- 1Centre for Ecosystem Science, School of Biological, Environmental and Earth Sciences, UNSW, Sydney, NSW, Australia
- 2New South Wales Department of Planning, Industry and Environment, Parramatta, NSW, Australia
The Murray-Darling Basin (the Basin) is the largest river system in Australia, supplying about 40% of the country’s irrigated agricultural output. Associated water resource development has come with a heavy price for the Basin’s freshwater ecosystems degrading them over decades. Australian governments are attempting to achieve environmental sustainability by returning water to the environment through buy-back of irrigation licences and improved water efficiencies. To determine effectiveness, basin-wide management objectives were established for key indicators, including waterbird populations and life histories which can effectively indicate ecosystem function and condition, driven by flow and flooding regimes. Ongoing monitoring of waterbird numbers indicates continued declines. We evaluated the feasibility of meeting established waterbird objectives under existing and predicted climates. We modelled long-term waterbird numbers using one of the world’s largest ongoing waterbird surveys (1983–2020), covering about 13.5% of the area of the entire Basin. Our findings suggest that under near future climate change projections, waterbird numbers will likely continue to decline, and remain below restoration targets set for the Basin. We discuss the current policy settings for using environmental water to support waterbird populations, recommending adjustments to restore the Basin’s waterbird populations and their wetlands in order to meet Australia’s conservation targets in relation to the ongoing global crisis of biodiversity loss.
Introduction
Fresh water is a fundamental resource for life on Earth (Carpenter et al., 2011) including humanity’s dramatic development but also contributed to its demise when scarce (Diamond, 2011). Increased reliance on fresh water for food and other commodities has led to extensive modifications of the world’s freshwater ecosystems, transforming their physical, chemical, and biological character, often leading to loss of function and services (Junk et al., 2012), such as grazing, filtration, flood mitigation, and carbon storage (Keddy et al., 2009; Everard et al., 2019). Freshwater ecosystems are now among the most altered ecosystems on the planet (Nilsson et al., 2005; Grill et al., 2019), in many areas nearing their natural limits for human use and effectively exceeding their renewable supply (Millennium Ecosystem Assessment 2005). Climate change is already disrupting global water cycles (Jiménez Cisneros et al., 2014), further threatening regional water availability and global water security (Dai 2013; Masson-Delmotte et al., 2018). Current projections of climate change are predicted to increase the risk of wetland loss and continued biodiversity degradation (Kingsford, 2011; Xi et al., 2021). Understanding these effects on biodiversity and developing robust conservation strategies to mitigate the impacts of both water resource development and climate change are urgently required.
To address degradation of freshwater biodiversity and loss of ecosystem services, initiatives and policy frameworks are often multi scaled, developed and implemented from local, grass roots initiatives (Casagrande, 1997; Zaldívar-Jiménez et al., 2017), to regional (Giakoumi et al., 2013; Kark et al., 2015), and global such as the Convention on Biological Diversity (CBD) and the UN’s Sustainable Development Goals (SDGs; e.g., SDG 6 https://unric.org/en/sdg-6/). Regardless of the scale, conservation efforts are reliant on accurately assessing the condition of biodiversity (Keith et al., 2015), tracking changes over time (Mace and Baillie 2007; Matthews et al., 2009; Romañach et al., 2021), predicting future states (White et al., 2021), and progression towards restoration targets (Kentula, 2000; Ferraro and Pattanayak, 2006; Hobbs, 2007; Zogaris et al., 2017). These require a good understanding of the ecosystems in question, hinged on accurate conceptualization of their inherent processes and dynamics, as well as threats (Zedler, 2000; Kingsford and Biggs, 2012). Consequently, appropriate indicators able to represent critical components of ecosystem are monitored, and inform evaluation frameworks that, ideally, form part of an adaptive management framework (O’Donnell and Galat, 2008; Williams, 2011; Navarro et al., 2017). If indicators deviate from desired conditions or trajectories, corrective actions are required, either in the form of management actions or possibly, re-evaluating achievability of defined targets.
The Murray-Darling Basin is one of Australia’s larger river basins, spanning five states and the Australian Capital Territory, predominantly characterized ecologically by expansive inland, semi-arid floodplain wetlands (Sims et al., 2011; Brooks 2017). It is also one of Australia’s most important food bowls, providing annual agricultural produce valued at $24 billion (https://www.agriculture.gov.au/water/mdb), supported by extensive water resource infrastructure and dams that divert almost half of the Basin’s annual surface water for irrigated agriculture and other uses (Leblanc et al., 2012; Gawne et al., 2019). Water resource development has come at the expense of altering the natural flow regimes of the Basin’s rivers, in turn impacting its ecological integrity (Kingsford, 2000; Arthington and Pusey, 2003; Pittock and Finlayson, 2011; Sims et al., 2011; Gawne et al., 2019). Of the Basin’s 16 Ramsar-listed wetlands of international importance, three have been formally acknowledged by the Australian Government to fail in maintain their ecological character since listing, primarily a consequence of water resource development (Kirsch et al., 2021). In response to ongoing degradation, the Australian government enacted national legislation (Water Act 2007) to protect and restore the Basin’s freshwater ecosystems through water buy-backs and infrastructure at a cost exceeding $A13 billion (Swirepik et al., 2016; Wheeler et al., 2020). Most of these funds were focused on returning a target of 2,750 GL per year back to the rivers by buying back water from willing irrigators and improving efficiency of irrigation system to allow for more environmental water (Wheeler et al., 2020). The legislation established the Murray–Darling Basin Authority (MDBA) and development and implementation of a “Basin Plan”, aimed at sustainability for the Basin (Swirepik et al., 2016), establishing environmental objectives and an environmental watering strategy (2014). The Basin Plan established Sustainable Development Limits for each of the rivers but failed to consider possible policy and management responses to climate change (Pittock and Finlayson, 2011; Pittock and Hartmann, 2011). The Basin Plan also guides environmental water delivery to support key ecological indicators while monitoring and evaluating progress towards desired environmental objectives (floodplain vegetation, fish, and waterbirds). The Basin’s waterbird objectives focus on maintaining species’ diversity, improving breeding success, and achieving waterbird numbers, comparable to those recorded in the early 1990s (Bino et al., 2014a; MDBA, 2020). Waterbirds respond strongly to the condition of freshwater ecosystems (Bino et al., 2015; Kingsford et al., 2017), influenced by availability and accessibility of habitat, resources, and breeding sites (Green and Robins, 1993; Clausen, 2000; Guillemain et al., 2000; Kingsford et al., 2010) which determine community composition, abundances, and breeding (Kingsford and Auld, 2005; Brandis et al., 2011; Arthur et al., 2012).
In this paper, we evaluated waterbird responses across the Basin, examining total abundances, abundances of five functional response groups (ducks, herbivores, large wading birds, piscivores, and shorebirds), and the number of breeding waterbirds. We utilize the longest running annual aerial survey of waterbirds in Australia (1983–2020) and assess historic numbers while also considering the dynamics of hydrological condition. We modelled annual waterbirds in relation to water availability and predicted responses under varying flow management and climate change scenarios to evaluate the likelihood of meeting the Basin’s waterbird restoration targets under current and projected future climates.
Methods
Study Areas
We studied waterbird responses in the Murray-Darling Basin (the Basin), one of Australia’s larger river basins, spanning five states and the Australian Capital Territories, covering approximate one million km2, Figure 1). The Basin encompasses a wide range of climatic conditions, receiving between 2,100 mm of annual rainfall in the highland areas in the southeast to 300 mm in the semi-arid areas in the west (https://www.mdba.gov.au). The Basin has a mapped wetland area of approximately 55,000 km2, predominantly palustrine (Bino et al., 2016).
