- 1Institute for Water and Wastewater Technology (IWWT), Durban University of Technology (DUT), Durban, South Africa
- 2Department of Microbiology, University of Uyo, Uyo, Nigeria
- 3Department of Community Health Studies, Faculty of Health Sciences, Durban University of Technology (DUT), Durban, South Africa
- 4Department of Environmental Health Sciences, College of Health Sciences, University of Sharjah, Sharjah, United Arab Emirates
- 5SAMRC Microbial Water Quality Monitoring Centre, University of For Hare, Alice, South Africa
The World Health Organization in 2017 listed vancomycin-resistant enterococci (VRE) among those with high priority for research. This study determined the efficiency of two wastewater treatment plants (WWTPs) in removing both vancomycin-resistant enterococci (VRE) and vancomycin-sensitive enterococci (VSE) from wastewater and the effect of their effluents on the receiving water bodies being reused. VRE and total enterococci (TE) respectively were isolated using Slanetz and Bartley agar with and without vancomycin from wastewater and river samples. Isolate speciation was confirmed by PCR and Matrix-Assisted Laser Desorption/Ionization-Time of Flight (MALDI-TOF) mass spectrometry (MS). Molecular methods were used for confirmation of presumptive VRE and for detection of van genes. Resistance to antibiotics was determined by the disk diffusion method. The TE and VRE counts of the two WWTPs influents ranged from 6.1 to 7.2 log10 CFU/100 ml and 4.3 to 6.7 log10 CFU/100 ml respectively while the effluent counts ranged from 0 to 4.4 log10 CFU/100 ml and 0 to 3.4 log10 CFU/100 ml for the chlorinated effluents. The TE and VRE counts of the recipient river samples were higher than the effluents. Exactly 186 (80.1%) isolates tallied with PCR as Enterococcus while 22 (9.5%) were positive by PCR but negative with MALDI-TOF. Enterococcus faecium and E. faecalis were the most abundant species. The isolates showed 34–100% resistance to quinopristin-dalfopristin, streptomycin, doxycycline, tetracycline, ciprofloxacin and cefixime. VanA (73.8%) were dominant among the isolates. The two WWTPs were efficient in reducing the VRE counts. Thus, the VRE in the river is most likely due to contamination from other sources and it may result in threat to human health when reused.
Introduction
Enterococci are Gram-positive coccoidal bacteria that mainly occur in pairs or short chains, and share some phenotypic attributes with streptococci (Vu and Carvalho, 2011). They consist of several species. Among these, E faecalis and E faecium are normal commensals of the gastrointestinal tract of humans and some other animals with typical concentration in human stool of 104–107 CFU per Gram (Fisher and Phillips, 2009; Boehm and Sassoubre, 2014; Puchter et al., 2018). Enterococci can be recovered from human infection, vegetations and surface water, the latter two partly due to contamination by animal excreta, untreated sewage and poorly treated wastewater (Jett et al., 1994; Hammerum, 2012; Sanderson et al., 2020; Ping et al., 2020). Several species such as E hirae, E faecalis, E casseliflavus, E faecium, E mundtii and E sulfureus have been shown to be associated with vegetations such as forage crops (Cai, 1999; Muller et al., 2001).
Being part of the normal flora of the gastrointestinal tract, Enterococcus species are faecal indicator used in water quality control EU, 2006; USEPA, 2006; and WHO, 2006; Boehm and Sassoubre, 2014. Enterococci can tolerate environmental stressors such as temperature (5–50°C), variable pH (4.5–9.9), 40% bile salts and 6.5% NaCl, desiccation, and oxidative stress (Bradley and Fraise, 1996; Fisher and Phillips, 2009; Arias and Murray, 2012). The resilient ability of enterococci may help them withstand some less stringent WWTP processes. This is evident from the detection of enterococci from chlorinated effluent samples tested by Rosenberg-Goldstein et al. (2014), though the concentration of chlorine was not stated.
Many species of Enterococci are opportunistic pathogens and cause some life-threatening infections such as nosocomial bacteremia, urinary tract infection and wound infection etc. Mortality associated with antibiotic resistant enterococcal bacteremia is several times higher than when associated with susceptible enterococcal bacteremia (Puchter et al., 2018; Athan et al., 2019). Athan et al. (2019) described enterococcal bacteremia as silent but deadly and therefore recommended that the patients with enterococcal bacteraemia should be assessed for colorectal neoplasia. According to European Centre for Disease Prevention and Control, (ECDC, 2012), the prevalence of vancomycin resistance between 2005 and 2010 was much more frequently associated with E. faecium (20.6% United Kingdom, 26.4% Portugal, 32.3% Greece, 35.2% Ireland) than with E. faecalis (2.1% United Kingdom, 4.0% Portugal, 5.3% Greece, 2.1% Ireland). In a study to characterize presumptive vancomycin-resistant enterococci recovered during infection control surveillance in Dallas, Texas, United States, using Spectra VRE agar containing 6 μg/ml vancomycin, robust identification of E. faecium was made by Ping et al. (2020). This shows that the bacteria still have a public health significance worthy of consideration for surveillance.
Vancomycin, is an important glycopeptide antibiotic and the drug of choice against enterococcal infection due to resistance to other antibiotics (Varela et al., 2013). Emergence and spread of vancomycin-resistant enterococci (VRE) are a public health concern and a threat to antibiotic therapy and can pose serious threat if contained in wastewater for reuse. In the US, the prevalence of VRE in health care units in 2003 was 28.5% (Varela et al., 2013) noted, this continues to be significant (Ping et al., 2020). VRE in poorly treated wastewater recycle back directly or indirectly to humans (Rizzo et al., 2013) and so constitute a serious threat to public health. Transmission may be through food crops irrigated with reclaimed water and effluents from WWTPs or discharged directly into streams and thereafter used as irrigation water.
Several studies on the occurrence of antibiotic resistant bacteria in WWTPs and effluents detected VRE at different stages of the wastewater treatment processes and even in the final effluents (Kotzamanidis et al., 2009; Nagulapally et al., 2009; Araújo et al., 2010; Luczkiewicz et al., 2010; Morris et al., 2012; Sanderson et al., 2020). Sadowy and Luczkiewicz (2014) showed that WWTPs constitute an important source of enterococcal strains carrying antimicrobial resistance determinants (ARDs). The ARDs are often associated with the presence of mobile genetic elements (MGE), thus increasing a pool of such genes for potential transfer to other organisms in the environment. Sanderson et al. (2020) reported the detection of VRE isolates, containing E. faecalis, E. faecium, E. casseliflavus and E. gallinarum from the WWTP AMR phenotype.
Managing and minimizing the level of VRE in the effluents from wastewater treatment is an important aspect in reducing infection risk among the exposed individuals when the water is reused. It will also reduce the risk of acquiring antimicrobial resistance by other bacterial species through genetic recombination in the environment. It is therefore important to assess the efficiency of WWTPs in removing VRE and subsequent measures to be taken to curb dissemination of these high-risk bacteria in South Africa because of the immense human health risks such pathogenic bacteria may have via exposure (World Health Organization, 2017; Adegoke et al., 2020; Nzima et al., 2020).
