- 1Department of Civil and Environmental Engineering, Colorado School of Mines, Golden, CO, United States
- 2Research Applications Laboratory, United States National Center for Atmospheric Research, Boulder, CO, United States
- 3Shiley School of Engineering, University of Portland, Portland, OR, United States
- 4Biology Department, Southern California Coastal Water Research Project, Costa Mesa, CA, United States
Anthropogenic development has adversely affected river habitat and species diversity in urban rivers, and existing habitats are jeopardized by future uncertainties in water resources management and climate. The Los Angeles River (LAR), for example, is a highly modified system that has been mostly channelized for flood control purposes, has altered hydrologic and hydraulic conditions, and is thermally altered (warmed), which severely limits the habitat suitability for cold water fish species. Efforts are currently underway to provide suitable environmental flows and improve channel hydraulic conditions, such as depth and velocity, for adult fish migration from the Pacific Ocean to upstream spawning areas. However, the thermal responses of restoration alternatives for resident and migrating cold water fish have not been fully investigated. Using a mechanistic model, we simulated the LAR’s water temperature under baseline conditions and future alternative restoration scenarios for migration of the native, anadromous steelhead trout in Southern California and the historically resident Santa Ana sucker. We considered three scenarios: 1) increasing roughness of the low-flow channel, 2) increasing the depth and width of the low-flow channel, and 3) allowing subsurface inflow to the river at a soft bottom reach in the LA downtown area. Our analysis indicates that the maximum weekly average temperature (MaxWAT) in the baseline condition was 28.9°C, suggesting that the current river temperatures would act as a limiting factor during the steelhead migration season and habitat for Santa Ana sucker. The MaxWAT dropped about 3%–28°C after applying all the considered scenarios at the study site, which is 3°C higher than the determined steelhead survival threshold. Our simulations suggest that without consideration of thermal restoration, restoring hydraulic conditions may be insufficient to support cold water fish migration or year-round resident native fish populations, particularly with potential river temperature increases due to climate change.
1 Introduction
Like many urban rivers, the Los Angeles River (LAR) is experiencing a renaissance and is now viewed as a valuable ecosystem to be restored as a community amenity as opposed to the old paradigm where it was considered a source of flooding to be controlled and a conveyance for treated wastewater to the ocean (Beach, 2001; Everard and Moggridge, 2012). Given that the LAR flows through one of the largest and most urbanized cities in the United States, complete restoration to an undisturbed condition is not achievable through reclamation efforts. Instead, the overall goal is to improve ecological function through targeted remediation efforts to provide a more ecologically dynamic state (Bernhardt and Palmer, 2007). This aligns with similar projects across the world where there is an integrated and pragmatic approach to urban river restoration to improve biodiversity and achieve overall ecosystem function and resilience (Palmer et al., 2005; Smith et al., 2014; Chou, 2016).
Identifying an ecological endpoint is one of the standards for successful river restoration (Palmer et al., 2005; Zhang et al., 2018; O’Brien et al., 2020). The anadromous steelhead trout (Oncorhynchus mykiss) is classified as endangered in southern California (U.S. Department of Commerce, 1997). For the LAR, improving river connectivity for steelhead from the sea to their native spawning grounds in southern California is a priority ecological endpoint (City of Los Angeles, 2007). Connecting urban rivers to healthy reaches can be successful as flow and sediment are more likely to be in balance and the fish can exploit new areas (Findley and Taylor, 2006). In addition to meeting the trout’s physical habitat requirements, because fish are ectotherms, water temperature must be within a defined thermal range for migrating fish to survive. Stream temperature influences the distribution of fish, food availability, body growth, movement, fecundity, and spawning success (Caissie, 2006).
In this study, we assessed how physical restoration scenarios focused on improving connectivity will alter stream temperatures to better support migrating steelhead. To do so, we evaluated how restoration measures within the LAR may improve stream temperature during steelhead migration and support other native fish habitat from January through June which is primarily the migration season in southern California (Moyle et al., 2008). Stream temperature is a function of flow, depth, velocity, and substrate connections, all of which may be altered during the LAR stream restoration. Gu and Li (2002) found that the sensitivity of stream temperature to river flow rate is as significant as that to weather. Therefore, the other physical parameters that are controlled by flow (i.e., depth and velocity) also affect water temperature. Furthermore, water temperature in riverine systems within highly urbanized areas can be elevated through modifications in riparian landcover (by affecting the shading on the water surface), as well as surface and subsurface inflows (LeBlanc et al., 1997; Chen et al., 1998; Van Buren et al., 2000; Sridhar et al., 2004; Herb et al., 2009; Sun et al., 2015; Abdi and Endreny, 2019). Like other similar projects, remediation of the LAR has the best chance of success if efforts improve ecological function and the river can support self-sustaining populations (Palmer et al., 2005).
Fixing hydrologic and hydraulic conditions of urban rivers through physical modification is often the focus of river restoration projects (Barber and Gleason, 2017), yet seldom do restoration efforts look at how stream temperature can be improved for native fish. River temperature is a critical factor in riverine networks, as it controls the saturation of dissolved oxygen (Sand-Jensen and Pedersen, 2005; Null et al., 2017). In addition, while many rehabilitation projects do concentrate on improving water quality to address water contamination, stream temperature is often overlooked (Purcell et al., 2002; Walsh et al., 2005; Pander and Geist 2013). Rivers are often highly thermally polluted due to industrial discharges (e.g., thermoelectric power plants return flows; Madden et al., 2013) or due to associated land-use change e.g., deforestation and urbanization; (Parker and Krenkel, 1969; Wunderlich, 1972; Walsh et al., 2005; Poshtiri and Pal, 2016; Rogers et al., 2021). Increasing air temperatures from climate change are expected to increase river temperatures as well (Eaton and Scheller, 1996).
In addition to air temperature, substrate inflow as groundwater and hyporheic exchange regulates river water temperature during wet and dry weather (Risley et al., 2010; Kurylyk et al., 2016), which depending on site conditions (e.g., hard or soft bottom, and urbanized or forested area) and seasonality may vary (Poole and Berman, 2001). The upwelling in the LAR is important due to the condition of the river however, the hyporheic exchange inflow is negligible due to hardening of the floodplain (Paulinski et al., 2021). Further, riparian zone shade effects from tree canopy, hillslope, and buildings are also factors reducing river water temperature by providing terrestrial-based reduction in direct and diffuse solar radiation and the view-to-sky factor for the river, which influences longwave radiation (Boyd and Kasper, 2003). Recent advances in temperature modeling now provide the tools to explore how managing flows and riparian shading can influence thermal conditions within desired migration corridors.
This study aims to assess the river temperature condition for steelhead migration on the LAR mainstem and evaluate the cooling or warming effects of potential restoration scenarios. The applied restoration scenarios are suggested by the United States Bureau of Reclamation (U.S. Bureau of Reclamation, 2019): 1) increasing roughness of the low-flow channel (see Supplementary Figure S11 for an example of a low-flow channel) to reduce velocity, 2) increasing the depth and width of the low-flow channel in addition to increasing the roughness, and 3) applying additional subsurface upwelling to Scenario 2. Our central research questions are: 1) to what degree does river temperature limit steelhead migration in LAR mainstem from the Pacific Ocean to the soft bottom section of the LAR (Glendale Narrows)? 2) how does river temperature respond to restoration scenarios to facilitate steelhead migration? and 3) how would simulated thermal changes limit the year-round resident native fish in alternative restoration scenarios? Modeling results could provide a better understanding of the role of water temperature as a limiting factor for steelhead migration in the LAR. Our study had two hypotheses: 1) that warm water temperature is a limiting factor for cold water migrating fish in LAR and 2) the proposed USBR restoration actions cannot address the temperature problems for both species. Findings from this work will provide the LAR water managers with a more holistic understanding of the capabilities and consequences of river restoration alternatives.
2 Methods
We estimated the optimum thermal suitability ranges in the determined river reach of the LAR based on the desirable thermal condition for steelhead (see section 2.3). We then simulated the water temperature for current conditions and under the restoration alternatives for the LAR based on the considered thermal metrics.
2.1 Study Area
We evaluated the thermal impacts of the alternative scenarios that were originally proposed by the United States Department of the Interior Bureau of Reclamation (U.S. Bureau of Reclamation, 2019) for steelhead migration on the LAR. The study area is an approximately 19.6 km reach of the LAR, from the confluence with Arroyo Seco tributary to the confluence with the Rio Hondo (Figure 1). The selected study reach overlaps with the domain considered in other restoration analyses of the river system (U.S. Bureau of Reclamation, 2019; Reaches 7 and 8 in; U.S. Army Corps of Engineers, 2016).
