- 1Emerging Pathogens Institute and Department of Plant Pathology, University of Florida, Gainesville, FL, United States
- 2Faculty of Organic Agricultural Sciences, Ecological Plant Protection, University of Kassel, Witzenhausen, Germany
- 3Department of Life and Environment Science, Hangzhou Normal University, Zhejiang, China
- 4Department of Environmental Sciences, Soil Physics and Land Management Group, Wageningen University, Wageningen, Netherlands
The herbicide glyphosate interferes with the shikimate pathway in plants and in major groups of microorganisms impeding the production of aromatic amino acids. Glyphosate application on plants results in a slow death, accelerated by reduced resistance to root pathogens. Extensive glyphosate use has resulted in increasing residues in soil and waterways. Although direct glyphosate effects on animals are limited, major concerns have arisen about indirect harmful side effects. In this paper, we focus on indirect effects of sublethal concentrations of glyphosate on plant, animal and human health due to shifts in microbial community compositions in successive habitats. Research results of glyphosate effects on microbial communities in soil, rhizosphere and animal guts have been contradictory due to the different integration levels studied. Most glyphosate studies have tested short-term treatment effects on microbial biomass or general community composition at higher taxonomic levels in soil, rhizosphere or animal intestinal tracts, and found little effect. More detailed studies showed reductions in specific genera or species as well as biological processes after glyphosate application. Plant growth promoting rhizobacteria and beneficial intestinal bacteria often are negatively affected, while pathogenic bacteria and fungi are enhanced. Such shifts in microbial community composition have been implicated in enhanced susceptibility of plants to Fusarium and Rhizoctonia, of birds and mammals to toxic Clostridium and Salmonella species, and of bees to Serratia and Deformed Wing Virus. In animals and humans, glyphosate exposure and concentrations in urine have been associated with intestinal diseases and neurological as well as endocrine problems, but cause-effect relationships need to be determined in more detail. Nevertheless, outbreaks of several animal and plant diseases have been related to glyphosate accumulation in the environment. Long-term glyphosate effects have been underreported, and new standards will be needed for residues in plant and animal products and the environment.
Introduction
The herbicide glyphosate, N-(phosphonomethyl) glycine, is a biocide with a broad-spectrum activity. It interferes with the enzyme 5-enolpyruvylshikimate-3-phosphate synthase (EPSPS) in the shikimate pathway in plants and major groups of fungi, bacteria, archaea and protozoa, impeding the production of aromatic amino acids (Rueda-Ruzafa et al., 2019; van Bruggen et al., 2018; Vázquez et al., 2021). These amino acids contribute to the production of lignin and antimicrobial phytoalexins that defend plants against pathogens (Duke, 2018). Consequently, death of glyphosate-treated non-genetically-modified plants is hastened by root pathogens (Defarge et al., 2018; Hammerschmidt, 2018; Rosenbaum et al., 2014).
Glyphosate is formulated as a salt with various adjuvants (Defarge et al., 2018), primarily surfactants such as polyoxyethylene amine (POEA, for example in Roundup®) to enhance uptake and translocation of the active ingredient in plants. The formulated product is transported throughout plants, including roots, resulting in plant death accelerated by reduced resistance to root pathogens (Defarge et al., 2018). Surfactants such as POEA have broad toxicity themselves (Defarge et al., 2018; Hammerschmidt, 2018; Straw et al., 2021; Woźniak et al., 2018), including negative effects on key biosynthetic pathways and on overall growth rates, for example of plant-beneficial Pseudomonas sp (Mendonca et al., 2019) and Lactobacillus sp (Clair E. et al., 2012). Because of its wide-ranging toxicity, formulations with POEA have been restricted or banned in the EU since 2016 (EC, 2016; EC, 2017; Székács and Darvas, 2018). Nevertheless, similar formulations have still been in use, and proved to be detrimental to bumble bees, even without glyphosate (Straw et al., 2021).
Glyphosate-based herbicides (GBHs) are used primarily before planting of traditional agricultural crops, after planting of glyphosate-resistant (GR) crops, and as a desiccator to facilitate harvesting of crops (van Bruggen et al., 2018). Glyphosate has been also widely used between trees in groves (Qiao et al., 2020; Zhang et al., 2015), in vineyards (Daouk et al., 2013; La Cecilia, 2018; Mandl et al., 2018; Zaller et al., 2018), and in urban areas (Poiger et al., 2017; Tauchnitz et al., 2020).
The total area treated with glyphosate has increased dramatically (Figure 1), especially since the introduction of GR crops (Myers et al., 2016) and end-of-season use to facilitate harvesting (van Bruggen et al., 2018). In addition, the annual glyphosate application rates per ha have often increased due to the emergence of glyphosate resistant weeds (Miyazaki et al., 2019; van Bruggen et al., 2018). Glyphosate use for agricultural production is now widespread, both in industrialized and developing countries (Benbrook, 2016; Maggi et al., 2020). Globally, about 700 thousand tonnes of glyphosate were applied on 36 million km (Acosta-Cortés et al., 2019) in 2015 (Maggi et al., 2019).
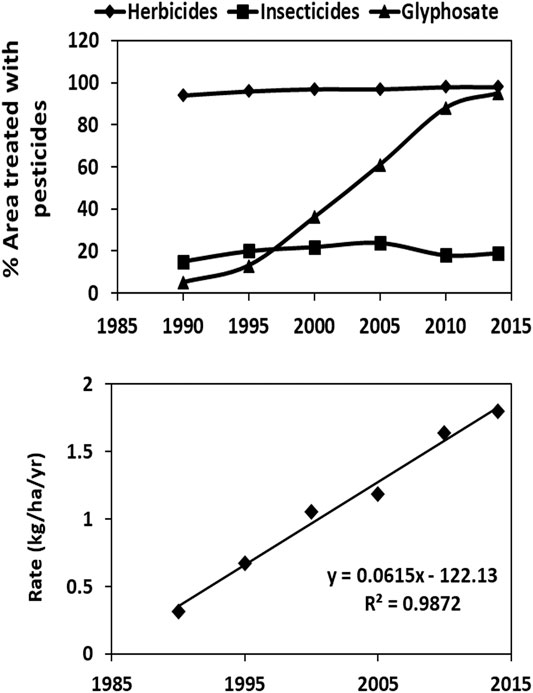
FIGURE 1. Increase in agricultural area treated with glyphosate (in comparison with total herbicides and insecticides) and in application rate on GR soybeans in the USA between 1990 and 2014 extracted from the United States Agricultural Statistics Service (NASS) website. http://www.nass.usda.gov/Surveys/Guide_to_NASS_Surveys.
Glyphosate is resistant to complete degradation due to the inert C-P linkage in the molecule (van Bruggen et al., 2018). It is broken down slowly in dead plant material, soil and water by various microorganisms (Carles et al., 2019; Grube et al., 2019; Helander et al., 2018; La Cecilia et al., 2018; Masotti et al., 2021; Xu et al., 2019; Zhao et al., 2015). The first decomposition product often is aminomethyl phosphonic acid, AMPA (Brock et al., 2019; Romano-Armada et al., 2019; Tang et al., 2019; Xu et al., 2019). In second generation GR crops that contain a gene coding for the enzyme glyphosate oxidase the first decomposition step is initiated in living plants (Arregui et al., 2004). Thus, residues of both glyphosate and AMPA can be found in plant products, plant debris and soil. AMPA has a similar toxicity spectrum as glyphosate (Daouk et al., 2013; Gomes et al., 2016; Katholm, 2016; Kwiatkowska et al., 2014; Woźniak et al., 2018). Current soil residues of glyphosate and AMPA (with maxima ranging from 2.1 to 1.9 mg/kg in Europe to 39.1 and 14.6 mg/kg in Brazil), enhanced by applying contaminated manure, may delay germination, root growth and yield of subsequent crops (Bento et al., 2019; da Silva et al., 2021; Fernandes et al., 2020; Helander et al., 2019; Muola et al., 2021; Silva et al., 2018; Weng et al., 2019; Zhang et al., 2015).
In recent years, major concerns have arisen about harmful side effects of glyphosate and AMPA on plant, animal and human health due to the large-scale and intensive use of glyphosate and its accumulation in the environment and edible products worldwide (van Bruggen et al., 2018; van Bruggen et al., 2019). Based on several publications on potential chronic side effects of GBHs on human health the World Health Organization reclassified the herbicide glyphosate as probably carcinogenic to humans in 2015 (International Agenc, 2015). Nevertheless, the US Environmental Protection Agency (EPA) and the European Food Safety Authority (EFSA) judged that carcinogenic hazards to humans were unlikely at the recommended application rate and expected residue levels in most plant products, based partially on non-peer-reviewed reports (Benbrook, 2019; EFSA, 2015; EPA, 2017). Despite various potential negative side effects of GBHs for human beings and the environment (van Bruggen et al., 2018), the European Union authorized glyphosate use for five more years in 2017, but POEA-containing herbicides were prohibited (European Commission, 2017; Rueda-Ruzafa et al., 2019). Since that time, many additional scientific papers have been published on potential side effects of glyphosate and GBHs on plants and animals. Several of those papers have indicated that indirect effects on plant and animal health through changes in the associated microbiomes may be more important than direct effects on plant and animal physiology (Kiefer et al., 2021; Miyazaki et al., 2019; Motta et al., 2020). Since the review on glyphosate side effects by the first three authors (van Bruggen et al., 2018) many more papers have been published on effects of glyphosate on microbiomes and on plant and animal diseases, but not on both types of effects in plants and animals.
In this paper, we focus on indirect effects of sublethal concentrations of glyphosate and GBH on the health of terrestrial plants, animals and humans due to shifts in microbial community composition in various habitats, ranging from soil to plant and animal surfaces and animal and human intestinal tracts (Figure 2). The objectives are to present an overview of the scientific literature on 1) glyphosate accumulation in the environment, and in plant and animal products 2) its effects on microbial communities in soil, plants, animals and humans, and 3) potential effects of shifts in microbial community composition on plant, animal and human health.
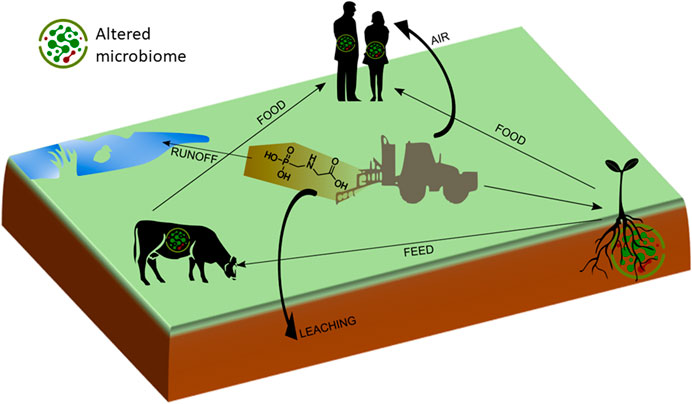
FIGURE 2. Depiction of glyphosate flows in the agricultural production chain. Microbiomes consist of a wide range of commensals, beneficials, and pathogens.
Glyphosate Fate in the Environment
Glyphosate in Soil and Water
Glyphosate applied on soil undergoes a decay in 2 phases. In the soil solute phase the initial decay is quite fast—showing a half-life of several days. In this phase AMPA, the main metabolite, is formed. Glyphosate and AMPA are then both adsorbed to clay and organic matter particles (Banks et al., 2014; Okada et al., 2016; Tang et al., 2019). Once adsorbed their degradation is very slow and both compounds are characterized by EFSA as persistent in soils (EFSA, 2015). The period required for 90 percent dissipation of glyphosate and AMPA (DT90) is estimated to be more than 1,000 days (EFSA, 2015), depending on the soil type, environmental conditions and prior exposure of soil microorganisms to the herbicide (Bento et al., 2016; Bento et al., 2019; Fei et al., 2013; Guijarro et al., 2018; Pérez Rodríguez et al., 2019; Weng et al., 2019; Zhao et al., 2015). Thus, glyphosate may decay partially in a few months, but its degradation product AMPA mostly persists for more than a year in soils with high clay content (Brock et al., 2019; Guijarro et al., 2018; Okada et al., 2016).
Despite its adsorption to clay and organic matter particles, parts of glyphosate and AMPA end up in the dissolved phase in ground water after heavy rain fall due to colloid transport processes in macropores (La Cecilia et al., 2018; Maqueda et al., 2017; Yang et al., 2015; Weng et al., 2019). Glyphosate residues have been documented up to 4.8 m depth in Germany (Tauchnitz et al., 2018). Soil particles with glyphosate and AMPA can also be transported by runoff or wind erosion to surface waters (Geng et al., 2021; Hofman, 2020; Silva et al., 2018; Yang et al., 2015), where they can remain in the particulate phase, be dissolved in water or adsorbed onto the bottom sediment (Maqueda et al., 2017). Glyphosate and AMPA are now widespread in soil and a variety of natural waters and sediments (Battaglin et al., 2014; Bento et al., 2019; Carles et al., 2019; Daouk et al., 2013; da Silva et al., 2021; Geng et al., 2021; Maqueda et al., 2017; Peruzzo et al., 2008; Poiger et al., 2017; Silva et al., 2018; Tauchnitz et al., 2020). It has also been detected in air and falling rain (Battaglin et al., 2014; Chang et al., 2011), irrigation water (Gomes et al., 2020), and in outlets from wastewater treatment plants (Poiger et al., 2017). The highest concentrations in surface waters occur in North and South America (Battaglin et al., 2014; Marques et al., 2021; van Bruggen et al., 2018), with values up to 700 μg/L in Argentina (Peruzzo et al., 2008). Although genetically modified crops are not grown in most European countries, glyphosate has been detected in various water sources there (van Bruggen et al., 2018). The glyphosate concentrations in ground- and surface waters were generally low (<2.5 μg/L) in several European and Asian countries, but higher in France (Geng et al., 2021; Poiger et al., 2017; van Bruggen et al., 2018). Glyphosate and AMPA are commonly detected in drinking-water (Mas et al., 2020; Parvez et al., 2018; O (World Health Organiz, 2005), but at concentrations below the acceptable daily intake (ADI) as determined in 1997 (O (World Health Organiz, 2005).
Glyphosate residues in Plant and Animal Products
Maximum residue limits (MRLs) in feed and food were established for glyphosate at the time of registration of the herbicide for use in agriculture in 1974 (CODEX Alimentarius, 2013). At that time, AMPA was not included in the MRLs. Since it was realized that glyphosate is partially broken down to AMPA in living plants (Arregui et al., 2004) and that AMPA is also toxic to various organisms (Gomes et al., 2016; Kwiatkowska et al., 2014; Woźniak et al., 2018), both glyphosate and AMPA have been included in residue analyses (Supplementary Table S1) and regulations by many agencies in recent years (CODEX Alimentarius, 2013; EPA, 2020; (European Commission), 2020; FAO, 2005). Under the auspices of the Food and Agriculture Organization of the United Nations (FAO), residues of glyphosate and AMPA in plant products were determined in standard experiments taking good agricultural practices (GAP) into account (FAO, 2005). These practices are focused on strict recommendations about GBH use (FAO, 2005). Updated MRL values were suggested from the highest residues obtained in trials that were carried out according to GAP requirements. When trial practices did not match GAP requirements the obtained results were omitted. No trials were conducted in Argentina where application rates and residues are exceptionally high (Cuhra, 2015).
The MRLs of glyphosate plus AMPA in farm products vary widely (Supplementary Table S1), depending on commodity and regulatory agency, ranging from 0.05 mg/kg for most animal products (except for meat byproducts), 0.1—40 mg/kg in many plant products for human consumption, and up to 530 mg/kg in grass and fodder (CODEX Alimentarius, 2013; Cuhra, 2015; (European Commission), 2020; EPA, 2020). The MRLs in animal feeds have been adjusted upwards over time when the original levels were exceeded too frequently and lower levels did not seem practical (Benbrook, 2016). The MRLs for most food and feed categories have been further increased (European Commission, 2020; EPA, 2020) since our previous glyphosate review published in 2018 (van Bruggen et al., 2018).
The observed concentrations of glyphosate plus AMPA in farm products vary widely too, ranging from 0.1–100 mg/kg in legumes (including soybeans), 0.1—85 mg/kg in grains and oil seeds, and 1—1,099 mg/kg in grassland and fodder (Supplementary Table S1). Extensive sampling of corn, soybeans, milk and eggs by the US Food and Drug Administration, using a specific analysis method, resulted in residues in a substantial percentage of the plant samples but not above the then current MRLs (FDA, 2017). However, very high concentrations of glyphosate were sometimes found in feed given to farm animals in Denmark that subsequently suffered from diseases such as infertility and malformation of pigs (Krüger et al., 2014b), botulism in cows (Gerlach et al., 2014; Krüger et al., 2013), and pathogenic Salmonella species in chickens (Krüger et al., 2013; Shehata et al., 2014).
Residues of glyphosate and AMPA in plant products and water are taken up by animals and humans and largely (60–70%) excreted in their feces and urine (Bus, 2015; Faniband et al., 2021; Heymann et al., 2021; Niemann et al., 2015; von Soosten et al., 2016; FAO and WHO, 2016). Glyphosate was detected in the urine of up to 96% of farm animals, with a maximum of 164 μg/L (Krüger et al., 2014a; Krüger et al., 2014b; Schrödl et al., 2014). Glyphosate and AMPA were also found frequently in the urine of dogs and cats, at relatively high concentrations (34–111 μg/L) (Karthikraj and Kannan, 2019). These concentrations are reflecting the high glyphosate residue levels in various pet food brands (Zhao et al., 2018).
The percentage of farmers with glyphosate in their urine was also high (up to 90%) with a maximum concentration of 233 μg/L in South Carolina (Acquavella et al., 2006). Glyphosate and AMPA were found in 60–95% of urine samples of the general public, including children, in the USA and in 40–50% of people in Europe (Gillezeau et al., 2019; Krüger et al., 2014a; Lemke et al., 2021; Niemann et al., 2015; Parvez et al., 2018; Soukup et al., 2020). The mean concentrations in human urine samples were low, 2–3 μg/L in the USA and <1 μg/L in Europe (Gillezeau et al., 2019; Lemke et al., 2021; Niemann et al., 2015; Parvez et al., 2018). In children, the highest average glyphosate concentration found was 4.04 μg/L with a peak of 18 μg/L in the USA (Gillezeau et al., 2020).
Recently, the herbicide was detected in urine samples of 30% of neonate babies (<30 days old; from Washington State) at very low concentrations (<1.06 ng/ml) in a small study (Ntotal = 108; Nneonates = 10) in New York State (Trasande et al., 2020). This finding suggests that glyphosate could have been transmitted through the placenta (Aris and Leblanc, 2011), Alternatively, it could have occurred in human breast milk or in baby formula possibly containing soy milk (Ehling and Reddy, 2015) or diluted with contaminated drinking water (O World Health Organiz, 2005). Glyphosate residues in breast milk have been reported unofficially (Honeycutt and Rowlands, 2014), but not in refereed journals (Bus, 2015; Ehling and Reddy, 2015; Steinborn et al., 2016). Glyphosate has rarely been detected in cow milk, including formula milk, but not above the MRL of 0.05 mg/L milk (Schnabel et al., 2017; van Eenennaam and Young, 2017; von Soosten et al., 2016).