Vancomycin affects the cell wall synthesis by targeting the D-Alanyl-D-Alanine terminus. Thus, resistance by enterococci involves two component systems where the cell wall composition is altered from the peptidoglycan precursor D-Ala-D-Ala (vancomycin-susceptible) to D-Ala-D-lactate (D-Lac) or D-Ala-D-Serine (D-Ser). The D-Ala-D-lactate (D-Lac) and D-Ala-D-Serine (D-Ser) have many times less affinity for vancomycin, thus the susceptible target is removed (Fisher and Philips, 2009). There are six types of resistance to vancomycin which are van A, B, C, D, E, and G. The differences between these phenotypes are based on the composition of their peptidoglycan precursors in response to vancomycin, their inducibility, and in the level of resistance conferred. VanA, VanB- and VanD-types of resistance result from the preferential incorporation of D-alanyl – D-lactate precursors in peptidoglycan (Bugg et al., 1991; Evers et al., 1994; Depardieu et al., 2004), while VanC, VanE and VanG (Arias et al., 2000; Abadia et al., 2002; Depardieu et al., 2003), relate to the ligase that produces D-alanyl – D-serine rather than D-alanyl – D-lactate (Reynolds et al., 1994).
The first outbreak of VRE in a haematology unit in Durban, South Africa was reported in 2016 (Mahabeer et al., 2016). This has raised the need for increased surveillance in clinical settings and the environment. Determination of the prevalence of VRE from the WWTPs and their receiving water bodies will help to reinforce control measures to avoid subsequent outbreaks when treated wastewater is reused along with the recipient surface water. Thus, this paper evaluated the prevalence of VRE in WWTPs and their receiving water bodies, the antibiotic resistant pattern and the putative resistance genes present in these isolates. This will reveal the potential human health risks associated with the reuse for agriculture and domestic purposes.
Materials and Methods
Sample Collection and Brief Description of the Sampling Sites
The sampling sites were two WWTPs collecting municipal wastewater from Durban metropolis and the water bodies receiving their effluents. The choice of the WWTP sites was based on the size, plant configuration and the sources of their wastewater. Plant I has a design capacity of 18.80 ML/d and serves about 30,000 people (working capacity = 10.98 ML/d) while Plant II is slightly bigger; 25 ML/d serving approximately 70,000 people (working capacity = 22.0 ML/d). Plant I is based totally on Trickling Filters with four primary settling tanks, six trickling filters, six secondary settling tanks and three anaerobic digesters (unheated and unmixed). Plant II uses the Activated Sludge System composed of two primary settling tanks, activated sludge reactor, three clarifiers, and three anaerobic digesters (heated and mixed). Both plants have a post-chlorination step where the effluent is disinfected before it is discharged to the respective recipient river.
Samples collected include wastewater (influent, biofilter/clarifier, and the final effluent at the point of discharge to the receiving water body) and the receiving water body samples from both upstream (1 km before) and downstream (1 km after) the discharge point. Samples were collected in parallel. There were nine sampling events spread across a year from July 2016 to May 2017. All samples were collected and transported in appropriate bottles in a cold chain analysed within 12 h.
Isolation and Enumeration of Enterococci from Samples
Standard membrane filtration (EPA, 2002) and spread plate methods were used depending on the expected concentration ranges to quantify total enterococci (TE) and VRE from the samples collected. A modified procedure as used by Rosenberg-Goldstein et al. (2014) for culturing and enumeration of TE and VRE were employed. Ten-fold serial dilutions in the range between 1 and 0.001 ml of the influent and the biofilter/clarifier samples were prepared using sterilized phosphate buffered saline (PBS) due to their expected high microbial counts. Then, 100 µL of each dilution was inoculated onto the medium by the spread plate method in duplicate on membrane-Enterococcus Slanetz and Bartley (mS&B) agar to isolate total enterococci, and mS&B agar modified with 6 μg/ml of vancomycin added to isolate VRE (Anderson et al., 2013; CLSI, 2020; Gazel et al., 2021). For the effluent and river samples 10 ml or 100 ml duplicate aliquots were filtered through 0.45 µm, 47 mm membrane filters (Millipore, Billerica, MA). Filters were placed on the media as above. Plates were incubated at 35°C for 4 h and then at 44°C for 44 h. Colonies with pink to maroon red colours were considered presumptive TE and VRE respectively. Distinct colonies with various colours (pink to maroon red) were selected. Depending on the count, one out of every five colonies were chosen with at least two isolates from each plate. These colonies were purified on Brain Heart Infusion (BHI) agar.
For preliminary identification, purified colonies were tested for Gram reaction, catalase and pyrrolidonyl peptidase (Pyrase test) and growth on bile aesculine agar. All Gram-positive cocci in pairs or short chains, catalase negative, pyrrolidonyl peptidase positive which produced dark colouration on bile aesculin agar were considered to be presumptive Enterococcus and thus stored in BHI broth with 25% glycerol at −80°C for further studies.
DNA Isolation
DNA extraction was carried out using the boiling method as described by Micallef et al. (2013). The glycerol stock isolates were streaked on Brain Heart Infusion Agar. A loopful of 24 h respective isolate cultures were transferred to 2 ml Eppendorf tubes with 500 µL of sterile distilled water. These were heated, in a heat block (Benchmark Digital heat block) at 100°C for 10 min and then centrifuged at 14,000rpm for 15 min. Then 300 µL of the supernatant, which contained the DNA, was carefully taken and stored in −20°C. This served as DNA template for all the PCR based reactions.
Generic and Species-Specific Identification of the Isolate by Polymerase Chain Reaction
Both the genus and species primers were synthesized by Inqaba Biotech., South Africa. For genus identification, Enterococcus primer, El (5′ TCA ACC GGG GAG GGT 3′) and E2 (5′ ATT ACT AGC GAT TCC GG 3′) and PCR condition developed by Deasy et al. (2000) was used. Briefly, the DNA amplification was carried out in a final volume of 20 µL containing 10 µL of Hotstart green mastermix (Thermofisher), 20 µM each of the forward and reverse primers, water and 5 µL of DNA template. The cycling condition in a thermocycler (Biorad, South Africa) consisted of an initial denaturation step of 95°C for 5 min, 25 cycles in a denaturation at 94°C for 1 min, annealing at 60°C for 1 min, followed by polymerization at 72°C for 1 min. Five microlitres of the PCR product was electrophoresed on a 2% (w/v) agarose gel using Ix TAE buffer (40 mM Tris acetate, 1 mM EDTA, pH 8) stained with gel red and a 100-bp ladder was used as a marker. This was run at 100 V for 60 min and visualized with UV transilluminator (BIORAD, South Africa) and photographed.