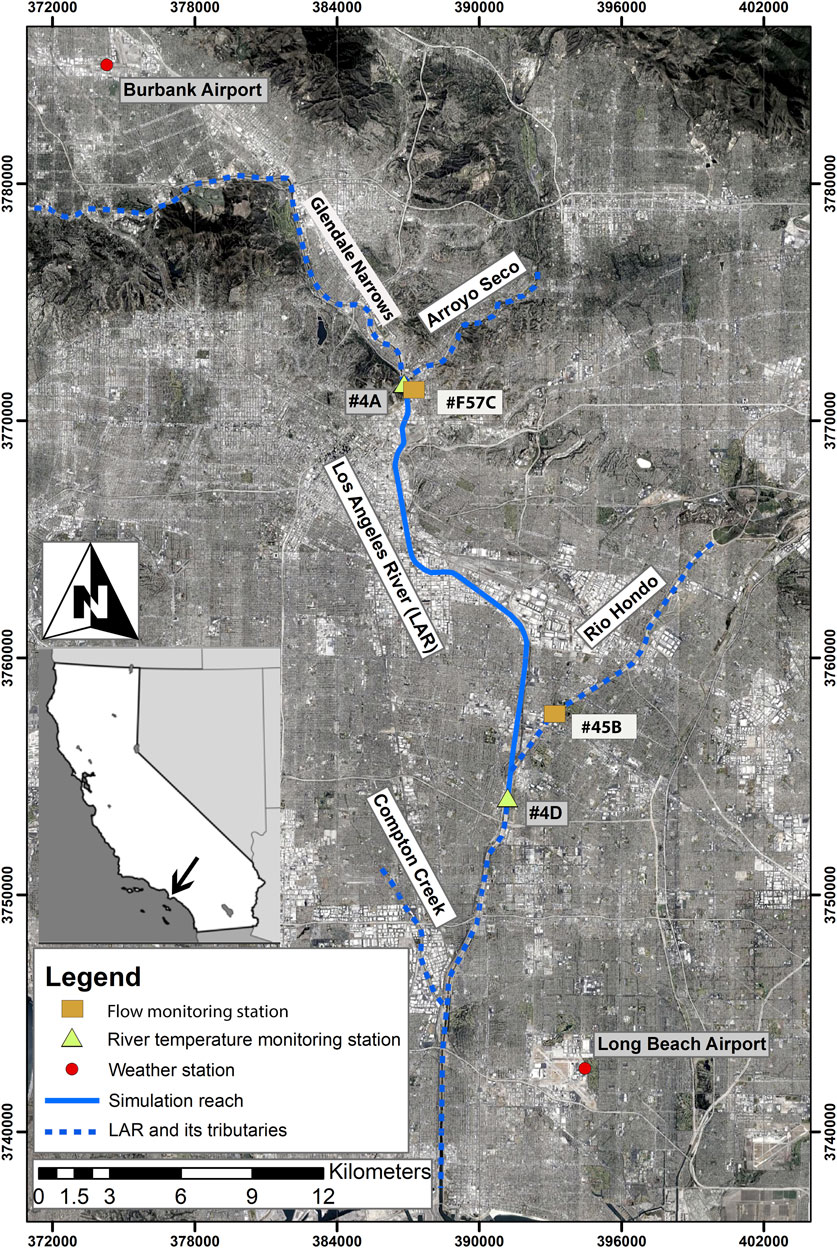
FIGURE 1. Study area on LAR’s mainstem. The figure includes the weather stations and river temperature monitoring stations as well the flow monitoring stations that are represented by the arrows The inset with an arrow shows the site location within the state of California, United States.
The study reach drains a 1,270 km2 area located entirely within the alluvial, coastal LAR watershed. The LAR watershed has a mild semi-arid Mediterranean climate with seasonal precipitation occurring primarily in the winter months (October through March). The study reach includes the physical and thermal contributions of the Arroyo Seco tributary and effluent from three water reclamation plants that discharge to the LAR upstream of the channel. Flows within the river are primarily wastewater-dominated, particularly in the summer months, when the three water reclamation plants collectively contribute over 70% of the total river flow (Stein, et al., 2021b). The water reclamation plants are upstream of the study reach and their influence on the water temperature is already captured by the upstream river temperature boundary condition. The mainstem of the LAR is primarily concrete-lined for flood control purposes (Mika et al., 2017; Read et al., 2019) except for a 17.7 km reach in the Glendale Narrows, a 3.9 km reach upstream of Sepulveda Dam, and the estuary (Figure 1). The hard-bottom section of the river is armored with uniform geometry to expedite stormwater removal and provide flood protection. The area under study is notable for its channelized and mostly trapezoidal cross-section form, gray concrete armoring, lack of subsurface inflows due to groundwater upwelling, and absence of riffle-pool bedform morphology that could provide thermal refugia, and lack of riparian vegetation.
Discharge within the LAR mainstem and tributaries is heavily managed through dams and reservoirs, distributed stormwater capture systems, spreading grounds, and water reclamation facilities. The hydrology within the watershed is constantly changing due to the complexity of the system, the need to balance existing water supplies, and the uncertainty of climate change impacts. For example, municipalities within Los Angeles are seeking to reuse wastewater for water supply, which would have significant impacts on hydrologic and hydraulic conditions in the effluent-dominated LAR. Since wastewater effluent is typically warm, an increase in discharge may elevate river temperatures outside the thermal tolerance range for some fish; a decrease in discharge may also have temperature effects. In the study herein, however, we focus on the impacts of restoration alternatives on river temperature under existing hydrologic conditions to isolate their effects.
Migrating steelhead were present in the LAR until 1940 when urbanization began to rapidly increase. Changes to velocity, depth, temperature, and refuge habitat are all considered to be contributing factors to migration no longer occurring. Although migration has not been observed since then, resident populations still exist in the upper watershed tributaries (Stein et al., 2020). Current restoration efforts are aimed at creating conditions along the mainstem that would once again allow migration to occur between the ocean and the extant upper watershed populations. High temperatures are considered one of the key limiting factors for steelhead migration under current conditions (Stillwater Sciences, 2020). Exposure to high temperatures can result in acute and chronic stress for migrating adults, which can lead to secondary stress effects such as increased energy use, immunosuppression, depressed reproductive maturation, and overall could reduce growth and reproductive fitness and lead to mortality if exposure is prolonged i.e., 7 days; (Myrick and Cech, 2000; A. Myrick and Cech, 2005; Boughton et al., 2015; Stillwater Sciences, 2020).
2.2 Model Setup
We used a one-dimensional hydraulic model, HEC-RAS (U.S. Army Corps of Engineers, 2016), under steady-state conditions to calculate water surface profile data coupled with the i-Tree Cool River model (Abdi and Endreny, 2019; Abdi et al., 2020b) to simulate water temperature. The HEC-RAS model was a previously-created hydraulic model of the LAR, as documented in Stein et al. (2021a.) Briefly, the model was compiled from various sources and channel geometry was validated with LiDAR data, as-builts, and Google Earth, to confirm that low-flow channel geometry was correct (U.S. Army Corps of Engineers, 2004; Environmental Science Associates, 2018; HDR CDM, 2011; U.S. Army Corps of Engineers, 2005). The final hydraulic model used in this study was from station #4A through station #4D (Figure 1) with a length of almost 19.6 km and 440 cross sections.
We applied the Arroyo Seco tributary inflows to the main channel in HEC-RAS to generate depth and velocity data to evaluate migration feasibility (i.e., minimum depth and max velocity to support migration) as well as seven other hydraulic parameters including cross-sectional distances, flow, minimum channel elevation, and water surface elevation to calculate the water column depth, top width, flow area, and wetted perimeter. These hydraulic parameters were used as inputs to the i-Tree Cool River model. The one-dimensional steady flow component in HEC-RAS uses the standard step method for the solution of steady gradually varied flow (Chow, 1959). The i-Tree Cool River model applies the standard advection, dispersion, reaction equation to the water surface profile outputs, generated by HEC-RAS, to simulate river water temperature (Abdi et al., 2020a).
2.3 Ecological Metrics
Our focus was to evaluate the thermal impacts of alternative restoration scenarios on native year-round resident fish populations and steelhead migration from the estuary to the soft bottom habitat in the Glendale Narrows. From the Glendale Narrows the anadromous trout can reach potential spawning grounds in upper tributaries. The restoration scenarios will also improve physical habitat for other native fish that could be reintroduced such as the endangered Santa Ana sucker (Catastomus santanae; U.S. Fish and Wildlife Service, 2000; U.S. Fish and Wildlife Service, 2011).
Steelhead are anadromous and migrate into freshwater to spawn between the middle of January to the middle of June each year in California (Santa Ynez River Technical Advisory Committee (SYRTAC), 2000), which generally coincides with high flows in the LAR. The steelhead are a large-bodied fish; adults average 721 mm in length across their native range (Quinn, 2018). A minimum depth of 0.3 m (1 ft) of water is needed for the adult migrating trout to swim up the river, while greater depths closer to 0.6 m (2 ft) are required for adults to rest periodically during migration (U.S. Bureau of Reclamation, 2019). They are strong swimmers, capable of swimming 1.5–3 m/s for prolonged distances and 4–8 m/s for burst speed (U.S. Bureau of Reclamation, 2019). When migrating to spawning grounds, the trout also need locations to rest and recover at speeds below 1.5 m/s. The goal of the planned river restoration is to provide habitat and hydraulic conditions that are passable by migrating adult trout to return to spawning grounds upstream of the study reach. While the planned river restoration considers stream velocities and depths appropriate for steelhead, it overlooks temperature as a limiting factor. Steelhead are cold water stenotherms that cannot survive in water above 25–30°C (Hokanson et al., 1977; Myrick and Cech, 2000; A. Myrick and Cech, 2005).
Other smaller-bodied native fish species remain in freshwater year-round, inhabiting the LAR during the high flows when steelhead are migrating and during the low-flows in the summer. Other native fish considered in this analysis include the threatened Santa Ana sucker, a small (16 cm) fish that prefers low to mid gradient streams with coarse substrate, a minimum depth of 40 cm, and temperatures below 22°C (U.S. Fish and Wildlife Service, 2011; Haglund et al., 2001; Haglund and Baskin, 2003). Different studies have observed mortality of Santa Ana sucker at temperatures between 22 and 32.8°C, we therefore used 22°C as their critical thermal maximum (Moyle, 2002; U.S. Fish and Wildlife Service, 2011).
The temperature threshold for Steelhead ranges from 24–32°C (Lee and Rinne, 1980; Myrick and Cech, 2000; Sloat and Osterback, 2013; Spina, 2007 and references therein) under lab conditions, where loss of equilibrium or death occurs within 7 days, through direct mortality, or indirect mortality from impairment of function. Steelhead temperature preference has been reported between 17.8–24.6°C (Verhille et al., 2016), however, optimum swimming speed has been documented as 14–15°C (Myrick and Cech, 2000), temperatures higher than the optimum may hinder swimming ability making it more challenging for fish to swim against the velocities considered in restoration. A barrier to migration has been estimated at 21–24°C (Stabler, 1981; Washington State Department of Ecology (WDOE), 2002), at which point individuals will start expressing avoidance behavior by sheltering in cooler tributaries, refusing to migrate, or migrating back downstream (McCullough et al., 2001). The critical thermal maxima for Steelhead, wherein fish lose equilibria after 24 h of exposure, has been observed to be 25°C (Myrick and Cech, 2000). While prolonged exposure, i.e., 7 days at 21°C, however, can lead to mortality, therefore the U.S. Environmental Protection Agency (USEPA) (2003) recommends a maximum weekly maximum temperature (MWMT) of 20°C for migratory corridors.