Van Eenennaam and Young (2017) found little evidence of accumulation of glyphosate in animal tissues except in kidneys and liver. Indeed, kidneys are highly sensitive to glyphosate exposure (Gao et al., 2019). Summarizing WHO data, Bus (2015) indicated that most glyphosate administered to rats ended up in the bones, followed by the liver. Overall, up to 1.35% of glyphosate administered to rats was recovered in various tissues, amounting to 0.04 mg glyphosate per kg rat (Bus, 2015). Relatively high concentrations (5–20 mg/kg) of glyphosate were found in several other organs of malformed pigs, dairy cows and fattening rabbits (Krüger et al., 2014a; Krüger et al., 2014b). On average, 2 mg/kg were detected in the livers of malformed pigs in Denmark (Krüger et al., 2014b). However, 5–16 mg/kg were registered in the livers of experimental pigs that had received glyphosate amended feed (10–40 mg/kg, the maximum tolerable concentration) in China, while glyphosate was not found in the livers of the control group (Fu et al., 2020).
Glyphosate Effects on Microbial Communities in Soil, Plants, Animals and Humans
The shikimate pathway is present not only in plants but also in fungi, bacteria, archaea, and protozoa, rendering many taxa of microorganisms sensitive to glyphosate (Duke, 2018). The sensitivity of microorganisms having the shikimate pathway depends on the class of EPSPS they have. Traditionally, two classes were distinguished: the glyphosate sensitive class I EPSPS and the glyphosate tolerant class II EPSPS (Funke et al., 2007; Mesnage and Antoniou, 2020; Priestman et al., 2005). Recently, four groupings were distinguished based on variation in DNA sequences coding for the EPSPS enzyme (Leino et al., 2021; Rainio et al., 2021). The original two classes occurred most frequently. Classes III and IV were associated with glyphosate resistance, mainly in some bacterial and archaeal species (Leino et al., 2021).
Intensive and long-term glyphosate use has led to the selection of bacterial and fungal strains with low sensitivity to glyphosate through various resistance mechanisms, ranging from low permeability of the cell wall, active removal from the cell, to changes in the EPSPS binding site (Liu et al., 2013; Massot et al., 2019; Priestman et al., 2005; Rainio et al., 2021; Staub et al., 2012; van Bruggen et al., 2018). Some glyphosate-resistant E. coli and Pseudomonas strains contain a gene coding for an ABC transporter that enhances the efflux of glyphosate from the cell (Staub et al., 2012). Such resistance mechanisms may have led to the cross-resistance against antibiotics observed for E. coli, Salmonella sp. and other environmental bacteria (Kurenbach et al., 2015; Kurenbach et al., 2018; van Bruggen et al., 2018; Wicaksono et al., 2021). Other glyphosate resistance mechanisms have not been associated with antibiotic resistance (Pöppe et al., 2020).
In the past 50 years of glyphosate use, strains of various bacterial species have emerged that can break down the herbicide; these include potential animal/human pathogens (Acosta-Cortés et al., 2019; Fei et al., 2013; Funke et al., 2007; Grube et al., 2019; Liu et al., 2013; Pérez Rodríguez et al., 2019; Priestman et al., 2005; Xu et al., 2019). For instance, glyphosate can be detoxified through N-acetylation by several bacterial species, including Bacillus species like B. cereus and B. anthracis (Acosta-Cortés et al., 2019). Thus, long-term glyphosate use may lead to increased levels of B. anthracis (causal agent of anthrax) in the environment. Differences in sensitivity among microorganisms have affected the microbial composition of various habitats harboring glyphosate, including soil, plant surfaces and animal intestinal tracts (van Bruggen et al., 2018).
Effects on Microorganisms in Bulk Soil, Rhizosphere and Plants
Glyphosate is taken up by the foliage of plants and transported throughout the plant and into the rhizosphere and bulk soil (Walker and Oliver, 2008; Zobiole et al., 2010). Because many microorganisms are sensitive to glyphosate, its application likely affects the microbial composition and enzymatic activity in the rhizosphere and surrounding bulk soil (Arango et al., 2014; Banks et al., 2014; Schafer et al., 2014; Zobiole et al., 2010). There is still controversy about the ultimate effects of glyphosate on microbial communities in soil (Allegrini et al., 2015; Kepler et al., 2020; Mandl et al., 2018; Tang et al., 2019; Wolmarans and Swart, 2014). Most researchers have compared a single or double application of glyphosate or GBH with untreated control soil and evaluated short-term treatment effects on global microbial community composition or diversity (Arango et al., 2014; Banks et al., 2014; Tang et al., 2019; Vázquez et al., 2021; Wolmarans and Swart, 2014; Zhou et al., 2020). The communities seemed to recover from such short-term treatments (Arango et al., 2014). Even after long-term glyphosate use in no-till systems, only minor changes were detected in microbial communities of the wheat rhizosphere (Lupwayi et al., 2020), even though significant negative effects recently were observed for fungi (Vázquez et al., 2021). However, the methods used to study microbial communities in soil were mostly restricted to those measuring general microbial community structure, diversity, biomass or activity so that only minor or no effects were found (Allegrini et al., 2015; Banks et al., 2014; Bottrill et al., 2020; Lupwayi et al., 2020; Zabaloy et al., 2016), probably due to the great diversity and compensatory ability of microorganisms within such large groupings.
Deep sequencing of extracted DNA or RNA can detect rare microorganisms, shifts in microbial composition, and changes in metabolic functions resulting from glyphosate applications of glyphosate isopropylamine or potassium salt plus a formulation blank (Newman et al., 2016; Schafer et al., 2014; Schlatter et al., 2018). However, large sequence data sets are mostly analyzed at higher taxonomic units such as orders, classes or families of microorganisms (Kepler et al., 2020; Lupwayi et al., 2020; Mandl et al., 2018; Schlatter et al., 2018; Tang et al., 2019; Zhou et al., 2020), often masking differences in glyphosate sensitivity at lower taxonomic levels. The lack of observed glyphosate effects on higher taxonomic units was sometimes attributed to the overwhelming differences due to farming systems, seasons and locations (Kepler et al., 2020; Lupwayi et al., 2020; Schlatter et al., 2018). The limited discriminatory power of sequence analysis at higher taxonomic units was not discussed. Even when the relative frequencies of higher taxonomic units were not affected by glyphosate, interdependence bacterial networks showed distinct effects of glyphosate application on microbial communities in soil that had not been exposed to the herbicide previously, while bacterial networks did not change when glyphosate was applied to soils that had had prior glyphosate treatments for five or 10 years (Guijarro et al., 2018). Thus, the difficulties finding glyphosate-free control soil (Silva et al., 2018) may contribute to the difficulties detecting effects of glyphosate at higher integration levels.
When deep sequencing data were analyzed at lower taxonomic levels or for specific processes, glyphosate application did have significant effects on microbial composition and specific processes in soil. For example, in various no-till systems glyphosate application (formulation not mentioned) to kill cover crops resulted in changes in the nitrogen cycle compared to crop-killing by frost: nitrification and denitrification genes were reduced while the ammonia-oxidation genes were unaffected by glyphosate compared to frost (Romdhane et al., 2019). The changes in the nitrogen cycle were related to differences in bacterial composition at the genus level (Romdhane et al., 2019). Similarly, glyphosate application (Yates Zero® at the recommended rate) decreased the nitrification and denitrification rates in sugar cane soil (Zhang et al., 2018). In addition, new analysis techniques like artificial neural network and Random Forest analyses were able to distinguish microbial communities and identify specific bacterial genera associated with changes in the communities exposed to glyphosate (Janßen et al., 2019).
Changes in functional genes were also detected in the rhizosphere after glyphosate application at the recommended dose (3 L/ha) of a liquid glyphosate formulation onto EPSPS-transgenic soybean plants (Lu et al., 2018). Although microbial diversity in the rhizosphere of these plants was not affected, functional genes involved in plant growth promotion such as nitrogen fixation genes were affected negatively by glyphosate application (Figure 3).
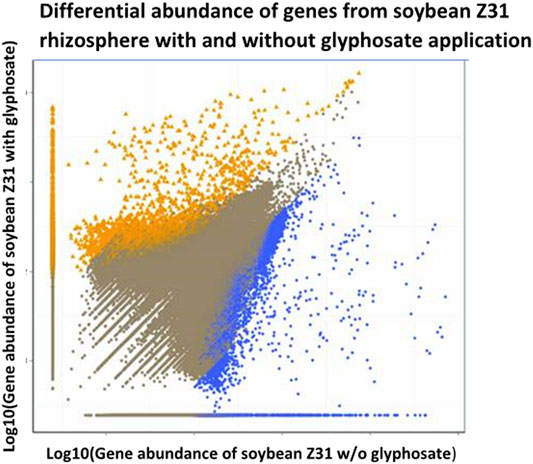
FIGURE 3. Effects of glyphosate on prokaryote communities on roots of EPSPS-transgenic soybeans cultivar Z31 sprayed with dilute glyphosate (y-axis) or water (x-axis), as determined by shotgun metagenome sequencing analysis of 16S rDNA, modified from Lu et al. (2018). Yellow and blue data points indicate genes with significantly higher and lower relative abundance, respectively, in Z31 plants sprayed with glyphosate than in those sprayed with water. Brown points represent genes without significant difference between the two treatments.
Microorganisms inside plants (the endosphere) originate partially from the microbiome in the rhizosphere or phyllosphere (Frank et al., 2017). Thus, effects of glyphosate on the rhizosphere microbiome, as described above, also impact the endosphere microbiome (Kuklinsky-Sobral et al., 2005). Very little is known about the effects of glyphosate on the composition of bacteria and fungi in the phyllosphere and endosphere (Lu et al., 2018), but distinctly different genera were detected in the endosphere of soybeans in glyphosate-treated compared to untreated soil (Kuklinsky-Sobral et al., 2005). Some species were resistant to glyphosate. For example, a glyphosate degrading bacterium, Achromobacter xylosoxidans was abundant in the endosphere of grapevine canes in vineyards that had been exposed frequently to glyphosate sprays (Deyett et al., 2017).
Effects on Microorganisms in Animals and Humans
Glyphosate-contaminated animal feed and water affects intestinal microbial communities, including archaea, bacteria, protozoa and fungi (van Bruggen et al., 2019). The effects depend on the form and concentration of glyphosate that is present in the different compartments of the intestinal tract. For calculation of possible concentrations encountered by the intestinal microbiome several assumptions need to be made, for example about potential concentrations in animal feed and reductions in the intestinal tract by degradation and absorption through intestinal walls (Katholm, 2016). The thus calculated concentrations were in the range of 2—20 mg L−1, although potentially higher concentrations were documented (Katholm, 2016; Krüger et al., 2014b). The minimum inhibitory concentrations (MIC) for bacteria are generally higher than the estimated concentrations in the intestinal tract but sensitive commensals like Bifidobacterium sp. or Bacterioides sp. can be exposed to concentrations that inhibit their growth (Nielsen et al., 2018; Shehata et al., 2013). The differences in sensitivity hold for both aerobic (Shehata et al., 2013) and anaerobic conditions (Nielsen et al., 2018), although the MIC values are generally higher under anaerobic conditions (Nielsen et al., 2018). Data on effects of actual glyphosate concentrations in animal feed and the intestinal tract on microbial communities and animal health are generally scarce (Katholm, 2016).
In the intestinal tract of mammals, the bacterial community is dominated by Firmicutes (mostly Gram-positive) and Bacteroidetes (mostly Gram-negative), as well as Actinobacteria and Proteobacteria phyla (Nielsen et al., 2018; Rueda-Ruzafa et al., 2019). Intestinal prokaryotes and eukaryotes vary considerably in sensitivity to glyphosate depending on the type of EPSPS they have. Based on knowledge of the DNA sequences coding for the different EPSPS types, it was estimated that 12–26% of bacteria in the human intestinal tract would be sensitive to glyphosate (Leino et al., 2021). Analysis of DNA sequences available from the Human Microbiome Project showed that almost all bacteria in the human intestinal tract possess the shikimate pathway (Mesnage and Antoniou, 2020). Contrary to the percentages glyphosate-sensitive bacteria estimated by Leino et al. (2021), Mesnage and Antoniou (2020) found that most of the gut bacteria in the Human Microbiome Project were sensitive to glyphosate. Comparison of paired metagenomes and metatranscriptomes obtained from the Inflammatory Bowel Disease Multi’omics Database indicated that there would be limited transcription of DNA coding for enzymes in the shikimate pathway, suggesting that biosynthesis of aromatic aminoacids via the shikimate pathway may not be essential for the gut microbiota residing in an amino acid-rich environment (Mesnage and Antoniou, 2020). However, the gut microbiomes of patients with irritable bowel syndrome are likely different from those of healthy people (Rueda-Ruzafa et al., 2019; Scotti et al., 2017). Thus, the potential effects of glyphosate residues on the in vivo gut microbiome still needs to be investigated in more detail.
In vitro, many potentially pathogenic bacteria are more tolerant to glyphosate and GBH than commensals (Bote et al., 2019a; Rueda-Ruzafa et al., 2019; van Bruggen et al., 2018). Technical grade glyphosate was used in few studies only; most researchers tested sensitivity to GBH (various Roundup or Glyfonova formulations) (Supplementary Table S2). The differences in sensitivity hold for both aerobic (Shehata et al., 2013) and anaerobic conditions (Nielsen et al., 2018). For instance, strains of Bifidobacterium sp. or Bacterioides sp were more sensitive to commercially formulated glyphosate than pathogenic Escherichia coli and Salmonella enterica under aerobic conditions (Shehata et al., 2013). The same beneficial bacteria were more sensitive to GBH than pathogenic Enterococcus faecalis and Salmonella enterica under anaerobic conditions (Supplementary Table S2) (Nielsen et al., 2018). The MIC values of Lactobacillus species varied considerably (Clair E. et al., 2012; Shehata et al., 2013; Nielsen et al., 2018), and those of Clostridium species were relatively high under aerobic (Shehata et al., 2013) but not under anaerobic conditions (Nielsen et al., 2018). Apparently, MIC values depend on the culturing and measuring methods used (Nielsen et al., 2018; Vicini et al., 2019).
Considering all papers with MIC values found for this review (Supplementary Table S2), more than ten-fold differences in MIC values were due to the method used to measure growth (with a turbidity meter versus visual observations), and much less by aerobic or anaerobic incubation conditions used (Table 1). Overall, pathogenic bacteria are less sensitive than non-pathogenic bacteria irrespective of the method used to assess growth. MIC values also depend on the year of isolation of the cultures studied relative to the time since glyphosate introduction into the environment (Bote et al., 2019a; Grube et al., 2019; Massot et al., 2019). Recent isolates are less sensitive to glyphosate and GBH than older isolates.

TABLE 1. Means and standard errors of minimal inhibitory concentrations (MIC, mg/ml) of glyphosate-based herbicides for N bacterial strains, as determined in 9 different studies. The means were calculated from the original published data (Supplementary Table S2). The test wells (micro titre plates) were incubated under aerobic or anaerobic conditions, and bacterial growth was assessed either by turbidometer or visually. Plates that were assessed with a turbidometer had not been incubated under anaerobic conditions.
In a study on dairy cows, lactic acid producing bacteria were more sensitive to glyphosate than toxin-producing Clostridium species (Krűger et al., 2013; Shehata et al., 2013). Also in mono-gastric animals, glyphosate ingestion led to serious Clostridium bacteremia (You et al., 2015). However, Riede et al., 2016 did not find effects of glyphosate on ruminal microbial communities in an in vitro study using single strand conformation polymorphism (SSCP) analysis on amplified extracted DNA. This is not surprising as Riede et al. (2016) looked for global metabolic changes and similarities of microbial communities at higher integration levels like in the studies of glyphosate effects on soil microbial communities (Allegrini et al., 2015; Kepler et al., 2020). Nevertheless, based on the study by Riede et al. (2016), EFSA decided that there was no significant effect of glyphosate on the ruminal microbial community ((European Food Safet, 2018). In a more recent in vitro bovine ruminal study, there were no effects of formulated glyphosate (10 mg L−1) on the decline curves of pathogenic isolates of E. coli and Salmonella Typhimurium (Bote et al., 2019b) as these genera are generally relatively resistant to glyphosate (Shehata et al., 2013; Pöppe et al., 2019).
Glyphosate in animal feed and water can affect intestinal fungi as well. A positive correlation was found between glyphosate concentrations in urine and the density of Mucorales in the rumen of dairy cows in Germany (Schrödl et al., 2014). Particularly, populations of Lichtheimia corymbifera and L. ramosa, members of the Mucorales, were significantly more abundant in animals with high glyphosate concentrations (>40 ng/ml) in their urine. These changes in the fungal community could have come about through a disturbance of the intestinal microbiota in general because members of the Mucorales were relatively resistant to glyphosate in vitro (Schrödl et al., 2014).
Experiments on effects of glyphosate on the gut microbiome of pigs were somewhat contradictory. No effects on bacterial families were detected by 16S rRNA sequencing after Roundup® LB plus (2.85 mg/kg body weight per day) had been added to bacterial cultivation fluid from pig colons in chemostats (Krause et al., 2020). However, small effects were observed at the functional level by metaproteomics (Krause et al., 2020). Potential effects on lower taxonomic units were not tested.
In mice, glyphosate-based herbicide (Roundup®) administered orally at high concentrations (250 or 500 mg a.i./kg bw/day for 6 or 12 weeks) reduced the abundance of Firmicutes, including Lactobacillus, and Bacteroidetes, but also potentially harmful Corynebacterium sp (Ait Bali et al., 2018). In a study with male rats that received 2.5 or 25 mg/kg bw/day orally for 2 weeks, the relative abundances of bacterial classes and phyla, estimated from 16rDNA sequencing, hardly changed (Nielsen et al., 2018). The absence of major effects was attributed to the availability of sufficient aromatic amino acids in the diet. Yet, at the genus level some significant treatment effects were obtained, for example increased Clostridium sensu stricto levels at 2.5 mg/kg bw/day (Nielsen et al., 2018). Conversely, Tang et al. (2020) demonstrated significant changes in composition and diversity of the gut microbiome of rats gavaged with diluted technical grade glyphosate for 35 days (for a total of 5–500 mg/kg body weight), using the same DNA sequencing techniques. The relative abundances of Firmicutes and Lactobacillus decreased, while potential bacterial pathogens increased. Firmicutes produce butyrate, which plays a role in maintaining the integrity of the intestinal wall and mucosal immunity (Canani et al., 2011; Fu et al., 2019).
When technical grade glyphosate or commercially available Roundup was administered in drinking water to maternal rats at a more realistic dose (at the US ADI of 1.75 mg/kg bw/day) for 6 or 13 weeks in the so-called Ramazzini study, significant changes were detected in bacterial composition (16S rRNA sequences) in the pups of the mother rats exposed to either treatment (Mao et al., 2018). Relative abundances of Bacteriodetes (especially the potential oral pathogen Prevotella) were increased while the Firmicutes (in particular the commensal Lactobacillus) were reduced by the glyphosate treatments. In a similar long-term study with female rats exposed to technical grade glyphosate or commercial Roundup MON 52276 (0.5, 50, or 175 mg glyphosate equivalent/kg BW/day in drinking water) the microbial metabolome in the cecum (upper part of the colon) was more affected by the formulated product than by glyphosate (Mesnage et al., 2021). No differences were found in alpha diversity and the most common bacteria in major taxonomic units, but four unrelated species (Acinetobacter, Akkermansia, Shinella, and the potential pathogen Eggerthella) were more abundant in both or either of the glyphosate treatments (Mesnage et al., 2021). In another long-term study with male rats exposed to pure glyphosate (1% aqueous solution) with or without two common food additives (1% solution each) in drinking water for 42 days, potentially pathogenic enterobacteria (Klebsiella, Citrobacter, and Enterobacter spp.), Pseudomonas sp. and Candida sp. were isolated more frequently from their feces than from control rats, while populations of commensal bacteria like Bifidobacterium and Lactobacillus were unaffected (Bilan et al., 2019).
