- 1Department of Ecology and Evolutionary Biology, School of Biological Sciences, The University of Adelaide, Adelaide, SA, Australia
- 2Australian Rivers Institute, Griffith University, Nathan, QLD, Australia
- 3Southern Seas Ecology Laboratories, School of Biological Sciences and The Environment Institute, The University of Adelaide, Adelaide, SA, Australia
- 4Water Studies, School of Chemistry, Monash University, Melbourne, VIC, Australia
- 5Centre for Water and Spatial Science, UWA School of Agriculture and Environment, The University of Western Australia, Perth, WA, Australia
- 6Marine Data Technology Hub, College of Science and Engineering, James Cook University, Townsville, QLD, Australia
Estuaries host unique biodiversity and deliver a range of ecosystem services at the interface between catchment and the ocean. They are also among the most degraded ecosystems on Earth. Freshwater flow regimes drive ecological processes contributing to their biodiversity and economic value, but have been modified extensively in many systems by upstream water use. Knowledge of freshwater flow requirements for estuaries (environmental flows or E-flows) lags behind that of rivers and their floodplains. Generalising estuarine E-flows is further complicated by responses that appear to be specific to each system. Here we critically review the E-flow requirements of estuaries to 1) identify the key ecosystem processes (hydrodynamics, salinity regulation, sediment dynamics, nutrient cycling and trophic transfer, and connectivity) modulated by freshwater flow regimes, 2) identify key drivers (rainfall, runoff, temperature, sea level rise and direct anthropogenic) that generate changes to the magnitude, quality and timing of flows, and 3) propose mitigation strategies (e.g., modification of dam operations and habitat restoration) to buffer against the risks of altered freshwater flows and build resilience to direct and indirect anthropogenic disturbances. These strategies support re-establishment of the natural characteristics of freshwater flow regimes which are foundational to healthy estuarine ecosystems.
1 Introduction
In many aquatic ecosystems, the freshwater flow regime, defined as the quality, quantity and timing of flows (Kotzé, 2016), is regarded as the key variable shaping ecosystem processes (Bunn and Arthington, 2002; Poff and Zimmerman, 2010). Any modification to the delivery of freshwater flows has an impact on the functioning of aquatic ecosystem processes and associated biological, chemical or physical responses (Van Niekerk et al., 2012). Importantly, flow modifications that elicit major responses threaten the ability of an ecosystem to support and maintain a diverse and resilient community of organisms (Andreasen et al., 2001; Adams, 2014).
The flow regime influences ecosystem processes from the headwaters of rivers and their catchments through to the marine environment, and sometimes as far as the continental shelf (Jutras et al., 2020; Liang et al., 2020). For example, slow-flowing headwaters provide adequate duration for organic matter decomposition and delivery of nutrient-rich water into the ocean, stimulating productivity over the continental shelf (McClelland et al., 2012). At the end of river catchments where freshwaters transition into the sea, estuaries form an important conduit between marine, freshwater and terrestrial realms (Gillanders et al., 2011; Arthington, 2012; Adams et al., 2016a; Kotzé, 2016). Strong physical, chemical and biological gradients are generated by site-specific interactions of inflowing saline and freshwater sources (Thrush et al., 2013; Kim et al., 2017) which drive estuarine ecosystem processes (Figure 1), such as salinity stratification, sediment erosion, flocculation and deposition, and the cycling of nutrients. These processes interact over a variety of spatial and temporal scales driven by variations of freshwater flow (Belmar et al., 2019; Clark and O’Connor, 2019). Because they have sharp environmental gradients, estuaries are complex systems hosting a diversity of habitats (e.g., open water, seagrass beds, mudflats, mangrove forests) that support a large diversity of organisms (Barbier et al., 2011; Pinto and Marques, 2015).
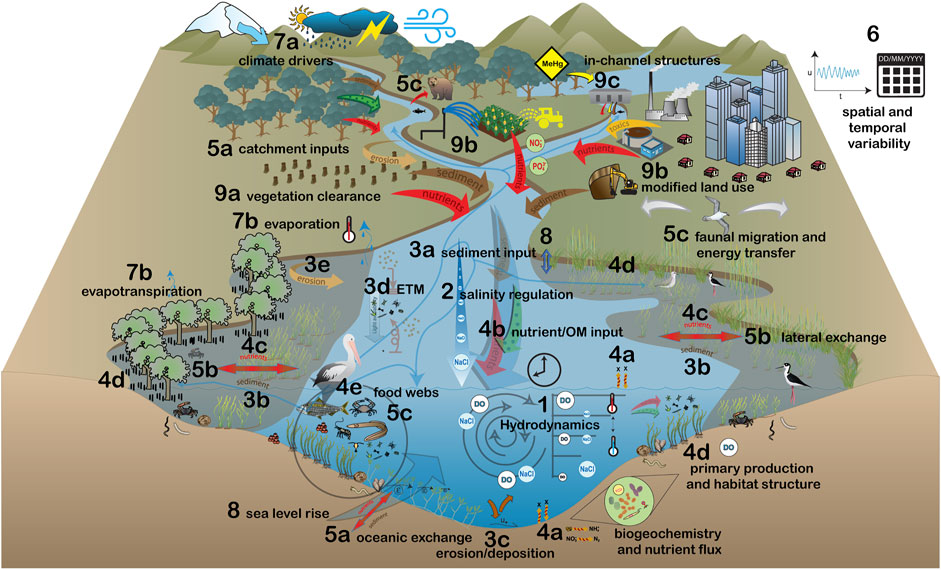
FIGURE 1. Conceptual diagram demonstrating the influences of freshwater flows on important estuarine ecosystem processes: 1) hydrodynamics, 2) salinity regulation, 3a–e) sediment dynamics (a: catchment sediment input, b: lateral sediment exchange, c: mouth erosion/deposition, d: estuarine turbidity maximum (ETM), e: lateral erosion), 4a–e) nutrient cycling and trophic transfer (a: biogeochemical processing and exchange between sediments, open water and atmosphere, b: catchment nutrient and organic matter (OM) inputs, c: lateral nutrient and energy exchange, d: primary production, e: food webs), and 5a–c) hydrological connectivity (a: longitudinal connectivity, b: lateral connectivity, c: energy transfer and faunal migrations), 6) spatial and temporal variability, and the drivers which change the influence of freshwater flows upon the ecosystem processes: 7a-b) climate (a: rainfall, temperature, snowmelt, storms and droughts, b: evaporation and evapotranspiration, 8) sea level rise and 9a–c) direct anthropogenic drivers (a: vegetation clearance, b: land use modification, c: in-channel structures).
Despite their ecological significance, estuaries are some of the most degraded ecosystems on Earth (Gillanders et al., 2011; Vermeiren and Sheaves, 2014; Kotzé, 2016). This degradation is often rapid, due to their susceptibility from both coastal and catchment pressures induced by climate change and direct anthropogenic stressors (Figure 1; Waltham and Sheaves, 2015). The widespread degradation of estuaries is notably caused due to eutrophication (Pinckney et al., 2001; Davis and Koop, 2006; Maier et al., 2009; Howarth et al., 2011) and has been the focus of research for many decades (e.g., Barlow et al., 1963; Caperon et al., 1971; Livingston, 1996). However, we also highlight the degradation estuaries have experienced as a consequence of modifications to freshwater flow regimes (Arthington, 2012; Kiwango et al., 2015; Stein et al., 2021), resulting in a decline in estuarine habitat quality due to altered ecosystem processes (Pinckney et al., 2001; Mbandzi et al., 2018). This can induce problems, such as eutrophication (see Section 4), due to biological responses (e.g., algal blooms, seagrass dieback) associated with modified ecosystem structure and function (Cottingham et al., 2018; Scharler et al., 2020).
Freshwater flow requirements to support fully functional, healthy estuaries are largely ignored compared with those of river and floodplain environments (Peñas et al., 2013; Adams, 2014; Kiwango et al., 2015; Van Niekerk et al., 2019b; Stein et al., 2021). This has been attributed to a lack of understanding of both the mechanisms which drive estuarine ecological functioning (Gippel et al., 2009; Peñas et al., 2013) and the responses to changes in flow (Gippel et al., 2009; Peñas et al., 2013; Adams, 2014; Van Niekerk et al., 2019b), both of which are attributable to a lack of long-term data (Peñas et al., 2013; Van Niekerk et al., 2019b). Where E-flow strategies have been implemented, they are usually tailored to individual estuaries and general principles and lessons learnt have not been synthesised to provide transferability to other estuary types with different geomorphological or hydrological characteristics (Taljaard et al., 2004; Peñas et al., 2013).
Here we seek to address the issue of specificity of E-flow applications to individual estuaries by considering the major underlying mechanisms that govern estuarine dynamics. We avoided focusing on specific resources or ecosystem states (Van Niekerk et al., 2019b) for the purpose of providing a general conceptual understanding of how E-flow requirements vary among estuaries. A critical review of the literature was undertaken to:
1) Identify key ecological processes influenced by the freshwater flow regime (hydrodynamics, salinity regulation, sediment dynamics, nutrient cycling and trophic transfer, and connectivity),
2) Identify key drivers (rainfall, runoff, temperature, sea level rise and direct anthropogenic) that generate changes to the magnitude, quality and timing of freshwater flows, and
3) Propose how direct and indirect anthropogenic alterations to these key drivers can be mitigated to buffer against the risks of altered freshwater flows and maintain ecological resilience of estuaries.
Our synthesis can help guide estuarine catchment management to define appropriate freshwater flow strategies and limits of acceptable change in the face of current and future climate and direct anthropogenic pressures including climate change.
2 Key Estuarine Ecosystem Processes
The major processes driving estuarine ecosystems are hydrodynamics (e.g., water circulation, mixing and flushing), salinity regulation, sediment dynamics (e.g., sediment delivery, deposition and erosion), nutrient cycling and trophic transfer, and hydrological connectivity (e.g., longitudinal and lateral exchange of water; #1–5, Figure 1). These processes are driven by the interactions of freshwater flow and tides, which modify the physical structure of the estuary, biogeochemical transformations and the behaviour of organisms (Thrush et al., 2013; Belmar et al., 2019). Variability in physical and biogeochemical process pathways creates a wide range of ecological niches and unique patterns of connectivity between them. This supports biodiversity (Sun et al., 2013; Sun et al., 2015), and many organisms have evolved life history traits tuned to the wide variations in physicochemical conditions (Sun et al., 2015; Zhang M. et al., 2017; Duggan et al., 2019; Izegaegbe et al., 2020).
2.1 Hydrodynamics
Freshwater flows influence the estuary hydrodynamics, i.e., the circulation of water and associated hydrologic transport and mixing of constituents (#1 Figure 1; Wolanski and Elliot, 2016). The degree to which flow affects estuarine hydrodynamics is mediated by the local tidal regime, the geomorphology of the estuary and local climate, such as the predominant wind speed and direction (Goodrich et al., 1987; Scully, 2010). Substantial freshwater flow can destabilise vertical stratification and ultimately flush out brackish water (Scharler et al., 2020). Conversely, when freshwater flow is small, it may not prevent seawater ingress, with freshwater remaining largely intact as a buoyant overflow above the saline water derived from the ocean (Ortmann et al., 2011; Cloern et al., 2017). Salinity stratification, characterised by a salt wedge, is often associated with a turbidity maximum (#3d Figure 1) and anoxic deep waters as the density stratification isolates the deeper waters from the atmosphere and largely negates atmospheric re-aeration (Bruce et al., 2014; Wolanski and Elliot, 2016). Importantly, there is enormous variability generated by the mixing of fresh and salt waters, flows operating at multiple temporal scales (interannual, seasonal, diurnal, tidal and sub-tidal) and spatial variability from the furthest marine influence (water level variations or saline intrusion) to the estuary mouth, often encompassing major geomorphological changes that both influence, and are influenced by the estuary hydrodynamics.
The residence time of water in an estuary (inversely related to the flushing rate, #1 Figure 1) is strongly influenced by freshwater flow (Wolanski et al., 2006; Huang et al., 2020). It affects the distribution of salinity, dissolved oxygen and resident organisms, sedimentation rates of particulates, processing times of nutrients, contaminants (e.g., toxins, heavy metals) and pathogens, and contaminant exposure risk to resident organisms (Cottingham et al., 2018; Clark and O'Connor, 2019; Fonseca et al., 2020). Residence time can be highly variable across a range of time scales, from interannual to tidal, and modified by estuarine morphology.
2.2 Salinity Regulation
The length of an estuary is defined by the furthest point of tidal influence where saline water penetrates from the mouth upstream to the point of inflowing freshwater (#2 Figure 1; Kim et al., 2017). Typically, a longitudinal salinity gradient runs from the upstream areas where freshwater enters the estuary to marine conditions at the mouth. Where estuaries receive little freshwater, this salinity gradient can sometimes be reversed (i.e., an inverse estuary, where the salinity is lowest at the mouth and increases with distance upstream; Sheaves, 1996; 1998; Potter et al., 2010). Varying salinity generated by inflowing freshwater provides a basis for estuary classification and biological community composition, as salinity is a key determinant of species distributions (Doering et al., 2002; Kanaya et al., 2011; Arthington, 2012; Peñas et al., 2013; Lee and Kuhn, 2019). Seasonal changes in salinity driven by variations in freshwater discharge result in shifts in biological communities (Collocott et al., 2014), promoting species diversity by controlling dominant species, such as the mangrove Kandelia obovate in the Tanshui River estuary, Taiwan, allowing for succession (Shih et al., 2011). These fluctuations may also facilitate adaptation to highly varying salinity conditions, promoting species with wide distributions and competitive advantages (Sheaves, 1998). Important life cycle events, such as the reproduction and recruitment of fishes, jellyfish, shrimp, crabs and prawns (Sun et al., 2013; Sun et al., 2015) and the germination of macrophyte seedlings (Kim et al., 2013; Kim et al., 2015) are triggered by seasonal shifts in salinity. Furthermore, these shifts can maintain a greater phytoplankton biodiversity and promote carbon and nutrient transport via phytoplankton (Ortmann et al., 2011). Large flow events (e.g., 10-year or 100-year floods) can benefit estuarine biodiversity, promoting high phytoplankton productivity as freshwater flows subside (Steichen et al., 2020), as well as favouring opportunistic microbenthic species over the incumbent dominant species (Izegaegbe et al., 2020). However, there are often adverse effects of large flow events on estuaries (Osburn et al., 2019; Hu et al., 2020), such as changes to geomorphology and salinity, which can cause stress to and mortality of organisms through increased flushing and osmotic stress (Park et al., 2014).
2.3 Sediment Dynamics
The delivery, deposition and erosion of sediments in estuaries shape their geomorphology (Kench, 1999). Freshwater inflows transport sediment particulate material to estuaries (#3a Figure 1) that settles out in areas of low velocity (#3b Figure 1; Russ and Palinkas, 2020). Settling rates can be enhanced by flocculation associated with increasing salinity (#2 Figure 1; Yan et al., 2020). Sediment delivery is important for building habitat structure in estuaries (#4d Figure 1; Le Pape et al., 2013; Li et al., 2019). The deposition of fine-grained particles allows for colonisation by plants (e.g., saltmarsh, mangroves) and infauna (e.g., polychaetes; Le Pape et al., 2013; Sottolichio et al., 2013; Sampath and Boski, 2016; Li et al., 2019; Adams, 2020).
Flow-driven resuspension of particles (#3c and #3d Figure 1) in addition to tidal currents, wind-induced surface waves and internal waves scour and erode sediments and sand bars (Adams et al., 2016b; Lund-Hansen et al., 1999). Flow-induced resuspension of recently deposited sediments can stimulate primary productivity and establish an estuarine turbidity maximum (ETM, #3d Figure 1; Wolanski et al., 2006; Yan et al., 2021). The ETM can be important for fish species by providing light contrast needed to detect prey (Hasenbein et al., 2013) or reducing light to avoid predators during juvenile life stages (Stewart et al., 2020).
Freshwater flow interacts with marine sediments at the mouth of estuaries. Turbulent wave action in the coastal surf zone resuspends sediments which are transported by flood tides and deposited in the mouths of estuaries, forming sand bars (Webster 2010; Whitfield et al., 2012). Scouring by freshwater flows can reduce sand bar development and maintain a connection to the sea (#3c Figure 1; Kjerfve, 1994; Webster, 2010; Whitfield et al., 2012). An open connection to the sea allows for flushing of sediments, nutrients and contaminants out of the estuary (Adams et al., 2020). Scouring of bank sediments (#3e Figure 1) can facilitate control of invasive macrophytes and maintain channel width and open water habitat (Belmar et al., 2019).
Sediment delivery, deposition and erosion dynamics are important processes within estuaries due to their strong influence on the geomorphology, water quality and habitat availability, and freshwater flows are critical to their provision. The delivery of macronutrients, nitrogen (N) and phosphorus (P) by freshwater flows are similarly important to estuarine ecosystem functioning.
2.4 Nutrient Cycling and Trophic Transfer
Freshwater flows affect processing rates of materials and energy flows within estuaries (Vinagre et al., 2011a; Shen et al., 2019). The mixing of fresh and saline water influences biogeochemical processes (#4a Figure 1) through controls on elemental concentrations via geochemical processes (e.g., flocculation, adsorption, desorption, precipitation, dissolution and redox fronts) and uptake, storage and transformation by microorganisms (Jensen et al., 1995; Conley, 2000; Gaonkar and Matta, 2019).
The dual role of estuaries as carbon sink and source is mediated by freshwater flows (Gorman et al., 2020; Jutras et al., 2020). River discharge promotes flushing (#1 Figure 1) of organic matter to adjacent marine waters which may stimulate offshore productivity and support commercial fisheries (Shen et al., 2019). As a carbon sink, estuaries are considered efficient “filters” that trap and process a large fraction of catchment-derived organic carbon delivered by inflowing freshwater (#4b Figure 1) and store carbon in the sediments via burial (#4a and c Figure 1; Hu et al., 2020; Wu et al., 2020). Primary producers (#4d Figure 1) take up and store carbon via photosynthesis along with bioavailable nutrients (Liang et al., 2020). Large vegetation, such as seagrass beds and mangrove forests, provide long-term carbon storage through their large standing stock (Thrush et al., 2013) and is influenced by sediment deposition (#3c Figure 1; Krauss et al., 2014) and salinity distribution (#2 Figure 1; Krauss et al., 2014; Riddin and Adams, 2010).
