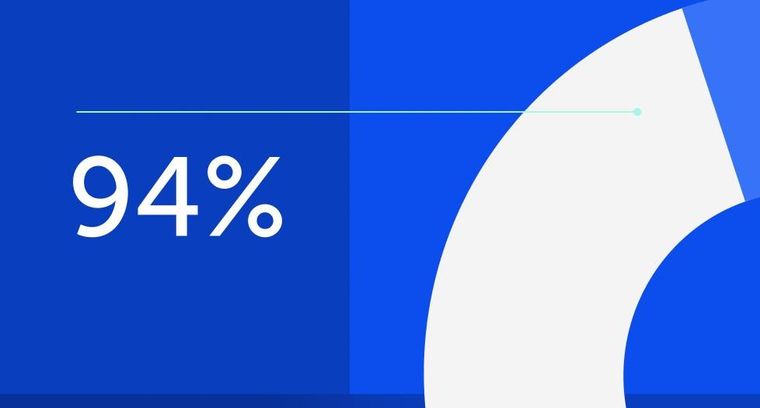
94% of researchers rate our articles as excellent or good
Learn more about the work of our research integrity team to safeguard the quality of each article we publish.
Find out more
ORIGINAL RESEARCH article
Front. Environ. Sci., 03 November 2021
Sec. Soil Processes
Volume 9 - 2021 | https://doi.org/10.3389/fenvs.2021.765696
In this study the impacts of urbanity on physical soil properties were explored by measuring water stable aggregates, combined particle size, infiltration rate and hydrophobicity across an urban gradient. The use of a gradient allowed for the relative importance of different environmental drivers to be assessed. We sampled 54 sites across Berlin and used a pre-existing database of environmental variables to extract three main axes of variation relating to urbanity, soil nutrient content, and heavy metal content. These axes, along with site age, were used to explore the drivers of changes in physical properties across an urban landscape. The percentage of water stable aggregates was found to decrease with urbanity, whilst infiltration rate was found to increase. Hydrophobicity did not appear to be influenced by urbanity but interacted with both infiltration rate and water stable aggregates. Combined particle sizes in the soil were found to increase with urbanity. Our findings provide evidence for urbanity being an important driver of variation in physico-chemical soil properties, which has implications for the provision of ecosystem services by these soils.
Since the middle of the 20th Century, the global urban population has been rapidly increasing, from 751 million individuals in 1950 to an estimated 4.2 billion in 2018, which accounts for roughly 55% of the total global population (United Nations, 2018). It has been estimated that whilst less than 0.5% of total global land surface is covered in built-up urban developments, this small percentage represented a total of 0.5 million km2 at the turn of the last century (Goldewijk et al., 2010), although estimates vary (Potere and Schneider, 2007). These urban ecosystems not only provide services to their inhabitants but are also often hotspots for global change factors such as increased temperature (Arnfield, 2003), salinisation (Equiza et al., 2017), and the presence of pollutants such as heavy metals (Plyaskina and Ladonin, 2009). Hence, urban soils can provide valuable information when it comes to understanding the impacts of global change factors on soil ecosystems and their functioning, which might impact human health (Brevik and Burgess, 2014). In these systems, anthropogenic interference is commonplace; this can include the management of soils (e.g. mowing and irrigation in urban parks), transportation of soils (e.g. due to construction; Hooke, 2000), soil sealing, and the addition of waste and construction material, such as building sand (Bridges, 1991). Urban landscapes have previously been associated with generally high levels of compaction (Lehmann and Stahr, 2007), although this may only be true in localised areas of high intensity usage (Edmondson et al., 2011). Geochemical cycling, hydrosystems and biodiversity have been demonstrated to change with rising urbanity (Grimm et al., 2008), however, many of the underlying soil characteristics which determine ecosystem processes have not been studied. For example, whilst aggregate stability is known to be a vital component of soil structure and functioning (Bronick and Lal, 2005), its response to urbanity has not previously been investigated and it is not known whether responses are universally similar (as could be expected according to the “urban ecosystem convergence hypothesis” proposed by Pouyat et al., 2003).
Urban soils provide a range of important ecosystem services themselves, such as hydrological control through infiltration, and as the substrate for plant growth (Morel et al., 2015). Via this impact on local flora, they not only support food production but also the parks and green spaces which have been demonstrated to support the wellbeing of urban residents (Tzoulas et al., 2007; Diaz et al., 2018). Inputs of fertilizers, elevated levels of N deposition and altered irrigation, as well as the removal of organic matter, cause altered nutrient cycling compared to natural ecosystems (Lorenz and Lal, 2009), with the new equilibrium varying across climatic regions, parent materials and socioeconomic areas (Pickett et al., 2001). The functioning of soil is closely related to its structure, which determines the availability of air, water, nutrients and pollutants to the microbial life which inhabits it. This relationship is of course not one sided, with soil microbes playing an important role in shaping soils through both their chemical and (in the case of filamentous fungi) physical properties. In this study, four soil physico-chemical parameters were measured across an urban gradient in Berlin. These properties were selected for their importance for soil functioning and their likelihood of showing a response to urbanity, they are: the percentage of water stable aggregates (WSA%), combined particle size (in this case referring to the soil fractions separated by simple dry sieving, comprising separate measures of mean weight diameter, coefficient of curvature, and uniformity coefficient), water infiltration rate and hydrophobicity.
The key building blocks of soils are aggregates, the stability and structure of which determine many of the ecosystem services provided by soil (Six et al., 2004; Baer and Birge, 2018). Aggregates provide a variety of niches for microbial life due to the oxygen gradients within them (Wilpiszeski et al., 2019; Cui et al., 2020) and impact soil hydrology through influencing infiltration and water holding capacity (Baer and Birge, 2018). Aggregates also provide surfaces for the adsorption of nutrients (e.g. Thao et al., 2008) and heavy metals (Huang et al.,2020), thus determining their availability. The ability of aggregates to maintain their structural integrity in the face of drying, wetting (Caron et al., 1996), freezing, thawing (Layton et al., 1993), and rain drop impact (Ramos and Nacci, 2003) is a key factor in the prevention of slaking and maintaining soil porosity. The increased levels of disturbance associated with urban systems have also been hypothesised to disrupt aggregate formation, with one notable experimental study by Chen et al. (2014). Observational studies by Jim (1998a, 1998b) reported a wide variety of levels of aggregation in urban soils in Hong Kong, however, in the absence of a non-urban comparison or an urbanity gradient, the impact of urbanity remains unclear. In general, observational studies of urban aggregate presence are lacking. Here, we used mean weight diameter and WSA% to test if soil aggregation properties change in relation to urbanity.
Infiltration is a macroscopic event, but it is largely determined by microscopic factors. Soil pores and crevices provide avenues for water infiltration, whilst hydrophobic compounds coating surfaces prevent flow. The infiltration of water carries both nutrients and pollutants through the soil matrix; the impacts of infiltration rate are therefore manifold, ranging from flood management to influencing plant growth (Assouline, 2013). If the rate of infiltration is low, water supply can easily exceed it, resulting in ponding on the surface and consequently run-off or even flooding (Morbidelli et al., 2018). These macro-scale services of infiltration rate have caused urban infiltration studies to generally focus on a large geographic scale where anthropogenic soil sealing (i.e. paving) is the determining factor of infiltration rate (e.g. Haase and Nuissl, 2007; Perry and Nawaz, 2008), however, it is not clear whether non-sealed areas within the urban landscape also express altered infiltration rates. These areas are likely to receive run-off from sealed areas, the implications of which are uncertain. Hypothetically these run-off events could lead to the destruction of aggregates and the loss of finer soil fractions, resulting in an increased infiltration rate. If this were to be the case, un-sealed soils may buffer the loss of infiltration potential in proximal sealed areas. Some studies suggest that urban soils express low infiltration rates due to soil compaction (Yang and Zhang, 2011), with construction and its associated heavy machinery having a particularly pronounced impact (Gregory et al., 2006). However, increased compaction in urban systems is unlikely to be universal (Edmondson et al., 2011) and a wide variety of other factors are thought to play a role (e.g. crust formation according to Assouline, 2004), therefore, it is difficult to predict the impacts of urbanity upon infiltration.
