- 1GFL Environmental Inc., Toronto, ON, Canada
- 2School of Engineering, Newcastle University, Newcastle Upon Tyne, United Kingdom
- 3Department of Mechanical and Construction Engineering, Northumbria University, Newcastle Upon Tyne, United Kingdom
- 4Departamento de Engenharia Sanitária e Ambiental, Federal University of Minas Gerais (UFMG), Belo Horizonte, Brazil
The removal mechanisms and extent of degradation of 28 chemicals (triclosan, fifteen polycyclic aromatic hydrocarbons, four estrogens, and eight polybrominated diphenyl ether congeners) in different biological treatment systems [activated sludge, up-flow anaerobic sludge blanket reactor (UASB) and waste stabilization pond (WSP)] was investigated to provide insights into the limits of engineered biological treatment systems. This was done through degradation experiments with inhibition and abiotic controls in static reactors under aerobic and anaerobic conditions. Estrogens showed higher first order degradation rates (0.1129 h−1) under aerobic conditions with activated sludge inocula followed by low molecular weight (LMW) PAHs (0.0171 h−1), triclosan (0.0072 h−1), middle (MMW) (0.0054 h−1) and high molecular weight PAHs (HMW) (0.0033 h−1). The same trend was observed under aerobic conditions with a facultative inoculum from a WSP, although at a much slower rate. Biodegradation was the major removal mechanism for these chemicals in the activated sludge and WSP WWTPs surveyed. Photodegradation of these chemicals was also observed and varied across the group of chemicals (estrogens (light rate = 0.4296 d−1; dark = 0.3900 d−1) degraded faster under light conditions while reverse was the case for triclosan (light rate = 0.0566 d−1; dark = 0.1752 d−1). Additionally, all the chemicals were resistant to anaerobic degradation with UASB sludge, which implies that their removal in the UASB of the surveyed WWTP was most likely via sorption onto solids. Importantly, the first order degradation rate determined in this study was used to estimate predicted effluent concentrations (PECs). The PECs showed good agreement with the measured effluent concentrations from a previous study for these treatment systems.
Introduction
Knowledge on the most important mechanisms for micropollutant (chemical contaminants) removal, and the rate of those mechanisms is essential to understand the limits of current wastewater treatment technologies and determine how they might be improved. Biodegradation and sorption have been identified as the main mechanisms of xenobiotic removal in wastewater treatment plants, with volatilization only playing a minor role (Verlicchi et al., 2012). During biological wastewater treatment, micropollutants are either sorbed onto solids or biodegraded–via complete mineralization or incomplete degradation and formation of biotransformation products (Luo et al., 2014). Photodegradation has also been reported as a removal mechanism in waste stabilization ponds (Gomez et al., 2007) and high rate algal ponds (Villar-Navarro et al., 2018). Recently, Falas et al. demonstrated that biodegradation was the main fate mechanism for a group of ≥20 structurally diverse non-volatile low-sorbing pharmaceuticals in laboratory experiments using unacclimated sludge as an inoculum in different treatment configurations and conditions. However, there has been relatively little systematic research into the fate and mechanisms of chemicals across different use-classes, especially in the context of microbial inocula from range of real full-scale treatment processes (Falås et al., 2016).
In our previous work, the occurrence and removal of 28 chemicals from four different use-classes; steroidal hormones (estrogens- E1, E2, E3 and EE2), personal care products (triclosan), industrial chemicals and flame retardants (polyaromatic hydrocarbons PAHs; and polybrominated diphenyl ethers PBDEs) in different full-scale Brazilian wastewater treatment plants were reported (Komolafe et al., 2021). These plants differed in their treatment processes and energy requirements (from energy-intensive activated sludge systems, to low-energy UASBs plus trickling filters system, and passive energy waste stabilization ponds), and operate under various redox conditions (aerobic, anaerobic and facultative). It is important to get more detailed insight into the fate of the chemicals in these technologically diverse biological treatment systems in order to assess their limits in pursuit of more sustainable and capable removal systems. Such systems would benefit the globe especially low-middle income countries (LMICs) that have little/no wastewater treatment infrastructure but are exposed to an equal or even greater environmental and human health risk from micropollutants.
After initial sorption, biodegradation of estrogen, triclosan, and PAHs removal has been attributed to different mechanisms including deconjugation, co-metabolism with nitrifying biomass or heterotrophic bacteria (Ren et al., 2007; Haritash and Kaushik, 2009; Roh et al., 2009; Racz and Goel, 2010). Furthermore, removal pathways and degradation efficiencies of these chemicals in WWTPs are dependent on the prevailing redox conditions-aerobic, anoxic or anaerobic (Joss et al., 2004; Xue et al., 2010). A review by Liu et al. shows that several studies have compared the fate of estrogens under relevant aerobic and anaerobic wastewater treatment conditions (Liu et al., 2015). However, while the fate of triclosan under aerobic conditions is well documented, there is limited information on its fate under anaerobic conditions especially with anaerobic inocula from real WWTPs (Chen et al., 2011). Most studies on PAH biodegradation have focused on their anaerobic and aerobic digestion in sewage sludge rather than wastewater treatment (Trably et al., 2005; Cea-Barcia et al., 2013). There is also only limited work on the biodegradation of polybrominated diphenyl ethers (PBDEs) under aerobic and anaerobic conditions; Stiborova et al. quantified degradation of eight PBDE congeners by activated sludge under aerobic conditions (Stiborova et al., 2015). Furthermore, the fate of these chemicals in WSPs (waste stabilization ponds) in unknown.