Allochthonous organic matter and nutrients (#4b Figure 1) are delivered by freshwater flows, stimulating primary productivity (#4d Figure 1) with flow-on effects through the food web both within the estuary (#4d Figure 1; Piazza and La Peyre, 2012; Le Pape et al., 2013; Ruibal-Conti et al., 2013; Dan et al., 2019; Vinagre et al., 2019) and in the nearshore coastal environment (Porter et al., 2010; Niemistö and Lund-Hansen, 2019). Productivity is enhanced by flows via a number of mechanisms. Vertical mixing (#1 Figure 1) can increase the flux of nutrients from the sediments to the water column, which can then be redistributed by baroclinic cycling and transported into offshore coastal waters (Markull et al., 2014). Conversely, increased water column stratification (#1 Figure 1) from freshwater inputs can lead to decreased oxygen concentrations in the lower water layer, leading to increased fluxes of ammonium from the sediments (#4a Figure 1). These conditions have been found to stimulate flagellate (dinoflagellates, cryptophytes, chrysophytes, prymnesiophytes, euglenophytes and prasinophytes) production, which has in turn been suggested to favour trophic transfer to zooplankton and reduce phytoplankton which dominate under low-flow conditions (McNaughton, 2018). Low oxygen concentrations also increase nitrogen and phosphorus cycling rates from the benthos, which can act as a positive feedback maintaining the persistence of extensive hypoxia in bottom waters (Conley et al., 2009; Testa and Kemp, 2012). Enhanced productivity as a result of catchment-derived nutrient inputs can persist for several months after flows have receded (Vinagre et al., 2011a; Vinagre et al., 2011b; Dias et al., 2016).
2.5 Hydrological Connectivity
Hydrological connectivity can be generated by freshwater flows in two planes; longitudinally (#5a Figure 1) from catchment to ocean, and laterally from exchanges with intertidal and littoral habitats, and adjacent wetlands (#5b Figure 1; Duggan et al., 2019). Longitudinal connectivity is promoted by open mouth connections to the ocean that allow passage for motile organisms (Drinkwater and Frank, 1994) which need access to important estuarine breeding and nursery habitat, such as penaeid prawns (Duggan et al., 2019) and diadromous fish species (Nordlie, 2003; Milton, 2009; Pasquaud et al., 2015; Merg et al., 2020; Scharler et al., 2020), in addition to species which migrate to the marine environment after spending time in the estuary (Drinkwater and Frank, 1994; Milton, 2009; Pasquaud et al., 2015).
Lateral connection to intertidal habitats and adjacent coastal wetlands has benefits for pelagic and intertidal organisms (Clark and O’Connor, 2019) by allowing accessibility to habitat and food resources, and stimulating benthic primary production (Vinagre et al., 2011b; Piazza and La Peyre, 2011; Raman et al., 2020). For example, in northern Australia, wet-season flows connect habitats laterally (Waltham et al., 2019), promoting fish larval recruitment (Godfrey et al., 2017) and stimulating productivity of fisheries (Duggan et al., 2019).
Freshwater flows facilitate connectivity through resource provision for terrestrial animals and birds from flow-stimulated primary and secondary productivity (#5c Figure 1; Belmar et al., 2019). This links estuaries to habitats further inland via terrestrial fauna migrations (Kiwango et al., 2015), and to other ecosystems at local, regional and continental scales via bird migrations (Buelow and Sheaves, 2015). The habitat requirements of migrating organisms are often shaped by freshwater flows (Schrandt et al., 2015). For example, spring freshwater flows decrease salinity in the Fraser River Estuary, Canada, enabling development of microalgal biofilms high in lipids, which are key energy-rich food items for the migrating western sandpipers (Calidris mauri; Schnurr et al., 2020).
3 Drivers of Change
3.1 Changes in Flow to Estuaries
Climate and direct anthropogenic forcing are changing freshwater flow regimes in non-uniform ways around the globe (Haddeland et al., 2014; Greve et al., 2018; Gudmundsson et al., 2021). Both the flow magnitude (e.g., volume) and distribution (e.g., low, average and high flows) are changing (Gudmundsson et al., 2019). Flow is decreasing in many river-catchments (Table 1) and generally across regions (Table 2) due to in-channel engineering structures, over-extraction and reduced precipitation, whilst increasing in some rivers and regions due to increased rainfall and reduced snowpack attributable to a changing climate (Gudmundsson et al., 2019; Gudmundsson et al., 2021). At the regional scale, the change in direction of the flow distribution is consistent whereby minimum flows (minimum and 10th percentile), average flows (mean and median) and high flows (maximum and 90th percentile) tend to be either all increasing or decreasing (Gudmundsson et al., 2019). However, at the catchment scale, variability in the direction of changes has been observed between flow indices (Douglas et al., 2000) and the same flow indicator at different parts of the catchment (Fleming et al., 2020). Recent declines in freshwater flows have been observed in southern Australia (Zhang et al., 2016; Huang et al., 2020), the Mediterranean (Haddeland et al., 2014; Greve et al., 2018), southern Africa (Haddeland et al., 2014) and southern Asia (Mondal and Mujumdar, 2012). Conversely, increases have been observed for rivers flowing to the Arctic Ocean (Durocher et al., 2019), northern Europe (Haddeland et al., 2014; Greve et al., 2018) northern Asia (Tananaev et al., 2016) and northern North America (Durocher et al., 2019).

TABLE 1. Changes in flow volumes reaching the sea from river-catchments and regions over the past century as a result of climate change and direct anthropogenic catchment modifications. Multiple volume change values denote a range.
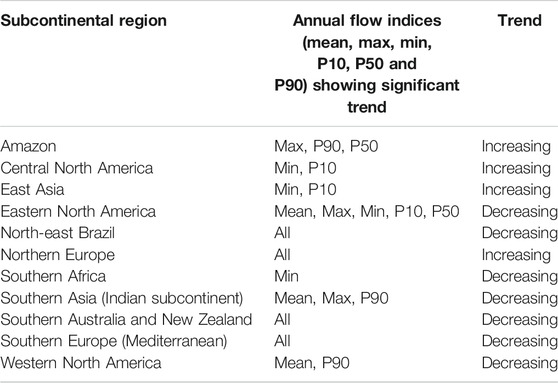
TABLE 2. Significant trends in regional streamflow from around the world, between 1971 and 2010. Annual flow indices are: mean (mean flow), min (minimum flow), max (maximum flow), P10 (10th percentile flow volume), P50 (50th percentile or the median flow volume) and P90 (90th percentile flow volume; Gudmundsson et al., 2019).
3.2 Changing Hydro-Climatological Regimes
River flow regimes are largely determined by meteorological processes (e.g., precipitation from rainfall or snowfall, and air temperature, which affect snowmelt and evaporation rates; #7a and b Figure 1), and by human alterations to watercourses (e.g., dams and water extraction; Leblanc et al., 2012; Zeiringer et al., 2018, as well as straightening and channelisation). Urbanisation affects the volume and quality of river flow from increases in impervious surfaces and modification of rainfall-runoff ratios and water quality (McGrane, 2016; Strohbach et al., 2019; Wałęga et al., 2019) as well as increases in temperature.
Seasonal peaks in flow (#6 Figure 1) can vary with latitude, altitude and the degree of river regulation (Haines et al., 1988; Naiman et al., 2008; Zeiringer et al., 2018). Glacial regimes tend to be characterised by high summer flow and diurnal peaks from air temperature increasing glacial melt during the day (Zeiringer et al., 2018; Durocher et al., 2019). Nival regimes are similar to glacial regimes but mostly occur in lower altitude areas with spring peaks of flow in response to glacial melt (Zeiringer et al., 2018). Pluvial regimes occur in the temperate and arid to semi-arid zones and tend to be stochastic and unpredictable, associated with sporadic rainfall events (Naiman et al., 2008) interspersed by prolonged dry periods (Loik et al., 2004; Bunn et al., 2006; Datry et al., 2018). The tropics are characterised by distinct wet and dry seasons (Warfe et al., 2011). Life histories of estuarine organisms have evolved to allow adaptation to these diverse flow regimes (Lytle and Poff, 2004).
Seasonal demand for irrigation water, changes in rainfall distribution and increased glacial melt shift the timing and magnitude of peak flow (IPCC, 2014; Rottler et al., 2020). With climate change, flows are predicted to increase due to greater rainfall, particularly in high-latitude arctic regions with glacial and nival hydrological regimes. Flow may be approximately 30% greater while ice cover may decrease by 50–80% by the end of the 21st century as a result of increased air temperature and reduced snowfall (Andersson et al., 2015). In contrast, mid-latitude temperate regions and the wet-dry tropics may experience an overall drying trend, with a decrease in mean precipitation and more frequent and prolonged heat waves (Greve et al., 2018).
An increase in global temperature of 0.85°C between 1880 and 2012 has led to greater evaporation and evapotranspiration rates (#7b Figure 1), reducing the volume of water delivered to estuaries (Nijssen et al., 2001; IPCC, 2014). Floods and droughts are predicted to become more severe and frequent with climate change (IPCC, 2014), altering flows (Gillson and Suthers, 2012). Storms can potentially deliver the annual average inflow to an estuary within a day or two (Steichen et al., 2020). Conversely, droughts significantly reduce flows to estuaries, causing serious hydrological imbalance (Ibáñez and Caiola, 2013; Brookes et al., 2015; Dittmann et al., 2015; Leterme et al., 2015). Storms and droughts are intrinsic components of natural flow regimes and can maintain biodiversity over evolutionary time scales as organisms adapt to them (Lytle and Poff, 2004; Naiman et al., 2008). However, they may be detrimental if their frequency or magnitude does not allow system recovery (Thrush et al., 2008). Precipitation and evaporation interact with the bio-geophysical characteristics of the catchment (e.g., drainage area, elevation, topography, drainage network patterns, soil type, soil moisture content, vegetation type and cover, human land use type and cover) to determine the volume of runoff or groundwater recharge reaching estuaries (Ruibal-Conti et al., 2013).
3.3 Sea Level Rise
Sea levels (#8 Figure 1) rose by an average 2 mm/year around the globe between 1971 and 2010 (IPCC, 2014) but between 1993 and 2012, rates of increase of 8–14 mm/year were recorded in the western Pacific Ocean, along with the coastal regions of south-east Asia, eastern Japan and north-eastern Australia (Church et al., 2013). Western coastlines of North and South America and parts of the eastern coastline of the United States are less affected by sea level rise (Church et al., 2013). Sea level rise alters the geomorphological structure and physicochemical characteristics of estuaries (Kimmerer and Weaver, 2013; Arellano et al., 2019; Khojasteh et al., 2021). Seawater is likely to intrude further inland, particularly where elevation gradients are low and where there are reductions in freshwater flows (Payne et al., 2019; Khojasteh et al., 2021). This may threaten freshwater habitat and water for human consumption (Hong et al., 2014; Haddout and Maslouhi, 2018; Wang and Hong, 2021). Increased salinity may also increase density stratification and persistence of salt wedges (Krvavica et al., 2017), leading to anoxia of bottom sediments and loss of benthic biota (Kimmerer and Weaver, 2013). Where littoral structures (e.g., levees) have been erected, intertidal habitat areas are likely to reduce in size as marine water intrudes up to these barriers (Colombano et al., 2021; Khojasteh et al., 2021). This has implications for intertidal species distributions and associated organisms due to excessive inundation and salinity (Smith et al., 2009; Payne et al., 2019). These impacts may vary depending upon the degree of sea level rise, which is non-uniform globally, although sea level will increase in 95% of the world’s ocean by 2100 (Church et al., 2013; IPCC, 2014).
3.4 Direct Anthropogenic Drivers
Globally, only a small fraction of catchments, covering a mere 0.16% of the Earth’s surface, are unaffected by human activities, and few rivers retain natural flow regimes (Vörösmarty et al., 2010). There are multiple demands for freshwater resources, reducing water volumes of streams and wetlands (Gillanders and Kingsford, 2002; Vörösmarty et al., 2010; Abbott et al., 2019; Dudgeon, 2019). Alterations to watercourses include the clearing of native vegetation (#9a Figure 1) for agricultural, industrial and urban land use (#9b Figure 1), and the development of in-channel structures (#9c Figure 1) to capture and divert water for irrigation, hydropower and consumptive use (Bunn et al., 2014). These activities change the quantity (Vörösmarty and Sahagian, 2000; Alber, 2002; Kingsford et al., 2011), quality (Liu M. et al., 2019) and timing of flows (Cai et al., 2019).
3.4.1 Water Diversions and In-Channel Structures
Of the 31% of total global runoff that is accessible to humans, more than half is either extracted or withheld behind in-channel structures (Postel et al., 1996). Approximately 60% of the world’s freshwater storage is behind dams, equating to five times the volume of the Earth’s rivers (Vörösmarty and Sahagian, 2000). Dams change the timing and magnitude of flow, and also affect longitudinal connectivity between catchments and rivers, posing a barrier to the transport of water, sediment, organic matter and nutrients, and the upstream and downstream movements of organisms (Poff et al., 2007; Bunn et al., 2014; Chen et al., 2016). This is highlighted by the negative impacts of dam construction for reproduction and abundance of anadromous and catadromous fishes (Drinkwater and Frank, 1994). Dams may also cause severe water quality problems through methyl-mercury (MeHg) production associated with stratification and anoxia, with MeHg delivered to estuaries on release of water (Hsu-Kim et al., 2018). Inter-basin transfers, where water is shifted between catchments, is driven by human water requirements for agriculture and energy, and exacerbates changes to the flow regime in both the donor (Micklin, 1988) and recipient water catchments (Shumilova et al., 2018; Yu et al., 2018; Dudgeon, 2019). These transfers may also introduce foreign material and organisms to the donor basin (Yu et al., 2018; Dudgeon, 2019).
3.4.2 Vegetation Clearance and Land-Use Modification
In undisturbed catchments, nutrient transport is regulated by the volume of freshwater runoff and the type and extent of vegetation within the catchment (Harris, 2001; Adams et al., 2020). The majority of nutrients exported in freshwater flows are in organic form (e.g., DON; Conley, 2000; Harris, 2001). However, as catchments are modified and the vegetation is cleared, nutrient export increases (Adams et al., 2020) and the ratio of bioavailable to total nutrients increases (Harris, 2001), with concomitant changes in nutrient ratios (Conley, 2000). Wide-scale catchment vegetation clearance and human industrial activities (e.g., mining) may also be responsible for increased sediment loads due to soil disturbance (Thrush et al., 2004; Norkko et al., 2006). Furthermore, rivers are often used for waste disposal contributing nutrients, contaminants and pollutants from agricultural, industrial and urban settings (Van Niekerk et al., 2019a; Gaonkar and Matta, 2019; Robins et al., 2019).
4 Risks to Ecosystem Functioning Under Modified Flow
Increases in flow (Figure 2), decreases in flow (Figure 3) and changes to the natural timing of flow (Figure 4) affect estuarine hydrodynamics, salinity, water quality, biogeochemical cycling and geomorphology, and alter the suitability of habitat for resident and migratory organisms that have adapted to the natural variations of the flow regime (Arthington, 2012; Sun et al., 2015; Zhang H. et al., 2017).
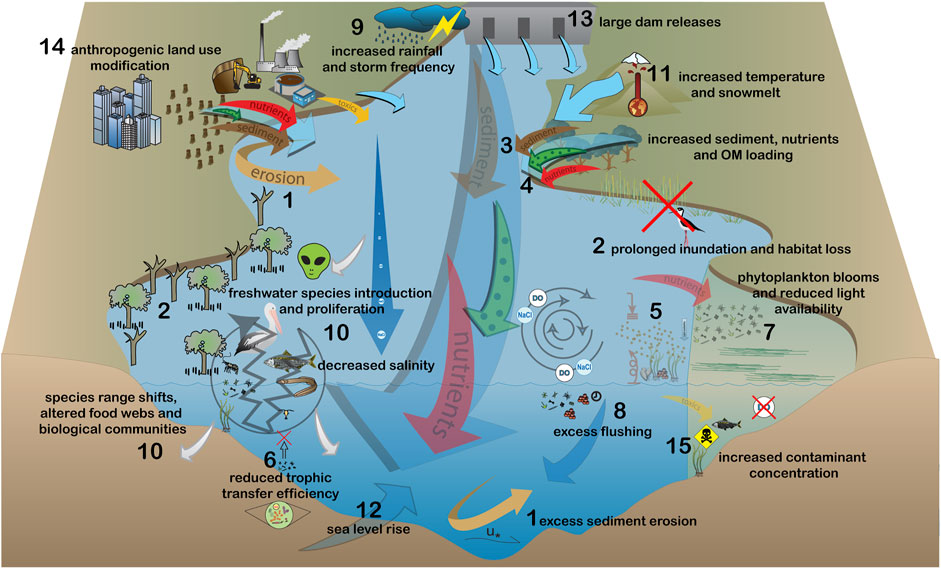
FIGURE 2. Modified estuarine ecosystem processes under long-term increases in flow and/or increases in the frequency and magnitude of large flow events. Impacts of this flow scenario are: 1) excess sediment erosion, 2) prolonged inundation of intertidal habitat, 3) increased sediment and 4) organic matter and nutrient loading, 5) reduced light availability and excess sediment impacts on benthic fauna, 6) reduced trophic transfer efficiency 7) algal bloom formation and associated water quality decline, 8) excess flushing, 10) decreasing salinity throughout the estuary and associated changes to species distribution, and 15) bioaccumulation of toxins in organisms. Principal drivers of change are: 9) increased rainfall and frequency and magnitude of storms, 11) increased snow melt, 12) sea level rise, 13) large dam releases and 14) anthropogenic land use modification.
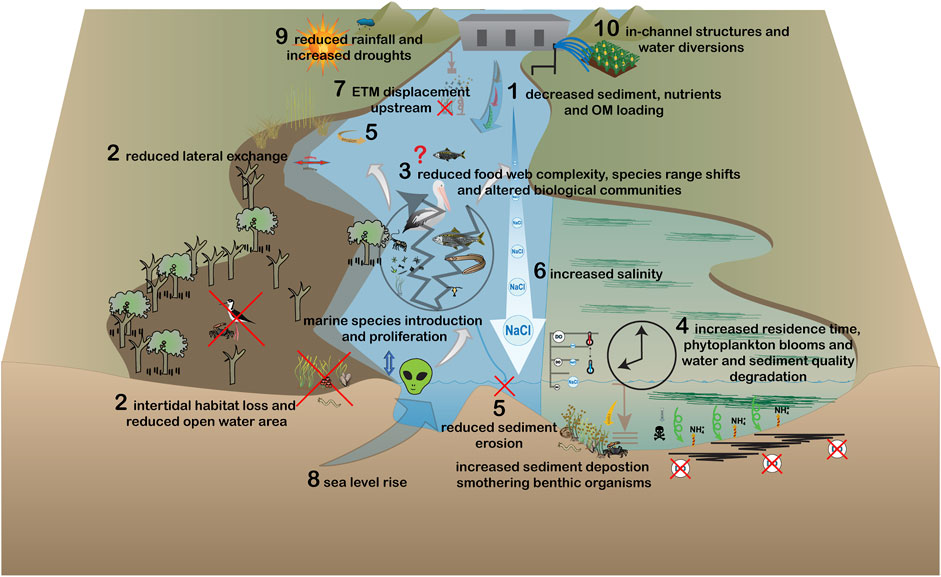
FIGURE 3. Modified estuarine ecosystem processes under permanent decreases in flow volume and/or increases in the frequency and magnitude of drought. Impacts of this flow scenario are: 1) reduced delivery of organic matter, nutrients and sediments, 2) losses to intertidal and pelagic habitat structure, 3) reduced nutrient and energy transfer through the food web, 4) increased residence time with associated water quality decline, 5) reduced sediment scouring, 6) increased salinity and 7) a shift upstream of the ETM. Principal drivers of change are: 8) sea level rise, 9) reduced rainfall and increasing droughts, and 10) in-channel structures and water diversions.