In a long-term poultry study, Japanese quails were exposed to glyphosate-based herbicide (Roundup Flex®) at a common concentration in feed (160 mg/kg of glyphosate), and their fecal microbiomes were subjected to DNA extraction and sequencing (Ruuskanen et al., 2020a). The occurrence of Firmicutes, specifically Lactobacillus, decreased, especially in young female birds, while Actinobacteria increased during exposure to glyphosate (Ruuskanen et al., 2020a). In another poultry study, pathogenic bacteria like Salmonella and Clostridium spp. isolated from chickens were less sensitive to glyphosate in vitro (MIC = 1.2–5 mg/g) than the commensals Enterococcus and Bifidobacterium spp (MIC = 0.08–0.15 mg/g) (Shehata et al., 2013). Recently isolated Salmonella strains were more resistant to glyphosate than isolates collected between 1981 and 1990, suggesting adaptation and selection after repeated exposure to the herbicide (Pöppe et al., 2019). Similarly, there was a broad range of MIC values (1–80 mg/ml of the glyphosate salt) for isolates of E. coli from a variety of animals, including humans (Bote et al., 2019a). Recently isolated cultures of E. coli had higher MIC values than the standard historic isolates of E. coli. Pathogenic E. coli had slightly but significantly higher MIC values than commensal E. coli and isolates from poultry were more tolerant of glyphosate than isolates from pigs or cattle (Bote et al., 2019a).
Glyphosate can spread throughout the plant, including the flowers and pollen (Walker and Oliver, 2008). Thus, bees could take up glyphosate with the nectar exuded by flowers or on pollen (El Agrebi et al., 2020; Graystock et al., 2017). Pollen have their own characteristic microbiome (Dharampal et al., 2019; Frank et al., 2017) that could be altered by low concentrations of glyphosate. Part of the pollen microbiomes are transmitted to pollinators such as bees (Manirajan et al., 2018). Indeed, associations have been found between core microbes of domesticated and wild bees and pollen provisions (Graystock et al., 2017; Manirajan et al., 2018). Both glyphosate and affected bacterial communities associated with the pollen are transferred into the hive and change the microbiome of the brood as part of the microbial cycle (van Bruggen et al., 2019). When young bee workers were fed with a glucose solution or glucose with technical grate glyphosate solution (5 or 10 mg/L) and then returned to the hive, after 3 days the total intestinal bacterial populations (16 S rDNA copies) were reduced by the glyphosate treatment and the bacteria composition was altered (Motta et al., 2018). The core bee gut species, Snodgrassella alvi, with the sensitive class I EPSPS gene, was significantly reduced in the microbiome with potentially disastrous effects on survival in the presence of a bee pathogen (see paragraph on disease effects below). When honey bee larvae were reared in vitro with 0, 0.8, 4 or 20 mg technical grade glyphosate/L, changes in the midgut bacterial composition, species diversity and richness were detected at the highest concentration, while brood survival and larval weight were slightly negatively affected at 4 and 20 mg/L (Dai et al., 2018). Thus, glyphosate can have sublethal effects on the honeybee microbiota and possibly its health (see paragraph on disease effects below).
Potential Effects of Shifts in Microbial Community Composition on Plant, Animal and Human Health
In the course of the last decade, it has become increasingly clear that the health of individual macro-organisms and their populations is related to the diversity and relative stability of the microbial communities associated with these organisms (Lloyd-Price et al., 2016; Rosenberg and Zilber-Rosenberg, 2016; Scotti et al., 2017; van Bruggen et al., 2015; van Bruggen et al., 2019). As microorganisms are transferred among organisms, mostly through food webs, it has been suggested that the health conditions of all organisms in an ecosystem are interconnected through the cycling of subsets of microbial communities, primarily through food chains (van Bruggen et al., 2019). Thus, glyphosate can have a multitude of indirect effects on plant, animal and human health (van Bruggen et al., 2018).
Indirect effects of Glyphosate on Plant Health
Indirect effects of glyphosate and AMPA on plant health are possible through changes in the endophytic and rhizosphere microbiome (Berg et al., 2014; Kremer et al., 2005; Kuklinsky-Sobral et al., 2005). The importance of the plant microbiome for plant health and growth promotion has been known for a long time. Disruption of the microbiome in the rhizosphere, phyllosphere and endosphere of plants by glyphosate can result in reduced competition for attachment sites and reduced antimicrobial production against pathogens (van Bruggen et al., 2015). In addition, increased exudation of carbohydrates and amino acids from roots of glyphosate-exposed plants can attract plant pathogens more efficiently (Kremer et al., 2005; Kremer and Means, 2009), and reduced mechanical and chemical defenses resulting from disruptions in the aromatic amino acid production can facilitate entry of pathogens into plants (Duke, 2018; Fuchs et al., 2021; Hammerschmidt, 2018). Here, we focus on indirect health effects through changes in the plant microbiome.
Infection by Fusarium species was more severe in fields where glyphosate was applied at recommended rates (0.84–1.2 kg ae/ha) before planting of a soybean or wheat crop compared with untreated control fields (Johal and Huber, 2009; Kremer et al., 2005; Kremer and Means, 2009; Sanogo et al., 2000). Similarly, infection of sugar beet by weakly pathogenic Fusarium and Rhizoctonia species was enhanced after glyphosate application before planting sugar beet seeds (Larson et al., 2006). Soybean sudden death syndrome (caused by Fusarium virguliforme) was often increased by application of glyphosate-based herbicides in both glyphosate-tolerant and sensitive cultivars (Kremer and Means, 2009; Sanogo et al., 2000), but not always (Duke et al., 2012; Kandel et al., 2015). Hammerschmidt (2018) argued against increased disease severity by glyphosate applications on GR soybean plants and attributed increased disease risk to pathogen growth in dying and dead plant materials. More recently however, glyphosate (Roundup ControlMax® at 30, 100, and 300 mmol L−1 or 5.1, 16.9, and 50.7 mg ml−1 of glyphosate) also increased disease severity on seedlings of transgenic maize inoculated with Fusarium graminearum, F. verticillioides, and F. oxysporum under controlled conditions, when no plant debris was present (Figure 4) (Carranza et al., 2019).
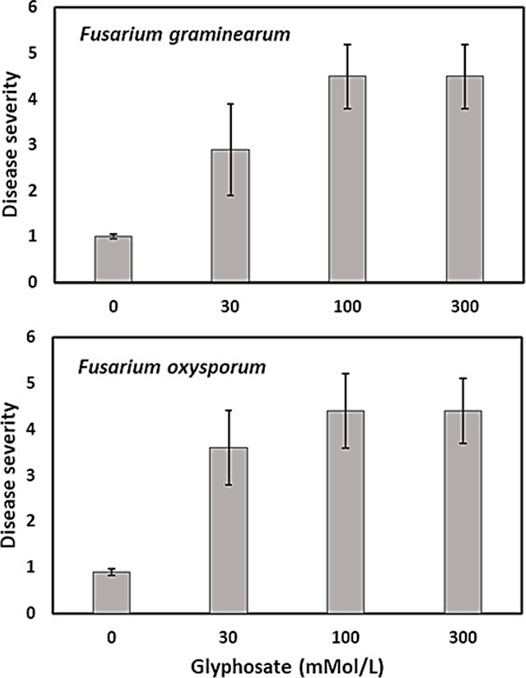
FIGURE 4. Effect of glyphosate (mmol/L) on Fusarium disease severity caused by F. verticillioides and F. oxysporum on glyphosate-resistant maize seedlings, modified from Carranza et al. (2019).
Despite the observed increases in root disease severity, spore germination and mycelium growth of these and other pathogens are mostly delayed and reduced by glyphosate in vitro (Figure 5) (Barnett et al., 2012; Benito et al., 2020; Carranza et al., 2019; Duke, 2018; Larson et al., 2006; Mengistu et al., 2013; Sanogo et al., 2000). This supports the notion that increased root disease in glyphosate treated soil comes about through reduced plant resistance or suppression of beneficial microorganisms rather than enhanced growth of plant pathogens like Fusarium and Rhizoctonia species (Duke, 2018). At the same time, mycotoxin production is sometimes enhanced at low (but realistic) glyphosate concentrations, for example aflatoxin production by Aspergillus flavus at 50 mM glyphosate formulated as Roundup-Controlmax® added to maize grains (Benito et al., 2020).
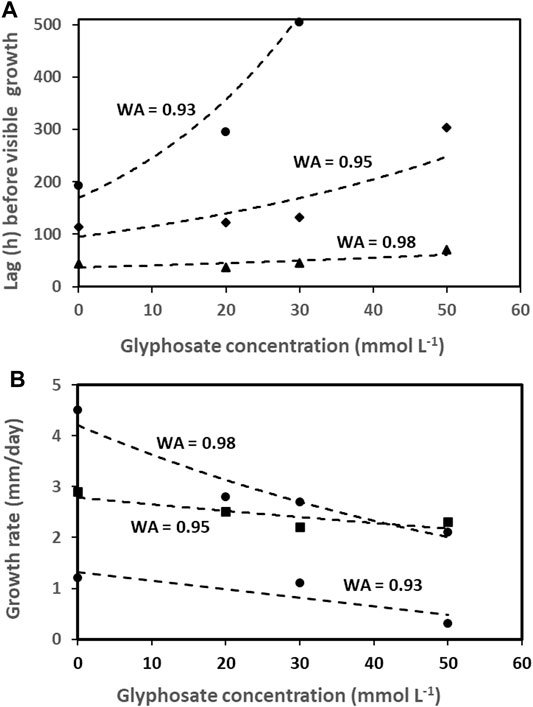
FIGURE 5. Effect of glyphosate (mmol/L) on the growth lag phase, h (A) and growth rate, mm/day (B) of Fusarium graminearum and F. oxysporum at three different water activities (WA) in vitro, prepared from data in Carranza et al. (2019).
Unlike soil-borne root pathogens, foliar plant pathogens may be less influenced by the surrounding microbial community. Very few reports describe effects of glyphosate on foliar pathogens. Goss’s bacterial wilt and leaf blight, a systemic maize disease caused by Clavibacter michiganensis ssp. Nebraskensis. became an increasingly severe problem since the introduction and expansion of GR maize (Langemeier, 2012). A significant, positive correlation was observed between glyphosate application and the detection of C. michiganensis ssp. nebraskensis in corn leaf samples (Langemeier, 2012). However, in field experiments with glyphosate-resistant and -sensitive maize with and without glyphosate application (Roundup at 1.68 kg ae/ha) and pathogen inoculation, no differences in disease severity were observed (Williams et al., 2015). Recently, only a weak correlation was detected between Goss’s wilt and leaf blight incidence and glyphosate application in farmers’ fields (Langemeier et al., 2017). Relations between Goss’s wilt and the endospheric microbial community were not investigated. Strawberry anthracnose, caused by the fungus Colletotrichum gloeosporioides, also became an increasingly severe problem after widespread use of glyphosate (Kao et al., 2019). Hyphal growth, conidial production and germination of C. gloeosporioides were promoted by glyphosate, and survival of this pathogen was enhanced in glyphosate treated soil. Anthracnose was more severe on strawberry leaves inoculated with the pathogen immediately after a foliar spray or soil drench with glyphosate (2.5 g/L, formulation not mentioned) than on control plants without the herbicide (Kao et al., 2019), suggesting a weakening of plant defenses. A reduction in phytoalexin production (enhancing plant defense) after glyphosate application had been shown convincingly previously (Johal and Rahe, 1990). This resulted in increased lesion expansion by Colletotrichum lindemuthianum on beans (Johal and Rahe, 1990). Conversely, alfalfa anthracnose by Colletotrichum trifolii, a hemibiotrophic pathogen, was reduced after glyphosate application on GR alfalfa plants (Samac and Foster-Hartnett, 2012). Similarly, the severity of several foliar rust diseases was reduced by glyphosate (Dos Santos et al., 2018; Duke, 2018; Hammerschmidt, 2018; Samac and Foster-Hartnett, 2012). This may be due to the damage to chlorophyll by glyphosate (Gomes et al., 2016; van Bruggen et al., 2018), resulting in a negative effect on biotrophic fungi like the rust fungi.
In their review of glyphosate effects on plant diseases and health, Martinez et al. (2018) considered health not only in terms of absence of disease, but also with respect to a balanced microbiome and availability and uptake of plant nutrients. Nutrient uptake can be impacted by glyphosate through its negative effects on microorganisms that make plant nutrients available (particularly N and P) as well as through its metal chelating properties, limiting the availability of nutrients such as copper, iron, manganese and zinc (Bott et al., 2011; Martinez et al., 2018). They attributed the documented increase in root diseases to disruptions to rhizosphere microbial ecology resulting in suppression of pathogen antagonists, nutritional stimulation of pathogen growth (from dying or dead plants and microorganisms, increased exudation, and the extra phosphorus contained in glyphosate), and reduced physiological plant defenses (Fuchs et al., 2021; Martinez et al., 2018). Although ultimate effects of glyphosate-based herbicides on plant disease development depend on environmental conditions and farming practices, Martinez et al. (2018) caution against over-reliance on these herbicides in agricultural production.
Indirect effects of Glyphosate on Animal and Human Health
Relationships between microbiomes and human or animal health have received much attention in recent years (Berg et al., 2014; Scotti et al., 2017). Despite the proliferation of literature in this area, many of the studies are primarily descriptive, with small sample sizes, and do not include deep sequencing data at the genus or species level; for these reasons, care needs to be taken in interpretation of these data. However, recent controlled studies with bees have addressed effects of glyphosate on microbiomes, immune indicators, infection by added pathogens, disease symptoms and survival in the same experiments (Castelli et al., 2021; Motta et al., 2020). This type of research has not been done with mammals as far as we know.
The initial animal microbiome is formed at birth, largely from the mother’s microbiome, but changes over time, as affected by microorganisms on food or feed, medicines, contaminants, and environmental factors (Lloyd-Price et al., 2016). The dynamic balance can be disturbed by extreme events, such as pesticide poisoning, and may or may not return to its original healthy state depending on the resilience of the original community (Lloyd-Price et al., 2016).
Intestinal microbiomes assist in the bioconversion of nutrients and detoxification, determine host immunity, protect against pathogenic microorganisms, and promote health (Raymann and Moran, 2018; Rinninella et al., 2019; Scotti et al., 2017). Changes in the intestinal composition can lead to dysbiosis, characterized by an imbalance between beneficial and pathogenic microorganisms (Rinninella et al., 2019; Rueda-Ruzafa et al., 2019; Scotti et al., 2017; Wicaksono et al., 2021). A balanced gut microbiome does not only affect the functioning and health of the gastrointestinal tract by defending against pathogen invasion, but also interacts with the endocrine and nervous systems affecting the functioning and health of the whole host system (Clair É. et al., 2012; Ingaramo et al., 2020; Maddalon et al., 2021; Rinninella et al., 2019; Scotti et al., 2017).
Intestinal microbial communities can be affected directly by glyphosate in contaminated animal feed and the environment. Indirectly, the intestinal microbiome is also changed by the microbial communities that enter the intestinal tract on/in glyphosate-exposed plant products (Lloyd-Price et al., 2016; van Bruggen et al., 2019). Subsequently, changes in these communities affect the immune system (Maddalon et al., 2021; Mendler et al., 2020; Peillex and Pelletier, 2020) and can be detrimental to animal and human health (Ait Bali et al., 2018; Tsiaoussis et al., 2019). For example, an increase in the ratio of Firmicutes to Bacteroidetes in the intestinal tract of humans has been associated with irritable bowel syndrome and obesity (Crovesy et al., 2020; Rueda-Ruzafa et al., 2019). Pathogens that are less sensitive or even insensitive to glyphosate, like Staphylococcus aureus or Clostridium perfringens, can emerge in the environment and cause serious disease symptoms (Funke et al., 2007; Priestman et al., 2005).
In the poultry studies mentioned above (Ruuskanen et al., 2020a; Ruuskanen et al., 2020b; Ruuskanen et al., 2020c; Shehata et al., 2013; Shehata et al., 2014), long-term exposure to glyphosate-based herbicide administered in the feed resulted in increased oxidative stress, lower testosterone levels and delayed development of female birds, which could have been affected by changes in the microbiome in the exposed birds (Ruuskanen et al., 2020c). Ultimate reproductive capacity was not affected in this bird study. However, chickens that were exposed to glyphosate in commercial feed (370 ± 92 μg/kg) showed typical disease symptoms associated with elevated intestinal Clostridium levels (Shehata et al., 2014). These symptoms were suppressed by humic acids that bind to glyphosate molecules in the intestinal tract (Shehata et al., 2014).
In pig studies, high concentrations of glyphosate (1.8 mg/ml) added to a liquid feed medium as Roundup® LB plus (equivalent to 2.85 mg glyphosate/kg body weight per day) were associated with only minor changes in bacterial families isolated from pig guts, but with significant changes in their metabolism (Krause et al., 2020). Yet, Roundup administered to pigs in their feed at moderate to high concentrations (10–40 mg glyphosate/kg body weight) resulted in increased permeability of the intestinal wall (Qiu et al., 2020), increased oxidative stress, liver damage and high glyphosate concentrations (6–16 mg/kg) in the liver (Fu et al., 2020). Serious stomach inflammation and enlarged uteri was observed more frequently in pigs that received a diet with glyphosate-resistant ingredients than a diet without genetically modified ingredients for 23 weeks (Carman et al., 2013). Also, high concentrations of glyphosate were found in various organs (up to 80 mg/kg) of piglets that showed major malformations (Krüger et al., 2013). Finally, sperm motility and viability were negatively affected by pure glyphosate at the highest concentration tested (360 mg/L), while Roundup was detrimental at much lower glyphosate concentrations (motility at ≥ 5 mg/L, mitochondrial activity at ≥25 mg/L, and viability at >100 mg/L (Nerozzi et al., 2020). Despite the intestinal and potential endocrine and reproduction problems (Jarrell et al., 2020), pig growth was not affected by glyphosate (Carman et al., 2013; Fu et al., 2020), and relationships of health problems to the pig microbiome are not clear.
In a feeding study with dairy cows, where glyphosate-contaminated feedstuffs (122.7 μg GL/kg BW for 16 weeks) were compared with control feed, no direct effects of glyphosate were detected on various blood and liver parameters (Heymann et al., 2021; Schnabel et al., 2020), as well as the general health condition of the cows (Schnabel et al., 2017). Nevertheless, long-term indirect health effects via changes in the microbiome have been documented (Gerlach et al., 2014; Krüger et al., 2013). Lactic acid producing bacteria generally were negatively affected by Roundup® (Clair E. et al., 2012; Krüger et al., 2013). These bacteria normally produce antibiotics and can suppress pathogenic bacteria such as Clostridium botulinum (Krüger et al., 2013; Rodloff and Krüger, 2012) and botulism has increasingly been found in cows that had high concentrations of glyphosate in their feed and urine (Gerlach et al., 2014; Krüger et al., 2013; Krüger et al., 2014a). During in vitro fermentation in bovine rumen fluid, several species of bacteria and protozoa were suppressed after exposure to glyphosate (Ackermann et al., 2015). Botulinum neurotoxin concentration was enhanced at the highest level of glyphosate (1,000 mg/L). High concentrations (>40 ng/ml) of glyphosate in the urine of dairy cows were also associated with relatively large, detrimental populations of Lichtheimia corymbifera and L. ramosa (Mucorales) and lower IgA antibodies against these fungi than in control cows (Schrödl et al., 2014). IgM antibodies and lipopolysaccharide-binding protein (LPB) were negatively correlated with glyphosate concentration in the urine, suggesting that glyphosate influenced the innate immune system of the cows, possibly through its toxic effects on the liver (Schrödl et al., 2014).