Soil hydrophobicity, which causes soil water repellency, is an important factor determining the infiltration rate of water into soil, thereby controlling whether precipitation can contribute to the groundwater recharge or is repelled as surface run-off. Having been largely ignored until the 1960s, this factor has gone from being considered an obscurity to being considered a ubiquitous factor in many ecosystems around the globe (DeBano, 2000; Mao et al., 2019). Despite this, it is only in the past few years that the practicalities of exploring the underlying causes of hydrophobicity have been surmounted. The consensus is now that long-chain carbon molecules coat the outer layers of soil particles, creating a thin hydrophobic layer (Doerr et al., 2000; Mao et al., 2019). Consequently, many studies report sandy, large-particle soils as expressing the greatest levels of hydrophobicity (Savage et al., 1969; McGhie and Posner, 1981; York and Canaway, 2000). However, this is not universal; in some cases, clay-rich soils also present high levels of hydrophobicity. In these situations, hydrophilic particles aggregate together, surrounded by hydrophobic particles, thus producing one large aggregate with a low surface area and a water-repellent surface (Bisdom et al., 1993; Doerr et al., 2000). Other research has pointed to hydrophobicity occurring in even the finest fractions of soils (de Jong et al., 1999), suggesting that fine particles of organic matter may themselves exhibit hydrophobicity. The source of hydrophobic compounds in soils appears to vary significantly, some of those identified include fires, plant roots, leaf detritus, soil microbes, and untreated wastewater (Mao et al., 2019). The impacts of soil hydrophobicity are also varied, with some reporting an increase in erosion due to increased water run-off (Miyata et al., 2007) and others suggesting that hydrophobicity increases aggregate resilience (Giovannini and Lucchesi, 1983; Korenkova and Matus, 2015). Hydrophobicity is known to be temporary, normally appearing after drought and lasting until a minimum wetting level has been reached (DeBano, 1971; Doerr et al., 2000; Dekker et al., 2001). The spatial layout of hydrophobicity also often varies in soils, with a hydrophobic surface layer concealing a hydrophilic layer beneath (Doerr et al., 2000; Mao et al., 2019). Hydrophobicity is a key component determining the infiltration of water and may well be influenced by urbanity; however, the total infiltration rate is also influenced by a variety of other factors, including soil structure and climatic conditions. As such, separate measures of hydrophobicity and infiltration rate were carried out in this study.
Urbanity is not a binary factor; urban systems are normally heterogenous landscapes expressing a wide variety of biotopes impacted by a variety of urban related and non-urban related variables. Urbanity itself is a qualitative measure, for which there is no universal method of quantification (Moll et al., 2019). By limiting this study to grasslands and comparing their key physical soil properties to the wide array of data already gathered on the study sites, an insight into the ways in which urbanity influences Berlin’s soil can be gained. A selection of these variables can also be identified which are associated with urbanity, and thus used to create a post-hoc scale of urbanity amongst sites. Thereby, the impact of urbanity on important components of soil functioning can be understood in more detail.
Berlin, Germany’s capital and largest city has triggered a long-lasting history of urban ecological research (Kowarik, 2020). Based on this tradition, the establishment of the CityScapeLab experimental research platform (von der Lippe et al., 2020) allows for the investigation of urban effects on biodiversity and ecological functioning independently from the vast heterogeneity of urban habitats. The grassland branch of the CityScapeLab consists of 56 plots (16 m2 each) situated in patches of dry grasslands across Berlin and its surrounding federal state, Brandenburg. These sites represent a spectrum of urbanity, ranging from grasslands in forested or agricultural contexts, to historical parks, to novel sites with artificial or heavily modified soils, such as road verges and vacant land (e.g. abandoned rail yards and airfields). A database of environmental parameters for these study sites was established in the summer of 2017 and consists of soil chemical data as well as habitat connectivity, site age, plant cover, and a variety of measures of urban factors, including population density, soil sealing, road density and floor area ratio (see database information below). For this study, two sites were discounted due to missing data, leaving a total of 54 sites (Figure 1).
FIGURE 1. (A) A map of Berlin and the surrounding state, Brandenburg, showing the locations of the 54 CityScapeLab sites used in this investigation and their PCA axis 1 scores (see results), representing a gradient of urbanity. Grey areas denote built-on and traffic areas. Photographs are examples of grassland patches at (B) low (PCA axis 1 score: −4.71) (C) intermediate (PCA axis 1 score: 0.08) and (D) high (PCA axis 1 score: 7.4) urbanity levels.
The 54 study sites encompassed dry grasslands which ranged in pH from 4.1 to 7.5. Moreover, the sites represented a range of urban impact from locations where the population density was as high as 97 people per hectare within a 100 m buffer and sites where 70% of the soil within a 100 m buffer was sealed, to rural sites where both these values were 0 (see database information below). The climate in Berlin is temperate with an average precipitation of 576 mm per year and an average annual temperature of 9.9°C, although recent years have witnessed hotter, drier periods (von der Lippe et al., 2020). All of Berlin’s soil has a high sand content, with textures limited to sand, medium loamy sand and medium silty sand (Gerstenberg, 2017). During the latter half of the 19th century Berlin expanded rapidly due to industrialisation and its selection as the new capital of Germany. In the 20th century, Berlin experienced the destruction of one third of its housing during the second world war, followed by the creation of the Berlin wall in 1961, which cut a swathe through the city. The destruction of the Berlin wall and German reunification in 1989 and 1990 led to the creation of many brownfield sites (meaning; sites previously built on and/or possibly polluted) and parks, as well as the abandonment of many buildings (Arandjelovic and Bogunovich, 2014). Many of the sites in this study will have been directly impacted by this tumultuous history and have either been left as brownfield sites or been turned into urban parks (e.g. Park am Nordbahnhof, a former location of the Berlin Wall).
Infiltration was measured in-situ using a Mini-Disk Infiltrometer (METER Group, Inc., Pullman, WA, United States). Three distinct flat areas were located within each field site and surface detritus was removed. Sampling locales were evenly spread across the study site and were selected for their ability to represent visible within-plot variation. At each of the three flat areas the infiltration rate was measured for 5 min with the Mini-Disk Infiltrometer set to a suction rate of 2 cm. Infiltration rates were then calculated using a tool provided by the Meter Group for use with the infiltrometer. The mean infiltration value was then calculated for each site, as well as standard error, which was used as a measure of within-site variation. This method was selected due to the ease with which field measurement can be taken with the Mini-Disk Infiltrometer, requiring only small amounts of water (<100 ml) and time for each replicate (Bát’ková et al., 2020). All infiltration measurements and sampling were performed between June and August 2020.
From within each of the 4 m × 4 m CityScapeLab plots three replicates of 30–40 g of topsoil were taken and placed in falcon tubes. The sampling locales within the plot were decided upon due to their ability to represent within-plot variation. The impacts of urbanity were expected to be concentrated at the soil surface; therefore, samples were taken from the top 2–3 cm of soil using a flat-bladed wide-headed spatula. Care was taken to not compress samples during extraction. Samples were air dried in the opened falcon tubes before being stored at 4°C until use.