The objective of this study was to systematically investigate the biodegradability of the selected group of micropollutants under different redox conditions (aerobic or anaerobic) using biomass from activated sludge, UASB reactors and WSP WWTPs, which are typical of those in warm LMICs. The photodegradation potential of the micropollutants was also investigated with biomass from the WSP. Understanding the extent of degradation (degradation rates) of these chemicals in aerobic and anaerobic systems is required to understand the limits of engineering biological systems (e.g., required hydraulic retention time, HRT) for their removal and predict effluent concentrations (PECs).
Experimental Methods
Materials
A standard solution of 15 mixed priority PAHs (including naphthalene, acenaphthylene, acenaphthene, fluorene, phenanthrene, anthracene, fluoranthene, pyrene, benz(a)anthracene, chrysene, benzo(b)fluoranthene, chrysene, indeno (1,2,3-cd)pyrene, dibenz (a,h)anthracene and benzo (ghi)perylene at 2 mg/ml in dichloromethane), and triclosan (100 mg) were purchased from Sigma, (United Kingdom). A certified standard solution mix of PBDEs (>98% purity) containing eight primary congeners including BDE 28, BDE 47, BDE 99, BDE 100, BDE 153, BDE 154, BDE 183 and BDE 209 was obtained from Accustandard Inc. via Kinesis (United Kingdom). The concentration of the congeners was 2.5 μg/ml in isooctane, except BDE 209 which was present at 25 μg/ml. Surrogate standards including isotope labeled 13C12-Triclosan (50 μg/ml in methanol), PCB 209 (10 μg/ml in heptane) and 4PC-BDE-208 (50 μg/ml in toluene) (surrogate for PBDEs), and a mix of deuterated PAHs (including acenaphthene-d10, phenanthrene-d10, chrysene-d12 and perylene-d12 at 2 mg/ml in dichloromethane) were purchased from Wellington Laboratories (via Greyhound Chromatography, United Kingdom), Sigma Aldrich (United Kingdom) and Accustandard (via Kinesis United Kingdom) respectively, with purities higher than 98%. Estrogen standards E1, E2, E3 and EE2 (>98% purity) were purchased from Sigma Aldrich (United Kingdom). Deuterated labeled internal standards of estrogens (E1-d4, E2-d4, E3-d2 and EE2-d4) were purchased from C/D/N Isotopes (QMX Laboratories, United Kingdom) (>98% purity). Derivatization reagent BSTFA with 1% TCMS was also purchased from Sigma Aldrich (United Kingdom).
Stock solutions were prepared by dissolving the reference and surrogate standards in methanol or acetone. Working solutions were then prepared by diluting the stock solutions in acetone or methanol and dichloromethane or ethylacetate for sample fortification and instrumental analysis respectively (see details of solvent used for different chemicals in the Supporting Information, Supplementary Information S1). All solutions were stored at 4 C and allowed to reach room temperature for 15 min before use. Ultra-trace grade of methanol, acetone, MTBE, dichloromethane and isopropanol were obtained from Casa Lab or Biosan (Brazil). Cartridges used for solid phase extraction were Isolute C18 (1000mg, 6 ml) and Isolute C18 (500 mg, 6 ml) were purchased from Biotage (United Kingdom), while Oasis HLB (200 mg, 6 ml) was purchased from Waters (United Kingdom). Glass microfiber filters were purchased from Sartorius (MGB filters, 0.7 mm thick, 1.0 μm particle retention).
Wastewater Treatment Plants and Sampling
Sludge samples were collected from three municipal WWTPs around Belo Horizonte (Brazil) with different treatment processes (activated sludge WWTP, UASB WWTP, and WSP) between December 2016–February 2017. Population equivalent, operational flowrate, plant configuration, hydraulic retention times (HRTs) and solid retention times (SRTs) of these WWTPs are reported elsewhere (Komolafe et al., 2021). A facultative inoculum (inoculum adapted to both aerobic and anaerobic environment) was collected from the facultative pond of the WSP. Samples were collected in cleaned and disinfected (with 1% Virkron for 24 h, followed by several rinses cycles with distilled water), 5 L high-density polyethylene (HDPE) containers; analysis of the empty rinsed containers showed no contamination with any of the target compounds. Samples were stored at 4°C upon arrival at the laboratory and were used within 24 h.