A multiplex convectional PCR as adapted from Jackson et al. (2004) was used to identify the species of the confirmed Enterococcus isolates. This was modified by using DNA template instead of the whole cell suspension. The twenty-three Enterococcus species and the multiplex groups as adapted from Jackson et al. (2004) were used (Table 1). PCR was carried out in a 25 µL reaction volume consisting of; 12.5 µL PCR mastermix (DreamTag MM Thermofisher), 0.2 µL each of the forward and reverse primers for genus and species; nuclease free water; and 5 µL of DNA template. The primers for the reaction are listed in Table 1. The thermocycling conditions were as follows; initial denaturation at 95°C for 4 min, products were amplified by 30 cycles of denaturation at 95°C for 30 s, annealing at 55°C (groups 1, 2, 5, and 6) or 60°C (groups 3, 4, and 7) for 1 min, and elongation at 72°C for 1 min. This was followed by a final extension at 72°C for 7 min. Five microliters of product was electrophoresed on a 2% 1 × Tris-acetate-EDTA agarose gel containing gel red stain. DNA molecular weight marker (100 bp; Roche) was used as the standard. The gel was run for 1 h at 100 V, the expected band size being 733 bp.
Further Confirmatory Identification by Matrix-Assisted Laser Desorption/Ionization Time-of-Flight Mass Spectrometry
Selected isolates were sent to Department of Infectious Diseases, University of Gothenburg, Sweden for further confirmatory identification using Matrix-assisted laser desorption/ionization time-of-flight mass spectrometry (MALDI-TOF-MS).
Antibiotic Susceptibility Testing
Inoculum for AST was standardized to 0.5 McFarland standards by the methods of Komolafe and Adegoke (2008). Four pure colonies of each isolate from a 24 h Brain Heart Infusion plate culture were inoculated into 2 ml sterile peptone water broth. This was incubated at 37°C for 6 h and the turbidity adjusted by diluting with PBS (pH 7.2) to 0.5 McFarlands (105 CFU/ml). Hundred microliter (100 µL) of this culture dilution was used to inoculate Muller-Hinton agar (MHA) plates using sterile swab stick to obtain a uniform spread. Antibiotic discs were aseptically placed on the inoculated MHA plate and were cultures read after 24 h incubation at 37°C. The following antibiotics were used: Azithromycin (15 µg), Vancomycin (30 µg), Imipenem (10 µg), Teicoplanin (30 µg), Tetracycline (30 µg), Doxycline (30 µg), Amoxyl-clav (30 µg), Gentamicin (10 µg), Streptomycin (10 µg), Ciprofloxacin (5 µg), Cefixime (5 µg), and Quinupristin-dalfopristin (15 µg). The choice of these antibiotics is based on their reported activity against gram-positive bacteria as well as being on the list of WHO first line of antibiotics (WHO, 2015). Since vancomycin used in the primary isolation medium was 6 μg/ml, a higher dose was used during susceptibility testing to evaluate if a higher dose could inhibit growth. The zone of inhibition was measured and then interpreted using CLSI (2016) and BSAC (2015) standards.
The antibiogram was presented to depict the spread of resistance across the various sampling points.
Multiple Antibiotic Resistance Index
The multiple antibiotic resistance index (MARI) was determined following the methods of Krumperman (1983) and Adegoke and Okoh (2014). This was calculated as the ratio of the number of the antibiotics to which resistance occurred by the isolates (A) to the total number of antibiotics to which the isolates were tested (B).
All the antibiotics except vancomycin and teicoplanin used in the AST were also considered for MARI determinations as organisms’ exhibit unequal profile to antibiotics within the same class. Teicoplanin is a glycopeptide as vancomycin and vancomycin was excluded in the MARI determination, because it was the original supplement in the isolation medium.
Detection of Van A, B, C1 and C2/3 genes.
Multiplex PCR as adapted from Nam et al. (2013) was used for the detection of Van A, B, C1 and C2/3 genes. The reaction mix was composed of 12.5 µL PCR mastermix (DreamTag MM Thermofisher), 20 µM of each forward and reverse primers, water, 5 µL of the DNA template in a final reaction volume of 25 µL. The cycling condition involves the initial denaturation at 94°C for 5 mins, 35 cycles consisting of 45 s at 94°C, 60 s at 54°C, and 90 s at 72°C, with final extension for 15 min at 72°C. Products were resolved by electrophoresis in 2% agarose gel using 110 V for 45 min. Results were determined using the expected band size on 100 bp ladder. The list of the primers as adapted by Nam et al. (2013) are summarised in Table 2.
Statistical Analysis
Descriptive statistics were used to analyze the result of the antibiotic susceptibility test and the putative antibiotic resistance genes. T-test was used to compare the enterococci count between relevant sampling points and p value of less than or equal to 0.05 was considered to be significant. Correlation analysis was conducted in terms of the putative antibiotic resistance genes.
Results
Enumeration of Enterococci
The TE counts ranged between 6,2 and 7,2 log10 CFU/100 ml in wastewater influent in both WWTPs. The VRE counts were persistently higher in WWTP II than in WWTP I. The counts in both plants did not show any seasonal variation. Plant II had a higher percentage of VRE (4.2%) than Plant I (1.1%), except in March, when Plant I was 0.3% higher (Figure 1 and Table 3). On two sampling months (July and February), no VRE was detected from Plant I but occurred in Plant II. Figure 1 shows the TE and VRE counts in the influents of the two WWTPs.

TABLE 3. Mean counts of the presumptive enterococci of various sampling points expressed as Log10/100 ml values.
Varying TE- and VRE log10 removal occurred over the various steps of the treatment processes. Log10 removal of TE in the secondary treatment (biofiltration) ranged from log10 0.99 to 2.47 (89.9–99.9%) for Plant I and log10 1,22 to 2,55 (94–98%) for Plant II. Log10 removal of VRE at this secondary treatment step also was in the same range or higher, ranging from 1,17 to 4.88 (95–100%) for Plant I and 1,06 to 4,85 (96–100%) for Plant II. There was 100% removal efficiency of both TE and VRE at the Plant I, but Plant II had between 94.0 and 100% removal efficiency (Table 3).
Even though the TE counts in the influents of the two plants is high, the VRE counts was low (0.5 -3.7%) for Plant I but higher in Plant II WWTP where the VRE count ranged from 1.2 – 35%.
Effect of the Effluents on the Recipient
The upstream count (TE and VRE) of both recipients of both plants were higher than at their respective downstream location (Table 4). Also, the downstream counts of TE and VRE were higher than that of the effluents. There was no measurable effect of the effluent on the downstream count of Plant II.
Confirmation of the Isolates
Out of the 205 VRE isolates which were gram-positive cocci in chains, catalase negative, pyrase positive and formed dark colonies on bile aesculine agar, 186 were confirmed by PCR as Enterococcus species. The species diversities include E. faecium and E. faecalis with E. casseliflavus as the least abundant. Others include E. durans, E. hirae, E. cecorium. and E. gallinarum (Figure 2).
