity of microorganisms was shown as combined UV-BTF> the single BTF > INO. Because a variety of intermediates in combined UV-BTF could be carbon sources for microorganisms, a diverse microbial community formed. Compared with the single BTF, the stronger microbial flora in the combined UV-BTF improved the removal of cyclohexane and maintained the stability of the entire reaction system.
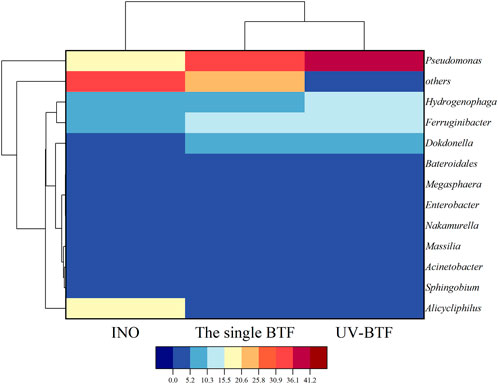
FIGURE 4. Hierarchical cluster analysis of INO, the single BTF and combined UV-BTF bacterial communities.
3.4.2 Microbial communities in bioaerosols in biotrickling filter exhaust
The bacterial and fungal aerosol diversity index analysis is shown in Table 2. Compared to the sample IB, the Chao index of microorganisms in the sample SBB increased. Higher Shannon and lower Simpson suggested that microbial diversity were much richer in bioaerosols. The Chao indices for sample UVB was roughly the same as the ones for sample IB, indicating that UV inactivation was efficient. Although as previously mentioned, the UV-BTF system had a higher microbial richness and diversity, the abundance of bacteria and fungi in aerosols reached the inlet level. As for bacteria, the Shannon index and Simpson index of SBB and UVB were not much different from IB, and sample SBB was higher. Under UV inactivation, the bacterial aerosol diversity in the sample UVB was lower than that in sample IB. Compared to bacteria, the diversity and richness of fungi were low for three samples, indicated that the fungi accounted for less in the bioaerosol. Compared with sample IB, the diversity and richness increased to a certain extent for sample UVB, and the increase for sample SBB was more obvious. Such results suggested that most fungi were more likely to enter the gas phase with the airflow to became microbial aerosols (Ferdowsi et al., 2017). Since both BTFs were dominated by bacteria, in general, the abundance and diversity of bacteria in aerosols were greater than those of fungi, which was consistent with the previous conclusion about the proportion of bacterial and fungal aerosol concentrations (Ibanga et al., 2018). After the fungi were inactivated by UV, the diversity was slightly higher than that of sample IB but significantly lower than that of sample SBB.
The community composition is shown in Figure 5. The genera with a higher average relative abundance of bacterial aerosols in sample IB were Acinetobacter (28.5%), Enterobacter (10%), Kocuria (9.8%), Massilia (8.4%), and Bacillus (2.8%). Acinetobacter and Enterobacter were reported to be isolated from wastewater treatment plant aerosols (Uhrbrand et al., 2017; Wang et al., 2019b). Bacillus sp. was reported to be the most predominant genus of bacterial bioaerosols generated from the waste treatment facility and its vicinity (Pahari et al., 2016).For sample SBB, the bacteria genera with the highest relative abundance were replaced by Pseudomonas (19.2%), and the relative abundances of Microbacterium (17.5%), Acinetobacter (14%), Lysinibacillus (4.4%), and Roseomonas (3.4%) were also higher. Pseudomonas is common in sewage treatment plants and it can biodegrade many organics (Wang et al., 2018), which was the dominant bacteria in the bacterial aerosols emitting from the BTF in the present study. Compared with the sample IB, after the community succession process under the interference of pollutants, the degrading bacteria related to cyclohexane gradually became the dominant flora. The bacteria in the aerosol originated from the reactor, and it could be seen that the same genera with higher abundance were Acinetobacter, Dokdonella, Enterobacter, and Pseudomonas. These bacterial aerosol particles carried some microorganisms that posed a potential threat to human health. Studies have shown that Pseudomonas widely existed in soil and air, with many species, and contained opportunistic pathogens closely related to human health (Uhrbrand et al., 2017). Acinetobacter is a gram-negative bacteria and is an opportunistic pathogen. After inhalation through the oropharynx, it may cause