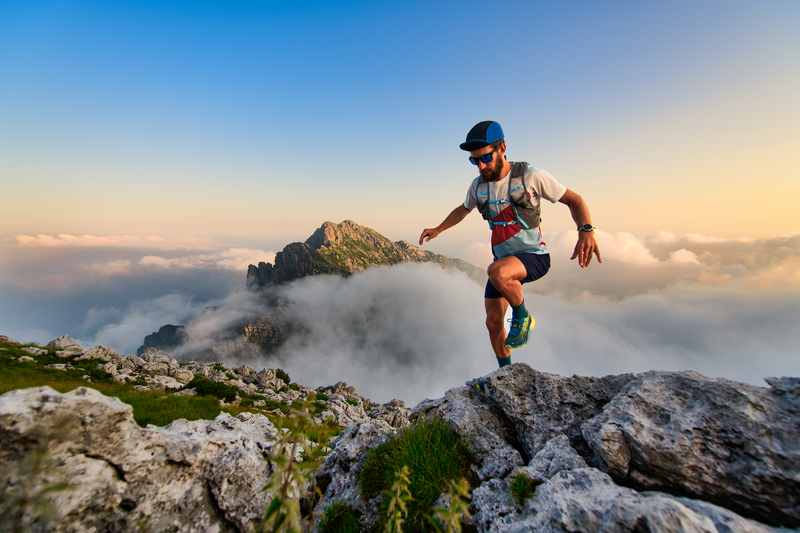
95% of researchers rate our articles as excellent or good
Learn more about the work of our research integrity team to safeguard the quality of each article we publish.
Find out more
ORIGINAL RESEARCH article
Front. Environ. Sci. , 29 July 2021
Sec. Biogeochemical Dynamics
Volume 9 - 2021 | https://doi.org/10.3389/fenvs.2021.717041
This article is part of the Research Topic Climate Change and Anthropogenic Impacts on Soil Organic Matter View all 5 articles
Biochar has received much attention as a strategy to enhance soil carbon (C) sequestration and mitigate climate change. Previous studies found that the feedstock and pyrolysis temperature can largely determine biochar properties, which in turn, impact the stability of native soil organic matter (SOM) and soil microorganisms. The Schima superba and Cunninghamia lanceolata are two tree species widely distributed in the subtropical region of southern China, but how the biochars from these two species influence the soil C sequestration and microbial communities of plantation remain poorly understood. In this study, we produced biochars from these two different feedstocks (13C-labeled S. superba and C. lanceolata litters) at three pyrolysis temperatures (350°C, 550°C, 750°C), then added them to the soils from C. lanceolata plantation, and maintained the experiments at 25°C for 112 days. We found both C mineralization and soil microbial community structures were strongly, but inconsistent, affected by biochar feedstock and pyrolysis temperature. The C. lanceolata biochar triggered the negative priming effect faster and greater compared with the S. superba biochar amendment. Biochars produced at 550°C showed the most significant negative priming effect during the whole incubation period, regardless of the different feedstocks. The cumulative amount of CO2 derived from biochars was significantly decreased with pyrolysis temperature (p < 0.05), indicating that biochars prepared at higher temperatures were more stable in the soil. Further, the soil microbial community structure was only affected by biochar pyrolysis temperature rather than biochar feedstock and their interaction. Together, our results reveal that biochar feedstock and pyrolysis temperature may play more important roles in dictating the priming effect than the structure of microbial community for C. lanceolata plantation. Overall, we concluded that the biochars prepared at 550°C could rapidly decrease the turnover of native SOM in a short term and biochar amendment has the potential to be a management practice for soil C sequestration in the C. lanceolata plantation.
Forest plantations in China account for about one-third of the global area of plantation and contributed about 80% of the total forest C sink increment in China (Fang et al., 2014; Li et al., 2018). Management practices such as fertilization and harvest residue may notably alter soil C storage and affect the C sequestration of forest plantations (Vogel et al., 2015; Wan et al., 2018). Soil organic matter (SOM) mineralization is a major pathway of soil C emission (Guillaume et al., 2015; Huang et al., 2019). Therefore, the understanding of the SOM mineralization in response to management practice and environmental change is crucial for mitigating the greenhouse gas emission and optimizing the climatic impacts of forest ecosystems (Post et al., 1982; Trumbore, 2006). Biochar is a carbon-rich product produced by plant or animal residues at high temperatures pyrolysis under limited or no supply of oxygen (Woolf et al., 2010; Hansen et al., 2016). It has received much attention as an effective soil amendment due to its beneficial properties for improving soil fertility and mitigating climate change in recent years (Fowles, 2007; Gomez et al., 2014; Fatima et al., 2021). Biochar is mainly made of highly stable forms of C, then it can remain in the soil for thousands to dozens of thousands years (Leng et al., 2019). For example, the decomposition rate of biochar was only between 0.4 and 0.6% after incubation experiment for 336 days, while that of fresh plant residue was about 25% (Naisse et al., 2015). Therefore, the potential of biochar for soil C stability and sequestration is extremely essential and enormous (Yuan et al., 2019), and the influence of biochar on C sequestration must be better understood.
Several studies have shown that biochar incorporation increased the soil C pool through affecting the mineralization of native SOM (Kuzyakov et al., 2009; Zimmerman et al., 2011; Cui et al., 2017; Luo et al., 2017). However, the influence of biochar on native SOM decomposition is a complex interaction process. Specifically, it depends on the biochar feedstock and pyrolysis temperature (Gomez et al., 2014; Ding et al., 2017; Yu et al., 2018), soil types (Lehmann et al., 2011) and incubation conditions (Maestrini et al., 2015), which can generate positive (stimulation), negative (suppression), and nonsignificant priming effect (Kuzyakov et al., 2000; Zimmerman et al., 2011; Luo et al., 2017). For example, Keith et al. (2011) found that the addition of wood biochar (450°C and 550°C pyrolysis of Eucalyptus salinga, 2% of the soil mass) can promote the slightly native SOM mineralization. In contrast, Rittl et al. (2015) showed that biochar (380°C pyrolysis of Jatropha curcas L., 1% of the soil mass) had a significant negative priming effect on native SOM after 30 days of incubation. Therefore, it is especially essential to study the specific conditions and their mechanisms to achieve C sequestration for the biochar amendment.