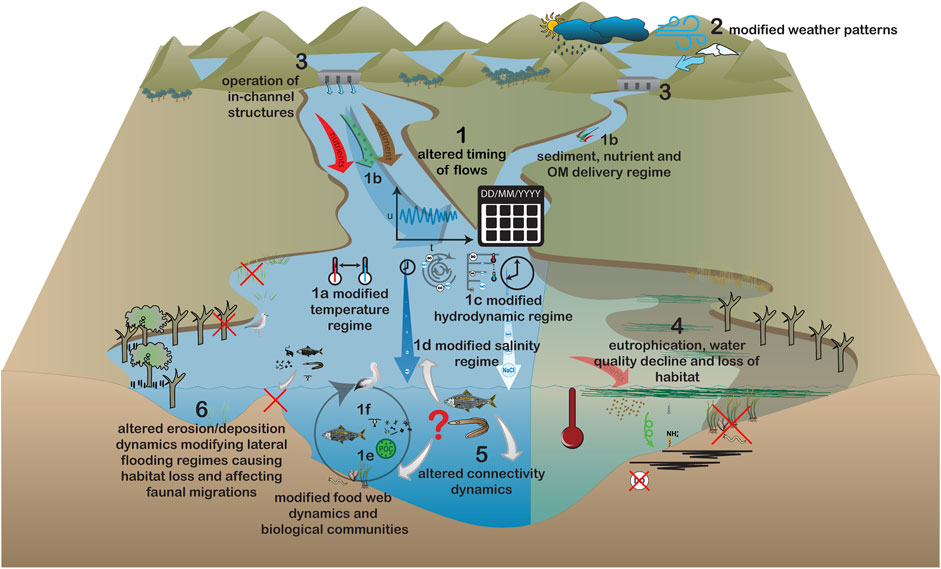
FIGURE 4. Modified estuarine ecosystem processes under alterations to the timing of flows. Impacts of this flow scenario are: 1a–f) effects of modified seasonal flow patterns on a) water temperature, b) sediment, nutrient and organic matter inputs, c) hydrodynamics, d) salinity, e) food webs and f) biological community composition. This can cause 4) increased nutrient availability, phytoplankton blooms and eutrophication, 5) altered connectivity dynamics affecting migratory patterns and biological community functioning, and 6) altered mouth scouring and associated impacts upon migration of organisms, salinity and intertidal flooding regimes. Principal drivers are: 2) modified weather patterns as a result of climate change and 3) the presence and operation of in-channel structures.
4.1 Increasing and Decreasing Flows
Long- and short-term increases in flow can lead to changes to geomorphology from sediment erosion (#1 Figure 2; Park et al., 2014) and prolonged inundation of intertidal habitat (#2 Figure 2; Adams, 2020), both resulting in habitat loss. Elevated flow may transport more sediment, humic substances and dissolved organic matter (DOC, including chromophoric components) from catchments (#3 and #4 Figure 2) which act to reduce light availability (#5 Figure 2) to primary producers (Andersson et al., 2015; Glover et al., 2019). Excess sediment may impact benthic fauna through interference with filter feeding and smothering of larvae (#5 Figure 2; Huang et al., 2016). Increased allochthonous organic matter and nutrient loading (#4 Figure 2) can increase heterotrophy in estuaries and reduce efficiency of food web transfers (#6 Figure 2; Wikner and Andersson, 2012; Soares and Berggren, 2019). It can also stimulate primary production which may be assimilated into the food web increasing productivity, but can also lead to algal blooms (#7 Figure 2) which compromise water quality and may reduce dissolved oxygen upon bloom collapse (Woodland et al., 2015; Claassens et al., 2020; Steichen et al., 2020). Recruitment and survival of planktonic larvae may be reduced from excess flushing (#8 Figure 2; Lueangthuwapranit et al., 2011; Park et al., 2014), which can be exacerbated by breaching of natural barriers due to increased flow and/or increases in the frequency and magnitude of storms (#9 Figure 2; Li et al., 2019).
In contrast, flow reductions decrease the delivery of organic matter and nutrients (#1 Figure 3; Alber, 2002; Alvarez-Lajonchère et al., 2018), and sediments to estuaries (#1 Figure 3; Vörösmarty et al., 2003; Liu C. et al., 2019). Reduced sediment, nutrient and organic matter delivery may negatively impact the provision of intertidal (#2 Figure 3; Sottolichio et al., 2013; Adams, 2020) and pelagic (Hasenbein et al., 2013; Stewart et al., 2020) habitats. The extent of inundation of intertidal areas is reduced, diminishing the transport of nutrients, sediments and organisms between pelagic and littoral habitats and exposing intertidal areas to desiccation (Adams, 2020). Biological community structure is then impacted through reductions to primary productivity and nutrient and energy flow through the food web (#3 Figure 2; Vinagre et al., 2011b; Clark and O'Connor, 2019). The hydrodynamics of the estuary are altered through reductions to vertical mixing and flushing, increasing the retention of particles with consequent nutrient enrichment, phytoplankton blooms, dissolved oxygen reduction and pollution problems (#4 Figure 3; Drinkwater and Frank, 1994; Waltham et al., 2013; Cottingham et al., 2018; Scharler et al., 2020). Reduced flows decrease sediment scouring at the mouth and along the banks (#5 Figure 3), creating sandbars and a barrier to migration of fauna (Nelson et al., 2013; Pasquaud et al., 2015), as well as reducing open water habitat area (Belmar et al., 2019). Reduced flows also lead to a reduction in the area and volume of the halocline which is an important region for estuarine productivity and a breeding area for some fish species, such as black bream (Acanthopagrus butcheri; Jenkins et al., 2010; Williams et al., 2012; Williams et al., 2013).
Modified flow regimes alter salinity, whereby flow increases reduce salinity throughout the estuary and push the salinity gradient closer to the mouth (#10 Figure 2; Adams, 2020; Steichen et al., 2020). In contrast, flow reductions increase estuarine salinity with the intrusion of seawater (#6 Figure 3), driving the salinity gradient further upstream (Sheaves et al., 2007; Hallett et al., 2018) and can result in the development of a reverse salinity gradient and hypersaline conditions from evaporation in warm, dry climates (Whitfield et al., 2012). Altered salinity can create barriers to the movement of organisms, removing access to important habitat and resources (Romañach et al., 2019; Santos et al., 2019) and can shift the distribution of species, causing it to contract or fragment (Alber, 2002; Park et al., 2014; Lauchlan and Nagelkerken, 2020). This can affect population dynamics by increasing the intensity of competition when distributions overlap (Shih et al., 2011) or by promoting the expansion of invasive or dominant species (Shih et al., 2011; Rodríguez-Climent et al., 2013). In some cases, prolongation of these impacts can lead to local extinctions (Nicol et al., 2018).
Increasing seawater penetration up the estuary in response to flow reductions can alter the position of the ETM (#7 Figure 3), moving it to the shallower upper reaches inhabited by benthic primary producers where reductions to light availability, in addition to salinity-induced osmotic stress, decreases growth and survival (Sottolichio et al., 2013; Robins et al., 2016). This can impact the organisms which use these areas as habitat, such as juvenile fish and invertebrates (Zhang H. et al., 2017; Belmar et al., 2019; Henderson, 2019). Increasing salinity in the upper reaches may also cause the transition of fringing riparian woodlands to saltmarshes or mangroves as a result of prolonged inundation and osmotic stress (Conner and Askew, 1992; Brinson et al., 1995), which can permanently alter estuary ecosystem structure and function (Brinson et al., 1995).
4.2 Interactive Effects From Climate and Direct Anthropogenic Drivers
The impacts of long-term changes to flow volumes may be exacerbated by climate change (Lauchlan and Nagelkerken, 2020). Greater snowmelt (#11 Figure 2) and precipitation (#9 Figure 2) may act in unison to drive major increases in freshwater flows to high-latitude estuaries (Andersson et al., 2015). Sea level rise (#12 Figure 2), increased frequency and magnitude of storms (#9 Figure 2) and large dam releases (#13 Figure 2) in response to increased precipitation and snowmelt may act accordantly to exacerbate the impacts of increased flows (Figure 2), which may be reflected in severe scouring of sediments at the estuary channel mouth (Riddin and Adams, 2010; Whitfield et al., 2012).
Conversely, sea level rise (#8 Figure 3) may offset losses from flow reductions by maintaining an open channel mouth, allowing for transfer of organisms, nutrients and sediments between intertidal and pelagic areas (Lester et al., 2013). The importance of marine-derived organic matter, nutrients and sediments relative to catchment inputs may affect biogeochemical activity, impacting estuarine organisms and food webs as river water is typically more nutrient-rich than seawater (Statham, 2012). Sea level rise associated tidal incursion may cause erosion and act with decreasing freshwater inputs to reduce intertidal habitat (Whitfield et al., 2012; Arellano et al., 2019). The collective impacts of reductions in rainfall, increased frequency of drought (#9 Figure 3) and freshwater extraction and diversion (#10 Figure 3), together with greater saline intrusion, impact species distributions and can affect drinking water availability (Kingsford et al., 2011; Romañach et al., 2019; Wang and Hong, 2021).
Anthropogenic development in estuaries and their catchments (#14 Figure 2) can act accordantly with climate change to exacerbate the impacts from increased flows (Hu et al., 2020). Flooding may lead to overflows of untreated sewage in urbanised and industrialised areas, increasing contaminant and pathogen concentrations (Olds et al., 2018). Where there are mining activities, it may transport large quantities of heavy metals (e.g., mercury). These metals bioaccumulate in commercially important fisheries species (#15 Figure 2) to levels exceeding the limit for human food consumption (Gamboa-García et al., 2020).
4.3 Changes to the Timing of Flows
Modifications to the timing of flow delivery can have profound impacts on estuarine processes (Figure 4) with consequences for species which are adapted to these natural variations (Bunn et al., 2014; Cai et al., 2019; Izegaegbe et al., 2020). Modified seasonal flow patterns (#1 Figure 4) interact with temperature (#1a Figure 4) and the delivery of nutrients, organic matter and sediments (#1b Figure 4), to alter the hydrodynamics (#1c Figure 4), salinity (#1d Figure 4), resource availability (#1e Figure 4), predator-prey cycles and food web functioning (#1f Figure 4; Hallett et al., 2018; Hamilton et al., 2020). Changes to the seasonality of flow due to climate change (#2 Figure 4) alters the timing of bioavailable dissolved nutrient export to estuaries, particularly where there are high delivery rates of these nutrients (e.g., agriculturally developed catchments), which can lead to increased phytoplankton biomass and reduced dissolved oxygen concentrations (Wagena et al., 2018). This can alter the spatial-temporal dynamics of phytoplankton blooms and hypoxia (#4 Figure 4), as observed in Chesapeake Bay, United States, where spring flows are shifting earlier in the season and stimulating an earlier onset and upstream shift of the spring bloom (Testa et al., 2018). Flow increases in warmer, drier, months (e.g., summer, tropics dry season) due to climate change and dam operations (#3 Figure 4) increases resource delivery to warmer estuarine waters (#4 Figure 4; Bunn et al., 2014; Cai et al., 2019). Under these conditions, phytoplankton activity becomes greatly stimulated and can lead to persistent blooms, eutrophication, anoxia and associated physiological stress for organisms (Hallett et al., 2018). Changes to seasonal peaks in flow impacts species migratory patterns (#5 Figure 4) which are often cued to seasonal changes in water chemistry brought about by freshwater flows (Drinkwater and Frank, 1994; Saintilan and Wen, 2012). Changes in migration patterns have consequences for species interactions and food web functioning (Hallett et al., 2018). For example, changes to the timing of flows from spring to autumn have been attributed to the decline of the endangered delta smelt (Hypomesus transpacificus) in the San Francisco Bay Estuary, United States, due to decreases in the abundance of its copepod prey. Lower temperatures together with the presence of a bivalve predator at the time of flow delivery act to reduce copepod biomass (Hamilton et al., 2020).
In estuaries which intermittently close to the ocean (#6 Figure 4), sudden influxes of freshwater during warmer months, when the mouth is closed, can cause a sharp reduction in salinity, threatening brackish and marine species that cannot migrate to preferred salinities (Scharler et al., 2020). Furthermore, these flows can increase the inundation of intertidal and littoral areas, causing loss of habitat and associated organisms due to rapid freshwater transitions and physiological stress (Adams, 2020).
5 Strategies for Risk Mitigation From Modified Flows
The flow regime is critically linked to key estuarine ecosystem processes (Figure 5) that are potentially amenable to restoration strategies involving changes in flow dynamics. Re-establishment of key elements of the natural freshwater flow regime is required to mitigate the multiple and interrelated risks posed to ecosystem processes. Natural flow regimes typically consist of the following principal components: low flow (e.g., drought), base flow, inter-annual to annual peak flow and decadal peak flow (e.g., 10–100-year flood recurrence). Strategies to achieve this are discussed in the following sections.
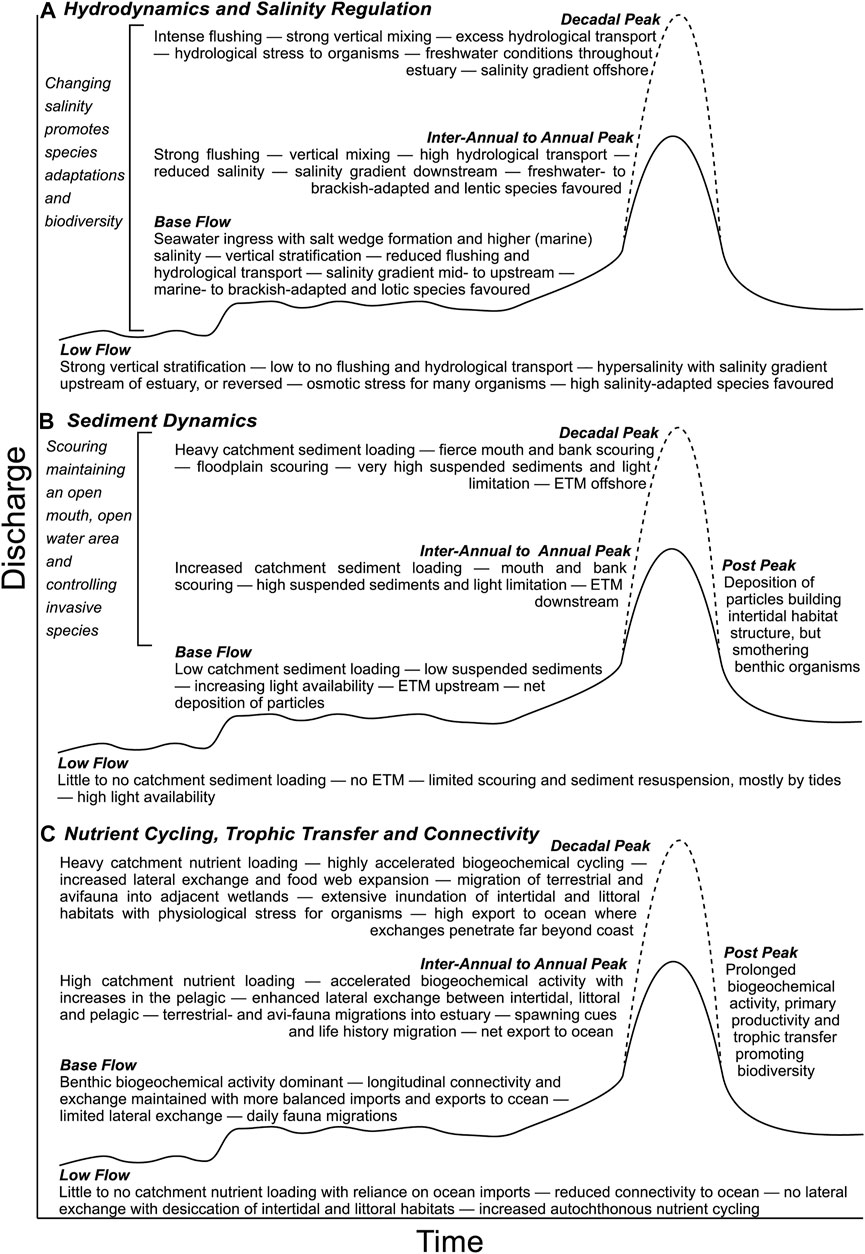
FIGURE 5. Conceptual hydrographs displaying the effects of varying flow components (low flow, base flow, inter-annual to annual peak, decadal peak and post peak) of the freshwater flow regime on the functioning of the key ecosystem processes: hydrodynamics and salinity regulation (A), sediment dynamics (B), and nutrient cycling, trophic transfer and connectivity (C).
5.1 Estuary Water Requirement
Preceding any ecological restoration actions, it is critical to define desirable physical, chemical (e.g., salinity) and biological (e.g., habitat availability, species migrations) conditions to support the suitable estuarine ecosystem processes and function outlined in Figure 5 (Perry and Hershner, 1999; Kiwango et al., 2015; Hallett et al., 2018). Often, the above criteria can be defined quantitatively using water quality characteristics which serve as a basis for assessing the attainment of designated uses and measuring progress toward meeting goals (Tango and Batiuk, 2013; Zhang et al., 2018). The flow regime required to deliver desired conditions has been referred to as an estuary’s water requirement (Adams et al., 2002) and needs to account for volume (amount) and intra-annual and inter-annual variability over the estuarine domain (Peñas et al., 2013; Zhang H. et al., 2017; Van Niekerk et al., 2019b). Once desirable conditions are defined, freshwater flow volumes can be linked through approaches such as expert opinion, or statistical or deterministic models to a given preferred physical state [e.g., salinity, nutrient, dissolved oxygen, water depth and mouth morphology (closed/open)] and the presence and abundance of desired organisms.