To achieve a measure of the percentage of water stable aggregates (WSA%), 4.0 g of soil was wet sieved using a wet sieving apparatus (Eijkelkamp, Netherlands) fitted with 0.25 mm sieves and the WSA% was calculated in accordance with Kemper and Rosenau (1986). There were three technical replicates per site, from which an average WSA% was calculated.
Combined particle size was measured through dry sieving: 8.0 g of soil were passed through a 4 mm sieve to remove excess debris before being passed through 2 mm, 1 mm, 500 μm, 100 and 50 µm sieves. The fractions held by each sieve were used to calculate the mean weight diameter (MWD), coefficient of curvature and uniformity coefficient (Samtani, 2006) for each replicate. A mean value for each measurement was then calculated for each site. MWD is often used in addition to wet sieving to measure the size of soil aggregates (van Bavel, 1950), however, here it was used to measure the size of all soil particles including sand. The coefficient of curvature is a measure of how well a soil is graded (i.e. contains a continuous range of particle sizes) and the uniformity coefficient is a measure of how similar in size the particles in the soil are. Together, these parameters can indicate if additional material has been imported to the site (e.g. larger diameter gravel for construction).
Hydrophobicity was measured using the Molarity of Ethanol Drop (MED) test, based on the protocol of Doerr (1998). Soil samples were placed into a pipetting reservoir with sloped sides to maximise available surface area for testing. Organic detritus and solid substrate particles larger than ⌀4 mm were removed and the surface was gently smoothed. ∼⌀5 mm droplets of ethanol in concentrations of 0, 4, 8, 12, 16, 20, 24, 28, and 32 percent were pipetted onto the soil surface and the lowest concentration whereby the drop infiltrated within 3 s was recorded. This was repeated for three technical replicates per site, from which an average ethanol concentration was calculated.
Due to all soils being dried before analysis, the levels of hydrophobicity measured may not exactly represent the levels found in-situ. However, given that sampling took place over a period of weeks pre-drying was appropriate. Hydrophobicity is generally most pronounced in surface soils, with localised areas of hydrophilic soil beneath them creating “finger flow” during wetting events, it was therefore decided to focus only on surface soil in order to sample comparable soils from each site.
A database of variables (von der Lippe et al., 2021) measured mostly in 2017 was used to provide information about the study sites. This database comprised of soil chemical measurements such as pH, concentrations of heavy metals (Zn, Cd, Pb, Ni, Cu), soil nutrients (N, P, K, S, organic C), water content, cation exchange capacity, electrical conductivity, as well as variables such as climate and weather measures, site connectivity, density of roads and railways, distance to roads and railways, soil sealing, population density, floor area ratio (FAR; a measure of building density) and an index of urbanity, combining FAR, soil sealing and population density. The sites were also categorised as either “old” or “new” depending on whether they existed as a grassland prior to 1940. For further information on all these parameters, please see: von der Lippe et al. (2020). Additionally, the distance from each site to the city centre point (Flächenschwerpunkt stone, Berlin) was calculated and included.
We decided that the inclusion of the soil chemical data, which could be considered to some extent transitory, was appropriate for this study. The reason for this decision was that the main causes of change for these values would be land-use or climate change. As land use could be expected to remain relatively constant in the intervening years between sampling and change in climate should have impacted all sites relatively evenly, it was decided that the benefits of including these data outweighed any possible limitations.
Due to many of the database variables being highly collinear, a PCA of variables was carried out using the ade4 package (Thioulouse et al., 2018) to resolve the main axes of variation (as discussed in: du Toit and Cilliers, 2011; McDonnell et al., 2012; Moll et al., 2019). Axes to keep for further inclusion in analyses were selected qualitatively, depending on whether they summarised a discernible syndrome that could be expected to influence soil properties. Kendall correlations (using R package Corrr; Kuhn et al., 2020) were used to correlate the physical soil properties measured with the axis scores of the PCA. Wilcoxon rank sum tests were used to test for differences in the physical properties between age groups of sites. Physical properties were correlated with each other using Kendall correlations. Non-parametric tests were used due to infiltration rate, standard error of infiltration rate, hydrophobicity and PCA axis 1 scores being non-normally distributed. Due to the sample size, correlations were more appropriate than nonparametric alternatives to regression models. All statistics were carried out in R (R Core Team, 2020). All plots were created using the ggplot2 package (Wickham, 2016), and aggregated using the ggpubr package (Kassambara, 2020). Figure 1 was created using the sf (Pebesma, 2018) and ggspatial (Dunnington, 2021) packages, with colours provided by the viridis package (Garnier et al., 2021).
Raw soil physico-chemical data and PCA axis scores are available via the Figshare online repository (Whitehead et al., 2021).
A summary of all PCA variable loadings can be found in Supplementary Table S1.
The first three PCA axes of variation were selected for the further analysis in this study due to the relatively clear syndromes they represented: Axis 1 comprised of mainly urban-related variables, relating to roads, railways, soil sealing, population, and distance from the city centre, and explained 24.3% of the variation seen in the data. Axis 2 explained 9.4% of the variation in the data and comprised of the soil nutrients N, S and organic C, as well as water content. Axis 3 explained only 6.9% of the variation in the data and comprised of heavy metals, particularly Ni and Cd, and site connectivity. pH was found to be a contributor to axis 3, but to a lesser extent than the heavy metals (Figure 2 and Supplementary Figure S1). Further PCA axes were excluded due to their failure to represent interpretable environmental syndromes.
FIGURE 2. Significant variables in each of the three PCA axes; only variables with a loading of >0.175 or < −0.175 are plotted. The total amount of variation in the dataset that the axis accounts for is stated alongside the axis name. For variables which were calculated within multiple different distance buffers surrounding the study sites, the size of the distance buffer is presented on the bar. For biplots of axes see Supplementary Figure S1.
It was found that axis 1 scores were significantly higher for new sites than old sites (Wilcoxon rank sum W = 623, p < 0.001), whilst axes 2 and 3 did not appear to be related to site age (Supplementary Figure S2).
A summary of results for all response variables is presented in Supplementary Table S2.
WSA% showed a negative correlation with axis 1 (Kendall’s Tau = −0.20, p = 0.03) and a positive trend with axis 2 (Kendall’s Tau = −0.17, p = 0.07) (Figure 3A). Old sites had a higher WSA% than new sites (Wilcoxon rank sum W value = 234, p = 0.04) (Figure 3B).
FIGURE 3. Relationships between soil parameters versus PCA axes and site age (N = New, O = Old). (A) WSA% vs. PCA axes (B) WSA% vs. Age (C) MWD vs. PCA axes (D) MWD vs. Age (E) Infiltration Rate vs. PCA axes (F) Infiltration Rate vs. Age. Lines shown are simple linear regression lines and grey areas represent standard error, values of Kendall’s Tau and P are presented in each plot. Boxplots show median, first and third quantiles, with whiskers showing range, Wilcoxon Rank Sum W and p values are presented in each plot.
Mean weight diameter showed a positive correlation with axis 1 (Kendall’s Tau = 0.23, p = 0.01) and a negative trend with axis 3 (Kendall’s Tau = −0.17, p = 0.07) (Figure 3C). There was also a significant difference in MWD between old and new sites, with newer sites having a higher MWD (Wilcoxon rank sum W = 497, p = 0.01) (Figure 3D). Both the coefficient of uniformity and coefficient of curvature did not relate to any of the PCA axes.
Infiltration rate had a positive correlation with axis 1 (Kendall’s Tau = 0.25, p = 0.009) and a negative correlation with axis 2 (Kendall’s Tau = −0.20, p = 0.03) (Figure 3E). The standard error of infiltration rate also showed a negative correlation with axis 2 (Kendall’s Tau = −0.20, p = 0.03). No significant difference in infiltration rate between old and new sites was observed (Figure 3F).
Hydrophobicity did not show clear trends with any of the PCA axes or site age.