Santa Ana Sucker have been documented in large temperature ranges from 8 to 26°C (Saiki et al., 2007) but are typically found in temperatures below 22°C (Moyle, 2002). Limited information describing tolerances to water temperature is available, however, mortality has occurred at elevated water temperatures, i.e., 27–33°C (San Marino Environmental Associates (SMEA), 2010; U.S. Fish and Wildlife Service, 2014), and their physical condition has been noted to worsen in average temperatures of 19.3°C (range 14.4 – 25.9°C, Saiki et al., 2007).
To evaluate whether the proposed restoration scenarios would provide thermal habitat to support the native fish species in the migration season, we used observed and modeled data to calculate thermal metrics to compare to fishes critical thermal maximas. Temperatures can be limiting to fish in two ways, exceeding maximums over short term exposures can lead to death while exceeding optimal weekly average temperatures can reduce survivability by inducing avoidance behavior that could impact migration success (McCullough et al., 2001), increasing metabolic costs that can impact viability of eggs (Sauter et al., 2001), and causing impairment. The first metric, the Maximum Weekly Maximum Temperature (MaxWMT) is the 7 days moving average of daily maximum temperatures. The second is the Maximum Weekly Average Temperature (MaxWAT), defined as the 7 days moving average of daily mean temperatures (Table 1). The third thermal metric is the Minimum Weekly Minimum Temperature (MinWMT), which is the 7-days moving minimum of daily minimum temperatures. We compared these calculated metrics from the observed and modeled data to the critical thermal maxima of each of the fishes of interest in this study.

TABLE 1. River temperature metrics used to evaluate the model results from different restoration scenarios in terms of fish thermal habitat suitability.
2.4 Input Data and Scenarios
2.4.1 Station #4A: Upstream Boundary Condition
River temperature monitoring station #4A, immediately downstream of the LAR and Arroyo Seco tributary confluence, was the boundary condition for the simulations (Figure 1). hourly observed water temperature data at this station was provided by Mongolo et al. (2017) for the dry season (i.e., with no storm event) in June 10–July 18, 2016 but not the migration season (February 1–May 31). Continuous river temperature data is rare on the LAR; however, single layer or multilayer regression relationships have been used to estimate water temperatures (Mohseni et al., 1998; Caissie, 2006; Neitsch et al., 2011). To get the river temperature at the upstream boundary condition for the desired migration season (February 1–May 31, 2016), we trained a multilayer linear regression machine learning (ML) algorithm (Murtagh, 1991; Pedregosa et al., 2011) on Google’s TensorFlow model version 2.3.1 (Abadi et al., 2015) using Keras artificial neural network (ANN) library (Chollet et al., 2015) on Python 3. We used the observed hourly river temperature data at LAR station #4A 936 observations, (Mongolo et al., 2017) as the dependent variable for the model training and testing. We used hourly weather data, including air temperature, wind speed, station pressure, and relative humidity as the independent variables as the predictive features. We obtained the weather data for training the model for station #4A from Burbank Airport weather station for the same time window (Supplementary Table S1).
After data gathering, cleaning2 and organizing, we used the available observed river temperature data for June 10–July 18, 2016 for our ML algorithm and used 0.6, 0.2, and 0.2 ratios for the training, validating, and testing phases, respectively. Mean absolute error (MAE) was used as the target error optimization parameter. The MAE decreased to 1.1°C after 100 iterations. The R2 for the testing process was 0.78 with a p-value of 0.202 (>α = 0.05) for a two-sample t-test, showing that there was no significant difference between the observed and predicted river temperatures.
By applying the observed weather data in the migration season (February 1–May 31, 2016; see Supplementary Table S2 for more statistical details) on the trained ML algorithm, we predicted the upstream water temperature boundary condition for the i-Tree Cool River model. Supplementary Table S3 shows the statistical properties of the prediction and Supplementary Figure S2A demonstrates the scatter plot between the observed air temperatures and predicted river water temperatures. Based on the predictions from our trained algorithm, the water temperature in migration season had an average of 23.7°C with 25th and 75th percentiles of 22.0°C and 25.4°C respectively, and a standard deviation of 2.5°C (Supplementary Table S3).
2.4.2 Station #4D: Downstream Control Point
We used the observed water temperature data provided by Mongolo et al. (2017) to determine river water temperature at the downstream boundary, station #4D (Figure 1). We trained a separate multilayer linear regression ML algorithm for station #4D to predict water temperature in the migration season (February 1–May 31, 2016). We used the observed hourly river temperature data on LAR stations #4D (Mongolo et al., 2017) as the dependent variables for the ML algorithm and similar independent features (air temperature, wind speed, station pressure, and relative humidity) obtained from Long Beach Airport weather station (Supplementary Table S1).
After data cleaning, for June 10–July 18, 2016, the ML algorithm with 0.6, 0.2, and 0.2 ratios for the training, validating, and testing phases respectively was applied to the station #4D dataset. The MAE was 2.5°C after 100 iterations (Supplementary Table S1). The R2 for the testing process was 0.68 with a p-value of 0.16 (>α = 0.05) for a two-sample t-test, showing that there was no significant difference between the observed and predicted river temperatures.
Using the trained ML algorithm, we predicted water temperature at station #4D in the migration season (February 1–May 31, 2016) using weather data from the Long Beach Airport weather station (see Supplementary Table S2) as the independent variables. The observed air temperature and predicted water temperature showed a similar pattern on variations in the migration season (Supplementary Figure S2B) and as shown in Supplementary Table S3, the predicted water temperature, had an average of 21.2°C with a 25th and 75th percentiles of 17.6°C and 24.7°C respectively, and a standard deviation of 5.2°C. As seen in Supplementary Tables S1, S2, S3, the observed air and water temperatures support the variation of predicted water temperature in stations #4A and #4D.
2.4.3 Input Data for Simulations
To check the accuracy of the simulated river temperatures along the LAR in the study reach, we calibrated and validated the i-Tree Cool River model for the migration season (February 1–May 31, 2016). For this study, we simulated hourly water temperatures using the i-Tree Cool River model. We used hourly weather data obtained from the Burbank Airport weather station as was used in the ML model training procedure. For the direct and diffuse shortwave radiations, we used hourly data from National Renewable Energy Laboratory’s National Solar Radiation Database NREL NSRDB; (Sengupta et al., 2018) for the station location on the LAR. We obtained solar radiation data from NREL’s NSRDB (station ID #83948 located at 34.09N, 118.22 W). In the simulation period, the average air temperature was 17.1°C and the average relative humidity was 53.9% (Supplementary Table S2).
According to Risley et al. (2010), we considered the long-term observed flow data for the flow gaging stations in the study area during the simulation time frame (February to May from 1985 to present). We used the observed flow data from the LA County stations #F57C for the LAR mainstem and #45B for the Rio Hondo tributary (Figure 1). Based on the assumption of 50% exceedance probability and assuming steady state for the simulations (Stein et al., 2021a), we assumed a constant flow of 3.74 m3/s (132 ft3/s) in the LAR mainstem and 0.03 m3/s (0.9 ft3/s) in the Rio Hondo tributary.
Using the calculated flow values from the observed data, we ran the HEC-RAS (U.S. Army Corps of Engineers, 2011) model in steady-state to get the water profile data for the cross-sections, including cross-sectional distances, flow, minimum channel elevation, and water surface elevation to calculate the water column depth, velocity in the channel, top width, flow area, and wetted perimeter. The average river water depth for the LAR in the study period was about 26 cm, the average water velocity was 0.8 m/s, and the average cross-sectional water level area was 5.9 m2. The i-Tree Cool River model uses an internal linear interpolation function to resample the HEC-RAS cross-sectional outputs to refine the spacing of cross-sections to 100 m and applies the spatial variation channel data and riparian features to simulate the river temperature (Abdi and Endreny, 2019; Abdi R. et al., 2021). Due to the bare concrete bed and lack of riparian shading in the river reach, we considered no subsurface inflow and no shading effect in the simulations.
2.4.4 Restoration Scenarios
Based on Manning’s equation, at low-flow values (less than about 5.7 m3/s (200 ft3/s)) a deepened and roughened low-flow channel could provide the minimum depth requirements and velocities suitable for resting and migration of steelhead in the LAR (U.S. Bureau of Reclamation, 2019). To do so, the underpinning concept of the fish passage design for the LAR is to increase the depth, width, and roughness of a low-flow channel that would fit within the larger concrete flood control channel and could accommodate the large-bodied trout (Fryirs and Brierley, 2000; U.S. Bureau of Reclamation, 2019).
Even low flows in the LAR tend to occur near critical depth (U.S. Bureau of Reclamation, 2019), meaning that increasing Manning’s roughness within the low-flow channel could increase the depth and reduce velocity to provide a passable condition without exhausting the trout during migration and allow the sucker viable habitat. For the first management scenario, we increased the roughness without changing the channel geometry. The Manning’s roughness coefficient in the baseline condition within the concrete bed material was 0.017 and as suggested by the U.S. Bureau of Reclamation (2019), we assumed that the low-flow channel roughness of the design concepts would be equivalent to that of a natural gravel or cobble bed stream with a Manning’s n-value of 0.035 (two times larger).
For the second scenario, in addition to changing the roughness, we modified the channel geometry to reach the desired ranges of depth and velocity. Based on the U.S. Bureau of Reclamation (2019), we applied a design flow of 8.5 m3/s (300 ft3/s) for the low-flow channel capacity, which corresponds to the 10 percentile of the annual exceedance for mean daily flows during the 1985 to 2017 period. Increasing flows in the low-flow channel area would improve habitat conditions but when the low-flow channel capacity is exceeded, habitat conditions decline (U.S. Bureau of Reclamation, 2019). As a result, we selected 8.5 m3/s (300 ft3/s) as the optimal design flow to balance habitat at base flow and higher flows. For the design flow, assuming a uniform trapezoidal low-flow channel, we used the top width of 20 m (65 ft) and the depth of 0.6 m (2 ft). We considered the cross-sectional design as the starting point for the design of the alternative management scenario and adjusted the mentioned values for the HEC-RAS cross-sections. We also assumed that the top elevation of the designed low-flow channel designs matches the elevation of the existing concrete near the channel center and excavating a wider and deeper.
