- 1Departamento de Hidráulica e Saneamento, Escola de Engenharia de São Carlos, Universidade de São Paulo, São Carlos, Brazil
- 2Departamento de Geociências, Universidade Federal de São João del-Rei, São João del-Rei, Brazil
- 3Departamento de Ciências Ambientais, Universidade Federal de São Carlos, São Carlos, Brazil
Riparian areas are recognized for their buffering capacity regarding phosphorus and nitrogen from agricultural and urban runoff. However, their role in attenuating nutrient loads of rivers receiving point source nutrient inputs (e.g., from wastewater treatment plants, WWTPs) is still little understood. Here, we investigated whether ammonium (NH4-N), nitrate (NO3-N), and soluble reactive phosphorus (SRP) retention were influenced by the riparian land use in three Brazilian rivers receiving WWTP effluents. We hypothesized that nutrient attenuation would be potentially influenced by the hydrological connectivity between the main channel and riparian areas with native vegetation. We estimated retention from longitudinal patterns of dilution-corrected nutrient concentrations below the WWTPs. We assessed nutrient retention during periods with high (i.e., the wet) and low connectivity (i.e., the dry season). Relationships between non-conservative (nutrients) and conservative (chloride) solutes in both seasons were used to identify potential changes in the river chemistry due to the hydrological connectivity with the riparian areas. We also evaluated the relationship between net uptake velocities (Vf-net) and the accumulated percent native vegetation cover in the 100-m buffer using linear regressions, comparing the response for each nutrient between seasons with Analysis of Covariance. Slopes of regressions between nutrients and chloride significantly differed between seasons for NO3-N and SRP but not for NH4-N. The relationships between Vf-net and accumulated native vegetation in the riparian buffer presented steeper slopes for SRP in the wet than in the dry season. No significant relationships between NO3-N Vf-net and native vegetation cover were observed in either season. In contrast, increases in Vf-net with increasing vegetation cover were observed for NH4-N in the dry season. In periods with expected higher connectivity, NO3-N and SRP concentrations tended to be lower relative to chloride concentrations, with a potential effect of native vegetation in the riparian area on SRP retention. Our results suggest that seasonal connectivity between nutrient-rich river water and riparian areas is likely to induce changes in the predominant nutrient transformation processes, thereby favoring either nutrient retention or export in such rivers.
Introduction
Anthropogenic impacts, such as land-use change, water pollution, hydraulic alteration, and geomorphologic simplification, degrade freshwaters and adversely affect ecosystem services provided by natural aquatic systems, such as water supply and nutrient abatement (Dodds et al., 2013). Streams are not just the “gutters down which run the ruins of continents” (Leopold et al., 1964); they can effectively process and retain materials that enter them (e.g., Mulholland et al., 2008). Humans have exploited the natural self-purification capacity of streams and rivers for many decades. Wastewater treatment plants (WWTPs) are core components of sanitation infrastructure designed to improve wastewater quality before its discharge into surface waters. Nonetheless, depending on treatment technology and efficiency, treated effluents can still be a major source of several contaminants to the receiving aquatic systems (Meng et al., 2013; Mußmann et al., 2013; Carr et al., 2016; Aubertheau et al., 2017). Such inputs, for example, can induce a suite of changes in the water chemistry and ecosystem functioning in different ways, from shifts in nutrient availability shaping the biological community’s structure to alterations in biogeochemical cycles and nutrient export to downstream waters (Gücker et al., 2006, Gücker et al., 2011; Atashgahi et al., 2015; Rodriguez-Castillo et al., 2017; Bernal et al., 2020). The impacts of WWTP effluents can be especially relevant in developing countries with economic limitations, where the removal of several particulate/dissolved compounds may not or only partially occur (Oliveira and von Sperling, 2011). Moreover, the limited treatment efficiency of WWTPs can be magnified by relatively large effluent discharges in developing tropical countries experiencing high urbanization rates due to population growth and rural-urban migration (UNFPA, 2019). Thus, improving the understanding of the impacts of potential pollution point sources (e.g., WWTPs) on riverine functioning is crucial for better water resource management of urban and peri-urban areas.
The discharge of WWTP effluents can strongly alter the capacity of the receiving systems to transform, retain and remove nutrients, such as nitrogen (N) and phosphorus (P) (Gücker and Pusch, 2006; Carey and Migliaccio, 2009), thus altering their self-purifying capacity, which is an important ecosystem service (Costanza et al., 1997, 2014). Increasing loads of dissolved inorganic nitrogen (DIN) and soluble reactive phosphorus (SRP) from WWTPs may alter river features associated with nutrient retention. Excessive nutrient availability is associated with the decreased efficiency of biota to take up nitrate-nitrogen (NO3-N), ammonium-nitrogen (NH4-N), and SRP (Gücker et al., 2006; Ruggiero et al., 2006; Merseburger et al., 2011; Figueroa-Nieves et al., 2016). The internal release of nutrients due to mineralization or abiotic release processes and the reduced adsorption capacities of nutrient-saturated sediments are also associated with the loss of efficiency of receiving rivers (Wakelin et al., 2008; Acuña et al., 2019). Receiving rivers can also experience large shifts in nutrient cycling due to increased concentrations and large inputs of exogenous microorganisms from treatment processes (Mußmann et al., 2013; Merbt et al., 2014).
Land use in the riparian zone can play a pivotal role in the nutrient dynamics of aquatic systems. This area surrounding streams and rivers constitutes a hot spot for ecosystem processes because it can control the fluxes of material and energy between watersheds and rivers (Hoffmann et al., 2009; Peralta-Maraver et al., 2021). Land use patterns in the riparian zone, for instance, can explain more of the variation in water quality and ecosystem processes in comparison to the land use in the whole watershed (Vidon et al., 2010; Silva-Junior et al., 2014; Tromboni and Dodds, 2017). Likewise, land cover conversion in the watershed can overcome the capacity of riparian zones to protect streams and rivers depending on its intensity and coverage (Allan, 2004). Additionally, the length of the vegetated riparian corridor is likely to be more influential on river water quality than riparian width, as relatively narrow riparian corridors already can have substantial beneficial effects (Monteiro et al., 2016; Hilary et al., 2021). The importance of riparian areas to reduce N and P loads into aquatic systems relies on two main functions performed by these areas. First, riparian vegetation can act as a buffer preventing nutrient loads from non-point sources via agricultural and urban surface runoff. The consolidation of riparian buffers (permanently vegetated areas between rivers and the surrounding landscape) is among the nutrient mitigation best management practices (Dauwalter et al., 2018; Krzeminska et al., 2019), also in the context of restoration initiatives (McMillan et al., 2014; Johnson et al., 2016). Second, the lateral hydrological connectivity between riparian areas and the main channel can stimulate riparian biogeochemical processes that can reduce nutrient loads transported downstream (Reckendorfer et al., 2013; Vigiak et al., 2016; Covino, 2017). However, studies exploring the role of riparian hydrological connectivity on the nutrient abatement in streams and rivers are still rare and more knowledge is needed, especially regarding urban areas.
N and P retention mediated by riparian areas can vary widely and be difficult to predict due to the riparian structure’s inherent complexity (Hill, 1996; Hoffmann et al., 2009). The influence of riparian areas to reduce nutrient availability depends on factors such as the delivery pathway, the form of the delivered nutrient, the biogeochemical conditions in the riparian area, the level of hydrological connectivity with the main channel, the presence and structure of the riparian vegetation, and other environmental characteristics (Fisher and Acreman, 2004; Mayer et al., 2007; Roberts et al., 2012; Covino, 2017). Several retention mechanisms described in the literature are coupled with the presence of riparian areas. For instance, riparian plant communities can strongly affect retention performance by influencing runoff hydrology, evapotranspiration, nutrient uptake, aquatic metabolism, and soil nutrient recycling (Lupon et al., 2015; Dodds et al., 2017; Hille et al., 2018). The riparian corridor structure, in turn, shapes overall stream morphology and nutrient attenuation by controlling the distribution of sediments, habitat patches, water flow, transient storage, and redox conditions (Pinay et al., 2018). The long residence times of water column solutes within stream-riparian edges (Schade et al., 2005) and hyporheic zones (Harvey et al., 2013; Li et al., 2020) potentially reduces nutrient concentrations (e.g., Gücker and Boëchat, 2004). The riparian canopy also controls seasonal changes in stream metabolism by regulating light and organic matter inputs to the channel (Sabater et al., 2000; Heffernan and Cohen, 2010; Lupon et al., 2016). Ultimately, soil and redox characteristics can favor predominant oxic or anoxic processes, essential for sorption/desorption (Bol et al., 2018; Vidon et al., 2019). Together, these processes mediate the amount of nutrients reaching the stream, and consequently, the temporal and spatial patterns of watershed nutrient exports.
Most of the mechanisms involved in nutrient transformations and their mediation by riparian areas have been intensively studied for streams. In theory, the interaction between riparian vegetation and ecosystem processing in low-order systems would be more pronounced because such systems are narrower and thus more directly affected by riparian land cover. However, the effects of riparian areas on nutrient transformations remain unclear for higher-order rivers. In larger systems, the riparian areas expand to floodplains surrounding the river channels. The strength of connections (via lateral connectivity) influences the efficiency of nutrient and organic matter retention (McMillan and Noe, 2017). Hydrologic connections across floodplains can occur via surface flow (sensu flood pulse concept, Junk et al., 1989) and subsurface pathways (Ward and Stanford, 1995). Repeated cycles of connection/disconnection of floodplain areas with the main river are induced by fluctuations in water level (Tockner et al., 1999), which can favor organic matter and nutrient retention (Malard et al., 2002). During high flow periods, the river generally connects with the floodplain via overbank flow. Subsequently, the direction of the connection reverses during lower flow states, with floodplain groundwater sustaining the main channel base flow (Covino, 2017). This bidirectional movement facilitates the exchange of substantial amounts of water, sediment, organic matter, and nutrients among rivers, floodplains, and riparian systems. Floodplains often show a considerable retention capacity for particles and a significant denitrification potential in cases of high organic matter availability and groundwater levels (Fisher and Acreman, 2004; Hoffmann et al., 2009). However, depending on the frequency of the fluxes between the floodplain and main river, the release of substantial amounts of nutrients to the river can be observed due to N and P mineralization from riparian sediments (Schönbrunner et al., 2012; Weigelhofer et al., 2015).
Urbanization and especially channelization (e.g., through concrete walls) have reduced terrestrial/aquatic connectivity worldwide, thereby depriving rivers of their riparian retention structures (Beaulieu et al., 2014; Hope et al., 2014). The role of riparian buffers in controlling non-point sources of pollution (i.e., agricultural and urban runoff) has been extensively studied in the last decades, especially in temperate regions, but such information is still scarce for tropical regions (but see Leal et al., 2016; Taniwaki et al., 2017; Tromboni and Dodds, 2017; Pissarra et al., 2019). Further, we identified less information on the importance of riparian retention mechanisms to mediate declines of nutrient concentrations in both temperate and tropical rivers receiving point source pollution (e.g., WWTPs). This is particularly important for developing countries where urban development has moved towards riparian areas in the last decades, in addition to the increasing effluent discharge into aquatic systems. The importance of riparian areas for maintaining water quality is a topic especially relevant in Brazil, where our study was carried out, because the Brazilian Forest Code (Law Number 12651/2012) has reduced the overall protection of riparian forests (e.g., in terms of widths), with potential implications for river nutrients dynamics.