In the Ramazzini study with rats mentioned above (Mao et al., 2018), significant changes were found in the bacterial composition in feces of young pups from mothers that had been exposed to glyphosate or Roundup at the US Acceptable Daily Intake dose (1.75 mg/kg bw/day). Relative abundances of Bacteroidetes were increased while the Firmicutes were reduced by the glyphosate treatments (Mao et al., 2018). The glyphosate concentrations in the urine increased with age, suggesting accumulation, and were similar for pure glyphosate and Roundup (Panzacchi et al., 2018). There were significant endocrine effects in female rats, which had higher testosterone levels and delayed first estrous (Manservisi et al., 2019). In male rats, there were also significant endocrine effects of glyphosate exposure at low concentrations (1 mg/L), but in this case, testosterone levels were decreased by 35% (Clair É. et al., 2012). In a human birth cohort study in the midwestern USA, more than 90% of pregnant women had detectable glyphosate levels in their urine (0.5–7.20 ng/ml), which correlated significantly with shortened pregnancy lengths but not with fetal growth indicators (Parvez et al., 2018). Similarly, glyphosate and AMPA concentrations in the urine of pregnant women were significantly correlated with pre-term births in Puerto Rico (Silver et al., 2021). Associations between pregnancy outcomes and changes in the microbiome were not explored in these studies.
Potential relationships between glyphosate exposure and immune-endocrine changes have not been studied in detail for humans (Maddalon et al., 2021). In a systematic review of ten associative epidemiological studies in Latin America, no direct relationships were found between occupational exposure of women to glyphosate and birth defects, except for an excess of Attention Deficit Hyperactivity Disorder or ADHD among children born to glyphosate-applying parents (de Araujo et al., 2016). Similarly, the increased use of glyphosate over time was significantly correlated with the increased occurrence of various neurological disorders such as Alzheimer’s, senile dementia, Parkinson’s, ADHD and autism in the USA (Swanson et al., 2014). Rueda-Ruzafa et al. (2019) reviewed the literature on gut microbiota and neurological effects of glyphosate. They concluded that there was a possible link between glyphosate-induced dysbiosis and neurodegenerative and neurodevelopmental pathologies. Indeed, positive correlations were found between toxic metabolites of pathogenic microorganisms, specifically Clostridium spp. and symptoms of autism spectrum disorders (Argou-Cardozo and Zeidán-Chuliá, 2018; Roman et al., 2018). Metabolites from Clostridium spp. may result in an excess of dopamine quinones, generating reactive oxygen species, leading to oxidative stress and mitochondrial dysfunction (Rueda-Ruzafa et al., 2019; Shaw, 2017). Contrary to pathogenic microorganisms, beneficial bacteria such as Bifidobacterium sp., were decreased in the guts of autistic children compared to that of healthy children (De Angelis et al., 2013), and administration of probiotic bacteria may be beneficial (Rueda-Ruzafa et al., 2019). Similarly, administration of probiotics containing Lactobacillus sp. and Bifidobacterium sp. ameliorated depression and anxiety disorders (Messaoudi et al., 2011).
Neuro-pathological effects of glyphosate were also found in controlled experiments with rodents. Anxiogenic and depressive behavior was related to a reduction in Firmicutes, including Lactobacillus, in the intestinal tract of mice exposed orally to high concentrations of glyphosate-based herbicide (250–500 mg/kg/day (Ait Bali et al., 2018) Although the official “no observed adverse effect level” is 1,000 mg/kg/day (Ait Bali et al., 2018), Lactobacillus delbrueckii is clearly suppressed by 1,000 mg/kg for 28 h (Figure 6) (Clair E. et al., 2012).
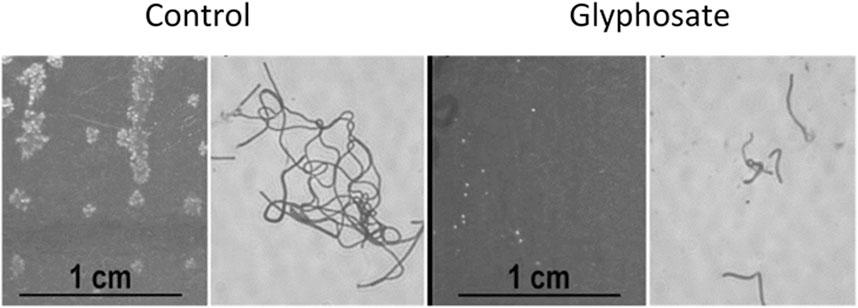
FIGURE 6. Effect of 1,000 mg/kg of glyphosate on macroscopic and microscopic growth of Lactobacillus delbrueckii subsp. bulgaricus in a solid growth medium for 48 h, modified from Clair E. et al. (2012).
Various neurochemical alterations and neurodevelopmental disorders were observed in pregnant rats treated with lower levels of glyphosate (50–70 mg/kg/day) and in their offspring (Cattani et al., 2017; Yu et al., 2018). Pregnant rats that received very low doses of glyphosate or Roundup (5 mg kg−1 d−1) reacted with a reduction in licking behavior towards their pups (Dechartres et al., 2019). Roundup exposure affected the maturation of doublecortin‐immunoreactive new neurons in the hippocampus of the mother (Dechartres et al., 2019). These neurological changes were associated with significant alterations of Bacteroidetes and Firmicutes in the mother’s gut. As with autism and depression mentioned above, administration of Lactobacillus and Bifidobacterium improved memory, learning behavior and oxidative stress in rats (Athari Nik Azm et al., 2018).
In ecological epidemiological studies the increased use of glyphosate has been followed by an increase in a wide variety of human diseases, including various forms of cancer, sometimes at a lag of several years (Swanson at al. 2014; Uyemura et al., 2017; Zhang et al., 2019). It should be emphasized that this does not imply a causal relationship (Mesnage and Antoniou, 2017). However, direct carcinogenic effects of chronic exposure to low doses of Roundup have been shown in experiments with laboratory rats (Mesnage et al., 2015b; Séralini et al., 2014). These results have been disputed, especially by researchers associated with the industry and regulatory agencies (Greim et al., 2015; Mesnage and Antoniou, 2017). The main argument was that no distinction was made between effects of the active ingredient and of the adjuvants (Davoren and Schiestl, 2018) and that the statistical analysis methods were deemed inappropriate (van Bruggen et al., 2018).
Nevertheless, a link has been suggested between pesticide use (with glyphosate as major component) and colon- and colorectal cancer (Uyemura et al., 2017). Intestinal inflammation and colorectal cancer are correlated with an overrepresentation of Proteobacteria including E. coli in the intestinal microbiome (Yang and Jobin, 2014). The risk of colon cancer is enhanced by strains of E. coli and Bacterioides fragilis that carry a gene coding for a cancer-promoting toxin (Hernández-Luna et al., 2019). E. coli strains that have the pathogenicity island pks (pks + E. coli), produce a toxin (colibactin) that induces distinct mutations in the host’s DNA in the colon epithelial cells. These mutations have been implicated in the development of cancer cells (Hernández-Luna et al., 2019; Pleguezuelos-Manzano et al., 2020). It is currently not known if colibactin-producing bacteria are more tolerant to glyphosate than regular commensals, but enhanced resistance to glyphosate of pathogenic E. coli (causing enteritis) compared to commensal E. coli has been documented (Bote et al., 2019a).
An alternative indirect effect of glyphosate on cancer development could be the inhibition of EPSPS of the gut microbiome resulting in accumulation of shikimate (Mesnage et al., 2021). Shikimate has been associated with the induction of various forms of cancer (Ma and Ning, 2019; Mesnage et al., 2021). Consequently, indirect carcinogenic effects of glyphosate through changes in the gut microbiome and/or its metabolism were suggested (Mesnage et al., 2021; Uyemura et al., 2017).
Concerns regarding the possible effect of glyphosate on microbiome and health have also been expressed for insects (Gómez-Gallego et al., 2020; Kiefer et al., 2021; Smith et al., 2021). For example, bee colony decline has been a well-known phenomenon in the past decade (Motta et al., 2018). The decline has been attributed to various factors, among others to bacterial and fungal pathogens. Honeybees (Apis mellifera) are dependent on bacterial symbionts such as Snodgrassella alvi, which has the class I ESPSP gene and is sensitive to glyphosate. After young honeybees were exposed to glyphosate at different doses (up to 169 mg L−1) affecting their microbiome as described above, challenge inoculation with the opportunistic pathogen Serratia marcescens resulted in up to 100% death, compared to 50% death by S. marcescens without glyphosate (Motta et al., 2018; Motta and Moran, 2020). The normal microbiome largely prevented infection by S. marcescens, while the microbiome altered by glyphosate could not prevent infection. Similar results were obtained in field experiments using 1 mM glyphosate or 0.1% Roundup in sucrose syrup combined with mark-and-recapture techniques (Motta et al., 2020). Fewer glyphosate-exposed bees were recaptured than control bees, and challenge inoculation with S. marcescens of the exposed bees resulted in increased death (Motta et al., 2020).
Glyphosate did not only enhance the susceptibility of bees to a bacterial pathogen, but also to a viral pathogen (Deformed Wing Virus) at 10 mg/L in sucrose syrup, despite an increase in immune activation indicators by glyphosate and the intestinal parasite Nosema ceranae (Castelli et al., 2021). The increased virus infection was associated with reduced bee survival (Castelli et al., 2021). When honeybees were artificially infected by N. ceranae, supplementation with Bifidobacteria and Lactobacilli in a sucrose solution partially controlled the infection (Baffoni et al., 2016). Although glyphosate changed the gut microbiome composition, sugar consumption, infection by N. ceranae and honeybee mortality were not affected in another study (Blot et al., 2019). Nevertheless, a recent meta-analysis of effects of different glyphosate formulations on bee mortality showed that glyphosate is indeed toxic to bees at realistic doses (Battisti et al., 2021). Additional effects of exposure of honeybees to glyphosate levels commonly found in agricultural settings are impaired cognitive abilities needed for a successful return to the hive. Thus, honeybees that had been fed with a solution containing 10 mg L−1 glyphosate (0.50 μg per individual bee) spent more time on homeward flights than control bees (Balbuena et al., 2015).
Solitary wild bees (such as Ceratina and Megalopta species) had a core microbiome that was partially shared with that of the pollen provision (Graystock et al., 2017). Lactobacillus was identified as core bacteria in all bee species and pollen sources investigated and was considered essential for a healthy microbiome (Graystock et al., 2017). Lactobacillus species are particularly sensitive to glyphosate (Baffoni et al., 2016), suggesting that sublethal glyphosate concentrations may have negative health effects on solitary bees too.
Discussion and Conclusion
Glyphosate use has increased worldwide more than ten-fold in the past 25 years (Székács and Darvas, 2018). As dissipation and degradation are much slower than anticipated originally, glyphosate residues have accumulated in soil and water bodies, and consequently have increased in plant and animal products (Battaglin, 2014; Carles, 2019; Silva et al., 2018). Tolerance levels were set originally based on acute oral toxicities and have been raised as the measured residues in food and feed products rose over time (Benbrook, 2016; Myers et al., 2016; Zhang et al., 2019). Although the toxicity to mammals was initially considered to be low (Greim et al., 2015; O World Health Organiz, 2009), there is increasing emphasis on potential chronic effects of glyphosate as it accumulates in the environment and the food chain (Jarrell et al., 2020; Greim et al., 2015; Mesnage et al., 2015a; Mesnage et al., 2015b; Myers et al., 2016; Zhang et al., 2019).
Correlations have been found between the increase in glyphosate use and several plant, animal and human diseases (Rueda-Ruzafa et al., 2019; Sanogo et al., 2000; Swanson et al., 2014; van Bruggen et al., 2018; Uyemura et al., 2017; Zhang et al., 2019). In 2015, the World Health Organization warned about the potential negative health effects of glyphosate, including the risk of cancer development based on experimental research results, and reclassified glyphosate as probably carcinogenic to humans ((International Agenc, 2015). Subsequently, several governments have partially restricted the use of glyphosate (https://www.baumhedlundlaw.com/toxic-tort-law/monsanto-roundup-lawsuit/where-is-glyphosate-banned/). Conversely, the Environmental Protection Agency (EPA) of the USA and the European Commission (EC), based on recommendations by EFSA and the German government, reviewed selected papers on the carcinogenic potential of glyphosate and decided to reregister the herbicide without additional restrictions (EPA, 2017; EC, 2017). Glyphosate and AMPA were not expected to have an impact on the health of various agricultural animals (and rumen microbial communities) at a maximal residue level of 292 mg kg-1 dry weight in fodder and 342–530 mg kg-1 in grass forage ((European Food Safet, 2018). These residue levels are approached frequently but rarely exceeded (Supplementary Table S1).
Despite the reregistration by international regulatory agencies, the potential negative side effects of glyphosate have remained controversial. The debate focused initially on toxic effects of additives in the formulations (Benbrook, 2016; Defarge et al., 2018; Hammerschmidt, 2018) and formulations with POEA were banned in some countries (EC, 2016; EC, 2017). Nevertheless, negative side effects continue to be reported by independent scientists, also for other formulations (Myers et al., 2016), and some countries are considering a complete ban of glyphosate (Peng et al., 2020).
In recent years, the emphasis in the debate has shifted to indirect side effects on plant, animal and human health via effects of glyphosate on the microbiomes associated with their hosts (Davoren and Schiestl, 2018). Direct effects of glyphosate on soil microbiota and plant- and animal microbiomes have been disputed by some researchers associated with government agencies (Duke, 2018; European Food Safet, 2018; EPA, 2017; Kepler et al., 2020; Schlatter et al., 2018) and private companies (Bus, 2015; Vicini et al., 2019). We noticed, however, that the detection of glyphosate effects on microbial community composition depends on the level of integration chosen for the analysis of microbiome DNA sequences: treatment effects on Phyla, Orders or Classes were generally not found. These levels of integration are not always relevant for finding shifts in microbiomes affecting health, because selection for resistance and pathogenicity commonly occurs at lower taxonomic levels (Bote et al., 2019a). The complex effects of glyphosate on microbiomes associated with the main macro-organism hosts discussed in this review are summarized in Supplementary Figure S1.
Glyphosate effects on microbial composition have been documented convincingly at the genus and species levels (Helander et al., 2018; Nielsen et al., 2018; Romdhane et al., 2019). For example, on Bacillus species, including B. anthracis (Acosta-Cortés et al., 2019; Yu et al., 2015) and Clostridium species, including C. botulinum (Argou-Cardozo and Zeidán-Chuliá, 2018; Gerlach et al., 2014; Krüger et al., 2013; Rodloff and Krüger, 2012) are often less sensitive or insensitive to glyphosate and may accumulate in the environment. Both genera produce survival spores under stress and can survive food and feed processing like pasteurization and fermentation (Driehuis et al., 2018). Additional research is needed to determine if glyphosate application to crops could lead to increased disease risk by these animal pathogens from contaminated and processed food or feed. In addition to effects of glyphosate on the microbiome at genus and species levels, subtle effects at the sub-species level may also be important (Bote et al., 2019a). It remains to be seen if pks + E. coli strains that enhance the risk of colon cancer are more tolerant to glyphosate than commensal E. coli strains.
The reasons for greater glyphosate resistance among animal pathogens such as Bacillus, Clostridium, Streptococcus, and Staphylococcus compared to non-pathogens such as Bacterioides and Bifidobacterium are not known. Cell wall thickness may be one reason (Ravishankar et al., 2020) as these animal pathogens are Firmicutes (Gram-positive) and thus have thicker cell walls. Lactobacillus is a Gram-positive commensal, and its MIC value is variable (Clair E. et al., 2012; Shehata et al., 2013; Nielsen et al., 2018). However, the main reasons for differential sensitivity to glyphosate likely are differences in EPSPS types (Leino et al., 2021; Rainio et al., 2021). Firmicutes have a resistant EPSPS type (Rainio et al., 2021). Nevertheless, also at the molecular interaction level there are exceptions, with Proteobacteria (Gram-negative) being either sensitive or resistant, the pathogens Legionella and Campylobacter being resistant and Yersinia and Neisseria sensitive (Rainio et al., 2021). This difference in sensitivity may be related to the extent of exposure to glyphosate in the environment (Rainio et al., 2021).
Deep-sequencing data at genus and species levels of the microbiomes of rhizosphere soil, plants and animals are still rare. However, significant effects of glyphosate on microbiomes have been demonstrated by deep sequencing recently (Lu et al., 2018; Mao et al., 2018; Motta et al., 2018; Romdhane et al., 2019). Again, the probability of demonstrating glyphosate effects depends on the depth of the analysis in terms of taxonomic and functional integration levels. Future improvements in deep sequencing and analysis techniques may be helpful in this respect.
Detecting glyphosate effects on microbiomes and their macro-organism hosts is also hampered because of increasing difficulties in finding:
1) Truly negative controls due to increasing residues in soil, water, food and feed (Séralini, 2020; Silva et al., 2018; de Araujo et al., 2016),
2) Differences in sensitivity of microorganisms as more and more species are becoming resistant to glyphosate (Grube et al., 2019; Pöppe et al., 2019), and
3) Actual residue levels above the maximum residue limits if the MRLs are raised over time (Benbrook, 2016; (European Commission), 2020).
Thus, documentation of negative side effects of glyphosate will become more and more difficult (Séralini, 2020). This does not mean that there are no negative side effects now compared to truly negative controls available 25 years ago.
In conclusion, we suggest that assessment and characterization of problems associated with the large scale and intensive use of glyphosate and other pesticides is much more daunting than originally anticipated by regulatory agencies, in particular due to the accumulation in soil and waterways (Geissen et al., 2021). Residual concentrations in manure and soil are getting so high that plant growth is affected negatively, and prospects for a circular agriculture are endangered (Fernandes et al., 2020; Gomes et al., 2020; Helander et al., 2018; Helander et al., 2019; Muola et al., 2021).
Glyphosate residues are increasing throughout the agri-foodsystem (Bøhn and Millstone, 2019; Benbrook, 2016). Taking chronic indirect effects of glyphosate into account, we agree with Myers et al. (2016) and Li and Jennings (2017), who recommend that regulatory agencies should reconsider legally binding tolerance levels of glyphosate plus AMPA in plant products and drinking water for animal and human consumption. Residues of glyphosate and AMPA are common in most plant products, especially from second generation GR crops with a gene encoding for the enzyme glyphosate oxidase that converts glyphosate into AMPA, so that animal and human diets contain residues of both compounds from many different sources. The decision about maximum residue levels should be risk-based (the combination of hazard and exposure), taking all possible exposure routes into account, including direct exposure through the skin, nasal exposure from aerosols, oral exposure with the consumption of plant- and animal products, and drinking water (Székács and Darvas, 2018). Maximal residues as observed in farm products (not only in experimental settings) should be used in the calculations (Bøhn and Millstone, 2019; Cuhra, 2015; Miyazaki et al., 2019), and exposures should be calculated over a lifetime, in recognition of the risk of chronic long-term effects (Greim et al., 2015; Mesnage et al., 2015b; Séralini et al., 2014). Hazard calculations need to include not only direct oral toxicity of the active ingredient resulting in liver and kidney disease and death (Gao et al., 2019; Trasande et al., 2020), but also long-term, sub-lethal exposures resulting in microbial dysbiosis, chronic kidney problems, carcinogenicity, reproductive and developmental toxicity, neurotoxicity and endocrine effects of the various formulations (Defarge et al., 2018; Ingaramo et al., 2020; Jarrell et al., 2020; Maddalon et al., 2021; Mesnage et al., 2015a; Székács and Darvas, 2018). In view of our recent understanding of the importance of the microbiome, not only for intestinal health but through the gut-brain axis also for immune, endocrine, neurodevelopmental and whole organism health, effects of glyphosate on pathogenic and beneficial bacterial and fungal species need to be taken into account (Davoren and Schiestl, 2018; Miyazaki et al., 2019).