The alternative design of the low-flow channel would require demolishing a portion of the existing concrete near the channel center and excavating a wider and deeper low-flow channel, which would also allow for subsurface upwelling. Groundwater in the basin is intensively managed for water supply and subsurface water quality reasons (Upper Los Angeles River Area Wastewater (ULARA), 2019). An estimate of groundwater upwelling was provided by the Los Angeles Department of Water and Power (LADWP) at a constant rate of 3,000 acre-ft/yr, or approximately 0.117 m3/s (4.14 ft3/s). We distributed the estimated upwelling over the simulation reach with a constant temperature, slightly adjusted, based on annual average air temperature at the Burbank Airport weather station (18.7°C) as suggested by Glose et al. (2017) and Abdi et al. (2020a).
To simulate the thermal impacts of the alternative restoration scenarios for the LAR downstream of the Glendale Narrows permeable soft bottom reach, we calibrated and validated the mechanistic river temperature model for the migration season under baseline conditions using the considered control point (station #4D). In the baseline condition thermal simulations, we used the HEC-RAS modeling’s outputs for steady-state conditions in the migration season. Applying values for the 440 cross-sections from the HEC-RAS model setup, we calibrated the temperature model based on solar radiation data and substrate temperature. In the calibration period (February 1–April 30, 2016), the coefficient of determination was 0.75 with and Nash Sutcliffe Efficiency (NSE) of 0.69 (Supplementary Figure S3A). In the validation period (May 1–31, 2016), the coefficient of determination was 0.66 and the NSE was 0.59 (Supplementary Figure S3B).
3 Results and Discussion
Results from baseline condition simulations, and under potential restoration scenarios, showed that during migration season, baseline thermal conditions would not support the steelhead or resident Santa Ana sucker. Even with restoration of hydraulic conditions, temperatures would exceed their optimal thermal maxima. Water temperature should therefore be considered a limiting factor in facilitating steelhead migration on the LAR or establishing Santa Ana sucker populations. On average, water temperature was about 4°C higher than the fish’s threshold (25°C). Even though management scenarios could improve physical conditions, other plans should be considered to reach the desired temperature thresholds.
The ML-based predictions of river temperature in the migration season for upstream and downstream of the study area showed that the calculated thermal metrics, MaxWMT and MaxWAT, exceeded the recommended 20°C MaxWMT for fish corridors and critical maxima survival threshold of 25°C for the steelhead, which is also above the 22°C maxima for the sucker (Figure 2). The median of MaxWMTs was 28.9°C at station #4A and reached 33.6°C at station #4D, an increase of 16% over baseline condition. The median of MaxWATs was 27.2°C at station #4A and reached 28.2°C at station #4D, an increase of 4%. We observed a 30% decrease in the median of the calculated MinWMTs from 20.1°C to 13.7°C. The 16% increase and a 30% decrease in the median of MaxWMTs and MinWMTs metrics respectively, showed that the diel variations of the water temperature at the downstream station were broader compared to at the upstream station. One explanation could be that the upstream station is in a soft bottom portion of the river while the downstream station is after 20 km of bare concrete channel, which can increase water temperature (Sun et al., 2016).
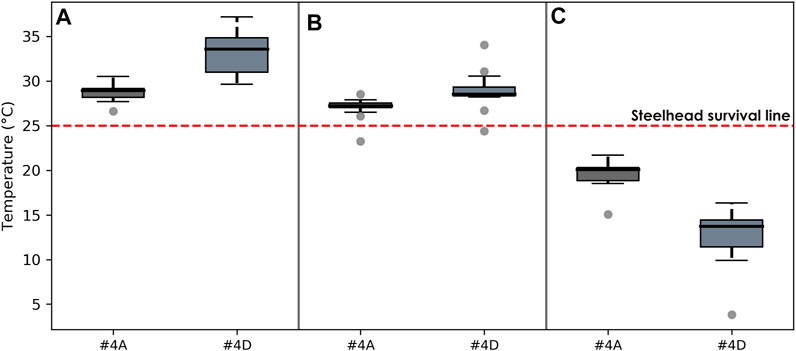
FIGURE 2. Boxplots show the variation of the calculated thermal metrics: MaxWMT (A), MaxWAT (B), and MinWMT (C) for the predicted water temperatures during the migration season in 2016 (February 1–May 31, 2016) for the upstream at station #4A and downstream at station #4D. The red dashed line shows the maximum temperature a steelhead could tolerate.
By increasing the Manning’s roughness in the low-flow channel from 0.017 to 0.035 (Scenario 1), the average cross-sectional water column depth increased by 50% to 39 cm and the average flow velocity decreased by 55% to 0.44 m/s (see Figure 3; Supplementary Table S4 for more details). Under this scenario, the water profile in the river channel was elevated due to the increased Manning’s roughness coefficient, and the estimated average diel change in the river temperature, based on the defined thermal metrics (MaxWMT-MinWMT), decreased by 30% from 20.6°C to 14.5°C. The average MaxWMT in the simulated migration season for Scenario 1 decreased by 2.3°C–31.1°C (7% compared to the baseline condition) and the average MinWMT increased by 3.8°C–16.6°C (29% compared to the baseline condition; Figure 4). The average MaxWAT didn’t change significantly compared to the other two metrics and decreased by only 0.1°C–28.8°C (0.3% compared to the baseline condition) and it was 3.8°C higher than the determined steelhead survival line (Table 2). As reported by Smith and Lavis (1975) and Ahmadi-Nedushan et al. (2007), the decrease and increase in the average MaxWMT and MinWMT, respectively, demonstrates that the relative change on water temperature occurs primarily through the associated impact of increase/decrease of the water column depth, which is also connected to the increased/decreased thermal inertia of the river.
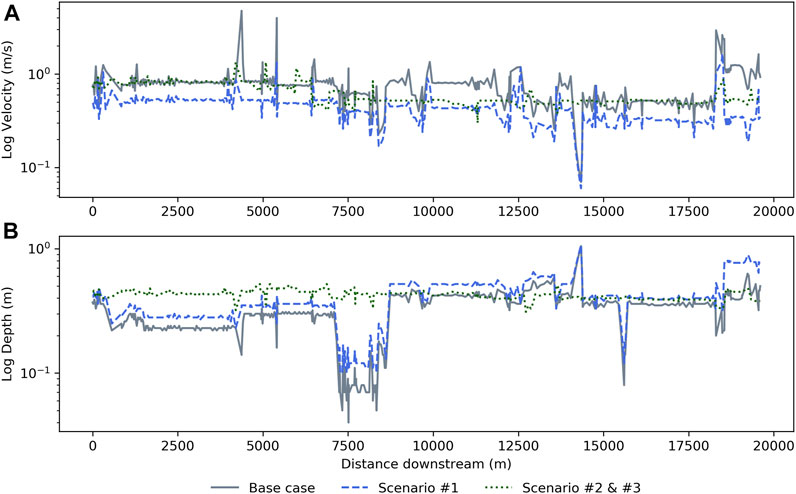
FIGURE 3. Variation of the cross-sectional velocity (A) and depth (B) in the base case condition and under determined management scenarios. The y-axis in both panels is in log format.
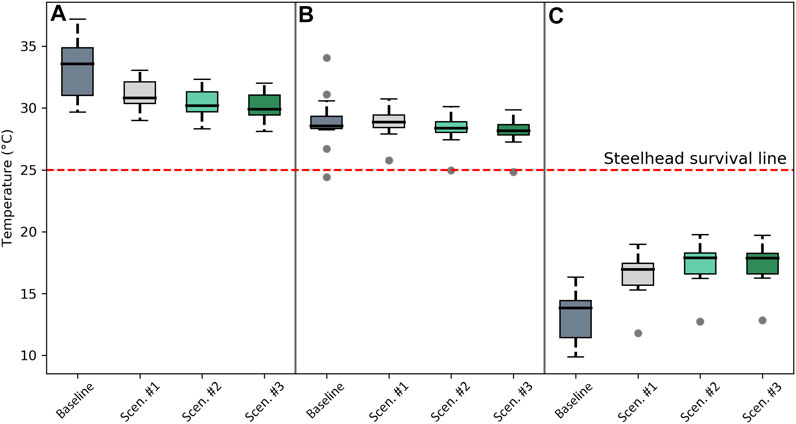
FIGURE 4. Boxplots showing the variation of the thermal metrices for the base case condition and under 3 applied scenarios. The figure shows the variation of three defined metrics MaxWMT (A), MaxWAT (B), and MinWMT (C) for the simulations in the migration season (February 1–May 31, 2016). The red dashed line shows the maximum temperature a steelhead could tolerate.

TABLE 2. Average thermal metrics of the river temperature (°C) in the downstream control station #4D for the baseline condition and under the alternative scenarios during the migration season (February 1–May 31, 2016).
Updating the cross-sections in the study area, in addition to increasing the Manning’s roughness (Scenario 2), caused an increase of 17 cm in the average flow depth compared to the baseline case (from 26 to 43 cm), and a 20% decrease in the average velocity (from 0.79 m/s cm to 0.63 m/s cm; Figure 3; Supplementary Table S4). The increase of flow depth starting at station 7,500 m for the next 1 km was more pronounced by about 40 cm. This increase has the potential to provide thermal refuge for the steelhead or sucker, however, the average MaxWAT in this reach was 28.1 C so we were not able to support this claim. Executing Scenario 2 demonstrated the same pattern of changes in the average MaxWMT and MinWMT that was noted for Scenario 1 (Table 2). The difference between the average MaxWMT and MinWMT for Scenario 2 was 12.9°C, which was 37% lower than the same difference for the base case and 11% lower than Scenario 1. A smaller difference between these thermal factors under Scenario 2 indicates that deeper in the water column caused fewer fluctuations in the diel river water temperatures as reported by Gu et al. (1999). The average MaxWMT for Scenario 2 dropped to 30.4°C, demonstrating a 9 and 2.2% decrease compared to the baseline case and Scenario 1, respectively. The average MinWMT for Scenario 2 increased to 17.5°C which was 36.7 and 5.4% higher than the base case and Scenario 1, respectively (see Figure 4). The average MaxWAT also was 28.3°C which, even though it was 0.6°C lower than the base case condition similar to Scenario 1, was higher than the considered steelhead survival line (by 3.3°C; Table 2).