Experimental Design of Biodegradation Assay
Batch reactors (glass test vessels) were used for the degradation experiments as they have previously been used to mimic biotransformation reactions and kinetics in full scale wastewater treatment plants -WWTPs (Helbling et al., 2012). The chemicals were added to test vessels in a solution of methanol or acetone–volume of solvent added was limited to 0.1% to minimize any potential effect. These solvents will not inhibit microbial action as methanol is non-toxic to microorganisms below 1,000 mg/L (Novak et al., 1985; Brasil Bernardelli et al., 2015) and acetone is non-toxic below 5% v/v in different redox conditions (González, 2006). For the aerobic experiment, 1,000 μg/L for triclosan, 200 μg/L for PAHs, 100 μg/L for estrogens and 30 μg/L for PBDEs were spiked into the test vessels. For the anaerobic experiment, 3,000 μg/L of triclosan, 600 μg/L of PAHs, 300 μg/L for estrogens and PBDEs at 30 μg/L. These concentrations are in the upper range of those found in sludge from municipal WWTPs (Pérez et al., 2001; Bester, 2005; Salgado et al., 2010; Chen et al., 2011; Jones et al., 2014; Pessoa et al., 2014). These high concentrations were chosen to help differentiate between background concentration of the chemicals in the sludge and reduce analytical bias/uncertainties. More details on spiking concentration of the chemicals are provided in the Supporting Information (Supplementary Information S1). The experiment was carried out at room temperature (30 ± 2°C) and the temperature for the experiment duration was recorded with a temperature logger (Lascar Electronics, EasyLog EL-USB-2-LCD). For the aerobic biodegradation experiments, dissolved oxygen (DO) and pH were monitored throughout the experiment to ensure that they were not limiting or excessive - especially as pH of the sludge has been reported to affect the adsorption of triclosan (Lindström et al., 2002). The DO and pH for the experiment with activated sludge ranged from 0.25–0.45 mg/L DO and pH of 6.6–7.3 respectively. This WWTP operates at a low dissolved oxygen concentration (0.2–0.3 mg/L) as they are not mandated to remove nitrogen, thereby saving on energy costs - hence the relatively low DO concentration. Furthermore, the total suspended solids (TSS) concentration of the sludge from this plant was 4,290 mg/L. For the experiment with facultative inocula, DO concentration and pH ranged from 6.1 to 7.0 mg/L and 7.5 to 9.5 respectively. The TSS concentration of this inocula was 75 mg/L. Furthermore, inhibition and abiotic controls were employed to check for losses due to non-biological degradation, hydrolysis and volatilization. This was done by autoclaving inocula in test vessels twice (24 h apart) at 121°C and 103 kPa for 20 min as described by Helbling et al. (Helbling et al., 2012). Further details of the aerobic and anaerobic experimental design including test vessels, experimental conditions, equipment and test duration is given in the supporting information (Supplementary Information S2).
Extraction and Instrumental Analysis
The compounds were extracted from the wastewater and biosolids by SPE and were analyzed by gas chromatography with mass spectrophotometry (GC-MS- for triclosan and PAHs), liquid chromatography with mass spectrophotometry (LC-MS/MS- for estrogens) or gas chromatography with electron capture detector (GC-ECD-for PBDEs) (Komolafe et al., 2019; Komolafe et al., 2021). The sludge was unfiltered before extraction to account for the chemicals in both the dissolved and solids/particulate phase. Information on the extraction and instrumental analysis of triclosan, PAHs, estrogens and PBDEs is reported elsewhere (Komolafe et al., 2021). SPE cartridges were stored at −20°C in Brazil, then transported in boxes with cooling packs to the UK, where elution and instrumental analysis was performed.
Quality Assurance and Data Analysis
Method accuracy was evaluated by performing recovery experiments in blanks (deionized water, n = 3) and matrix samples (final effluent and activated sludge, n = 3) at different fortification levels (Supplementary Information S1). The repeatability of the method was determined by the relative standard deviation (% RSD) from the recovery experiments in the fortified blank and matrix sample. The recovery rate of triclosan was 102% in effluent (RSD = 11%), and 101% in activated sludge (RSD = 2.5%) (Supplementary Tables S1, S3). The recoveries of PAHs ranged from 67 to 107% (RSD = 0.8–11%) in effluent, and 67–101% (RSD = 0.8–8.1%) in activated sludge (Supplementary Tables S2, S3). Recoveries of PBDEs and estrogens are reported elsewhere (Coello-Garcia et al., 2019; Komolafe et al., 2019). Method IDL, MDL and MQL have previously been reported (Komolafe et al., 2021). All samples were fortified with labeled internal standards- 13C12- triclosan, 13C12- methyl triclosan, acenaphthene-d10, phenanthrene-d10, chrysene-d12, E1-d4, E2-d4, E3-d2, EE2-d4 and perylene-d12 (except for PBDE analysis where unlabelled standards PCB 209 and 4PC-BDE-208 was used) for possible correction of matrix effect and losses during sample extraction. Five levels of concentration for each analyte was used for the calibration curve, and linearity was observed when the correlation coefficient was >0.99.
The reaction kinetics was evaluated by checking the fit of the degradation experimental data to zero-order, first-order, second-order and third-order. The degradation kinetics for all the chemicals follow first-order kinetics with the best linear regression values (R2) (See Supplementary Information S2, Table S1).