We recommend additional interdisciplinary research on the associations between low level chronic glyphosate exposure, distortions in microbial communities at the species level and the emergence of animal, human and plant diseases. A potential connection between glyphosate exposure, populations of pks + bacterial species such as E. coli and intestinal cancer development needs to be investigated (Davoren and Schiestle, 2018). Connections between glyphosate resistance in bacteria and antibiotic resistance also deserve more attention (Kurenbach et al., 2015; Kurenbach et al., 2018; Pöppe et al., 2020). As suggested by us earlier (van Bruggen et al., 2018), independent and trustworthy research is needed to revisit the tolerance thresholds for glyphosate residues in water, food and animal feed taking all possible health risks into account.
Author Contributions
AvB developed the ideas, collected literature, and wrote the manuscript. MF contributed ideas and suggestions for manuscript improvements, MH contributed references, CR made suggestions and edited the manuscript, PH prepared the glyphosate flow chart and made suggestions for the text, DK prepared Supplementary Table S1, and VG corrected the text on environmental fate of glyphosate and contributed ideas and suggestions for the manuscript as a whole.
Funding
Publication fees were paid by the SLM group of Wageningen University. We are grateful for collaboration and financial support of the SPRINT project funded by the European Commission through Horizon 2020, the EU research and innovation programme (grant agreement no. 862568).
Conflict of Interest
The authors declare that the research was conducted in the absence of any commercial or financial relationships that could be construed as a potential conflict of interest.
Publisher’s Note
All claims expressed in this article are solely those of the authors and do not necessarily represent those of their affiliated organizations, or those of the publisher, the editors and the reviewers. Any product that may be evaluated in this article, or claim that may be made by its manufacturer, is not guaranteed or endorsed by the publisher.
Acknowledgments
We are grateful to Prof. J.G. Morris, Jr, for reviewing an earlier version of this review. We also thank the reviewers of this paper.
Supplementary Material
The Supplementary Material for this article can be found online at: https://www.frontiersin.org/articles/10.3389/fenvs.2021.763917/full#supplementary-material
References
Ackermann, W., Coenen, M., Schrödl, W., Shehata, A. A., and Krüger, M. (2015). The Influence of Glyphosate on the Microbiota and Production of Botulinum Neurotoxin during Ruminal Fermentation. Curr. Microbiol. 70, 374–382. doi:10.1007/s00284-014-0732-3
Acosta-Cortés, A. G., Martinez-Ledezma, C., López-Chuken, U. J., Kaushik, G., Nimesh, S., and Villarreal-Chiu, J. F. (2019). Polyphosphate recovery by a native Bacillus cereus strain as a direct effect of glyphosate uptake. ISME J. 13, 1497–1505. doi:10.1038/s41396-019-0366-3
Acquavella, J. F., Alexander, B. H., Mandel, J. S., Burns, C. J., and Gustin, C. (2006). Exposure Misclassification in Studies of Agricultural Pesticides. Epidemiology 17 (1), 69–74. doi:10.1097/01.ede.0000190603.52867.22
Aitbali, Y., Ba-M'hamed, S., Elhidar, N., Nafis, A., Soraa, N., and Bennis, M. (2018). Glyphosate based- herbicide exposure affects gut microbiota, anxiety and depression-like behaviors in mice. Neurotoxicology and Teratology 67, 44–49. doi:10.1016/j.ntt.2018.04.002
Allegrini, M., Zabaloy, M. C., and Gómezdel, E. d. V. V. (2015). Ecotoxicological assessment of soil microbial community tolerance to glyphosate. Sci. Total Environ. 533, 60–68. doi:10.1016/j.scitotenv.2015.06.096
Arango, L., Buddrus-Schiemann, K., Opelt, K., Lueders, T., Haesler, F., Schmid, M., et al. (2014). Effects of glyphosate on the bacterial community associated with roots of transgenic Roundup Ready soybean. Eur. J. Soil Biol. 63, 41–48. doi:10.1016/j.ejsobi.2014.05.005
Argou-Cardozo, I., and Zeidán-Chuliá, F. (2018). Clostridium bacteria and autism spectrum conditions: a systematic review and hypothetical contribution of environmental glyphosate levels. Med. Sci. 6, 29. doi:10.3390/medsci6020029
Aris, A., and Leblanc, S. (2011). Maternal and fetal exposure to pesticides associated to genetically modified foods in Eastern Townships of Quebec, Canada. Reprod. Toxicol. 31 (4), 528–533. doi:10.1016/j.reprotox.2011.02.004
Arregui, M. C., Lenardón, A., Sanchez, D., Maitre, M. I., Scotta, R., and Enrique, S. (2004). Monitoring glyphosate residues in transgenic glyphosate-resistant soybean. Pest Manag. Sci. 60, 163–166. doi:10.1002/ps.775
Athari Nik Azm, S., Djazayeri, A., Safa, M., Azami, K., Ahmadvand, B., Sabbaghziarani, F., et al. (2018). Lactobacilli and bifidobacteria ameliorate memory and learning deficits and oxidative stress in β-amyloid (1-42) injected rats. Appl. Physiol. Nutr. Metab 43, 718–726.
Baffoni, L., Gaggìa, F., Alberoni, D., Cabbri, R., Nanetti, A., Biavati, B., et al. (2016). Effect of dietary supplementation of Bifidobacterium and Lactobacillus strains in Apis mellifera L. against Nosema Ceranae. Beneficial Microbes 7, 45–51. doi:10.3920/bm2015.0085
Balbuena, M. S., Tison, L., Hahn, M.-L., Greggers, U., Menzel, R., and Farina, W. M. (2015). Effects of sublethal doses of glyphosate on honeybee navigation. J. Exp. Biol. 218, 2799–2805. doi:10.1242/jeb.117291
Banks, M. L., Kennedy, A. C., Kremer, R. J., and Eivazi, F. (2014). Soil microbial community response to surfactants and herbicides in two soils. Appl. Soil Ecol. 74, 12–20.
Barnett, K. A., Sprague, C. L., Kirk, W. W., and Hanson, L. E. (2012). Influence of glyphosate on Rhizoctonia crown and root rot (Rhizoctonia solani) in glyphosate-resistant sugarbeet. Weed Sci. 60, 113–120. doi:10.1614/ws-d-11-00027.1
Battaglin, W. A., Meyer, M. T., Kuivila, K. M., and Dietze, J. E. (2014). Glyphosate and its degradation product AMPA occur frequently and widely in U.S. soils, surface water, groundwater, and precipitation. J. Am. Water Resour. Assoc. 50, 275–290. doi:10.1111/jawr.12159
Battisti, L., Potrich, M., Sampaio, A. R., de Castilhos Ghisi, N., Costa-Maia, F. M., Abati, R., et al. (2021). Is glyphosate toxic to bees? A meta-analytical review. Sci. Total Environ. 767, 145397. doi:10.1016/j.scitotenv.2021.145397
Benbrook, C. M. (2019). How did the US EPA and IARC reach diametrically opposed conclusions on the genotoxicity of glyphosate-based herbicides? Environ. Sci. Eur. 31, 2. doi:10.1186/s12302-018-0184-7
Benbrook, C. M. (2016). Trends in glyphosate herbicide use in the United States and globally. Environ. Sci. Eur. 28, 3. doi:10.1186/s12302-016-0070-0
Benito, N., Magnoli, K., Carranza, C. S., Aluffi, M. E., Magnoli, C. E., and Barberis, C. L. (2020). Influence of a glyphosate-based herbicide on growth parameters and aflatoxin B1 production by Aspergillus section Flavi on maize grains. Rev. Argent. Microbiol., S0325–S7541. doi:10.1016/j.ram.2020.09.004
Bento, C. P. M., van der Hoeven, S., Yang, X., Riksen, M. M. J. P. M., Mol, H. G. J., Ritsema, C. J., et al. (2019). Dynamics of glyphosate and AMPA in the soil surface layer of glyphosate-resistant crop cultivations in the loess Pampas of Argentina. Environ. Pollut. 244, 323–331. doi:10.1016/j.envpol.2018.10.046
Bento, C. P. M., Yang, X., Gort, G., Xue, S., van Dam, R., Zomer, P., et al. (2016). Persistence of glyphosate and aminomethylphosphonic acid in loess soil under different combinations of temperature, soil moisture and light/darkness. Sci. Total Environ. 572, 301–311. doi:10.1016/j.scitotenv.2016.07.215
Berg, G., Gruber, M., Schloter, M., and Smalla, K. (2014). The plant microbiome and its importance for plant and human health. Front. Microbiol. 5, 491. doi:10.3389/fmicb.2014.00491
Bilan, M. V., Lieshchova, M. A., Tishkina, N. M., and Brygadyrenko, V. V. (2019). Combined effect of glyphosate, saccharin and sodium benzoate on the gut microbiota of rats. Regul. Mech. Biosyst. 10 (2), 228–232. doi:10.15421/021934
Blot, N., Veillat, L., Rouzé, R., and Delatte, H. (2019). Glyphosate, but not its metabolite AMPA, alters the honeybee gut microbiota, PLoS One, 14. e0215466. doi:10.1371/journal.pone.0215466
Bøhn, T., and Millstone, E. (2019). The Introduction of Thousands of Tonnes of Glyphosate in the food Chain-An Evaluation of Glyphosate Tolerant Soybeans. Foods 8, 669. doi:10.3390/foods8120669
Bote, K., Pöppe, J., Merle, R., Makarova, O., and Roesler, U. (2019a). Minimum Inhibitory Concentration of Glyphosate and of a Glyphosate-Containing Herbicide Formulation for Escherichia coli Isolates - Differences Between Pathogenicand Non-pathogenic Isolates and Between Host Species. Front. Microbiol. 10, 932. doi:10.3389/fmicb.2019.00932
Bote, K., Pöppe, J., Riede, S., Breves, G., and Roesler, U. (2019b). Effect of a glyphosate-containing herbicide on Escherichia coli and Salmonella ser. Typhimurium in an in vitro rumen simulation system. Eur. J. Microbiol. Immunol. 9 (3), 94–99. doi:10.1556/1886.2019.00010
Bott, S., Tesfamariam, T., Kania, A., Eman, B., Aslan, N., Römheld, V., et al. (2011). Phytotoxicity of glyphosate soil residues re-mobilised by phosphate fertilisation. Plant Soil 342 (1), 249–263. doi:10.1007/s11104-010-0689-3
Bottrill, D., Ogbourne, S. M., Citerne, N., Smith, T., Farrar, M. B., Hu, H.-W., et al. (2020). Short-term application of mulch, roundup and organic herbicides did not affect soil microbial biomass or bacterial and fungal diversity. Chemosphere 244, 125436. doi:10.1016/j.chemosphere.2019.125436
Brock, A. L., Rein, A., Polesel, F., Nowak, K. M., Kästner, M., and Trapp, S. (2019). Microbial turnover of glyphosate to biomass: utilization as nutrient source and formation of AMPA and biogenic NER in an OECD 308 test. Environ. Sci. Technol. 53, 5838–5847. doi:10.1021/acs.est.9b01259
Bus, J. S. (2015). Analysis of Moms across America report suggesting bioaccumulation of glyphosate in U.S. mother's breast milk: Implausibility based on inconsistency with available body of glyphosate animal toxicokinetic, human biomonitoring, and physico-chemical data. Regul. Toxicol. Pharmacol. 73 (3), 758–764. doi:10.1016/j.yrtph.2015.10.022
Canani, R. B., Costanzo, M. D., Leone, L., Pedata, M., Meli, R., and Calignano, A. (2011). Potential beneficial effects of butyrate in intestinal and extraintestinal diseases. Wjg 17, 1519–1528. doi:10.3748/wjg.v17.i12.1519
Carles, L., Gardon, H., Joseph, L., Sanchís, J., Farré, M., and Artigas, J. (2019). Meta-analysis of glyphosate contamination in surface waters and dissipation by biofilms. Environ. Int. 124, 284–293. doi:10.1016/j.envint.2018.12.064
Carman, J. A., Vlieger, H. R., Ver Steeg, L. J., Sneller, V. E., Robinson, G. W., Clinch-Jones, C. A., et al. (2013). A long-term toxicology study on pigs fed a combined genetically modified (GM) soy and GM maize diet. J. Org. Syst. 8 (1), 38–54.
Carranza, C. S., Aluffi, M. E., Benito, N., Magnoli, K., Barberis, C. L., and Magnoli, C. E. (2019). Effect of in vitro glyphosate on Fusarium spp. growth and disease severity in maize. J. Sci. Food Agric. 99, 5064–5072. doi:10.1002/jsfa.9749
Castelli, L., Balbuena, S., Branchiccela, B., Zunino, P., Liberti, J., Engel, P., et al. (2021). Impact of Chronic Exposure to Sublethal Doses of Glyphosate on Honey Bee Immunity, Gut Microbiota and Infection by Pathogens. Microorganisms 9, 845. doi:10.3390/microorganisms9040845
Cattani, D., Cesconetto, P. A., Tavares, M. K., Parisotto, E. B., De Oliveira, P. A., Rieg, C. E. H., et al. (2017). Developmental exposure to glyphosate-based herbicide and depressive-like behavior in adult offspring: implication of glutamate excitotoxicity and oxidative stress. Toxicology 387, 67–80. doi:10.1016/j.tox.2017.06.001
Chang, F.-c., Simcik, M. F., and Capel, P. D. (2011). Occurrence and fate of the herbicide glyphosate and its degradate aminomethylphosphonic acid in the atmosphere. Environ. Toxicol. Chem. 30, 548–555. doi:10.1002/etc.431
Clair, E., Linn, L., Travert, C., Amiel, C., Séralini, G.-E., and Panoff, J.-M. (2012b). Effects of Roundup and Glyphosate on Three Food Microorganisms: Geotrichum candidum, Lactococcus lactis subsp. cremoris and Lactobacillus delbrueckii subsp. bulgaricus. Curr. Microbiol. 64, 486–491. doi:10.1007/s00284-012-0098-3
Clair, É., Mesnage, R., Travert, C., and Séralini, G.-É. (2012a). A glyphosate-based herbicide induces necrosis and apoptosis in mature rat testicular cells in vitro, and testosterone decrease at lower levelsA glyphosate-based herbicide induces necrosis and apoptosis in mature rat testicular cells in vitro, and testosterone decrease at lower levels. Toxicol, In. Toxicol. Vitro 26 (2), 269–279. doi:10.1016/j.tiv.2011.12.009
CODEX Alimentarius (2013). Pesticide residues in food and feed: 158 Glyphosate. Food Agric. Organ. World Health Organ. [accessed: 3/25/2020] http://www.fao.org/fao-who-codexalimentarius/codex-texts/dbs/pestres/en/.
Crovesy, L., Masterson, D., and Rosado, E. L. (2020). Profile of the gut microbiota of adults with obesity: a systematic review. Eur. J. Clin. Nutr. 74, 1251–1262. doi:10.1038/s41430-020-0607-6
Cuhra, M. (2015). Review of GMO safety assessment studies: glyphosate residues in Roundup Ready crops is an ignored issue. Environ. Sci. Eur. 27, 20. doi:10.1186/s12302-015-0052-7
Da Silva, K. A., Beltrame Nicola, V., Tavares Dudas, R., Demetrio, D. C., dos Santos Maia, L., Cunha, L., et al. (2021). Pesticides in a case study on no-tillage farming systems and surrounding forest patches in Brazil. Nat. Scientifc Rep. 11, 9839. doi:10.1038/s41598-021-88779-3
Dai, P., Yan, Z., Ma, S., Yang, Y., Wang, Q., Hou, C., et al. (2018). The Herbicide Glyphosate Negatively Affects Midgut Bacterial Communities and Survival of Honey Bee during Larvae Reared In Vitro. J. Agric. Food Chem. 66, 7786–7793. doi:10.1021/acs.jafc.8b02212
Daouk, S., Copin, P.-J., Rossi, L., Chèvre, N., and Pfeifer, H.-R. (2013). Dynamics and environmental risk assessment of the herbicide glyphosate and its metabolite AMPA in a small vineyard river of the Lake Geneva catchment. Environ. Toxicol. Chem. 32, 2035–2044. doi:10.1002/etc.2276
Davoren, M. J., and Schiestl, R. H. (2018). Glyphosate-based herbicides and cancer risk: a post-IARC decision review of potential mechanisms, policy and avenues of research. Carcinogenesis 39 (10), 1207–1215. doi:10.1093/carcin/bgy105
De Angelis, M., Piccolo, M., Vannini, L., Siragusa, S., De Giacomo, A., Serrazzanetti, D. I., et al. (2013). Fecal microbiota and metabolome of children with autism and pervasive developmental disorder not otherwise specified. PLoS One 8, e76993. doi:10.1371/journal.pone10.1371/journal.pone.0076993
de Araujo, J. S. A., Delgado, I. F., and Paumgartten, F. J. R. (2016). Glyphosate and adverse pregnancy outcomes, a systematic review of observational studies. BMC Public Health 16, 472. doi:10.1186/s12889-016-3153-3
Dechartres, J., Pawluski, J. L., Gueguen, M. M., Jablaoui, A., Maguin, E., Rhimi, M., et al. (2019). Glyphosate and glyphosate-based herbicide exposure during the peripartum period affects maternal brain plasticity, maternal behaviour and microbiome. J. Neuroendocrinol 31 (9), e12731. doi:10.1111/jne.12731
Defarge, N., Spiroux de Vendômois, J., and Séralini, G. E. (2018). Toxicity of formulants and heavy metals in glyphosate-based herbicides and other pesticides. Toxicol. Rep. 5, 156–163. doi:10.1016/j.toxrep.2017.12.025
Deyett, E., Roper, M. C., Ruegger, P., Yang, J.-I., Borneman, J., and Rolshausen, P. E. (2017). Microbial Landscape of the Grapevine Endosphere in the Context of Pierce's Disease. Phytobiomes J. 1, 138–149. doi:10.1094/pbiomes-08-17-0033-r
Dharampal, P. S., Carlson, C., Currie, C. R., and Steffan, S. A. (2019). Pollen-borne microbes shape bee fitness. Proc. R. Soc. B. 286 (1904), 20182894. doi:10.1098/rspb.2018.2894
Driehuis, F., Wilkinson, J. M., Jiang, Y., Ogunade, I., and Adesogan, A. T. (2018). Silage review: Animal and human health risks from silage. J. Dairy Sci. 101 (5), 4093–4110. doi:10.3168/jds.2017-13836
Druille, M., Cabello, M. N., García Parisi, P. A., Golluscio, R. A., and Omacini, M. (2015). Glyphosate vulnerability explains changes in root-symbionts propagules viability in pampean grasslands. Agric. Ecosyst. Environ. 202, 48–55. doi:10.1016/j.agee.2014.12.017
Duke, S. O. (2018). Interaction of chemical pesticides and their formulation ingredients with microbes associated with plants and plant pests. J. Agric. Food Chem. 66, 7553–7561. doi:10.1021/acs.jafc.8b02316
Duke, S. O., Lydon, J., Koskinen, W. C., Moorman, T. B., Chaney, R. L., and Hammerschmidt, R. (2012). Glyphosate effects on plant mineral nutrition, crop rhizosphere microbiota, and plant disease in glyphosate-resistant crops. J. Agric. Food Chem. 60, 10375–10397. doi:10.1021/jf302436u
EC (European Commission) (2020). EU Pesticides Database – MRLs. [accessed: 3/14/2020]. https://ec.europa.eu/food/plant/pesticides/eu-pesticides-database/public/?event=download.MRLhttps://ec.europa.eu/food/plant/pesticides/eu-pesticides-database/public/?event=pesticide.residue.selection& language=EN.
EC (European Commission) (2016). Frequently Asked Questions: Glyphosate. [accessed: 08/23/2021]. https://ec.europa.eu/commission/presscorner/detail/en/MEMO_16_2012.