This study aimed to analyze whether NH4-N, NO3-N, and SRP declines were influenced by hydrological connectivity and riparian land use in three Brazilian 4th-order rivers receiving WWTP inputs. We estimated nutrient retention based on longitudinal nutrient concentration patterns in each river downstream of WWTPs following the nutrient spiraling concept (Newbold et al., 1981). We specifically focused on nutrient retention patterns during periods of high (i.e., rainy season) and low hydrological connectivity (i.e., dry season) due to fluctuations in river levels. As we did not directly measure biogeochemical processes in the riparian areas, we considered the changes in the conservative (i.e., biologically non-reactive) solute chloride (Stream Solute Workshop, 1990) as a proxy for identifying hydrochemical shifts due to the connectivity between riparian areas and river water. Changes in non-conservative solutes (i.e., biologically reactive nutrients) relative to conservative ones, in turn, were attributed to the occurrence of biogeochemical processes influencing increases or decreases of nutrient concentrations in the water column. We addressed the following research questions: 1) does the riparian land use along the studied reaches act as a potential control of nutrient abatement in tropical rivers receiving WWTP effluents? and 2) which nutrient abatement mechanisms are potentially related to the presence of native vegetation in riparian zones? Our study was based on the following hypotheses: 1) During periods of high connectivity between rivers and riparian zones (i.e., the rainy season with higher river water levels), conservative (i.e., chloride: Cl−) and non-conservative (i.e., nutrients) solutes should show divergent development of concentrations due to biogeochemical nutrient retention occurring in the riparian areas. The riparian retention mechanisms that potentially emerge from the connection would affect nutrient concentrations, whereas no changes in chloride concentrations would be expected. 2) In contrast, as a consequence of low connectivity in the dry season, we expected a more similar development of non-conservative and conservative solute concentrations due to reduced effects of riparian retention and the dominance of the effects of river transport processes on longitudinal solute patterns.
Materials and Methods
Study Sites
This study was conducted in the municipalities of São Carlos (Monjolinho and Quilombo rivers) and Vinhedo (Pinheiros River), both located in the central region of the state of São Paulo, southeastern Brazil. Over the last centuries, the landscape in this region has been strongly modified by deforestation, agriculture (primarily for sugarcane cultivation), and, more recently (especially after the 1950s), by industry and urbanization with potential impacts on the local water resources (Fracassi and Lollo, 2013; Gomes et al., 2019). We selected three 4th-order rivers to examine how seasonal riparian connectivity influenced nutrient retention downstream of WWTPs. The selected river catchments were representative of the Cerrado/Atlantic Rainforest biome transition. The elevation at our study sites ranged from 473 to 686 m.a.s.l. The climate in both the São Carlos and Vinhedo regions is defined as tropical with hot and wet summers and dry winters (i.e., Cwa, Köppen-Geiger’s classification; Kottek et al., 2006). A well-defined dry season occurs from April to September (fall to winter) and a rainy season from October to March (spring to summer). Total precipitation in the São Carlos region usually averages 1,400 mm year−1, with December and August as the wettest (276 mm) and driest (35 mm) months. In the Vinhedo region, the total precipitation averages 1,300 mm year−1, with January (226 mm) and May (45 mm) as the extremes (INMET, 2020).
We selected a reach located downstream the discharge of treated effluents from a WWTP in each receiving river. The total length of the reaches ranged from 600 m to 7.4 km depending on river current velocity and effluent discharge. We selected 4 to 6 sampling sites sufficiently downstream of each WWTP to ensure complete mixing between river water and effluents, allowing us to observe a significant decline in nutrient concentrations. The Monjolinho and Pinheiros Rivers had small tributaries that join the studied reach (two and one tributaries, respectively). However, their contribution to the main river’s discharge and nutrient and chloride contents was minimal (unpublished data), and the WWTP effluent was the major source of nutrients and chloride to these rivers.
We divided reaches below WWTPs into subreaches between the sampling sites (e.g., from WWTP to station 1, from station 1 to station 2, etc.) and delineated a 100-m buffer strip around the subreaches to characterize riparian land use (Figure 1). A riparian width of 100 m was chosen as the minimum width to accurately map riparian areas due to the resolution of available land use maps. This land-use characterization included four categories (i.e., urban area, native vegetation cover, agriculture, and pasture) and was restricted to the studied reaches below the respective WWTPs. The land use of the reaches’ entire drainage areas covered a wide array of urban, peri-urban, and natural conditions within the riparian areas (Supplementary Table S1).
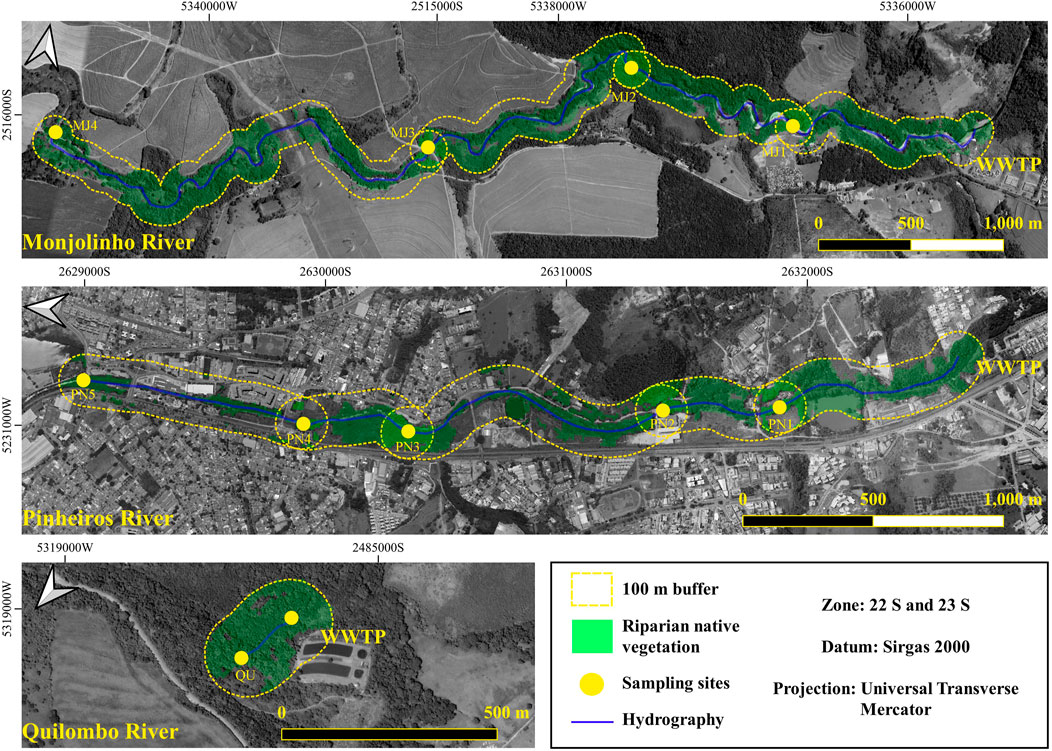
FIGURE 1. Reaches of Monjolinho, Pinheiros, and Quilombo rivers located below the WWTP and its respective sampling sites analyzed in this study. Sampling sites and native vegetation in the 100-m riparian buffer are indicated as yellow dots and green areas, respectively. MJ, Monjolinho; PN, Pinheiros; and QU, Quilombo.
The analyzed WWTPs had differences regarding the treatment technology (ARES-PCJ, 2020; SAAE, 2020). The biological treatment of the Monjolinho WWTP consisted of different modules of upflow anaerobic sludge blanket (UASB) reactors. The Santa Eudóxia WWTP discharged its effluents into the Quilombo River and performed biological treatment by anaerobic stabilization ponds followed by facultative ponds. The Pinheirinho WWTP performed biological treatment with activated sludge with extended aeration, discharging its effluents into the Pinheiros River. Among the studied WWTPs, only the Monjolinho WWTP effectively removed nutrients (i.e., tertiary treatment using dissolved air flotation technology, which removed, on average, 80% of total phosphorus). Moreover, the average volumes of treated effluent, removal efficiency, and post-treatment also differed among WWTPs (Table 1).
Data Collection
The field and sampling activities were carried out in March and July 2019, and March 2020. Total precipitation in the São Carlos and Vinhedo regions was 189.0 and 133.2 mm in March 2019, 36.2 and 68.8 mm in July 2019, and 94.8 and 113.0 mm in March 2020, respectively, (INMET, 2020). On each sampling date, we measured the river discharge upstream of the WWTP effluent discharge and at the downstream sampling sites. Discharge was estimated from width, depth, and water velocity measurements. Water velocity was the average of 10–15 evenly spaced measures performed in a cross-section of the river using a current meter. After collection, water samples were kept on ice and transported to the laboratory, where they were immediately filtered through fiberglass filters (GF/C Glass Microfiber Membranes, 0.45 μm, Whatman International, Kent, United Kingdom). Filtered samples were kept in the freezer at −18°C until analysis. Nutrient concentration samples were always collected and analyzed in triplicate via colorimetric methods. We determined NH4-N concentrations by the phenol hypochlorite method (Solorzano, 1969), modified for a 7 ml sample volume, NO3-N concentrations by the ultraviolet spectrophotometric screening method (American Public Health Association, 2012), SRP concentrations by the ascorbic acid method (American Public Health Association, 2012) and Cl− concentrations by the mercuric thiocyanate method (American Public Health Association, 2012).
Nutrient Retention Estimates
Nutrient retention for each river was estimated from the longitudinal patterns in dilution-corrected nutrient concentration along the study reach downstream the WWTPs (Martí et al., 2004; Haggard et al., 2005). Nutrient uptake length (SW) is a standard metric used to describe nutrient retention and transport. It can be estimated from combined short-term nutrient and conservative tracer additions (Mulholland et al., 2002). According to the nutrient spiraling approach (Newbold et al., 1981), SW is defined as the distance traveled by a nutrient atom before being removed from the water column by physical-chemical and biological mechanisms. Short SW indicates high nutrient retention. However, SW can be strongly influenced by stream velocity and depth, potentially leading to interpretation bias, especially in cross-site comparisons. The mass transfer coefficient or uptake velocity (Vf) normalizes SW for depth (d) and water velocity (u) (Vf = [u*d]/SW) and is thus more appropriate for comparing retention efficiency across streams (Stream Solute Workshop, 1990). Opposite to SW, greater Vf indicates higher retention.
Nutrient inputs from WWTPs can be substantial so that the effluent can represent a continuous nutrient source or injected solute, similar to a nutrient addition experiment. We used nutrient spiraling metrics and calculations to describe our results (Stream Solute Workshop 1990), but the interpretation is different from a typical nutrient addition experiment due to the permanent nature of the nutrient source. The longitudinal pattern in ambient concentrations downstream of the WWTP inputs reflects the net result of removal (e.g., biotic assimilation, denitrification, sorption) and release (e.g., mineralization) processes under long-term conditions, where nutrient levels are elevated beyond typical nutrient-saturated conditions (Haggard et al., 2001; Gibson and Meyer, 2007). Therefore, like in other studies on WWTP effects on nutrient spiraling (e.g., Haggard et al., 2001; Martí et al., 2004; Gibson and Meyer 2007; Figueroa-Nieves et al., 2016), we calculated the net nutrient retention metrics SW-net and Vf-net.