The obtained first-order biodegradation rates were used to predict effluent concentrations and assess the likelihood of risk when these chemicals are discharged into water bodies. In a completely stirred tank reactor (CSTR), effluent concentration is given by the equation below (Levenspiel, 1999);
Where k = biodegradation constant (h−1), HRT = hydraulic retention time of the activated sludge plant sampled, and concentrations are in ng/L.
Results and Discussion
Biodegradation Under Aerobic Conditions
Triclosan
Biotransformation of Triclosan With Activated Sludge Inocula
Under aerobic conditions, triclosan concentration in the batch tests decreased by 74% over the duration of the experiment (168 h) (Figure 1, Supplementary Figure S1). Chen et al., reported a similar observation of 86% degradation of triclosan spiked at 500 μg/L after 168 h (Chen et al., 2011). Interestingly, this low DO (0.2–0.45 mg/L) activated sludge inocula performed like a traditional activated sludge inocula with DO of 4 mg/L and above (Bester, 2005; Chen et al., 2011). This implies that activated sludge-based treatment plants operating with low DO concentrations (typical in warmer climates with no nitrogen removal requirements) can remove triclosan as effectively as the traditional ones. The biodegradation of triclosan followed first order kinetics with a rate constant of 0.0072 h−1 and an estimated half-life of 96 h (Table 1, Supplementary Table S5). This rate is similar to those reported by (Chen et al., 2011), but slower than those reported by (Armstrong et al., 2018)–perhaps due to the two fold lower starting concentration (Supplementary Table S5).
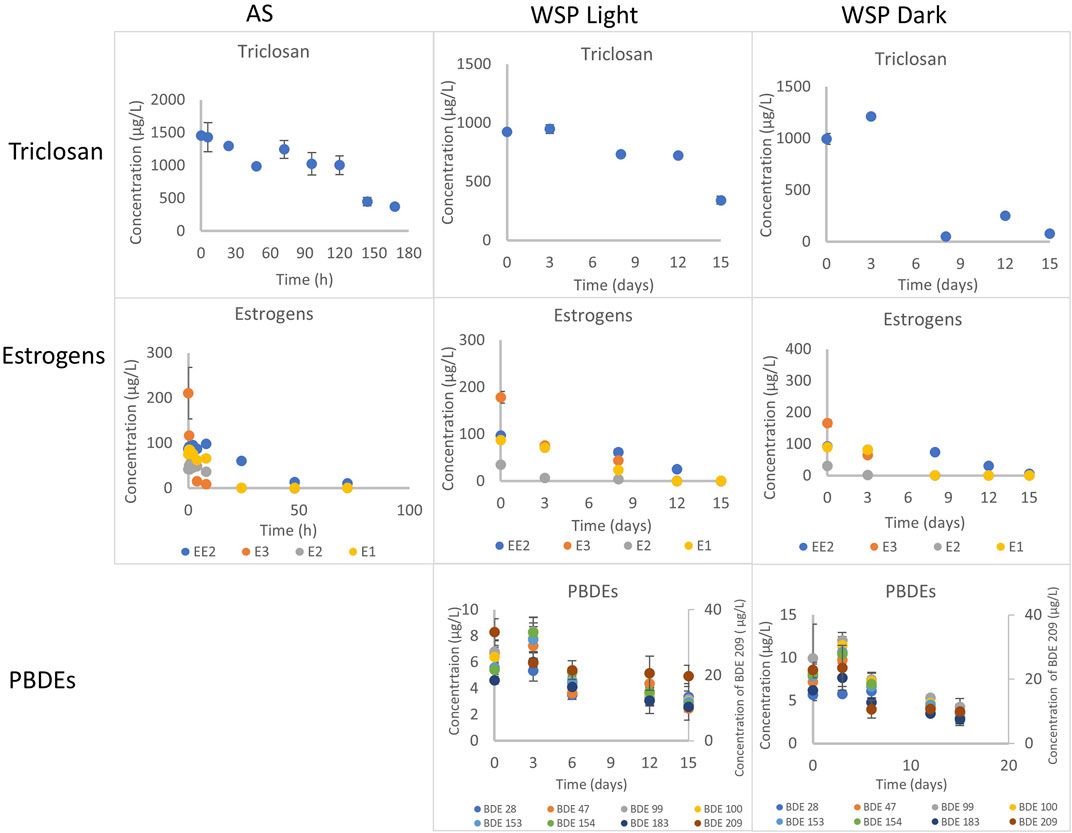
FIGURE 1. Degradation of triclosan, estrogens and PBDEs under aerobic conditions with activated sludge and facultative inocula in the light and dark. Concentration determined in the combined phase (dissolved and particulate). AS refers to activated sludge, WSP refers to waste stabilization pond.