A guiding principle behind past estuarine water requirement determination methods has been to return as far as practicable to pre-regulation flow regimes without disrupting societal function (Acreman et al., 2014). However, under global climate change, modifications to the freshwater flow regime and its influence on estuarine processes are inevitable (IPCC, 2014) and constraining future flow regimes to historical targets can be not only counterproductive, but incorrect, as it is necessary to consider natural changes inherent to each system in addition to global climate change (Acreman et al., 2014; Poff, 2018). Reference conditions by which E-flow targets are determined are not static (Poff, 2018) and may require non-stationary target conditions as systems change and new reference conditions emerge (Arthington et al., 2018; Poff, 2018). Ideally, research and monitoring can be used to define limits of acceptable change for site-specific system attributes (e.g., salinity timing and concentration thresholds for fish recruitment or light thresholds for seagrass growth). Monitoring programmes are then required to support this approach, enabling qualitative and quantitative measures of change (Claassens et al., 2020).
5.2 Modelling the Natural Flow Regime
Models are key tools to assist with understanding, planning for, and mitigating impacts from rapid environmental change. For estuaries, process-based models have been proven to be particularly useful for exploring a range of flow regimes, including reference conditions, target hydrological conditions and extreme events (Beilfuss and Brown, 2010; Van Niekerk et al., 2019b). Most models focus on simulating the prevailing hydrodynamics and thermodynamics (Duarte et al., 2014; Biguino et al., 2021), fewer examine geomorphological evolution (Deng et al., 2017), and even fewer consider ecological states (Panda et al., 2015; Weng et al., 2020). As flow volumes to estuaries are naturally stochastic (Gillanders and Kingsford, 2002), model simulations need to include a range of relevant temporal variations that conform to the historical and future variance distribution in terms of flow exceedance likelihoods (Beilfuss and Brown, 2010; Van Niekerk et al., 2019b). Model outputs can then be used to define physicochemical or biological states for different flow regimes (Peñas et al., 2013; Van Niekerk et al., 2019b). For fully coupled hydrodynamic-ecological models, different flow scenarios have been developed to consider factors leading to adverse water quality outcomes, such as cyanobacteria blooms (Robson and Hamilton, 2004) and hypoxia (Huang et al., 2018). These mechanistic models can help to optimise biodiversity under altered flow regimes and look for new opportunities (i.e., novel flow regimes) to promote resilience (Hipsey et al., 2015; Tonkin et al., 2021).
A long-standing example is the coupled watershed-estuary model of Chesapeake Bay which has been in continuous operation since 1982 (Shenk and Linker, 2013). The Chesapeake Bay Program Partnership has been developing, updating, and applying a complex linked system of watershed, airshed, and estuary models which is used as a planning tool to inform strategic management decisions and restoration efforts. The model has been attributed to playing a crucial role in pollutant load reductions, reduced anoxic “dead zone” volume and increased submerged aquatic plant cover in the bay (Hood et al., 2021).”
Long-term datasets are essential for ecohydrological simulations to provide key boundary condition and within-domain validation data, and develop confidence that the model captures the dominant processes which drive estuarine ecosystem functioning (Adams et al., 2016a; Van Niekerk et al., 2019b; Claassens et al., 2020). Monitoring needs to occur at time and space scales that are relevant to model input requirements (Adams et al., 2016a). Most of our current models are challenged by lags and hysteresis relationships that exist between flow and biological responses and further efforts are required to prove the current generation of models are fit for purpose in this regard (Hipsey et al., 2020). Ultimately, models can then be used to quantify the risks associated with a given pattern of water delivery in terms of likelihood of crossing the limits of acceptable change.
In estuaries where there are sparse observation networks and limited data, E-flow requirements may be difficult to define (Adams, 2014). For such cases, rainfall-runoff models can be used to simulate hydrological data from within the catchment, or nearby catchments may be used to infer relationships between rainfall and runoff (Van Niekerk et al., 2019b). Where long-term physicochemical or biological data is missing, recent or current salinity data can be used as an indicator or proxy for the relationship between freshwater inflow and the functioning of estuaries, due to the transferability of salinity effects to multiple ecosystem elements (Peñas et al., 2013).
5.3 Dam, Agricultural and Wastewater Operations to Benefit Environmental Flows
Throughout the globe, dam construction has impaired the natural flow regime, with significant consequences for river and estuarine ecosystem functioning (Poff et al., 1997). Typically, there are multiple stakeholders and competing objectives for water (e.g., hydrological power, water supply) and releases from dams will differ to the natural flow regime in terms of volume and timing (Richter and Thomas, 2007; Watts et al., 2011). However, there are opportunities to modify the operation of dams to work towards restoring natural flow regimes (Bednarek and Hart, 2005; Richter and Thomas, 2007; Morais, 2008; Watts et al., 2011) or design “novel” flow regimes (Tonkin et al., 2021) to prioritise the characteristics of the flow regime which supply the most ecological benefit. Richter and Thomas (2007) proposed a six-step framework for planning and implementing dam re-operation involving: 1) the assessment of dam-induced alterations to the flow regime compared to pre-dam flows, 2) describing the ecological and social consequences of modified flows due to dam operation, 3) specifying goals (e.g., targeted flows for ecological outcomes) for re-operation, 4) designing re-operation strategies to achieve goals, 5) implementation of strategies and 6) assessing results against goals.
Water consumption from irrigation can be reduced through improvements to infrastructure and on-farm irrigation technology, and by establishing legislation that requires acquisition of licenses or permits, and sets allocation limits to the volume of water extracted and the number of irrigators in a catchment (Gippel et al., 2009). Effective monitoring and enforcement are necessary to administer these measures (Adams et al., 2020). Additionally, in estuaries and catchments with intense agricultural production, alternative farming practices can be introduced to reduce the use of fertilisers, herbicides and pesticides, for example by balancing fertiliser use with plant requirements and adopting integrated pest management strategies to decrease nutrient and contaminant loads (Olsen et al., 2006; Claassens et al., 2020).
In estuaries and catchments with urban development, storm water management can be improved by increasing permeable surface area and implementing alternative solutions, such as rain gardens, to reduce excessive flow peaks, nutrient loads, toxins and species invasions (McGrane, 2016). Increasingly, large-scale areas are being targeted to reduce impervious surfaces and flood risks in what has been termed “sponge cities” (Zevenbergen et al., 2018). Improved infrastructure to effectively treat and recycle wastewater can also reduce nutrient and contaminant loads. This requires effective governance through routine monitoring and compliance (Claassens et al., 2020).
5.4 Complementary Restoration Strategies
The implementation of strategies to determine and deliver the most suitable E-flows alone may not address all of the threats to estuarine processes. Non-flow related environmental factors, such as various land use practices (e.g., vegetation clearance), sediment modification (e.g., infilling, dredging), pollution and invasive species proliferation, may also challenge the ecological health of estuaries (Dias et al., 2016; Roebig et al., 2017; Poff, 2018). Consequently, other strategies may be necessary to restore degraded estuaries and complement environmental flow management strategies.
Artificial modifications to the geomorphology of an estuary, such as dredging, may be used as a restoration method (Whitfield et al., 2012; Park et al., 2014; Belmar et al., 2019). Where prolonged reductions in freshwater inflows have occurred and the mouth of an estuary is constricted, it may be necessary to artificially breach the sand bar (Adams et al., 2016a). This enables connection to the ocean, flushing of pollutants, return of marine water incursions and passage for species migrations (Whitfield et al., 2012). However, artificial breaching and channel modification may have contrasting effects as a result of physicochemical disturbances due to the increase in flushing and subsequent tidal influx (Schallenberg et al., 2010), expelling large numbers of hyperbenthic macroinvertebrates into the ocean (Lill et al., 2012) and causing macrophyte die-off due to water level decline and osmotic stress (dos Santos and Esteves, 2002; dos Santos et al., 2006). Subsequent decomposition of the dead macrophyte material can result in nutrient enrichment (dos Santos et al., 2006), negating the benefits to eutrophication from increased flushing. Prior understanding of estuary specific factors (e.g., geomorphology, degree of tidal incursion, presence/absence of macrophytes and benthic fauna) that influence the natural mouth opening regime is critical to artificial geomorphological restoration works (Schallenberg et al., 2010).
Conversely, artificially infilling a naturally opened barrier may also be used as a conservation strategy (Park et al., 2014). For example, in Mobile Bay, United States, Hurricane Katrina scoured a new channel to the ocean by cutting Dauphin Island in two. The increased salinity and flushing in the bay negatively impacted resident biota, in particular a large oyster population. In response, infilling the breach in Dauphin Island improved estuarine conditions with subsequent increases in oyster abundance (Park et al., 2014). However, it is important to note that artificially changing the geomorphology of estuaries may have negative consequences for ecological health, often not evident in short term assessments and implementation (Widdows et al., 2007; Belmar et al., 2019).
Artificial structures, such as barriers, levees and sea walls, can be used to manage increasing tidal incursions and protect shorelines from increased wave and tidal energy due to sea level rise (Koraim et al., 2011). Barriers, such as locks and gates, can be used to manage tidal flow and provide protection against tidal flooding, and have been operational in the River Thames Estuary, United Kingdom, since the 1980s (Lavery and Donovan, 2005). Levees and sea walls provide geomorphological protection by fixing the shoreline in place and are widely used in estuaries around the world (Koraim et al., 2011). These structures can be implemented in conjunction with intertidal and littoral habitat restoration to enhance shoreline protection from erosion and flooding (Pinto et al., 2018). However, shoreline protection structures are expensive to erect and maintain, are susceptible to damage from large storm events, can alter erosion/deposition dynamics and tend to not be as effective as natural shorelines at attenuating wave and tide energy (Koraim et al., 2011). In addition, they can pose a barrier to lateral connectivity and cause intertidal and littoral habitats to retract in response to changes in distribution as a result of sea level rise induced salinity alterations (Colombano et al., 2021; Khojasteh et al., 2021).
Artificial oxygenation may be used to locally negate the negative impacts of low dissolved oxygen in the bottom waters of vertically stratified and eutrophic estuaries, and in regions upstream of weirs that restrict saline intrusion (Huang et al., 2018; Larsen et al., 2019). Artificial oxygenation is an engineering measure to supply oxygen generated mechanically to anoxic or hypoxic bottom waters, aerating the water and increasing redox potential at the sediment-water interface to reduce sediment nutrient release (Toffolon et al., 2013). This technique has been more commonly used in deep freshwater lakes and reservoirs (Gantzer et al., 2009; Toffolon et al., 2013), but was successfully applied to shallow freshwaters upstream of the Swan River Estuary, Perth, whereby dissolved oxygen concentrations increased immediately in the water column post installation, with improvements after several days at the sediment-water interface increasing oxygen fluxes into the sediment (Larsen et al., 2019). Previous attempts to address deoxygenation in the salt wedge region of the Swan River Estuary were undertaken through artificial destratification by application of bubble plumes to mix surface and bottom waters (Hamilton et al., 2001). Despite generating complete vertical mixing, this prototype was not extended to full scale because of its localised influence (30 m radius around the bubble plume) and inability to extend over tidal excursions (Hamilton et al., 2001).
5.5 Holistic Management
Holistic catchment-to-coast management and restoration planning is critical to mitigate the risks from the drivers of change (Gippel et al., 2009; Watts et al., 2011; Arthington et al., 2018; Stewardson and Guarino, 2018; Van Niekerk et al., 2019a). A holistic approach aims to address the water requirements of the entire river-catchment ecosystem, including principal and tributary river channels, groundwater, floodplains, lakes, estuaries and near-shore marine ecosystems (Arthington et al., 2003). Environmental flow delivery needs to be intertwined with other strategies, such as nutrient management and climate adaptation, and a holistic assessment must consider the consequences of water releases, such as increasing nutrient loads and productivity which can potentially lead to eutrophication where flushing is not adequate.
Stakeholders play an integral role in the definition and execution of environmental flow targets as reliable knowledge of environmental conditions, both past and present, can be contributed by scientific and non-scientific groups (Olsen et al., 2006). However, difficulties can arise in achieving the appropriate balance of flow delivery as there is often a large number of stakeholders with varied, and sometimes opposing, motivations and desired outcomes for E-flow targets (Gippel et al., 2009). Holistic catchment management requires sound understanding of the knowledge and interests of the many relevant groups (e.g., individuals, local communities, organisations, government agencies, private enterprises) and good communication, including the discussion of the consequences of potential courses of action to seek consensus and enable E-flow targets to be met (Olsen et al., 2006; Watts et al., 2011). This may require trade-offs and targeted restoration of the processes within estuaries that provide the greatest benefit for desired outcomes (e.g., increased biodiversity, improved water quality; Van Niekerk et al., 2019a).
Despite potential communication and motivational limitations, holistic management is achievable. This is highlighted by a long-standing holistic management system in Chesapeake Bay, United States where the Chesapeake Bay Program, a partnership including six states (Virginia, Maryland, Pennsylvania, West Virginia, Delaware and New York), the district of Columbia and hundreds of federal, state and local government agencies, academic institutions and not-for-profit organisations, formed in 1983 to guide and foster restoration of Chesapeake Bay and its catchment (Hood et al., 2021).
Given the rate of climate change and human impacts, long-term changes to estuarine ecosystem structure and functioning are inevitable (Arthington et al., 2018; Lauchlan and Nagelkerken, 2020). Environmental flows and restoration of estuaries need to build resilience and buffer against the impacts enabling organisms and ecosystems to adapt (Sun et al., 2013).
6 Conclusion
Knowledge of freshwater flow regimes to support fully functional, healthy estuaries is still lacking compared to river and floodplain ecosystems. Key estuarine ecosystem processes that are mediated by freshwater flow regimes are hydrodynamics, salinity regulation, sediment dynamics, nutrient cycling and trophic transfer, and connectivity. These processes promote estuarine biodiversity and support a wide range of ecosystem services, but are threatened by changes to the magnitude and timing of flows as a result of climate change and direct anthropogenic stressors. Mitigation of these stressors can be achieved through a number of strategies: defining desired physical and biological conditions based on non-stationary target conditions, using numerical models to simulate flow scenarios to predict the flows required to produce desired conditions, modifying dam, agricultural and wastewater operations to help to deliver these flows, performing restoration strategies to complement flow delivery, and managing E-flows holistically from catchment to coast and balancing the needs of various stakeholders. By focusing on the ecosystem processes influenced by the freshwater flow regime, we provide greater transferability of E-flow requirements amongst estuaries. Transferability of concepts around the ecology of complex systems such as estuaries is difficult. We believe this synthesis has drawn out the key concepts related to the effects of flow on estuaries and presented them in a generally transferable way. This review can help guide estuarine catchment management to define appropriate freshwater flow strategies that provide resilience to the impacts of current and future climate and direct anthropogenic pressures.
Author Contributions
All authors listed have made a substantial, direct, and intellectual contribution to the work and approved it for publication.
Funding
IN was supported by a Discovery Projects grant from the Australian Research Council (grant no. DP170101722). MH was supported by the Australian Research Council Linkage program (LP150100451). PC was supported by the Australian Research Council grant no. LP140100087.
Conflict of Interest
The authors declare that the research was conducted in the absence of any commercial or financial relationships that could be construed as a potential conflict of interest.
Publisher’s Note
All claims expressed in this article are solely those of the authors and do not necessarily represent those of their affiliated organizations, or those of the publisher, the editors, and the reviewers. Any product that may be evaluated in this article or claim that may be made by its manufacturer is not guaranteed or endorsed by the publisher.
Acknowledgments
We kindly thank the University of Adelaide for providing access to resources and materials. Further, we are very thankful for the constructive critique by the reviewers and would like to thank the organisers of this special issue.
References
Abbott, B. W., Bishop, K., Zarnetske, J. P., Minaudo, C., Chapin, F. S., Krause, S., et al. (2019). Human Domination of the Global Water Cycle Absent from Depictions and Perceptions. Nat. Geosci. 12 (7), 533–540. doi:10.1038/s41561-019-0374-y
Acreman, M., Arthington, A. H., Colloff, M. J., Couch, C., Crossman, N. D., Dyer, F., et al. (2014). Environmental Flows for Natural, Hybrid, and Novel Riverine Ecosystems in a Changing World. Front. Ecol. Environ. 12 (8), 466–473. doi:10.1890/130134
Adams, J. B. (2014). A Review of Methods and Frameworks Used to Determine the Environmental Water Requirements of Estuaries. Hydrological Sci. J. 59 (3-4), 451–465. doi:10.1080/02626667.2013.816426
Adams, J. B., Bate, G. C., Harrison, T. D., Huizinga, P., Taljaard, S., van Niekerk, L., et al. (2002). A Method to Assess the Freshwater Inflow Requirements of Estuaries and Application to the Mtata Estuary, South Africa. Estuaries 25 (6), 1382–1393. doi:10.1007/bf02692232
Adams, J. B., Cowie, M., and Van Niekerk, L. (2016a). Assessment of Completed Ecological Water Requirement Studies for South African Estuaries and Responses to Changes in Freshwater Inflow. Gezina, South Africa: Water Research Commission.