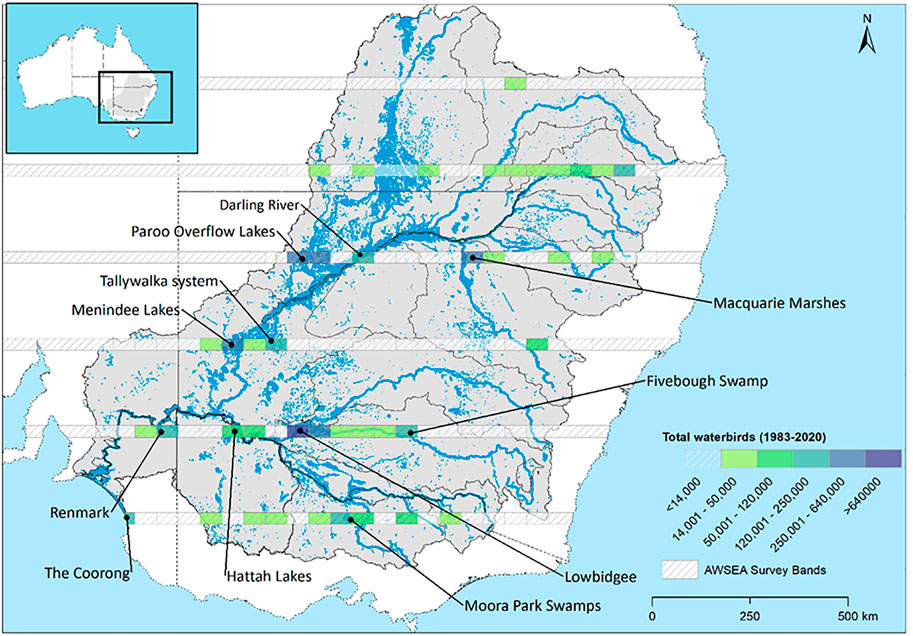
FIGURE 1. The Murray–Darling Basin, Water Resource Planning Areas (outlines, Supplementary Appendix S1 for labels), six of ten survey bands of the Aerial Survey of Waterbirds in Eastern Australia intersecting with the Basin (hatched and shaded summary of totals 1983–2020 as 30 × 50 km grid cells), and labelled wetland complexes supporting high waterbird abundance (1983–2020) which intersected with the survey bands.
Waterbird Surveys
We used waterbird counts collected as part of the Aerial Survey of Waterbirds in Eastern Australia (1983–2020) (Kingsford et al., 2020). Surveys were done annually (October) across ten systematic survey bands spanning eastern Australia, each separated by about 200 km. For most surveys, the high-winged aircraft (e.g., Cessna 206 or 208) was flown at a height of 30–46 m and a speed of 167–204 km/h (90–110 knots), within 150 m of the shoreline, where waterbirds usually congregate and two observers counted on either side to provide a total or proportion count (Kingsford and Porter, 1994; Kingsford, 1999; Kingsford et al., 2020). For some wetlands transects were used and a 200 m wide strip (100 m each side of the aircraft) delineated across the wetland, as the high–winged aircraft. Each wetland surveyed was given a unique code so that survey records could be matched between the two observers in each aircraft. The two counts of observers were then added together to form a combined count for a particular wetland. Each wetland was surveyed for the full complement of waterbirds, including cormorants, grebes, herons, egrets, ibis, waterfowls, and wading birds. More than 50 taxa have been identified over the years, mostly to species level although grebes, egrets, and waders have been grouped because of difficulties in differentiation (Kingsford et al., 2020). Waterbirds and nesting activity were identified, and their numbers estimated and immediately recorded. In this study, we included the seven surveys bands which cover the Murray–Darling Basin, about 13.5% of its land surface area (1,061,469 km2, Bino et al., 2016). We evaluated seven waterbird metrics: 1) total annual abundance; 2–6) total annual abundance for each of the five functional response groups (ducks, waders, shorebirds, piscivores, herbivores) and; 7) total annual abundance of breeding waterbirds.
We classified water availability in years, corresponding with the waterbird surveys (1983–2020) into five Surface Water Conditions (SWC): Very Dry, Dry, Moderate, Wet, and Very Wet. To do this, we identified 18 flow gauges across the Basin’s main rivers, and collated daily flow volume data from state data servers (Supplementary Appendix S2) between 1980 and 2020. We then calculated the annual (water year: October–September) cumulative flow volumes. For a Basin wide SWC index, we calculated the standardized average annual flow volume across all 18 gauges and classified each year into five quantiles. Using the annual percentile each year and the those in the previous year, we designated the SWC (Supplementary Appendix S3). Following a similar process, we also classified SWCs for each of the Basin’s Water Resource Planning Areas (WRPAs) using the corresponding river gauges (Supplementary Appendix S2).
Modelling Waterbird Responses
To predict waterbird responses under a range of flow management and climate change scenarios (1900–2008, see details below), we modelled annual waterbird responses to water availability. We calculated the 3, 6, 12, 24-months total discharge recorded in the months prior to aerial surveys (October) across the Basin (Supplementary Appendix S2 for details on gauges). Likewise, we calculated the 3, 6, 12, 24-months total rainfall recorded in the months prior to aerial surveys (October) across the Basin, as well as annual rainfall across northern, southern, and eastern Australia (Supplementary Appendix S2 for details on gauges). We also considered the annual (water year) monthly average Southern Oscillation Indices, given El Nino and La Nina cycles associate with major periods of flooding and drying in Australia (Puckridge et al., 2000). We modelled seven waterbird responses: 1) total annual abundance, 2–6) total annual abundance for each of the five functional response groups (ducks, waders, shorebirds, piscivores, herbivores), and 7) total annual abundance of breeding waterbirds. We log-transformed all response variables prior to analysis to meet Gaussian model requirements (Supplementary Appendix S2).
We used two distinct approaches to modelling waterbird responses. The first was Generalized Linear Models (GLMs) using a Bayesian Model Averaging (BMA), which enabled combining predictions from several candidate models, while accounting for model uncertainty. BMA combines the predictions of a large sample of possible models using a weighted averaging algorithm based on Bayes’ theorem, with weights proportional to the posterior probability representing the relative strength of evidence in favour of each model (Raftery, 1995; Wintle et al., 2003). The BMA process assumes that all considered models partially explain the data, but only a subset chosen on the basis of the Bayes information criterion (BIC), are combined to improve the overall predictive power of the final model (Hoeting et al., 1999). The final model incorporated the covariates and their averaged coefficients weighted according to the BIC scores. We implemented this using the BAS package (Clyde et al., 2011) within the R statistical software. The second approach was Random Forests (Cutler et al., 2007), which employs a classification algorithm to identify binary partitioning in the response data, which minimises variance using the best predictor variable. The partitioning continues until further subdivision no longer improve model performance (using the Gini index). The Random Forest algorithm uses bootstrapping to sample the data (∼63% of the data) and fits multiple trees on each subset. Accuracy and error rates are calculated for each tree using the reminder data (∼37), out-of-bag observations and predictor variable importance determined. We implemented this using the randomForest package (Liaw and Wiener 2002) within the R statistical software (R Core Team 2018).
We used predicted flow scenarios to assess the benefits and impacts to waterbirds under varying management and climate scenarios (MDBA, 2011b). We used flow scenarios developed by CSIRO which have provided a modelling framework for developing the Basin’s Sustainable Yields project (CSIRO, 2008; Podger et al., 2010; MDBA, 2011a). Flow scenarios provided predicted flow discharge rages for 1902–2008 (MDBA, 2010). We considered two foundation model scenarios which provide historical and contemporary estimates of the Basin’s water resources: without water resource development (R844) and baseline (pre-Basin Plan) with historical climate (R871). The without-development scenario (R844) represents the Basin as a natural system with no development or associated consumptive use while the baseline scenario (R871) includes water use in 2009, prior to establishing the Basin Plan. Differences between the two provided an estimate on the effects of development on the natural flow regime. We also considered flows scenarios developed for identifying environmentally sustainable level of consumptive use which included reduced annual diversions of 2,750 GL (R971) and 3,200 GL (R862). We also examined the impacts of climate change (1°C increase) on the baseline models under a dry (R893), medium (R892) and wet (R894) near future climate for the year 2030 (Chiew et al., 2010). We then predicted waterbird abundances under each flow scenario between 1900 and 2008 and summarized results by SWC. Flow scenarios considering higher global warming were not available. In addition, a flow scenario which incorporated both reduced diversions and climate change does not exist. Given the highly regulated rivers in the Basin, water available for waterbirds cannot be effectively predicted using rainfall alone (Kingsford et al., 2017).