To estimate these metrics, we sampled water at several sites downstream of the WWTP (4–6 sampling sites). Lagrangian sample collection was performed within 2 h to ensure minimal variation in effluent volume and composition during sampling. We also collected samples from the WWTP output (i.e., effluents) and upstream of the effluent release. All water samples were analyzed for NH4-N, NO3-N, SRP, and Cl−. Considering that WWTP effluents can be a major source of Cl− (i.e., a conservative solute) to the stream, we used the downstream changes in Cl− concentrations to correct nutrient concentrations for dilution along the reach (Stream Solute Workshop, 1990).
The percentage of water dilution (D) along the reaches from the longitudinal decline in Cl− concentrations was estimated through Eq. 1 (Martí et al., 2004):
where Clx and Cl1 are Cl− concentrations at the sampling site x and at the top of the reach (where the effluent has completely mixed with the stream water).
The SW-net for each reach below the WWTP was estimated through Eq. 2.
where Cx is the nutrient concentration at distance x from the top of the study reach; C1 is the nutrient concentration at the top of the study reach; and k is the first-order uptake coefficient. We used simple linear regression (α = 0.05) of ln (dilution-corrected nutrient concentrations) versus downstream distance to estimate k. The negative inverse of the slope (–1/k) represents SW-net.
While the value of SW-net represents the retention of the whole study reach below the WWTP (i.e., considering all sampling sites), we calculated Vf-net for each sampling site (Eq. 3).
where Q is discharge and w is the average wetted width at each sampling site (Stream Solute Workshop 1990).
Statistical Analysis
We evaluated the relationship between non-conservative (NH4-N, NO3-N, SRP) and conservative (Cl−) solutes in the wet and dry seasons to identify changes in the river chemistry due to the potential hydrological connectivity with the riparian areas. Separate analyses of covariance (ANCOVA) were carried out for each nutrient to identify significant differences between seasons. We tested whether both the first-order uptake coefficient (k, Eq. 2) and net nutrient uptake lengths (SW-net) were related with both the nutrient concentrations upstream the WWTP and the response ratio ln (downstream/upstream), where upstream and downstream are the mean nutrient concentrations upstream the WWTP and at the first sampling station downstream the WWTP (i.e., C1 in Eq. 2), respectively (as also performed by Figueroa-Nieves et al., 2016). We also evaluated the relationship between Vf-net (representative of each sampling site) and the accumulated upstream percent cover of native vegetation in the 100-m buffer (total % of vegetated riparian area from the WWTP to the sampling station of interest) (Supplementary Figure S1). For this analysis, we conducted simple linear regressions and compared the response for each nutrient in both seasons with ANCOVA. All variables used in the linear models were ln-transformed, and the residuals were graphically checked to evaluate the premises. Results at p < 0.05 were considered significant. These analyses were carried out with the rstatix R package (Kassambara, 2021) in R (R Core Team, 2020).
Results
The river discharge (including sites upstream of the WWTP) varied from 0.2 to 7.5 m³ s−1 across all sampling sites, whereas river water velocity varied from 0.31 to 0.75 m s−1 (Table 2). Ambient nutrient concentrations (i.e., upstream of the WWTP outfall) were generally higher in the Monjolinho and Pinheiros Rivers than in the Quilombo River (Figure 2). Also, WWTP inputs raised nutrient levels more strongly in Pinheiros than in Monjolinho, whereas almost no effects were observed in the Quilombo River (Figure 2). Nutrient (non-conservative solutes) concentrations were positively related to chloride (conservative solute) in both the wet (high connectivity) and the dry (low connectivity) seasons (Figure 3). The relationship between NH4-N and Cl− did not differ between seasons, both in relation to slopes (F1,38 = 3.24, p > 0.05) and intercepts (F1,39 = 0.253, p > 0.05), suggesting negligible hydrological connectivity effects on NH4-N concentrations. In contrast, significant seasonal differences in slopes were observed for NO3-N (F1,38 = 5.54, p = 0.024) and SRP (F1,38 = 5.19, p = 0.028). Concentrations of both NO3-N and SRP were higher relative to chloride in periods of low connectivity (dry season) than in periods of high connectivity (29 and 46% higher, respectively), suggesting effects of riparian hydrological connectivity on decreased ambient concentrations for both nutrients.
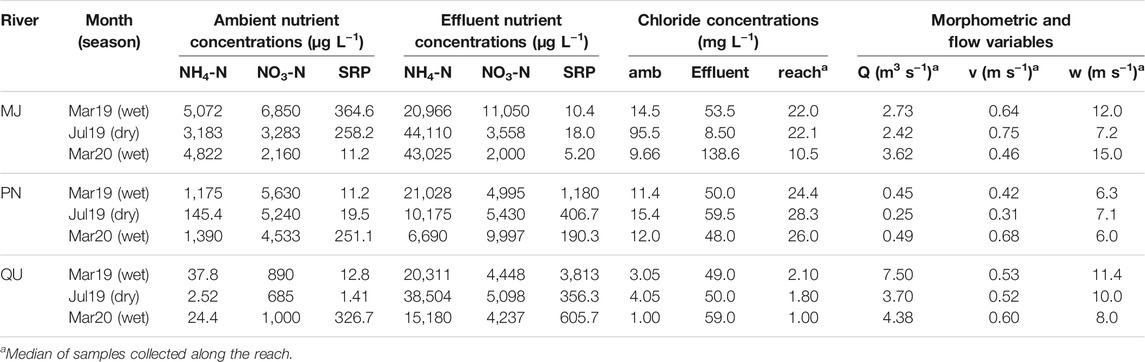
TABLE 2. Concentrations of NH4-N, NO3-N, SRP, and chloride, as well as morphometric and flow variables during three samplings across our rivers. For nutrients, we presented values for ambient (upstream WWTP) and effluent (at WWTP outfall) concentrations. For chloride, the ambient (amb), effluent, and the observed median values along the reach are shown. For discharge (Q), water velocity (v), and wetted width (w), the medians for all sampling sites are shown in each case.
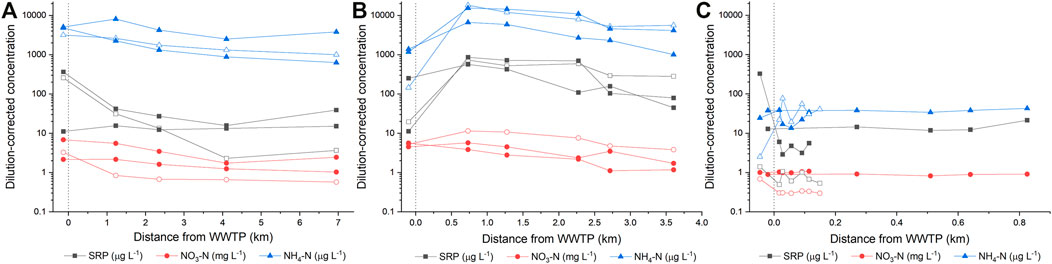
FIGURE 2. Variation of mean dilution-corrected nutrient concentrations upstream and downstream WWTPs (indicated by a dotted line) in the (A) Monjolinho, (B) Pinheiros, and (C) Quilombo river, sampled in periods of high (closed symbols) and low (open symbols) hydrological connectivity.
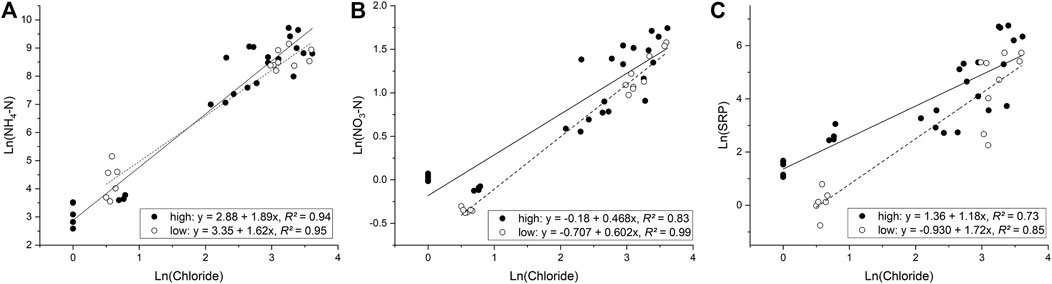
FIGURE 3. Relationships between ambient (A) NH4-N, (B) NO3-N, and (C) SRP concentrations and chloride during periods of high (closed circles) and low (open circles) hydrological connectivity at the sampling sites below WWTP inputs. Lines are fitted linear regressions for high (continuous lines) and low (dashed lines) connectivity periods.
Values for SW-net downstream the WWTP differed between nutrients and seasons for the three analyzed rivers (Table 3). In Monjolinho, similar values were found for NH4-N (ranging between 5.15 and 6.41 km) in the two seasons, but values for NO3-N in the dry season (17.24 km) were almost twice those in the wet season (Table 3). Moreover, in the dry season, SW-net for SRP was 2.48 km in the Monjolinho River, but in the wet season, no significant relationship was found between nutrient concentrations and distance from the WWTP, indicating no retention of this nutrient. In the Pinheiros River, consistent decreases were found for all nutrients, with similar values for NH4-N and NO3-N SW-net in both seasons, whereas SRP SW-net values were more than two times higher in the dry than in the wet season (Table 3). In contrast, no significant relationships were found for the studied nutrient forms in the Quilombo River. We observed that the effect of WWTP effluents on the increased nutrient concentrations was more apparent in some rivers than in others and varied depending on upstream conditions (Figure 4). There was a negative relationship between the first-order uptake coefficient (k) and NO3-N concentrations upstream of the WWTP, indicating background effects on the longitudinal pattern of concentrations (Figure 4A). We also found a negative relationship between k and the response ratio (downstream/upstream) for SRP (Figure 4B), indicating that both first-order uptake coefficient, and therefore SW-net, depended on how much the WWTP increased background SRP levels. Variation in k was not related to either NH4-N response ratio or upstream NH4-N concentrations. Likewise, WWTP effluents had little impact on nutrient concentrations for the Quilombo River, so that estimates of SW-net could not be calculated for that river. Consequently, we did not consider the data from this river for further analyses.
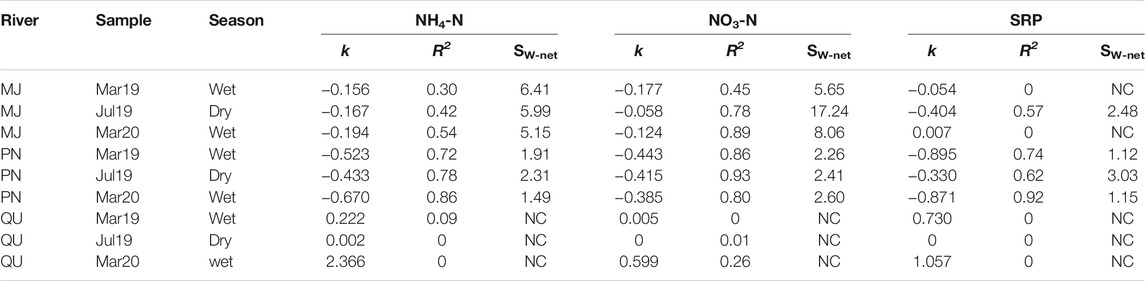
TABLE 3. Linear regression results to estimate net nutrient uptake lengths (SW-net) based on the first-order uptake coefficient (k). NC = no significant change in concentration observed along the studied reach.