Adams, J. B. (2020). Salt Marsh at the Tip of Africa: Patterns, Processes and Changes in Response to Climate Change. Estuarine, Coastal Shelf Sci. 237, 106650. doi:10.1016/j.ecss.2020.106650
Adams, J., Taljaard, S., van Niekerk, L., and Lemley, D. (2020). Nutrient Enrichment as a Threat to the Ecological Resilience and Health of South African Microtidal Estuaries. Afr. J. Aquat. Sci. 45, 23–40. doi:10.2989/16085914.2019.1677212
Adams, M. P., Hovey, R. K., Hipsey, M. R., Bruce, L. C., Ghisalberti, M., Lowe, R. J., et al. (2016b). Feedback between Sediment and Light for Seagrass: Where Is it Important? Limnol. Oceanogr. 61 (6), 1937–1955. doi:10.1002/lno.10319
Alber, M. (2002). A Conceptual Model of Estuarine Freshwater Inflow Management. Estuaries 25 (6), 1246–1261. doi:10.1007/bf02692222
Alvarez-Lajonchère, L., Laíz-Averhoff, O., and Perigó-Arnaud, E. (2018). River Dam Effects on Cuban Fisheries and Aquaculture Development with Recommendations for Mitigation. Environ. Eng. Manag. J. 17 (2), 491–512. doi:10.30638/eemj.2018.050
Andersson, A., Meier, H. E. M., Ripszam, M., Rowe, O., Wikner, J., Haglund, P., et al. (2015). Projected Future Climate Change and Baltic Sea Ecosystem Management. Ambio 44, 345–356. doi:10.1007/s13280-015-0654-8
Andreasen, J. K., O’Neill, R. V., Noss, R., and Slosser, N. C. (2001). Considerations for the Development of a Terrestrial index of Ecological Integrity. Ecol. Indicators 1, 21–35. doi:10.1016/s1470-160x(01)00007-3
Arellano, A. R., Bianchi, T. S., Osburn, C. L., D'Sa, E. J., Ward, N. D., Oviedo‐Vargas, D., et al. (2019). Mechanisms of Organic Matter export in Estuaries with Contrasting Carbon Sources. J. Geophys. Res. Biogeosci. 124 (10), 3168–3188. doi:10.1029/2018JG004868
Arthington, A. H. (2012). “Estuaries, Threats and Flow Requirements,” in Environmental Flows: Saving Rivers in the Third Millenium. 1 ed (USA: University of California Press), 192–200. doi:10.1525/california/9780520273696.003.0018
Arthington, A. H., Kennen, J. G., Stein, E. D., and Webb, J. A. (2018). Recent Advances in Environmental Flows Science and Water Management-Innovation in the Anthropocene. Freshw. Biol. 63 (8), 1022–1034. doi:10.1111/fwb.13108
Arthington, A. H., Tharme, R. E., Brizga, S. O., Pusey, B. J., and Kennard, M. J. (2003). “Environmental Flow Assessment with Emphasis on Holistic Methodologies,” in Proceedings of the Second International Symposium on the Management of Large Rivers for Fisheries. Editors R. L. Welcomme, and T. Petr (Bangkok, Thailand: FAO Regional Office for Asia and the Pacific).
Barbier, E. B., Hacker, S. D., Kennedy, C., Koch, E. W., Stier, A. C., and Silliman, B. R. (2011). The Value of Estuarine and Coastal Ecosystem Services. Ecol. Monogr. 81 (2), 169–193. doi:10.1890/10-1510.1
Barlow, J. P., Lorenzen, C. J., and Myren, R. T. (1963). Eutrophication of a Tidal Estuary. Limnol. Oceanogr. 8 (2), 251–262. doi:10.4319/lo.1963.8.2.0251
Bednarek, A. T., and Hart, D. D. (2005). Modifying Dam Operations to Restore Rivers: Ecological Responses to Tennessee River Dam Mitigation. Ecol. Appl. 15 (3), 997–1008. doi:10.1890/04-0586
Beilfuss, R., and Brown, C. (2010). Assessing Environmental Flow Requirements and Trade-Offs for the Lower Zambezi River and delta, Mozambique. Int. J. River Basin Manag. 8 (2), 127–138. doi:10.1080/15715121003714837
Belmar, O., Ibáñez, C., Forner, A., and Caiola, N. (2019). The Influence of Flow Regime on Ecological Quality, Bird Diversity, and Shellfish Fisheries in a lowland Mediterranean River and its Coastal Area. Water 11 (5), 918. doi:10.3390/w11050918
Biguino, B., Sousa, F., and Brito, A. C. (2021). Variability of Currents and Water Column Structure in a Temperate Estuarine System (Sado Estuary, Portugal). Water 13 (2), 187. doi:10.3390/w13020187
Brinson, M. M., Christian, R. R., and Blum, L. K. (1995). Multiple States in the Sea-Level Induced Transition from Terrestrial forest to Estuary. Estuaries 18 (4), 648–659. doi:10.2307/1352383
Brookes, J. D., Aldridge, K. T., Bice, C. M., Deegan, B., Ferguson, G. J., Paton, D. C., et al. (2015). Fish Productivity in the Lower Lakes and Coorong, Australia, during Severe Drought. Trans. R. Soc. South Aust. 139 (2), 189–215. doi:10.1080/03721426.2015.1074338
Bruce, L. C., Cook, P. L. M., Teakle, I., and Hipsey, M. R. (2014). Hydrodynamic Controls on Oxygen Dynamics in a Riverine Salt Wedge Estuary, the Yarra River Estuary, Australia. Hydrol. Earth Syst. Sci. 18 (4), 1397–1411. doi:10.5194/hess-18-1397-2014
Buelow, C., and Sheaves, M. (2015). A Birds-Eye View of Biological Connectivity in Mangrove Systems. Estuarine, Coastal Shelf Sci. 152, 33–43. doi:10.1016/j.ecss.2014.10.014
Bunn, S. E., and Arthington, A. H. (2002). Basic Principles and Ecological Consequences of Altered Flow Regimes for Aquatic Biodiversity. Environ. Manage. 30 (4), 492–507. doi:10.1007/s00267-002-2737-0
Bunn, S. E., Bond, N. R., Davis, J. A., Gawne, B., Kennard, M. J., King, A. J., et al. (2014).Ecological Responses to Altered Flow Regimes: Synthesis Report. In Water for a Healthy Country Flagship. Australia: CSIRO.
Bunn, S. E., Thoms, M. C., Hamilton, S. K., and Capon, S. J. (2006). Flow Variability in Dryland Rivers: Boom, Bust and the Bits in between. River Res. Applic. 22 (2), 179–186. doi:10.1002/rra.904
Cai, H., Zhang, X., Zhang, M., Guo, L., Liu, F., and Yang, Q. (2019). Impacts of Three Gorges Dam's Operation on Spatial-Temporal Patterns of Tide-River Dynamics in the Yangtze River Estuary, China. Ocean Sci. 15 (3), 583–599. doi:10.5194/os-15-583-2019
Caiola, N., Rodríguez-Climent, S., and Ibáñez, C. (2013). Salinity as the Main Factor Structuring Small-Bodied Fish Assemblages in Hydrologically Altered Mediterranean Coastal Lagoons. Sci. Mar. 77 (1), 37–45. doi:10.3989/scimar.03698.26B
Caperon, J., Cattell, S. A., and Krasnick, G. (1971). Phytoplankton Kinetics in a Subtropical Estuary: Eutrophication1. Limnol. Oceanogr. 16 (4), 599–607. doi:10.4319/lo.1971.16.4.0599
Chen, J., Finlayson, B. L., Wei, T., Sun, Q., Webber, M., Li, M., et al. (2016). Changes in Monthly Flows in the Yangtze River, China - with Special Reference to the Three Gorges Dam. J. Hydrol. 536, 293–301. doi:10.1016/j.jhydrol.2016.03.008
Church, J. A., Clark, P. U., Cazenave, A., Gregory, J. M., Jevrejeva, S., Levermann, A., et al. (2013). “Sea Level Change,” in Climate Change 2013: The Physical Science Basis. Contribution of Working Group I to the Fifth Assessment Report of the Intergovernmental Panel on Climate Change. Editors T. F. Stocker, D. Qin, G.-K. Plattner, M. Tignor, S. K. Allen, and J. Boschung (Cambridge, United Kingdom and New York, NY, USA: Cambridge University Press).
Claassens, L., Barnes, R., Wasserman, J., Lamberth, S., Miranda, N., van Niekerk, L., et al. (2020). Knysna Estuary Health: Ecological Status, Threats and Options for the Future. Afr. J. Aquat. Sci. 45 (1-2), 65–82. doi:10.2989/16085914.2019.1672518
Clark, R., and O'Connor, K. (2019). A Systematic Survey of Bar-Built Estuaries along the California Coast. Estuarine, Coastal Shelf Sci. 226, 106285. doi:10.1016/j.ecss.2019.106285
Cloern, J. E., Jassby, A. D., Schraga, T. S., Nejad, E., and Martin, C. (2017). Ecosystem Variability along the Estuarine Salinity Gradient: Examples from Long‐term Study of San Francisco Bay. Limnol. Oceanogr. 62 (S1), S272–S291. doi:10.1002/lno.10537
Collocott, S., Vivier, L., and Cyrus, D. (2014). Prawn Community Structure in the Subtropical Mfolozi-Msunduzi Estuarine System, KwaZulu-Natal, South Africa. Afr. J. Aquat. Sci. 39 (2), 127–140. doi:10.2989/16085914.2014.925419
Colombano, D. D., Litvin, S. Y., Ziegler, S. L., Alford, S. B., Baker, R., Barbeau, M. A., et al. (2021). Climate Change Implications for Tidal Marshes and Food Web Linkages to Estuarine and Coastal Nekton. Estuaries and Coasts 44, 1637–1648. doi:10.1007/s12237-020-00891-1
Conley, D. J. (2000). Biogeochemical Nutrient Cycles and Nutrient Management Strategies. Hydrobiologia 410, 87–96.
Conley, D. J., Carstensen, J., Vaquer-Sunyer, R., and Duarte, C. M. (2009). Ecosystem Thresholds with Hypoxia. Hydrobiologia 629 (1), 21–29. doi:10.1007/s10750-009-9764-2
Conner, W. H., and Askew, G. R. (1992). Response of Baldcypress and Loblolly pine Seedlings to Short-Term Saltwater Flooding. Wetlands 12 (3), 230–233. doi:10.1007/bf03160614
Cottingham, A., Huang, P., Hipsey, M. R., Hall, N. G., Ashworth, E., Williams, J., et al. (2018). Growth, Condition, and Maturity Schedules of an Estuarine Fish Species Change in Estuaries Following Increased Hypoxia Due to Climate Change. Ecol. Evol. 8 (14), 7111–7130. doi:10.1002/ece3.4236
Dan, S. F., Liu, S.-M., Udoh, E. C., and Ding, S. (2019). Nutrient Biogeochemistry in the Cross River Estuary System and Adjacent Gulf of Guinea, South East Nigeria (West Africa). Continental Shelf Res. 179, 1–17. doi:10.1016/j.csr.2019.04.001
Datry, T., Foulquier, A., Corti, R., von Schiller, D., Tockner, K., Mendoza-Lera, C., et al. (2018). A Global Analysis of Terrestrial Plant Litter Dynamics in Non-perennial Waterways. Nat. Geosci 11 (7), 497–503. doi:10.1038/s41561-018-0134-4
Davis, J. R., and Koop, K. (2006). Eutrophication in Australian Rivers, Reservoirs and Estuaries - A Southern Hemisphere Perspective on the Science and its Implications. Hydrobiologia 559 (1), 23–76. doi:10.1007/s10750-005-4429-2
Deng, J., Woodroffe, C. D., Rogers, K., and Harff, J. (2017). Morphogenetic Modelling of Coastal and Estuarine Evolution. Earth-Science Rev. 171, 254–271. doi:10.1016/j.earscirev.2017.05.011
Dias, E., Morais, P., Cotter, A., Antunes, C., and Hoffman, J. (2016). Estuarine Consumers Utilize marine, Estuarine and Terrestrial Organic Matter and Provide Connectivity Among These Food Webs. Mar. Ecol. Prog. Ser. 554, 21–34. doi:10.3354/meps11794
Dittmann, S., Baring, R., Baggalley, S., Cantin, A., Earl, J., Gannon, R., et al. (2015). Drought and Flood Effects on Macrobenthic Communities in the Estuary of Australia's Largest River System. Estuarine, Coastal Shelf Sci. 165, 36–51. doi:10.1016/j.ecss.2015.08.023
Doering, P. H., Chamberlain, R. H., and Haunert, D. E. (2002). Using Submerged Aquatic Vegetation to Establish Minimum and Maximum Freshwater Inflows to the Caloosahatchee Estuary, Florida. Estuaries 25 (6), 1343–1354. doi:10.1007/BF02692229
Douglas, E. M., Vogel, R. M., and Kroll, C. N. (2000). Trends in Floods and Low Flows in the United States: Impact of Spatial Correlation. J. Hydrol. 240, 90–105. doi:10.1016/s0022-1694(00)00336-x
Drinkwater, K. F., and Frank, K. T. (1994). Effects of River Regulation and Diversion on marine Fish and Invertebrates. Aquat. Conserv: Mar. Freshw. Ecosyst. 4, 135–151. doi:10.1002/aqc.3270040205
Duarte, P., Alvarez-Salgado, X. A., Fernández-Reiriz, M. J., Piedracoba, S., and Labarta, U. (2014). A modeling study on the hydrodynamics of a coastal embayment occupied by mussel farms (Ria de Ares-Betanzos, NW Iberian Peninsula). Estuarine, Coastal Shelf Sci. 147, 42–55. doi:10.1016/j.ecss.2014.05.021
Dudgeon, D. (2019). Multiple Threats Imperil Freshwater Biodiversity in the Anthropocene. Curr. Biol. 29 (19), R960–R967. doi:10.1016/j.cub.2019.08.002
Duggan, M., Bayliss, P., and Burford, M. A. (2019). Predicting the Impacts of Freshwater-Flow Alterations on Prawn (Penaeus Merguiensis) Catches. Fish. Res. 215, 27–37. doi:10.1016/j.fishres.2019.02.013
Durocher, M., Requena, A. I., Burn, D. H., and Pellerin, J. (2019). Analysis of Trends in Annual Streamflow to the Arctic Ocean. Hydrological Process. 33 (7), 1143–1151. doi:10.1002/hyp.13392
Fleming, B. J., Archfield, S. A., Hirsch, R. M., Kiang, J. E., and Wolock, D. M. (2020). Spatial and Temporal Patterns of Low Streamflow and Precipitation Changes in the Chesapeake Bay Watershed. J. Am. Water Resour. Assoc. 57 (1), 96–108. doi:10.1111/1752-1688.12892
Fonseca, V. F., Reis-Santos, P., Duarte, B., Cabral, H. N., Caçador, M. I., Vaz, N., et al. (2020). Roving Pharmacies: Modelling the Dispersion of Pharmaceutical Contamination in Estuaries. Ecol. Indicators 115, 106437. doi:10.1016/j.ecolind.2020.106437
Gamboa-García, D. E., Duque, G., Cogua, P., and Marrugo-Negrete, J. L. (2020). Mercury Dynamics in Macroinvertebrates in Relation to Environmental Factors in a Highly Impacted Tropical Estuary: Buenaventura Bay, Colombian Pacific. Environ. Sci. Pollut. Res. 27 (4), 4044–4057. doi:10.1007/s11356-019-06970-6
Gantzer, P. A., Bryant, L. D., and Little, J. C. (2009). Effect of Hypolimnetic Oxygenation on Oxygen Depletion Rates in Two Water-Supply Reservoirs. Water Res. 43 (6), 1700–1710. doi:10.1016/j.watres.2008.12.053
Gaonkar, C. V., and Matta, V. M. (2019). Impact of Mining on Metal Concentration in Waters of the Zuari Estuary, India. Environ. Monit. Assess. 191 (6). doi:10.1007/s10661-019-7506-0
Gillanders, B., and Kingsford, M. (2002). Impact of Changes in Flow of Freshwater on Estuarine and Open Coastal Habitats and the Associated Organisms. Oceanography Mar. Biol. 40, 233–309. doi:10.1201/9780203180594.ch5
Gillanders, B. M., Elsdon, T. S., Halliday, I. A., Jenkins, G. P., Robins, J. B., and Valesini, F. J. (2011). Potential Effects of Climate Change on Australian Estuaries and Fish Utilising Estuaries: a Review. Mar. Freshw. Res. 62, 1115–1131. doi:10.1071/MF11047
Gillson, J., Suthers, I., and Scandol, J. (2012). Effects of Flood and Drought Events on Multi-Species, Multi-Method Estuarine and Coastal Fisheries in Eastern Australia. Fish. Manag. Ecol. 19 (1), 54–68. doi:10.1111/j.1365-2400.2011.00816.x
Gippel, C., Anderson, B., Harty, C., Bond, N., Sherwood, J., and Pope, A. (2009). Gap Analysis and Strategy Development for National Level Estuary Environmental Flows Policies. Canberra, ACT: National Water Commission.