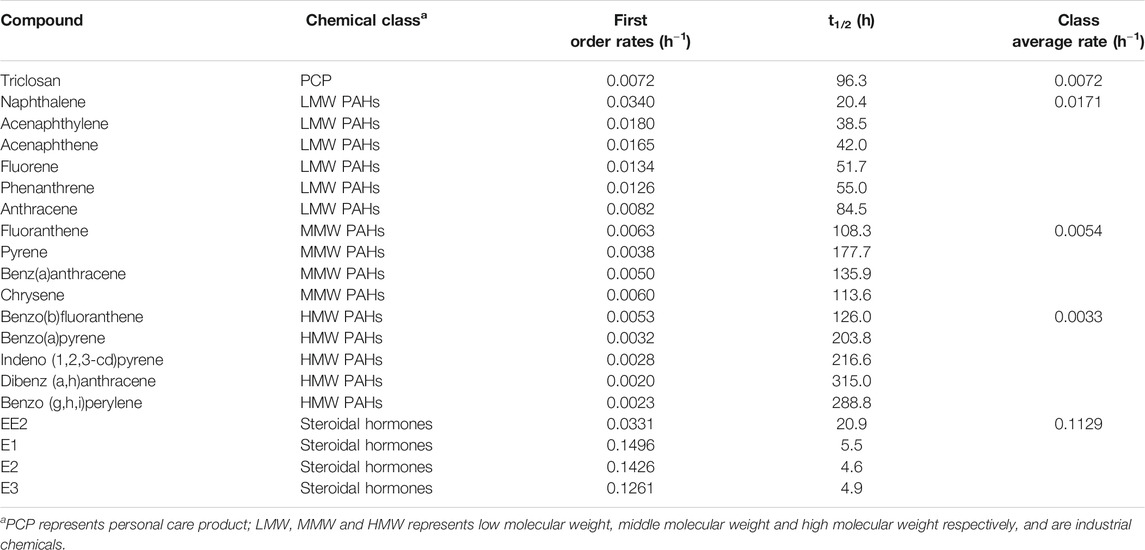
TABLE 1. Degradation rates of different classes of chemicals under aerobic conditions with activated sludge inocula.
Methyl triclosan was produced in the batch tests, and concomitantly increased with decreasing triclosan concentration (Supplemntary Figure S1), thereby supporting reports that methyl triclosan is biotransformation product of triclosan under aerobic conditions (Heidler and Halden, 2007). Methyl triclosan is known to be more persistent, bio-accumulative and lipophilic than triclosan (Lindström et al., 2002; Balmer et al., 2004). However, only 4.8% of triclosan was bio-transformed to methyl triclosan, which suggests the formation of other major triclosan transformation products. Phenol, catechol and 2, 4- dichlorophenol have previously been identified as the major bio-transformation products of triclosan under aerobic conditions with pure bacteria strains isolated from activated sludge (Veetil et al., 2012). The observed methylation of triclosan in this study (4.8%) was higher than the 1% reported by (Chen et al., 2011) but lower than the 42% reported by (Armstrong et al., 2018).
Surprisingly, results from the inactivated control (autoclaved sludge) showed losses after 168 h. This was possibly due to incomplete inactivation of the inocula during the adopted autoclaving process (Helbling et al., 2012). The methylation of triclosan observed in the inhibition control (Supplementary Figure S1) indicated incomplete inactivation of the sludge, since such a transformation is unlikely to occur without a biological catalyst. Therefore, associated reductions of triclosan in this control may be attributed to biodegradation, not abiotic losses. Experimental data from previous UK studies (where controls worked) also indicated that removal of triclosan was by degradation (data not shown). Hence, reduction observed was mainly due to degradation. Furthermore, sorption contributes majorly to triclosan removal in wastewater as our previous study reported that 59–92% of triclosan was sorbed onto suspended solids (Komolafe et al., 2021).
Biotransformation of Triclosan With WSP (Facultative) Inocula
The concentration of triclosan decreased by 63 and 92% under light and dark conditions respectively in 15 days with inocula obtained from the WSP (WWTP C) (Figure 1).
The biodegradation of triclosan followed first order kinetics under both conditions with half-life of 12 days (rate constant of 0.0566 d−1) in the light and 4 days (rate constant of 0.1752 d−1) in the dark (Table 2, Supplementary Table S6). Photodegradation has been reported to play a role in triclosan elimination from the environment due to its degradation when irradiated with UV light and sunlight (Chen et al., 2008; Buth et al., 2010; Tamura and Yamamoto, 2012). A study reported 63% photodegradation of triclosan (at a first order rate of 0.087 d−1) and formation of 2,8-dichlorodibenzo-p-dioxin (2,8-DCDD) in fresh water after irradiation with white light (fluorescent lamp), and no degradation under dark conditions (Aranami and Readman, 2007). In contrast, triclosan degraded faster under dark conditions in this study using a facultative inoculum. This might be due to more intense competition between autotrophs and heterotrophs for nutrients under light conditions leading to less degradation of triclosan. On the other hand, the death of autotrophs and subsequent release of nutrients might have favored the co-metabolic degradation of triclosan by the heterotrophs. Anaerobic conditions were not maintained in these experiments and being an inoculum sourced from a facultative pond, the co-existence of aerobic and anaerobic conditions might have confounding effects on the system. Similar to the aerobic experiment with activated sludge, the inactivated control (autoclaved inocula) also showed reductionof triclosan over time (data not shown), most likely for the same reasons as in the activated sludge aerobic experiment above. Hence, reductions observed was mainly due to degradation.