endogenous infection, resulting in respiratory tract inflammation such as bronchitis and bronchitis (Fracchia et al., 2006). After UV inactivation, the microbial diversity and bioaerosol concentration decreased, and the genera with higher relative abundance changed to Pseudomonas (35.9%), Bacillus (21.1%), Acinetobacter (14.2%), and Microbacterium (6.9%). It can be seen that UV has a good inactivation effect on some bacteria such as Microbacterium, the inactivation of the dominant degrading bacteria (Pseudomonas, Acinetobacter) and bacteria with a special tolerance to UV (Bacillus) in the system is not complete. The renewal and shedding of biofilms stabilize the cyclohexane-related degrading bacteria in both systems, so these bacteria inevitably appeared in the bioaerosol; the UV intensity mentioned before may make them easier to detect at UVB. Bacillus was difficult to inactivate, which was reported to have UV protecting spore coats and DNA repair machinery in the endospores (Setlow, 2006).
Compared with bacteria, there were fewer fungal species in the aerosols, mainly Aspergillus which reached 100% relative abundance in sample IB. Aspergillus species are pathogens that cause respiratory or lung infections (Breza-Boruta, 2012). The Shannon index in sample SBB was 2.49 times higher than that in sample IB, and the genera with higher relative abundance were Aspergillus (99.8%), Irpex (22.7%), Trametes (19.4%), and Penicillium (4%). Penicillium can cause penicillin disease, and can enter the body through the respiratory tract to cause lung infection or invade blood vessels, causing tissue necrosis, which seriously threatens the health and safety of humans and animals (Cruz-Perez et al., 2001). UV wiped out Irpex and Trametes, but there were some remnants of Aspergillus and Penicillium. The inactivation effect of post-UV light on fungi was not as good as that of bacteria. The reason might be that the surface of fungi was less hydrophobic, so it was easier to form a water film and reduce UV damage to it (Li et al., 2021c). In conclusion, post-UV had a greater impact on the species and quantity of both bacteria and fungi, but mainly on the quantity of bacteria.
4 Conclusion
This paper focused on the comparison of gaseous cyclohexane treatment by the combined UV-BTF and single BTF, alone with the evaluation of the inactivation effect on bioaerosols in the tail gas by UV. Results showed that combined UV-BTF had better REs and operational performance, which was attributed to UV photolysis to obtain more biodegradable intermediate products. These abundant carbon sources allowed the subsequent BTF to have a better microbial community. UV radiation had a certain inactivation effect on the bioaerosols sourcing from BTF, and the gas velocity had an impact on the concentration and particle distribution of bioaerosols. The particle size of the residual bioaerosol after inactivation was mainly <4.7 μm. After UV inactivation, the residual bacteria were mainly related to the cyclohexane’s degrader and special UV-tolerant bacteria, the fungi were mainly Penicillium and Aspergillus.
Data availability statement
The original contributions presented in the study are included in the article/Supplementary Material, further inquiries can be directed to the corresponding author.
Author contributions
Designed and performed the experiment: ZS, ZC, PL, and PZ. Analyzed the data and wrote the manuscript: ZS, ZC. Contributed reagents/materials/analysis tools and assisted guide: ZC, JC, JY , DC, PZ.
Conflict of interest
The authors declare that the research was conducted in the absence of any commercial or financial relationships that could be construed as a potential conflict of interest.
Publisher’s note
All claims expressed in this article are solely those of the authors and do not necessarily represent those of their affiliated organizations, or those of the publisher, the editors and the reviewers. Any product that may be evaluated in this article, or claim that may be made by its manufacturer, is not guaranteed or endorsed by the publisher.