Antibiotic Resistant Pattern of the VRE Isolates
In total, 186 VRE isolates were subjected to antibiotic susceptibility tests. As shown in Table 5, there were multidrug resistant isolates showing high resistance to cefixime (100%), ciprofloxacin and tetracycline (90.1%) and streptomycin (91.1%). For Quinopristin-dalfopristin, there was a marked zone of inhibition with most isolates but it was not large enough to be interpreted as sensitive. Overall there was a greater number of the VRE isolates interpreted as intermediate or resistant than sensitive to Quinopristin-dalfopristin. Highest sensitivity was observed with Amoxy-clav followed by Imipenem, Azithromycin and Gentamicin. Since Vancomycin was used in the isolation medium, resistance to Teicoplanin and Vancomycin, were expected because they are related glycopeptides. Apart from the isolate, E casseflavus that was sensitive to Teicoplanin and Vancomycin. The summary is shown in Tables 5.
The spread of the resistance on various sampling points revealed relatively similar trend, but on one sampling occasion, effluents from Plant II had the highest resistance (compared to other points) to Azithromycin (37.5%). On the same sampling occasion, 5 isolates from Plant II effluent and 1 isolate from Plant II secondary treatment stage (clarifiers), which were all Enterococcus faecium were resistant to all the 12 antibiotics used.
These highly resistant E. faecium were, however, not detected in Plant I and the two recipients. Plant II downstream isolates showed 14.8% resistance to imipenem and 7.4% resistance to amoxicillin-clavulanic acid.
MARI of ≥0.2 was observed among all the isolates with the highest percentage (50.3%) of the isolates having MARI of 0.5. About 3.4% of the isolates showed resistance to all the antibiotics used in the study (MARI = 1). The details of the MARI and the percentage of the isolates are depicted in Figure 3.
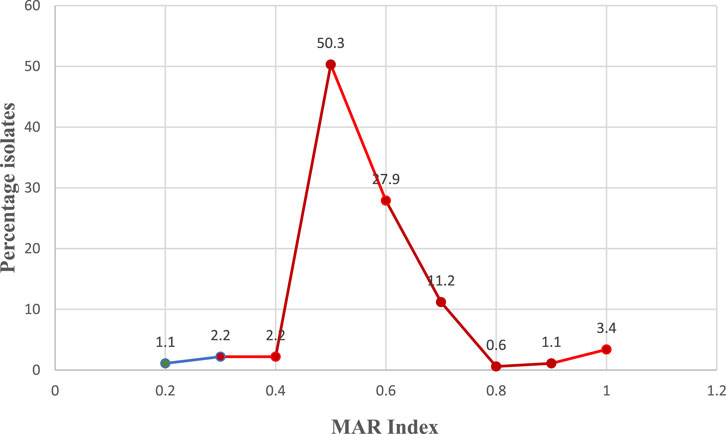
FIGURE 3. Multiple antibiotic resistant index (MAR Index) of the VRE isolates and percentage involved. *Red zone revealed the epidemiological high-risk isolates zone (MAR Index >0.2). The subsequent MAR Index value of the VRE isolates after 0.2 is 0.3 from which the red zone on the figure started.
Detection of Van Genes
One hundred and eighty VRE isolates were tested for presence of Van A, B, C1 and C2/3 genes. Each of the isolates showed the presence of, at least, one of the van genes by multiplex PCR. Van A, C1 and C2/3 were detected. Greater percentage of the isolates (80.3%) had van A as seen clearly by the expected band size followed by van C1 (18.4). Presence of van C1 was detected only in E. gallinarum and E. cecorum while van A was common among E. faecalis, E. faecium and other enterococci (unspeciated). A band size of 420bp, which is not the expected band size for van B was formed, when a singleplex PCR was done using van B primers thus confirming vanB not present.
Discussion
WWTPs are described as hotspots for dissemination of VRE. This is due to high concentration and variety of bacteria carrying resistance genes from different sources and thus enabling environment for horizontal gene transfer (Rizzo et al., 2013). Improper wastewater treatment plant processes could lead to pollution of their receiving water bodies with harmful pathogens such as VRE. This study was designed to determine the efficiency of two WWTPs in removing VRE from wastewater and thus their impact in their receiving water bodies. Our findings showed that VRE count in the influent of the two plants ranged between 4,46 and 5,87 log10 CFU/100 ml. The VRE counts were reduced down by the treatment process such that their effluents had very little or no influence on the receiving water bodies.
Our study coincides with data from other studies where VRE were detected in wastewater samples including effluents in this study. Our findings differed by the increased concentration of VRE isolates involved as compared to their fewer VRE isolate (Nagulapally et al., 2009; Kotzamanidis et al., 2009; Araújo et al., 2010; Luczkiewicz et al., 2010; Morris et al., 2012). However, our use of media supplemented with Vancomycin during the primary isolation could contribute to possible detection of all VRE present in the samples analysed. Enterococci are normal gastrointestinal flora and will be present in large number in influent samples as can be seen in high counts of TE (6,2 _ 6,7 for Plant I; 6,3 – 7,2 for Plant II) and VRE (4,0 – 4,9 for Plant I; 4,4 – 5,8 for Plant II) in our study.
Treatment processes are expected to bring about the reduction of pathogen concentration. A high reduction occurred in the counts from influent to effluent with varying efficiencies with plant (100% in Plant I; 94–100% in Plant II) mainly as a result of the effect of chlorination. Chlorination does not necessarily kill all bacterial pathogens, but it renders some non-culturable, even when they are still viable (Rizzo et al., 2004; Oliver et al., 2005; Ding et al., 2017). The non-culturable bacteria may still be a threat in conferring both virulence and antibiotic resistance genes on other bacteria in the receiving water environment (Rizzo et al., 2004). There was no clear impact of the receiving water with viable Enterococci by the effluent in this study as the upstream samples had higher concentration than the downstream although the difference is not statistically significant (p > 0.05). Contamination of the receiving surface water might most probably emanate from other sources. Surface water are exposed to pollution from multiple sources in addition to wastewater effluents like farm yard runoff and other human activities (Pignata et al., 2012).
The proportion of VRE to TE isolates were different between the two plants, with consistently lower values in Plant I samples (both wastewater and river samples) than from Plant II. The difference in VRE count of influent between the two plants are statistically significant (p < 0.05). These differences could be due to geographic differences in human VRE infection rates (Bouchillon et al., 2005; Rosenberg-Goldstein et al., 2014) or due to their different sources of wastewater. Plant I is located in a local township area while Plant II is in a suburban setting with larger population and thus may account for the higher concentration of VRE Plant II. In addition, the design and working capacity of Plant II is larger than Plant I. In addition, there is a livestock farm close to Plant II and on several sampling occasions, the livestock were seen grazing around the stream. This could have contributed to the prevalence of VRE in the stream samples rather than from the wastewater effluents.