Soil microorganisms play an important role in regulating the priming effect (Gomez et al., 2014; Mitchell et al., 2015; Yu et al., 2018), but soil microbial communities often vary with exogenous C (Semenov et al., 2012). Studies indicate that the enhanced microbial biomass or activity may cause increased native SOM mineralization by biochar (Hamer et al., 2004; Fang et al., 2015). It possibly results from biological co-metabolism by labile components in biochar (Kuzyakov et al., 2009; Luo et al., 2018) or other nutrients supplied from biochar (Quilliam et al., 2012). Conversely, some mechanisms of negative priming effect by biochar have also been proposed. For example, the negative priming effect can be caused by the stabilization of native SOM by biochar combined with soil minerals and aggregation (Keith et al., 2011; Herath et al., 2013), or the inhibitory effect of volatile organic compounds in biochar on soil microbial activity (Spokas et al., 2010; Fang et al., 2015). Therefore, as a mediator, the soil microorganisms may play a central role in controlling the direction and intensity of the priming effect (Mitchell et al., 2015; Tian et al., 2019). It is critical to explore the changes in microbial community structure to better understand the mechanism of the priming effect.
China has a wide area of Cunninghamia lanceolata plantations with acidic and scarcely fertile soils (Duan et al., 2019), its area is more than 1.10×107 hm2, accounting for ca. 6.66% of the total forest area in China (Yu et al., 2017). Clear-cutting and slash burning are common silvicultural practices in subtropical China (Guo et al., 2016). Although it is convenient for farmers to plant seedlings, such disturbance also causes serious environmental pollution and soil erosion (Yang et al., 2005). If the harvesting residues and litter can be used to produce biochar and return it to the soil, thus the issues caused by direct fire may be avoided, and soil fertility of the plantation would be improved. Previous study indicated that biochar is a potentially effective material for soil C sequestration in C. lanceolata plantations (Li et al., 2019). However, the effects of biochar on soil C sequestration may largely depend on its properties (Lin et al., 2017), and how the feedstock and pyrolysis temperature determine biochar properties remains poorly studied (Rafael et al., 2019), although these two factors may have strong impacts on the stability of native SOM and soil microorganisms (Jindo and Sonoki, 2019).
By using an in vitro incubation experiment, we measured the CO2 emissions and microbial community structures from soils amended with biochars with two contrasting feedstocks (S. superba or C. lanceolate litter) produced at three pyrolysis temperatures (350°C, 550°C and 750°C) to study the effects of biochar feedstock and pyrolysis temperature on SOM mineralization and microbial community structures of forest soils. Here, we hypothesized that both biochar feedstock and pyrolysis temperature would have strong effects on SOM mineralization and microbial community structures, being soil microbial community structures and soil properties largely determining the SOM mineralization due to the decomposition activity of microorganisms.
Soils used in this experiment were taken from the field at Wanmulin Nature Reserve of Fujian province, which is located in northern Fujian Province, southeastern China (27°03′N, 118°09 E). The area has a mid-subtropical monsoon climate with an average annual temperature of 19.4°C and an average annual precipitation of 1731 mm. The soil has developed from granite and is an Ultisol in USDA Soil Taxonomy (Ma et al., 2019). In april 2017, soil (about 5 kg) was collected from the 0–20 cm layer under a C. lanceolata plantation about 36-year-old. Visible plant residues were removed, and then the soil was air-dried and passed through a 2 mm sieve. Soil pH was determined in distilled water suspension (1:2.5, weight/volume) by a pH meter (Starter 300, United States) (Luo et al., 2011), whereas total C (TC) and total N (TN) were determined by dry combustion using a Vario MAX CN analyzer (Elementar, Germany) (Tian et al., 2019). Soil particle size was determined by laser diffraction method as described by Yang et al. (2015), soil sample (300 mg) was treated in 10% H2O2 solution for 48 h with occasional stirring (5 times) to remove the organic material; carbonates were then removed using 0.2% HCl solution, and soil aggregates were dispersed using 0.5 M Na6(PO3)6 solution. The contents of sand (2000–20 μm), silt (20–2 μm) and clay fractions (<2 μm) were measured using a Mastersizer 2000 particle-size analyzer (Malvern, United Kingdom). The properties of the tested soil were as follows: pH 5.7, TC 22.80 g kg−1, TN 1.77 g kg−1, sand 40.8%, silt 45.4%, and clay 13.8%.
Biochars were produced from 13C-labeled S. superba and C. lanceolata litter as feedstocks, which were generated by a pulse-labelling method (Yin et al., 2010). Before the pyrolysis, the two types of feedstock were dried at 70°C for 24 h and milled to <2 mm. The <2 mm fractions (15.0 g) were placed in sealed porcelain crucibles and underwent pyrolysis in the muffle furnace with peak temperatures of 350°C, 550°C, and 750°C for 2 h (Peng et al., 2011). The crucibles were closed with a cap and wrapped with tin foil to reduce the oxygen entry. It took about 35 min to reach the final temperature. After cooling, biochar samples produced from each feedstock with eight replicates were pooled (Hamer et al., 2004). S. superba-derived biochars at 350°C, 550°C and 750°C pyrolysis were named as SB350, SB550 and SB750, while C. lanceolata-derived biochars at 350°C, 550°C, and 750°C pyrolysis temperatures were named as CB350, CB550, and CB750. For each biochar, the pH was measured by a pH meter in distilled water (1:15, weight/volume), whereas total dissolved organic C (DOC) was extracted with distilled water (1:10, weight/volume) and measured with a TOC-VCPH analyzer (Shimadzu, Japan) (Luo et al., 2011). The TC and TN were determined with a Vario EL III CN analyzer (Elementar, Germany) (Yang et al., 2005). The δ13C value (‰) of biochar was determined using a MAT 253 IRMS connected with Flash 2000HT elemental analyzer (Thermo Scientific, United States) (Cui et al., 2017). Volatile matter was determined by weight loss after heating; the temperature of the muffle furnace was raised to 900°C where it remained for 10 min (Crombie et al., 2013). The biochars properties are shown in Table 1.