Under Scenario 3, including groundwater upwelling in the study area, the average MaxWMT and MaxWAT values for the simulation period were 30.1°C and 28°C (Table 2). Although Scenario 3 provided the lowest values for the higher ranges of the determined thermal metrics, they were still higher than the desired temperature range for the steelhead migration and resident sucker (Figure 4; Table 2). Subsurface inflow temperature and volume parameters are typically sensitive parameters that affect river water temperature significantly (Abdi and Endreny, 2019; Abdi et al., 2020b). By studying the Shasta River tributary in northern California, Nichols et al. (2014) showed that subsurface upwelling inflows could act as reservoir releases to decrease water temperature. Loheide and Gorelick (2006) noted the importance of hyporheic exchange, as an important form of subsurface inflows in Cottonwood Creek in northern California during summer dry weather. They demonstrated that the absence of the subsurface inflows could lead to river temperatures warming in the downstream direction. In winter, Risley et al. (2010) and Kurylyk et al. (2016) documented that groundwater temperature could also contribute a warming effect where the river water temperature is typically below subsurface water temperatures. During the summer, in the LAR, Abdi et al. (2020a) simulated a similar phenomenon of groundwater cooling by redirecting warm surface inflows to infiltration via constructed riffles and pools, which then entered the river as cooler groundwater inflows. These findings indicate how pronounced the subsurface inflows are for reaching determined thermal thresholds for steelhead migration feasibility.
Other studies have demonstrated the impact of flow change on water temperature due to the hydraulics of river flow (Gu et al., 1999; Sinokrat and Gulliver, 2010; Abdi B. et al., 2021). Gu and Li (2002) showed that river water temperature’s sensitivity to flow and its properties (in first 20% flow change) is as significant as that to climate data such as air temperature, humidity, and solar radiation. Hockey et al. (1982) studied the variation of water temperature in the Hurunui River in New Zealand and found that for every 1 m3/s reduction in river flow, water temperature increased by 0.1°C. Garner et al. (2017) studied a 1,050 m reach of Girnock Burn River basin in east Scotland and found that for the scenarios with low gradient velocities (0.023 m/s), the water stayed longer within the reach, causing higher maximum and lower minimum temperatures. The findings from Garner et al. (2017) support our results showing the effect of lower water velocities on simulated water temperature due to enhanced heat accumulation and dissipation. However, since we didn’t increase the canopy density in our scenarios, we didn’t get significant changes in water temperatures (Figure 2). Flow velocities in even lower gradients could cause a warming effect in the water temperatures as well, where the residence time would be too high which allows water more time to heat up from solar radiation. Therefore, depending on other factors (riparian shading, upwelling, and inflows), a threshold should be considered for the residence time in the rivers to avoid unwanted warming of the water. Comparing the findings of other studies and our results shows a mixed, sensitive, and uncertain response of water temperature to variation of flow depth and velocity. Therefore, water temperature needs to be considered as a limiting factor based on the results from our simulations and the overall uncertainty of water temperature’s response to changes in depth and velocity.
Ecological restoration scenarios such as riparian shading from tree canopy, cooler substrate temperature (Trimmel et al., 2018; Abdi R. et al., 2021), as well as additional groundwater recharge and hyporheic exchange inflow could be considered to decrease water temperatures (Saha et al., 2017). Sun et al. (2015) simulated river temperature along six separate reaches of Mercer Creek in Washington State and found that tree and hillslope shading reduced the annual maximum temperatures by 4°C. Further, Dbouk (2017) noted that conductive heat transfer approaches and embedding conduit materials with a high thermal conductivity into substrate materials that have a much lower thermal conductivity could be used to act as cooling channels. For subsurface inflows, as suggested by Abdi and Endreny (2019), the relative contribution of groundwater and hyporheic exchange inflow with river water varies by site conditions and seasonality. For the LAR, Abdi et al. (2020a) showed that an 18% groundwater inflow contribution during dry weather in only a 0.5 km reach decreased LAR water temperature by 0.3°C. The need for additional restoration actions will only be exacerbated by climate change (Justice et al., 2017; Merriam and Petty, 2019), which is projected to increase river temperatures in the West (Risley et al., 2010; Rheinheimer et al., 2015).
The baseline temperature conditions of the LAR are not cool enough to support the native Santa Ana sucker nor migrating steelhead. The trout have a thermal maxima of 25°C (Myrick and Cech, 2000; A. Myrick and Cech, 2005) and the sucker’s maxima has been observed to be 22°C (Moyle, 2002). Temperatures at or near the steelhead’s maxima have been observed to be a source of chronic stress in other southern California streams, which may make them vulnerable to other poor water quality conditions and physiological stress (Materna, 2001; Dagit et al., 2009). Warm stream temperatures will also block the migration of salmonids, temperatures above 23°C have prevented steelhead migration in the Northwestern United States (McCullough et al., 2001). The results of our temperature modeling demonstrate that the three restoration scenarios selected for this study do not improve thermal conditions enough in the LAR to support the return of these fishes.
Despite a generally thermally inhospitable environment, thermal refugia can occur in association with cold water patches created from tributaries, groundwater seeps and springs, and shade. Trout have been observed to occupy thermal refugia in streams that exceeded their thermal tolerance where the refugia were 3–8°C colder than ambient stream temperatures (Ebersole et al., 2001). Thermal refugia could be present throughout the LAR, providing a reprieve from warm stream temperatures which would allow trout and sucker populations to survive. However, areas for thermal refugia may be limited due to the primarily engineered nature of the channel. The temperature modeling conducted here did not evaluate fine-scale micro-habitats, instead, the stream temperature was modeled on a reach-scale. Our modeling results suggest that overall, the stream temperatures are not hospitable to the steelhead and Santa Ana sucker even in consideration of proposed restoration alternatives. Support of suitable habitat for resident and migratory fish will require additional measures to ameliorate thermal conditions and allow fish to reach upstream areas with more suitable physical and thermal habitats.
This work may be indicative of other urban rivers, in which restoration alternatives have been proposed to restore physical river parameters but water quality is not explicitly modeled or considered (Wohl et al., 2015). In addition to temperature, cold water fish are sensitive to other pollutants, like metals (Ingersoll and Mebane, 2014; Naddy et al., 2015) and trace organics contaminants (Petrovic et al., 2002), such as those from tire wear (Tian et al., 2021). Water quality stressors, in addition to changing water management regimes and the effects of climate change, should be studied in tandem with optimal hydraulic and temperature parameters for target species. Our work suggests that future studies and management recommendations should consider environmental conditions that are holistically needed to support target species. Further, this work serves as an illustration of the challenge of habitat restoration in urban rivers, given the uncertain climate future.
4 Conclusion
Like most other urban rivers, the LAR is severely impacted by anthropogenic development and urban activities and since it has been channelized and confined, it suffers from a decline in biological habitat and species diversity. Hydraulic conditions in the LAR channel are not suitable for many of the native fish fauna because of shallow depths and high velocities. Several restoration scenarios have been suggested to provide increased flow complexity and habitat heterogeneity within this confined urban stream, such as increasing the roughness of the channel substrate and redesigning the cross-sectional channel area (USBR, 2019). Restoration scenarios could facilitate the migration of the steelhead in the river, specifically targeting the passage from the Pacific Ocean to the upstream Glendale Narrows soft bottom area and upper tributaries. However, previous work focused on improving the depth and velocity for the fish habitat suitability while the thermal condition of the river in the migration season was overlooked.
Our simulations of the baseline condition showed the river temperature was about 4°C higher than the determined threshold for fishes, therefore river temperature in the migration season would not support sustainable migrating steelhead or resident sucker populations despite suitable water column depths and average velocities. Hence, river temperature should be considered as a limiting factor for habitat suitability in facilitating steelhead migration plans in the LAR. Further, after applying the developed restoration scenarios in the study area, our simulations showed that even though the restoration scenarios decreased the 4°C thermal gap, they were still higher than the desired temperature range for the steelhead migration and the resident Santa Ana sucker (about 3°C after applying three considered scenarios combined). This indicates that additional ecological restoration actions, such as shading, should be considered and applied to further decrease the water temperature in the river passage during the migration season and to support year-round resident native fish such as the Santa Ana sucker.
Data Availability Statement
The raw data supporting the conclusions of this article will be made available by the authors, without undue reservation.
Author Contributions
Conceptualization, RA, AR, JW, KQ., and KI; methodology, RA and DP; software, RA; validation, RA and JR; formal analysis, RA; investigation, RA, and AR; resources, RA, AR, JW, KQ, and KI, ES; data curation, RA, KQ; writingoriginal draft preparation, RA; writing review and editing, RA, AR, JW, KQ, KI, ES, TH; visualization, RA; supervision, ES, TH; project administration, ES, TH; funding acquisition, ES, TH.
Funding
The funding was provided through interagency agreements (MOAs) from Los Angeles Department of Water and Power, City of Los Angeles Bureau of Sanitation, Los Angeles County Flood Control District, Los Angeles County Sanitation Districts. Principal funding was provided by the City of Los Angeles, the Los Angeles Department of Water and Power (LADWP), and the Bureau of Sanitation (BoS). Additional funding was provided by Los Angeles County Department of Public Works (LADPW), Los Angeles County Flood Sanitation Districts (LACSD), the Watershed Conservation Authority (WCA), a joint powers authority between the Rivers and Mountains Conservancy (RMC) and the Los Angeles County Flood Control District, and the Mountains Recreation and Conservation Authority (MRCA), a joint power of the Santa Monica Mountains Conservancy, the Conejo Recreation and Park District and the Ranch Simi Recreation and Park District.