Average infiltration rate demonstrated a strong negative correlation with WSA% (Kendall’s Tau = −0.33, p = <0.001) (Figure 4A) and a negative correlation with hydrophobicity (Kendall’s Tau = −0.22, p = 0.02), although this relationship appeared to be more complicated than a simple linear relationship (Figure 4B). There was a strong positive correlation between the WSA% and hydrophobicity (Kendall’s Tau = 0.34, p < 0.001) (Figure 4C). It should also be noted that as the infiltration rate increased, so too did the within-site variation, as measured by the standard-error of the infiltration rate (Kendall’s Tau = 0.55, p < 0.001).
FIGURE 4. Relationships between soil properties. (A) Infiltration rate vs. WSA% (B) Infiltration rate vs. hydrophobicity (C) Hydrophobicity vs. WSA%. Lines shown are simple linear regression lines and grey areas represent standard error. Kendall’s Tau and p values are presented in each plot.
We found no significant relation between the coefficient of curvature and coefficient of uniformity, meaning that there was no notable connection between the grading of the soil particles and the variety of sizes of soil particles. However, the coefficient of uniformity did show a strong positive correlation with MWD (Kendall’s Tau = 0.49, p < 0.001), meaning that as average particle size got larger so too did the variety of particle sizes.
The PCA of variables led to the identification of three distinguishable main groups of explanatory variables. Axis 1 clearly related to urbanity, axis 2 related to soil nutrient content (specifically N, S and organic C) and water content, and axis 3 is related to heavy metals and site connectivity. Soil pH, often a key parameter in soil science, contributed to axis 3 but to a relatively minor extent. Electrical conductivity, a proxy for salinity, did not contribute significantly to any of the environmental axes. In urban research salinity is often a significant variable, however, in Berlin the use of salt as a de-icing agent has been banned since 2013 (Berliner Naturschutzgesetz, 2013), explaining its lack of contribution to the variance within our environmental dataset.
WSA% showed a significant trend for lower values in newer, more urban sites. Observations of urban impacts on soil structure have generally related to compaction, a reduction of WSA in relation to urbanity does not appear to have previously been reported. Due to the interest in compaction, bulk density is often the main parameter measured in studies of urban soil properties (Scharenbroch et al., 2005; Hagan et al., 2012; da Silva et al., 2017, Nero and Anning, 2018). Despite aggregate stability being discussed in association with urban soil bulk density measures (Matziris et al., 2016), direct observations of aggregate stability in relation to urbanity are lacking. Low to medium levels of aggregate stability have been recorded in an urban context before (Jim, 1998a; Jim, 1998b), but the findings were in relation to tree growth and no gradient in urbanity was reported. The use of bulk density is often chosen for its relation to abiotic ecosystem services such as infiltration, however, our findings about aggregate stability provide evidence for important biological and nutrient cycling ramifications of urbanity. A reduction in WSA% with increasing urbanity has implications for urban soil biodiversity due to the important role that bacteria and fungi (Lehmann et al., 2017), in particular arbuscular mycorrhizal fungi (Lehman et al., 2020), play in aggregate formation, thus a reduction in WSA% in urban soils may be a result of reduced microbial activity. However, this relationship is not unidirectional and may not tell the full story. If WSA are lost in an urban setting due to external mechanical stresses (e.g. trampling), then a reduction in WSA% may be a cause of, rather than a symptom of, a reduction in microbial activity. The chemical concentration gradients within WSA provide niches for organisms, in particular nitrifying bacteria, which are protected within their core (Wilpiszeski et al., 2019). A loss of WSA therefore heralds a loss of microbial functional diversity. Either way, their loss in an urban environment is deeply troubling and signals a disruption of nutrient cycling. WSA are also important due to their ability to prevent erosion and absorb nutrients, in a polluted environment they may also absorb heavy metals (Fan et al., 2013; Xu et al., 2017). The nutrient cycling function of WSA may be demonstrated by examining the relationship between WSA% and axis 2; both axes 2 and 3 appear to show a positive relationship with WSA%. This positive relationship to both axes is likely due to the increased sorption capacity of soil with a high WSA%, however, the weaker relationship with axis 3 is probably due to the compounding impact of axis 1, whereby more urban areas (which also tend to have higher heavy metal content) had lower WSA%. It seems unlikely that site connectivity, another component of axis 3, could directly impact WSA%. The increased WSA% in old sites may also be explained by an increased colonization of these soils by roots and microorganisms sensitive to disturbance; arbuscular mycorrhizal fungi, which are known to be sensitive to disturbance and land use change (Trejo et al., 2016) could explain this trend through the important role they play in aggregate formation (Rillig et al., 2010). Microbial life may also explain the link between high WSA% and high hydrophobicity due to the hydrophobins bacteria and fungi have been demonstrated to excrete to expediate the process of aggregation (Lehmann et al., 2017; Lehmann et al., 2020). Former studies have demonstrated a close link between hydrophobicity and aggregate stability (Zheng et al., 2016), with research demonstrating that hydrophobicity improves aggregate stability during wetting (Vogelmann et al., 2013). As such, a positive feedback loop between aggregate presence and hydrophobicity may be present. Hydrophobicity is often associated with sandy soils, where smaller surface areas are more easily coated by hydrophobic carbon-chain compounds. However, it appears that in this case hydrophobicity showed no relationship to MWD.
The findings of this study suggest that an increase in urbanity is associated with a loss in soil structure through reduced aggregate stability. It is, however, interesting to note that individual sites did not abide by this trend. Indeed, the highest levels of WSA% were seen at a surprising mix of sites; the highest was in a small grass patch between a new road development and a forest in Brandenburg, the second highest levels were observed in an abandoned underpass development in Schöneberg, a district in Southwest Berlin, and the third highest was in a grassland patch in Spandauer forest, near the Brandenburg-Berlin border. In accordance with the relationship between WSA% and axis 1, two of these sites were in comparatively rural locations, however, when the urban underpass development was sampled it was noted that moss and lichens were forming a crust and the previously cleared ground appeared to be experiencing secondary succession. Unsurprisingly all the sites with lowest WSA% were newer sites, with two of the lowest on central verges between roads. However, the very lowest WSA% was observed on parkland. This site was formerly the runway for Flugplatz Johannisthal, the second oldest airfield in the world. It seems that the remediation work that transformed this site into a non-industrial site had not yet resulted in substantial soil aggregate formation.
The positive trend between combined particle size and axis 1 is unlikely to be the result of the size of aggregates that withstood sieving, due to the negative relationship between WSA% and axis 1. The relationship between MWD and axis 1 is therefore likely due to sand and gravel particles. The cause for such a relationship could either be the removal of the finer fraction by erosion, or the addition of a larger fraction. It is possible that in urban grasslands, which experience heightened water flow because of proximal soil sealing, the finer fraction would be eroded over time. Alternatively, the importation of gravel for construction and the addition of fragments of building waste from anthropogenic activity may have supplied larger soil particles. The positive relationship between the uniformity coefficient and MWD suggests that the addition of new, larger particle size fractions is the cause of the aforementioned increase in MWD, rather than the removal of smaller soil fractions. All three of the sites with highest MWD were found near railway lines, with the highest MWD being in Natur-Park Schöneberger Südgelände, which was previously a rail yard and is proximal to a railway still in active use. Of the other two sites, one was on the embankment of a railway and the other was in a field around 140 m away from a railway. The site with the smallest MWD was a grassland formerly under the Berlin wall, previously mentioned due to its high infiltration rate. The other two sites with the lowest MWD were both grassland patches located in the Spandauer forest. The high MWD in railway-related sites is a clear indication that the urban-related increase in MWD observed in the study may be due to the use of gravel in the construction of railways.