Seven treatments were examined: 1) the soil with no amendment (CK), 2) soil amended with SB350 (SS350), 3) soil amended with SB550 (SS550), 4) soil amended with SB750 (SS750), 5) soil amended with CB350 (SC350), 6) soil amended with CB550 (SC550), 7) soil amended with CB750 (SC750). The experiment was a completely randomized design with three replicates for each treatment. Air-dried soils (equivalent to 50 g oven-dry basis) were separately mixed with the biochars at an application of 20 g kg−1 soil (dry weight basis). Soil moisture was adjusted to 60% water holding capacity (WHC) by the addition of deionized water. These mixed soil substrates were put inside 60 ml small vials, which were placed inside a 1 L glass jar with a rubber stopper, with 10 ml 0.5 M NaOH in a vial and 10 ml deionized water at the bottom of jar. The NaOH trap vials were collected and replaced by new vials at 3, 7, 14, 28, 56, 84, and 112 days, respectively. The sampling time intervals were selected according to Luo et al. (2011) and Cui et al. (2017). During the incubation, all treatments were maintained in a chamber at 25°C for 112 days in the dark. We adjusted soil moisture for every sampling. After the incubation, each soil was divided into two subsamples. One subsample was for the determination of soil properties, and the other was freeze-dried for the phospholipid fatty acid (PLFA) analysis.
To quantify the organic matter mineralization, the cumulative amount of total CO2 in each period was measured by the titration of 0.25 M HCl and BaCO3 precipitation (Aoyama et al., 2000). The δ13C value (‰) of BaCO3 was determined using the MAT 253 IRMS equipped with a Gasbench device (Thermo Scientific, United States) (Cui et al., 2017), and that of soil was determined using the same procedure as used for the biochar.
To examine the response of the soil microbial community structure to biochars application, we extracted the soil phospholipid fatty acids (PLFAs) following the method described in Denef et al. (2007), then the contents of PLFAs were determined by a gas chromatograph (Agilent 6890 N, United States) combined with the MIDI Sherlock Microbial Identification System (MIDI Inc., United States). The PLFAs are denoted by standard PLFA nomenclature (Frostegård et al., 2011; Wan et al., 2015) and grouped to the Gram-positive bacteria (G+) (14:0 iso, 15:0 iso, 15:0 anteiso, 16:0 iso, 17:0 iso, and 17:0 anteiso), Gram-negative bacteria (G−) (16:1 ω9c, 16:1ω7c, 17:0 cyclo ω7c, 18:1 ω7c, 18:1 ω5c, and 19:0 cyclo ω7c), Fungi (18:2 ω6c and 18:1 ω9c) and Actinomycetes (ACT) (16:0 10-methyl, 17:0 10-methyl, and 18:0 10-methyl). Total microbial PLFAs were determined as the sum of all PLFAs above and Non-specific bacteria (14:00, 15:00, 16:00, 17:00, and 18:00), while total bacterial PLFA contents were calculated as the sum of PLFAs attributed to G+ and G−.
The fraction of CO2 production derived from biochar was calculated as,
Where δ13CO2,biochar and δ13CO2,control are the δ13C values of CO2 produced from soils with and without biochar, respectively, while δ13Cbiochar and δ13Csoil are the δ13C values of biochars and soil (Luo et al., 2011).
The contribution of native SOC to CO2 production in soils with biochar was determined as,
Where CO2,biochar was the total CO2 production in soil with biochar addition.
Priming effect (PE) on CO2 production from SOC was defined as,
where CO2,control was the total CO2 production in soil without biochar.
Statistical analyses were performed using the SPSS 19.0 software package for Windows (SPSS Inc. Chicago, IL, United States), OriginPro 9.0 (Origin Lab, Northampton, MA, United States) and R 3.6.0. Effects of biochar preparation feedstock, pyrolysis temperature, and their interaction on soil microbial groups were tested using repeated measures ANOVA. Significant differences between treatments were calculated using the Duncan test. Principal component analyses (PCA) using the absolute content of microbial groups in the soil were performed to compare the microbial community structures in the different treatments. Correlation analyses among parameters were performed by Spearman’s coefficient test. Statistical significance was assigned at the p < 0.05 level.
The cumulative amounts of CO2 derived from biochar and native SOM were significantly affected by biochar feedstock, pyrolysis temperature, and their interaction (Figure 1, Supplementary Table S1). Specifically, the cumulative amount of CO2 from biochar (i.e., the sum of the amount of CO2 from biochar from 0 to 112 days) was significantly greater in the S. superba-derived biochar treatment than in the C. lanceolata-derived biochar treatment, while it was significantly decreased with the pyrolysis temperature in the two feedstock treatments (p < 0.05). Besides, the cumulative amount of CO2 dervied from native SOM was significantly reduced after biochars amendment (except for SS750), and the C. lanceolata-derived biochar treatments had a greater influence and achieved the lowest amount after the biochar produced at 550°C. Surprisingly, the biochar stimulated and suppressed the priming effect on CO2 production from native SOM during the early and later stages of incubation, respectively (Figure 2). Biochars prepared at 750°C showed the greatest positive priming effect in the two feedstock treatments, with 6.3–71.9% (S. superba) and 38.0% (C. lanceolata), respectively. During the whole 112 days experiment, both S. superba and C. lanceolata-derived biochars produced at 550 °C had the most significant negative priming effect, with −10.9% and −15.0%, respectively.
FIGURE 1. Cumulative amount of CO2 derived from two sources with different biochars. Data are mean ± standard deviation (n = 3). Blank and grey bars represent the cumulative amount of CO2 derived from native SOM and biochars, respectively. Their significant differences among different treatments were also indicated by lowercase and uppercase letters, respectively (at p < 0.05 level). CK: the soil with no amendment as control; SS350: soil with 350°C S. superba biochar amendment; SS550: soil with 550°C S. superba biochar amendment; SS750: soil with 750°C S. superba biochar amendment; SC350: soil with 350°C C. lanceolata biochar amendment; SC550: soil with 550 °C C. lanceolata biochar amendment; SC750: soil with 750°C C. lanceolata biochar amendment.
FIGURE 2. Priming effects of biochar amendment over incubation time. Data are mean ± standard deviation (n = 3).