Supplementary material
The Supplementary Material for this article can be found online at: https://www.frontiersin.org/articles/10.3389/fenvs.2022.1010980/full#supplementary-material
References
Andrade, M. A., Mestre, A. S., Carvalho, A. P., Pombeiro, A. J. L., and Martins, L. (2020). The role of nanoporous carbon materials in catalytic cyclohexane oxidation. Catal. Today 357, 46–55. doi:10.1016/j.cattod.2019.07.036
Andersen, A. A. (1958). New sampler for the collection, sizing, and enumeration of viable airborne particles. J Bacteriol 76 (5), 471–484.
Bester, E., Kroukamp, O., Hausner, M., Edwards, E. A., and Wolfaardt, G. M. (2011). Biofilm form and function: Carbon availability affects biofilm architecture, metabolic activity and planktonic cell yield. J. Appl. Microbiol. 110 (2), 387–398. doi:10.1111/j.1365-2672.2010.04894.x
Bordoloi, A., and Gostomski, P. A. (2019). Fate of degraded pollutants in waste gas biofiltration: An overview of carbon end-points. Biotechnol. Adv. 37 (4), 579–588. doi:10.1016/j.biotechadv.2018.09.002
Bretschneider, L., Heuschkel, I., Buhler, K., Karande, R., and Buhler, B. (2022). Rational orthologous pathway and biochemical process engineering for adipic acid production using Pseudomonas taiwanensis VLB120. Metab. Eng. 70, 206–217. doi:10.1016/j.ymben.2022.01.014
Breza-Boruta, B. (2012). Bioaerosols of the municipal waste landfill site as a source of microbiological air pollution and health hazard. Ecol. Chem. Eng. 19 (8), 12.
Campbell, M. L. (2011). “Cyclohexane,” in Ullmann's encyclopedia of industrial chemistry (New Jersey: Wiley VCH).
Cheng, Q., Thomas, S. M., Kostichka, K., Valentine, J. R., and Nagarajan, V. (2000). Genetic analysis of a gene cluster for cyclohexanol oxidation in Acinetobacter sp strain SE19 by in vitro transposition. J. Bacteriol. 182 (17), 4744–4751. doi:10.1128/jb.182.17.4744-4751.2000
Cheng, Y. T., Li, X., Liu, H. Y., Yang, C. P., Wu, S. H., Du, C., et al. (2020). Effect of presence of hydrophilic volatile organic compounds on removal of hydrophobic n-hexane in biotrickling filters. Chemosphere 252, 126490. doi:10.1016/j.chemosphere.2020.126490
Cheng, Z. W., Zhang, L. L., Chen, J. M., Yu, J. M., Gao, Z. L., and Jiang, Y. F. (2011a). Treatment of gaseous alpha-pinene by a combined system containing photo oxidation and aerobic biotrickling filtration. J. Hazard. Mater. 192 (3), 1650–1658. doi:10.1016/j.jhazmat.2011.06.092
Cheng, Z. W., Zhang, L. L., Xi, J. Y., Chen, J. M., Hu, H. Y., and Jiang, Y. F. (2011b). Recovery of biological removal of gaseous alpha-pinene in long-term vapor-phase bioreactors by UV photodegradation. Chem. Eng. J. 175, 316–323. doi:10.1016/j.cej.2011.09.113
Cruz-Perez, P., Buttner, M. P., and Stetzenbach, L. D. (2001). Detection and quantitation of Aspergillus fumigatus in pure culture using polymerase chain reaction. Mol. Cell. Probes 15 (2), 81–88. doi:10.1006/mcpr.2000.0343
Den, W., Ravindran, V., and Pirbazari, M. (2006). Photooxidation and biotrickling filtration for controlling industrial emissions of trichloroethylene and perchloroethylene. Chem. Eng. Sci. 61 (24), 7909–7923. doi:10.1016/j.ces.2006.09.015
Estrada, J. M., Kraakman, N., Munoz, R., and Lebrero, R. (2011). A comparative analysis of odour treatment technologies in wastewater treatment plants. Environ. Sci. Technol. 45 (3), 1100–1106. doi:10.1021/es103478j
Ferdowsi, M., Ramirez, A. A., Jones, J. P., and Heitz, M. (2017). Elimination of mass transfer and kinetic limited organic pollutants in biofilters: A review. Int. Biodeterior. Biodegrad. 119, 336–348. doi:10.1016/j.ibiod.2016.10.015
Ferguson, R. M. W., Neath, C. E., Dumbrell, A. J., Whitby, C., Colbeck, I., et al. (2017). Novel insights into the size distribution of bacterial bioaerosols at composting sites, in: The Aerosol Society Focus Meeting 10-Bioaerosols (University of Bristol, UK: The Aeorosl Society).