Glover, H. E., Ogston, A. S., Miller, I. M., Eidam, E. F., Rubin, S. P., and Berry, H. D. (2019). Impacts of Suspended Sediment on Nearshore Benthic Light Availability Following Dam Removal in a Small Mountainous River: In Situ Observations and Statistical Modeling. Estuaries and Coasts 42 (7), 1804–1820. doi:10.1007/s12237-019-00602-5
Godfrey, P. C., Arthington, A. H., Pearson, R. G., Karim, F., and Wallace, J. (2017). Fish Larvae and Recruitment Patterns in Floodplain Lagoons of the Australian Wet Tropics. Mar. Freshw. Res. 68 (5), 964–979. doi:10.1071/mf15421
Goodrich, D. M., Boicourt, W. C., Hamilton, P., and Pritchard, D. W. (1987). Wind-induced Destratification in Chesapeake Bay. J. Phys. Oceanogr. 17 (12), 2232–2240. doi:10.1175/1520-0485(1987)017<2232:widicb>2.0.co;2
Gorman, D., Sumida, P. Y. G., Figueira, R. C. L., and Turra, A. (2020). Improving Soil Carbon Estimates of Mudflats in Araçá Bay Using Spatial Models that Consider Riverine Input, Wave Exposure and Biogeochemistry. Estuarine, Coastal Shelf Sci. 238, 106734. doi:10.1016/j.ecss.2020.106734
Greve, P., Gudmundsson, L., and Seneviratne, S. I. (2018). Regional Scaling of Annual Mean Precipitation and Water Availability with Global Temperature Change. Earth Syst. Dynam. 9 (1), 227–240. doi:10.5194/esd-9-227-2018
Gudmundsson, L., Boulange, J., Do, H. X., Gosling, S. N., Grillakis, M. G., Koutroulis, A. G., et al. (2021). Globally Observed Trends in Mean and Extreme River Flow Attributed to Climate Change. Science 371, 1159–1162. doi:10.1126/science.aba3996
Gudmundsson, L., Leonard, M., Do, H. X., Westra, S., and Seneviratne, S. I. (2019). Observed Trends in Global Indicators of Mean and Extreme Streamflow. Geophys. Res. Lett. 46 (2), 756–766. doi:10.1029/2018gl079725
Haddeland, I., Heinke, J., Biemans, H., Eisner, S., Flörke, M., Hanasaki, N., et al. (2014). Global Water Resources Affected by Human Interventions and Climate Change. Proc. Natl. Acad. Sci. USA 111 (9), 3251–3256. doi:10.1073/pnas10.1073/pnas.1222475110
Haddout, S., and Maslouhi, A. (2018). One-dimensional Hydraulic Analysis of the Effect of Sea Level Rise on Salinity Intrusion in the Sebou Estuary, Morocco. Mar. Geodesy 41 (3), 270–288. doi:10.1080/01490419.2017.1420713
Haines, A. T., Finlayson, B. L., and McMahon, T. A. (1988). A Global Classification of River Regimes. Appl. Geogr. 8 (4), 255–272. doi:10.1016/0143-6228(88)90035-5
Hallett, C. S., Hobday, A. J., Tweedley, J. R., Thompson, P. A., McMahon, K., and Valesini, F. J. (2018). Observed and Predicted Impacts of Climate Change on the Estuaries of South-Western Australia, a Mediterranean Climate Region. Reg. Environ. Change 18 (5), 1357–1373. doi:10.1007/s10113-017-1264-8
Hamilton, D. P., Chan, T., Robb, M. S., Pattiaratchi, C. B., and Herzfeld, M. (2001). The Hydrology of the Upper Swan River Estuary with Focus on an Artificial Destratification Trial. Hydrol. Process. 15 (13), 2465–2480. doi:10.1002/hyp.298
Hamilton, S., Bartell, S., Pierson, J., and Murphy, D. (2020). Factors Controlling Calanoid Copepod Biomass and Distribution in the Upper San Francisco Estuary and Implications for Managing the Imperiled Delta Smelt (Hypomesus Transpacificus). Environ. Manage. 65 (5), 587–601. doi:10.1007/s00267-020-01267-8
Harris, G. P. (2001). Biogeochemistry of Nitrogen and Phosphorus in Australian Catchments, Rivers and Estuaries: Effects of Land Use and Flow Regulation and Comparisons with Global Patterns. Mar. Freshw. Res. 52, 139–149. doi:10.1071/mf00031
Hasenbein, M., Komoroske, L. M., Connon, R. E., Geist, J., and Fangue, N. A. (2013). Turbidity and Salinity Affect Feeding Performance and Physiological Stress in the Endangered delta Smelt. Integr. Comp. Biol. 53 (4), 620–634. doi:10.1093/icb/ict082
Henderson, P. A. (2019). A Long-Term Study of whiting, Merlangius Merlangus (L) Recruitment and Population Regulation in the Severn Estuary, UK. J. Sea Res. 155, 101825. doi:10.1016/j.seares.2019.101825
Hipsey, M. R., Gal, G., Arhonditsis, G. B., Carey, C. C., Elliott, J. A., Frassl, M. A., et al. (2020). A System of Metrics for the Assessment and Improvement of Aquatic Ecosystem Models. Environ. Model. Softw. 128, 104697. doi:10.1016/j.envsoft.2020.104697
Hipsey, M. R., Hamilton, D. P., Hanson, P. C., Carey, C. C., Coletti, J. Z., Read, J. S., et al. (2015). Predicting the Resilience and Recovery of Aquatic Systems: A Framework for Model Evolution within Environmental Observatories. Water Resour. Res. 51 (9), 7023–7043. doi:10.1002/2015wr017175
Hoerling, M., Barsugli, J., Livneh, B., Eischeid, J., Quan, X., and Badger, A. (2019). Causes for the century-long Decline in Colorado River Flow. J. Clim. 32 (23), 8181–8203. doi:10.1175/jcli-d-19-0207.1
Hong, X., Huang, W., Johnson, E., Lou, S., and Wan, W. (2014). “Effects of Sea Level Rise on Salinity Intrusion in St. Marks River Estuary, Florida, U.S.A,” in Climate Change Impatcs on Surface-Water Systems. Editors W. Huang, and S. C. Hagen (Coconut Creek, Florida, USA: Journal of Coastal Research, Special Issue).
Hood, R. R., Shenk, G. W., Dixon, R. L., Smith, S. M. C., Ball, W. P., Bash, J. O., et al. (2021). The Chesapeake Bay Program Modeling System: Overview and Recommendations for Future Development. Ecol. Model. 456, 109635. doi:10.1016/j.ecolmodel.2021.109635
Howarth, R., Chan, F., Conley, D. J., Garnier, J., Doney, S. C., Marino, R., et al. (2011). Coupled Biogeochemical Cycles: Eutrophication and Hypoxia in Temperate Estuaries and Coastal marine Ecosystems. Front. Ecol. Environ. 9 (1), 18–26. doi:10.1890/100008
Hsu-Kim, H., Eckley, C. S., Achá, D., Feng, X., Gilmour, C. C., Jonsson, S., et al. (2018). Challenges and Opportunities for Managing Aquatic Mercury Pollution in Altered Landscapes. Ambio 47 (2), 141–169. doi:10.1007/s13280-017-1006-7
Hu, X., Yao, H., Staryk, C. J., McCutcheon, M. R., Wetz, M. S., and Walker, L. (2020). Disparate Responses of Carbonate System in Two Adjacent Subtropical Estuaries to the Influence of Hurricane Harvey - A Case Study. Front. Mar. Sci. 7. doi:10.3389/fmars.2020.00026
Huang, P., Hennig, K., Kala, J., Andrys, J., and Hipsey, M. R. (2020). Climate Change Overtakes Coastal Engineering as the Dominant Driver of Hydrological Change in a Large Shallow Lagoon. Hydrol. Earth Syst. Sci. 24 (11), 5673–5697. doi:10.5194/hess-24-5673-2020
Huang, P., Kilminster, K., Larsen, S., and Hipsey, M. R. (2018). Assessing Artificial Oxygenation in a Riverine Salt-Wedge Estuary with a Three-Dimensional Finite-Volume Model. Ecol. Eng. 118, 111–125. doi:10.1016/j.ecoleng.2018.04.020
Huang, W., Hagen, S. C., Wang, D., Hovenga, P. A., Teng, F., and Weishampel, J. F. (2016). Suspended Sediment Projections in Apalachicola Bay in Response to Altered River Flow and Sediment Loads under Climate Change and Sea Level Rise. Earth's Future 4 (10), 428–439. doi:10.1002/2016EF000384
IPCC (2014). in Climate Change 2014: Synthesis Report. Contribution of Working Groups I, II and III to the Fifth Assessment Report of the Intergovernmental Panel on Climate Change. Editors R. K. Pachauri, and L. A. Meyer (Geneva, Switzerland: IPCC).
Ibáñez, C., and Caiola, N. (2013). Impacts of Water Scarcity and Drought on Iberian Aquatic Ecosystems, in Drought in Arid and Semi-Arid Regions: A Multi-Disciplinary and Cross-Country Perspective, 169–184. doi:10.1007/978-94-007-6636-5_9
Izegaegbe, J., Vivier, L., and Mzimela, H. (2020). Macrobenthic Community Structure of the Mhlathuze Estuary, a Permanently Open Estuarine Embayment in KwaZulu-Natal, South Africa. Afr. J. Aquat. Sci. 45 (1-2), 95–107. doi:10.2989/16085914.2020.1719818
Jenkins, G., Conron, S., and Morison, A. (2010). Highly Variable Recruitment in an Estuarine Fish Is Determined by Salinity Stratification and Freshwater Flow: Implications of a Changing Climate. Mar. Ecol. Prog. Ser. 417, 249–261. doi:10.3354/meps08806
Jensen, H. S., Mortensen, P. B., Andersen, F., Rasmussen, E., and Jensen, A. (1995). Phosphorus Cycling in a Coastal marine Sediment, Aarhus Bay, Denmark. Limnol. Oceanogr. 40 (5), 908–917. doi:10.4319/lo.1995.40.5.0908
Jutras, M., Mucci, A., Sundby, B., Gratton, Y., and Katsev, S. (2020). Nutrient Cycling in the Lower St. Lawrence Estuary: Response to Environmental Perturbations. Estuarine, Coastal Shelf Sci. 239, 106715. doi:10.1016/j.ecss.2020.106715
Kanaya, G., Suzuki, T., and Kikuchi, E. (2011). Spatio-temporal Variations in Macrozoobenthic Assemblage Structures in a River-Affected Lagoon (Idoura Lagoon, Sendai Bay, Japan): Influences of Freshwater Inflow. Estuarine, Coastal Shelf Sci. 92 (1), 169–179. doi:10.1016/j.ecss.2010.12.029
Kattel, G. R., Dong, X., and Yang, X. (2016). A century-scale, Human-Induced Ecohydrological Evolution of Wetlands of Two Large River Basins in Australia (Murray) and China (Yangtze). Hydrol. Earth Syst. Sci. 20 (6), 2151–2168. doi:10.5194/hess-20-2151-2016
Kench, P. S. (1999). Geomorphology of Australian Estuaries: Review and prospect. Aust. J. Ecol. 24, 367–380. doi:10.1046/j.1442-9993.1999.00985.x
Khojasteh, D., Glamore, W., Heimhuber, V., and Felder, S. (2021). Sea Level Rise Impacts on Estuarine Dynamics: A Review. Sci. Total Environ. 780, 146470. doi:10.1016/j.scitotenv.2021.146470
Kim, D., Aldridge, K. T., Ganf, G. G., and Brookes, J. D. (2015). Physicochemical Influences on Ruppia Tuberosa Abundance and Distribution Mediated through Life Cycle Stages. Iw 5 (4), 451–460. doi:10.5268/iw-5.4.709
Kim, D. H., Aldridge, K. T., Brookes, J. D., and Ganf, G. G. (2013). The Effect of Salinity on the Germination of Ruppia Tuberosa and Ruppia Megacarpa and Implications for the Coorong: A Coastal Lagoon of Southern Australia. Aquat. Bot. 111, 81–88. doi:10.1016/j.aquabot.2013.06.008
Kim, Y. H., Hong, S., Song, Y. S., Lee, H., Kim, H.-C., Ryu, J., et al. (2017). Seasonal Variability of Estuarine Dynamics Due to Freshwater Discharge and its Influence on Biological Productivity in Yeongsan River Estuary, Korea. Chemosphere 181, 390–399. doi:10.1016/j.chemosphere.2017.04.085
Kimmerer, W., and Weaver, M. J. (2013). Vulnerability of Estuaries to Climate Change, in Climate Vulnerability: Understanding and Addressing Threats to Essential Resources, 271–292. doi:10.1016/b978-0-12-384703-4.00438-x
Kingsford, R. T., Walker, K. F., Lester, R. E., Young, W. J., Fairweather, P. G., Sammut, J., et al. (2011). A Ramsar Wetland in Crisis - the Coorong, Lower Lakes and Murray Mouth, Australia. Mar. Freshw. Res. 62, 255–265. doi:10.1071/mf09315
Kiwango, H., Njau, K. N., and Wolanski, E. (2015). The Need to Enforce Minimum Environmental Flow Requirements in Tanzania to Preserve Estuaries: Case Study of Mangrove-Fringed Wami River Estuary. Ecohydrology & Hydrobiology 15 (4), 171–181. doi:10.1016/j.ecohyd.2015.09.002
Kjerfve, B. (1994). Chapter 1 Coastal Lagoons, in Coastal Lagoon Processes, 1–8. doi:10.1016/s0422-9894(08)70006-0
Koraim, A. S., Heikal, E. M., and Abo Zaid, A. A. (2011). Different Methods Used for Protecting Coasts from Sea Level Rise Caused by Climate Change. Curr. Dev. Oceanography 3 (1), 33–66.
Kotzé, P. (2016). Protecting Nature's Nurseries - Study Highlights Importance of Natural Flow to Estuaries. The Water Wheel 15 (4), 12–16.
Krauss, K. W., McKee, K. L., Lovelock, C. E., Cahoon, D. R., Saintilan, N., Reef, R., et al. (2014). How Mangrove Forests Adjust to Rising Sea Level. New Phytol. 202 (1), 19–34. doi:10.1111/nph.12605
Krvavica, N., Travaš, V., and Ožanić, N. (2017). Salt-Wedge Response to Variable River Flow and Sea-Level Rise in the Microtidal Rječina River Estuary, Croatia. J. Coastal Res. 33 (4), 802–814. doi:10.2112/jcoastres-d-16-00053.1
Larsen, S. J., Kilminster, K. L., Mantovanelli, A., Goss, Z. J., Evans, G. C., Bryant, L. D., et al. (2019). Artificially Oxygenating the Swan River Estuary Increases Dissolved Oxygen Concentrations in the Water and at the Sediment Interface. Ecol. Eng. 128, 112–121. doi:10.1016/j.ecoleng.2018.12.032
Lauchlan, S. S., and Nagelkerken, I. (2020). Species Range Shifts along Multistressor Mosaics in Estuarine Environments under Future Climate. Fish Fish 21 (1), 32–46. doi:10.1111/faf.12412
Lavery, S., and Donovan, B. (2005). Flood Risk Management in the Thames Estuary Looking Ahead 100 Years. Phil. Trans. R. Soc. A. 363 (1831), 1455–1474. doi:10.1098/rsta.2005.1579
Le Pape, O., Modéran, J., Beaunée, G., Riera, P., Nicolas, D., Savoye, N., et al. (2013). Sources of Organic Matter for Flatfish Juveniles in Coastal and Estuarine nursery Grounds: A Meta-Analysis for the Common Sole (Solea solea) in Contrasted Systems of Western Europe. J. Sea Res. 75, 85–95. doi:10.1016/j.seares.2012.05.003
Leblanc, M., Tweed, S., Van Dijk, A., and Timbal, B. (2012). A Review of Historic and Future Hydrological Changes in the Murray-Darling Basin. Glob. Planet. Change 80-81, 226–246. doi:10.1016/j.gloplacha.2011.10.012
Lee, W., and Kuhn, N. (2019). Multivariate Approach for Identifying Environmental Indicator Species in Estuarine Systems. Mar. Ecol. Prog. Ser. 611, 19–29. doi:10.3354/meps12864
Lester, R. E., Fairweather, P. G., Webster, I. T., and Quin, R. A. (2013). Scenarios Involving Future Climate and Water Extraction: Ecosystem States in the Estuary of Australia's Largest River. Ecol. Appl. 23 (5), 984–998. doi:10.2307/2344160110.1890/12-1331.1
Leterme, S. C., Allais, L., Jendyk, J., Hemraj, D. A., Newton, K., Mitchell, J., et al. (2015). Drought Conditions and Recovery in the Coorong Wetland, South Australia in 1997-2013. Estuarine, Coastal Shelf Sci. 163, 175–184. doi:10.1016/j.ecss.2015.06.009
Li, X., Leonardi, N., and Plater, A. J. (2019). Impact of Barrier Breaching on Wetland Ecosystems under the Influence of Storm Surge, Sea-Level Rise and Freshwater Discharge. Wetlands 40, 771–785. doi:10.1007/s13157-019-01219-x
Liang, B., Hu, J. T., Li, S. Y., Ye, Y. X., Liu, D. H., and Huang, J. (2020). Carbon System Simulation in the Pearl River Estuary, China: Mass Fluxes and Transformations. J. Geophys. Res. Biogeosci. 125 (2). doi:10.1029/2019JG005012
Lill, A. W. T., Closs, G. P., Schallenberg, M., and Savage, C. (2012). Impact of Berm Breaching on Hyperbenthic Macroinvertebrate Communities in Intermittently Closed Estuaries. Estuaries and Coasts 35, 155–168. doi:10.1007/s12237-011-9436-0
Liu, C., Yu, M., Cai, H., and Chen, X. (2019a). Recent Changes in Hydrodynamic Characteristics of the Pearl River Delta during the Flood Period and Associated Underlying Causes. Ocean Coastal Manag. 179, 104814. doi:10.1016/j.ocecoaman.2019.104814
Liu, M., Xie, H., He, Y., Zhang, Q., Sun, X., Yu, C., et al. (2019b). Sources and Transport of Methylmercury in the Yangtze River and the Impact of the Three Gorges Dam. Water Res. 166, 115042. doi:10.1016/j.watres.2019.115042
Livingston, R. J. (1996). Eutrophication in Estuaries and Coastal Systems: Relationships of Physical Alterations, Salinity Stratification, and Hypoxia. Columbia: Univ South Carolina Press.
Loik, M. E., Breshears, D. D., Lauenroth, W. K., and Belnap, J. (2004). A Multi-Scale Perspective of Water Pulses in Dryland Ecosystems: Climatology and Ecohydrology of the Western USA. Oecologia 141 (2), 269–281. doi:10.1007/s00442-004-1570-y
Lueangthuwapranit, C., Sampantarak, U., and Wongsai, S. (2011). Distribution and Abundance of Phytoplankton: Influence of Salinity and Turbidity Gradients in the Na Thap River, Songkhla Province, Thailand. J. Coastal Res. 27 (3), 585–594. doi:10.2112/JCOASTRES-D-10-00123.1
Lund-Hansen, L. C., Petersson, M., and Nurjaya, W. (1999). Vertical Sediment Fluxes and Wave-Induced Sediment Resuspension in a Shallow-Water Coastal Lagoon. Estuaries 22 (1), 39–46. doi:10.2307/1352925
Lytle, D. A., and Poff, N. L. (2004). Adaptation to Natural Flow Regimes. Trends Ecol. Evol. 19 (2), 94–100. doi:10.1016/j.tree.2003.10.002
Maier, G., Nimmo-Smith, R. J., Glegg, G. A., Tappin, A. D., and Worsfold, P. J. (2009). Estuarine Eutrophication in the UK: Current Incidence and Future Trends. Aquat. Conserv: Mar. Freshw. Ecosyst. 19 (1), 43–56. doi:10.1002/aqc.982
Markull, K., Lencart E Silva, J. D., Simpson, J. H., and Dias, J. M. (2014). The Influence of the Maputo and Incomati Rivers on the Mixing and Outflow of Freshwater from Maputo Bay (Mozambique). J. Coastal Res. 70, 580–585. doi:10.2112/SI70-098.1
Mbandzi, N., Wasserman, R. J., Deyzel, S. H. P., Vine, N. G., and Whitfield, A. K. (2018). River Flow, Zooplankton and Dominant Zooplanktivorous Fish Dynamics in a Warm-Temperate South African Estuary. J. Fish. Biol. 92 (6), 1747–1767. doi:10.1111/jfb.13617
McClelland, J. W., Holmes, R. M., Dunton, K. H., and Macdonald, R. W. (2012). The Arctic Ocean Estuary. Estuaries and Coasts 35 (2), 353–368. doi:10.1007/s12237-010-9357-3
McGrane, S. J. (2016). Impacts of Urbanisation on Hydrological and Water Quality Dynamics, and Urban Water Management: a Review. Hydrological Sci. J. 61 (13), 2295–2311. doi:10.1080/02626667.2015.1128084
McNaughton, C. (2018). Linking Flow, Nutrients and Fish: An Integrated Approach to Estuary Management. PhD: Monash University.