Conflict of Interest
The authors declare that the research was conducted in the absence of any commercial or financial relationships that could be construed as a potential conflict of interest.
Publisher’s Note
All claims expressed in this article are solely those of the authors and do not necessarily represent those of their affiliated organizations, or those of the publisher, the editors and the reviewers. Any product that may be evaluated in this article, or claim that may be made by its manufacturer, is not guaranteed or endorsed by the publisher.
Acknowledgments
We thank Rosi Dagit, Resource Conservation District of Santa Monica Mountains, for providing us with the observed temperature data. We thank Elizabeth Jachens, Ph.D., with the United States Geological Survey (USGS), for sharing the groundwater modeling outcomes in the LAR watershed. We thank all members of the Stakeholder Workgroup and the Technical Advisory Group who provided critical input, advice, and review throughout this project. Additional project information is available at https://www.waterboards.ca.gov/water_issues/programs/larflows.html.
Supplementary Material
The Supplementary Material for this article can be found online at: https://www.frontiersin.org/articles/10.3389/fenvs.2021.749085/full#supplementary-material
Footnotes
1Figures and tables with “S” are presented in the supplementary materials.
2Process of preparing the data for the ML algorithms by removing or modifying inaccurate, corrupted, or improperly formatted data (https://www.sisense.com/glossary/data-cleaning/).
References
Abadi, M., Agarwal, A., Barham, P., Brevdo, E., Chen, Z., Citro, C., et al. (2015). TensorFlow: Large-Scale Machine Learning on Heterogeneous Systems. Software available from tensorflow.org.
Abdi, B., Bozorg-Haddad, O., and Chu, X. (2021a). Uncertainty Analysis of Model Inputs in Riverine Water Temperature Simulations. Sci. Rep. 11, 19908. doi:10.1038/s41598-021-99371-0
Abdi, R., Endreny, T., and Nowak, D. (2020a). A Model to Integrate Urban River thermal Cooling in River Restoration. J. Environ. Manage. 258, 110023. doi:10.1016/j.jenvman.2019.110023
Abdi, R., and Endreny, T. A. (2019). Urban River Temperature Modeling of Unsteady Stormwater Inflows and Riparian Shading. Water 11 (5), 1060.
Abdi, R., Endreny, T., and Nowak, D. (2020b). I-Tree Cool River: An Open Source, Freeware Tool to Simulate River Water Temperature Coupled with HEC-RAS. MethodsX 7, 100808. doi:10.1016/j.mex.2020.100808
Abdi, R., Rogers, J. B., Rust, A., Wolfand, J. M., Philippus, D., Taniguchi-QuanRogers, K., et al. (2021b). Simulating the thermal Impact of Substrate Temperature on Ecological Restoration in Shallow Urban Rivers. J. Environ. Manage. 289, 112560. doi:10.1016/j.jenvman.2021.112560
Ahmadi-Nedushan, B., St-Hilaire, A., Ouarda, T. B. M. J., Bilodeau, L., Robichaud, É., Thiémonge, N., et al. (2007). Predicting River Water Temperatures Using Stochastic Models: Case Study of the Moisie River (Québec, Canada). Hydrol. Process. 21, 21–34. doi:10.1002/hyp.6353
A. Myrick, C., and Cech, J. J. (2005). Temperature Effects on Juvenile Anadromous Salmonids in California's central valley: what Don't We Know. Rev. Fish. Biol. Fish. 14 (1), 113–123. doi:10.1007/s11160-004-2739-5
Barber, C., and Gleason, C. J. (2017). Verifying the Prevalence, Properties, and Congruent Hydraulics of at-many-stations Hydraulic Geometry (AMHG) for Rivers in the continental United States. J. Hydrol. 556, 625–633. doi:10.1016/j.jhydrol.2017.11.038
Beach, D. (2001). Coastal Sprawl. The Effects of Urban Design on Aquatic Ecosystems in the United States Pews Ocean Commission. Arlington VA.
Bernhardt, E. S., and Palmer, M. A. (2007). Restoring Streams in an Urbanizing World. Freshw. Biol 52 (4), 738–751. doi:10.1111/j.1365-2427.2006.01718.x
Boughton, D. A., Harrison, L. R., Pike, A. S., Arriaza, J. L., and Mangel, M. (2015). Thermal Potential for Steelhead Life History Expressionin a Southern California Alluvial River. Trans. Am. Fish. Soc. 144, 258–273. doi:10.1080/00028487.2014.986338
Boyd, M., and Kasper, B. (2003). Analytical Methods for Dynamic Open Channel Heat and Mass Transfer: Methodology for Heat Source Model Version 7.0. Portland, OR, USA: Watershed Sciences Inc.
Caissie, D. (2006). The thermal Regime of Rivers: a Review. Freshw. Biol 51 (8), 1389–1406. doi:10.1111/j.1365-2427.2006.01597.x
C. G. Ingersoll, and C. A. Mebane (Editors) (2014). Acute and Chronic Sensitivity of white sturgeon (Acipenser transmontanus) and Rainbow trout (Oncorhynchus mykiss) to Cadmium, Copper, lead, or Zinc in Laboratory Water-Only Exposures (U.S: Geological Survey Scientific Investigations Report 2013–5204), 70.
Chen, Y. D., Carsel, R. F., McCutcheon, S. C., and Nutter, W. L. (1998). Stream Temperature Simulation of Forested Riparian Areas: I. Watershed-Scale Model Development. J. Environ. Eng. 124, 304–315. doi:10.1061/(asce)0733-9372(1998)124:4(304)
Chou, R.-J. (2016). Achieving Successful River Restoration in Dense Urban Areas: Lessons from Taiwan. Sustainability 8 (11), 1159. doi:10.3390/su8111159
City of Los Angeles (2007). Los Angeles River Revitalization Master Plan. City of Los Angeles, Department of Public Works, Bureau of Engineering. Available online at: https://boe.lacity.org/lariverrmp/CommunityOutreach/masterplan_download.htm.
Dagit, R., Adams, S., and Drill, S. (2009). Die off and Current Status of Southern Steelhead Trout (Oncorhynchus mykiss) in Malibu Creek, Los Angeles County, USA. Bull. South. Calif. Acad. Sci. 108 (1), 1–15. doi:10.3160/0038-3872-108.1.1
Dbouk, T. (2017). A Review about the Engineering Design of Optimal Heat Transfer Systems Using Topology Optimization. Appl. Therm. Eng. 112, 841–854. doi:10.1016/j.applthermaleng.2016.10.134
Eaton, J. G., and Scheller, R. M. (1996). Effects of Climate Warming on Fish thermal Habitat in Streams of the United States. Limnol. Oceanogr. 41 (5), 1109–1115. doi:10.4319/lo.1996.41.5.1109
Ebersole, J. L., Liss, W. J., and Frissell, C. A. (2001). Relationship between Stream Temperature, thermal Refugia and Rainbow trout Oncorhynchus mykiss Abundance in Arid-Land Streams in the Northwestern United States. Ecol. Freshw. Fish 10 (1), 1–10. doi:10.1034/j.1600-0633.2001.100101.x
Environmental Science Associates (2018). HEC-RAS Model for Glendale 2018 Wastewater Change Petition.
Everard, M., and Moggridge, H. L. (2012). Rediscovering the Value of Urban Rivers. Urban Ecosyst. 15 (2), 293–314. doi:10.1007/s11252-011-0174-7
Findley, S. J., and Taylor, M. P. (2006). Why Rehabilitate Urban River Systems. Area 38 (3), 312–325.
Fryirs, K., and Brierley, G. (2000). A Geomorphic Approach to the Identification of River Recovery Potential. Phys. Geogr. 21, 244–277. doi:10.1080/02723646.2000.10642708
Garner, G., Malcolm, I. A., Sadler, J. P., and Hannah, D. M. (2017). The Role of Riparian Vegetation Density, Channel Orientation and Water Velocity in Determining River Temperature Dynamics. J. Hydrol. 553, 471–485. doi:10.1016/j.jhydrol.2017.03.024
Glose, A., Lautz, L. K., and Baker, E. A. (2017). Stream Heat Budget Modeling with HFLUX: Model Development, Evaluation, and Applications across Contrasting Sites and Seasons. Environ. Model. Softw. 92, 213–228. doi:10.1016/j.envsoft.2017.02.021
Gu, R., McCutcheon, R., and Chen, C. J. (1999). Development of Weather-dependent Flow Requirements for River Temperature Control. Environ. Manage. 24 (4), 529–540. doi:10.1007/s002679900252
Gu, R. R., and Li, Y. (2002). River Temperature Sensitivity to Hydraulic and Meteorological Parameters. J. Environ. Manage. 66, 43–56. doi:10.1006/jema.2002.0565
Haglund, T. R., and Baskin, J. N. (2003). Habitat and Resources Utilization by the Santa Ana Sucker (Catostomus Santaanae) and the Santa Ana Speckled Dace (Rhinichtys Osculus Ssp) in the East Fork of the San Gabriel River. Contract No. P9985307: Unpublished report prepared for the California Department of Fish and Game.
Haglund, T. R., Baskin, J. N., and Swift, C. C. (2001). Results of the Year 1 Implementation of the Santa Ana Sucker Conservation Program for the Santa Ana River. Unpublished report prepared for Santa Ana Watershed Project Authority.
HDR CDM. (2011). HEC-RAS Model for San Gabriel River, San Jose Creek, Compton Creek, Upper Rio Hondo, Coyote Creek, Verdugo Wash. Arroyo Seco.
Herb, W. R., Janke, B., Mohseni, O., and Stefan, H. G. (2009). Runoff Temperature Model for Paved Surfaces. J. Hydrol. Eng. 14, 1146–1155. doi:10.1061/(asce)he.1943-5584.0000108
Hockey, J. B., Owen, I. F., and Tapper, N. J. (1982). Empirical and Theoretical Models to Isolate the Effect of Discharge on Summer Water Temperatures in the Hurunui River. J. Hydrol. (N.Z.) 21, 1–12.