Finlay, W. H., and Darquenne, C. (2020). Particle size distributions. J. Aerosol Med. Pulm. Drug Deliv. 33 (4), 178–180. doi:10.1089/jamp.2020.29028.whf
Fracchia, L., Pietronave, S., Rinaldia, M., and Martinotti, M. G. (2006). Site-related airborne biological hazard and seasonal variations in two wastewater treatment plants. Water Res. 40 (10), 1985–1994. doi:10.1016/j.watres.2006.03.016
Ghosh, B., Lal, H., and Srivastava, A. (2015). Review of bioaerosols in indoor environment with special reference to sampling, analysis and control mechanisms. Environ. Int. 85, 254–272. doi:10.1016/j.envint.2015.09.018
Hao, F., Gao, Y. F., Liu, J. N., Dudek, R., Neal, L., Wang, S., et al. (2021). Zeolite-assisted core-shell redox catalysts for efficient light olefin production via cyclohexane redox oxidative cracking. Chem. Eng. J. 409, 128192. doi:10.1016/j.cej.2020.128192
Hu, X. R., Han, M. F., Wang, C., Yang, N. Y., Wang, Y. C., Duan, E. H., et al. (2020). A short review of bioaerosol emissions from gas bioreactors: Health threats, influencing factors and control technologies. Chemosphere 253, 126737. doi:10.1016/j.chemosphere.2020.126737
Hwang, K. C., and Sagadevan, A. (2014). One-pot room-temperature conversion of cyclohexane to adipic acid by ozone and UV light. Science 346 (6216), 1495–1498. doi:10.1126/science.1259684
Ibanga, I. E., Fletcher, L. A., Noakes, C. J., King, M. F., and Steinberg, D. (2018). Pilot-scale biofiltration at a materials recovery facility: The impact on bioaerosol control. Waste Manag. 80, 154–167. doi:10.1016/j.wasman.2018.09.010
Jiang, L. Y., Li, H., Chen, J. M., Zhang, D., Cao, S. L., and Ye, J. X. (2016). Combination of non-thermal plasma and biotrickling filter for chlorobenzene removal. J. Chem. Technol. Biotechnol. 91 (12), 3079–3087. doi:10.1002/jctb.4984
Jiang, T., Kennedy, M. D., De Schepper, V., Nam, S. N., Nopens, I., Vanrolleghem, P. A., et al. (2010). Characterization of soluble microbial products and their fouling impacts in membrane bioreactors. Environ. Sci. Technol. 44 (17), 6642–6648. doi:10.1021/es100442g
Kennes, C., Rene, E. R., and Veiga, M. C. (2009). Bioprocesses for air pollution control. J. Chem. Technol. Biotechnol. 84 (10), 1419–1436. doi:10.1002/jctb.2216
King, B., Kesavan, J., and Sagripanti, J.-L. (2011). Germicidal UV sensitivity of bacteria in aerosols and on contaminated surfaces. Aerosol Sci. Technol. 45 (5), 645–653. doi:10.1080/02786826.2010.550959
Ko, G., First, M. W., and Burge, H. A. (2000). Influence of relative humidity on particle size and UV sensitivity of Serratia marcescens and Mycobacterium bovis BCG aerosols. Tuber. Lung Dis. 80 (4-5), 217–228. doi:10.1054/tuld.2000.0249
Kuisiene, N., Raugalas, J., Sproer, C., Kroppenstedt, R. M., and Chitavichius, D. (2008). Bacillus butanolivorans sp nov., a species with industrial application for the remediation of n-butanol. Int. J. Syst. Evol. Microbiol. 58, 505–509. doi:10.1099/ijs.0.65332-0