Medeiros dos Santos, A., and de Assis Esteves, F. (2002). Primary Production and Mortality of Eleocharis Interstincta in Response to Water Level Fluctuations. Aquat. Bot. 74, 189–199. doi:10.1016/s0304-3770(02)00082-7
Merg, M.-L., Dézerald, O., Kreutzenberger, K., Demski, S., Reyjol, Y., Usseglio-Polatera, P., et al. (2020). Modeling Diadromous Fish Loss from Historical Data: Identification of Anthropogenic Drivers and Testing of Mitigation Scenarios. PLoS One 15 (7), e0236575. doi:10.1371/journal.pone.0236575
Micklin, P. P. (1988). Desiccation of the Aral Sea: a Water Management Disaster in the Soviet Union. Science 241 (4870), 1170–1176. doi:10.1126/science.241.4870.1170
Milton, D. A. (2009). Living in Two Worlds: Diadromous Fishes, and Factors Affecting Population Connectivity between Tropical Rivers and Coasts, in Ecological Connectivity among Tropical Coastal Ecosystems, 325–355. doi:10.1007/978-90-481-2406-0_9
Mondal, A., and Mujumdar, P. P. (2012). On the basin‐scale Detection and Attribution of Human‐induced Climate Change in Monsoon Precipitation and Streamflow. Water Resour. Res. 48 (10). doi:10.1029/2011wr011468
Morais, P. (2008). Review on the Major Ecosystem Impacts Caused by Damming and Watershed Development in an Iberian basin (SW-Europe): Focus on the Guadiana Estuary. Ann. Limnol. - Int. J. Lim. 44 (2), 105–117. doi:10.1051/limn:2008012
Naiman, R. J., Latterell, J. J., Pettit, N. E., and Olden, J. D. (2008). Flow Variability and the Biophysical Vitality of River Systems. Comptes Rendus Geosci. 340 (9-10), 629–643. doi:10.1016/j.crte.2008.01.002
Nelson, S. M., Zamora-Arroyo, F., Ramírez-Hernández, J., and Santiago-Serrano, E. (2013). Geomorphology of a Recurring Tidal Sandbar in the Estuary of the colorado River, Mexico: Implications for Restoration. Ecol. Eng. 59, 121–133. doi:10.1016/j.ecoleng.2012.12.095
Nicol, J., Gehrig, S., Ganf, G. G., and Paton, D. C. (2018). “Aquatic and Littoral Vegetation,” in Natural History of the Coorong, Lower Lakes, and Murray Mouth Region (Yarluwar-Ruwe). Editors L. Mosley, Q. Ye, S. Shepherd, S. Hemming, and R. Fitzpatrick (Adelaide, South Australia: University of Adelaide Press), 292–316. doi:10.20851/natural-history-cllmm-3.2
Niemistö, J., and Lund-Hansen, L. C. (2019). Instantaneous Effects of Sediment Resuspension on Inorganic and Organic Benthic Nutrient Fluxes at a Shallow Water Coastal Site in the Gulf of Finland, Baltic Sea. Estuaries and Coasts 42 (8), 2054–2071. doi:10.1007/s12237-019-00648-5
Nijssen, B., O'Donnell, G. M., Hamlet, A. F., and Lettenmaier, D. P. (2001). Hydrologic Sensitivity of Global Rivers to Climate Change. Clim. Change 50, 143–175. doi:10.1023/a:1010616428763
Nordlie, F. G. (2003). Fish Communities of Estuarine Salt Marshes of Eastern North America, and Comparisons with Temperate Estuaries of Other Continents. Rev. Fish Biol. Fish. 13, 281–325. doi:10.1023/b:rfbf.0000033050.51699.84
Norkko, J., Hewitt, J. E., and Thrush, S. F. (2006). Effects of Increased Sedimentation on the Physiology of Two Estuarine Soft-Sediment Bivalves, Austrovenus Stutchburyi and Paphies Australis. J. Exp. Mar. Biol. Ecol. 333 (1), 12–26. doi:10.1016/j.jembe.2005.11.015
Olds, H. T., Corsi, S. R., Dila, D. K., Halmo, K. M., Bootsma, M. J., and McLellan, S. L. (2018). High Levels of Sewage Contamination Released from Urban Areas after Storm Events: A Quantitative Survey with Sewage Specific Bacterial Indicators. Plos Med. 15 (7), e1002614. doi:10.1371/journal.pmed.1002614
Olsen, S. B., Padma, T. V., and Richter, B. D. (2006). Managing Freshwater Inflows to Estuaries: A Methods Guide. Washington, U.S.A.: U.S. Agency for International Development.
Ortmann, A., Metzger, R., Liefer, J., and Novoveska, L. (2011). Grazing and Viral Lysis Vary for Different Components of the Microbial Community across an Estuarine Gradient. Aquat. Microb. Ecol. 65 (2), 143–157. doi:10.3354/ame01544
Osburn, C. L., Rudolph, J. C., Paerl, H. W., Hounshell, A. G., and Van Dam, B. R. (2019). Lingering Carbon Cycle Effects of Hurricane Matthew in North Carolina's Coastal Waters. Geophys. Res. Lett. 46 (5), 2654–2661. doi:10.1029/2019GL082014
Panda, U. S., Mahanty, M. M., Ranga Rao, V., Patra, S., and Mishra, P. (2015). Hydrodynamics and Water Quality in Chilika Lagoon-A Modelling Approach, in Procedia Engineering, 639–646.
Park, K., Powers, S. P., Bosarge, G. S., and Jung, H.-S. (2014). Plugging the Leak: Barrier Island Restoration Following Hurricane Katrina Enhances Larval Retention and Improves Salinity Regime for Oysters in Mobile Bay, Alabama. Mar. Environ. Res. 94, 48–55. doi:10.1016/j.marenvres.2013.12.003
Pasquaud, S., Vasconcelos, R. P., França, S., Henriques, S., Costa, M. J., and Cabral, H. (2015). Worldwide Patterns of Fish Biodiversity in Estuaries: Effect of Global vs. Local Factors. Estuarine, Coastal Shelf Sci. 154, 122–128. doi:10.1016/j.ecss.2014.12.050
Payne, A. R., Burdick, D. M., and Moore, G. E. (2019). Potential Effects of Sea-Level Rise on Salt Marsh Elevation Dynamics in a New Hampshire Estuary. Estuaries and Coasts 42 (6), 1405–1418. doi:10.1007/s12237-019-00589-z
Peñas, F. J., Juanes, J. A., Galván, C., Medina, R., Castanedo, S., Álvarez, C., et al. (2013). Estimating Minimum Environmental Flow Requirements for Well-Mixed Estuaries in Spain. Estuarine, Coastal Shelf Sci. 134, 138–149. doi:10.1016/j.ecss.2013.05.020
Perry, J. E., and Hershner, C. H. (1999). Temporal Changes in the Vegetation Pattern in a Tidal Freshwater Marsh. Wetlands 19 (1), 90–99. doi:10.1007/bf03161737
Piazza, B. P., and La Peyre, M. K. (2012). Measuring Changes in Consumer Resource Availability to Riverine Pulsing in Breton Sound, Louisiana, USA. PLoS ONE 7 (5), e37536. doi:10.1371/journal.pone.0037536
Piazza, B. P., and La Peyre, M. K. (2011). Nekton Community Response to a Large-Scale Mississippi River Discharge: Examining Spatial and Temporal Response to River Management. Estuarine, Coastal Shelf Sci. 91 (3), 379–387. doi:10.1016/j.ecss.2010.11.001
Pinckney, J. L., Paerl, H. W., Tester, P., and Richardson, T. L. (2001). The Role of Nutrient Loading and Eutrophication in Estuarine Ecology. Environ. Health Perspect. 109 (5), 699–706. doi:10.2307/3454916
Pinto, P. J., Kondolf, G. M., and Wong, P. L. R. (2018). Adapting to Sea Level Rise: Emerging Governance Issues in the San Francisco Bay Region. Environ. Sci. Pol. 90, 28–37. doi:10.1016/j.envsci.2018.09.015
Pinto, R., and Marques, J. C. (2015). “Ecosystem Services in Estuarine Systems: Implications for Management,” in Ecosystem Services and River Basin Ecohydrology. Editors L. Chicharo, F. Müller, and N. Fohrer (Dordrecht, NL: Springer). doi:10.1007/978-94-017-9846-4_16
Poff, N. L., Allan, J. D., Bain, M. B., Karr, J. R., Prestegaard, K. L., Richter, B. D., et al. (1997). The Natural Flow Regime. Bioscience 47 (11), 769–784. doi:10.2307/1313099
Poff, N. L. (2018). Beyond the Natural Flow Regime? Broadening the Hydro-Ecological Foundation to Meet Environmental Flows Challenges in a Non-stationary World. Freshw. Biol. 63 (8), 1011–1021. doi:10.1111/fwb.13038
Poff, N. L., Olden, J. D., Merritt, D. M., and Pepin, D. M. (2007). Homogenization of Regional River Dynamics by Dams and Global Biodiversity Implications. Proc. Natl. Acad. Sci. 104 (14), 5732–5737. doi:10.1073/pnas.0609812104
Poff, N. L., and Zimmerman, J. K. H. (2010). Ecological Responses to Altered Flow Regimes: a Literature Review to Inform the Science and Management of Environmental Flows. Freshw. Biol. 55 (1), 194–205. doi:10.1111/j.1365-2427.2009.02272.x
Porter, E., Mason, R., and Sanford, L. (2010). Effect of Tidal Resuspension on Benthic-Pelagic Coupling in an Experimental Ecosystem Study. Mar. Ecol. Prog. Ser. 413, 33–53. doi:10.3354/meps08709
Postel, S. L., Daily, G. C., and Ehrlich, P. R. (1996). Human Appropriation of Renewable Fresh Water. Science 271 (5250), 785–788. doi:10.1126/science.271.5250.785
Potter, I. C., Chuwen, B. M., Hoeksema, S. D., and Elliott, M. (2010). The Concept of an Estuary: a Definition that Incorporates Systems Which Can Become Closed to the Ocean and Hypersaline. Estuarine, Coastal Shelf Sci. 87, 497–500. doi:10.1016/j.ecss.2010.01.021
Raman, R. K., Naskar, M., Sahu, S. K., Chandra, G., and Das, B. K. (2020). A Multi-Species Modelling Approach to Assess the Influence of Hydrological Regime on Commercial Fisheries in a Tropical River-Estuary System. Reg. Stud. Mar. Sci. 34, 101035. doi:10.1016/j.rsma.2019.101035
Richter, B. D., and Thomas, G. A. (2007). Restoring Environmental Flows by Modifying Dam Operations. Ecol. Soc. 12 (1), 26. doi:10.5751/es-02014-120112
Riddin, T., and Adams, J. B. (2010). The Effect of a Storm Surge Event on the Macrophytes of a Temporarily Open/closed Estuary, South Africa. Estuarine, Coastal Shelf Sci. 89 (1), 119–123. doi:10.1016/j.ecss.2010.06.004
Robins, P. E., Farkas, K., Cooper, D., Malham, S. K., and Jones, D. L. (2019). Viral Dispersal in the Coastal Zone: A Method to Quantify Water Quality Risk. Environ. Int. 126, 430–442. doi:10.1016/j.envint.2019.02.042
Robins, P. E., Skov, M. W., Lewis, M. J., Giménez, L., Davies, A. G., Malham, S. K., et al. (2016). Impact of Climate Change on UK Estuaries: A Review of Past Trends and Potential Projections. Estuarine, Coastal Shelf Sci. 169, 119–135. doi:10.1016/j.ecss.2015.12.016
Robson, B. J., and Hamilton, D. P. (2004). Three-dimensional Modelling of a Microcystis Bloom Event in the Swan River Estuary, Western Australia. Ecol. Model. 174 (1-2), 203–222. doi:10.1016/j.ecolmodel.2004.01.006
Roebig, J. H., McLaughlin, J. K., and Feller, M. J. (2017). Environmental Reviews and Case Studies: Restoring a Salt Marsh in a Highly Urbanized Environment of New York City: The Alley Park Restoration Project. Environ. Pract. 14 (1), 68–78. doi:10.1017/s1466046611000512
Romañach, S. S., Beerens, J. M., Patton, B. A., Chapman, J. P., and Hanson, M. R. (2019). Impacts of Saltwater Intrusion on Wetland Prey Production and Composition in a Historically Freshwater Marsh. Estuaries and Coasts 42 (6), 1600–1611. doi:10.1007/s12237-019-00572-8
Rottler, E., Francke, T., Bürger, G., and Bronstert, A. (2020). Long-term Changes in central European River Discharge for 1869-2016: Impact of Changing Snow Covers, Reservoir Constructions and an Intensified Hydrological Cycle. Hydrol. Earth Syst. Sci. 24 (4), 1721–1740. doi:10.5194/hess-24-1721-2020
Ruibal-Conti, A. L., Summers, R., Weaver, D., and Hipsey, M. R. (2013). Hydro-climatological Non-stationarity Shifts Patterns of Nutrient Delivery to an Estuarine System. Hydrol. Earth Syst. Sci. Discuss. 10, 11035–11092. doi:10.5194/hessd-10-11035-2013
Russ, E., and Palinkas, C. (2020). Evolving Sediment Dynamics Due to Anthropogenic Processes in Upper Chesapeake Bay. Estuarine, Coastal Shelf Sci. 235, 106596. doi:10.1016/j.ecss.2020.106596
Saintilan, N., and Wen, L. (2012). Environmental Predictors of Estuarine Fish Landings along a Temperate Coastline. Estuarine, Coastal Shelf Sci. 113, 221–230. doi:10.1016/j.ecss.2012.08.007
Sampath, D. M. R., and Boski, T. (2016). Morphological Response of the Saltmarsh Habitats of the Guadiana Estuary Due to Flow Regulation and Sea-Level Rise. Estuarine, Coastal Shelf Sci. 183, 314–326. doi:10.1016/j.ecss.2016.07.009
Santos, A. M. d., Amado, A. M., Minello, M., Farjalla, V. F., and Esteves, F. A. (2006). Effects of the Sand Bar Breaching on Typha Domingensis (PERS.) in a Tropical Coastal Lagoon. Hydrobiologia 556 (1), 61–68. doi:10.1007/s10750-005-1084-6
Santos, R. O., Schinbeckler, R., Viadero, N., Larkin, M. F., Rennert, J. J., Shenker, J. M., et al. (2019). Linking Bonefish (Albula vulpes) Populations to Nearshore Estuarine Habitats Using an Otolith Microchemistry Approach. Environ. Biol. Fish. 102 (2), 267–283. doi:10.1007/s10641-018-0839-7
Schallenberg, M., Larned, S. T., Hayward, S., and Arbuckle, C. (2010). Contrasting Effects of Managed Opening Regimes on Water Quality in Two Intermittently Closed and Open Coastal Lakes. Estuarine, Coastal Shelf Sci. 86 (4), 587–597. doi:10.1016/j.ecss.2009.11.001
Scharler, U., Lechman, K., Radebe, T., and Jerling, H. (2020). Effects of Prolonged Mouth Closure in a Temporarily Open/closed Estuary: a Summary of the Responses of Invertebrate Communities in the uMdloti Estuary, South Africa. Afr. J. Aquat. Sci. 45 (1-2), 121–130. doi:10.2989/16085914.2019.1689911
Schnurr, P. J., Drever, M. C., Elner, R. W., Harper, J., and Arts, M. T. (2020). Peak Abundance of Fatty Acids from Intertidal Biofilm in Relation to the Breeding Migration of Shorebirds. Front. Mar. Sci. 7. doi:10.3389/fmars.2020.00063
Schrandt, M. N., Powers, S. P., and Mareska, J. F. (2015). Habitat Use and Fishery Dynamics of a Heavily Exploited Coastal Migrant, Spanish Mackerel. North Am. J. Fish. Manag. 35 (2), 352–363. doi:10.1080/02755947.2015.1009659
Scully, M. E. (2010). Wind Modulation of Dissolved Oxygen in Chesapeake Bay. Estuaries and Coasts 33 (5), 1164–1175. doi:10.1007/s12237-010-9319-9
Serrano, M. A., Cobos, M., Magaña, P. J., and Díez-Minguito, M. (2020). Sensitivity of Iberian Estuaries to Changes in Sea Water Temperature, Salinity, River Flow, Mean Sea Level, and Tidal Amplitudes. Estuarine, Coastal Shelf Sci. 236, 106624. doi:10.1016/j.ecss.2020.106624
Sheaves, M. (1996). Do spatial Differences in the Abundance of Two Serranid Fishes in Estuaries of Tropical Australia Reflect Long-Term Salinity Patterns? Mar. Ecol. Prog. Ser. 137, 39–49. doi:10.3354/meps137039
Sheaves, M., Duc, N. H., and Khoa, N. X. (2008). Ecological Attributes of a Tropical River basin Vulnerable to the Impacts of Clustered Hydropower Developments. Mar. Freshw. Res. 59 (11), 971–986. doi:10.1071/mf08029
Sheaves, M., Johnston, R., Molony, B., and Shepard, G. (2007). The Effect of Impoundments on the Structure and Function of Fish Fauna in a Highly Regulated Dry Tropics Estuary. Estuaries Coasts: J. ERF 30 (3), 507–517. doi:10.1007/bf02819397
Sheaves, M. (1998). Spatial Patterns in Estuarine Fish Faunas in Tropical Queensland: A Reflection of Interaction between Long-Term Physical and Biological Processes? Mar. Freshw. Res. 49, 31–40. doi:10.1071/mf97019
Shen, C., Testa, J. M., Ni, W., Cai, W. J., Li, M., and Kemp, W. M. (2019). Ecosystem Metabolism and Carbon Balance in Chesapeake Bay: A 30‐Year Analysis Using a Coupled Hydrodynamic‐Biogeochemical Model. J. Geophys. Res. Oceans 124 (8), 6141–6153. doi:10.1029/2019JC015296
Shenk, G. W., and Linker, L. C. (2013). Development and Application of the 2010 Chesapeake Bay Watershed Total Maximum Daily Load Model. J. Am. Water Resour. Assoc. 49 (5), 1042–1056. doi:10.1111/jawr.12109
Shih, S.-S., Yang, S.-C., Lee, H.-Y., Hwang, G.-W., and Hsu, Y.-M. (2011). Development of a Salinity-Secondary Flow-Approach Model to Predict Mangrove Spreading. Ecol. Eng. 37 (8), 1174–1183. doi:10.1016/j.ecoleng.2011.02.018
Shumilova, O., Tockner, K., Thieme, M., Koska, A., and Zarfl, C. (2018). Global Water Transfer Megaprojects: A Potential Solution for the Water-Food-Energy Nexus? Front. Environ. Sci. 6. doi:10.3389/fenvs.2018.00150
Smith, B. D., Braulik, G., Strindberg, S., Mansur, R., Diyan, M. A. A., and Ahmed, B. (2009). Habitat Selection of Freshwater-dependent Cetaceans and the Potential Effects of Declining Freshwater Flows and Sea-Level Rise in Waterways of the Sundarbans Mangrove forest, Bangladesh. Aquat. Conserv: Mar. Freshw. Ecosyst. 19 (2), 209–225. doi:10.1002/aqc.987
Soares, A. R. A., and Berggren, M. (2019). Indirect Link between Riverine Dissolved Organic Matter and Bacterioplankton Respiration in a Boreal Estuary. Mar. Environ. Res. 148, 39–45. doi:10.1016/j.marenvres.2019.04.009
Sottolichio, A., Hanquiez, V., Périnotto, H., Sabouraud, L., and Weber, O. (2013). Evaluation of the Recent Morphological Evolution of the Gironde Estuary through the Use of Some Preliminary Synthetic Indicators. J. Coastal Res. 165, 1224–1229. doi:10.2112/SI65-20710.2112/si65-207.1
Statham, P. J. (2012). Nutrients in Estuaries - an Overview and the Potential Impacts of Climate Change. Sci. Total Environ. 434, 213–227. doi:10.1016/j.scitotenv.2011.09.088
Steichen, J. L., Labonté, J. M., Windham, R., Hala, D., Kaiser, K., Setta, S., et al. (2020). Microbial, Physical, and Chemical Changes in Galveston Bay Following an Extreme Flooding Event, Hurricane Harvey. Front. Mar. Sci. 7. doi:10.3389/fmars.2020.00186
Stein, E. D., Gee, E. M., Adams, J. B., Irving, K., and Van Niekerk, L. (2021). Advancing the Science of Environmental Flow Management for Protection of Temporarily Closed Estuaries and Coastal Lagoons. Water 13 (5), 595. doi:10.3390/w13050595
Stewardson, M. J., and Guarino, F. (2018). Basin‐scale Environmental Water Delivery in the Murray-Darling, Australia: A Hydrological Perspective. Freshw. Biol. 63 (8), 969–985. doi:10.1111/fwb.13102
Stewart, J., Hughes, J. M., Stanley, C., and Fowler, A. M. (2020). The Influence of Rainfall on Recruitment success and Commercial Catch for the Large Sciaenid, Argyrosomus Japonicus, in Eastern Australia. Mar. Environ. Res. 157, 104924. doi:10.1016/j.marenvres.2020.104924
Strohbach, M. W., Döring, A. O., Möck, M., Sedrez, M., Mumm, O., Schneider, A.-K., et al. (2019). The "Hidden Urbanization": Trends of Impervious Surface in Low-Density Housing Developments and Resulting Impacts on the Water Balance. Front. Environ. Sci. 7 (29). doi:10.3389/fenvs.2019.00029
Sun, T., Xu, J., and Yang, Z. F. (2013). Environmental Flow Assessments in Estuaries Based on an Integrated Multi-Objective Method. Hydrol. Earth Syst. Sci. 17 (2), 751–760. doi:10.5194/hess-17-751-2013
Sun, T., Zhang, H., Yang, Z., and Yang, W. (2015). Environmental Flow Assessments for Transformed Estuaries. J. Hydrol. 520, 75–84. doi:10.1016/j.jhydrol.2014.11.015
Taljaard, S., Adams, J. B., Turpie, J. K., Van Niekerk, L., Demetriades, N., Bate, G. C., et al. (2004). Water Resource protection and Assessment Policy Implementation Process. Resource Directed Measures for protection of Water Resource: Methodology for the Determination of the Ecological Water Requirements for Estuaries. Pretoria, South Africa: Department of Water Affairs and Forestry.