The contents of soil total PLFA, G+, G−, and ACT, the G+/G− and Fungi/Bacteria ratios were significantly affected by the biochar pyrolysis temperature, while the effects of feedstock and their interactions were not significant (Supplementary Table S2). Specifically, both S. superba and C. lanceolata-derived biochars prepared at 350°C significantly reduced the contents of total PLFA compared with CK treatment. Furthermore, the PLFA contents of other microbial groups (G+, G−, bacteria, fungi and ACT) tended to be lowest in the 350°C treatments, especially in the C. lanceolata-derived biochar treatment, which was significantly lower than in the control treatment (Figure 3). Similarly, the principal component analysis (PCA) also showed the microbial community structure was only affected by biochar pyrolysis temperature rather than biochar feedstock and their interaction (Figure 4; Supplementary Tables S3, S4), with the total variance explained by the first two axes was 51.79% (30.88% axis 1 and 20.91% axis 2).
FIGURE 3. Microbial PLFA content of the different treatments. Data are mean ± standard deviation (n = 3). Different letters indicate significant differences between the treatments (p < 0.05).
The influences of soil microorganisms on C mineralization in the two feedstock treatments were different (Table 2). The correlation analysis showed that the cumulative amounts of CO2 derived from biochar and native SOM were negatively correlated (p < 0.01) with the total PLFA as well as other microbial groups for C. lanceolata-derived biochar treatment but did not correlate with microbial groups for S. superba-derived biochar treatment (Table 2). Soil properties also affected the C mineralization in the S. superba-derived biochar treatment. Specifically, the cumulative amount of CO2 derived from biochar was positively and negatively related to soil TN (p < 0.01) and C/N (p < 0.01), respectively. Furthermore, soil pH was negatively correlated (p < 0.01) with S. superba-derived biochar mineralization but positively correlated (p < 0.05) with the cumulative amount of CO2 derived from the biochar or native SOM in the C. lanceolata-derived biochar treatment.
TABLE 2. Correlation coefficients between the cumulative CO2 and the microbial PLFA content or soil properties (n = 9).
Our results showed that different types of feedstock and pyrolysis temperature in biochars would differently influence the mineralization of biochars (Figure 1). We found that the cumulative amount of CO2 derived from S. superba-derived biochar was higher than that from C. lanceolata-derived biochar at the same pyrolysis temperature. That may be because the S. superba is a broadleaved species, thus its biochar enriched with higher total N, volatile matter and DOC contents (Table 1), which are extremely unstable and can be preferentially utilized by soil microorganisms (Whitman et al., 2014). In contrast, the C. lanceolate is a conifer species, and its biochar has less volatile matter and nutrient to soil microorganisms, and therefore would have lower mineralization rate. Furthermore, we also found the cumulative amount of CO2 derived from biochars decreased with increasing pyrolysis temperature, which may result from the decreased volatile matter and DOC contents at higher temperatures. In addition, the higher-temperature biochars were more stable in the soil probably related to an increasing degree of aromaticity and aromatic condensation (Peng et al., 2011). This result agreed with Jindo and Sonoki (2019), which found that the stability of biochar derived from different feedstocks and increased with the increasing pyrolysis temperature.
Our study supports that biochar feedstock and pyrolysis temperature play important roles in determining the magnitude of priming effect. The C. lanceolata-derived biochar triggered the negative priming effect more faster and greater compared with the S. superba-derived biochar amendment. This phenomenon may be associated with the higher volatile matter and DOC contents of S. superba-derived biochar, which stimulated the special microbial activity related to the native SOM mineralization, then caused weaker negative priming effect. Further, we found biochars produced at 550°C regardless of the feedstock had the most notable influence compared to the other two temperature treatments. Such result was probably due to the fact that the biochar produced at intermediate temperature has a moderate proportion of unstable C and specific surface area to increase microbial habitability, which can slow down microbial degradation, then had a suppression effect on native SOM mineralization. Of note, for all treatments, the priming effect changed from positive to negative over time. This process may be caused by several interacting factors. Initially, an “apparent positive priming” effect would like to emerge, which can be linked to the accelerated turnover of native microbial biomass C (Blagodatskaya and Kuzyakov, 2008). Additionally, labile C in biochar can accelerate the activity of soil microorganisms in the early stage, then enhance the mineralization of native SOM, as a result of co-metabolism (Lehmann et al., 2011). In contrast, the negative effect at later stages may result from the stabilisation of native SOM through biochar-induced organo-mineral interactions and soil aggregation (Keith et al., 2011; Herath et al., 2013; Fang et al., 2015). Toxicity of biochar such as ethylene may be another negative priming mechanism, which has been shown to reduce microbial activities (Spokas et al., 2010). Moreover, Sagrilo et al. (2015) indicated that the negative effect may attribute to the labile fraction of biochar, which might be consumed in the later period. Similar to our study, Zimmerman et al. (2011) also found that there had a positive priming effect response to the application of biochars (oak and pine, 525°C) in the early stage (0–90 days), whereas turned to negative during the later phase, and they attributed this change to the evolution of the physical properties of biochar. Overall, we suggest that such transition is likely driven by the complicated interactions rather than a single mechanism.
Several studies suggested that biochar may stimulate soil microorganism activities and shift microbial community composition (Hardy et al., 2019; Tian et al., 2019). This may be owing to the improvement in the physical and chemical characteristics of the soil by biochar addition (Palansooriya et al., 2019). In our study, the response of soil microorganisms to biochar addition was mainly driven by the biochar pyrolysis temperature but largely independent of the feedstock and their interaction. Specifically, the biochars prepared at 350°C both significantly decreased the total PLFA contents, and the C. lanceolata-derived biochar produced at 350°C also significantly reduced all microbial PLFA contents compared with the control treatment. A possible reason for this is that the low temperature biochars contain toxic substances ranging from dioxins, furans, phenols and polyaromatic hydrocarbons to ethylene, some of which reduced microbial activity (Spokas et al., 2010; Zimmerman et al., 2011). Especially, both inhibitory and stimulatory effects have found to be associated with volatile matter (Zimmerman et al., 2011), which was most abundant in biochars produced at 350°C (Table 1). Furthermore, the observed decrease in PLFA content with biochar addition may also result from biochar sorption of PLFAs (Gomez et al., 2014).