Results
Total waterbird numbers have declined at an annual rate of 4.8% across the Basin since the Eastern Australia Waterbird Surveys (EAWS) began in 1983, (Figure 2). During this time, Surface Water Conditions (SWC) have steadily declined across the Basin, with very low water availability since 2002, apart for 2010–2013 (Figure 2). Since 1983, the Basin has experienced similar frequencies of annual SWC, with most of the “very dry” and “dry” years occurring since 2000, corresponding with the Millennium (1997–2009) and the 2017–2019 droughts (http://www.bom.gov.au/climate/drought/knowledge-centre/previous-droughts.shtml). Annual waterbird abundances corresponded with SWC (Table 1; Figure 3), with low numbers observed during dry conditions. During “very dry” conditions, total waterbird abundances across the Basin averaged 63,546 ± 30,441SD, compared to an average of 138,115 ± 74,606SD under “moderate” conditions, and an average of 298,240 ± 208,494SD under “very wet” conditions (Table 1; Figure 3; Supplementary Appendix S3). Four of the five waterbird functional response groups were responsive to SWC, most notable for ducks, herbivores, and piscivores (Table 1; Figure 3). Large waders and total breeding (mostly consistent of large wading birds) also responded in “dry” and “moderate” SWCs, reflective of strong responses in 2010 following a break in a decade long drought (Millennium Drought) as well as in 2016. Shorebird abundance did not fluctuate significantly by SWC.

TABLE 1. Average annual water bird abundance (Nos +/− SD) from the Eastern Australia Waterbird Survey (EAWS) across the Murray-Darling Basin (1983–2020), stratified by five Surface Water Conditions (SWC) and separated into total numbers, the five functional response groups (Du-ducks; He-herbivores, La-large waders, Pi-piscivores, Sh-shorebirds) and total waterbird breeding.
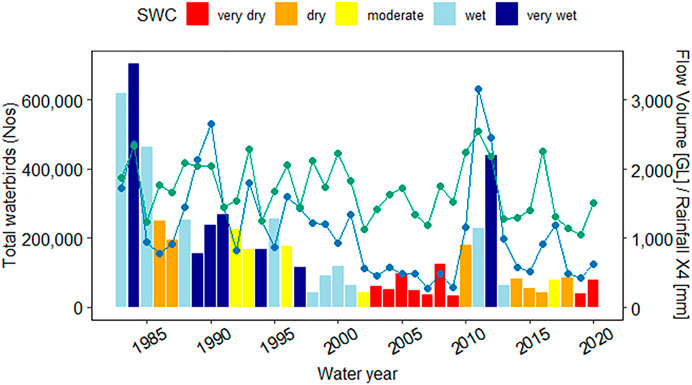
FIGURE 2. Annual (1983–2020) waterbird abundance (columns, coloured by SWC) and average flow volume (blue line and points) and rainfall (green line and points) across the Murray–Darling Basin (Supplementary Appendix S2 for gauge information).
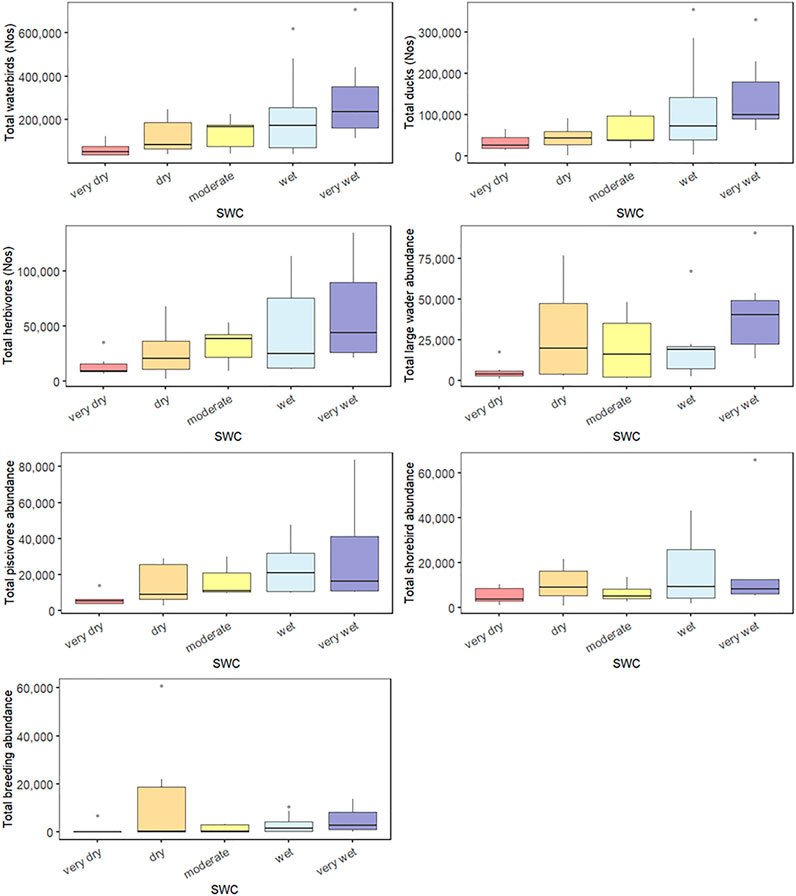
FIGURE 3. Box plots of annual (1983–2020) waterbird abundance (total, five functional response groups, and total breeding) from the Eastern Australia Waterbird Surveys across the Murray-Darling Basin by five Surface Water Conditions (SWC).
Four Water Resource Planning Areas (WRPAs) (Figure 1) represented the largest proportions of surveyed waterbird abundances: the Murrumbidgee (annual average proportion 0.33 ± 0.22SD), Intersecting Streams (p = 0.17 ± 0.17SD), NSW Murray and Lower Darling (0.11 ± 0.11SD), Northern Victoria (0.11 ± 0.11SD), as well as the Macquarie-Castlereagh (0.06 ± 0.04SD) and South Australian Murray Region (0.06 ± 0.09SD) (Figure 4). Under “very dry” and “dry” SWCs, the Murrumbidgee and Intersecting Streams supported the largest number of waterbirds, while under “moderate” SWC the Murrumbidgee, NSW Murray and Lower Darling, Macquarie-Castlereagh, and Intersecting Streams supported the larger proportion of waterbirds. Under “wet” and “very wet” SWCs the largest proportion of total waterbirds were surveyed in the Murrumbidgee, Intersecting Streams, and NSW Murray and Lower Darling (Figure 4).
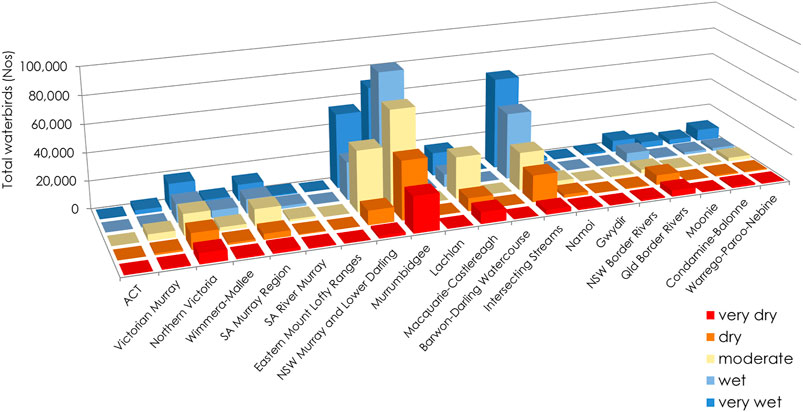
FIGURE 4. Average annual (1983–2020) waterbird abundance from the Eastern Australia Waterbird Survey in each of the Water Resource Planning Area across the Murray-Darling Basin (Supplementary Appendix S1 for map with labels), stratified by five Surface Water Conditions (SWC).