This study showed even distribution of Enterococcus species in both WWTPs and the receiving water, though there is none or just a few in the effluents of Plant I and Plant II. E. faecium and E. faecalis dominated the isolates and also dominate the Enterococci in the normal flora of the gastrointestinal tract (Agudelo and Huycke, 2013; Lebreton et al., 2014). Several other studies have also identified E faecium and E faecalis as the most common species of VRE isolated from wastewater Talebi et al. (2008); Morris et al. (2012); Rosenberg-Goldstein et al. (2014). Other species such as E durans, E hirae, E cecorium and E gallinarum are normally less abundant than E faecium and E faecalis as evidenced in this study and in line with the report of Leclercq et al. (2013).
Although the antibiotic resistance pattern of the VRE isolates showed sensitivity to Amoxy-clav, Imipenem and to some extent Gentamicin and Azithromycin, their high resistance to Quinopristin/dalfopristin and Streptomycin is of concern. This was in tandem with the report of Gotkowska-Płachta (2020) that majority of the E. faecalis and E. faecium isolated were highly resistant to Streptomycin. This implies that Quinopristin/dalfopristin which has been a valuable alternative to the treatment of VRE infections (Wang et al., 2016) may no longer be as potent for chemotherapy. Since Vancomycin was used in the isolation medium, resistance to Teicoplanin and Vancomycin, were expected as they are glycopeptides and as such are related. The World Health Organization has in early 2017 listed VRE among those with highest priority for further surveillance and research, both among humans and in the receiving aquatic environment. Resistance to imipenem and cefixime in secondary treatment stage, effluent and downstream samples of Plant II also showed that the isolates from these sample sites are potential beta lactamase producers with immense public health importance (Radhouani et al., 2014). Resistance to all the 12 antibiotics used as observed in Plant II effluent showed that potentially difficult-to-treat isolates are being released at the plant. Varying antibiotic susceptibility profiles are expected from various sectors of one health continuum due to varying application of antibiotics (Zaheer et al., 2020). This is expected to select for the emergence of multidrug resistance based on what number of antibiotics an isolate has been exposed (Adegoke et al., 2016). Generally, an observation of MARI >0.2 shows that 98.9% of the isolates are from high risk to very high risk sources, reflecting potential antibiotic abuse and high selective pressure (Suresh et al., 2000) or the potential release of multiple antibiotic resistant strains into the environment from the hospital patients or convalescent individuals at home (Adegoke et al., 2016; Adegoke et al., 2020). It also showed the epidemiological high-risk isolates zone (MARI >0.2), too high for reuse of the sampled water source. The subsequent MARI value of the VRE isolates after 0.2 is 0.3 from which the red zone on the figure started (Figure 3). However, following a longitudinal surveillance of antibiotic resistance in Escherichia coli and Enterococcus spp. from a Wastewater Treatment Plant in Kwazulu Natal, South Africa, Mbanga et al. (2021) observed a lower MARI that ranged from 1.83 to 3.98 among Enterococcus spp.
VanC1 was detected in all the E gallinarum of VRE isolated which portrays the intrinsic vanC resistance specific to E gallinarum. However, vanA was detected in 80.3% of the VRE isolates while vanB was not detected. This differs from the previous findings by Iweriebor et al. (2015) who found that Enterococcus isolated from wastewater and their effluents in Alice, South Africa, contained vanB, vanC1 and vanC2/3 but no vanA genes; and Nam et al. (2013) who detected only vanC1 and vanC2/3 genes from Korean aquatic environments. Gotkowska-Płachta (2020) reported that the Enterococcal strains isolated from wastewater and downstream bear van genes and showed 57% phenotypic resistance, in which majority bore vanC1 genes. VanA type of resistance is mostly inducible and confers high level of resistance (resistance to high concentration) to both Vancomycin and Teicoplanin (Fisher and Phillips, 2009). The vanA gene has been shown to be on transposons (Arthur et al., 1993) and thus can easily be transferred. This may explain their detection in the majority (80.3%) of the VRE isolates in this study. According to Giraffa, (2002), resistance genes can be transferred to both antibiotics susceptible enterococci and other pathogens. In the same way, vanA resistance gene can easily be transferred to other pathogens within the environment. Thus, a high prevalence of vanA genes among the isolates is a public health risk.
Conclusion
The two WWTPs showed efficient in removing VRE from wastewater. VRE present in the stream samples that receive their effluents were not as a result of the discharged effluents but from other sources since the upstream counts were higher than that of the downstream. The use of MALDI-ToF-MS added values to the speciation process and infer acceptable concord with PCR for continuing use. The presence of vanA genes in greater percentage of the isolates has potential public health impact since they can be easily transferred to other pathogens. The treated wastewater can be put to cautious reuse because of the very low level of Enterococcus contaminants in them. Further work on the proper risk assessment of using Plant I and Plant II recipient water as sources of water for agricultural and other purposes should be done.
Data Availability Statement
The original contributions presented in the study are included in the article/Supplementary Material, further inquiries can be directed to the corresponding author.
Author Contributions
TS and AA conceptualized the concepts. All authors contributed in developing the concept. AA and CM carried out the laboratory analysis with contributions from all authors. All authors contributed in the development of the manuscript.
Funding
The South African Research Chairs Initiative (SARChI), Durban University of Technology (DUT), and the University of Fort Hare (UFH) supported the research and publication.
Conflict of Interest
The authors declare that the research was conducted in the absence of any commercial or financial relationships that could be construed as a potential conflict of interest.
Publisher’s Note
All claims expressed in this article are solely those of the authors and do not necessarily represent those of their affiliated organizations, or those of the publisher, the editors and the reviewers. Any product that may be evaluated in this article, orclaim that may be made by its manufacturer, is not guaranteed or endorsed by the publisher.
Acknowledgments
We appreciate the workers of the two wastewater treatment plants for their assistance during sample collection. Thanks to South African Chair Initiative (SARChI) for funding the research and Institute for Water and Wastewater Technology, Durban University of Technology, Durban for providing us with an enabling research environment.
Abbreviations
mS&B, membrane-Enterococcus Slanetz and Bartley; TE, Total Enterococci; WWTP, Wastewater treatment plant.