Hokanson, K. E. F., Kleiner, C. F., and Thorslund, T. W. (1977). Effects of Constant Temperatures and Diel Temperature Fluctuations on Specific Growth and Mortality Rates and Yield of Juvenile Rainbow trout, Salmo Gairdneri. J. Fish. Board Can. 34 (5), 194–200. doi:10.1139/f77-100
Justice, C., White, S. M., McCullough, D. A., Graves, D. S., and Blanchard, M. R. (2017). Can Stream and Riparian Restoration Offset Climate Change Impacts to salmon Populations. J. Environ. Manage. 188, 212–227. doi:10.1016/j.jenvman.2016.12.005
Kurylyk, B. L., Moore, R. D., and Macquarrie, K. T. B. (2016). Scientific Briefing: Quantifying Streambed Heat Advection Associated with Groundwater-Surface Water Interactions. Hydrol. Process. 30, 987–992. doi:10.1002/hyp.10709
LeBlanc, R. T., Brown, R. D., and FitzGibbon, J. E. (1997). Modeling the Effects of Land Use Change on the Water Temperature in Unregulated Urban Streams. J. Environ. Manage. 49, 445–469. doi:10.1006/jema.1996.0106
Lee, R. M., and Rinne, J. N. (1980). Critical thermal Maxima of Five trout Species in the Southwestern United States. Trans. Am. Fish. Soc. 109 (6), 632–635. doi:10.1577/1548-8659(1980)109<632:ctmoft>2.0.co;2
Loheide, S. P., and Gorelick, S. M. (2006). Quantifying Stream−Aquifer Interactions through the Analysis of Remotely Sensed Thermographic Profiles and In Situ Temperature Histories. Environ. Sci. Technol. 40, 3336–3341. doi:10.1021/es0522074
Madden, N., Lewis, A., and Davis, M. (2013). Thermal Effluent from the Power Sector: an Analysis of Once-Through Cooling System Impacts on Surface Water Temperature. Environ. Res. Lett. 8, 035006. doi:10.1088/1748-9326/8/3/035006
Materna, E. (2001). Issue Paper 4. Temperature Interaction. Prepared as part of U.S. EPA Region 10 Temperature Water Quality Criteria Guidance Development ProjectEPA-910-D-01-004.
McCullough, D. A., Spalding, S., Sturdevant, D., and Hicks, M. (2001). Issue Paper 5. Summary of Technical Literature Examining the Physiological Effects of Temperature on Salmonids. EPA-910-D-01-005, prepared as part of U.S. EPA Region 10. Temperature Water Quality Criteria Guidance Development Project.
Merriam, E. R., and Petty, J. T. (2019). Stream Channel Restoration Increases Climate Resiliency in a Thermally Vulnerable Appalachian River. Restor Ecol. 27 (6), 1420–1428. doi:10.1111/rec.12980
Mika, K., Gallo, E., Read, L., Edgley, R., Truong, K., Hogue, T. S., et al. (2017). LA Sustainable Water Project. Los Angeles River Watershed UCLA Institute of the Environment and Sustainability, 144.
Mohseni, O., Stefan, H. G., and Erickson, T. R. (1998). A Nonlinear Regression Model for Weekly Stream Temperatures. Water Resour. Res. 34 (10), 2685–2692. doi:10.1029/98wr01877
Mongolo, J., Trusso, N., Dagit, R., Aguilar, A. A., and Drill, S. L. (2017). A Longitudinal Temperature Profile of the Los Angeles River from June through October 2016: Establishing a Baseline. Southern California Academy of Sciences, 26.
Moyle, P. B., Israel, J. A., and Purdy, S. E. (2008). Salmon, Steelhead and trout in California, Status of an Emblematic Fauna; a Report Commissioned by California Trout. UC Davis Center for Watershed Sciences.
Murtagh, F. (1991). Multilayer Perceptrons for Classification and Regression. Neurocomputing 2 (5-6), 183–197. doi:10.1016/0925-2312(91)90023-5
Myrick, C. A., and Cech, J. J. (2000). Growth and thermal Biology of Feather River Steelhead under Constant and Cyclical temperaturesFish, and Conservation Biology. Davis, Davis, CA: Department of Water Resources ContractFinal Report, Department of WildlifeUniversity of California, 20.
Naddy, R. B., Cohen, A. S., and Stubblefield, W. A. (2015). The Interactive Toxicity of Cadmium, Copper, and Zinc toCeriodaphnia Dubiaand Rainbow trout (Oncorhynchus mykiss). Environ. Toxicol. Chem. 34 (4), 809–815. doi:10.1002/etc.2870
Neitsch, S. L., Arnold, J. G., Kiniry, J. R., and Williams, J. R. (2011). Soil and Water Assessment Tool Theoretical Documentation Version 2009. Texas Water Resources Institute.
Nichols, A. L., Willis, A. D., Jeffres, C. A., and Deas, M. L. (2014). Water Temperature Patterns below Large Groundwater Springs: Management Implications for Coho Salmon in the Shasta River, California. River Res. Applic. 30, 442–455. doi:10.1002/rra.2655
Null, S. E., Mouzon, N. R., and Elmore, L. R. (2017). Dissolved Oxygen, Stream Temperature, and Fish Habitat Response to Environmental Water Purchases. J. Environ. Manage. 197, 559–570. doi:10.1016/j.jenvman.2017.04.016
O’Brien, G. C., Dickens, C., Hines, E., Wepener, V., Stassen, R., Quayle, L., et al. (2020). A Regional-Scale Ecological Risk Framework for Environmental Flow Evaluations. Hydrol. Earth Syst. Sci. 22, 957–975.
Palmer, M. A., Bernhardt, E. S., Allan, J. D., Lake, P. S., Alexander, G., Brooks, S., et al. (2005). Standards for Ecologically Successful River Restoration. J. Appl. Ecol. 42 (2), 208–217. doi:10.1111/j.1365-2664.2005.01004.x
Pander, J., and Geist, J. (2013). Ecological Indicators for Stream Restoration success. Ecol. Indicators 30, 106–118. doi:10.1016/j.ecolind.2013.01.039
Parker, F. L., and Krenkel, P. A. (1969). Thermal Pollution: Status of the Art; Report 3. Nashville, TN, USA: Department of Environmental and Resource Engineering, Vanderbilt University.
Paulinski, S., Pedregosa, F., Varoquaux, G., Gramfort, A., Michel, V., Thirion, B., et al. (2021). Development of a Groundwater-Simulation Model in the Los Angeles Coastal Plain, Los Angeles County, CaliforniaScikit-Learn: Machine Learning in Python. J. Machine Learn. Res. 12, 4892825–4892830. doi:10.3133/sir20215088
Petrovic, M., Solé, M., López De Alda, M. J., and Barceló, D. (2002). Endocrine Disruptors in Sewage Treatment Plants, Receiving River Waters, and Sediments: Integration of Chemical Analysis and Biological Effects on Feral Carp. Environ. Toxicol. Chem. 21 (10), 2146–2156. doi:10.1002/etc.5620211018
Poole, G. C., and Berman, C. H. (2001). An Ecological Perspective on In-Stream Temperature: Natural Heat Dynamics and Mechanisms of Human-CausedThermal Degradation. Environ. Manage. 27 (6), 787–802. doi:10.1007/s002670010188
Poshtiri, M. P., and Pal, I. (2016). Patterns of Hydrological Drought Indicators in Major U.S. River Basins. Climatic Change 134, 549–563. doi:10.1007/s10584-015-1542-8
Purcell, A. H., Friedrich, C., and Resh, V. H. (2002). An Assessment of a Small Urban Stream Restoration Project in Northern California. Restor Ecol. 10 (4), 685–694. doi:10.1046/j.1526-100x.2002.01049.x
Quinn, T. P. (2018). “The Behavior and Ecology of Pacific salmon and Trout,” in Association with the American Fisheries Society (Bethesda, Maryland: University of Washington Press).The Behavior and Ecology of Pacific salmon and trout
Read, L. K., Hogue, T. S., Edgley, R., Mika, K., and Gold, M. (2019). Evaluating the Impacts of Stormwater Management on Streamflow Regimes in the Los Angeles River. J. Water Resour. Plann. Manage. 145 (10), 05019016. doi:10.1061/(asce)wr.1943-5452.0001092
Rheinheimer, D. E., Null, S. E., and Lund, J. R. (2015). Optimizing Selective Withdrawal from Reservoirs to Manage Downstream Temperatures with Climate Warming. J. Water Resour. Plann. Manage. 141 (4), 04014063. doi:10.1061/(asce)wr.1943-5452.0000447
Risley, J. C., Constantz, J., Essaid, H., and Rounds, S. (2010). Effects of Upstream Dams versus Groundwater Pumping on Stream Temperature under Varying Climate Conditions. Water Resour. Res. 46, 6. doi:10.1029/2009wr008587
Rogers, J. B., Stein, E. D., Beck, M. W., Flint, K., Kinoshita, A., and Ambrose, R. F. (2021). Modeling Future Changes to the Hydrological and thermal Regime of Unaltered Streams Using Projected Changes in Climate to Support Planning for Sensitive Species Management. Ecohydrology 14, e2299. doi:10.1002/eco.2299
Saha, G. C., Li, J., Thring, R. W., Hirshfield, F., and Paul, S. S. (2017). Temporal Dynamics of Groundwater-Surface Water Interaction under the Effects of Climate Change: A Case Study in the Kiskatinaw River Watershed, Canada. J. Hydrol. 551, 440–452. doi:10.1016/j.jhydrol.2017.06.008
Saiki, M. K., Martin, B. A., Knowles, G. W., and Tennant, P. W. (2007). Life History and Ecological Characteristics of the Santa Ana Sucker, Catostomus Santaanae. Calif. Fish Game 93 (2), 87–101.
San Marino Environmental Associates (SMEA) (2010). Santa Ana Sucker Research Progress Report: December 2010. Santa Ana sucker population monitoring, 20015–22010.