Models of total annual waterbird abundances, when using GLMs, were principally driven by cumulative annual (water year) flow volumes (Marginal Posterior Inclusion Probability of p = 0.88) and annual rainfall in south-eastern Australia (p = 0.13) (Supplementary Appendix S4). Model fit for total waterbirds was moderate with pseudo-R2 of 0.5. When using Random Forests, annual rainfall in south-eastern Australia and cumulative annual and 3-months flow volumes were the chief predictors (Supplementary Appendix S4), with an improved model fit of pseudo-R2 of 0.88. Although modelled waterbird responses, using a classification tree approach offered better fit to observed data, they are known to fail predicting responses which are outside the range of observed values (1983–2020), thus likely underestimated waterbird numbers under the unregulated flow scenario which would have supported greater numbers compared to those observed since surveys began in 1983.
Models predicted a current average total abundance between 163,798 (GLM: 90% CI: 81,712–274,403) and 152,836 (RF 90% CI: 76,236–241,265) under the post–Basin Plan scenario with 2,750 GL reduction in water abstraction (R971, Figure 5). This represented a decline between 26% (GLM) and 16% (RF) over the without development scenario (R844) which predicted an annual average between 220,051 (GLM 90% CI 113,636–340,651) and 180,958 (RF 90% CI 104,493–256,095). The post–Basin Plan flow scenario (R971) predicted an increase between 12% (GLM) to 9% (RF) over the pre-Basin Plan (R871), predicted to support an annual average between 145,618 (GLM 90% CI 71,137–252,559) and 139,778 (RF 90% CI 66,345–232,648). Under a projected dry climate change scenario (R893), waterbird abundances were significantly lower, predicting a decline between 32% (GLM) and 21% (RF) compared to pre-Basin Plan with historical climate (R871, Figure 5). Under a median climate change scenario (R892), declines were predicted to be between 12% (GLM) and 6% (RF), while under a wet climate change scenario (R894), annual average waterbird abundances were predicted to increase between 13% (GLM) to 7% (RF) (Figure 5). Detailed predicted responses are summarized in Supplementary Appendix S5.
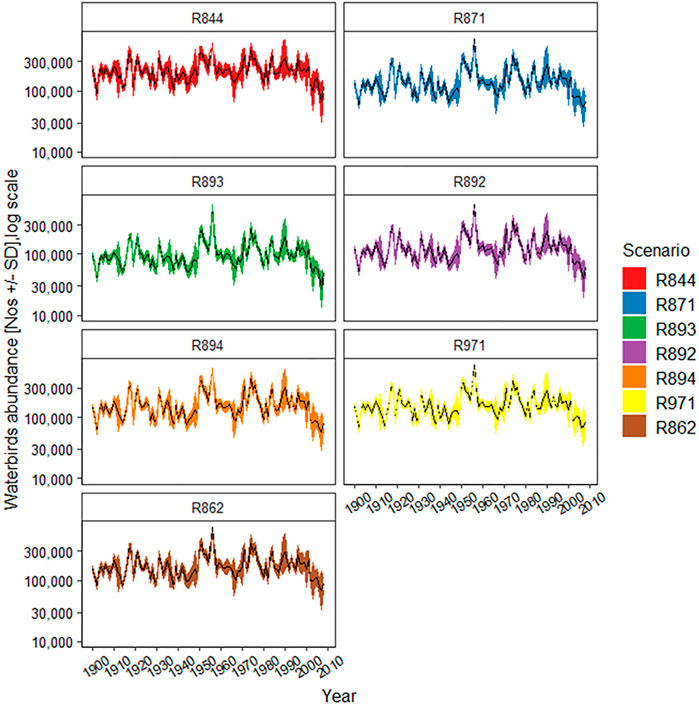
FIGURE 5. Predicted annual waterbird abundances based on GLMs (see Random Forest predictions in Supplementary Appendix S5) under the seven management/flow Scenarios in the Murray-Darling Basin 1900–2008 (MDBA, 2011b): without water resource development (R844), pre-Basin Plan with historical climate (R871), without-development scenario (R844), reduced annual diversions of 2,750 GL (R971) and 3,200 GL (R862), and climate change 1°C increase on the R871 under a dry (R893), medium (R892) and wet (R894) near future climate for the year 2030 (Chiew et al., 2010).
Discussion
The world’s freshwater biodiversity is in decline, predominantly from the impacts of water resource developments, including dams, diversions of water and development of floodplains and wetlands (Junk et al., 2012; Kingsford et al., 2016). The increasing effects of climate change, particularly reductions in rainfall and run-off and increased evaporation, are further decreasing flow and flooding regimes and contributing to biodiversity loss (Dai, 2013; Xi et al., 2021). Since last evaluated (1983–2014) (Kingsford et al., 2017), long-term declines in waterbirds have continued, mainly from river regulation effects but also exacerbated by dry conditions, likely associated with a changing climate. In this paper we expand on (Kingsford et al., 2017) and evaluated the continued impacts of river regulation and climate change on waterbirds in Australia’s most developed river basin, the Murray-Darling Basin. We also improved utility for setting restoration targets which are tailored to water availability conditions (Surface Water Conditions) at multiple scales (Basin, Water Resource Planning Areas, wetlands).
The restoration plan for the Murray-Darling Basin (MDBA, 2012), aims to restore the ecological integrity and function of its many degrading freshwater ecosystems. The chief tool for restoring the system was setting sustainable diversion limits to restrict water use and securing water for the environment. Setting diversion thresholds are reliant on data, particularly useful if it extends prior to degradation (Jackson et al., 2001), rarely the case prior to regulation of the Basin’s freshwater ecosystems (Kingsford et al., 2015). In their absence, models able to predict responses under varying conditions can provide useful tools for setting management targets. In the Basin, environmental objectives have been identified for four key components: river flows and connectivity, native vegetation, waterbirds, and fish (MDBA, 2020). In the case of waterbirds, models (Bino et al., 2014a; MDBA, 2020) were used to set restoration targets to be achieved from 2024: 1. Increase abundance by 20–25%; 2. Increase frequency of breeding events by 50% and breeding abundances by 30–40%; and 3. maintain species’ diversity across the Basin and those of migratory species in the Coorong (MDBA, 2020). Waterbird numbers have already declined by more than 70% since 1983 (Kingsford et al., 2017). Using available flow scenarios, we predicted that the Basin would support 25% less waterbirds compared to pre-regulation flows with the current extent of regulation, though this analysis is limited by waterbird responses since 1983, long after extensive water resource development (e.g., dams), (Kingsford et al., 2011), occurred in the Basin. This limit predicting waterbird responses, particularly when using classification trees, which have likely been significantly larger than those recorded in the early 1980’s.
The high dependency of waterbirds on river flows and flooding regimes and projected drying under climate change will continue to impact river flows and dependent waterbirds. Climate change in the next decade (2030) were projected to further decrease the flows and consequently waterbird populations by ∼30–20%, compared to the pre-Basin Plan period. Current restoration efforts, primarily by allocating water for the environment, are predicted to support an increase of ∼12% in waterbirds numbers over the pre-Basin Plan but will only halve the projected impacts due to climate change. This translates to a net loss of ∼20–10% in waterbird numbers over the pre-Basin Plan period. Under a less likely median climatic near future (2030), environmental flow restoration may offset projected declines, but will still be lower than intended restoration targets for the waterbirds of the Murray-Darling Basin. Only under an unlikely wet climatic near future could restoration objectives for waterbird be attained. The lack of available flow scenarios which combine climate change as well as reduced diversions are in development, but their absence increases uncertainty in predicted waterbird responses in the next decade. Such uncertainty stalls developing better predictions and appropriate and timely management and policy actions.