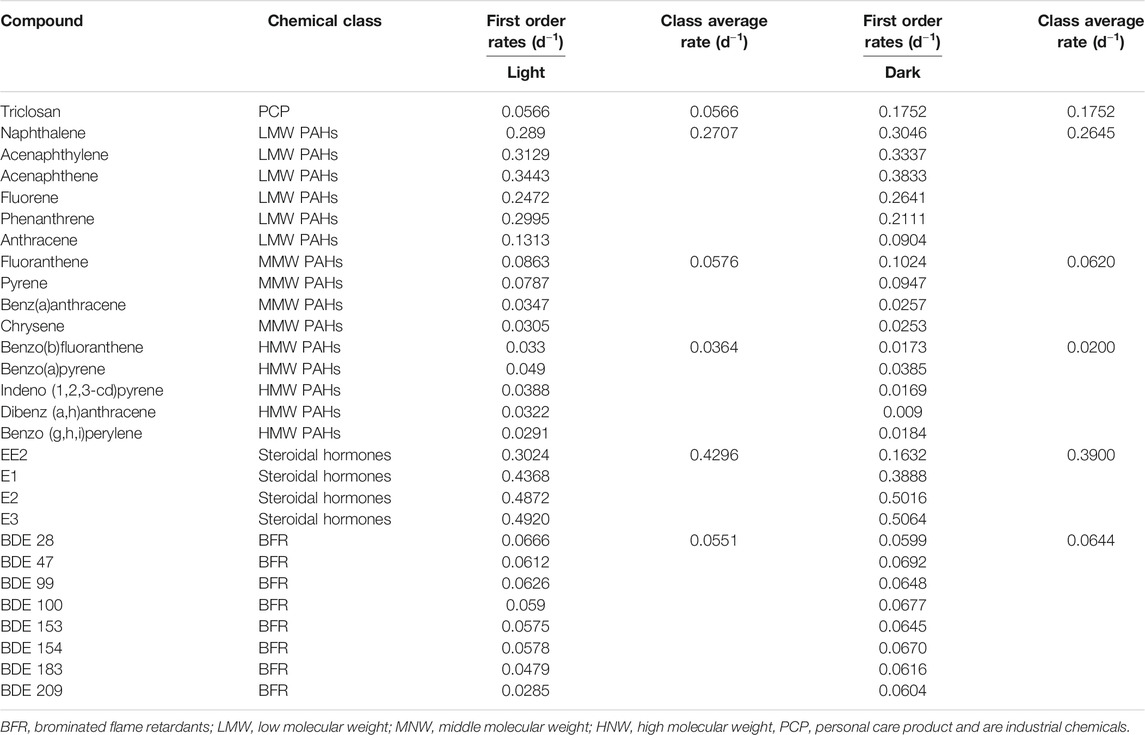
TABLE 2. Degradation rates of different classes of chemicals under aerobic conditions with facultative pond inocula.
Polycyclic Aromatic Hydrocarbons
Biotransformation of Polycyclic Aromatic Hydrocarbons With Activated Sludge Inocula
Reduction of low molecular weight (LMW) PAHs ranged from 64% for anthracene to 97% for naphthalene after 144 h (Figure 1, Supplementary Figure S3). By comparison, reduction of middle molecular weight (MMW) PAHs ranged from 57% for chrysene to 61% for fluoranthene (Figure 2, Supplementary Figure S4), while high molecular weight (HMW) PAHs ranged from 56% for benzo(b)fluoranthene to 21% for dibenz (a,h)anthracene (Figure 2, Supplementary Figure S5). The inactivated control (autoclaved activated sludge) showed reduction of some LMW PAHs (naphthalene, acenaphthylene, acenaphthene and fluorene) - suggesting that volatilization contributed to the reduction of LMW PAHs. Volatilization tendency for these four chemicals was estimated to be between 0.5–2% at 25°C and atmospheric pressure (see calculation in S4), but eration during activated sludge treatment has been reported to intensify volatilization rates (Luo et al., 2014). Lighter PAHs tend to volatilize as a result of their relatively lower melting point and higher water solubility compared to heavier PAHs (Trably et al., 2005). The inactivated control for MMW and HMW PAHs showed no loses (Supplementary Figures S3, S4) indicating that reductions observed was solely due to degradation. However, sorption contributes majorly to the removal of PAHs in wastewater as our previous study reported that 60–97% of PAHs was sorbed onto suspended solids (Komolafe et al., 2021).