EC (European Commission) (2017). Summary report of the Appeal Committee Phytopharmaceuticals - Plant Protection Products – Legislation. , [accessed: 3/14/2020]. https://ec.europa.eu/food/sites/food/files/plant/docs/sc_phyto_20171127_pppl_summary.pdf.
EFSA (European Food Safety Authority) (2015). Conclusion on the peer review of the pesticide risk assessment of the active substance glyphosate. EFSA J. 201513 (11), 4302, 107. pp. 10.2903/j.efsa.2015.4302. [accessed: 3/25/2020].
EFSA (European Food Safety Authority) (2018). Evaluation of the impact of glyphosate and its residues in feed on animal health. EFSA J. 16 (55283), e05283. pp. doi.org/10.2903/j.efsa.2018.5283. [accessed: 3/25/2020]. doi:10.2903/j.efsa.2018.5283
Ehling, S., and Reddy, T. M. (2015). Analysis of Glyphosate and Aminomethylphosphonic Acid in Nutritional Ingredients and Milk by Derivatization with Fluorenylmethyloxycarbonyl Chloride and Liquid Chromatography-Mass Spectrometry. J. Agric. Food Chem. 63, 10562–10568. doi:10.1021/acs.jafc.5b04453
El Agrebi, N., Tosi, S., Wilmart, O., Scippo, M.-L., de Graaf, D. C., and Saegerman, C. (2020). Honeybee and consumer's exposure and risk characterisation to glyphosate-based herbicide (GBH) and its degradation product (AMPA): Residues in beebread, wax, and honey. Sci. Total Environ. 704, 135312. doi:10.1016/j.scitotenv.2019.135312
EPA (Environmental Protection Agency) (2020). “Electronic Code of Federal Regulations, Title 40: Protection of Environment. PART 180-Tolerances and Exemptions for Pesticide Chemical Residues in Food,” in Subpart C-specific Tolerances (Washington, DC: Environmental Protection Agency). 02.tpl [accessed: 3/14/2020]. http://www.ecfr.gov/cgi-bin/textidx?tpl=/ecfrbrowse/Title40/40cfr180_main_.
EPA (Environmental Protection Agency) (2017). Revised Glyphosate Issue Paper: Evaluation of Carcinogenic Potential. EPA’s Office of Pesticide Programs. [accessed: 3/14/2020]. https://cfpub.epa.gov/si/si_public_record_Report.cfm?Lab=OPP& dirEntryId=337935.
Faniband, M. H., Norén, E., Littorin, M., and Lindh, C. H. (2021). Human experimental exposure to glyphosate and biomonitoring of young Swedish adults. Int. J. Hyg. Environ. Health 231, 113657. doi:10.1016/j.ijheh.2020.113657
FAO (Food and Agricultural Organization) and WHO (World Health Organization) (2016). “Pesticide Residues in Food 2016,” in Report of the Special Session of the Joint Meeting of the FAO Panel of Experts on Pesticide Residues in Food and the Environment and the WHO Core Assessment Group on Pesticide Residues Geneva (Switzerland: FAO Plant Production and Protection Paper 227), 9–13. May 2016.
FAO (Food and Agricultural Organization) (2005). Report of the Joint Meeting on Pesticide Residues, 20–29 September 2005 in Geneva, Switzerland. . [accessed: 3/25/2020] http://www.fao.org/3/a0209e/a0209e0d.htm#TopOfPage.
FDA (US Food and Drug Administration) (2017). Pesticide Residue Monitoring Program Fiscal Year 2016 Pesticide Report. . [accessed: 3/25/2020] https://www.fda.gov/food/pesticides/pesticide-residue-monitoring-2016-report-and-data.
Fei, Y.-Y., Gai, J.-Y., and Zhao, T.-J. (2013). Identification of regulated genes conferring resistance to high concentrations of glyphosate in a new strain ofEnterobacter. FEMS Microbiol. Lett. 349, 135–143. doi:10.1111/1574-6968.12306
Fernandes, B., Soares, C., Braga, C., Rebotim, A., Ferreira, R., Ferreira, J., et al. (2020). Ecotoxicological Assessment of a Glyphosate-Based Herbicide in Cover Plants: Medicago sativa L. as a Model Species. Appl. Sci. 10, 5098. doi:10.3390/app10155098
Frank, A., Saldierna Guzmán, J., and Shay, J. (2017). Transmission of bacterial endophytes. Microorganisms 5, 70. doi:10.3390/microorganisms.504007010.3390/microorganisms5040070
Fu, H., Qiu, S., Yao, X., Gao, F., Tan, P., Teng, T., et al. (2020). Toxicity of glyphosate in feed for weanling piglets and the mechanism of glyphosate detoxification by the liver nuclear receptor CAR/PXR pathway. J. Hazard. Mater. 387 (5), 121707. doi:10.1016/j.jhazmat.2019.121707
Fu, X., Liu, Z., Zhu, C., Mou, H., and Kong, Q. (2019). Nondigestible carbohydrates, butyrate, and butyrate-producing bacteria. Crit. Rev. Food Sci. Nutr. 59 (Suppl. 1), S130–S152. 1549-7852. doi:10.1080/10408398.2018.1542587
Fuchs, B., Saikkonen, K., and Helander, M. (2021). Glyphosate-modulated biosynthesis driving plant defense and species interactions. Trends Plant Sci. 26 (4), 312–323. doi:10.1016/j.tplants.2020.11.004
Funke, T., Healy-Fried, M. L., Han, H., Alberg, D. G., Bartlett, P. A., and Schönbrunn, E. (2007). Differential Inhibition of Class I and Class II 5-Enolpyruvylshikimate-3-phosphate Synthases by Tetrahedral Reaction Intermediate Analogues,. Biochemistry 46, 13344–13351. doi:10.1021/bi701095u
Gao, H., Chen, J., Ding, F., Chou, X., Zhang, X., Wan, Y., et al. (2019). Activation of the N ‐methyl‐ d ‐aspartate receptor is involved in glyphosate‐induced renal proximal tubule cell apoptosis. J. Appl. Toxicol. 39 (8), 1096–1107. doi:10.1002/jat.3795
Geissen, V., Silva, V., Lwanga, E. H., Beriot, N., Oostindie, K., Bin, Z., et al. (2021). Cocktails of pesticide residues in conventional and organic farming systems in Europe - Legacy of the past and turning point for the future. Environ. Pollut. 278, 116827. doi:10.1016/j.envpol.2021.116827
Geng, Y., Jiang, L., Zhang, D., Liud, B., Zhang, J., Cheng, H., et al. (2021). Glyphosate, aminomethylphosphonic acid, and glufosinate ammonium in agricultural groundwater and surface water in China from 2017 to 2018: Occurrence, main drivers, and environmental risk assessment. Sci. Total Environ. 769, 0048–9697. doi:10.1016/j.scitotenv.2020.144396
Gerlach, H., Gerlach, A., Schrödl, W., Schottdorf, B., Haufe, S., Helm, H., et al. (2014). Oral application of charcoal and humic acids to dairy cows influences Clostridium botulinum blood serum antibody level and glyphosate excretion in urine. J. Clin. Toxicol. 4, 186. doi:10.4172/2161-0495.186
Gillezeau, C., Lieberman-Cribbin, W., and Taioli, E. (2020). Update on human exposure to glyphosate, with a complete review of exposure in children. Environ. Health 19, 115. doi:10.1186/s12940-020-00673-z
Gillezeau, C., van Gerwen, M., Shaffer, R. M., Rana, I., Zhang, L., Sheppard, L., et al. (2019). The evidence of human exposure to glyphosate: a review. Environ. Health 18, 2. doi:10.1186/s12940-018-0435-5
Gomes, M. P., Le Manac'h, S. G., Maccario, S., Labrecque, M., Lucotte, M., and Juneau, P. (2016). Differential effects of glyphosate and aminomethylphosphonic acid (AMPA) on photosynthesis and chlorophyll metabolism in willow plants. Pestic. Biochem. Physiol. 130, 65–70. doi:10.1016/j.pestbp.2015.11.010
Gomes, M. P., Rocha, D. C., Moreira de Brito, J. C., Tavares, D. S., Marques, R. Z., Soffiatti, P., et al. (2020). Emerging contaminants in water used for maize irrigation: Economic and food safety losses associated with ciprofloxacin and glyphosate. Ecotoxicology Environ. Saf. 196, 110549. doi:10.1016/j.ecoenv.2020.110549
Gómez-Gallego, C., Rainio, M. J., Collado, M. C., Mantziari, A., Salminen, S., Saikkonen, K., et al. (2020). Glyphosate-based herbicide affects the composition of microbes associated with Colorado potato beetle (Leptinotarsa decemlineata). FEMS Microbiol. Lett., 367. doi:10.1093/femsle/fnaa050
Graystock, P., Rehan, S. M., and McFrederick, Q. S. (2017). Hunting for healthy microbiomes: determining the core microbiomes of Ceratina, Megalopta, and Apis bees and how they associate with microbes in bee collected pollen. Conserv Genet. 18 (3), 701–711. doi:10.1007/s10592-017-0937-7
Greim, H., Saltmiras, D., Mostert, V., and Strupp, C. (2015). Evaluation of carcinogenic potential of the herbicide glyphosate, drawing on tumor incidence data from fourteen chronic/carcinogenicity rodent studies. Crit. Rev. Toxicol. 45, 185–208. doi:10.3109/10408444.2014.1003423
Grube, M., Kalnenieks, U., and Muter, O. (2019). Metabolic response of bacteria to elevated concentrations of glyphosate-based herbicide. Ecotoxicology Environ. Saf. 173, 373–380. doi:10.1016/j.ecoenv.2019.02.045
Guijarro, K. H., Aparicio, V., De Gerónimo, E., Castellote, M., Figuerola, E. L., Costa, J. L., et al. (2018). Soil microbial communities and glyphosate decay in soils with different herbicide application history. Sci. Total Environ. 634, 974–982. doi:10.1016/j.scitotenv.2018.03.393
Hammerschmidt, R. (2018). How glyphosate affects plant disease development: it is more than enhanced susceptibility. Pest Manag. Sci. 74, 1054–1063. doi:10.1002/ps.4521
Helander, M., Pauna, A., Saikkonen, K., and Saloniemi, I. (2019). Glyphosate residues in soil affect crop plant germination and growth. Sci. Rep. 9, 19653. doi:10.1038/s41598-019-56195-3
Helander, M., Saloniemi, I., Omacini, M., Druille, M., Salminen, J.-P., and Saikkonen, K. (2018). Glyphosate decreases mycorrhizal colonization and affects plant-soil feedback. Sci. Total Environ. 642, 285–291. doi:10.1016/j.scitotenv.2018.05.377
Hernández-Luna, M. A., López-Briones, S., and Luria-Pérez, R. (2019). The four horsemen in colon cancer. J. Oncol. 5636272, 12. doi:10.1155/2019/5636272
Heymann, A.-K., Schnabel, K., Billenkamp, F., Bühler, S., Frahm, J., Kersten, S., et al. (2021). Effects of glyphosate residues and different concentrate feed proportions in dairy cow rations on hepatic gene expression, liver histology and biochemical blood parameters. PLoS ONE 16 (2), e0246679. doi:10.1371/journal.pone.0246679
Honeycutt, Z., and Rowlands, H. (2014). Glyphosate Testing Report: Findings in American Mothers’ Breast Milk, Urine and Water. Moms Across America Sust. Pulse. https://www.momsacrossamerica.com/glyphosate_testing_results.
IARC (International Agency for Research on Cancer) (2015). Evaluation of five organophosphate insecticides and herbicides. IARC Monographs 112, World Health Organization, International Agency for Research on Cancer. Lyon: France. Volume112.pdf. [accessed: 3/25/2020] http://www.iarc.fr/en/media-centre/iarcnews/pdf/Monograph.
Ingaramo, P., Alarcón, R., Muñoz-de-Toro, M., and Luque, E. H. (2020). Are glyphosate and glyphosate-based herbicides endocrine disruptors that alter female fertility? Mol. Cell Endocrinol. 518, 110934. doi:10.1016/j.mce.2020.110934
Janßen, R., Zabel, J., von Lukas, U., and Labrenz, M. (2019). An artificial neural network and Random Forest identify glyphosate-impacted brackish communities based on 16S rRNA amplicon MiSeq read counts. Mar. Pollut. Bull. 149, 110530. doi:10.1016/j.marpolbul.2019.110530
Jarrell, Z. R., Ahammad, M. U., and Benson, A. P. (2020). Glyphosate-based herbicide formulations and reproductive toxicity in animals. Vet. Anim. Sci. 10, 100126. doi:10.1016/j.vas.2020.100126
Johal, G. S., and Huber, D. M. (2009). Glyphosate effects on diseases of plants. Eur. J. Agron. 31, 144–152. doi:10.1016/j.eja.2009.04.004
Johal, G. S., and Rahe, J. E. (1990). Role of phytoalexins in the suppression of resistance ofPhaseolus vulgaristoColletotrichum lindemuthianumby glyphosate. Can. J. Plant Pathol. 12 (3), 225–235. doi:10.1080/07060669009500992
Kandel, Y. R., Bradley, C. A., Wise, K. A., Chilvers, M. I., Tenuta, A. U., Davis, V. M., et al. (2015). Effect of glyphosate application on sudden death syndrome of glyphosate-resistant soybean under field conditions. Plant Dis. 99, 347–354. doi:10.1094/pdis-06-14-0577-re
Kao, H.-Y., Chung, K.-R., and Huang, J.-W. (2019). Paraquat and glyphosate increase severity of strawberry anthracnose caused by Colletotrichum gloeosporioides. J. Gen. Plant Pathol. 85, 23–32. doi:10.1007/s10327-018-0823-2
Karthikraj, R., and Kannan, K. (2019). Widespread occurrence of glyphosate in urine from pet dogs and cats in New York State, USA. Sci. Total Environ. 659, 790–795. doi:10.1016/j.scitotenv.2018.12.454
Katholm, C. L. (2016). “Effects of Roundup (glyphosate) on gut microorganisms of farm animals (Effekter af Roundup (glyfosat) på mikroorganismer fra husdyr). Master Thesis, Matriculation number 20095408, Department of Animal Science,” in Research Centre Foulum (Denmark: Aarhus University). [accessed: 3/25/2020] https://www.coursehero.com/file/28993562/Katholm-2016pdf/.
Kepler, R. M., Schmidt, D. J. E., Yarwood, S. A., Cavigelli, M. A., Reddy, K. N., Duke, S. O., et al. (2020). Erratum for Kepler et al., "Soil Microbial Communities in Diverse Agroecosystems Exposed to the Herbicide Glyphosate". Appl. Environ. Microbiol. 86 (5), e01744–19. doi:10.1128/AEM.01744-1910.1128/AEM.02262-20
Kiefer, J. S. T., Batsukh, S., Bauer, E., Hirota, B., Weiss, B., Wierz, J. C., et al. (2021). Inhibition of a nutritional endosymbiont by glyphosate abolishes mutualistic benefit on cuticle synthesis in Oryzaephilus surinamensis. Commun. Biol. 4, 554. doi:10.1038/s42003-021-02057-6
Krause, J. L., Haange, S.-B., Schäpe, S. S., Engelmann, B., Rolle-Kampczyk, U., Fritz-Wallace, K., et al. (2020). The glyphosate formulation Roundup LB plus influences the global metabolome of pig gut microbiota in vitro. Sci. Total Environ. 745, 140932. doi:10.1016/j.scitotenv.2020.140932.004
Kremer, R. J., and Means, N. E. (2009). Glyphosate and glyphosate-resistant crop interactions with rhizosphere microorganisms. Eur. J. Agron. 31, 153–161. doi:10.1016/j.eja.2009.06.004
Kremer, R., Means, N., and Kim, S. (2005). Glyphosate affects soybean root exudation and rhizosphere micro-organisms. Int. J. Environ. Anal. Chem. 85, 1165–1174. doi:10.1080/03067310500273146
Krüger, M., Schledorn, P., Schrödl, W., Hoppe, H.-W., Lutz, W., and Shehata, A. A. (2014a). Detection of glyphosate residues in animals and humans. J. Environ. Anal. Toxicol. 4, 2. doi:10.4172/2161-0525.1000210
Krüger, M., Schrödl, W., Pedersen, I., Schledorn, P., and Shehata, A. A. (2014b). Detection of glyphosate in malformed piglets. J. Environ. Anal. Toxicol. 04, 5. doi:10.4172/2161-0525.1000230
Krüger, M., Shehata, A. A., Schrödl, W., and Rodloff, A. (2013). Glyphosate suppresses the antagonistic effect of Enterococcus spp. on Clostridium botulinum. Anaerobe 20, 74–78. doi:10.1016/j.anaerobe.2013.01.005
Kuklinsky-Sobral, J., Araújo, W. L., Mendes, R., Pizzirani-Kleiner, A. A., and Azevedo, J. L. (2005). Isolation and characterization of endophytic bacteria from soybean (Glycine max) grown in soil treated with glyphosate herbicide. Plant Soil 273, 91–99. doi:10.1007/s11104-004-6894-1
Kurenbach, B., Hill, A. M., Godsoe, W., van Hamelsveld, S., and Heinemann, J. A. (2018). Agrichemicals and antibiotics in combination increase antibiotic resistance evolution. PeerJ 6, e5801. doi:10.7717/peerj.5801
Kurenbach, B., Marjoshi, D., Amábile-Cuevas, C. F., Ferguson, G. C., Godsoe, W., Gibson, P., et al. (2015). Sublethal Exposure to Commercial Formulations of the Herbicides Dicamba, 2,4-Dichlorophenoxyacetic Acid, and Glyphosate Cause Changes in Antibiotic Susceptibility in Escherichia coli and Salmonella enterica serovar Typhimurium. Mbio 6 (2), 13–30. doi:10.1128/mBio.00009-15
Kwiatkowska, M., Nowacka-Krukowska, H., and Bukowska, B. (2014). The effect of glyphosate, its metabolites and impurities on erythrocyte acetylcholinesterase activity. Environ. Toxicol. Pharmacol. 37, 1101–1108. doi:10.1016/j.etap.2014.04.008
La Cecilia, D., Tang, F. H. M., Coleman, N. V., Conoley, C., Vervoort, R. W., and Maggi, F. (2018). Glyphosate dispersion, degradation, and aquifer contamination in vineyards and wheat fields in the Po Valley, Italy. Water Res. 146, 37–54. doi:10.1016/j.watres.2018.09.008
Langemeier, C. B. (2012). Improved understanding of factors influencing the re-emergence of Goss’s bacterial wilt and blight of corn. Lincoln, NE, Master’s thesis: Univ. Nebraska. [accessed: 3/25/2020] https://digitalcommons.unl.edu/cgi/viewcontent.cgi?article=1061&context=agronhortdiss.