Climate change models used in these analyses were based on a 1°C warming. Already, mean annual temperatures in the Basin have risen by 1.4°C and are expected to continue to rise (Commonwealth of Australia 2020). Unfortunately, flow scenarios considering higher global warming were not available. Current climate change median projections predict a reduction of ∼20% in stream flows in the Basin under a likely 2°C warming, increasing the frequency of severe droughts (Chiew et al., 2009; Zheng et al., 2019). The realized impacts of climate change on stream flows will likely be exacerbated because of increased demand for fresh water, increased capture of water during extreme droughts in farm dams, outside of existing entitlements (Bond et al., 2008; Fowler et al., 2015; Matthews 2017). Already signs of lower-than-expected recovery of the Basin’s southern watersheds from past drought are indicative of failing resilience (Peterson et al., 2021). The impacts of climate change to the environment are predicted to be even greater under the current water sharing rules, as allocations of water to licenced users are adjusted annually based on water availability leading to water for the environment being reduced by a greater proportion than water for consumptive use during dry conditions (Prosser et al., 2021).
Climate change projections do not bode well for humanity as for the freshwater ecosystems of the Murray-Darling Basin. Degradation, already apparent on account of water resource development (Thoms and Sheldon, 2000; Leigh and Sheldon, 2008; Shaeri Karimi et al., 2021), has undermined the resilience of freshwater ecosystems to cope with extreme droughts (Colloff and Baldwin 2010; Sandi et al., 2020). Improving the likelihood of meeting environmental restoration targets, under a likely drying future requires substantial and urgent transformative strategies (Smith et al., 2011) for the upcoming review of the Basin Plan in 2026 (Gawne et al., 2021). The Basin’s waterbirds are indicators of the freshwater ecosystems and leave two options to consider in order to meet restoration and avoid possible collapse: 1) Increase water allocations for the environment and improve management effectiveness tailored to waterbird life histories such as opportunities for breeding and improved habitat quality or 2) reduce restoration targets for waterbirds for the Basin-wide environmental watering strategy set to be reviewed in 2022 while observing ongoing declines. For the former, significant social-economic barriers exist impeding future increases in water allocations for the environment (Bark et al., 2014; Colloff and Pittock, 2019). Improving awareness of the critical links between ecological integrity and societal well-being for the Basin’s communities and fostering a shared vision of a sustainable future are important. Alternative management strategies that may improve waterbird outcomes also exist. For example, changing water management rules by allowing increased storage of environmental flows in dams for supporting larger flooding could improve waterbird breeding outcomes (Kingsford and Auld, 2005; Bino et al., 2014b; Chen et al., 2020). Should the latter be preferred, quantifying waterbird numbers under different water availability conditions such as presented here (i.e., Surface Water Conditions) at multiple scales (Basin, Water Resource Planning Areas, wetlands) provides a framework of setting more plausible waterbird targets according to conditions. Uncertainty still exists in response models. In this study we used two very different modelling approaches. Exploring alternative modelling approaches is useful to evaluating uncertainties originating from model assumptions (Araújo and New, 2007). Although the decision tree approach offered better fit to observed data, such models can fail when attempting to predict beyond the range of available data. As such, predicted response prior to water resource development in the Basin using decision tree models are likely an underestimation. Decision trees predicted somewhat lower impacts of climate change compared to GLMs, offering perhaps some hope that declines might be less severe, but nonetheless still foreshadow a degradation of waterbirds populations. Developing models based on biological processes (Tonkin et al., 2019) of specific waterbird species can also improve quantifying requirements for viability and restoration (Brandis et al., 2021), but require good knowledge of life histories of waterbird species, including regional and continental interactions of waterbirds across wetlands (Bino et al., 2020). There is also urgent need to expand flow scenarios under a wider range of probable climate change projections and longer foresight (Prosser et al., 2021), improved interlinks between rivers with floodplains (Whipple and Viers 2019), and integrate future demand for freshwater (Loch et al., 2020). Regardless, developing more realistic and sustainable socio-ecological adaptations for the future of the Murray-Darling Basin are sorely needed if we are to avoid collapse of ecosystems.
Data Availability Statement
The datasets presented in this study can be found in online through: https://www.ecosystem.unsw.edu.au/research-projects/rivers-and-wetlands/waterbirds/eastern-australian-waterbird-survey.
Author Contributions
RK and JP collected the data, GB conceptualized the research and analysed the data, GB led the writing and all authors contributed to writing of the manuscript and gave final approval for publication.
Funding
This study was funded by ARC Linkage LP180100159 and partially by the Murray-Darling Basin Authority (MD5659).
Conflict of Interest
The authors declare that the research was conducted in the absence of any commercial or financial relationships that could be construed as a potential conflict of interest.
Publisher’s Note
All claims expressed in this article are solely those of the authors and do not necessarily represent those of their affiliated organizations, or those of the publisher, the editors and the reviewers. Any product that may be evaluated in this article, or claim that may be made by its manufacturer, is not guaranteed or endorsed by the publisher.
Supplementary Material
The Supplementary Material for this article can be found online at: https://www.frontiersin.org/articles/10.3389/fenvs.2021.785903/full#supplementary-material
References
Araújo, M. B., and New, M.(2007) Ensemble Forecasting of Species Distributions. Trends Ecol. Evol. 22 (4356), 42–47.
Arthington, A. H., and Pusey, B. J. (2003). Flow Restoration and protection in Australian Rivers. River Res. Applic. 19, 377–395. doi:10.1002/rra.745
Arthur, A. D., Reid, J. R. W., Kingsford, R. T., McGinness, H. M., Ward, K. A., and Harper, M. J. (2012). Breeding Flow Thresholds of Colonial Breeding Waterbirds in the Murray-Darling Basin, Australia. Wetlands 32, 257–265. doi:10.1007/s13157-011-0235-y
Bark, R., Kirby, M., Connor, J. D., and Crossman, N. D. (2014). Water Allocation Reform to Meet Environmental Uses while Sustaining Irrigation: a Case Study of the Murray-Darling Basin, Australia. Water Policy 16, 739–754. doi:10.2166/wp.2014.128
Bino, G., Brandis, K., Kingsford, R. T., and Porter, J. (2020). Waterbird Synchrony across Australia's Highly Variable Dryland Rivers - Risks and Opportunities for Conservation. Biol. Conservation 243, 108497. doi:10.1016/j.biocon.2020.108497
Bino, G., Kingsford, R. T., and Brandis, K. (2016). Australia's Wetlands - Learning from the Past to Manage for the Future. Pac. Conserv. Biol. 22, 116–129. doi:10.1071/pc15047
Bino, G., Kingsford, R. T., Brandis, K., and Porter, J. (2014a). Setting Waterbird Objective and Priorities for the Basin-wide Environmental Watering Strategy. Canberra, Australia: Murray-Darling Basin Authority.
Bino, G., Kingsford, R. T., and Porter, J. (2015). Prioritizing Wetlands for Waterbirds in a Boom and Bust System: Waterbird Refugia and Breeding in the Murray-Darling Basin. PloS one 10, e0132682. doi:10.1371/journal.pone.0132682
Bino, G., Steinfeld, C., and Kingsford, R. T. (2014b). Maximizing Colonial Waterbirds' Breeding Events Using Identified Ecological Thresholds and Environmental Flow Management. Ecol. Appl. 24, 142–157. doi:10.1890/13-0202.1
Bond, N. R., Lake, P. S., and Arthington, A. H. (2008). The Impacts of Drought on Freshwater Ecosystems: an Australian Perspective. Hydrobiologia 600, 3–16. doi:10.1007/s10750-008-9326-z
Brandis, K. J, Bino, G., and Kingsford, R. T (2021). More Than Just a Trend: Integrating Population Viability Models to Improve Conservation Management of Colonial Waterbirds. Environ. Manage. 68, 468–476. doi:10.1007/s00267-021-01507-5
Brandis, K. J., Kingsford, R. T., Ren, S., and Ramp, D. (2011). Crisis Water Management and Ibis Breeding at Narran Lakes in Arid Australia. Environ. Manage. 48, 489–498. doi:10.1007/s00267-011-9705-5
Brooks, S. (2017). Classification of Aquatic Ecosystems in the Murray-Darling Basin: 2017 Update. Canberra, Australia: Department of the Environment and Energy. Available at: https://data.gov.au/data/dataset/classification-of-aquatic-ecosystems-in-the-murray-darling-basin-2017-update.