Kujundzic, E., Hernandez, M., and Miller, S. L. (2007). Ultraviolet germicidal irradiation inactivation of airborne fungal spores and bacteria in upper-room air and HVAC in-duct configurations. J. Environ. Eng. Sci. 6 (1), 1–9. doi:10.1139/s06-039
Li, L., Ma, J. W., Yang, K. X., Chai, F. G., Liu, J. X., and Guo, X. S. (2021a). Microbial aerosol particles in four seasons of sanitary landfill site: Molecular approaches, traceability and risk assessment. J. Environ. Sci. 108, 120–133. doi:10.1016/j.jes.2021.01.013
Li, M. X., Shi, Y. T., Li, Y. X., Sun, Y. Z., Song, C. H., Huang, Z. Y., et al. (2019). Shift of microbial diversity and function in high-efficiency performance biotrickling filter for gaseous xylene treatment. J. Air & Waste Manag. Assoc. 69 (9), 1059–1069. doi:10.1080/10962247.2019.1600603
Li, P., Li, L., Wang, Y., Zheng, T., and Liu, J. (2021b). Characterization, factors, and UV reduction of airborne bacteria in a rural wastewater treatment station. Sci. Total Environ. 751, 141811. doi:10.1016/j.scitotenv.2020.141811
Li, P. Y., Li, L., Yang, K. X., Zheng, T. L., Liu, J. X., and Wang, Y. J. (2021c). Characteristics of microbial aerosol particles dispersed downwind from rural sanitation facilities: Size distribution, source tracking and exposure risk. Environ. Res. 195, 110798. doi:10.1016/j.envres.2021.110798
Lighthart, B., and Shaffer, B. T. (1997). Increased airborne bacterial survival as a function of particle content and size. Aerosol Sci. Technol. 27 (3), 439–446. doi:10.1080/02786829708965483
Mallin, H., Wulf, H., and Bornscheuer, U. T. (2013). A self-sufficient Baeyer-Villiger biocatalysis system for the synthesis of epsilon-caprolactone from cyclohexanol. Enzyme Microb. Technol. 53 (4), 283–287. doi:10.1016/j.enzmictec.2013.01.007
Montero, A., Dueker, M. E., and O'Mullan, G. D. (2016). Culturable bioaerosols along an urban waterfront are primarily associated with coarse particles. Peerj 4, e2827. doi:10.7717/peerj.2827
Moussavi, G., and Mohseni, M. (2007). Using UV pretreatment to enhance biofiltration of mixtures of aromatic VOCs. J. Hazard. Mater. 144 (1-2), 59–66. doi:10.1016/j.jhazmat.2006.09.086
Pahari, A. K., Dasgupta, D., Patil, R. S., and Mukherji, S. (2016). Emission of bacterial bioaerosols from a composting facility in Maharashtra, India. Waste Manag. 53, 22–31. doi:10.1016/j.wasman.2016.04.027
Pearson, C., Littlewood, E., Douglas, P., Robertson, S., Gant, T. W., and Hansell, A. L. (2015). Exposures and health outcomes in relation to bioaerosol emissions from composting facilities: A systematic review of occupational and community studies. J. Toxicol. Environ. Health Part B 18 (1), 43–69. doi:10.1080/10937404.2015.1009961
Quan, Y., Wu, H., Yin, Z., Fang, Y., and Yin, C. (2017). Effect of static magnetic field on trichloroethylene removal in a biotrickling filter. Bioresour. Technol. 239, 7–16. doi:10.1016/j.biortech.2017.04.121
Rene, E. R., Jin, Y. M., Veiga, M. C., and Kennes, C. (2009). Two-stage gas-phase bioreactor for the combined removal of hydrogen sulphide, methanol and -pinene. Environ. Technol. 30 (12), 1261–1272. doi:10.1080/09593330903196868