We found a negative correlation between these PLFAs and the cumulative CO2 derived from biochar or native SOM in the C. lanceolata-derived biochar treatment, which was not significant in the S. superba-derived biochar treatment. This means the microbial PLFAs content may not significantly contribute to the C mineralization of biochar and native SOM after 112 days. These results are consistent with a recent study which showed the priming effect was not significantly associated with changes in microbial biomass (Liu et al., 2020). The reason is that the priming effect strongly depends on the stimulation of the specific microbial groups rather than the change of total microbial biomass (Shahzad et al., 2019). In contrast, we did find some relation between C mineralization and soil chemical properties in the S. superba-derived biochar treatment. The cumulative amount of CO2 derived from S. superba biochar was positively correlated with TN and negatively correlated with C/N ratio (Table 2). This result may be due to the fact that the biochar is a significant source of N for microorganisms, which influence C and N dynamics in the soil (Nelissen et al., 2012; Taghizadeh-Toosi et al., 2012). In addition, it is possible that the CO2 derived from the abiotic release of inorganic C contained in the biochar, likely present in the form of carbonates, and this process need an acidic environment (Jones et al., 2011), so there was a negative correlation between the cumulative amount of CO2 derived from biochar and soil pH in the S. superba-derived biochar treatment. However, it was a positive correlation in the C. lanceolata-derived biochar treatment. Therefore, the influence of soil pH on biochar decomposition is likely to be feedstock-specific.
In summary, our study found that both biochar feedstock and pyrolysis temperature significantly affected the C mineralization, while pyrolysis temperature also had a strong influence on the microbial communities. The mineralization of S. superba-derived biochar was greater than that of C. lanceolata-derived biochar due to the different nutrient contents between two tree species, while biochars produced at higher temperatures were more stable in the soil because of their low proportion of labile fraction. We also found biochars prepared at 550°C had the most significant negative priming effect in native SOM mineralization, while the microbial PLFA contents tended to be lowest after the addition of biochars produced at 350°C. As a result, we concluded that the biochar prepared at 550°C could have the best effect for soil C sequestration in the C. lanceolata plantation in such a short period, without significant effect on soil microbial community structure. Nevertheless, the long-term impacts of different types and application rates of biochar under various pyrolysis conditions in the field still need to be further investigated. Especially, the interactions of soil, plant and biochar may have complex influences on forest soil C sequestration, and their responses to global climate change (such as elevated atmospheric CO2, O3, or temperature, and N deposition) need to be better understood. With the development of this research field, the biochar amendment combined with other management practices (such as chemical fertilizer and forest residues) would be an important option to improve soil quality and sustainable forest plantations.
The original contributions presented in the study are included in the article/Supplementary Material, further inquiries can be directed to the corresponding author.
XL: wrote the first draft. YY: did experimental analysis. SL: revised the manuscript. HM: designed the study. RG: designed the study and revised the manuscript. YY: conceived and designed the study and revised the manuscript. All authors contributed to the article and approved the submitted version.
This work was supported by the National Natural Science Foundation of China (No. 31470628, 31570607, 31770659) and by the Public Interest Project of Fujian Province (2020R1002005).
The authors declare that the research was conducted in the absence of any commercial or financial relationships that could be construed as a potential conflict of interest.
All claims expressed in this article are solely those of the authors and do not necessarily represent those of their affiliated organizations, or those of the publisher, the editors and the reviewers. Any product that may be evaluated in this article, or claim that may be made by its manufacturer, is not guaranteed or endorsed by the publisher.
The Supplementary Material for this article can be found online at: https://www.frontiersin.org/articles/10.3389/fenvs.2021.717041/full#supplementary-material
Aoyama, M., Angers, D. A., N'dayegamiye, A., and Bissonnette, N. (2000). Metabolism of 13C-Labeled Glucose in Aggregates from Soils with Manure Application. Soil Biol. Biochem. 32, 295–300. doi:10.1016/S0038-0717(99)00152-2
Blagodatskaya, Е., and Kuzyakov, Y. (2008). Mechanisms of Real and Apparent Priming Effects and Their Dependence on Soil Microbial Biomass and Community Structure: Critical Review. Biol. Fertil. Soils 45 (2), 115–131. doi:10.1007/s00374-008-0334-y
Crombie, K., Mašek, O., Sohi, S. P., Brownsort, P., and Cross, A. (2013). The Effect of Pyrolysis Conditions on Biochar Stability as Determined by Three Methods. GCB Bioenergy 5 (2), 122–131. doi:10.1111/gcbb.12030