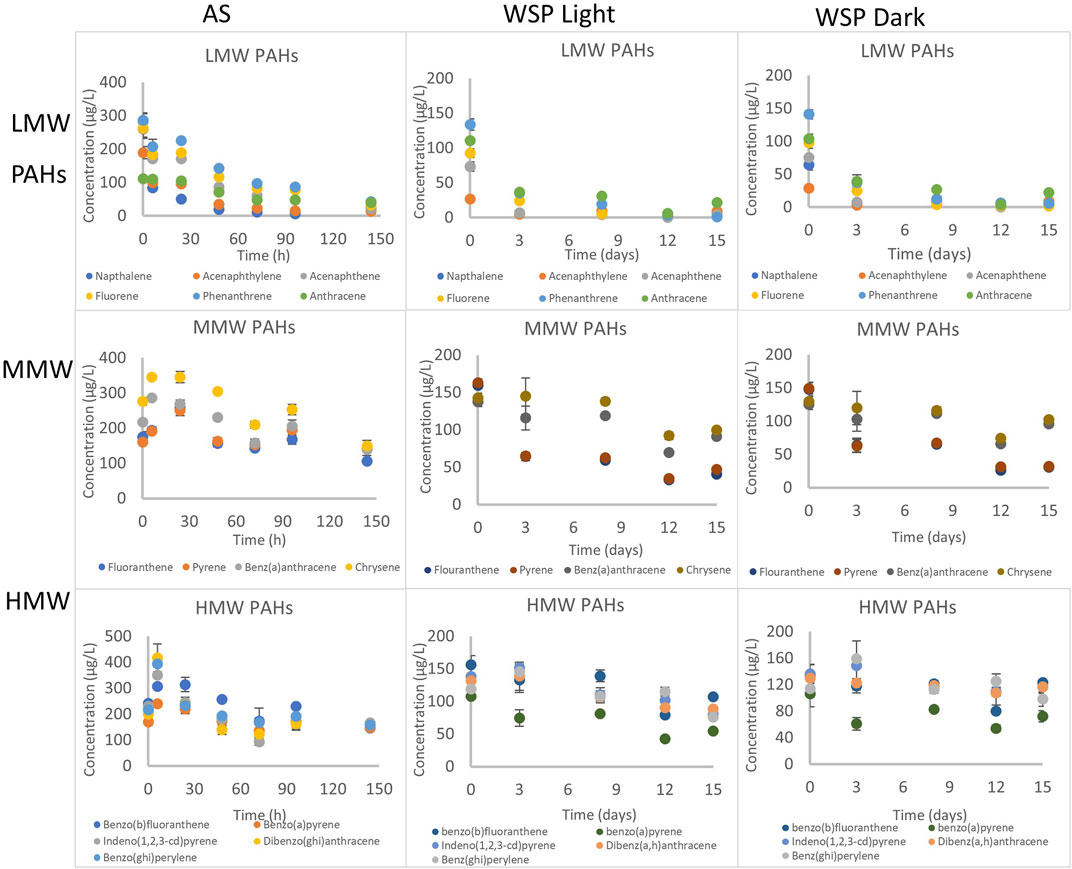
FIGURE 2. Disappearance of PAHs under aerobic conditions with activated sludge and facultative inocula (under light and dark conditions). Concentration determined in the combined phase (dissolved and particulate). AS refers to activated sludge, WSP refers to waste stabilization ponds. LMW, MMW and HMW refers to low molecular weight, middle molecular weight and high molecular weight respectively.
The biodegradation of PAHs followed first order kinetics and their half-lives ranged from 20 to 315 h (Table 1, Supplementary Table S7). The half-lives and first order rate constants of LMW PAHs ranged from 20 to 85 h (naphthalene to anthracene) and 0.0340–0.0082 h−1 (naphthalene to anthracene) respectively. In comparison, slower rates and longer half-lives were observed for MMW PAHs with half-lives and rate constants ranging from 110 to 182 h (fluoranthene-pyrene) and 0.0063–0.0038 h−1 (fluoranthene-pyrene) respectively (Table 1). HMW PAHs were the slowest to degrade with half-lives and rate constants ranging from 131 to 347 h and 0.0053–0.0020 h−1. This further suggests that degradation rates of PAHs increases from light to heavier PAH under aerobic conditions, and agrees with findings by several other reports (Trably et al., 2005; Ghosal et al., 2016).
Biotransformation of Polycyclic aromatic hydrocarbons With WSP (Facultative) Inocula
Degradation of 15 PAHs under aerobic conditions with facultative inocula was observed in the presence and absence of white light (fluorescent bulb placed 20 cm from the reactors) (Figure 2). The inactivated control (autoclaved sludge) failed in this experiment. However, some experimental data from previous UK studies (data not shown) showed that removal was mostly by degradation with minor volatilization. The reduction of LMW PAHs ranged from 80 to 98% and 79–99% (anthracene to naphthalene) under light and dark conditions respectively (Figure 2, Supplementary Figure S6). In comparison, the reduction of MMW PAHs ranged from 30 to 74% and 22 to 79% (chrysene to fluoranthene) under light and dark conditions respectively (Figure 2, Supplementary Figure S7). The extent of degradation of HMW PAHs was lowest, ranging from 31 to 45 and 8.2 to 32% (benzo(b)fluoranthene to benzo(a)pyrene) under the light and dark conditions respectively (Figure 2, Supplementary Figure S8).
The degradation of PAHs followed first order kinetics with half-lives ranging from 2 to 34 days and 2 to 77 days under light and dark conditions (Table 2, Supplementary Table S8). Degradation was slower with increasing molecular weight of the PAHs since rate constants were between 0.0863–0.3833 d−1, 0.0253–0.1024 d−1 and 0.0090–0.0490 d−1 for LMW, MMW and HMW PAHs respectively (Table 2). Similar degradation rates and half-lives were observed for LWM PAHs under light (2 days) and dark (2 days) conditions with similar rate constants, except for phenanthrene and anthracene, where the reaction was relatively faster in the light (half-life = 2 and 5 days respectively in light, 3 and 8 days in the dark) (Table 2). The degradation of MMW PAHs was faster under light (half-lives ranging from 8 to 23 days) than dark conditions (half-lives ranging from 7.7–27 days). Similarly, degradation of HMW PAHs was faster in the light (half-lives between 14–24 days), than dark conditions (half-lives between 18–77 days).