References
Abadía Patiño, L., Courvalin, P., and Perichon, B. (2002). vanE Gene Cluster of Vancomycin-Resistant Enterococcus faecalis BM4405. J. Bacteriol. 184 (23), 6457–6464. doi:10.1128/jb.184.23.6457-6464.2002
Adegoke, A. A., Madu, C. E., Aiyegoro, O. A., Stenström, T. A., and Okoh, A. I. (2020). Antibiogram and Beta-Lactamase Genes Among Cefotaxime Resistant E. coli from Wastewater Treatment Plant. Antimicrob. Resist. Infect. Control. 9, 1–2. doi:10.1186/s13756-020-0702-4
Adegoke, A. A., and Okoh, A. I. (2014). Species Diversity and Antibiotic Resistance Properties of Staphylococcus of Farm Animal Origin in Nkonkobe Municipality, South Africa. Folia Microbiol. 59 (2), 133–140. doi:10.1007/s12223-013-0275-1
Adegoke, A., Faleye, A., Singh, G., and Stenström, T. (2016). Antibiotic Resistant Superbugs: Assessment of the Interrelationship of Occurrence in Clinical Settings and Environmental Niches. Molecules 22 (1), 29. pii. doi:10.3390/molecules22010029
Agudelo, H. N. I., and Huycke, M. M. (2014). “Enterococcal Disease, Epidemiology, and Implications for Treatment,” in Enterococci: From Commensals to Leading Causes of Drug Resistant Infection Boston: Massachusetts Eye and Ear Infirmary. Editors M. S. Gilmore, D. B. Clewell, and Y. Ike. Available from: https://www.ncbi.nlm.nih.gov/books/NBK190429/.
Anderson, N. W., Buchan, B. W., Young, C. L., Newton, D. W., Brenke, C., Lapsley, L., et al. (2013). Multicenter Clinical Evaluation of VRESelect agar Foridentification of Vancomycin-Resistant Enterococcus faecalis and Enterococcus Faecium. J. Clin. Microbiol. 1 (8), 2758–2760. doi:10.1128/JCM.00979-13
Araújo, C., Torres, C., Silva, N., Carneiro, C., Gonçalves, A., Radhouani, H., et al. (2010). Vancomycin-resistant Enterococci from Portuguese Wastewater Treatment Plants. J. Basic Microbiol. 50, 605–609. doi:10.1002/jobm.201000102
Arias, C. A., Courvalin, P., and Reynolds, P. E. (2000). vanC Cluster of Vancomycin-Resistant Enterococcus Gallinarum BM4174. Antimicrob. Agents Chemother. 44 (6), 1660–1666. doi:10.1128/aac.44.6.1660-1666.2000
Arias, C. A., and Murray, B. E. (2012). The Rise of the Enterococcus: beyond Vancomycin Resistance. Nat. Rev. Microbiol. 10, 266–278. doi:10.1038/nrmicro2761
Arthur, M., Molinas, C., Depardieu, F., and Courvalin, P. (1993). Characterization of Tn1546, a Tn3-Related Transposon Conferring Glycopeptide Resistance by Synthesis of Depsipeptide Peptidoglycan Precursors in Enterococcus Faecium BM4147. J. Bacteriol. 175 (1), 117–127. doi:10.1128/jb.175.1.117-127.1993
Athan, E., Cabiltes, I., Coghill, S., and Bowe, S. J. (2019). Silent but Deadly: Patients with Enterococcal Bacteraemia Should Be Assessed for Colorectal Neoplasia. Med. J. Aust. 210 (2), 86. doi:10.5694/mja2.12027
Boehm, A. B., and Sassoubre, L. M. (2014). “Enterococci as Indicators of Environmental Fecal Contamination,” in Enterococci from Commensals to Leading Causes of Drug Resistant Infection. Editors M. S. Gilmore, D. B. Clewell, Y. Ike, and N. Shankar (Boston: Massachusetts Eye and Ear Infirmary). Available from: https://www.ncbi.nlm.nih.gov/books/NBK190429.
Bouchillon, S. K., Hoban, D. J., Johnson, B. M., Johnson, J. L., Hsiung, A., and Dowzicky, M. J. (2005). In Vitro activity of Tigecycline against 3989 Gram-Negative and Gram-Positive Clinical Isolates from the United States Tigecycline Evaluation and Surveillance Trial (TEST Program; 2004). Diagn. Microbiol. Infect. Dis. 52, 173–179. doi:10.1016/j.diagmicrobio.2005.06.004
Bradley, C. R., and Fraise, A. P. (1996). Heat and Chemical Resistance of Enterococci. J. Hosp. Infect. 34, 191–196. doi:10.1016/s0195-6701(96)90065-1
BSAC (2015). British Society for Antimicrobial Chemotherapy, Interpretation Guideline. version 14. Available from: http://bsac.org.uk/wp-content/uploads/2012/02/BSAC-disc-susceptibility-testing-method-Jan-2015.pdf.
Bugg, T. D. H., Dutka-Malen, S., Arthur, M., Courvalin, P., and Walsh, C. T. (1991). Identification of Vancomycin Resistance Protein VanA as a D-alanine:D-Alanine Ligase of Altered Substrate Specificity. Biochemistry 30 (8), 2017–2021. doi:10.1021/bi00222a002
Cai, Y. (1999). Identification and Characterization of Enterococcus Species Isolated from Forage Crops and Their Influence on Silage Fermentation. J. Dairy Sci. 82, 2466–2471. doi:10.3168/jds.s0022-0302(99)75498-6
CLSI (2016). Performance Standards for Antimicrobial Susceptibility Testing. M100-S25. Wayne, NJ: Clinical Laboratory Standard Institute.
CLSI (2020). Performance Standards for Antimicrobial Susceptibility Testing. M100-S30. Wayne, NJ: Clinical Laboratory Standard Institute.
Deasy, B. M., Rea, M. C., Fitzgerald, G. F., Cogan, T. M., and Beresford, T. P. (2000). A Rapid PCR Based Method to Distinguish between Lactococcus and Enterococcus. Syst. Appl. Microbiol. 23, 510–522. doi:10.1016/s0723-2020(00)80025-9
Depardieu, F., Bonora, M. G., Reynolds, P. E., and Courvalin, P. (2003). The vanG Glycopeptide Resistance Operon from Enterococcus faecalis Revisited. Mol. Microbiol. 50 (3), 931–948. doi:10.1046/j.1365-2958.2003.03737.x
Depardieu, F., Kolbert, M., Pruul, H., Bell, J., and Courvalin, P. (2004). VanD-type Vancomycin-Resistant Enterococcus Faecium and Enterococcus faecalis. Antimicrob. Agents Chemother. 48 (10), 3892–3904. doi:10.1128/aac.48.10.3892-3904.2004
Ding, T., Suo, Y., Xiang, Q., Zhao, X., Chen, S., Ye, X., et al. (2017). Significance of Viable but Nonculturable Escherichia coli: Induction, Detection, and Control. J. Microbiol. Biotechnol. 27 (3), 417–428. doi:10.4014/jmb.1609.09063
ECDC- (2012). European Centre for Disease Prevention and Control. Available from: http://ecdc.europa.eu/en/healthtopics/antimicrobial_resistance/Pages/index.aspx (Accessed August, 2017).
EPA (2002). Method1600: Enterococci in Water by Membrane Filtration Using Membrane- Enterococcus Indoxyl-B-D-Glucosideagar (mEI) 1600.