Cui, J., Ge, T., Kuzyakov, Y., Nie, M., Fang, C., Tang, B., et al. (2017). Interactions between Biochar and Litter Priming: A Three-Source 14C and δ13C Partitioning Study. Soil Biol. Biochem. 104, 49–58. doi:10.1016/j.soilbio.2016.10.014
Denef, K., Bubenheim, H., Lenhart, K., Vermeulen, J., Van Cleemput, O., Boeckx, P., et al. (2007). Community Shifts and Carbon Translocation within Metabolically-Active Rhizosphere Microorganisms in Grasslands under Elevated CO2. Biogeosciences 4, 769–779. doi:10.5194/bg-4-769-2007
Ding, Y., Liu, Y., Liu, S., Huang, X., Li, Z., Tan, X., et al. (2017). Potential Benefits of Biochar in Agricultural Soils: A Review. Pedosphere 27, 645–661. doi:10.1016/S1002-0160(17)60375-8
Duan, A., Lei, J., Hu, X., Zhang, J., Du, H., Zhang, X., et al. (2019). Effects of Planting Density on Soil Bulk Density, pH and Nutrients of Unthinned Chinese Fir Mature Stands in South Subtropical Region of China. Forests 10 (4), 351. doi:10.3390/f10040351
Fang, J., Guo, Z., Hu, H., Kato, T., Muraoka, H., and Son, Y. (2014). Forest Biomass Carbon Sinks in East Asia, with Special Reference to the Relative Contributions of forest Expansion and forest Growth. Glob. Change Biol. 20 (6), 2019–2030. doi:10.1111/gcb.12512
Fang, Y., Singh, B., and Singh, B. P. (2015). Effect of Temperature on Biochar Priming Effects and its Stability in Soils. Soil Biol. Biochem. 80, 136–145. doi:10.1016/j.soilbio.2014.10.006
Fatima, S., Riaz, M., Al-Wabel, M. I., Arif, M. S., Yasmeen, T., Hussain, Q., et al. (2021). Higher Biochar Rate Strongly Reduced Decomposition of Soil Organic Matter to Enhance C and N Sequestration in Nutrient-Poor Alkaline Calcareous Soil. J. Soils Sediments 21, 148–162. doi:10.1007/s11368-020-02753-6
Fowles, M. (2007). Black Carbon Sequestration as an Alternative to Bioenergy. Biomass and Bioenergy 31 (6), 426–432. doi:10.1016/j.biombioe.2007.01.012
Frostegård, Å., Tunlid, A., and Bååth, E. (2011). Use and Misuse of PLFA Measurements in Soils. Soil Biol. Biochem. 43 (8), 1621–1625. doi:10.1016/j.soilbio.2010.11.021
Gomez, J. D., Denef, K., Stewart, C. E., Zheng, J., and Cotrufo, M. F. (2014). Biochar Addition Rate Influences Soil Microbial Abundance and Activity in Temperate Soils. Eur. J. Soil Sci. 65 (1), 28–39. doi:10.1111/ejss.12097
Guillaume, T., Damris, M., and Kuzyakov, Y. (2015). Losses of Soil Carbon by Converting Tropical forest to Plantations: Erosion and Decomposition Estimated by δ 13 C. Glob. Change Biol. 21 (9), 3548–3560. doi:10.1111/gcb.12907
Guo, J. F., Chen, G. S., Xie, J. S., and Yang, Z. J. (2016). Clear-cutting and Slash Burning Effects on Soil CO2efflux Partitioning in Chinese Fir and evergreen Broadleaved Forests in Subtropical China. Soil Use Manage 32, 220–229. doi:10.1111/sum.12243
Hamer, U., Marschner, B., Brodowski, S., and Amelung, W. (2004). Interactive Priming of Black Carbon and Glucose Mineralisation. Org. Geochem. 35 (7), 823–830. doi:10.1016/j.orggeochem.2004.03.010.1016/j.orggeochem.2004.03.003
Hansen, V., Müller-Stöver, D., Munkholm, L. J., Peltre, C., Hauggaard-Nielsen, H., and Jensen, L. S. (2016). The Effect of Straw and wood Gasification Biochar on Carbon Sequestration, Selected Soil Fertility Indicators and Functional Groups in Soil: an Incubation Study. Geoderma 269, 99–107. doi:10.1016/j.geoderma.2016.01.033
Hardy, B., Sleutel, S., Dufey, J. E., and Cornelis, J.-T. (2019). The Long-Term Effect of Biochar on Soil Microbial Abundance, Activity and Community Structure Is Overwritten by Land Management. Front. Environ. Sci. 7, 110. doi:10.3389/fenvs.2019.00110
Herath, H. M. S. K., Camps-Arbestain, M., and Hedley, M. (2013). Effect of Biochar on Soil Physical Properties in Two Contrasting Soils: an Alfisol and an Andisol. Geoderma 209-210, 188–197. doi:10.1016/j.geoderma.2013.06.016
Huang, J., Lin, T.-C., Xiong, D., Yang, Z., Liu, X., Chen, G., et al. (2019). Organic Carbon Mineralization in Soils of a Natural forest and a forest Plantation of southeastern China. Geoderma 344, 119–126. doi:10.1016/j.geoderma.2019.03.012
Jindo, K., and Sonoki, T. (2019). Comparative Assessment of Biochar Stability Using Multiple Indicators. Agronomy 9 (5), 254. doi:10.3390/agronomy9050254
Jones, D. L., Murphy, D. V., Khalid, M., Ahmad, W., Edwards-Jones, G., and DeLuca, T. H. (2011). Short-term Biochar-Induced Increase in Soil CO2 Release Is Both Biotically and Abiotically Mediated. Soil Biol. Biochem. 43, 1723–1731. doi:10.1016/j.soilbio.2011.04.018
Keith, A., Singh, B., and Singh, B. P. (2011). Interactive Priming of Biochar and Labile Organic Matter Mineralization in a Smectite-Rich Soil. Environ. Sci. Technol. 45 (22), 9611–9618. doi:10.1021/es202186j
Kuzyakov, Y., Friedel, J. K., and Stahr, K. (2000). Review of Mechanisms and Quantification of Priming Effects. Soil Biol. Biochem. 32, 1485–1498. doi:10.1016/S0038-0717(00)00084-5
Kuzyakov, Y., Subbotina, I., Chen, H., Bogomolova, I., and Xu, X. (2009). Black Carbon Decomposition and Incorporation into Soil Microbial Biomass Estimated by 14C Labeling. Soil Biol. Biochem. 41, 210–219. doi:10.1016/j.soilbio.2008.10.016
Lehmann, J., Rillig, M. C., Thies, J., Masiello, C. A., Hockaday, W. C., and Crowley, D. (2011). Biochar Effects on Soil Biota - A Review. Soil Biol. Biochem. 43, 1812–1836. doi:10.1016/j.soilbio.2011.04.022