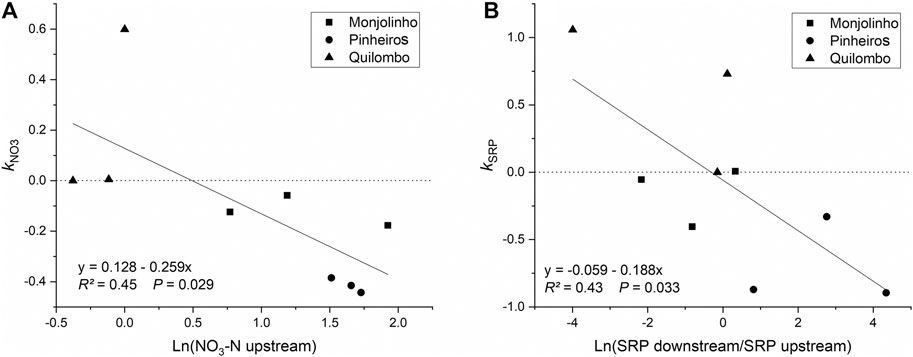
FIGURE 4. Relationship between the first-order uptake coefficient (k) and (A) upstream NO3-N concentrations and (B) SRP response ratios (downstream/upstream). Lines indicate linear regressions.
We found different relationships between Vf-net and the accumulated percent cover of riparian vegetation in the Monjolinho and Pinheiros rivers for the analyzed nutrient forms (Figures 5A–C). In the dry season, significant increases in Vf-net with increasing vegetation cover were observed for NH4-N, although no significant relationships were found in the wet season (Figure 5A). During the wet season, NH4-N Vf-net values were higher in areas with lower riparian vegetation cover (Figure 5A, left side), but as vegetation increased, velocities showed similar values compared to the dry season. There were no significant relationships between NO3-N Vf-net and accumulated percent cover of riparian vegetation in either season, but Vf-net values were higher in the wet than in the dry season (Figure 5B) (F1,15 = 18.3, p < 0.001). Since SW-net for SRP could not be estimated in the Monjolinho River in the wet season, we compared these relationships considering only sampling sites where effective nutrient retention occurred in both seasons (i.e., k < 0), though riparian vegetation cover in these cases was <33% (Figure 5C, left side). No significant differences in slopes (F1,11 = 0.048, p = 0.831) were found, but SRP Vf-net values were higher in the wet than in the dry season (F1,12 = 88.7, p < 0.001). These trends in Vf-net influenced the dilution-corrected concentrations of some nutrients so that concentrations declined with increasing vegetation cover along the 100-m buffer (Figures 5D–F). Decreases in NH4-N concentrations with increasing vegetation did not differ between seasons in relation to slopes (F1,23 = 2.25, p = 0.147) or intercepts (F1,24 = 0.255, p = 0.618), with a large variation during the wet season (Figure 5D). In contrast, there were significant differences in the slopes of the relationship for NO3-N (F1,23 = 25.46, p < 0.001), with a strong relationship in the dry season but no significant relationship in the wet season (Figure 5E). Finally, considering all data points for SRP, there were differences in slopes between seasons (F1,23 = 8.13, p = 0.009), with a steeper relationship in the dry season (Figure 5F).
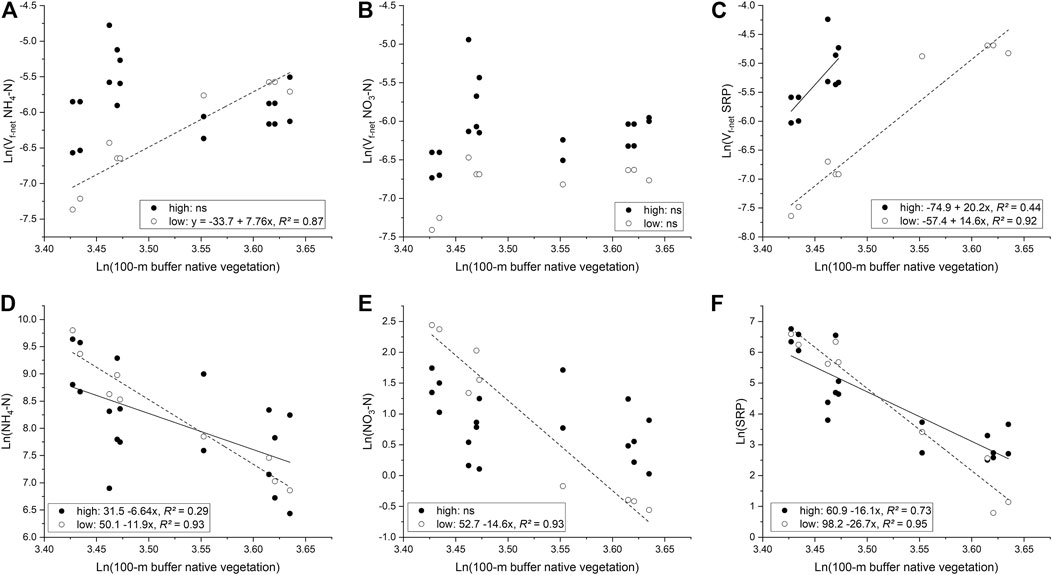
FIGURE 5. Relationships of net uptake velocities (Vf-net) of (A) NH4-N, (B) NO3-N, and (C) SRP, as well as dilution-corrected concentrations of (D) NH4-N, (E) NO3-N, and (F) SRP with the accumulated percent cover of native vegetation in the 100-m buffer during periods of high (closed circles) and low (open circles) hydrological connectivity at the sampling sites below the WWTP inputs. Lines are fitted linear regressions for high (continuous lines) and low (dashed lines) connectivity periods.
Discussion
This study showed that nutrient uptake downstream WWTPs could vary depending on the local environmental conditions and sampling period since one of the studied rivers did not present net uptake at all (i.e., Quilombo), whereas another river (Monjolinho) presented SRP uptake only in the dry season. This variation in nutrient uptake below WWTPs has been previously described in other studies. For example, Martí et al. (2004) found that from one-third to half of the studied streams in Catalonia presented no significant retention, whereas Figueroa-Nieves et al. (2016) found that net retention occurred in only about 13% of their studied streams in Puerto Rico. Our data showed that background concentrations (sampled upstream the WWTP) directly influenced NO3-N retention, with higher concentrations resulting in higher retention. In contrast, SRP downstream change was related to how much the WWTP increased background levels. For example, background concentrations were lower in the Quilombo River compared to the other rivers, and the WWTP did not raise nutrient concentrations enough to result in a nutrient uptake response. However, in the Monjolinho River, the WWTP did not raise background SRP concentrations significantly either, but the lack of significant SRP retention observed during the wet season was probably related to the connectivity of riparian areas and the main river channel, as discussed below.
Effects of Temporary Surface Water Connections With Riparian Areas on the Water Chemistry of Receiving Rivers
Although we acknowledge the limitation of this study regarding the small number of independent replicates (i.e., three tropical receiving rivers sampled in different seasons), we emphasize the significance of our data as the effects of point source pollution on nutrient processing in tropical rivers are still understudied. Additionally, our results showed nutrient concentration changed in the studied rivers between periods with different surface water connections to riparian areas. Especially for NO3-N and SRP, these changes were likely to arise from changes in biogeochemical processes due to the mixing effects between the main river and riparian waters during periods of higher hydrological connectivity (Lamers et al., 2006). While we did not investigate the processes underlying the observed hydrochemical changes, the different patterns observed between conservative and non-conservative solutes supported the hypothesis of altered biogeochemical processes due to connection. We found that in periods with expected higher connectivity (i.e., the wet season), NO3-N and SRP concentrations tended to be lower relative to chloride. This suggests that the investigated river-riparian systems may influence the observed declines in NO3-N and SRP contents during the wet season with the potential effect of riparian native vegetation on the retention process. During increases in water level due to precipitation events, river water initially floods riparian areas and is later exchanged in both directions. As the water level decreases, the riparian area and the main channel disconnect and become discrete compartments. The inherent characteristics of riparian areas differ from those of the main channel and may induce new biogeochemical processes which potentially modify the water chemistry in these zones. The main channel and riparian waters are then mixed again as a new connection arises in the short-term (i.e., hours or days) with consequential increases or decreases in nutrient availability of river water (Tockner et al., 1999). As we did not directly measure temporary water connectivity, for example, using water level variation data, we used the relationships between nutrients and chloride to assess hydrological connectivity indirectly (e.g., Weigelhofer et al., 2015). However, the riparian zone was indeed flooded and interacting with our rivers as overbank flow and the flooding of extensive floodplain areas during the rainy season was visually confirmed in the field. The potential mechanisms that occurred from this connection and might have influenced the observed declines in nutrient concentrations are shortly discussed below. We emphasize that further investigations are needed to confirm the potential processes influencing nutrient retention in riparian-river systems subject to high loads of nutrients, with focus on the main drivers of the biogeochemical mechanisms that emerge due to temporary water connection.
We observed SRP Vf-net increase with increasing native vegetation cover in the 100-m buffer during periods of both high and low hydrological connectivity, with higher values during the wet season. Additionally, SRP concentrations also decreased compared to chloride during the wet season. Together these results suggest that the vegetated riparian areas of the studied rivers potentially contribute to declines in SRP concentrations in the water column. Our results contrast with previous studies that have linked P mineralization (i.e., the release of inorganic phosphorus from sediments) in floodplain waterbodies to greater organic matter/nutrient contents, aerobic conditions, and moisture in riparian waters (Noe et al., 2013; Weigelhofer et al., 2015). In our receiving rivers, we expected that increases in nutrient and DOC (dissolved organic carbon) availability in the floodplain due to the surface water connection would increase SRP in the water column due to mineralization, especially during the wet season when connections are likely to occur. We suggest that the positive effect of vegetated riparian areas, decreasing SRP concentrations, could be associated with other retention processes such as sedimentation, assimilation, or adsorption. Since most phosphorus travels downstream bound to sediments or organic matter, the importation of the main river’s water into the floodplain may enhance the trapping of sediment, which has already been noted as an important mechanism of retention in riparian areas (Monteiro et al., 2016; Vigiak et al., 2016). In addition, total phosphorus within the floodplains can also be taken up, especially by herbaceous vegetation (Lyons et al., 2001), or be mineralized to SRP and later attached to clay particles through adsorption (Hoffmann et al., 2009). Floodplain sediments are typically effective in retaining total phosphorus but may have little effect on net dissolved phosphorus retention due to potential saturation, which will depend on factors such as cation exchange capacity or redox potential (House, 2003). The sediment-bound phosphorus trapped by riparian areas may slowly be leached into the stream, especially once the soil becomes P-saturated (Schönbrunner et al., 2012), though the river may still be protected from extreme nutrient pulses. Although we observed a potential positive effect of riparian areas on reducing phosphorus in the water column, riparian areas have the potential to act as a short-term sink for phosphorus, but in the long term, their effectiveness can be limited (Sharpley et al., 2013), especially in rivers receiving high P loads. Long-term management of phosphorus pollution should thus require effective on-site management of its sources (e.g., by controlling WWTP or urban runoff inputs).