Tananaev, N. I., Makarieva, O. M., and Lebedeva, L. S. (2016). Trends in Annual and Extreme Flows in the Lena River basin, Northern Eurasia. Geophys. Res. Lett. 43 (20), 10764–10772. doi:10.1002/2016gl070796
Tango, P. J., and Batiuk, R. A. (2013). Deriving Chesapeake Bay Water Quality Standards. J. Am. Water Resour. Assoc. 49 (5), 1007–1024. doi:10.1111/jawr.12108
Testa, J. M., and Kemp, W. M. (2012). Hypoxia-induced Shifts in Nitrogen and Phosphorus Cycling in Chesapeake Bay. Limnol. Oceanogr. 57 (3), 835–850. doi:10.4319/lo.2012.57.3.0835
Testa, J. M., Murphy, R. R., Brady, D. C., and Kemp, W. M. (2018). Nutrient- and Climate-Induced Shifts in the Phenology of Linked Biogeochemical Cycles in a Temperate Estuary. Front. Mar. Sci. 5. doi:10.3389/fmars.2018.00114
Thrush, S. F., Halliday, J., Hewitt, J. E., and Lohrer, A. M. (2008). The Effects of Habitat Loss, Fragmentation, and Community Homogenization on Resilience in Estuaries. Ecol. Appl. 18 (1), 12–21. doi:10.1890/07-0436.1
Thrush, S. F., Townsend, M., Hewitt, J. E., Davies, K., Lohrer, A., Lundquist, C., et al. (2013). “The many Uses and Values of Estuarine Ecosystems,” in Ecosystem Services in New Zealand: Conditions and Trends (Lincoln, New Zealand: Manaaki Whenua Press).
Thrush, S., Hewitt, J., Cummings, V., Ellis, J., Hatton, C., Lohrer, A., et al. (2004). Muddy Waters: Elevating Sediment Input to Coastal and Estuarine Habitats. Front. Ecol. Environ. 2 (6), 299–306. doi:10.1890/1540-9295(2004)002[0299:mwesit]2.0.co;2
Toffolon, M., Ragazzi, M., Righetti, M., Teodoru, C. R., Tubino, M., Defrancesco, C., et al. (2013). Effects of Artificial Hypolimnetic Oxygenation in a Shallow lake. Part 1: Phenomenological Description and Management. J. Environ. Manage. 114, 520–529. doi:10.1016/j.jenvman.2012.10.062
Tonkin, J. D., Olden, J. D., Merritt, D. M., Reynolds, L. V., Rogosch, J. S., and Lytle, D. A. (2021). Designing Flow Regimes to Support Entire River Ecosystems. Front. Ecol. Environ. 19 (6), 326–333. doi:10.1002/fee.2348
Van Niekerk, L., Adams, J. B., Allan, D. G., Taljaard, S., Weerts, S. P., Louw, D., et al. (2019a). Assessing and Planning Future Estuarine Resource Use: A Scenario-Based Regional-Scale Freshwater Allocation Approach. Sci. Total Environ. 657, 1000–1013. doi:10.1016/j.scitotenv.2018.12.033
Van Niekerk, L., Taljaard, S., Adams, J. B., Lamberth, S. J., Huizinga, P., Turpie, J. K., et al. (2019b). An Environmental Flow Determination Method for Integrating Multiple-Scale Ecohydrological and Complex Ecosystem Processes in Estuaries. Sci. Total Environ. 656, 482–494. doi:10.1016/j.scitotenv.2018.11.276
Van Niekerk, L., Taljaard, S., and Huizinga, P. (2012). An Evaluation of the Ecological Flow Requirements of South Africa's Estuaries from a Hydrodynamics Perspective. WRC Report No. KV 302/12. Gezina, South Africa: Water Research Commission.
Vermeiren, P., and Sheaves, M. (2014). Predictable Habitat Associations of Four Crab Species across the Low Intertidal Landscape of a Tropical Estuary over Time. Estuaries and Coasts 38 (1), 285–295. doi:10.1007/s12237-014-9799-0
Vinagre, C., Madeira, C., Dias, M., Narciso, L., and Mendonça, V. (2019). Reliance of Coastal Intertidal Food Webs on River Input - Current and Future Perspectives. Ecol. Indicators 101, 632–639. doi:10.1016/j.ecolind.2019.01.064
Vinagre, C., Máguas, C., Cabral, H. N., and Costa, M. J. (2011a). Spatial Variation in River Runoff into a Coastal Area - an Ecological Approach. J. Sea Res. 65 (3), 362–367. doi:10.1016/j.seares.2011.02.003
Vinagre, C., Salgado, J., Cabral, H. N., and Costa, M. J. (2011b). Food Web Structure and Habitat Connectivity in Fish Estuarine Nurseries-Impact of River Flow. Estuaries and Coasts 34 (4), 663–674. doi:10.1007/s12237-010-9315-0
Vörösmarty, C. J., McIntyre, P. B., Gessner, M. O., Dudgeon, D., Prusevich, A., Green, P., et al. (2010). Global Threats to Human Water Security and River Biodiversity. Nature 467 (7315), 555–561. doi:10.1038/nature09440
Vörösmarty, C. J., Meybeck, M., Fekete, B., Sharma, K., Green, P., and Syvitski, J. P. M. (2003). Anthropogenic Sediment Retention: Major Global Impact from Registered River Impoundments. Glob. Planet. Change 39 (1-2), 169–190. doi:10.1016/s0921-8181(03)00023-7
Vörösmarty, C. J., and Sahagian, D. (2000). Anthropogenic Disturbance of the Terrestrial Water Cycle. BioScience 50 (9), 253–265. doi:10.1641/0006-3568(2000)050
Wagena, M. B., Collick, A. S., Ross, A. C., Najjar, R. G., Rau, B., Sommerlot, A. R., et al. (2018). Impact of Climate Change and Climate Anomalies on Hydrologic and Biogeochemical Processes in an Agricultural Catchment of the Chesapeake Bay Watershed, USA. Sci. Total EnvironmentSci Total Environ 637-638, 1443–1454. doi:10.1016/j.scitotenv.2018.05.116
Wałęga, A., Radecki-Pawlik, A., Cupak, A., Hathaway, J., and Pukowiec, M. (2019). Influence of Changes of Catchment Permeability and Frequency of Rainfall on Critical Storm Duration in an Urbanized Catchment-A Case Study, Cracow, Poland. Water 11 (12), 2557. doi:10.3390/w11122557
Waltham, N. J., Burrows, D., Wegscheidl, C., Buelow, C., Ronan, M., Connolly, N., et al. (2019). Lost Floodplain Wetland Environments and Efforts to Restore Connectivity, Habitat, and Water Quality Settings on the Great Barrier Reef. Front. Mar. Sci. 6 (71). doi:10.3389/fmars.2019.00071
Waltham, N. J., and Sheaves, M. (2015). Expanding Coastal Urban and Industrial Seascape in the Great Barrier Reef World Heritage Area: Critical Need for Coordinated Planning and Policy. Mar. Pol. 57, 78–84. doi:10.1016/j.marpol.2015.03.030
Waltham, N. J., Teasdale, P. R., and Connolly, R. M. (2013). Use of Flathead Mullet (Mugil cephalus) in Coastal Biomonitor Studies: Review and Recommendations for Future Studies. Mar. Pollut. Bull. 69, 195–205. doi:10.1016/j.marpolbul.2013.01.012
Wang, J., and Hong, B. (2021). Threat Posed by Future Sea-Level Rise to Freshwater Resources in the Upper Pearl River Estuary. Jmse 9 (3), 291. doi:10.3390/jmse9030291
Warfe, D. M., Pettit, N. E., Davies, P. M., Pusey, B. J., Hamilton, S. K., Kennard, M. J., et al. (2011). The 'wet-Dry' in the Wet-Dry Tropics Drives River Ecosystem Structure and Processes in Northern Australia. Freshw. Biol. 56 (11), 2169–2195. doi:10.1111/j.1365-2427.2011.02660.x
Watts, R. J., Richter, B. D., Opperman, J. J., and Bowmer, K. H. (2011). Dam Reoperation in an Era of Climate Change. Mar. Freshw. Res. 62, 321–327. doi:10.1071/mf10047
Webster, I. T. (2010). Dynamic Assessment of Oceanic Connectivity in a Coastal Lagoon-The Coorong, Australia. J. Coastal Res. 27 (1), 131–139. doi:10.2112/jcoastres-d-10-00079.1
Weng, X., Jiang, C., Zhang, M., Yuan, M., and Zeng, T. (2020). Numeric Study on the Influence of Sluice-Gate Operation on Salinity, Nutrients and Organisms in the Jiaojiang River Estuary, China. Water 12 (7), 2026. doi:10.3390/w12072026
Whitfield, A., Bate, G., Adams, J., Cowley, P., Froneman, P., Gama, P., et al. (2012). A Review of the Ecology and Management of Temporarily Open/closed Estuaries in South Africa, with Particular Emphasis on River Flow and Mouth State as Primary Drivers of These Systems. Afr. J. Mar. Sci. 34 (2), 163–180. doi:10.2989/1814232x.2012.675041
Widdows, J., Bale, A. J., Brinsley, M. D., Somerfield, P., and Uncles, R. J. (2007). An Assessment of the Potential Impact of Dredging Activity on the Tamar Estuary over the Last century: II. Ecological Changes and Potential Drivers. Hydrobiologia 588, 97–108. doi:10.1007/s10750-007-0655-0
Wikner, J., and Andersson, A. (2012). Increased Freshwater Discharge Shifts the Trophic Balance in the Coastal Zone of the Northern Baltic Sea. Glob. Change Biol. 18 (8), 2509–2519. doi:10.1111/j.1365-2486.2012.02718.x
Williams, J., Hindell, J. S., Swearer, S. E., and Jenkins, G. P. (2012). Influence of Freshwater Flows on the Distribution of Eggs and Larvae of Black Bream Acanthopagrus butcheri within a Drought-Affected Estuary. J. Fish Biol. 80 (6), 2281–2301. doi:10.1111/j.1095-8649.2012.03283.x
Williams, J., Jenkins, G., Hindell, J., and Swearer, S. (2013). Linking Environmental Flows with the Distribution of Black Bream Acanthopagrus butcheri Eggs, Larvae and Prey in a Drought Affected Estuary. Mar. Ecol. Prog. Ser. 483, 273–287. doi:10.3354/meps10280
Wolanski, E., Chicharo, L., Chicharo, M. A., and Morais, P. (2006). An Ecohydrology Model of the Guadiana Estuary (South Portugal). Estuarine, Coastal Shelf Sci. 70 (1-2), 132–143. doi:10.1016/j.ecss.2006.05.029
Woodland, R. J., Thomson, J. R., Mac Nally, R., Reich, P., Evrard, V., Wary, F. Y., et al. (2015). Nitrogen Loads Explain Primary Productivity in Estuaries at the Ecosystem Scale. Limnol. Oceanogr. 60 (5), 1751–1762. doi:10.1002/lno.10136
Wu, B., Wu, X., Shi, X., Qiao, S., Liu, S., Hu, L., et al. (2020). Influences of Tropical Monsoon Climatology on the Delivery and Dispersal of Organic Carbon over the Upper Gulf of Thailand. Mar. Geology. 426, 106209. doi:10.1016/j.margeo.2020.106209
Yan, G., Labonté, J. M., Quigg, A., and Kaiser, K. (2020). Hurricanes Accelerate Dissolved Organic Carbon Cycling in Coastal Ecosystems. Front. Mar. Sci. 7 (248). doi:10.3389/fmars.2020.00248
Yan, Y., Song, D., Bao, X., and Wang, N. (2021). The Response of Turbidity Maximum to Peak River Discharge in a Macrotidal Estuary. Water 13 (1), 106. doi:10.3390/w13010106
Yu, M., Wang, C., Liu, Y., Olsson, G., and Wang, C. (2018). Sustainability of Mega Water Diversion Projects: Experience and Lessons from China. Sci. Total Environ. 619-620, 721–731. doi:10.1016/j.scitotenv.2017.11.006
Zeiringer, B., Seliger, C., Greimel, F., and Schmutz, S. (2018). “River Hydrology, Flow Alteration, and Environmental Flow,” in Riverine Ecosystem Management: Science for Governing towards a Sustainable Future. Editors S. Schmutz, and J. Sendzimir (Cham, Switzerland: Springer). doi:10.1007/978-3-319-73250-3_4
Zevenbergen, C., Fu, D., and Pathirana, A. (2018). Transitioning to Sponge Cities: Challenges and Opportunities to Address Urban Water Problems in China. Water 10 (9), 1230. doi:10.3390/w10091230
Zhang, H., Sun, T., Xue, S., Yang, W., and Yin, X. (2017a). Environmental Flow Assessment in Estuaries Taking into Consideration Species Dispersal in Fragmented Potential Habitats. Ecol. Indicators 78, 541–548. doi:10.1016/j.ecolind.2017.03.042
Zhang, M., Cui, B., Zhang, Z., and Jiang, X. (2017b). Salinity-oriented Environmental Flows for keystone Species in the Modaomen Estuary, China. Front. Earth Sci. 11 (4), 670–681. doi:10.1007/s11707-016-0609-9
Zhang, Q., Murphy, R. R., Tian, R., Forsyth, M. K., Trentacoste, E. M., Keisman, J., et al. (2018). Chesapeake Bay's Water Quality Condition Has Been Recovering: Insights from a Multimetric Indicator Assessment of Thirty Years of Tidal Monitoring Data. Sci. Total Environ. 637-638, 1617–1625. doi:10.1016/j.scitotenv.2018.05.025
Keywords: environmental flows (E-flows), estuaries, freshwater flow requirements, freshwater flow alteration, ecosystem process and function, anthropogenic disturbance, climate change, mitigation and adaptation
Citation: Chilton D, Hamilton DP, Nagelkerken I, Cook P, Hipsey MR, Reid R, Sheaves M, Waltham NJ and Brookes J (2021) Environmental Flow Requirements of Estuaries: Providing Resilience to Current and Future Climate and Direct Anthropogenic Changes. Front. Environ. Sci. 9:764218. doi: 10.3389/fenvs.2021.764218
Received: 25 August 2021; Accepted: 27 October 2021;
Published: 17 November 2021.
Edited by:
Avril C. Horne, The University of Melbourne, AustraliaReviewed by:
Reinaldo Luiz Bozelli, Federal University of Rio de Janeiro, BrazilQian Zhang, University of Maryland Center for Environmental Science (UMCES), United States
Copyright © 2021 Chilton, Hamilton, Nagelkerken, Cook, Hipsey, Reid, Sheaves, Waltham and Brookes. This is an open-access article distributed under the terms of the Creative Commons Attribution License (CC BY). The use, distribution or reproduction in other forums is permitted, provided the original author(s) and the copyright owner(s) are credited and that the original publication in this journal is cited, in accordance with accepted academic practice. No use, distribution or reproduction is permitted which does not comply with these terms.
*Correspondence: Daniel Chilton, ZGFuaWVsLmNoaWx0b25AYWRlbGFpZGUuZWR1LmF1