Infiltration rate appears to be positively linked with urbanity (axis 1) and negatively with soil nutrient content (axis 2). Urban environments are often thought to exhibit low infiltration across a large geographic area due to the creation of impervious surfaces sealing the soil, but the findings of our study suggest that there is not a simple trade-off when it comes to soil sealing and infiltration rate; a loss in infiltration through soil sealing may be buffered by an increase in infiltration rate in proximal non-sealed soils. Infiltration rate has previously been explored in urban ecosystems in relation to soil compaction (Gregory et al., 2006; Yang and Zhang, 2011), which resulted in reduced infiltration. However, the findings of our study suggest that this is not the case in Berlin’s grasslands. Increased infiltration in sites adjacent to sealed areas is discussed in Scalenghe and Ajmone-Marsan (2009), however, this situation does not appear to have been comprehensively studied elsewhere.
It is possible that the increase in infiltration rate we observed may be due to the loss of WSA in urban areas, a hypothesis which is supported by the negative relationship between infiltration rate and WSA%, and between WSA% and axis 1. The soils in Berlin contain a large sand fraction, and so a lack of WSA may indicate the presence of very sandy soil which (not considering hydrophobicity) offers little resistance to infiltration. Infiltration rate showed its most significant response to axis 2, which was dominated by N, S, organic C, and water content. This negative relationship may simply be due to the aforementioned lack of WSA in sites with high infiltration, however, there could also be a direct effect of high levels of water flowing through the soil matrix upon nutrient content; both N and S are susceptible to leaching, and although C loss from soil is more often related to microbial activity, leaching of organic C has been demonstrated to have a detectable impact on C levels in grasslands (Kindler et al., 2011).
The negative relationship between infiltration and hydrophobicity is unsurprising, as it could be expected that a major limiting factor for infiltration would be hydrophobicity. However, the absence of a relationship between hydrophobicity and axis 1 means it is unlikely that hydrophobicity explains the urban-related change in infiltration. The trend for decreasing infiltration rate with increasing hydrophobicity did, however, have some notable exceptions; three sites with both high infiltration rates and high levels of hydrophobicity were recorded. It is possible these sites were experiencing “finger flow,” allowing replicates with very high rates of infiltration to compensate for those with slow rates due to spatially variable hydrophobicity, a hypothesis supported by the increase in standard error of infiltration at sites with high average infiltration rates.
The highest levels of infiltration were observed in Park am Nordbahnhof, an urban park built on land previously covered by the Berlin wall. Tiergarten (the iconic park in the centre of Berlin) and a very sandy grassland patch on the edge of Bieselheide national forest (formerly covered by the Berlin wall) also demonstrated high infiltration rates. It is interesting that these sites all expressed high infiltration rates but clearly had different management practices and site histories. One possible unifying feature of these sites could be a high level of human visitation; the former two are in popular urban parks whilst the third is sandwiched between two poorly defined pathways on the border between a suburban neighbourhood and a forest. Previous research has reported a loss of soil aggregate structure and a reduction in water infiltration rate because of cattle trampling, however, the reduction in infiltration was apparently less notable in sandy loam than in soil with a high clay content (Pietola et al., 2005). Our findings suggest that sandy soils exposed to trampling in urban parks may exhibit an increase in infiltration, perhaps due to a loss of soil structure without the substantial increase in bulk density associated with trampling by cattle. The two lowest infiltration rates were observed in grassland patches in forests surrounding Berlin, whilst the third lowest was on a patch of grassland next to Berlin’s International Congress Center (the site with the highest axis 1 score), shunning the general trend of infiltration rate increasing with urbanity. In contrast to the sites expressing high levels of infiltration, these sites are all secluded; either in a rural forest or bordered by a conference centre and several busy roads. Our findings suggest that while axis 1 comprised of many urban related factors which drive infiltration rate, human visitation may also be an important driver. It seems likely that in a soil with higher clay content, this pattern could easily be reversed due to compaction at highly visited sites.
In the wider context of urban research, these findings show a mixed result in terms of supporting the “urban ecosystem convergence hypothesis” (Pouyat et al., 2003). Our results imply a loss of soil structure with urbanity, as hypothesised previously by Chen et al. (2014). A reduction in infiltration rate is not observed, conversely to what has previously been demonstrated with compacted urban soils (Yang and Zhang, 2011) and in fact demonstrated an increase. It seems likely that the sandy nature of Berlin’s soil greatly influences the impacts of urban soil degradation, resulting in increased infiltration rather than a reduction. The measurement of combined particle sizes provides additional evidence for the importance of anthropogenic artifacts in determining the structure of urban soils (Bridges et al., 1991; Lehmann and Stahr, 2007; Pouyat et al., 2010). Our findings demonstrate that urban soils do not necessarily converge in their structure and function, with important implications for the cycling of nutrients within them, and the ecosystem services they provide.
Our findings signal the important role urbanity has in determining soil structure and function. The loss in stable aggregates with urbanity will likely be associated with a reduction in microbial functional diversity and consequently a loss in nutrient cycling capacity. An increased risk of soil erosion is also likely to be present. Our observation of increased infiltration rate with urbanity suggests that urban soils have a capacity to buffer local soil sealing in terms of infiltration capacity, this may however, be because of a loss of soil structure and aggregates. Those managing urban soils should be aware that, at least in the case of sandy soils, high infiltration rates may come at the cost of a loss of the biotic functioning of soils. Therefore, a careful balancing of management activities fostering physical or biotic soil functions is required.
The datasets presented in this study can be found in online repositories. The names of the repository/repositories and accession number(s) can be found below: Figshare: https://doi.org/10.6084/m9.figshare.16775701, https://doi.org/10.6084/m9.figshare.16811701.
JW, SH, and MR designed the research. JW conducted fieldwork and lab analyses, and wrote the first draft of the paper. AH and ML provided field data. All authors added to and edited the text.
This work was funded by the German Federal Ministry of Education and Research (BMBF) within the collaborative Project “Bridging in Biodiversity Science (BIBS-phase 2)” (funding number 01LC1501A).
The authors declare that the research was conducted in the absence of any commercial or financial relationships that could be construed as a potential conflict of interest.
All claims expressed in this article are solely those of the authors and do not necessarily represent those of their affiliated organizations, or those of the publisher, the editors and the reviewers. Any product that may be evaluated in this article, or claim that may be made by its manufacturer, is not guaranteed or endorsed by the publisher.
We acknowledge support by the Open Access Publication Initiative of Freie Universität Berlin. We thank Lena Fiechter for her help in collecting environmental data, and our reviewers for their comments.
The Supplementary Material for this article can be found online at: https://www.frontiersin.org/articles/10.3389/fenvs.2021.765696/full#supplementary-material
Arandjelovic, B., and Bogunovich, D. (2014). City Profile: Berlin. Cities 37, 1–26. doi:10.1016/j.cities.2013.10.007
Arnfield, A. J. (2003). Two Decades of Urban Climate Research: A Review of Turbulence, Exchanges of Energy and Water, and the Urban Heat Island. Int. J. Climatol. 23 (1), 1–26. doi:10.1002/joc.859
Assouline, S. (2013). Infiltration into Soils: Conceptual Approaches and Solutions. Water Resour. Res. 49 (4), 1755–1772. doi:10.1002/wrcr.20155
Assouline, S. (2004). Rainfall-induced Soil Surface Sealing: A Critical Review of Observations, Conceptual Models, and Solutions. Vadose Zone J. 3 (2), 570–591. doi:10.2136/vzj2004.0570
Baer, S. G., and Birgé, H. E. (2018). “Soil Ecosystem Services: An Overview,”. Managing Soil Health for Sustainable Agriculture. Editor D. Reicosky (London: Burleigh Dodds Science Publishing Limited), 1, 1–22. doi:10.19103/as.2017.0033.02
Bát’ková, K., Mihalikova, M., and Matula, S. (2020). Hydraulic Properties of a Cultivated Soil in Temperate Continental Climate Determined by Mini Disk Infiltrometer. Water 12 (3), 21. doi:10.3390/w12030843
Bisdom, E. B. A., Dekker, L. W., and Schoute, J. F. T. (1993). Water Repellency of Sieve Fractions from Sandy Soils and Relationships with Organic Material and Soil Structure. Geoderma 56 (1-4), 105–118. doi:10.1016/b978-0-444-81490-6.50013-3
Brevik, E. C., and Burgess, L. C. (2014). The Influence of Soils on Human Health. Nat. Educ. Knowledge 5 (12), 1.