We detected significant decreases in NO3-N concentration relative to chloride during the wet season compared to the dry season. However, we did not observe increases in NO3-N Vf-net with increases in the accumulated native vegetation in the 100-riparian buffers, suggesting partial effects of riparian areas on NO3-N retention. While we did not directly estimate denitrification rates, previous studies have recognized this process as the main pathway of permanent N-removal in both floodplains (Roley et al., 2012) and rivers receiving WWTP inputs (Lofton et al., 2007; Ribot et al., 2012), as the complete denitrification process releases nitrogen gas to the atmosphere. In riparian areas, denitrification drivers may include NO3-N availability (Gift et al., 2010) and soil moisture due to its influence on redox conditions, as well as organic matter content (Groffman and Crawford, 2003). Indirect drivers of floodplain denitrification potentially include the duration of inundation and the type of riparian vegetation (McMillan et al., 2014). We, therefore, expected that the temporary influx of nutrient-rich waters into the floodplain would influence NO3-N removal via denitrification since high loads of effluent NO3-N and organic carbon are likely to be supplied by WWTPs in addition to the nearly anoxic conditions in these zones. The lack of significant relationships between NO3-N Vf-net and the presence of natural vegetation in the 100-m riparian buffer (Figures 5B,E) did not permit to attribute such declines in NO3-N concentrations to denitrification mediated by riparian native vegetation. However, we still observed potential effects of hydrological connectivity on declines of NO3-N concentrations during the wet season (Figure 3B). One possibility to explain such relationship is the temporary mixing between the main river’s water and floodplain that may supply the water column with labile dissolved organic carbon produced in riparian soils (e.g., via primary production or benthic microbial mineralization; Preiner et al., 2008; Stanley et al., 2012) which is a more bioavailable form of carbon for denitrification (Pulou et al., 2012; Ribot et al., 2019). Likewise, the evidence of steeper declines of NO3-N concentrations during the dry season suggests the potential denitrification across our rivers in both seasons since this process depends on low DO availability and high NO3-N concentrations, which are expected in periods with low discharges and river water volume. Further studies are necessary to confirm these processes and more deeply explore the biochemical mechanisms involved in NO3-N removal.
In our study, we observed a potential influence of the riparian areas on the retention of both NO3-N and SRP during periods of expected surface water connection (i.e., the wet season), but no influence was observed on NH4-N retention. The lack of significant differences between NH4-N concentrations and chloride in the wet and dry seasons suggests that the lateral hydrological connectivity with riparian areas did not affect NH4-N retention. In rivers receiving effluents, nitrification (i.e., NH4-N oxidation) has been noted as an important mechanism of NH4-N retention (Krasner et al., 2009; Mußmann et al., 2013; Merbt et al., 2014). In turn, nitrification is not expected in riparian areas due to predominant anaerobic conditions and the ammonification process within this zone (Wolf et al., 2013). However, we found significant effects of the accumulated riparian native vegetation on increases in NH4-N Vf-net during periods with low hydrological connectivity (i.e., the dry season), suggesting an influence of riparian vegetation on nutrient retention. Riparian vegetation has already been described as an important driver of nutrient retention in natural streams as leaf litter during early fall and winter may increase water transient storage zones and promote the interaction between stream biota and fresh organic matter, affecting processes such as ecosystem respiration (Silva-Junior et al., 2014) and nitrification (Bernal et al., 2012). However, the effects of leaf litter on water quality in urbanized rivers are not fully understood (but see Duan et al., 2014). Likewise, more studies are needed for understanding the interactions between riparian vegetated areas and receiving rivers that may influence NH4-N retention.
Implications for River and Land Use Management
Our study encompassed rivers receiving WWTP inputs and spanning a gradient of land use in the riparian area. We observed that WWTP inputs had a considerable effect on river nutrient processing with potential implications for downstream nutrient exports, which has already been observed in other studies (Martí et al., 2004; Gücker et al., 2006; Figueroa-Nieves et al., 2016; Bernal et al., 2020). In our study, the retention mechanisms that potentially emerged from the surface water connection with riparian areas determined the role of these areas as sources or sinks of nutrients (Tockner et al., 1999). However, further investigations are necessary to confirm the potential processes governing nutrient retention, focusing on the controls of the main mechanisms that occur due to the temporary hydrologic connection in riparian-river systems subject to high loads of nutrients and carbon. In contrast, previous studies have already demonstrated the significant effects of land use in the watershed on nutrient concentrations across the river networks with urbanization related to increased nutrient concentrations, potentially due to urban runoff and illegal/unregulated sewage discharges (Tromboni and Dodds, 2017). Additionally, vegetation in riparian areas may act as a buffer preventing nutrient inputs from reaching the river network (see Meynendonckx et al., 2006; Dosskey et al., 2010; Lupon et al., 2017), or as a hotspot for nutrient processing controlled by the hydrological connectivity with the main river (Covino, 2017), as also suggested by our results. The benefits provided by the vegetation in riparian zones in maintaining water quality (by inducing new biogeochemical processes of nutrient retention and buffering nutrient loads from the drainage area) are thus noteworthy in rivers subject to both point and non-point sources of pollution, such as the ones we considered in our study. Despite their direct influences on nutrient processing, as riparian systems become more established with increasingly stable and robust vegetation, the quality and quantity of soil carbon increases, accelerating microbial activity and nutrient removal (McMillan and Noe, 2017). Nowadays, the conservation of riparian vegetation is especially critical in Brazil as the most recent Forest Code implies reductions in the legal requirements for maintaining both permanent protection areas and legal reserve areas. Important changes in the legal framework also include the exclusion of the permanent protection area class “hill tops” and the reduction of buffer strip width for small rivers.
Our results are also important from a water resources management perspective because they may contribute to global nutrient exportation models in larger watersheds (Kroeze et al., 2012). In general, such models usually focus on non-point pollution sources such as urban and agriculture runoff influencing retention and transport of nutrients along river networks (but see Mayorga et al., 2010). However, studies have indicated that point sources (e.g., WWTPs effluents) may also be a relevant driver of nutrient availability and quality impairment in rivers (Carey and Migliaccio, 2009; Hutchins et al., 2018). Furthermore, understanding the importance of riparian areas for river nutrient concentrations can be equally relevant to global nutrient exportation models. The inclusion of habitat-specific biochemical reactions such as those occurring in riparian systems may enhance the reliability and sensitivity of estimates, incorporating actual process-based data to predict the fate and transport of solutes affecting overall water quality (e.g., Hassanzadeh et al., 2019; Pissarra et al., 2019).
Despite the potential benefits of river-riparian connectivity indicated by our results, these connections have been impaired in many landscapes and rivers as a consequence of numerous circumstances in the last decades (Kondolf et al., 2006). For instance, flow regulation (i.e., by upstream dams and reservoirs) has severely decreased flow variability in many systems. In addition, channelization has eliminated the lateral connectivity of rivers, especially in urban and agricultural areas. Minimized flow variability and channelization are expected to create partially positive effects, especially in urban areas subject to social and economic losses due to flooding events, but deprive rivers of this biogeochemically reactive connection. In addition, climate change has increasingly altered the frequency of extreme and severe events such as drought or heavy rainfall leading to the modification of hydrological regimes of rivers worldwide. Changes in extreme weather events may pose stronger threats to ecosystem functioning than global trends and shifts in average conditions (Jentsch and Beierkuhnlein, 2008). Under this scenario, the likelihood of the river reconnecting to the riparian area becomes increasingly improbable in both small and high order systems (Wollheim et al., 2008; Wohl and Beckman, 2014) with implications at the ecosystem level. For instance, many aquatic systems require high flow events with overbank flooding to transport sediment, maintain high groundwater levels, and sustain floodplain surface-water bodies (see flood pulse concept; Junk et al., 1989). Disconnecting rivers and riparian areas can lead to the loss of retention capacity with substantial implications for the downstream transport of water, sediment, organic material, and nutrients, particularly in watersheds with various pollution sources along the drainage network. In summary, the disconnection of rivers and riparian areas coupled with climate change can become a challenge for restoring riparian areas and rivers receiving inputs from WWTPs. Restoration strategies should aim to reconstruct this critical reactive connection as well as flow dynamics (Palmer and Ruhi, 2019) in rivers worldwide to increase their capacity to retain nutrients and maintain other ecosystem functions.
Our study suggested that high nutrient loads from WWTP effluents could be either attenuated or exacerbated depending on the type of land cover in the riparian zones. Biogeochemical processes such as denitrification and P release, for example, may be influenced by nutrient and DOC inputs which are directly associated with the technologies used in the WWTP (i.e., secondary and tertiary treatment). Direct measurements of denitrification rates and other mechanisms are fundamental next steps to confirm the importance of riparian zones for nutrient retention in the studied tropical rivers. The different patterns observed in our study for NH4-N versus NO3-N and SRP concerning hydrological connectivity also indicated that the treatment technology could influence the processing of N in the receiving systems. For example, anaerobic biological treatment (e.g., UASB reactors) tends to release more NH4-N, whereas aerobic treatment (i.e., activated sludge) tends to release more NO3-N to the receiving watercourses (Metcalf and Eddy, 2016). Additionally, water bodies may have a relatively low capacity to deal with excess nutrients from point-source inputs (e.g., Gücker and Pusch 2006), and downstream export of nutrients could become especially relevant during low flow periods or severe nutrient discharge episodes. Similarly, WWTP management strategies should aim to increase the self-purifying capacity of the receiving rivers, considering environmental aspects, ethics, and legal constraints.
In summary, we argue that riparian areas are potentially related to decreasing nutrient concentrations downstream of WWTPs. Hydrological connectivity appeared to play a role in nutrient dynamics as the temporary water connection may import river compounds to be processed into the riparian areas. Both the riparian biogeochemical properties and characteristics of river water can determine the role of riparian areas as a sink or sources of nutrients to the water column and the consequential retention or export of nutrients. Furthermore, the consequences of such temporary connections may exceed those of pure mixing effects between riparian and main channel water and may even lead to long-term downstream eutrophication due to internal loading effects in both riparian and river systems. To understand the implications of hydrological connectivity for the water quality of highly impacted water bodies, especially those receiving effluents, further studies are needed focusing on the impact of river water pulses on benthic biogeochemical processes and the coupling with the water column in riparian systems.
Data Availability Statement
The original contributions presented in the study are included in the article/Supplementary Materials, further inquiries can be directed to the corresponding author.
Author Contributions
NF and DC designed the framework of the study, conducted fieldwork and analyzed data. NF coordinated analyses of dissolved nutrients of the samples, and also the modelling of nutrient uptake metrics. MF conducted analyses of land use. MT, IB, and BG revised statistical analyses. All authors discussed the results and commented on the article.
Funding
The authors are grateful to the São Paulo Research Foundation (FAPESP) for the PhD scholarship provided to NF (FAPESP 2018/13171-1) and for the regular research grants provided to DC a (FAPESP 2018/21412-9). DC also thanks the Brazilian National Council for Scientific and Technological Development (CNPq) for the productivity grant (CNPq 310844/2020-7).
Conflict of Interest
The authors declare that the research was conducted in the absence of any commercial or financial relationships that could be construed as a potential conflict of interest.
Publisher’s Note
All claims expressed in this article are solely those of the authors and do not necessarily represent those of their affiliated organizations, or those of the publisher, the editors and the reviewers. Any product that may be evaluated in this article, or claim that may be made by its manufacturer, is not guaranteed or endorsed by the publisher.