Leng, L., Huang, H., Li, H., Li, J., and Zhou, W. (2019). Biochar Stability Assessment Methods: A Review. Sci. Total Environ. 647, 210–222. doi:10.1016/j.scitotenv.2018.07.402
Li, Y., Li, Y., Chang, S. X., Yang, Y., Fu, S., Jiang, P., et al. (2018). Biochar Reduces Soil Heterotrophic Respiration in a Subtropical Plantation through Increasing Soil Organic Carbon Recalcitrancy and Decreasing Carbon-Degrading Microbial Activity. Soil Biol. Biochem. 122, 173–185. doi:10.1016/j.soilbio.2018.04.019
Li, Y., Zhou, C., Qiu, Y., Tigabu, M., and Ma, X. (2019). Effects of Biochar and Litter on Carbon and Nitrogen Mineralization and Soil Microbial Community Structure in a China Fir Plantation. J. For. Res. 30 (5), 1913–1923. doi:10.1007/s11676-018-0731-5
Lin, Z., Liu, Q., Liu, G., Cowie, A. L., Bei, Q., Liu, B., et al. (2017). Effects of Different Biochars on Pinus Elliottii Growth, N Use Efficiency, Soil N 2 O and CH 4 Emissions and C Storage in a Subtropical Area of China. Pedosphere 27 (2), 248–261. doi:10.1016/S1002-0160(17)60314-X
Liu, X.-J. A., Finley, B. K., Mau, R. L., Schwartz, E., Dijkstra, P., Bowker, M. A., et al. (2020). The Soil Priming Effect: Consistent across Ecosystems, Elusive Mechanisms. Soil Biol. Biochem. 140, 107617. doi:10.1016/j.soilbio.2019.107617
Luo, Y., Durenkamp, M., De NobiliLin, M., Lin, Q., and Brookes, P. C. (2011). Short Term Soil Priming Effects and the Mineralisation of Biochar Following its Incorporation to Soils of Different pH. Soil Biol. Biochem. 43, 2304–2314. doi:10.1016/j.soilbio.2011.07.020
Luo, Y., Lin, Q., Durenkamp, M., and Kuzyakov, Y. (2018). Does Repeated Biochar Incorporation Induce Further Soil Priming Effect?. J. Soils Sediments 18 (1), 128–135. doi:10.1007/s11368-017-1705-5
Luo, Y., Zang, H., Yu, Z., Chen, Z., Gunina, A., Kuzyakov, Y., et al. (2017). Priming Effects in Biochar Enriched Soils Using a Three-Source-Partitioning Approach: 14C Labelling and 13C Natural Abundance. Soil Biol. Biochem. 106, 28–35. doi:10.1016/j.soilbio.2016.12.006
Ma, H., Yin, Y., Gao, R., Taqi, R., and He, X. (2019). Response of Nitrogen Transformation to Glucose Additions in Soils at Two Subtropical forest Types Subjected to Simulated Nitrogen Deposition. J. Soils Sediments 19, 2166–2175. doi:10.1007/s11368-018-02237-8
Maestrini, B., Nannipieri, P., and Abiven, S. (2015). A Meta-Analysis on Pyrogenic Organic Matter Induced Priming Effect. GCB Bioenergy 7 (4), 577–590. doi:10.1111/gcbb.12194
Mitchell, P. J., Simpson, A. J., Soong, R., and Simpson, M. J. (2015). Shifts in Microbial Community and Water-Extractable Organic Matter Composition with Biochar Amendment in a Temperate forest Soil. Soil Biol. Biochem. 81, 244–254. doi:10.1016/j.soilbio.2014.11.017
Naisse, C., Girardin, C., Davasse, B., Chabbi, A., and Rumpel, C. (2015). Effect of Biochar Addition on C Mineralisation and Soil Organic Matter Priming in Two Subsoil Horizons. J. Soils Sediments 15, 825–832. doi:10.1007/s11368-014-1002-5
Nelissen, V., Rütting, T., Huygens, D., Staelens, J., Ruysschaert, G., and Boeckx, P. (2012). Maize Biochars Accelerate Short-Term Soil Nitrogen Dynamics in a Loamy Sand Soil. Soil Biol. Biochem. 55, 20–27. doi:10.1016/j.soilbio.2012.05.019
Palansooriya, K. N., Wong, J. T. F., Hashimoto, Y., Huang, L., Rinklebe, J., Chang, S. X., et al. (2019). Response of Microbial Communities to Biochar-Amended Soils: a Critical Review. Biochar 1, 3–22. doi:10.1007/s42773-019-00009-2
Peng, X., Ye, L. L., Wang, C. H., Zhou, H., and Sun, B. (2011). Temperature- and Duration-dependent rice Straw-Derived Biochar: Characteristics and its Effects on Soil Properties of an Ultisol in Southern China. Soil Tillage Res. 112, 159–166. doi:10.1016/j.still.2011.01.002
Post, W. M., Emanuel, W. R., Zinke, P. J., and Stangenberger, A. G. (1982). Soil Carbon Pools and World Life Zones. Nature 298, 156–159. doi:10.1038/298156a0
Quilliam, R. S., Marsden, K. A., Gertler, C., Rousk, J., DeLuca, T. H., and Jones, D. L. (2012). Nutrient Dynamics, Microbial Growth and weed Emergence in Biochar Amended Soil Are Influenced by Time since Application and Reapplication Rate. Agric. Ecosyst. Environ. 158 (1), 192–199. doi:10.1016/j.agee.2012.06.011
Rafael, R. B. A., Fernández-marcos, M. L., Cocco, S., Ruello, M. L., Fornasier, F., and Corti, G. (2019). Benefits of Biochars and NPK Fertilizers for Soil Quality and Growth of Cowpea (Vigna Unguiculata L. Walp.) in an Acid Arenosolfits of Biochars and NPK Fertilizers for Soil Quality and Growth of Cowpea (Vigna Unguiculata L. Walp.) in an Acid Arenosol. Pedosphere 29, 311–333. doi:10.1016/S1002-0160(19)60805-2
Rittl, T. F., Novotny, E. H., Balieiro, F. C., Hoffland, E., Alves, B. J. R., and Kuyper, T. W. (2015). Negative Priming of Native Soil Organic Carbon Mineralization by Oilseed Biochars of Contrasting Quality. Eur. J. Soil Sci. 66 (4), 714–721. doi:10.1111/ejss.12257
Sagrilo, E., Jeffery, S., Hoffland, E., and Kuyper, T. W. (2015). Emission of CO2from Biochar-Amended Soils and Implications for Soil Organic Carbon. GCB Bioenergy 7, 1294–1304. doi:10.1111/gcbb.12234
Semenov, A. V., Pereira e Silva, M. C., Szturc-Koestsier, A. E., Schmitt, H., Falcão Salles, J., and van Elsas, J. D. (2012). Impact of Incorporated Fresh 13C Potato Tissues on the Bacterial and Fungal Community Composition of Soil. Soil Biol. Biochem. 49, 88–95. doi:10.1016/j.soilbio.2012.02.016