Bridges, E. M. (1991). Waste Materials in Urban Soils. Oxford: Blackwell Scientific Publications Ltd, 28–46.
Bronick, C. J., and Lal, R. (2005). Soil Structure and Management: a Review. Geoderma 124 (1-2), 3–22. doi:10.1016/j.geoderma.2004.03.005
Caron, J., Espindola, C. R., and Angers, D. A. (1996). Soil Structural Stability during Rapid Wetting: Influence of Land Use on Some Aggregate Properties. Soil Sci. Soc. America J. 60 (3), 901–908. doi:10.2136/sssaj1996.03615995006000030032x
Chen, Y., Day, S. D., Wick, A. F., and McGuire, K. J. (2014). Influence of Urban Land Development and Subsequent Soil Rehabilitation on Soil Aggregates, Carbon, and Hydraulic Conductivity. Sci. Total Environ. 494-495, 329–336. doi:10.1016/j.scitotenv.2014.06.099
Cui, H., Ou, Y., Lv, D., Wang, L. X., Liang, A. Z., Yan, B. X., et al. (2020). Aggregate-related Microbial Communities and Nutrient Stoichiometry under Different Croplands. Ecol. Process. 9 (1), 9. doi:10.1186/s13717-020-00239-4
de Jonge, L. W., Jacobsen, O. H., and Moldrup, P. (1999). Soil Water Repellency: Effects of Water Content, Temperature, and Particle Size. Soil Sci. Soc. America J. 63 (3), 437–442. doi:10.2136/sssaj1999.03615995006300030003x
DeBano, L. F. (1971). The Effect of Hydrophobic Substances on Water Movement in Soil during Infiltration. Soil Sci. Soc. America J. 35 (2), 340–343. doi:10.2136/sssaj1971.03615995003500020044x
DeBano, L. F. (2000). The Role of Fire and Soil Heating on Water Repellency in Wildland Environments: a Review. J. Hydrol. 231-232, 195–206. doi:10.1016/s0022-1694(00)00194-3
Dekker, L. W., Doerr, S. H., Oostindie, K., Ziogas, A. K., and Ritsema, C. J. (2001). Water Repellency and Critical Soil Water Content in a Dune Sand. Soil Sci. Soc. Am. J. 65 (6), 1667–1674. doi:10.2136/sssaj2001.1667
Díaz, S., Pascual, U., Stenseke, M., Martín-López, B., Watson, R. T., Molnár, Z., et al. (2018). Assessing Nature's Contributions to People. Science 359 (6373), 270–272. doi:10.1126/science.aap8826
Doerr, S. H. (1998). On Standardizing the ,water Drop Penetration Time' and the ,molarity of an Ethanol Droplet' Techniques to Classify Soil Hydrophobicity: A Case Study Using Medium Textured Soils. Earth Surf. Process. Landforms 23 (7), 663–668. doi:10.1002/(sici)1096-9837(199807)23:7<663:aid-esp909>3.0.co;2-6
Doerr, S. H., Shakesby, R. A., and Walsh, R. P. D. (2000). Soil Water Repellency: its Causes, Characteristics and Hydro-Geomorphological Significance. Earth-Science Rev. 51 (1-4), 33–65. doi:10.1016/s0012-8252(00)00011-8
du Toit, M. J., and Cilliers, S. S. (2011). Aspects Influencing the Selection of Representative Urbanization Measures to Quantify Urban-Rural Gradients. Landscape Ecol. 26 (2), 169–181. doi:10.1007/s10980-010-9560-4
Dunnington, D. (2021). Ggspatial: Spatial Data Framework for Ggplot2. R package version 1.1.5. Available at: https://CRAN.R-project.org/package=ggspatial.
Edmondson, J. L., Davies, Z. G., McCormack, S. A., Gaston, K. J., and Leake, J. R. (2011). Are Soils in Urban Ecosystems Compacted? A Citywide Analysis. Biol. Lett. 7 (5), 771–774. doi:10.1098/rsbl.2011.0260
Equiza, M. A., Calvo-Polanco, M., Cirelli, D., Señorans, J., Wartenbe, M., Saunders, C., et al. (2017). Long-term Impact of Road Salt (NaCl) on Soil and Urban Trees in Edmonton, Canada. Urban For. Urban Green. 21, 16–28. doi:10.1016/j.ufug.2016.11.003
Fan, J., Ding, W., and Ziadi, N. (2013). Thirty-Year Manuring and Fertilization Effects on Heavy Metals in Black Soil and Soil Aggregates in Northeastern China. Commun. Soil Sci. Plant Anal. 44 (7), 1224–1241. doi:10.1080/00103624.2012.756002
Garnier, S., Ross, N., Rudis, R., Camargo, P. A., Sciaini, M., and Scherer, C. (2021). Viridis - Colorblind-Friendly Color Maps for R. R package version 0.6.1. Available online at: https://sjmgarnier.github.io/viridis/.
Gerstenberg, J. (2017). 01.06.1 Bodenarten. Available online at: https://www.berlin.de/umweltatlas/boden/bodenkundliche-kennwerte/2015/karten/artikel.948554.php (Accessed 06 18, 2021).
Giovannini, G., and Lucchesi, S. (1983). Effect of Fire on Hydrophobic and Cementing Substances of Soil Aggregates1. Soil Sci. 136 (4), 231–236. doi:10.1097/00010694-198310000-00006
Gregory, J. H., Dukes, M. D., Jones, P. H., and Miller, G. L. (2006). Effect of Urban Soil Compaction on Infiltration Rate. J. Soil Water Conservation 61 (3), 117–124.
Grimm, N. B., Faeth, S. H., Golubiewski, N. E., Redman, C. L., Wu, J., Bai, X., et al. (2008). Global Change and the Ecology of Cities. Science 319 (5864), 756–760. doi:10.1126/science.1150195
Haase, D., and Nuissl, H. (2007). Does Urban Sprawl Drive Changes in the Water Balance and Policy? The Case of Leipzig (Germany) 1870–2003. Landscape Urban Plann. 80 (1), 1–13. doi:10.1016/j.landurbplan.2006.03.011
Hagan, D., Dobbs, C., Timilsina, N., Escobedo, F., Toor, G. S., and Andreu, M. (2012). Anthropogenic Effects on the Physical and Chemical Properties of Subtropical Coastal Urban Soils. Soil Use Manage. 28 (1), 78–88. doi:10.1111/j.1475-2743.2011.00379.x
Hooke, R. L. (2000). On the History of Humans as Geomorphic Agents. Geology 28 (9), 843–846. doi:10.1130/0091-7613(2000)028<0843:othoha>2.3.co;2
Huang, B., Yuan, Z., Li, D., Zheng, M., Nie, X., and Liao, Y. (2020). Effects of Soil Particle Size on the Adsorption, Distribution, and Migration Behaviors of Heavy Metal(loid)s in Soil: a Review. Environ. Sci. Process. Impacts 22 (8), 1596–1615. doi:10.1039/d0em00189a
Jim, C. Y. (1998b). Physical and Chemical Properties of a Hong Kong Roadside Soil in Relation to Urban Tree Growth. Urban Ecosyst. 2 (2), 171–181. doi:10.1023/a:1009585700191
Jim, C. Y. (1998a). Urban Soil Characteristics and Limitations for Landscape Planting in Hong Kong. Landscape Urban Plann. 40 (4), 235–249. doi:10.1016/s0169-2046(97)00117-5
Kassambara, A. (2020). Ggpubr: ',ggplot2' Based Publication Ready Plots. R package version 0.4.0. Available online at: https://CRAN.R-project.org/package=ggpubr.