Salamanca, D., Buhler, K., Engesser, K. H., Schmid, A., and Karande, R. (2021). Whole-cell biocatalysis using the Acidovorax sp. CHX100 Delta 6HX for the production of omega-hydroxycarboxylic acids from cycloalkanes. New Biotechnol. 60, 200–206. doi:10.1016/j.nbt.2020.10.009
Salamanca, D., Dobslaw, D., and Engesser, K. H. (2017). Removal of cyclohexane gaseous emissions using a biotrickling filter system. Chemosphere 176, 97–107. doi:10.1016/j.chemosphere.2017.02.078
Salamanca, D., and Engesser, K. H. (2014). Isolation and characterization of two novel strains capable of using cyclohexane as carbon source. Environ. Sci. Pollut. Res. 21 (22), 12757–12766. doi:10.1007/s11356-014-3206-z
Setlow, P. (2006). Spores of Bacillus subtilis: Their resistance to and killing by radiation, heat and chemicals. J. Appl. Microbiol. 101 (3), 514–525. doi:10.1111/j.1365-2672.2005.02736.x
Spigno, G., Pagella, C., Fumi, M. D., Molteni, R., and De Faveri, D. M. (2003). VOCs removal from waste gases: Gas-phase bioreactor for the abatement of hexane by Aspergillus Niger. Chem. Eng. Sci. 58 (3-6), 739–746. doi:10.1016/s0009-2509(02)00603-6
Staudt, S., Bornscheuer, U. T., Menyes, U., Hummel, W., and Groger, H. (2013). Direct biocatalytic one-pot-transformation of cyclohexanol with molecular oxygen into epsilon-caprolactone. Enzyme Microb. Technol. 53 (4), 288–292. doi:10.1016/j.enzmictec.2013.03.011
Sun, Y. L., Xue, S., Li, L., Ding, W. J., Liu, J. X., and Han, Y. P. (2018). Sulfur dioxide and o-xylene co-treatment in biofilter: Performance, bacterial populations and bioaerosols emissions. J. Environ. Sci. 69, 41–51. doi:10.1016/j.jes.2017.03.039
Uhrbrand, K., Schultz, A. C., Koivisto, A. J., Nielsen, U., and Madsen, A. M. (2017). Assessment of airborne bacteria and noroviruses in air emission from a new highly-advanced hospital wastewater treatment plant. Water Res. 112, 110–119. doi:10.1016/j.watres.2017.01.046
Wang, C., Lu, S., and Zhang, Z. (2019a). Inactivation of airborne bacteria using different UV sources: Performance modeling, energy utilization, and endotoxin degradation. Sci. Total Environ. 655, 787–795. doi:10.1016/j.scitotenv.2018.11.266
Wang, C., Xi, J. Y., and Hu, H. Y. (2009a). Reduction of toxic products and bioaerosol emission of a combined ultraviolet-biofilter process for chlorobenzene treatment. J. Air & Waste Manag. Assoc. 59 (4), 405–410. doi:10.3155/1047-3289.59.4.405
Wang, C., Xi, J. Y., Hu, H. Y., and Yao, Y. (2009b). Advantages of combined UV photodegradation and biofiltration processes to treat gaseous chlorobenzene. J. Hazard. Mater. 171 (1-3), 1120–1125. doi:10.1016/j.jhazmat.2009.06.129
Wang, Y. J., Li, L., Han, Y. P., Liu, J. X., and Yang, K. X. (2018). Intestinal bacteria in bioaerosols and factors affecting their survival in two oxidation ditch process municipal wastewater treatment plants located in different regions. Ecotoxicol. Environ. Saf. 154, 162–170. doi:10.1016/j.ecoenv.2018.02.041