Supplementary Material
The Supplementary Material for this article can be found online at: https://www.frontiersin.org/articles/10.3389/fenvs.2021.709922/full#supplementary-material
Supplementary Figure 1 | Experimental design and procedures of the present study. (A) Details of the spiraling metrics (SW-net and Vf-net, net uptake length and net uptake velocity, respectively) calculated below each WWTP; and (B) estimation of land use in the riparian area between sampling sites. See the Methods section for more details. Qn: discharge at the sampling site n; 1 to n: sampling sites; x: downstream distance from the WWTP; Cx: nutrient concentration at sampling site of interest; C1: nutrient concentration at the first sampling site below WWTP; k: first-order uptake coefficient; Sw-net: net uptake length; Vf-net n: net uptake velocity at the sampling site n, wn: wetted width at the sampling site n; ARPn: accumulated percent of upstream land use in the 100-m buffer (from WWTP to the sampling site of interest) at the sampling site n.
References
Acuña, V., Casellas, M., Font, C., Romero, F., and Sabater, S. (2019). Nutrient Attenuation Dynamics in Effluent Dominated Watercourses. Water Res. 160, 330–338. doi:10.1016/j.watres.2019.05.093
Allan, J. D. (2004). Landscapes and Riverscapes: The Influence of Land Use on Stream Ecosystems. Annu. Rev. Ecol. Evol. Syst. 35, 257–284. doi:10.1146/annurev.ecolsys.35.120202.110122
American Public Health Association (2012). Standard Methods for the Examination of Water and Wastewater. 22nd ed. Washington: American Water Works Assn.
ARES-PCJ (2020). Agência Reguladora dos Serviços de Saneamento das Bacias dos Rios Piracicaba, Capivari e Jundiaí. Relatório Fisc. técnica dos Sist. água e esgoto Do município Vinhedo, 26. Available at: http://www.arespcj.com.br/arquivos/57190_relatório_de_não_conformidades_Vinhedo.pdf (Accessed July 15, 2021).
Atashgahi, S., Aydin, R., Dimitrov, M. R., Sipkema, D., Hamonts, K., Lahti, L., et al. (2015). Impact of a Wastewater Treatment Plant on Microbial Community Composition and Function in a Hyporheic Zone of a Eutrophic River. Sci. Rep. 5, 1–13. doi:10.1038/srep17284
Aubertheau, E., Stalder, T., Mondamert, L., Ploy, M.-C., Dagot, C., and Labanowski, J. (2017). Impact of Wastewater Treatment Plant Discharge on the Contamination of River Biofilms by Pharmaceuticals and Antibiotic Resistance. Sci. Total Environ. 579, 1387–1398. doi:10.1016/j.scitotenv.2016.11.136
Beaulieu, J. J., Mayer, P. M., Kaushal, S. S., Pennino, M. J., Arango, C. P., Balz, D. A., et al. (2014). Effects of Urban Stream Burial on Organic Matter Dynamics and Reach Scale Nitrate Retention. Biogeochemistry 121, 107–126. doi:10.1007/s10533-014-9971-4
Bernal, S., Drummond, J., Castelar, S., Gacia, E., Ribot, M., and Martí, E. (2020). Wastewater Treatment Plant Effluent Inputs Induce Large Biogeochemical Changes during Low Flows in an Intermittent Stream but Small Changes in Day-Night Patterns. Sci. Total Environ. 714, 136733. doi:10.1016/j.scitotenv.2020.136733
Bernal, S., Von Schiller, D., Martí, E., and Sabater, F. (2012). In-stream Net Uptake Regulates Inorganic Nitrogen export from Catchments under Base Flow Conditions. J. Geophys. Res. 117, a–n. doi:10.1029/2012JG001985
Bol, R., Gruau, G., Mellander, P.-E., Dupas, R., Bechmann, M., Skarbøvik, E., et al. (2018). Challenges of Reducing Phosphorus Based Water Eutrophication in the Agricultural Landscapes of Northwest Europe. Front. Mar. Sci. 5, 1–16. doi:10.3389/fmars.2018.00276
Carey, R. O., and Migliaccio, K. W. (2009). Contribution of Wastewater Treatment Plant Effluents to Nutrient Dynamics in Aquatic Systems: A Review. Environ. Manage. 44, 205–217. doi:10.1007/s00267-009-9309-5
Carr, S. A., Liu, J., and Tesoro, A. G. (2016). Transport and Fate of Microplastic Particles in Wastewater Treatment Plants. Water Res. 91, 174–182. doi:10.1016/j.watres.2016.01.002
Costanza, R., d'Arge, R., de Groot, R., Farber, S., Grasso, M., Hannon, B., et al. (1997). The Value of the World's Ecosystem Services and Natural Capital. Nature 387, 253–260. doi:10.1038/387253a0
Costanza, R., de Groot, R., Sutton, P., van der Ploeg, S., Anderson, S. J., Kubiszewski, I., et al. (2014). Changes in the Global Value of Ecosystem Services. Glob. Environ. Change 26, 152–158. doi:10.1016/j.gloenvcha.2014.04.002
Covino, T. (2017). Hydrologic Connectivity as a Framework for Understanding Biogeochemical Flux through Watersheds and along Fluvial Networks. Geomorphology 277, 133–144. doi:10.1016/j.geomorph.2016.09.030
Dauwalter, D. C., Fesenmyer, K. A., Miller, S. W., and Porter, T. (2018). Response of Riparian Vegetation, Instream Habitat, and Aquatic Biota to Riparian Grazing Exclosures. North. Am. J. Fish. Manage. 38, 1187–1200. doi:10.1002/nafm.10224
Dodds, W. K., Tromboni, F., Aparecido Saltarelli, W., and Fernandes Cunha, D. G. (2017). The Root of the Problem: Direct Influence of Riparian Vegetation on Estimation of Stream Ecosystem Metabolic Rates. Limnol. Oceanogr. 2, 9–17. doi:10.1002/lol2.10032
Dosskey, M. G., Vidon, P., Gurwick, N. P., Allan, C. J., Duval, T. P., and Lowrance, R. (2010). The Role of Riparian Vegetation in Protecting and Improving Chemical Water Quality in Streams1. J. Am. Water Resour. Assoc. 46, 261–277. doi:10.1111/j.1752-1688.2010.00419.x
Duan, S., Delaney-Newcomb, K., Kaushal, S. S., Findlay, S. E. G., and Belt, K. T. (2014). Potential Effects of Leaf Litter on Water Quality in Urban Watersheds. Biogeochemistry 121, 61–80. doi:10.1007/s10533-014-0016-9
Figueroa-Nieves, D., McDowell, W. H., Potter, J. D., and Martínez, G. (2016). Limited Uptake of Nutrient Input from Sewage Effluent in a Tropical Landscape. Freshw. Sci. 35, 12–24. doi:10.1086/684992
Fisher, J., and Acreman, M. C. (2004). Wetland Nutrient Removal: a Review of the Evidence. Hydrol. Earth Syst. Sci. 8, 673–685. doi:10.5194/hess-8-673-2004
Fracassi, P. C., and Lollo, J. A. (2013). Urban Sprawl in Small Cities, Analysis of the Municipality of São Pedro (Sp): Potentials and Constrains. J. Urb. Environ. Engng 7, 64–73. doi:10.4090/juee.2013.v7n1.064073
Gibson, C. A., and Meyer, J. L. (2007). Nutrient Uptake in a Large Urban River. J. Am. Water Resour. Assoc 43, 576–587. doi:10.1111/j.1752-1688.2007.00041.x
Gift, D. M., Groffman, P. M., Kaushal, S. S., and Mayer, P. M. (2010). Denitrification Potential, Root Biomass, and Organic Matter in Degraded and Restored Urban Riparian Zones. Restor. Ecol. 18, 113–120. doi:10.1111/j.1526-100X.2008.00438.x
Gomes, L., Simões, S., Dalla Nora, E., de Sousa-Neto, E., Forti, M., and Ometto, J. (2019). Agricultural Expansion in the Brazilian Cerrado: Increased Soil and Nutrient Losses and Decreased Agricultural Productivity. Land 8, 12–26. doi:10.3390/land8010012
Groffman, P. M., and Crawford, M. K. (2003). Denitrification Potential in Urban Riparian Zones. J. Environ. Qual. 32, 1144–1149. doi:10.2134/jeq2003.1144
Gücker, B., and Boëchat, I. G. (2004). Stream Morphology Controls Ammonium Retention in Tropical Headwaters. Ecology 85, 2818–2827. doi:10.1890/04-0171
Gücker, B., Brauns, M., and Pusch, M. T. (2006). Effects of Wastewater Treatment Plant Discharge on Ecosystem Structure and Function of lowland Streams. J. North Am. Benthological Soc. 25, 313–329. doi:10.1899/0887-3593(2006)25[313:eowtpd]2.0.co;2
Gücker, B., Brauns, M., Solimini, A. G., Voss, M., Walz, N., and Pusch, M. T. (2011). Urban Stressors Alter the Trophic Basis of Secondary Production in an Agricultural Stream. Can. J. Fish. Aquat. Sci. 68, 74–88. doi:10.1139/F10-126
Gücker, B., and Pusch, M. T. (2006). Regulation of Nutrient Uptake in Eutrophic lowland Streams. Limnol. Oceanogr. 51, 1443–1453. doi:10.4319/lo.2006.51.3.1443
Haggard, B. E., Stanley, E. H., and Storm, D. E. (2005). Nutrient Retention in a point-source-enriched Stream. J. North Am. Benthological Soc. 24, 29–47. doi:10.1899/0887-3593(2005)024<0029:NRIAPS>2.0.CO;2
Haggard, B. E., Storm, D. E., and Stanley, E. H. (2001). Effect of a point Source Input on Stream Nutrient Retention. J. Am. Water Resour. Assoc 37, 1291–1299. doi:10.1111/j.1752-1688.2001.tb03639.x
Harvey, J. W., Böhlke, J. K., Voytek, M. A., Scott, D., and Tobias, C. R. (2013). Hyporheic Zone Denitrification: Controls on Effective Reaction Depth and Contribution to Whole-Stream Mass Balance. Water Resour. Res. 49, 6298–6316. doi:10.1002/wrcr.20492
Hassanzadeh, Y. T., Vidon, P. G., Gold, A. J., Pradhanang, S. M., and Lowder, K. A. (2019). RZ-TRADEOFF: A New Model to Estimate Riparian Water and Air Quality Functions. Water 11, 769. doi:10.3390/w11040769
Heffernan, J. B., and Cohen, M. J. (2010). Direct and Indirect Coupling of Primary Production and Diel Nitrate Dynamics in a Subtropical spring-fed River. Limnol. Oceanogr. 55, 677–688. doi:10.4319/lo.2010.55.2.0677
Hilary, B., Chris, B., North, B. E., Angelica Maria, A. Z., Sandra Lucia, A. Z., Carlos Alberto, Q. G., et al. (2021). Riparian Buffer Length Is More Influential Than Width on River Water Quality: A Case Study in Southern Costa Rica. J. Environ. Manage. 286, 112132. doi:10.1016/j.jenvman.2021.112132
Hill, A. R. (1996). Nitrate Removal in Stream Riparian Zones. J. Environ. Qual. 25, 743–755. doi:10.2134/jeq1996.00472425002500040014x
Hille, S., Larsen, S. E., Rubæk, G. H., Kronvang, B., and Baattrup-Pedersen, A. (2018). Does Regular Harvesting Increase Plant Diversity in Buffer Strips Separating Agricultural Land and Surface Waters? Front. Environ. Sci. 6, 1–10. doi:10.3389/fenvs.2018.00058
Hoffmann, C. C., Kjaergaard, C., Uusi-Kämppä, J., Hansen, H. C. B., and Kronvang, B. (2009). Phosphorus Retention in Riparian Buffers: Review of Their Efficiency. J. Environ. Qual. 38, 1942–1955. doi:10.2134/jeq2008.0087
Hope, A. J., McDowell, W. H., and Wollheim, W. M. (2014). Ecosystem Metabolism and Nutrient Uptake in an Urban, Piped Headwater Stream. Biogeochemistry 121, 167–187. doi:10.1007/s10533-013-9900-y
House, W. A. (2003). Geochemical Cycling of Phosphorus in Rivers. Appl. Geochem. 18, 739–748. doi:10.1016/S0883-2927(02)00158-0
Hutchins, M. G., Abesser, C., Prudhomme, C., Elliott, J. A., Bloomfield, J. P., Mansour, M. M., et al. (2018). Combined Impacts of Future Land-Use and Climate Stressors on Water Resources and Quality in Groundwater and Surface Waterbodies of the Upper Thames River basin, UK. Sci. Total Environ. 631-632, 962–986. doi:10.1016/j.scitotenv.2018.03.052
INMET (2020). National Intitute of Metereology. Repos. Meteorol. Data – BDMEP. Available at: https://bdmep.inmet.gov.br/ (Accessed July 15, 2021).