Evers, S., Reynolds, P. E., and Courvalin, P. (1994). Sequence of the vanB and Ddl Genes Encoding D-Alanine:d-Lactate and D-Alanine:d-Alanine Ligases in Vancomycin-Resistant Enterococcus faecalis V583. Gene 140 (1), 97–102. doi:10.1016/0378-1119(94)90737-4
Fisher, K., and Phillips, C. (2009). The Ecology, Epidemiology and Virulence of Enterococcus. Microbiol 155, 1749–1757. doi:10.1099/mic.0.026385-0
Gazel, D., Erinmez, M., Büyüktaş Manay, A., and Zer, Y. (2021). Investigation of Heteroresistant Vancomycin Intermediate Staphylococcus aureus Among MRSA Isolates. J. Infect. Dev. Ctries 15 (1), 89–94. doi:10.3855/jidc.12799
Giraffa, G. (2002). Enterococci from Foods. FEMS Microbiol. Rev. 26, 163–171. doi:10.1111/j.1574-6976.2002.tb00608.x
Gotkowska-Płachta, A. (20202021). The Prevalence of Virulent and Multidrug-Resistant Enterococci in River Water and in Treated and Untreated Municipal and Hospital Wastewater. Ijerph 18, 563. doi:10.3390/ijerph18020563
Hammerum, A. M. (2012). Enterococci of Animal Origin and Their Significance for Public Health. Clin. Microbiol. Infect. 18, 619–625. doi:10.1111/j.1469-0691.2012.03829.x
Jackson, C. R., Fedorka-Cray, P. J., and Barrett, J. B. (2004). Use of a Genus- and Species-specific Multiplex PCR for Identification of Enterococci. J. Clin. Microbiol. 42 (8), 3558–3565. doi:10.1128/jcm.42.8.3558-3565.2004
Jett, B. D., Huycke, M. M., and Gilmore, M. S. (1994). Virulence of Enterococci. Clin. Microbiol. Rev. 7, 462–478. doi:10.1128/cmr.7.4.462
Komolafe, A. O., and Adegoke, A. A. (2008). Incidence of Bacterial Septicaemia in Ile-Ife Metropolis, Nigeria. Malaysian J. Microbiol. 4 (2), 51–61. doi:10.21161/mjm.13008
Kotzamanidis, C., Zdragas, A., Kourelis, A., Moraitou, E., Papa, A., Yiantzi, V., et al. (2009). Characterization ofvanA-typeEnterococcus Faeciumisolates from Urban and Hospital Wastewater and Pigs. J. Appl. Microbiol. 107, 997–1005. doi:10.1111/j.1365-2672.2009.04274.x
Krumperman, P. H. (1983). Multiple Antibiotic Resistance Indexing of Escherichia coli to Identify High-Risk Sources of Fecal Contamination of Foods. Appl. Environ. Microbiol. 46 (1), 165–170. doi:10.1128/aem.46.1.165-170.1983
Lebreton, F., Willems, R. J. L., and Gilmore, M. S. (2014). “Enterococcus Diversity, Origins in Nature, and Gut Colonization,” in Enterococci: From Commensals to Leading Causes of Drug Resistant Infection. Editors M. S. Gilmore, D. B. Clewell, and Y. Ike. (Boston: Massachusetts Eye and Ear Infirmary). Available from: https://www.ncbi.nlm.nih.gov/books/NBK190427/.
Leclercq, R., Oberl�, K., Galopin, S. ½., Cattoir, V., Budzinski, H. ½. ½., and Petit, F. (2013). Changes in Enterococcal Populations and Related Antibiotic Resistance along a Medical Center-Wastewater Treatment Plant-River Continuum. Appl. Environ. Microbiol. 79 (7), 2428–2434. doi:10.1128/aem.03586-12
Luczkiewicz, A., Jankowska, K., Fudala-Ksiazek, S., and Olanczuk-Neyman, K. (2010). Antimicrobial Resistance of Fecal Indicators in Municipal Wastewater Treatment Plant. Water Res. 44, 5089–5097. doi:10.1016/j.watres.2010.08.007
Mahabeer, Y., Lowman, W., Govind, C. N., Swe-Swe-Han, K., and Mlisana, K. P. (2016). First Outbreak of Vancomycin-Resistant Enterococcus in a Haematology Unit in Durban, South Africa. South. Afr. J. Infect. Dis. 31 (1), 20–24. doi:10.4102/sajid.v31i1.100
Mbanga, J., Abia, A. L. K., Amoako, D. G., and Essack, S. Y. (2021). Longitudinal Surveillance of Antibiotic Resistance in Escherichia coli and Enterococcus Spp. From a Wastewater Treatment Plant and its Associated Waters in KwaZulu-Natal, South Africa. Microb. Drug Resist. 27 (7), 904–918. doi:10.1089/mdr.2020.0380
Micallef, S. A., Rosenberg Goldstein, R. E., George, A., Ewing, L., Tall, B. D., Boyer, M. S., et al. (2013). Diversity, Distribution and Antibiotic Resistance of Enterococcus Spp. Recovered from Tomatoes, Leaves, Water and Soil on U.S. Mid-Atlantic Farms. Food Microbiol. 36, 465–474. doi:10.1016/j.fm.2013.04.016
Morris, D., Galvin, S., Boyle, F., Hickey, P., Mulligan, M., and Cormican, M. (2012). Enterococcus Faecium of thevanAGenotype in Rural Drinking Water, Effluent, and the Aqueous Environment. Appl. Environ. Microbiol. 78, 596–598. doi:10.1128/aem.06636-11
Muller, T., Ulrich, A., Ott, E.-M., and Muller, M. (2001). Identification of Plant-Associated Enterococci. J. Appl. Microbiol. 91, 268–278. doi:10.1046/j.1365-2672.2001.01373.x
Nagulapally, S. R., Ahmad, A., Henry, A., Marchin, G. L., Zurek, L., and Bhandari, A. (2009). Occurrence of Ciprofloxacin-, Trimethoprim-Sulfamethoxazole-, and Vancomycin-Resistant Bacteria in a Municipal Wastewater Treatment Plant. Water Environ. Res. 81, 82–90. doi:10.2175/106143008x304596
Nam, S., Kim, M.-j., Park, C., Park, J.-G., Maeng, P. J., and Lee, G.-C. (2013). Detection and Genotyping of Vancomycin-Resistant Enterococcus Spp. By Multiplex Polymerase Chain Reaction in Korean Aquatic Environmental Samples. Int. J. Hyg. Environ. Health 216, 421–427. doi:10.1016/j.ijheh.2012.12.004
Nzima, B., Adegoke, A. A., Ofon, U. A., Al-Dahmoshi, H. O. M., Saki, M., Ndubuisi-Nnaji, U. U., et al. (2020). Resistotyping and Extended-Spectrum Beta-Lactamase Genes Among Escherichia coli from Wastewater Treatment Plants and Recipient Surface Water for Reuse in South Africa. New Microbes and New Infections 38, 100803. doi:10.1016/j.nmni.2020.100803