Shahzad, T., Anwar, F., Hussain, S., Mahmood, F., Arif, M. S., Sahar, A., et al. (2019). Carbon Dynamics in Surface and Deep Soil in Response to Increasing Litter Addition Rates in an Agro-Ecosystem. Geoderma 333, 1–9. doi:10.1016/j.geoderma.2018.07.018
Spokas, K. A., Baker, J. M., and Reicosky, D. C. (2010). Ethylene: Potential Key for Biochar Amendment Impacts. Plant Soil 333 (1-2), 443–452. doi:10.1007/s11104-010-0359-5
Taghizadeh-Toosi, A., Clough, T. J., Sherlock, R. R., and Condron, L. M. (2012). Biochar Adsorbed Ammonia Is Bioavailable. Plant Soil 350 (1-2), 57–69. doi:10.1007/s11104-011-0870-3
Tian, X., Wang, L., Hou, Y., Wang, H., Tsang, Y. F., and Wu, J. (2019). Responses of Soil Microbial Community Structure and Activity to Incorporation of Straws and Straw Biochars and Their Effects on Soil Respiration and Soil Organic Carbon Turnover. Pedosphere 29, 492–503. doi:10.1016/S1002-0160(19)60813-1
Trumbore, S. (2006). Carbon Respired by Terrestrial Ecosystems - Recent Progress and Challenges. Glob. Change Biol 12 (2), 141–153. doi:10.1111/j.1365-2486.2006.01067.x
Vogel, J. G., He, D., Jokela, E. J., Hockaday, W., and Schuur, E. A. G. (2015). The Effect of Fertilization Levels and Genetic Deployment on the Isotopic Signature, Constituents, and Chemistry of Soil Organic Carbon in Managed Loblolly pine (Pinus Taeda L.) Forests. For. Ecol. Manag. 355, 91–100. doi:10.1016/j.foreco.2015.05.020
Wan, X., Huang, Z., He, Z., Yu, Z., Wang, M., Davis, M. R., et al. (2015). Soil C:N Ratio Is the Major Determinant of Soil Microbial Community Structure in Subtropical Coniferous and Broadleaf forest Plantations. Plant Soil 387 (1-2), 103–116. doi:10.1007/s11104-014-2277-4
Wan, X., Xiao, L., Vadeboncoeur, M. A., Johnson, C. E., and Huang, Z. (2018). Response of mineral Soil Carbon Storage to Harvest Residue Retention Depends on Soil Texture: a Meta-Analysis. For. Ecol. Manag. 408, 9–15. doi:10.1016/j.foreco.2017.10.028
Whitman, T., Enders, A., and Lehmann, J. (2014). Pyrogenic Carbon Additions to Soil Counteract Positive Priming of Soil Carbon Mineralization by Plants. Soil Biol. Biochem. 73, 33–41. doi:10.1016/j.soilbio.2014.02.009
Woolf, D., Amonette, J. E., Street-Perrott, F. A., Lehmann, J., and Joseph, S. (2010). Sustainable Biochar to Mitigate Global Climate Change. Nat. Commun. 1, 1–9. doi:10.1038/ncomms1053
Yang, X., Zhang, Q., Li, X., Jia, X., Wei, X., and Shao, M. A. (2015). Determination of Soil Texture by Laser Diffraction Method. Soil Sci. Soc. America J. 79 (6), 1556–1566. doi:10.2136/sssaj2015.04.0164
Yang, Y. S., Guo, J., Chen, G., Xie, J., Gao, R., Li, Z., et al. (2005). Carbon and Nitrogen Pools in Chinese Fir and evergreen Broadleaved Forests and Changes Associated with Felling and Burning in Mid-subtropical China. For. Ecol. Manag. 216, 216–226. doi:10.1016/j.foreco.2005.05.030
Yin, Y. F., Yang, Y. S., Gao, R., Ma, H. L., and Lu, Q. (2010). A Preliminary Study on Phyto-Enrichment 13C Labeling Technique. Acta Pedol Sin 47 (4), 790–793. doi:10.1080/00949651003724790
Yu, Y., Yang, J., Zeng, S., Wu, D., Jacobs, D. F., and Sloan, J. L. (2017). Soil pH, Organic Matter, and Nutrient Content Change with the Continuous Cropping of Cunninghamia Lanceolata Plantations in South China. J. Soils Sediments 17 (9), 2230–2238. doi:10.1007/s11368-016-1472-8
Yu, Z., Chen, L., Pan, S., Li, Y., Kuzyakov, Y., Xu, J., et al. (2018). Feedstock Determines Biochar-Induced Soil Priming Effects by Stimulating the Activity of Specific Microorganisms. Eur. J. Soil Sci. 69 (3), 521–534. doi:10.1111/ejss.12542
Yuan, P., Wang, J., Pan, Y., Shen, B., and Wu, C. (2019). Review of Biochar for the Management of Contaminated Soil: Preparation, Application and prospect. Sci. Total Environ. 659, 473–490. doi:10.1016/j.scitotenv.2018.12.400
Keywords: biochar, feedstock, pyrolysis temperature, priming effect, microbial community structure, mineralization
Citation: Lu X, Yin Y, Li S, Ma H, Gao R and Yin Y (2021) Effects of Biochar Feedstock and Pyrolysis Temperature on Soil Organic Matter Mineralization and Microbial Community Structures of Forest Soils. Front. Environ. Sci. 9:717041. doi: 10.3389/fenvs.2021.717041
Received: 30 May 2021; Accepted: 20 July 2021;
Published: 29 July 2021.
Edited by:
Lu-Jun Li, Northeast Institute of Geography and Agroecology (CAS), ChinaReviewed by:
Jun-Jian Wang, Southern University of Science and Technology, ChinaCopyright © 2021 Lu, Yin, Li, Ma, Gao and Yin. This is an open-access article distributed under the terms of the Creative Commons Attribution License (CC BY). The use, distribution or reproduction in other forums is permitted, provided the original author(s) and the copyright owner(s) are credited and that the original publication in this journal is cited, in accordance with accepted academic practice. No use, distribution or reproduction is permitted which does not comply with these terms.
*Correspondence: Ren Gao, cmVuLmdhb0Bmam51LmVkdS5jbg==; Yunfeng Yin, eXVuZmVuZ3lpbkAxNjMuY29t
Disclaimer: All claims expressed in this article are solely those of the authors and do not necessarily represent those of their affiliated organizations, or those of the publisher, the editors and the reviewers. Any product that may be evaluated in this article or claim that may be made by its manufacturer is not guaranteed or endorsed by the publisher.
Research integrity at Frontiers
Learn more about the work of our research integrity team to safeguard the quality of each article we publish.