Jentsch, A., and Beierkuhnlein, C. (2008). Research Frontiers in Climate Change: Effects of Extreme Meteorological Events on Ecosystems. Comptes Rendus Geosci. 340, 621–628. doi:10.1016/j.crte.2008.07.002
Junk, W. J., Bayley, P. B., and Sparks, R. E. (1989). The Flood Pulse Concept in River. Can. Spec. Publ. Fish. Aquat. Sci. 106, 110–127.
Kassambara, A. (2021). Rstatix: Pipe-Friendly Framework for Basic Statistical Tests for R. Available at:https://cran.r-project.org/package=rstatix.
Kondolf, G. M., Boulton, A. J., O'Daniel, S., Poole, G. C., Rahel, F. J., Stanley, E. H., et al. (2006). Process-based Ecological River Restoration: Visualizing Three-Dimensional Connectivity and Dynamic Vectors to Recover Lost Linkages. E&S 11. doi:10.5751/ES-01747-110205
Kottek, M., Grieser, J., Beck, C., Rudolf, B., and Rubel, F. (2006). World Map of the Köppen-Geiger Climate Classification Updated. metz 15, 259–263. doi:10.1127/0941-2948/2006/0130
Krasner, S. W., Westerhoff, P., Chen, B., Rittmann, B. E., Nam, S.-N., and Amy, G. (2009). Impact of Wastewater Treatment Processes on Organic Carbon, Organic Nitrogen, and DBP Precursors in Effluent Organic Matter. Environ. Sci. Technol. 43, 2911–2918. doi:10.1021/es802443t
Kroeze, C., Bouwman, L., and Seitzinger, S. (2012). Modeling Global Nutrient export from Watersheds. Curr. Opin. Environ. Sustainability 4, 195–202. doi:10.1016/j.cosust.2012.01.009
Krzeminska, D., Kerkhof, T., Skaalsveen, K., and Stolte, J. (2019). Effect of Riparian Vegetation on Stream Bank Stability in Small Agricultural Catchments. Catena 172, 87–96. doi:10.1016/j.catena.2018.08.014
Lamers, L. P. M., Loeb, R., Antheunisse, A. M., Miletto, M., Lucassen, E. C. H. E. T., Boxman, A. W., et al. (2006). Biogeochemical Constraints on the Ecological Rehabilitation of Wetland Vegetation in River Floodplains. Hydrobiologia 565, 165–186. doi:10.1007/s10750-005-1912-8
Leal, C. G., Pompeu, P. S., Gardner, T. A., Leitão, R. P., Hughes, R. M., Kaufmann, P. R., et al. (2016). Multi-scale Assessment of Human-Induced Changes to Amazonian Instream Habitats. Landscape Ecol. 31, 1725–1745. doi:10.1007/s10980-016-0358-x
Li, A., Bernal, S., Kohler, B., Thomas, S. A., Martí, E., and Packman, A. I. (2021). Residence Time in Hyporheic Bioactive Layers Explains Nitrate Uptake in Streams. Water Res. 57, e2020WR027646. doi:10.1029/2020wr027646
Lofton, D. D., Hershey, A. E., and Whalen, S. C. (2007). Evaluation of Denitrification in an Urban Stream Receiving Wastewater Effluent. Biogeochemistry 86, 77–90. doi:10.1007/s10533-007-9146-7
Lupon, A., Gerber, S., Sabater, F., and Bernal, S. (2015). Climate Response of the Soil Nitrogen Cycle in Three forest Types of a Headwater Mediterranean Catchment. J. Geophys. Res. Biogeosci. 120, 859–875. doi:10.1002/2014jg002791
Lupon, A., Martí, E., Sabater, F., and Bernal, S. (2016). Green Light: Gross Primary Production Influences Seasonal Stream N export by Controlling fine-scale N Dynamics. Ecology 97, 133–144. doi:10.1890/14-2296.1
Lupon, A., Sabater, F., and Bernal, S. (2017). The Influence of Mediterranean Riparian Forests on Stream Nitrogen Dynamics: A Review from a Catchment Perspective. Limnetica 36, 507–523. doi:10.23818/limn.36.18
Lyons, J., Thimble, S. W., and Paine, L. K. (2001). Grass versus Trees: Managing Riparian Areas to Benefits Stream Os Cnetral North America. J. Am. Water Resour. Assoc. 36, 919–930.
Malard, F., Tockner, K., Dole-Olivier, M.-J., and Ward, J. V. (2002). A Landscape Perspective of Surface-Subsurface Hydrological Exchanges in River Corridors. Freshw. Biol. 47, 621–640. doi:10.1046/j.1365-2427.2002.00906.x
Martí, E., Aumatell, J., Godé, L., Poch, M., and Sabater, F. (2004). Nutrient Retention Efficiency in Streams Receiving Inputs from Wastewater Treatment Plants. J. Environ. Qual. 33, 285–293. doi:10.2134/jeq2004.2850
Mayer, P. M., Reynolds, S. K., McCutchen, M. D., and Canfield, T. J. (2007). Meta-Analysis of Nitrogen Removal in Riparian Buffers. J. Environ. Qual. 36, 1172–1180. doi:10.2134/jeq2006.0462
Mayorga, E., Seitzinger, S. P., Harrison, J. A., Dumont, E., Beusen, A. H. W., Bouwman, A. F., et al. (2010). Global Nutrient Export from WaterSheds 2 (NEWS 2): Model Development and Implementation. Environ. Model. Softw. 25, 837–853. doi:10.1016/j.envsoft.2010.01.007
McMillan, S. K., and Noe, G. B. (2017). Increasing Floodplain Connectivity through Urban Stream Restoration Increases Nutrient and Sediment Retention. Ecol. Eng. 108, 284–295. doi:10.1016/j.ecoleng.2017.08.006
McMillan, S. K., Tuttle, A. K., Jennings, G. D., and Gardner, A. (2014). Influence of Restoration Age and Riparian Vegetation on Reach‐Scale Nutrient Retention in Restored Urban Streams. J. Am. Water Resour. Assoc. 50, 626–638. doi:10.1111/jawr.12205
Meng, F., Huang, G., Yang, X., Li, Z., Li, J., Cao, J., et al. (2013). Identifying the Sources and Fate of Anthropogenically Impacted Dissolved Organic Matter (DOM) in Urbanized Rivers. Water Res. 47, 5027–5039. doi:10.1016/j.watres.2013.05.043
Merbt, S. N., Auguet, J.-C., Blesa, A., Martí, E., and Casamayor, E. O. (2014). Wastewater Treatment Plant Effluents Change Abundance and Composition of Ammonia-Oxidizing Microorganisms in Mediterranean Urban Stream Biofilms. Microb. Ecol. 69, 66–74. doi:10.1007/s00248-014-0464-8
Merseburger, G., Martí, E., Sabater, F., and Ortiz, J. D. (2011). Point-source Effects on N and P Uptake in a Forested and an Agricultural Mediterranean Streams. Sci. Total Environ. 409, 957–967. doi:10.1016/j.scitotenv.2010.11.014
Metcalf, L., and Eddy, H. P. (2016). Tratamento de Efluentes e Recuperação de Recursos. 5 ed. Porto Alegre: AMGH.
Meynendonckx, J., Heuvelmans, G., Muys, B., and Feyen, J. (2006). Effects of Watershed and Riparian Zone Characteristics on Nutrient Concentrations in the River Scheldt Basin. Hydrol. Earth Syst. Sci. 10, 913–922. doi:10.5194/hess-10-913-2006
Monteiro, J. A. F., Kamali, B., Srinivasan, R., Abbaspour, K., and Gücker, B. (2016). Modelling the Effect of Riparian Vegetation Restoration on Sediment Transport in a Human-Impacted Brazilian Catchment. Ecohydrol. 9, 1289–1303. doi:10.1002/eco.1726
Mulholland, P. J., Tank, J. L., Webster, J. R., Bowden, W. B., Dodds, W. K., Gregory, S. V., et al. (2002). Can Uptake Length in Streams Be Determined by Nutrient Addition Experiments? Results from an Interbiome Comparison Study. J. North Am. Benthological Soc. 21, 544–560. doi:10.2307/1468429
Mußmann, M., Ribot, M., von Schiller, D., Merbt, S. N., Augspurger, C., Karwautz, C., et al. (2013). Colonization of Freshwater Biofilms by Nitrifying Bacteria from Activated Sludge. FEMS Microbiol. Ecol. 85, 104–115. doi:10.1111/1574-6941.12103
Newbold, J. D., Elwood, J. W., O'Neill, R. V., and Winkle, W. V. (1981). Measuring Nutrient Spiralling in Streams. Can. J. Fish. Aquat. Sci. 38, 860–863. doi:10.1139/f81-114
Newcomer Johnson, T., Kaushal, S., Mayer, P., Smith, R., and Sivirichi, G. (2016). Nutrient Retention in Restored Streams and Rivers: A Global Review and Synthesis. Water 8, 116–128. doi:10.3390/w8040116
Noe, G. B., Hupp, C. R., and Rybicki, N. B. (2013). Hydrogeomorphology Influences Soil Nitrogen and Phosphorus Mineralization in Floodplain Wetlands. Ecosystems 16, 75–94. doi:10.1007/s10021-012-9597-0
Oliveira, S. C., and von Sperling, M. (2011). Performance Evaluation of Different Wastewater Treatment Technologies Operating in a Developing Country. J. Water Sanit. Hyg. Dev. 1, 37–56. doi:10.2166/washdev.2011.022
Palmer, M., and Ruhi, A. (2019). Linkages between Flow Regime, Biota, and Ecosystem Processes: Implications for River Restoration. Science 365, eaaw2087. doi:10.1126/science.aaw2087
Peralta-Maraver, I., Stubbington, R., Arnon, S., Kratina, P., Krause, S., de Mello Cionek, V., et al. (2021). The Riverine Bioreactor: An Integrative Perspective on Biological Decomposition of Organic Matter across Riverine Habitats. Sci. Total Environ. 772, 145494. doi:10.1016/j.scitotenv.2021.145494
Pinay, G., Bernal, S., Abbott, B. W., Lupon, A., Marti, E., Sabater, F., et al. (2018). Riparian Corridors: A New Conceptual Framework for Assessing Nitrogen Buffering across Biomes. Front. Environ. Sci. 6, 1–11. doi:10.3389/fenvs.2018.00047
Pissarra, T. C. T., Valera, C. A., Costa, R. C. A., Siqueira, H. E., Martins Filho, M. V., Valle Júnior, R. F. d., et al. (2019). A Regression Model of Stream Water Quality Based on Interactions between Landscape Composition and Riparian Buffer Width in Small Catchments. Water 11, 1757. doi:10.3390/w11091757
Preiner, S., Drozdowski, I., Schagerl, M., Schiemer, F., and Hein, T. (2008). The Significance of Side-Arm Connectivity for Carbon Dynamics of the River Danube, Austria. Freshw. Biol. 53, 238–252. doi:10.1111/j.1365-2427.2007.01888.x
Pulou, J., Tournebize, J., Chaumont, C., Haury, J., and Laverman, A. M. (2012). Carbon Availability Limits Potential Denitrification in Watercress Farm Sediment. Ecol. Eng. 49, 212–220. doi:10.1016/j.ecoleng.2012.08.002
R Core Team (2020). R: A Language and Environment for Statistical Computing. Available at: https://www.r-project.org/.