Kemper, W. D., and Rosenau, R. C. (1986). Aggregate Stability and Size Distribution. Methods Soil Anal. 175, 425–442. doi:10.2136/sssabookser5.1.2ed.c17
Kindler, R., Siemens, J., Kaiser, K., Walmsley, D. C., Bernhofer, C., Buchmann, N., et al. (2011). Dissolved Carbon Leaching from Soil Is a Crucial Component of the Net Ecosystem Carbon Balance. Glob. Change Biol. 17 (2), 1167–1185. doi:10.1111/j.1365-2486.2010.02282.x
Klein Goldewijk, K., Beusen, A., and Janssen, P. (2010). Long-term Dynamic Modeling of Global Population and Built-Up Area in a Spatially Explicit Way: HYDE 3.1. The Holocene 20 (4), 565–573. doi:10.1177/0959683609356587
Korenkova, L., and Matus, P. (2015). Role of Water Repellency in Aggregate Stability of Cultivated Soils under Simulated Raindrop Impact. Eurasian Soil Sci. 48 (7), 754–758. doi:10.1134/s1064229315070054
Kowarik, I. (2020). Herbert Sukopp - an Inspiring pioneer in the Field of Urban Ecology. Urban Ecosyst. 23 (3), 445–455. doi:10.1007/s11252-020-00983-7
Kuhn, M., Jackson, S., and Cimentada, J. (2020). Corrr: Correlations in R. R package version 0.4.3. Available online at: https://CRAN.R-project.org/package=corrr.
Layton, J. B., Skidmore, E. L., and Thompson, C. A. (1993). Winter-associated Changes in Dry-Soil Aggregation as Influenced by Management. Soil Sci. Soc. America J. 57 (6), 1568–1572. doi:10.2136/sssaj1993.03615995005700060029x
Lehmann, A., Zheng, W., Ryo, M., Soutschek, K., Roy, J., Rongstock, R., et al. (2020). Fungal Traits Important for Soil Aggregation. Front. Microbiol. 10, 2904. doi:10.3389/fmicb.2019.02904
Lehmann, A., and Stahr, K. (2007). Nature and Significance of Anthropogenic Urban Soils. J. Soils Sediments 7 (4), 247–260. doi:10.1065/jss2007.06.235
Lehmann, A., Zheng, W., and Rillig, M. C. (2017). Soil Biota Contributions to Soil Aggregation. Nat. Ecol. Evol. 1 (12), 1828–1835. doi:10.1038/s41559-017-0344-y
Lorenz, K., and Lal, R. (2009). Biogeochemical C and N Cycles in Urban Soils. Environ. Int. 35 (1), 1–8. doi:10.1016/j.envint.2008.05.006
Mao, J., Nierop, K. G. J., Dekker, S. C., Dekker, L. W., and Chen, B. (2019). Understanding the Mechanisms of Soil Water Repellency from Nanoscale to Ecosystem Scale: a Review. J. Soils Sediments 19 (1), 171–185. doi:10.1007/s11368-018-2195-9
Matziris, E., Stefanou, S., Papazafeiriou, A. Z., Seilopoulos, D., and Papaioannou, A. (2016). Impacts of Human Activities on Soil Physical Properties of Urban green Areas: a Case Study in Thessaloniki City, Greece. Carpathian J. Earth Environ. Sci. 11 (2), 381–394.
McDonnell, M. J., Hahs, A. K., and Pickett, S. T. A. (2012). Exposing an Urban Ecology Straw Man: Critique of Ramalho and Hobbs. Trends Ecol. Evol. 27 (5), 255–256. doi:10.1016/j.tree.2012.01.009
McGhie, D., and Posner, A. (1981). The Effect of Plant Top Material on the Water Repellence of Fired Sands and Water Repellent Soils. Aust. J. Agric. Res. 32 (4), 609–620. doi:10.1071/ar9810609
Miyata, S., Kosugi, K. i., Gomi, T., Onda, Y., and Mizuyama, T. (2007). Surface Runoff as Affected by Soil Water Repellency in a Japanese cypress forest. Hydrol. Process. 21 (17), 2365–2376. doi:10.1002/hyp.6749
Moll, R. J., Cepek, J. D., Lorch, P. D., Dennis, P. M., Tans, E., Robison, T., et al. (2019). What Does Urbanization Actually Mean? A Framework for Urban Metrics in Wildlife Research. J. Appl. Ecol. 56 (5), 1289–1300. doi:10.1111/1365-2664.13358
Morbidelli, R., Corradini, C., Saltalippi, C., Flammini, A., Dari, J., and Govindaraju, R. S. (2018). Rainfall Infiltration Modeling: A Review. Water 10 (12), 20. doi:10.3390/w10121873
Morel, J. L., Chenu, C., and Lorenz, K. (2015). Ecosystem Services provided by Soils of Urban, Industrial, Traffic, Mining, and Military Areas (SUITMAs). J. Soils Sediments 15 (8), 1659–1666. doi:10.1007/s11368-014-0926-0
Naturschutzgesetz, B. (2013). Section 39: Streusalzverbot. Permanent Link. Available online at: https://gesetze.berlin.de/perma?d=jlr-NatSchGBE2013pP39.
Nero, B. F., and Anning, A. K. (2018). Variations in Soil Characteristics Among Urban Green Spaces in Kumasi, Ghana. Environ. Earth Sci. 77 (8). doi:10.1007/s12665-018-7441-3
Pebesma, E. (2018). Simple Features for R: Standardized Support for Spatial Vector Data. R. J. 10, 439–446. doi:10.32614/rj-2018-009
Perry, T., and Nawaz, R. (2008). An Investigation into the Extent and Impacts of Hard Surfacing of Domestic Gardens in an Area of Leeds, United Kingdom. Landscape Urban Plann. 86 (1), 1–13. doi:10.1016/j.landurbplan.2007.12.004
Pickett, S. T. A., Cadenasso, M. L., Grove, J. M., Nilon, C. H., Pouyat, R. V., Zipperer, W. C., et al. (2001). Urban Ecological Systems: Linking Terrestrial Ecological, Physical, and Socioeconomic Components of Metropolitan Areas. Annu. Rev. Ecol. Syst. 32, 127–157. doi:10.1146/annurev.ecolsys.32.081501.114012
Pietola, L., Horn, R., and Yli-Halla, M. (2005). Effects of Trampling by Cattle on the Hydraulic and Mechanical Properties of Soil. Soil Tillage Res. 82 (1), 99–108. doi:10.1016/j.still.2004.08.004
Plyaskina, O. V., and Ladonin, D. V. (2009). Heavy Metal Pollution of Urban Soils. Eurasian Soil Sc. 42 (7), 816–823. doi:10.1134/s1064229309070138
Potere, D., and Schneider, A. (2007). A Critical Look at Representations of Urban Areas in Global Maps. GeoJournal 69 (1), 55–80. doi:10.1007/s10708-007-9102-z
Pouyat, R. V., Russell-Anelli, J., Yesilonis, I. D., and Groffman, P. M. (2003). “Soil Carbon in Urban forest Ecosystems,” in The Potential of U.S. forest Soils to Sequester Carbon and Mitigate the Greenhouse Effect. Editors J. M. Kimble, L. S. Heath, R. A. Birdsey, and R. Lal (U.S.: CRC Press), 347–362.