Oliver, J. D., Dagher, M., and Linden, K. (2005). Induction of Escherichia coli and Salmonella typhimurium into the Viable but Nonculturable State Following Chlorination of Wastewater. J. Water Health 3, 249–257. doi:10.2166/wh.2005.040
Pignata, C., Fea, E., Rovere, R., Degan, R., Lorenzi, E., de Ceglia, M., et al. (2012). Chlorination in a Wastewater Treatment Plant: Acute Toxicity Effects of the Effluent and of the Recipient Water Body. Environ. Monit. Assess. 184, 2091–2103. doi:10.1007/s10661-011-2102-y
Ping, S., Mayorga-Reyes, N., Price, V. J., Onuoha, M., Bhardwaj, P., Rodrigues, M., et al. (2020). Characterization of Presumptive Vancomycin-Resistant Enterococci Recovered during Infection Control Surveillance in Dallas, Texas. Br. Med. J. 23, 1–21. doi:10.1101/2020.10.09.20209569
Puchter, L., Chaberny, I. F., Schwab, F., Vonberg, R.-P., Bange, F.-C., and Ebadi, E. (2018). Economic burden of Nosocomial Infections Caused by Vancomycin-Resistant Enterococci. Antimicrob. Resist. Infect. Control. 7, 1. doi:10.1186/s13756-017-0291-z
Radhouani, H., Silva, N., Poeta, P., Torres, C., Correia, S., and Igrejas, G. (2014). Potential Impact of Antimicrobial Resistance in Wildlife, Environment and Human Health. Front. Microbiol. 5, 23–12. doi:10.3389/fmicb.2014.00023
Reynolds, P. E., Snaith, H. A., Maguire, A. J., Dutka-Malen, S., and Courvalin, P. (1994). Analysis of Peptidoglycan Precursors in Vancomycin-Resistant Enterococcus Gallinarum BM4174. Biochem. J. 301 (1), 5–8. doi:10.1042/bj3010005
Rizzo, L., Belgiorno, V., and Napoli, R. M. A. (2004). Regrowth Evaluation of Coliform Bacteria Injured by Low Chlorine Doses Using Selective and Nonselective media. J. Environ. Sci. Health A 39 (8), 2081–2092. doi:10.1081/ese-120039376
Rizzo, L., Manaia, C., Merlin, C., Schwartz, T., Dagot, C., Ploy, M. C., et al. (2013). Urban Wastewater Treatment Plants as Hotspots for Antibiotic Resistant Bacteria and Genes Spread into the Environment: A Review. Sci. Total Environ. 447, 345–360. doi:10.1016/j.scitotenv.2013.01.032
Rosenberg Goldstein, R. E., Micallef, S. A., Gibbs, S. G., George, A., Claye, E., Sapkota, A., et al. (2014). Detection of Vancomycin-Resistant Enterococci (VRE) at Four U.S. Wastewater Treatment Plants that Provide Effluent for Reuse. Sci. Total Environ. 466-467, 404–411. doi:10.1016/j.scitotenv.2013.07.039
Sadowy, E., and Luczkiewicz, A. (2014). Drug-resistant and Hospital-Associated Enterococcus Faecium from Wastewater, Riverine Estuary and Anthropogenically Impacted marine Catchment basin. BMC Microbiol. 14 (66), 66–2180. doi:10.1186/1471-2180-14-66
Suresh, T., Srinivasan, D., Hatha, A. A. M., and Lakshmanaperumalsamy, P. (2000). The Incidence, Antibiotic Resistance and Survival of Salmonella and Escherichia coli Isolated from Broiler Chicken Retail Outlets. Microb. Environ. 15, 173–181. doi:10.1264/jsme2.2000.173
Talebi, M., Rahimi, F., Katouli, M., Möllby, R., and Pourshafie, M. R. (2008). Epidemiological Link between Wastewater and Human Vancomycin-Resistant Enterococcus Faecium Isolates. Curr. Microbiol. 56, 468–473. doi:10.1007/s00284-008-9113-0
U S Environmental Protection Agency (2006). Office of Water. Method 1106.1: Enterococci Inwater by Membrane Filtration Using Membrane-Enterococcus -Esculin Iron Agar (mEEIA). Washington D C.
Varela, A. R., Ferro, G., Vredenburg, J., Yanık, M., Vieira, L., Rizzo, L., et al. (2013). Vancomycin Resistant Enterococci: From the Hospital Effluent to the Urban Wastewater Treatment Plant. Sci. Total Environ. 450-451, 155–161. doi:10.1016/j.scitotenv.2013.02.015
Vu, J., and Carvalho, J. (2011). Enterococcus: Review of its Physiology, Pathogenesis, Diseases and the Challenges it Poses for Clinical Microbiology. Front. Biol. 6 (5), 357–366. doi:10.1007/s11515-011-1167-x
Wang, S., Guo, Y., Lv, J., Qi, X., Li, D., Chen, Z., et al. (2016). Characteristic of Enterococcus Faecium Clinical Isolates with Quinupristin/dalfopristin Resistance in China. BMC Microbiol. 16 (264), 246–255. doi:10.1186/s12866-016-0863-8
World Health Organization. (2017). Global Priority List of Antibiotic-Resistant Bacteria to Guide Research, Discovery, and Development of New Antibiotics. WHO-PPL-Short_Summary_25Feb-ET_NM_WHO.pdf
Keywords: vancomycin, wastewater reuse, enterococci, river water, antibiogram, van genes
Citation: Adegoke AA, Madu CE, Reddy P, Stenström TA and Okoh AI (2022) Prevalence of Vancomycin Resistant Enterococcus in Wastewater Treatment Plants and Their Recipients for Reuse Using PCR and MALDI-ToF MS. Front. Environ. Sci. 9:797992. doi: 10.3389/fenvs.2021.797992
Received: 19 October 2021; Accepted: 08 December 2021;
Published: 24 January 2022.
Edited by:
Khalid Muzamil Gani, National Institute of Technology, IndiaReviewed by:
Aalaa Samir Saad, Animal Health Research Institute, EgyptM. Jahangir Alam, University of Houston, United States
Copyright © 2022 Adegoke, Madu, Reddy, Stenström and Okoh. This is an open-access article distributed under the terms of the Creative Commons Attribution License (CC BY). The use, distribution or reproduction in other forums is permitted, provided the original author(s) and the copyright owner(s) are credited and that the original publication in this journal is cited, in accordance with accepted academic practice. No use, distribution or reproduction is permitted which does not comply with these terms.
*Correspondence: Anthony A. Adegoke, YW50aG9ueWFkZWdva2VAdW5pdXlvLmVkdS5uZw==, YWF5b2RlZ29rZUBnbWFpbC5jb20=