Reckendorfer, W., Funk, A., Gschöpf, C., Hein, T., and Schiemer, F. (2013). Aquatic Ecosystem Functions of an Isolated Floodplain and Their Implications for Flood Retention and Management. J. Appl. Ecol. 50, 119–128. doi:10.1111/1365-2664.12029
Ribot, M., Cochero, J., Vaessen, T. N., Bernal, S., Bastias, E., Gacia, E., et al. (2019). Leachates from Helophyte Leaf-Litter Enhance Nitrogen Removal from Wastewater Treatment Plant Effluents. Environ. Sci. Technol. 53, 7613–7620. doi:10.1021/acs.est.8b07218
Ribot, M., Martí, E., Von Schiller, D., Sabater, F., Daims, H., and Battin, T. J. (2012). Nitrogen Processing and the Role of Epilithic Biofilms Downstream of a Wastewater Treatment Plant. Freshw. Sci. 31, 1057–1069. doi:10.1899/11-161.1
Roberts, W. M., Stutter, M. I., and Haygarth, P. M. (2012). Phosphorus Retention and Remobilization in Vegetated Buffer Strips: A Review. J. Environ. Qual. 41, 389–399. doi:10.2134/jeq2010.0543
Rodríguez-Castillo, T., Barquín, J., Álvarez-Cabria, M., Peñas, F. J., and Álvarez, C. (2017). Effects of Sewage Effluents and Seasonal Changes on the Metabolism of Three Atlantic Rivers. Sci. Total Environ. 599-600, 1108–1118. doi:10.1016/j.scitotenv.2017.05.067
Roley, S. S., Tank, J. L., and Williams, M. A. (2012). Hydrologic Connectivity Increases Denitrification in the Hyporheic Zone and Restored Floodplains of an Agricultural Stream. J. Geophys. Res. 117, a–n. doi:10.1029/2012JG001950
Ruggiero, A., Solimini, A. G., Anello, M., Romano, A., De Cicco, M., and Carchini, G. (2006). Nitrogen and Phosphorus Retention in a Human Altered Stream. Chem. Ecol. 22, S1–S13. doi:10.1080/02757540600556753
SAAE (2020). Autonomous Service of Water and Wastewater of São Carlos. Relatório Qual. Do Esgoto Tratado, São Carlos., 15. Available at: https://www.saaesaocarlos.com.br/saaesc/index.php/esgoto/qualidade-do-esgoto-tratado.
Sabater, F., Butturini, A., MartÍ, E., Muñoz, I., Romaní, A., Wray, J., et al. (2000). Effects of Riparian Vegetation Removal on Nutrient Retention in a Mediterranean Stream. J. North Am. Benthological Soc. 19, 609–620. doi:10.2307/1468120
Schade, J. D., Welter, J. R., Martí, E., and Grimm, N. B. (2005). Hydrologic Exchange and N Uptake by Riparian Vegetation in an Arid-Land Stream. J. North Am. Benthological Soc. 24, 19–28. doi:10.1899/0887-3593(2005)024<0019:heanub>2.0.co;2
Schönbrunner, I. M., Preiner, S., and Hein, T. (2012). Impact of Drying and Re-flooding of Sediment on Phosphorus Dynamics of River-Floodplain Systems. Sci. Total Environ. 432, 329–337. doi:10.1016/j.scitotenv.2012.06.025
Sharpley, A., Jarvie, H. P., Buda, A., May, L., Spears, B., and Kleinman, P. (2013). Phosphorus Legacy: Overcoming the Effects of Past Management Practices to Mitigate Future Water Quality Impairment. J. Environ. Qual. 42, 1308–1326. doi:10.2134/jeq2013.03.0098
Silva-Junior, E. F., Moulton, T. P., Boëchat, I. G., and Gücker, B. (2014). Leaf Decomposition and Ecosystem Metabolism as Functional Indicators of Land Use Impacts on Tropical Streams. Ecol. Indicators 36, 195–204. doi:10.1016/j.ecolind.2013.07.027
Solórzano, L. (1969). DETERMINATION of AMMONIA IN NATURAL WATERS by the PHENOLHYPOCHLORITE METHOD 1 1 This Research Was Fully Supported by U.S. Atomic Energy Commission Contract No. ATS (11-1) GEN 10, P.A. 20. Limnol. Oceanogr. 14, 799–801. doi:10.4319/lo.1969.14.5.0799
Stanley, E. H., Powers, S. M., Lottig, N. R., Buffam, I., and Crawford, J. T. (2012). Contemporary Changes in Dissolved Organic Carbon (DOC) in Human-Dominated Rivers: Is There a Role for DOC Management? Freshw. Biol. 57, 26–42. doi:10.1111/j.1365-2427.2011.02613.x
Stream Solute Workshop (1990). Concepts and Methods for Assessing Solute Dynamics in Stream Ecosystems. J. North. Am. Benthol. Soc. 9, 95–119. doi:10.2307/1467445
Taniwaki, R. H., Cassiano, C. C., Filoso, S., Ferraz, S. F. d. B., Camargo, P. B. d., and Martinelli, L. A. (2017). Impacts of Converting Low-Intensity Pastureland to High-Intensity Bioenergy Cropland on the Water Quality of Tropical Streams in Brazil. Sci. Total Environ. 584-585, 339–347. doi:10.1016/j.scitotenv.2016.12.150
Tockner, K., Pennetzdorfer, D., Reiner, N., Schiemer, F., and Ward, J. V. (1999). Hydrological Connectivity, and the Exchange of Organic Matter and Nutrients in a Dynamic River-Floodplain System (Danube, Austria). Freshw. Biol. 41, 521–535. doi:10.1046/j.1365-2427.1999.00399.x
Tromboni, F., and Dodds, W. K. (2017). Relationships between Land Use and Stream Nutrient Concentrations in a Highly Urbanized Tropical Region of Brazil: Thresholds and Riparian Zones. Environ. Manage. 60, 30–40. doi:10.1007/s00267-017-0858-8
UNFPA (2019). The State of World Population 2019. Available at: https://www.unfpa.org/sites/default/files/pub-pdf/UNFPA_PUB_2019_EN_State_of_World_Population.pdfhttps://www.unfpa.org/sites/default/files/sowp/downloads/UNFPA_PUB_2017_EN_SWOP.pdf.
Vidon, P., Allan, C., Burns, D., Duval, T. P., Gurwick, N., Inamdar, S., et al. (2010). Hot Spots and Hot Moments in Riparian Zones: Potential for Improved Water Quality Management1. J. Am. Water Resour. Assoc. 46, 278–298. doi:10.1111/j.1752-1688.2010.00420.x
Vidon, P. G., Welsh, M. K., and Hassanzadeh, Y. T. (2019). Twenty Years of Riparian Zone Research (1997-2017): Where to Next? J. Environ. Qual. 48, 248–260. doi:10.2134/jeq2018.01.0009
Vigiak, O., Malagó, A., Bouraoui, F., Grizzetti, B., Weissteiner, C. J., and Pastori, M. (2016). Impact of Current Riparian Land on Sediment Retention in the Danube River Basin. Sustainability Water Qual. Ecol. 8, 30–49. doi:10.1016/j.swaqe.2016.08.001
Wakelin, S. A., Colloff, M. J., and Kookana, R. S. (2008). Effect of Wastewater Treatment Plant Effluent on Microbial Function and Community Structure in the Sediment of a Freshwater Stream with Variable Seasonal Flow. Appl. Environ. Microbiol. 74, 2659–2668. doi:10.1128/AEM.02348-07
Ward, J. V., and Stanford, J. A. (1995). Ecological Connectivity in Alluvial River Ecosystems and its Disruption by Flow Regulation. Regul. Rivers: Res. Mgmt. 11, 105–119. doi:10.1002/rrr.3450110109
Weigelhofer, G., Preiner, S., Funk, A., Bondar-Kunze, E., and Hein, T. (2015). The Hydrochemical Response of Small and Shallow Floodplain Water Bodies to Temporary Surface Water Connections with the Main River. Freshw. Biol. 60, 781–793. doi:10.1111/fwb.12532
Wohl, E., and Beckman, N. D. (2014). Leaky Rivers: Implications of the Loss of Longitudinal Fluvial Disconnectivity in Headwater Streams. Geomorphology 205, 27–35. doi:10.1016/j.geomorph.2011.10.022
Wolf, K. L., Noe, G. B., and Ahn, C. (2013). Hydrologic Connectivity to Streams Increases Nitrogen and Phosphorus Inputs and Cycling in Soils of Created and Natural Floodplain Wetlands. J. Environ. Qual. 42, 1245–1255. doi:10.2134/jeq2012.0466
Keywords: tropical rivers, point source pollution, nutrient spiraling, biogeochemistry, river riparian connectivity, river management
Citation: Finkler NR, Gücker B, Boëchat IG, Ferreira MS, Tanaka MO and Cunha DGF (2021) Riparian Land Use and Hydrological Connectivity Influence Nutrient Retention in Tropical Rivers Receiving Wastewater Treatment Plant Discharge. Front. Environ. Sci. 9:709922. doi: 10.3389/fenvs.2021.709922
Received: 19 May 2021; Accepted: 26 August 2021;
Published: 22 September 2021.
Edited by:
Paola Passalacqua, University of Texas at Austin, United StatesReviewed by:
Isabelle Combroux, Université de Strasbourg, FranceFernando Mayer Pelicice, Federal University of Tocantins, Brazil
Copyright © 2021 Finkler, Gücker, Boëchat, Ferreira, Tanaka and Cunha. This is an open-access article distributed under the terms of the Creative Commons Attribution License (CC BY). The use, distribution or reproduction in other forums is permitted, provided the original author(s) and the copyright owner(s) are credited and that the original publication in this journal is cited, in accordance with accepted academic practice. No use, distribution or reproduction is permitted which does not comply with these terms.
*Correspondence: N. R. Finkler, bmljb2xhcy5maW5rbGVyQHVzcC5icg==