Carpenter, S. R., Stanley, E. H., and Vander Zanden, M. J. (2011). State of the World's Freshwater Ecosystems: Physical, Chemical, and Biological Changes. Annu. Rev. Environ. Resour. 36, 75–99. doi:10.1146/annurev-environ-021810-094524
Casagrande, D. G. (1997). Restoration of an Urban Salt Marsh: An Interdisciplinary Approach. New Haven, Connecticut: Yale School of Forestry & Environmental Studies Bulletin Series, 100.
Chen, Y., Colloff, M. J., Lukasiewicz, A., and Pittock, J. (2020). A Trickle, Not a Flood: Environmental Watering in the Murray–Darling Basin, Australia. Mar. Freshw. Res. 72, 601–619. doi:10.1071/MF20172
Chiew, F. H. S., Kirono, D. G. C., Kent, D. M., Frost, A. J., Charles, S. P., Timbal, B., et al. (2010). Comparison of Runoff Modelled Using Rainfall from Different Downscaling Methods for Historical and Future Climates. J. Hydrol. 387, 10–23. doi:10.1016/j.jhydrol.2010.03.025
Chiew, F. H. S., Teng, J., Vaze, J., Post, D., Perraud, J., Kirono, D., et al. (2009). Estimating Climate Change Impact on Runoff across Southeast Australia: Method, Results, and Implications of the Modeling Method. Water Resour. Res. 45, 1–17. doi:10.1029/2008wr007338
Clausen, P. (2000). Modelling Water Level Influence on Habitat Choice and Food Availability for Zostera Feeding Brent Geese Branta bernicla in Non-tidal Areas. Wildl. Biol. 6, 75–87. doi:10.2981/wlb.2000.003
Clyde, M. A., Ghosh, J., and Littman, M. L. (2011). Bayesian Adaptive Sampling for Variable Selection and Model Averaging. J. Comput. Graphical Stat. 20, 80–101. doi:10.1198/jcgs.2010.09049
Colloff, M. J., and Baldwin, D. S. (2010). Resilience of Floodplain Ecosystems in a Semi-arid Environment. Rangel. J. 32, 305–314. doi:10.1071/rj10015
Colloff, M. J., and Pittock, J. (2019). Why We Disagree about the Murray-Darling Basin Plan: Water Reform, Environmental Knowledge and the Science-Policy Decision Context. Australas. J. Water Resour. 23, 88–98. doi:10.1080/13241583.2019.1664878
CSIRO (2008). Water Availability in the Murray‐Darling Basin, A Report to the Australian Government from the CSIRO Murray‐Darling Basin Sustainable Yields Projec. Australia: Commonwealth Scientific and Industrial Research Organisation.
Cutler, D. R., Edwards, T. C., Beard, K. H., Cutler, A., Hess, K. T., Gibson, J., et al. (2007). Random Forests for Classification in Ecology. Ecology 88, 2783–2792. doi:10.1890/07-0539.1
Dai, A. (2013). Increasing Drought under Global Warming in Observations and Models. Nat. Clim Change 3, 52–58. doi:10.1038/nclimate1633
Everard, M., Kangabam, R., Tiwari, M. K., McInnes, R., Kumar, R., Talukdar, G. H., et al. (2019). Ecosystem Service Assessment of Selected Wetlands of Kolkata and the Indian Gangetic Delta: Multi-Beneficial Systems under Differentiated Management Stress. Wetlands Ecol. Manage. 27, 405–426. doi:10.1007/s11273-019-09668-1
Ferraro, P. J., and Pattanayak, S. K. (2006). Money for Nothing? A Call for Empirical Evaluation of Biodiversity Conservation Investments. Plos Biol. 4, e105. doi:10.1371/journal.pbio.0040105
Fowler, K., Morden, R., Lowe, L., and Nathan, R. (2015). Advances in Assessing the Impact of hillside Farm Dams on Streamflow. Australas. J. Water Resour. 19, 96–108. doi:10.1080/13241583.2015.1116182
Gawne, B., Hale, J., Stewardson, M. J., Webb, J. A., Ryder, D. S., Brooks, S. S., et al. (2019). Monitoring of Environmental Flow Outcomes in a Large River basin: The Commonwealth Environmental Water Holder's Long‐term Intervention in the Murray-Darling Basin, Australia. River Res. Applic 36, 630–644. doi:10.1002/rra.3504
Gawne, B., Ryan, K. A., Coleman, M., Meehan, A., Davies, P. E., Sluggett, A., et al. (2021). Monitoring, Evaluation, and Adaptive Management in the Murray-Darling Basin. Australia: Murray-Darling Basin, 227–249. doi:10.1016/b978-0-12-818152-2.00011-5
Giakoumi, S., Sini, M., Gerovasileiou, V., Mazor, T., Beher, J., Possingham, H. P., et al. (2013). Ecoregion-based Conservation Planning in the Mediterranean: Dealing with Large-Scale Heterogeneity. PloS one 8, e76449. doi:10.1371/journal.pone.0076449
Green, R. E., and Robins, M. (1993). The Decline of the Ornithological Importance of the Somerset Levels and Moors, England and Changes in the Management of Water Levels. Biol. Conservation 66, 95–106. doi:10.1016/0006-3207(93)90140-v
Grill, G., Lehner, B., Thieme, M., Geenen, B., Tickner, D., Antonelli, F., et al. (2019). Mapping the World's Free-Flowing Rivers. Nature 569, 215–221. doi:10.1038/s41586-019-1111-9
Guillemain, M., Fritz, H., Guillon, N., and Guillon, N. (2000). Foraging Behavior and Habitat Choice of Wintering Northern Shoveler in a Major Wintering Quarter in France. Waterbirds: Int. J. Waterbird Biol. 23, 353–363. doi:10.2307/1522173
Hobbs, R. J. (2007). Setting Effective and Realistic Restoration Goals: Key Directions for Research. Restor Ecol. 15, 354–357. doi:10.1111/j.1526-100x.2007.00225.x
Hoeting, J. A., Madigan, D., Raftery, A. E., and Volinsky, C. T. (1999). Bayesian Model Averaging: a Tutorial. Stat. Sci. 14 (4), 382–401.
Jackson, J. B. C., Kirby, M. X., Berger, W. H., Bjorndal, K. A., Botsford, L. W., Bourque, B. J., et al. (2001). Historical Overfishing and the Recent Collapse of Coastal Ecosystems. Science 293, 629–637. doi:10.1126/science.1059199
Jiménez Cisneros, B. E., Oki, T., Arnell, N. W., Benito, G., Cogley, J. G., Döll, P., et al. (2014) “Freshwater resources. ,” in Climate Change 2014: Impacts, Adaptation, and Vulnerability. Part A: Global and Sectoral Aspects. Contribution of Working Group II to the Fifth Assessment Report of the Intergovernmental Panel on Climate Change. 1st Edn, Editor C. Field, V. Barros, D. Dokken, and K. Mach (Cambridge, UK: Cambridge University Press), Chap. 3, 229–269.