Pouyat, R. V., Szlavecz, K., Yesilonis, I. D., Groffman, P. M., and Schwarz, K. (2010). Chemical, Physical, and Biological Characteristics of Urban Soils. Madison, WI: Urban Ecosystem Ecology, 119–152.
R Core Team (2020). R: A Language and Environment for Statistical Computing. Vienna: R Foundation for Statistical Computing.
Ramos, M. C., Nacci, S., and Pla, I. (2003). Effect of Raindrop Impact and its Relationship with Aggregate Stability to Different Disaggregation Forces. Catena 53 (4), 365–376. doi:10.1016/s0341-8162(03)00086-9
Rillig, M. C., Mardatin, N. F., Leifheit, E. F., and Antunes, P. M. (2010). Mycelium of Arbuscular Mycorrhizal Fungi Increases Soil Water Repellency and Is Sufficient to Maintain Water-Stable Soil Aggregates. Soil Biol. Biochem. 42 (7), 1189–1191. doi:10.1016/j.soilbio.2010.03.027
Samtani, N. C. (2006). Soils and Foundations Reference Manual, 1. Washington D.C.: National Highway Institute, US Department of Transportation.
Savage, S. M., Martin, J. P., and Letey, J. (1969). Contribution of Some Soil Fungi to Natural and Heat‐Induced Water Repellency in Sand. Soil Sci. Soc. Am. J. 33 (3), 405–409. doi:10.2136/sssaj1969.03615995003300030021x
Scalenghe, R., and Marsan, F. A. (2009). The Anthropogenic Sealing of Soils in Urban Areas. Landscape Urban Plann. 90, 1–10. doi:10.1016/j.landurbplan.2008.10.011
Scharenbroch, B. C., Lloyd, J. E., and Johnson-Maynard, J. L. (2005). Distinguishing Urban Soils with Physical, Chemical, and Biological Properties. Pedobiologia 49 (4), 283–296. doi:10.1016/j.pedobi.2004.12.002
Silva, A. M. d., Urban, R. C., Manfré, L. A., Brossard, M., and Moreira, M. Z. (2017). Soil Quality Attributes Related to Urbanization in Brazilian Watershed. J. Environ. Eng. Landscape Manage. 25 (4), 317–328. doi:10.3846/16486897.2017.1296451
Six, J., Bossuyt, H., Degryze, S., and Denef, K. (2004). A History of Research on the Link between (Micro)aggregates, Soil Biota, and Soil Organic Matter Dynamics. Soil Tillage Res. 79 (1), 7–31. doi:10.1016/j.still.2004.03.008
Thao, H. T. B., George, T., Yamakawa, T., and Widowati, L. R. (2008). Effects of Soil Aggregate Size on Phosphorus Extractability and Uptake by rice (Oryza sativaL.) and Corn (Zea maysL.) in Two Ultisols from the Philippines. Soil Sci. Plant Nutr. 54 (1), 148–158. doi:10.1111/j.1747-0765.2007.00220.x
Thioulouse, J., Dray, S., Dufour, A., Siberchicot, A., Jombart, T., and Pavoine, S. (2018). Multivariate Analysis of Ecological Data with Ade4. Springer.
Trejo, D., Barois, I., and Sangabriel-Conde, W. (2016). Disturbance and Land Use Effect on Functional Diversity of the Arbuscular Mycorrhizal Fungi. Agroforest Syst. 90 (2), 265–279. doi:10.1007/s10457-015-9852-4
Tzoulas, K., Korpela, K., Venn, S., Yli-Pelkonen, V., Kaźmierczak, A., Niemela, J., et al. (2007). Promoting Ecosystem and Human Health in Urban Areas Using Green Infrastructure: A Literature Review. Landscape Urban Plann. 81 (3), 167–178. doi:10.1016/j.landurbplan.2007.02.001
United Nations, (2018). 2018 Revision of World Urbanization Prospects. Available at: https://www.un.org/development/desa/publications/2018-revision-of-world-urbanization-prospects.html (Accessed November 20, 2021)
van Bavel, C. H. M. (1950). Mean Weight-Diameter of Soil Aggregates as a Statistical Index of Aggregation. Soil Sci. Soc. America J. 14 (C), 20–23. doi:10.2136/sssaj1950.036159950014000c0005x
Vogelmann, E. S., Reichert, J. M., Prevedello, J., Awe, G. O., and Mataix-Solera, J. (2013). Can Occurrence of Soil Hydrophobicity Promote the Increase of Aggregates Stability. Catena 110, 24–31. doi:10.1016/j.catena.2013.06.009
von der Lippe, M., Buchholz, S., Hiller, A., Seitz, B., and Kowarik, I. (2020). CityScapeLab Berlin: A Research Platform for Untangling Urbanization Effects on Biodiversity. Sustainability 12 (6), 30. doi:10.3390/su12062565
von der Lippe, M., Fiechter, L., Hiller, A., and Whitehead, J. (2021). Data from: Environmental Data Used for PCA, and Site Age. Figshare. doi:10.6084/m9.figshare.16811701
Whitehead, J., Hempel, S., Hiller, A., von der Lippe, M., and Rillig, M. C. (2021). Data from: Soil Physico-Chemical Data and PCA axis Scores. Figshare. doi:10.6084/m9.figshare.16775701
Wilpiszeski, R. L., Aufrecht, J. A., Retterer, S. T., Sullivan, M. B., Graham, D. E., Pierce, E. M., et al. (2019)
Xu, L., Cui, H., Zheng, X., Zhou, J., Zhang, W., Liang, J., et al. (2017). Changes in the Heavy Metal Distributions in Whole Soil and Aggregates Affected by the Application of Alkaline Materials and Phytoremediation. RSC Adv. 7 (65), 41033–41042. doi:10.1039/c7ra05670b
Yang, J.-L., and Zhang, G.-L. (2011). Water Infiltration in Urban Soils and its Effects on the Quantity and Quality of Runoff. J. Soils Sediments 11 (5), 751–761. doi:10.1007/s11368-011-0356-1
Keywords: urban soil, soil aggregates, infiltration, hydrophobicity, particle size
Citation: Whitehead J, Hempel S, Hiller A, von der Lippe M and Rillig MC (2021) Soil Physico-Chemical Properties Change Across an Urbanity Gradient in Berlin. Front. Environ. Sci. 9:765696. doi: 10.3389/fenvs.2021.765696
Received: 27 August 2021; Accepted: 21 October 2021;
Published: 03 November 2021.
Edited by:
Rosa Francaviglia, Council for Agricultural and Economics Research (CREA), ItalyReviewed by:
Riccardo Scalenghe, University of Palermo, ItalyCopyright © 2021 Whitehead, Hempel, Hiller, von der Lippe and Rillig. This is an open-access article distributed under the terms of the Creative Commons Attribution License (CC BY). The use, distribution or reproduction in other forums is permitted, provided the original author(s) and the copyright owner(s) are credited and that the original publication in this journal is cited, in accordance with accepted academic practice. No use, distribution or reproduction is permitted which does not comply with these terms.
*Correspondence: Matthias C. Rillig, cmlsbGlnQHplZGF0LmZ1LWJlcmxpbi5kZQ==
Disclaimer: All claims expressed in this article are solely those of the authors and do not necessarily represent those of their affiliated organizations, or those of the publisher, the editors and the reviewers. Any product that may be evaluated in this article or claim that may be made by its manufacturer is not guaranteed or endorsed by the publisher.
Research integrity at Frontiers
Learn more about the work of our research integrity team to safeguard the quality of each article we publish.