Wang, Y. J., Li, L., Xiong, R., Guo, X. S., and Liu, J. X. (2019b). Effects of aeration on microbes and intestinal bacteria in bioaerosols from the BRT of an indoor wastewater treatment facility. Sci. Total Environ. 648, 1453–1461. doi:10.1016/j.scitotenv.2018.08.244
Wei, Z. S., Sun, J. L., Xie, Z. R., Liang, M. Y., and Chen, S. Z. (2010). Removal of gaseous toluene by the combination of photocatalytic oxidation under complex light irradiation of UV and visible light and biological process. J. Hazard. Mater. 177 (1-3), 814–821. doi:10.1016/j.jhazmat.2009.12.106
Wu, H., Yan, H. Y., Quan, Y., Zhao, H. Z., Jiang, N. Z., and Yin, C. R. (2018). Recent progress and perspectives in biotrickling filters for VOCs and odorous gases treatment. J. Environ. Manag. 222, 409–419. doi:10.1016/j.jenvman.2018.06.001
Wu, H., Yin, Z., Quan, Y., Fang, Y., and Yin, C. (2016). Removal of methyl acrylate by ceramic-packed biotrickling filter and their response to bacterial community. Bioresour. Technol. 209, 237–245. doi:10.1016/j.biortech.2016.03.009
Yeung, M., Saingam, P., Xu, Y., and Xi, J. Y. (2021). Low-dosage ozonation in gas-phase biofilter promotes community diversity and robustness. Microbiome 9 (1), 14. doi:10.1186/s40168-020-00944-4
Yu, J. M., Liu, W., Cheng, Z. W., Jiang, Y. F., Cai, W. J., and Chen, J. M. (2014). Dichloromethane removal and microbial variations in a combination of UV pretreatment and biotrickling filtration. J. Hazard. Mater. 268, 14–22. doi:10.1016/j.jhazmat.2013.12.068
Zhang, J. L., Huang, S. X., Tian, M. Y., Wu, X. R., Samaiti, D., Haheerman, A., et al. (2017). Microbial aerosol in beijing-tianjin-hebei region of eastern China. Int. J. Environ. Pollut. 61 (2), 89–97. doi:10.1504/ijep.2017.085650
Keywords: UV photodegradation, biotrickling filtration, removal performance, bioaerosols, microbial analysis
Citation: Sun Z, Cheng Z, Luo P, Chen J, Yu J, Chen D and Zhao P (2022) Cyclohexane removal and UV post-control of bioaerosols in a combination of UV pretreatment and biotrickling filtration. Front. Environ. Sci. 10:1010980. doi: 10.3389/fenvs.2022.1010980
Received: 03 August 2022; Accepted: 02 September 2022;
Published: 28 September 2022.
Edited by:
Can Wang, Tianjin University, ChinaReviewed by:
Guanlong Yu, Changsha University of Science and Technology, ChinaWei Li, Zhejiang University, China
Copyright © 2022 Sun, Cheng, Luo, Chen, Yu, Chen and Zhao. This is an open-access article distributed under the terms of the Creative Commons Attribution License (CC BY). The use, distribution or reproduction in other forums is permitted, provided the original author(s) and the copyright owner(s) are credited and that the original publication in this journal is cited, in accordance with accepted academic practice. No use, distribution or reproduction is permitted which does not comply with these terms.
*Correspondence: Zhuowei Cheng, endjaGVuZ0B6anV0LmVkdS5jbg==