Junk, W. J., An, S., Finlayson, C., Gopal, B., Květ, J., Mitchell, S. A., et al. (2012). Current State of Knowledge Regarding the World’s Wetlands and Their Future under Global Climate Change: A Synthesis. Aquat. Sci. 75 (1), 151–167. doi:10.1007/s00027-012-0278-z
Kark, S., Tulloch, A., Gordon, A., Mazor, T., Bunnefeld, N., and Levin, N. (2015). Cross-boundary Collaboration: Key to the Conservation Puzzle. Curr. Opin. Environ. sustainability 12, 12–24. doi:10.1016/j.cosust.2014.08.005
Keddy, P. A., Fraser, L. H., Solomeshch, A. I., Junk, W. J., Campbell, D. R., Arroyo, M. T. K., et al. (2009). Wet and Wonderful: The World's Largest Wetlands Are Conservation Priorities. BioScience 59, 39–51. doi:10.1525/bio.2009.59.1.8
Keith, D. A., Rodríguez, J. P., Brooks, T. M., Burgman, M. A., Barrow, E. G., Bland, L., et al. (2015). The IUCN Red List of Ecosystems: Motivations, Challenges, and Applications. Conservation Lett. 8, 214–226. doi:10.1111/conl.12167
Kentula, M. E. (2000). Perspectives on Setting success Criteria for Wetland Restoration. Ecol. Eng. 15, 199–209. doi:10.1016/s0925-8574(00)00076-8
Kingsford, R., and Biggs, H. (2012). Strategic Adaptive Management Guideline for Effective Conservation of Freshwater Ecosystems in and Around Protected Areas of the World. Sydney: IUCN WCPA Freshwater Taskforce, Australian Wetlands, Rivers, and Landscapes Centre. Available at: http://data.iucn.org/dbtw-wpd/edocs/2012-017.pdf.
Kingsford, R. T., Porter, J. L., Brandis, K. J., and Ryall, S. (2020). Aerial Surveys of Waterbirds in Australia. Sci. Data 7, 172–176. doi:10.1038/s41597-020-0512-9
Kingsford, R. T. (1999). Aerial Survey of Waterbirds on Wetlands as a Measure of River and Floodplain Health. Freshw. Biol. 41, 425–438. doi:10.1046/j.1365-2427.1999.00440.x
Kingsford, R. T., and Auld, K. M. (2005). Waterbird Breeding and Environmental Flow Management in the Macquarie Marshes, Arid Australia. River Res. Applic. 21, 187–200. doi:10.1002/rra.840
Kingsford, R. T., Basset, A., and Jackson, L. (2016). Wetlands: Conservation's Poor Cousins. Aquat. Conserv: Mar. Freshw. Ecosyst. 26, 892–916. doi:10.1002/aqc.2709
Kingsford, R. T., Bino, G., and Porter, J. L. (2017). Continental Impacts of Water Development on Waterbirds, Contrasting Two Australian River Basins: Global Implications for Sustainable Water Use. Glob. Change Biol. 23, 4958–4969. doi:10.1111/gcb.13743
Kingsford, R. T. (2011). Conservation Management of Rivers and Wetlands under Climate Change - a Synthesis. Mar. Freshw. Res. 62, 217–222. doi:10.1071/mf11029
Kingsford, R. T. (2000). Ecological Impacts of Dams, Water Diversions and River Management on Floodplain Wetlands in Australia. Austral Ecol. 25, 109–127. doi:10.1046/j.1442-9993.2000.01036.x
Kingsford, R. T., Mac Nally, R., King, A., Walker, K. F., Bino, G., Thompson, R., et al. (2015). A Commentary on 'Long-Term Ecological Trends of Flow-dependent Ecosystems in a Major Regulated River basin', by Matthew J. Colloff, Peter Caley, Neil Saintilan, Carmel A. Pollino and Neville D. Crossman. Mar. Freshw. Res. 66, 970–980. doi:10.1071/mf15185
Kingsford, R. T., and Porter, J. L. (1994). Waterbirds on an Adjacent Freshwater lake and Salt Lake in Arid Australia. Biol. Conservation 69, 219–228. doi:10.1016/0006-3207(94)90063-9
Kingsford, R. T., Roshier, D. A., and Porter, J. L. (2010). Australian Waterbirds - Time and Space Travellers in Dynamic Desert Landscapes. Mar. Freshw. Res. 61, 875–884. doi:10.1071/mf09088
Kingsford, R. T., Walker, K. F., Lester, R. E., Young, W. J., Fairweather, P. G., Sammut, J., et al. (2011). A Ramsar Wetland in Crisis - the Coorong, Lower Lakes and Murray Mouth, Australia. Mar. Freshw. Res. 62, 255–265. doi:10.1071/mf09315
Kirsch, E., Colloff, M. J., and Pittock, J. (2021). Lacking Character? A Policy Analysis of Environmental Watering of Ramsar Wetlands in the Murray–Darling Basin, Australia. Mar. Freshw. Res.. doi:10.1071/MF21036
Leblanc, M., Tweed, S., Van Dijk, A., and Timbal, B. (2012). A Review of Historic and Future Hydrological Changes in the Murray-darling Basin. Glob. Planet. Change 80-81, 20. doi:10.1016/j.gloplacha.2011.10.012
Leigh, C., and Sheldon, F. (2008). Hydrological Changes and Ecological Impacts Associated with Water Resource Development in Large Floodplain Rivers in the Australian Tropics. River Res. Applic. 24, 1251–1270. doi:10.1002/rra.1125
Loch, A., Adamson, D., and Auricht, C. (2020). (g)etting to the point: The Problem with Water Risk and Uncertainty. Water Resour. Econ. 32, 100154. doi:10.1016/j.wre.2019.100154
Mace, G. M., and Baillie, J. E. M. (2007). The 2010 Biodiversity Indicators: Challenges for Science and Policy. Conservation Biol. 21, 1406–1413. doi:10.1111/j.1523-1739.2007.00830.x
Masson-Delmotte, V., Zhai, P., Pörtner, H.-O., Roberts, D., Skea, J., Shukla, P. R., et al. (2018). Global Warming of 1.5 C. An IPCC Special Report on the Impacts of Global Warming of 1(5). Geneva, Switzerland: Intergovernmental Panel on Climate Change.
Matthews, J. W., Spyreas, G., and Endress, A. G. (2009). Trajectories of Vegetation-Based Indicators Used to Assess Wetland Restoration Progress. Ecol. Appl. 19, 2093–2107. doi:10.1890/08-1371.1
Matthews, K. (2017). Independent Investigation into NSW Water Management and Compliance–Final Report. Sydney, NSW: Department of Industry. Available at: https://www.industry.nsw.gov.au/__data/assets/pdf_file/0019/131905/Matthews-final-report-NSW-water-management-and-compliance.pdf.
MDBA (2020). Basin-wide Environmental Watering Strategy. Second edition. Canberra: Murray-Darling Basin Authority.
MDBA (2010). Guide to the Proposed Basin Plan: Technical Background. Canberra: Murray–Darling Basin Authority.
MDBA (2011a). The Proposed “Environmentally Sustainable Level of Take” for Surface Water of the Murray‐Darling Basin: Methods and Outcomes. MDBA publication no: 226/11. Canberra: Murray‐Darling Basin Authority.
MDBA (2011b). Timeseries Computer Model Data for Proposed Basin Plan. Canberra: Murray-Darling Basin Authority MDBA.
Millennium Ecosystem Assessment (2005). Ecosystems and Human Well-Being: Biodiversity Synthesis. Washington, DC, USA: World Resources Institute.