Langemeier, C. B., Robertson, A. E., Wang, D., Jackson-Ziems, T. A., and Kruger, G. R. (2017). Factors Affecting the Development and Severity of Goss's Bacterial Wilt and Leaf Blight of Corn, Caused by Clavibacter michiganensis subsp. nebraskensis. Plant Dis. 101, 54–61. doi:10.1094/pdis-01-15-0038-re
Larson, R. L., Hill, A. L., Fenwick, A., Kniss, A. R., Hanson, L. E., and Miller, S. D. (2006). Influence of glyphosate on Rhizoctonia and Fusarium root rot in sugar beet. Pest Manag. Sci. 62, 1182–1192. doi:10.1002/ps.1297
Leino, L., Tall, T., Helander, M., Saloniemi, I., Saikkonen, K., Ruuskanen, S., et al. (2021). Classification of the glyphosate target enzyme (5-enolpyruvylshikimate-3-phosphate synthase) for assessing sensitivity of organisms to the herbicide. J. Hazard. Mater. 408, 124556. doi:10.1016/j.jhazmat.2020.124556
Lemke, N., Murawski, A., Schmied-Tobies, M. I. H., Rucic, E., Hoppe, H.-W., Conrad, A., et al. (2021). Glyphosate and aminomethylphosphonic acid (AMPA) in urine of children and adolescents in Germany - Human biomonitoring results of the German Environmental Survey 2014-2017 (GerES V). Environ. Int. 156, 106769. doi:10.1016/j.envint.2021.106769
Liu, Y.-B., Long, M.-X., Yin, Y.-J., Si, M.-R., Zhang, L., Lu, Z.-Q., et al. (2013). Physiological roles of mycothiol in detoxification and tolerance to multiple poisonous chemicals in Corynebacterium glutamicum. Arch. Microbiol. 195, 419–429. doi:10.1007/s00203-013-0889-3
Lloyd-Price, J., Abu-Ali, G., and Huttenhower, C. (2016). The healthy human microbiome. Genome Med. 8, 51. doi:10.1186/s13073-016-0307-y
Lorch, M., Agaras, B., García-Parisi, P., Druille, M., Omacini, M., and Valverde, C. (2021). Repeated annual application of glyphosate reduces the abundance and alters the community structure of soil culturable pseudomonads in a temperate grassland. Agric. Ecosyst. Environ. 319, 107503. doi:10.1016/j.agee.2021.107503
Lu, G. H., Hua, X. M., Cheng, J., Zhu, Y. L., Wang, G. H., Pang, Y. J., et al. (2018). Impact of glyphosate on the rhizosphere microbial communities of an EPSPS-transgenic soybean line ZUTS31 by metagenome sequencing. Curr. Genomics 19, 36–49.
Lupwayi, N. Z., Fernandez, M. R., Kanashiro, D. A., and Petri, R. M. M. (2020). Profiles of wheat rhizobacterial communities in response to repeated glyphosate applications, crop rotation, and tillage. Can. J. Soil Sci. 101, 157–167. doi:10.1139/cjss-2020-0008
Ma, X., and Ning, S. (2019). Shikimic acid promotes estrogen receptor(ER)-positive breast cancer cells proliferation via activation of NF-Κb signaling. Toxicol. Lett. 312, 65–71. doi:10.1016/j.toxlet.2019.04.030
Maddalon, A., Galbiati, V., Colosio, C., Mandić-Rajčević, S., and Corsini, E. (2021). Glyphosate-based herbicides: Evidence of immune-endocrine alteration. Toxicology 459, 152851. doi:10.1016/j.tox.2021.152851
Maggi, F., la Cecilia, D., Tang, F. H. M., and McBratney, A. (2020). The global environmental hazard of glyphosate use. Sci. Total Environ. 717, 137167. doi:10.1016/j.scitotenv.2020.137167
Maggi, F., Tang, F. H. M., la Cecilia, D., and McBratney, A. (2019). PEST-CHEMGRIDS, global gridded maps of the top 20 crop-specific pesticide application rates from 2015 to 2025. Sci. Data 6, 170. doi:10.1038/s41597-019-0169-4
Mandl, K., Cantelmo, C., Gruber, E., Faber, F., Friedrich, B., and Zaller, J. G. (2018). Effects of Glyphosate-, Glufosinate- and Flazasulfuron-Based Herbicides on Soil Microorganisms in a Vineyard. Bull. Environ. Contam. Toxicol. 101, 562–569. doi:10.1007/s00128-018-2438-x
Manirajan, B. A., Maisinger, C., Ratering, S., Rusch, V., Schwiertz, A., Cardinale, M., et al. (2018). Diversity, specificity, co-occurrence and hub taxa of the bacterial-fungal pollen microbiome. FEMS Microbiol. Ecol. 94 (8), fiy112. doi:10.1093/femsec/fiy112
Manservisi, F., Lesseur, C., Panzacchi, S., Mandrioli, D., Falcioni, L., Bua, L., et al. (2019). The Ramazzini Institute 13-week pilot study glyphosate-based herbicides administered at human-equivalent dose to Sprague Dawley rats: effects on development and endocrine system. Environ. Health 18 (1), 15. doi:10.1186/s12940-019-0453-y
Mao, Q., Manservisi, F., Panzacchi, S., Mandrioli, D., Menghetti, I., Vornoli, A., et al. (2018). The Ramazzini Institute 13-week pilot study on glyphosate and Roundup administered at human-equivalent dose to Sprague Dawley rats: effects on the microbiome. Environ. Health 17, 50. doi:10.1186/s12940-018-0394-xb
Maqueda, C., Undabeytia, T., Villaverde, J., and Morillo, E. (2017). Behaviour of glyphosate in a reservoir and the surrounding agricultural soils. Sci. Total Environ. 593-594, 787–795. doi:10.1016/j.scitotenv.2017.03.202
Marques, J. G. C., Veríssimo, K. J. D. S., Fernandes, B. S., Ferreira, S. R. M., Montenegro, S. M. G. L., and Motteran, F. (2021). Glyphosate: A review on the current environmental impacts from a Brazilian perspective. Bull. Environ. Contam. Toxicol. 107, 385–397. doi:10.1007/s00128-021-03295-4
Martinez, D. A., Loening, U. E., and Graham, M. C. (2018). Impacts of glyphosate-based herbicides on disease resistance and health of crops: a review. Environ. Sci. Eur. 30, 2. doi:10.1186/s12302-018-0131-7
Mas, L. I., Aparicio, V. C., De Gerónimo, E., and Costa, J. L. (2020). Pesticides in water sources used for human consumption in the semiarid region of Argentina. SN Appl. Sci. 2, 691. doi:10.1007/s42452-020-2513-x
Masotti, F., Garavaglia, B. S., Piazza, A., Burdisso, P., Altabe, S., Gottig, N., et al. (2021). Bacterial isolates from Argentine Pampas and their ability to degrade glyphosate. Sci. Total Environ. 774, 145761. doi:10.1016/j.scitotenv.2021.145761
Massot, F., Gkorezis, P., McAmmond, B., d’Haen, J., Van Hamme, J., Merini, L. J., et al. (2019). First high-quality draft genome of Ochrobactrum haematophilum P6BS-III, a highly glyphosate-tolerant strain isolated from agricultural soil in Argentina. 3 Biotech. 9, 74. doi:10.1007/s13205-019-1606-y
Mendler, A., Geier, F., Haange, S.-B., Pierzchalski, A., Krause, J. L., Nijenhuis, I., et al. (2020). Mucosal-associated invariant T-Cell (MAIT) activation is altered by chlorpyrifos- and glyphosate-treated commensal gut bacteria. J. Immunotoxicology 17, 10–20. doi:10.1080/1547691X.2019.1706672
Mendonca, C. M., Reed, M. L., Kukurugya, M. A., and Aristilde, L. (2019). Adverse metabolic outcomes in soil Pseudomonas species exposed to polyethoxylated tallow amine and glyphosate. Environ. Sci. Technol. Lett. 6, 448–455. doi:10.1021/acs.estlett.9b00363
Mengistu, A., Reddy, K. N., Bellaloui, N., Walker, E. R., and Kelly, H. M. (2013). Effect of glyphosate on Macrophomina phaseolina in vitro and its effect on disease severity of soybean in the field. Crop Prot. 54, 23–28. doi:10.1016/j.cropro.2013.07.015
Mesnage, R., Teixeira, M., Mandrioli, D., Falcioni, L., Ducarmon, Q. R., Zwittink, R. D., et al. (2021). Use of shotgun metagenomics and metabolomics to evaluate the impact of glyphosate or Roundup Mon 52276 on the gut microbiota and serum metabolome of sprague-dawley rats. Environ. Health Perspect. 129 (1), 17005. doi:10.1289/EHP6990
Mesnage, R., and Antoniou, M. N. (2020). Computational modelling provides insight into the effects of glyphosate on the shikimate pathway in the human gut microbiome. Curr. Res. Toxicol. 1, 25–33. doi:10.1016/j.crtox.2020.04.001
Mesnage, R., and Antoniou, M. N. (2017). Facts and fallacies in the debate on glyphosate toxicity. Front. Public Health 5, 316. doi:10.3389/fpubh.2017.0031610.3389/fpubh.2017.00316
Mesnage, R., Arno, M., Costanzo, M., Malatesta, M., Séralini, G.-E., and Antoniou, M. N. (2015a). Transcriptome profile analysis reflects rat liver and kidney damage following chronic ultra-low dose Roundup exposure. Environ. Health 14, 70. doi:10.1186/s12940-015-0056-1
Mesnage, R., Defarge, N., Spiroux de Vendômois, J., and Séralini, G. E. (2015b). Potential toxic effects of glyphosate and its commercial formulations below regulatory limits. Food Chem. Toxicol. 84, 133–153. doi:10.1016/j.fct.2015.08.012
Messaoudi, M., Violle, N., Bisson, J.-F., Desor, D., Javelot, H., and Rougeot, C. (2011). Beneficial psychological effects of a probiotic formulation (Lactobacillus helveticusR0052 andBifidobacterium longumR0175) in healthy human volunteers. Gut Microbes 2, 256–261. doi:10.4161/gmic.2.4.16108
Miyazaki, J., Bauer-Panskus, A., Bøhn, T., Reichenbecher, W., and Then, C. (2019). Insufficient risk assessment of herbicide-tolerant genetically engineered soybeans intended for import into the EU. Environ. Sci. Eur. 31, 92. doi:10.1186/s12302-019-0274-1
Motta, E. V. S., Mak, M., De Jong, T. K., Powell, J. E., O'Donnell, A., Suhr, K. J., et al. (2020). Oral or topical exposure to glyphosate in herbicide formulation impacts the gut microbiota and survival rates of honey bees. Appl. Environ. Microbiol. 86, e01150–20. doi:10.1128/AEM.01150-20
Motta, E. V. S., and Moran, N. A. (2020). Impact of glyphosate on the honey bee gut microbiota: effects of intensity, duration, and timing of exposure. mSystems 5, e00268–20. doi:10.1128/mSystems.00268-20
Motta, E. V. S., Raymann, K., and Moran, N. A. (2018). Glyphosate perturbs the gut microbiota of honey bees. Proc. Natl. Acad. Sci. USA 115 (41), 10305–10310. doi:10.1073/pnas.1803880115
Muola, A., Fuchs, B., Laihonen, M., Rainio, K., Heikkonen, L., Ruuskanen, S., et al. (2021). Risk in the circular food economy: Glyphosate-based herbicide residues in manure fertilizers decrease crop yield. Sci. Total Environ. 750, 141422. doi:10.1016/j.scitotenv.2020.141422
Myers, J. P., Antoniou, M. N., Blumberg, B., Carroll, L., Colborn, T., Everett, L. G., et al. (2016). Concerns over use of glyphosate-based herbicides and risks associated with exposures: a consensus statement. Environ. Health 15, 19. doi:10.1186/s12940-016-0117-0
Nerozzi, C., Recuero, S., Galeati, G., Bucci, D., Spinaci, M., and Yeste, M. (2020). Effects of Roundup and its main component, glyphosate, upon mammalian sperm function and survival. Sci. Rep. 10, 11026. doi:10.1038/s41598-020-67538-w
Newman, M. M., Lorenz, N., Hoilett, N., Lee, N. R., Dick, R. P., Liles, M. R., et al. (2016). Changes in rhizosphere bacterial gene expression following glyphosate treatment. Sci. Total Environ. 553, 32–41. doi:10.1016/j.scitotenv.2016.02.078
Nielsen, L. N., Roager, H. M., Casas, M. E., Frandsen, H. L., Gosewinkel, U., Bester, K., et al. (2018). Glyphosate has limited short-term effects on commensal bacterial community composition in the gut environment due to sufficient aromatic amino acid levels. Environ. Pollut. 233, 364–376. doi:10.1016/j.envpol.2017.10.016
Niemann, L., Sieke, C., Pfeil, R., and Solecki, R. (2015). A critical review of glyphosate findings in human urine samples and comparison with the exposure of operators and consumers. J. Verbr. Lebensm. 10, 3–12. doi:10.1007/s00003-014-0927-3
Okada, E., Costa, J. L., and Bedmar, F. (2016). Adsorption and mobility of glyphosate in different soils under no-till and conventional tillage. Geoderma 263, 78–85. doi:10.1016/j.geoderma.2015.09.009
Panzacchi, S., Mandrioli, D., Manservisi, F., Bua, L., Falcioni, L., Spinaci, M., et al. (2018). The Ramazzini Institute 13-week study on glyphosate-based herbicides at human-equivalent dose in Sprague Dawley rats: study design and first in-life endpoints evaluation. Environ. Health 17, 52. doi:10.1186/s12940-018-0393-y
Parvez, S., Gerona, R. R., Proctor, C., Friesen, M., Ashby, J. L., Reiter, J. L., et al. (2018). Glyphosate exposure in pregnancy and shortened gestational length: a prospective Indiana birth cohort study. Environ. Health 17, 23. doi:10.1186/s12940-018-0367-0
Peillex, C., and Pelletier, M. (2020). The impact and toxicity of glyphosate and glyphosate-based herbicides on health and immunity. J. Immunotoxicology 17 (1), 163–174. doi:10.1080/1547691X.2020.1804492
Peng, W., Lam, S. S., and Sonne, C. (2020). Support Austria's glyphosate ban. Science 367 (6475), 257–258. doi:10.1126/science.aba5642
Pérez Rodríguez, M., Melo, C., Jiménez, E., and Dussán, J. (2019). Glyphosate bioremediation through the sarcosine oxidase pathway mediated by Lysinibacillus sphaericus in soils cultivated with potatoes. Agriculture 9 (10), 217. doi:10.3390/agriculture9100217
Peruzzo, P. J., Porta, A. A., and Ronco, A. E. (2008). Levels of glyphosate in surface waters, sediments and soils associated with direct sowing soybean cultivation in north pampasic region of Argentina. Environ. Pollut. 156, 61–66. doi:10.1016/j.envpol.2008.01.015
Pleguezuelos-Manzano, C., Puschhof, J., Rosendahl Huber, A., van Hoeck, A., Wood, H. M., Nomburg, J., et al. (2020). Mutational signature in colorectal cancer caused by genotoxic pks+ E. coli. Nature 580, 269–273. doi:10.1038/s41586-020-2080-8
Poiger, T., Buerge, I. J., Bächli, A., Müller, M. D., and Balmer, M. E. (2017). Occurrence of the herbicide glyphosate and its metabolite AMPA in surface waters in Switzerland determined with on-line solid phase extraction LC-MS/MS. Environ. Sci. Pollut. Res. 24, 1588–1596. doi:10.1007/s11356-016-7835-2
Pöppe, J., Bote, K., Ramesh, A., Murugaiyan, J., Kuropka, B., Kühl, M., et al. (2020). Selection for resistance to a glyphosate-containing herbicide in Salmonella enterica does not result in a sustained activation of the tolerance response or increased cross-tolerance and cross-resistance to clinically important antibiotics. Appl. Environ. Microbiol. 86, e01204–20. doi:10.1128/AEM.01204-20
Pöppe, J., Bote, K., Merle, R., Makarova, O., and Roesler, U. (2019). Minimum inhibitory concentration of glyphosate and a glyphosate-containing herbicide in Salmonella enterica isolates originating from different time periods, hosts, and serovars. Eur. J. Microbiol. Immunol. 9 (2), 35–41. doi:10.1556/1886.2019.00005
Priestman, M. A., Funke, T., Singh, I. M., Crupper, S. S., and Schönbrunn, E. (2005). 5-Enolpyruvylshikimate-3-phosphate synthase fromStaphylococcus aureusis insensitive to glyphosate. FEBS Lett. 579, 728–732. doi:10.1016/j.febslet.2004.12.057
Qiao, C., Wang, C., Pang, R., Tian, F., Han, L., Guo, L., et al. (2020). Environmental behavior and influencing factors of glyphosate in peach orchard ecosystem. Ecotoxicology Environ. Saf. 206, 111209. Environ. Safety. doi:10.1016/j.ecoenv.2020.111209
Qiu, S., Fu, H., Zhou, R., Yang, Z., Bai, G., and Shi, B. (2020). Toxic effects of glyphosate on intestinal morphology, antioxidant capacity and barrier function in weaned piglets. Ecotoxicology Environ. Saf. 187, 109846. doi:10.1016/j.ecoenv.2019.109846
Rainio, M. J., Ruuskanen, S., Helander, M., Saikkonen, K., Saloniemi, I., and Puigbò, P. (2021). Adaptation of bacteria to glyphosate: a microevolutionary perspective of the enzyme 5‐enolpyruvylshikimate‐3‐phosphate synthase. Environ. Microbiol. Rep. 13 (3), 309–316. doi:10.1111/1758-2229.12931
Ravishankar, A., Pupo, A., and Gallagher, J. E. G. (2020). Resistance mechanisms of Saccharomyces cerevisiae to commercial formulations of glyphosate involve DNA damage repair, the cell cycle, and the cell wall structure. Genes/Genome/Genetics 10, 2043–2056. doi:10.1534/g3.120.401183
Raymann, K., and Moran, N. A. (2018). The role of the gut microbiome in health and disease of adult honey bee workers. Curr. Opin. Insect Sci. 26, 97–104. doi:10.1016/j.cois.2018.02.012
Riede, S., Toboldt, A., Breves, G., Metzner, M., Köhler, B., Bräunig, J., et al. (2016). Investigations on the possible impact of a glyphosate-containing herbicide on ruminal metabolism and bacteriain vitroby means of the 'Rumen Simulation Technique'. J. Appl. Microbiol. 121, 644–656. doi:10.1111/jam.13190
Rinninella, E., Raoul, P., Cintoni, M., Franceschi, F., Miggiano, G., Gasbarrini, A., et al. (2019). What is the healthy gut microbiota composition? A changing ecosystem across age, environment, diet, and diseases. Microorganisms 7, 14. doi:10.3390/microorganisms7010014
Rodloff, A. C., and Krüger, M. (2012). Chronic Clostridium botulinum infections in farmers. Anaerobe 18, 226–228. doi:10.1016/j.anaerobe.2011.12.011
Roman, P., Rueda-Ruzafa, L., Cardona, D., and Cortes-Rodríguez, A. (2018). Gut-brain axis in the executive function of austism spectrum disorder. Behav. Pharmacol. 29, 654–663. doi:10.1097/fbp.0000000000000428
Romdhane, S., Spor, A., Busset, H., Falchetto, L., Martin, J., and Bizouard, F. (2019). Cover crop management practices rather than composition of cover crop mixtures affect bacterial communities in no-till agroecosystems. Front. Microbiol. doi:10.3389/fmicb.2019.01618
Rosenbaum, K. K., Miller, G. L., Kremer, R. J., and Bradley, K. W. (2014). Interactions between Glyphosate,FusariumInfection of Common Waterhemp (Amaranthus rudis), and Soil Microbial Abundance and Diversity in Soil Collections from Missouri. Weed Sci. 62, 71–82. doi:10.1614/ws-d-13-00071.1
Rosenberg, E., and Zilber-Rosenberg, I. (2016). Microbes drive evolution of animals and plants: the hologenome concept. Mbio 7 (2), e01395–15. doi:10.1128/mBio.01395-15
Rueda-Ruzafa, L., Cruz, F., Roman, P., and Cardona, D. (2019). Gut microbiota and neurological effects of glyphosate. NeuroToxicology 75, 1–8. doi:10.1016/j.neuro.2019.08.006
Ruuskanen, S., Rainio, M. J., Gómez-Gallego, C., Selenius, O., Salminen, S., Collado, M. C., et al. (2020a). Glyphosate-based herbicides influence antioxidants, reproductive hormones and gut microbiome but not reproduction: A long-term experiment in an avian model. Environ. Pollut. 266, 115108. doi:10.1016/j.envpol.2020.115108
Ruuskanen, S., Rainio, M. J., Kuosmanen, V., Laihonen, M., Saikkonen, K., Saloniemi, I., et al. (2020b). Female preference and adverse developmental effects of glyphosate-based herbicides on ecologically relevant traits in Japanese quails. Environ. Sci. Technol. 54, 1128–1135. doi:10.1021/acs.est.9b07331
Ruuskanen, S., Rainio, M. J., Uusitalo, M., Saikkonen, K., and Helander, M. (2020c). Effects of parental exposure to glyphosate-based herbicides on embryonic development and oxidative status: a long-term experiment in a bird model. Sci. Rep. 10, 6349. doi:10.1038/s41598-020-63365-1
Samac, D. A., and Foster-Hartnett, D. (2012). Effect of glyphosate application on foliar diseases in glyphosate-tolerant alfalfa. Plant Dis. 96, 1104–1110. doi:10.1094/pdis-08-11-0715-re
Sanogo, S., Yang, X. B., and Scherm, H. (2000). Effects of herbicides on Fusarium solani f. sp. glycines and development of sudden death syndrome in glyphosate-tolerant soybean. Phytopathology 90, 57–66. doi:10.1094/phyto.2000.90.1.57
Santos, S. A. d., Tuffi‐Santos, L. D., Tanaka, F. A. O., Sant'Anna‐Santos, B. F., Rodrigues, F. d. Á., and Alfenas, A. C. (2018). Carfentrazone‐ethyl and glyphosate drift inhibits uredinial formation of Austropuccinia psidii on Eucalyptus grandis leaves. Pest Manag. Sci. 75, 53–62. doi:10.1002/ps.5163
Schafer, J. R., Hallett, S. G., and Johnson, W. G. (2014). Rhizosphere microbial community dynamics in glyphosate-treated susceptible and resistant biotypes of giant ragweed (Ambrosia trifida). Weed Sci. 62, 370–381. doi:10.1614/ws-d-13-00164.1
Schlatter, D. C., Yin, C., Burke, I., Hulbert, S., and Paulitz, T. (2018). Location, root proximity, and glyphosate-use history modulate the effects of glyphosate on fungal community networks of wheat. Microb. Ecol. 76, 240–257. doi:10.1007/s00248-017-1113-9
Schnabel, K., Schmitz, R., Frahm, J., Meyer, U., Breves, G., and Dänicke, S. (2020). Functionality and DNA-damage properties of blood cells in lactating cows exposed to glyphosate contaminated feed at different feed energy levels. Arch. Anim. Nutr. 74 (2), 87–106. doi:10.1080/1745039X.2020.1718474
Schnabel, K., Schmitz, R., von Soosten, D., Frahm, J., Kersten, S., Meyer, U., et al. (2017). Effects of glyphosate residues and different concentrate feed proportions on performance, energy metabolism and health characteristics in lactating dairy cows. Arch. Anim. Nutr. 71, 413–427. doi:10.1080/1745039x.2017.1391487
Schrödl, W., Krüger, S., Konstantinova-Müller, T., Shehata, A. A., Rulff, R., and Krüger, M. (2014). Possible Effects of Glyphosate on Mucorales Abundance in the Rumen of Dairy Cows in Germany. Curr. Microbiol. 69, 817–823. doi:10.1007/s00284-014-0656-y
Scotti, E., Boue, S., Lo Sasso, G., Zanetti, F., Belcastro, V., Poussin, C., et al. (2017). Exploring the microbiome in health and disease: Implications for toxicology. Toxicol. Res. Applic 1, 1–37. doi:10.1177/2397847317741884
Séralini, G.-E., Clair, E., Mesnage, R., Gress, S., Defarge, N., Malatesta, M., et al. (2014). Republished study: long-term toxicity of a Roundup herbicide and a Roundup-tolerantgenetically modified maize. Environ. Sci. Eur. 26 (1), 14. doi:10.1186/s12302-014-0014-5
Séralini, G.-E. (2020). Update on long-term toxicity of agricultural GMOs tolerant to roundup. Environ. Sci. Eur. 32, 18. doi:10.1186/s12302-020-0296-8
Shaw, W. (2017). Elevated Urinary Glyphosate and Clostridia Metabolites with Altered Dopamine Metabolism in Triplets with Autistic Spectrum Disorder or Suspected Seizure Disorder: A Case Study. Integr. Med. (Encinitas) 16, 50–57.