Sand-Jensen, K., Pedersen, O., Binzer, T., and Borum, J. (2005). Contrasting Oxygen Dynamics in the Freshwater Isoetid Lobelia Dortmanna and the Marine Seagrass Zostera marina. Ann. Bot. 96 (4), 613–623. doi:10.1093/aob/mci214
Santa Ynez River Technical Advisory Committee (SYRTAC) (2000). Lower Santa Ynez River Fish Management Plan. Santa Ynez River Technical Advisory Committee, 180. Santa Ynez Consensus Committee.
Sauter, S. T., McMillan, J., and Dunham, J. (2001). Issue Paper 1. Salmonid Behavior and Water Tempera- Ture. Prepared as part of U.S. EPA Region 10 Temperature Water Quality Criteria Guidance Development Project. EPA-910-D-01-001.
Sengupta, M., Xie, Y., Lopez, A., Habte, A., Maclaurin, G., and Shelby, J. (2018). The National Solar Radiation Data Base (NSRDB). Renew. Sustain. Energ. Rev. 89, 51–60. doi:10.1016/j.rser.2018.03.003
Sinokrat, B. A., and Gulliver, J. S. (2010). In-stream Flow Impact on River Water Temperatures. J. Hydraulic Res. 5, 339–349.
Sloat, M. R., and Osterback, A.-M. K. (2013). Maximum Stream Temperature and the Occurrence, Abundance, and Behavior of Steelhead trout (Oncorhynchus mykiss) in a South ern California Stream. Can. J. Fish. Aquat. Sci. 70 (1), 64–73. doi:10.1139/cjfas-2012-0228
Smith, B., Clifford, N. J., and Mant, J. (2014). The Changing Nature of River Restoration. WIREs Water 1 (3), 249–261. doi:10.1002/wat2.1021
Smith, K., and Lavis, M. E. (1975). Environmental Influences on the Temperature of a Small upland Stream. Oikos 26 (2), 228–236. doi:10.2307/3543713
Spina, A. P. (2007). Thermal Ecology of Juvenile Steelhead in a Warm-Water Environment. Environ. Biol. Fish. 80 (1), 23–34. doi:10.1007/s10641-006-9103-7
Sridhar, V., Sansone, A. L., LaMarche, J., Dubin, T., and Lettenmaier, D. P. (2004). Prediction of Stream Temperature in Forested Watersheds. J. Am. Water Resour. Assoc 40, 197–213. doi:10.1111/j.1752-1688.2004.tb01019.x
Stabler, D. F. (1981). Effects of Altered Flow Regimes, Temperatures, and River Impoundment on Adult Steelhead trout and chinook salmon. Moscow: M.S. thesis, University of Idaho, 84.
Stein, E., Taniguchi-Quan, K., Wolfand, J., Gallo, E., Irving, K., Philippus, D., et al. (2021b). Process and Decision Support Tools for Evaluating Flow Management Targets to Support Aquatic Life and Recreational Beneficial Uses of the Los Angeles River: Los Angeles River Environmental Flows Project. Southern California Coastal Water Research Project, 63.
Stein, E., Wolfand, J., Abdi, R., Irving, K., Hennon, V., Taniguchi-Quan, K., et al. (2021a). Assessment of Aquatic Life Use Needs for the Los Angeles River. Southern California Coastal Water Research Project, 96.
Stillwater Sciences (2020). Conceptual Ecological Model and Limiting Factors Analysis for Steelhead in the Los Angeles River Watershed. Los AngelesPasadena, California: Final Technical MemorandumCalifornia for the Council for Watershed Health. Prepared by Stillwater Sciences.
Sun, N., Yearsley, J., Baptiste, M., Cao, Q., Lettenmaier, D. P., and Nijssen, B. (2016). A Spatially Distributed Model for Assessment of the Effects of Changing Land Use and Climate on Urban Stream Quality. Hydrol. Process. 30 (25), 4779–4798. doi:10.1002/hyp.10964
Sun, N., Yearsley, J., Voisin, N., and Lettenmaier, D. P. (2015). A Spatially Distributed Model for the Assessment of Land Use Impacts on Stream Temperature in Small Urban Watersheds. Hydrol. Process. 29 (10), 2331–2345. doi:10.1002/hyp.10363
Tian, Z., Zhao, H., PeterGonzalez, K. T. M., Gonzalez, M., Wetzel, J., Wu, C., et al. (2021). A Ubiquitous Tire Rubber-Derived Chemical Induces Acute Mortality in Coho salmon. Science 371 (6525), 185–189. doi:10.1126/science.abd6951
Trimmel, H., Weihs, P., Leidinger, D., Formayer, H., Kalny, G., and Melcher, A. (2018). Can Riparian Vegetation Shade Mitigate the Expected Rise in Stream Temperatures Due to Climate Change during Heat Waves in a Human-Impacted Pre-alpine River. Hydrol. Earth Syst. Sci. 22, 437–461. doi:10.5194/hess-22-437-2018
Upper Los Angeles River Area Wastewater (ULARA) (2019). Wastewater Service in the Upper Los Angeles River Area – 2017-18 Water Year. ULARA.
U.S. Army Corps of Engineers (2004). HEC-RAS Model for Stormwater Management Plan. US Army Corp of Engineers, Hydrologic engineering center.
U.S. Army Corps of Engineers (2005). HEC-RAS Model for Upper Los Angeles River and Tujunga Wash. US Army Corp of Engineers, Hydrologic engineering center.
U.S. Army Corps of Engineers (2016). HEC-RAS River Analysis System Hydraulic Reference Manual Version 5.0, CPD-68. US Army Corp of Engineers, Hydrologic engineering center, 960.
U.S. Army Corps of Engineers (2011). Los Angeles County Drainage Area, San Gabriel River, San Jose Creek Compton Creek, Upper Rio Hondo, Coyote Creek, Verdugo Wash HEC-RAS Hydraulic Models, 37. Los Angeles District: U.S. Army Corps of Engineers.
U.S. Bureau of Reclamation (2019). Design and Analysis of Ecosystem Features in Urban Flood Control ChannelsResearch and Development Office, Science and Technology Program. Final Report ST-2019-1726-01 .
U.S. Department of Commerce (1997). Endangered and Threatened Species: Listing of Several Evolutionary Significant Units (ESUs) of West Coast Steelhead, 50. Washington, DC: National Oceanic and Atmospheric Administration. CFR Parts 222 and 227.
U.S. Environmental Protection Agency (USEPA) (2003). EPA Region 10 Guidance for Pacific Northwest State and Tribal Water Quality Standards, 10. Seattle, WA: Region. EPA 910-B-03-002. 49pp.
U.S. Fish and Wildlife Service (2000). 65 FR 19686. Endangered and Threatened Wildlife and Plants; Threatened Status for the Santa Ana Sucker. Fed. Regist. 65, 19686–19698.
U.S. Fish and Wildlife Service (2014). Draft Recovery Plan for the Santa Ana Sucker. U.S. Fish and Wildlife Service, Pacific Southwest Region. Sacramento, California, 61. v +.
U.S. Fish and Wildlife Service (2011). Santa Ana Sucker (Catostomus Santaanae) 5-Year Review: Summary and Evaluation.
Van Buren, M., Watt, W. E., Marsalek, J., and Anderson, B. C. (2000). Thermal Enhancement of Stormwater Runoff by Paved Surfaces. Water Res. 34, 1359–1371. doi:10.1016/s0043-1354(99)00244-4
Verhille, C. E., English, K. K., Cocherell, D. E., Farrell, A. P., Fangue, N. A., Walsh, C. J., et al. (20162005). High thermal Tolerance of a Rainbow trout Population Near its Southern Range Limit Suggests Local thermal adjustmentConservation PhysiologyThe Urban Stream Syndrome: Current Knowledge and the Search for a Cure. Conserv Physiol. 424 (13), cow057706–723. doi:10.1093/conphys/cow057
Washington State Department of Ecology (WDOE) (2002). Evaluating Criteria for the Protection of Freshwater Aquatic Life in Washington’s Surface Water Quality Standards: Dissolved Oxygen. Draft Discussion Paper and Literature Summary. Publication Number 00-10-071. 90pp.
Wohl, E., Lane, S. N., and Wilcox, A. C. (2015). The Science and Practice of River Restoration. Water Resour. Res. 51 (8), 5974–5997. doi:10.1002/2014wr016874
Wunderlich, T. E. (1972). in Tennessee Valley Authority (USA: Norris Tennessee, TN).Heat and Mass Transfer between a Water Surface and the Atmosphere; Report No. 14; Water Resources Research Laboratory
Zhang, J., ZhangShi, C. W., and Fu, Y. (2018). Quantitative Evaluation and Optimized Utilization of Water Resources-Water Environment Carrying Capacity Based on Nature-Based Solutions. J. Hydrol. 568, 96–107. (https://www.sisense.com/glossary/data-cleaning/).
Keywords: river temperature, environmental flows, mechanistic modeling, multilayer linear regression, restoration, Los Angeles River, fish migration, climate change
Citation: Abdi R, Rust A, Wolfand JM, Taniguchi-Quan K, Irving K, Philippus D, Stein ED and Hogue TS (2022) Thermal Suitability of the Los Angeles River for Cold Water Resident and Migrating Fish Under Physical Restoration Alternatives. Front. Environ. Sci. 9:749085. doi: 10.3389/fenvs.2021.749085
Received: 28 July 2021; Accepted: 21 December 2021;
Published: 12 January 2022.
Edited by:
Zoe Courville, Cold Regions Research and Engineering Laboratory, United StatesReviewed by:
Gabrielle David, Cold Regions Research and Engineering Laboratory, United StatesMatthew Keefer, University of Idaho, United States
Copyright © 2022 Abdi, Rust, Wolfand, Taniguchi-Quan, Irving, Philippus, Stein and Hogue. This is an open-access article distributed under the terms of the Creative Commons Attribution License (CC BY). The use, distribution or reproduction in other forums is permitted, provided the original author(s) and the copyright owner(s) are credited and that the original publication in this journal is cited, in accordance with accepted academic practice. No use, distribution or reproduction is permitted which does not comply with these terms.
*Correspondence: Reza Abdi, rabdi@mines.edu