Navarro, L. M., Fernández, N., Guerra, C., Guralnick, R., Kissling, W. D., Londoño, M. C., et al. (2017). Monitoring Biodiversity Change through Effective Global Coordination. Curr. Opin. Environ. sustainability 29, 158–169. doi:10.1016/j.cosust.2018.02.005
Nilsson, C., Reidy, C. A., Dynesius, M., and Revenga, C. (2005). Fragmentation and Flow Regulation of the World's Large River Systems. Science 308, 405–408. doi:10.1126/science.1107887
O’Donnell, T. K., and Galat, D. L. (2008). Evaluating success Criteria and Project Monitoring in River Enhancement within an Adaptive Management Framework. Environ. Manage. 41, 90–105. doi:10.1007/s00267-007-9010-5
Peterson, T. J., Saft, M., Peel, M. C., and John, A. (2021). Watersheds May Not Recover from Drought. Science 372, 745–749. doi:10.1126/science.abd5085
Pittock, J., and Finlayson, C. M. (2011). Australia's Murray - Darling Basin: Freshwater Ecosystem Conservation Options in an Era of Climate Change. Mar. Freshw. Res. 62, 232–243. doi:10.1071/mf09319
Pittock, J., and Hartmann, J. (2011). Taking a Second Look: Climate Change, Periodic Relicensing and Improved Management of Dams. Mar. Freshw. Res. 62, 312–320. doi:10.1071/mf09302
Podger, G., Yang, A., Brown, A., Teng, J., Power, R., and Seaton, S. (2010). Proposed River Modelling Methods and Integrated River System Modelling Framework Design for Use in Basin Plan Modelling. Canberra, SA: CSIRO: Water for a Healthy Country National Research Flagship.
Prosser, I. P., Chiew, F. H. S., and Stafford Smith, M. (2021). Adapting Water Management to Climate Change in the Murray-Darling Basin, Australia. Water 13, 2504. doi:10.3390/w13182504
Puckridge, J. T., Walker, K. F., and Costelloe, J. F. (2000). Hydrological Persistence and the Ecology of Dryland Rivers. Regul. Rivers: Res. Mgmt. 16, 385–402. doi:10.1002/1099-1646(200009/10)16:5<385:aid-rrr592>3.0.co;2-w
R Core Team (2018). R: A Language and Environment for Statistical Computing, Vienna, Austria: R Foundation for Statistical Computing. R Foundation for Statistical Computing. ISBN 3-900051-07-0, Available at: http://www.R-project.org.
Raftery, A. E. (1995). Bayesian Model Selection in Social Research. Sociological Methodol. 25, 111–164. doi:10.2307/271063
Romañach, S. S., D'Acunto, L. E., Chapman, J. P., and Hanson, M. R. (2021). Small Mammal Responses to Wetland Restoration in the Greater Everglades Ecosystem. Restoration Ecol. 29, e13332. doi:10.1111/rec.13332
Sandi, S. G., Rodriguez, J. F., Saintilan, N., Wen, L., Kuczera, G., Riccardi, G., et al. (2020). Resilience to Drought of Dryland Wetlands Threatened by Climate Change. Sci. Rep. 10, 13232. doi:10.1038/s41598-020-70087-x
Shaeri Karimi, S., Saintilan, N., Wen, L., Valavi, R., and Cox, J. (2021). The Ecohydrological Impact of Water Resource Developments through Inundation Regime Analysis of a Large Semi-arid Floodplain. J. Hydrol. 596, 126127. doi:10.1016/j.jhydrol.2021.126127
Sims, N. C., Chariton, A. A., Jin, H., and Colloff, M. J. (2011). A Classification of Floodplains and Wetlands of the Murray-Darling Basin Based on Changes in Flows Following Water Resource Development. Wetlands 32, 239–248. doi:10.1007/s13157-011-0231-2
Smith, M. S., Horrocks, L., Harvey, A., and Hamilton, C. (2011). Rethinking Adaptation for a 4 ° C World. Phil. Trans. R. Soc. A. 369, 196–216. doi:10.1098/rsta.2010.0277
Swirepik, J. L., Burns, I. C., Dyer, F. J., Neave, I. A., O'brien, M. G., Pryde, G. M., et al. (2016). Establishing Environmental Water Requirements for the Murray-Darling Basin, Australia's Largest Developed River System. River Res. Applic. 32, 1153–1165. doi:10.1002/rra.2975
Thoms, M. C., and Sheldon, F. (2000). Water Resource Development and Hydrological Change in a Large Dryland River: the Barwon-Darling River, Australia. J. Hydrol. 228, 10–21. doi:10.1016/s0022-1694(99)00191-2
Tonkin, J. D., Poff, N. L., Bond, N. R., Horne, A., Merritt, D. M., Reynolds, L. V., et al. (2019). Prepare River Ecosystems for an Uncertain Future. Nature 570, 301–303. doi:10.1038/d41586-019-01877-1
Wheeler, S. A., Carmody, E., Grafton, R. Q., Kingsford, R. T., and Zuo, A. (2020). The Rebound Effect on Water Extraction from Subsidising Irrigation Infrastructure in Australia. Resour. Conservation Recycling 159, 104755. doi:10.1016/j.resconrec.2020.104755
Whipple, A., and Viers, J. (2019). Coupling Landscapes and River Flows to Restore Highly Modified Rivers. Water Resour. Res. 55, 4512–4532. doi:10.1029/2018wr022783
White, H. J., Caplat, P., Emmerson, M. C., and Yearsley, J. M. (2021). Predicting Future Stability of Ecosystem Functioning under Climate Change. Agric. Ecosyst. Environ. 320, 107600. doi:10.1016/j.agee.2021.107600
Williams, B. K. (2011). Adaptive Management of Natural Resources-Framework and Issues. J. Environ. Manage. 92, 1346–1353. doi:10.1016/j.jenvman.2010.10.041
Wintle, B. A., McCarthy, M. A., Volinsky, C. T., and Kavanagh, R. P. (2003). The Use of Bayesian Model Averaging to Better Represent Uncertainty in Ecological Models. Conservation Biol. 17, 1579–1590. doi:10.1111/j.1523-1739.2003.00614.x
Xi, Y., Peng, S., Ciais, P., and Chen, Y. (2021). Future Impacts of Climate Change on Inland Ramsar Wetlands. Nat. Clim. Chang. 11, 45–51. doi:10.1038/s41558-020-00942-2
Zaldívar-Jiménez, A., Ladrón-de-Guevara-Porras, P., Pérez-Ceballos, R., Díaz-Mondragón, S., and Rosado-Solórzano, R. (2017). US-Mexico Joint Gulf of Mexico Large marine Ecosystem Based Assessment and Management: Experience in Community Involvement and Mangrove Wetland Restoration in Términos Lagoon, Mexico. Environ. Dev. 22, 206–213.
Zedler, J. B. (2000). Progress in Wetland Restoration Ecology. Trends Ecol. Evol. 15, 402–407. doi:10.1016/s0169-5347(00)01959-5
Zheng, H., Chiew, F., Potter, N., and Kirono, D. (2019). “Projections of Water Futures for Australia: an Update,” in MODSIM2019, 23rd International Congress on Modelling and Simulation, Canberra, December 1–6, 2019 (Modelling and Simulation Society of Australia and New Zealand), 1–6.
Keywords: Murray-Darling Basin, waterbirds, river regulation, river restoration, climate change, aerial survey, Australia
Citation: Bino G, Brandis K, Kingsford RT and Porter J (2021) Shifting Goalposts: Setting Restoration Targets for Waterbirds in the Murray-Darling Basin Under Climate Change. Front. Environ. Sci. 9:785903. doi: 10.3389/fenvs.2021.785903
Received: 29 September 2021; Accepted: 19 November 2021;
Published: 09 December 2021.
Edited by:
Julius Kipkemboi, Egerton University, KenyaReviewed by:
Glenn Guntenspergen, United States Geological Survey (USGS), United StatesJeyaraj Antony Johnson, Wildlife Institute of India, India
Copyright © 2021 Bino, Brandis, Kingsford and Porter. This is an open-access article distributed under the terms of the Creative Commons Attribution License (CC BY). The use, distribution or reproduction in other forums is permitted, provided the original author(s) and the copyright owner(s) are credited and that the original publication in this journal is cited, in accordance with accepted academic practice. No use, distribution or reproduction is permitted which does not comply with these terms.
*Correspondence: Gilad Bino, Z2lsYWQuYmlub0B1bnN3LmVkdS5hdQ==