Shehata, A. A., Schrödl, W., Aldin, A. A., Hafez, H. M., and Krüger, M. (2013). The Effect of Glyphosate on Potential Pathogens and Beneficial Members of Poultry Microbiota In Vitro. Curr. Microbiol. 66, 350–358. doi:10.1007/s00284-012-0277-2
Shehata, A. A., Schrödl, W., Schledorn, P., and Krüger, M. (2014). Distribution of glyphosate in chicken organs and its reduction by humic acid supplementation. Jpn. Poult. Sci. 51, 334–338. doi:10.2141/jpsa.0130169
Silva, V., Montanarella, L., Jones, A., Fernández-Ugalde, O., Mol, H. G. J., Ritsema, C. J., et al. (2018). Distribution of glyphosate and aminomethylphosphonic acid (AMPA) in agricultural topsoils of the European Union. Sci. Total Environ. 621, 1352–1359. doi:10.1016/j.scitotenv.2017.10.093
Silver, M. K., Fernandez, J., Tang, J., McDade, A., Sabino, J., Rosario, Z., et al. (2021). Prenatal Exposure to Glyphosate and Its Environmental Degradate, Aminomethylphosphonic Acid (AMPA), and Preterm Birth: A Nested Case-Control Study in the PROTECT Cohort (Puerto Rico). Environ. Health Perspect. 129 (5), 057011. doi.org/10.1289/EHP7295. doi:10.1289/ehp7295
Smith, D. F. Q., Camacho, E., Thakur, R., Barron, A. J., Dong, Y., Dimopoulos, G., et al. (2021). Glyphosate inhibits melanization and increases susceptibility to infection in insects. Plos Biol. 19 (5), e3001182. doi:10.1371/journal.pbio.3001182
Soukup, S. T., Merz, B., Bub, A., Hoffmann, I., Watzl, B., Steinberg, P., et al. (2020). Glyphosate and AMPA levels in human urine samples and their correlation with food consumption: results of the cross-sectional KarMeN study in Germany. Arch. Toxicol. 94 (5), 1575–1584. doi:10.1007/s00204-020-02704-7
Staub, J. M., Brand, L., Tran, M., Kong, Y., and Rogers, S. G. (2012). Bacterial glyphosate resistance conferred by overexpression of an E. coli membrane efflux transporter. J. Ind. Microbiol. Biotechnol. 39, 641–647. doi:10.1007/s10295-011-1057-x
Steinborn, A., Alder, L., Michalski, B., Zomer, P., Bendig, P., Martinez, S. A., et al. (2016). Determination of glyphosate levels in breast milk samples from Germany by LC-MS/MS and GC-MS/MS. J. Agric. Food Chem. 64 (6), 1414–1421. doi:10.1021/acs.jafc.5b05852
Straw, E. A., Carpentier, E. N., and Brown, M. J. F. (2021). Roundup causes high levels of mortality following contact exposure in bumble bees. J. Appl. Ecol. 00, 1–10. doi:10.1111/1365-2664.13867
Swanson, N. L., Leu, A., Abrahamson, J., and Wallet, B. (2014). Genetically engineered crops, glyphosate and the deterioration of health in the United States of America. J. Org. Syst. 9, 6–37.
Székács, A., and Darvas, B. (2018). Re-registration challenges of glyphosate in the European Union. Front. Environ. Sci., 6. doi:10.3389/fenvs.2018.00078
Tang, F. H. M., Jeffries, T. C., Vervoort, R. W., Conoley, C., Coleman, N. V., and Maggi, F. (2019). Microcosm experiments and kinetic modeling of glyphosate biodegradation in soils and sediments. Sci. Total Environ. 658, 105–115. doi:10.1016/j.scitotenv.2018.12.179
Tang, Q., Tang, J., Ren, X., and Li, C. (2020). Glyphosate exposure induces inflammatory responses in the small intestine and alters gut microbial composition in rats. Environ. Pollut. 261, 114129. doi:10.1016/j.envpol.2020.114129
Tauchnitz, N., Kurzius, F., Rupp, H., Schmidt, G., Hauser, B., Schrödter, M., et al. (2020). Assessment of pesticide inputs into surface waters by agricultural and urban sources - A case study in the Querne/Weida catchment, central Germany. Environ. Pollut. 267, 115186. doi:10.1016/j.envpol.2020.115186
Tauchnitz, N., Schrödter, M., Schmidt, G., and Hauser, B. (2018). Pflanzenschutzmittelwirkstoffe in Oberflächengewässern – Eintragspfade und Reduzierungsmaßnahmen. 61. Deutsche Pflanzenschutztagung. Herausforderung Pflanzenschutz - Wege in die Zukunft, 364–365. 11.-14. Sept. 2018. Julius Kühn Institut, Universität Hohenheim.
Trasande, L., Aldana, S. I., Trachtman, H., Kannan, K., Morrison, D., Christakis, D. A., et al. (2020). Glyphosate exposures and kidney injury biomarkers in infants and young children. Environ. Pollut. 256, 113334. doi:10.1016/j.envpol.2019.113334
Tsiaoussis, J., Antoniou, M. N., Koliarakis, I., Mesnage, R., Vardavas, C. I., Izotov, B. N., et al. (2019). Effects of single and combined toxic exposures on the gut microbiome: Current knowledge and future directions. Toxicol. Lett. 312, 72–97. doi:10.1016/j.toxlet.2019.04.014
Uyemura, S. A., Stopper, H., Martin, F. L., and Kannen, V. (2017). A perspective discussion on rising pesticide levels and colon cancer burden in Brazil. Front. Public Health 5, 273. doi:10.3389/fpubh.2017.00273
van Bruggen, A. H. C., Goss, E. M., Havelaar, A., van Diepeningen, A. D., Finckh, M. R., and Morris, J. G. (2019). One Health - Cycling of diverse microbial communities as a connecting force for soil, plant, animal, human and ecosystem health. Sci. Total Environ. 664, 927–937. doi:10.1016/j.scitotenv.2019.02.091
van Bruggen, A. H. C., He, M. M., Shin, K., Mai, V., Jeong, K. C., Finckh, M. R., et al. (2018). Environmental and health effects of the herbicide glyphosate. Sci. Total Environ. 616-617, 255–268. doi:10.1016/j.scitotenv.2017.10.309
van Bruggen, A. H. C., Sharma, K., Kaku, E., Karfopoulos, S., Zelenev, V. V., and Blok, W. J. (2015). Soil health indicators and Fusarium wilt suppression in organically and conventionally managed greenhouse soils. Appl. Soil Ecol. 86, 192–201. doi:10.1016/j.apsoil.2014.10.014
van Eenennaam, A. L., and Young, A. E. (2017). Detection of dietary DNA, protein, and glyphosate in meat, milk, and eggs. J. Anim. Sci. 95, 3247–3269. doi:10.2527/jas2016.1346
Vázquez, M. B., Moreno, M. V., Amodeo, M. R., and Bianchinotti, M. V. (2021). Effects of glyphosate on soil fungal communities: A field study. Rev. Argent Microbiol. 2021, S0325–S7541. doi:10.1016/j.ram.2020.10.005
Vicini, J. L., Reeves, W. R., Swarthout, J. T., and Karberg, K. A. (2019). Glyphosate in livestock: feed residues and animal health1. J. Anim. Sci. 97 (11), 4509–4518. doi:10.1093/jas/skz295
von Soosten, D., Meyer, U., Hüther, L., Dänicke, S., Lahrssen-Wiederholt, M., Schafft, H., et al. (2016). Excretion pathways and ruminal disappearance of glyphosate and its degradation product aminomethylphosphonic acid in dairy cows. J. Dairy Sci. 99, 5318–5324. doi:10.3168/jds.2015-10585
Walker, E. R., and Oliver, L. R. (2008). Translocation and absorption of glyphosate in flowering sicklepod (Senna obtusifolia). Weed Sci. 56, 338–343. doi:10.1614/ws-07-069.1
Weng, Z., Rose, M. T., Tavakkoli, E., van Zwieten, L., Styles, G., Bennett, W., et al. (2019). Assessing plant-available glyphosate in contrasting soils by diffusive gradient in thin-films technique (DGT). Sci. Total Environ. 646, 735–744. doi:10.1016/j.scitotenv.2018.07.221
WHO (World Health Organization) (2005). Glyphosate and AMPA in Drinking-Water. http://www.who.int/water_sanitation_health/dwq/chemicals/glyphosateampa290605.pdf.2005Accessed: 3/25/2020]
WHO (World Health Organization) (2009). The WHO recommended classification of pesticides by hazard and guidelines to classification: 2009. Int. Program Chem. Saf. [accessed: 3/25/2020] http://www.who.int/ipcs/publications/pesticides_hazard_2009.pdf?ua=1.
Wicaksono, W. A., Kusstatscher, P., Erschen, S., Reisenhofer-Graber, T., Grube, M., Cernava, T., et al. (2021). Antimicrobial-specific response from resistance gene carriers studied in a natural, highly diverse microbiome. Microbiome 9, 29. doi:10.1186/s40168-020-00982-y
Williams, M. M., Bradley, C. A., Duke, S. O., Maul, J. E., and Reddy, K. N. (2015). Goss's Wilt Incidence in Sweet Corn Is Independent of Transgenic Traits and Glyphosate. horts 50 (12), 1791–1794. doi:10.21273/hortsci.50.12.1791
Wolmarans, K., and Swart, W. J. (2014). Influence of glyphosate, other herbicides and genetically modified herbicide-resistant crops on soil microbiota: a review. South Afr. J. Plant Soil 31, 177–186. doi:10.1080/02571862.2014.960485
Woźniak, E., Sicińska, P., Michałowicz, J., Woźniak, K., Reszka, E., Huras, B., et al. (2018). The mechanism of DNA damage induced by Roundup 360 PLUS, glyphosate and AMPA in human peripheral blood mononuclear cells - genotoxic risk assessement. Food Chem. Toxicol. 120, 510–522. doi:10.1016/j.fct.2018.07.035
Xu, B., Sun, Q.-J., Lan, J. C.-W., Chen, W.-M., Hsueh, C.-C., and Chen, B.-Y. (2019). Exploring the glyphosate-degrading characteristics of a newly isolated, highly adapted indigenous bacterial strain, Providencia rettgeri GDB 1. J. Biosci. Bioeng. 128 (1), 80–87. doi:10.1016/j.jbiosc.2019.01.012
Yang, X., Wang, F., Bento, C. P. M., Xue, S., Gai, L., van Dam, R., et al. (2015). Short-term transport of glyphosate with erosion in Chinese loess soil - A flume experiment. Sci. Total Environ. 512-513, 406–414. doi:10.1016/j.scitotenv.2015.01.071
Yang, Y., and Jobin, C. (2014). Microbial imbalance and intestinal pathologies: connections and contributions. Dis. Model. Mech. 7 (10), 1131–1142. doi:10.1242/dmm.016428
You, M. J., Shin, G. W., and Lee, C. S. (2015). Clostridium tertium bacteremia in a patient with glyphosate ingestion. Am. J. Case Rep. 16, 4–7. doi:10.12659/AJCR.891287
Yu, N., Tong, Y., Zhang, D., Zhao, S., Fan, X., Wu, L., et al. (2018). Circular RNA expression profiles in hippocampus from mice with perinatal glyphosate exposure. Biochem. Biophysical Res. Commun. 501, 838–845. doi:10.1016/j.bbrc.2018.04.200
Yu, X. M., Yu, T., Yin, G. H., Dong, Q. L., An, M., Wang, H. R., et al. (2015). Glyphosate biodegradation and potential soil bioremediation by Bacillus subtilis strain Bs-15. Genet. Mol. Res. 14 (4), 14717–14730. doi:10.4238/2015.november.18.37
Zabaloy, M. C., Carné, I., Viassolo, R., Gómez, M. A., and Gomez, E. (2016). Soil ecotoxicity assessment of glyphosate use under field conditions: microbial activity and community structure of Eubacteria and ammonia-oxidising bacteria. Pest Manag. Sci. 72, 684–691. doi:10.1002/ps.4037
Zaller, J. G., Cantelmo, C., Santos, G. D., Muther, S., Gruber, E., Pallua, P., et al. (2018). Herbicides in vineyards reduce grapevine root mycorrhization and alter soil microorganisms and the nutrient composition in grapevine roots, leaves, xylem sap and grape juice. Environ. Sci. Pollut. Res. 25, 23215–23226. doi:10.1007/s11356-018-2422-3
Zhang, C., Hu, X., Luo, J., Wu, Z., Wang, L., Li, B., et al. (2015). Degradation dynamics of glyphosate in different types of citrus orchard soils in China. Molecules 20, 1161–1175. doi:10.3390/molecules20011161
Zhang, L., Rana, I., Shaffer, R. M., Taioli, E., and Sheppard, L. (2019). Exposure to glyphosate-based herbicides and risk for non-hodgkin lymphoma: A meta-analysis and supporting evidence. Mutat. Research/Reviews Mutat. Res. 781, 186–206. doi:10.1016/j.mrrev.2019.02.001
Zhang, M., Wang, W., Tang, L., Heenan, M., and Xu, Z. (2018). Effects of nitrification inhibitor and herbicides on nitrification, nitrite and nitrate consumptions and nitrous oxide emission in an Australian sugarcane soil. Biol. Fertil. Soils 54, 697–706. doi:10.1007/s00374-018-1293-6
Zhao, H., Tao, K., Zhu, J., Liu, S., Gao, H., and Zhou, X. (2015). Bioremediation potential of glyphosate-degrading Pseudomonas spp. strains isolated from contaminated soil. J. Gen. Appl. Microbiol. 61, 165–170. doi:10.2323/jgam.61.165
Zhao, J., Pacenka, S., Wu, J., Richards, B. K., Steenhuis, T., Simpson, K., et al. (2018). Detection of glyphosate residues in companion animal feeds. Environ. Pollut. 243, 1113–1118. doi:10.1016/j.envpol.2018.08.100
Zhou, X., Liang, J., Luan, Y., Song, X., and Zhang, Z. (2020). The influence of genetically modified glyphosate-tolerant maize CC-2 on rhizosphere bacterial communities revealed by MiSeq sequencing. Plant Soil Environ. 66, 387–394. doi:10.17221/216/2020-pse
Keywords: health, microbiome, Roundup, residues, side effects, minimal inhibitory concentration
Citation: van Bruggen AHC, Finckh MR, He M, Ritsema CJ, Harkes P, Knuth D and Geissen V (2021) Indirect Effects of the Herbicide Glyphosate on Plant, Animal and Human Health Through its Effects on Microbial Communities. Front. Environ. Sci. 9:763917. doi: 10.3389/fenvs.2021.763917
Received: 24 August 2021; Accepted: 28 September 2021;
Published: 18 October 2021.
Edited by:
Anabela Cachada, University of Porto, PortugalReviewed by:
Pere Puigbo, University of Turku, FinlandFolarin Owagboriaye, Olabisi Onabanjo University, Nigeria
Federica Giambò, University of Messina, Italy
Copyright © 2021 van Bruggen, Finckh, He, Ritsema, Harkes, Knuth and Geissen. This is an open-access article distributed under the terms of the Creative Commons Attribution License (CC BY). The use, distribution or reproduction in other forums is permitted, provided the original author(s) and the copyright owner(s) are credited and that the original publication in this journal is cited, in accordance with accepted academic practice. No use, distribution or reproduction is permitted which does not comply with these terms.
*Correspondence: A. H. C. van Bruggen, YWhjdmFuYnJ1Z2dlbkB1ZmwuZWR1