Photolysis has been reported as a major abiotic degradation process for PAHs either via direct photooxidation (radiation absorbed directly by PAHs) or photosensitization (transformation mediated by other light absorbing substances) (Bertilsson and Widenfalk, 2002; Saeed et al., 2011). This explains the observed faster degradation under light conditions. Furthermore, photolytic degradation has been reported to be more rapid in higher molecular weight PAHs due to their lower quantum yields that leads to increased photoreactivity by a better overlap of their adsorption to solar spectrum (Kochany and Maguire, 1994). This also explains the extent of high molecular weight PAHs photodegradation in presence of light compared to low and middle molecular weight PAHs in this study.
Estrogens
Biotransformation of Estrogens With Activated Sludge Inocula
The reduction of all four estrogens observed in the experiment was solely due to biodegradation as no sign of abiotic losses was indicated by the inactivated control (autoclaved sludge) (Figure 1, Supplementary Figure S10). The concentration of E1, E2, E3 decreased by 99% in 72 h in the batch tests; with the most reduction (96–99%) occurring within 24 h (Supplementary Figure S10). EE2 was also reduced by 88% after 72 h (Supplementary Figure S10). Rapid transformation and high removal rate of E3, E2, E1 and EE2 has been reported to occur under nitrifying conditions with activated sludge and ammonia oxidizing bacteria (Dytczak et al., 2008; Gaulke et al., 2008; Haiyan et al., 2007). The simultaneous oxidation/removal of NH4+-N and organic micropollutants is a consequence of co-metabolic biotransformation induced by autotrophic aerobic bacteria present in the system (Fernandez-Fontaina et al., 2012; Helbling et al., 2012; Yi et al., 2006). Some authors suggested that the enzyme ammonium monooxygenase (AMO) is the catalyst responsible for the micropollutants and NH4+-N co-metabolism (Yi et al., 2006). In our study (Supplementary Figure S10), E3 and E1 were the least adsorbed estrogen as 96–97% and 92–96% respectively was present in the aqueous phase, compared to EE2 (25–47%) and E2 (8.2–8.5%). This might be due to their water solubility and Log Kow (2.47 for E3, 3.13 for E1, 3.67 for EE2 and 4.10 for E2) (Liu et al., 2009) and implies that the relatively highly hydrophobic EE2 and E2 possess higher tendency of adsorption when compared to E3 and E1, hence, their removal in treatment plants can be partly due to adsorption (Urase and Kikuta, 2005; Wang et al., 2013).
The biotransformation of the all four estrogens followed first order kinetics with estimated half-lives of 5.5, 4.6, 4.9, and 20.9 h for E1, E2, E3 and EE2 respectively (Table 1, Supplementary Table S5). Reported degradation rates of estrogens varies greatly in the literature because the biodegradation assays were conducted under different temperatures, inocula concentration and spiked concentrations (Liu et al., 2015) (Supplementary Table S5). The degradability of the estrogens in our study is E3/E2 > E1 > EE2. This observation is in agreement with previous studies (Petrie et al., 2014; Liu et al., 2015). However, Shi et al., reported that E3 was the most resistant to aerobic degradation (Shi et al., 2004) (Supplementary Table S9).
Biotransformation of Estrogens With WSP (Facultative) Inocula
The reduction of all four estrogens was observed in the aerobic batch tests under light and dark conditions (Figure 1, Supplementary Figure S11). Although, no other studies have used facultative inocula in their experiments, the degradation trend observed was similar to those reported with activated sludge inocula (Dytczak et al., 2008). About 99.5% of E1, E2 and E3 were degraded after 15 days under the light and dark conditions (Supplementary Figure S11). Also, more EE2 was degraded under light conditions (99.5%), than in the dark (93.5%) after 15 days (Supplementary Figure S11). Most of the losses of E1, E2 and E3 (>95%) occurred within 8 and 12 days under dark and light conditions respectively. However, degradation of EE2 was slower, with 93.5–99.5% removal after 15 days.
The degradation of all four estrogens under light and dark incubation conditions followed first order kinetics (Table 2, Supplementary Table S10). The reaction kinetics was similar for E1 and E3 under both light and dark conditions with estimated rate half-lives of 1.4 days (Table 2). Meanwhile the degradation reaction kinetics was faster under light conditions for E2 and EE2. The rate constant of E2 (half-life of 1.6 days) was 0.4368 d−1 under light conditions, compared to 0.3888 d−1 (half-life of 1.8 days) under dark conditions. Also, the degradation rate almost doubled in the light (k = 0.3024 d−1, half-life = 2.3 days) for EE2 compared to the dark conditions (k = 0.1632 d−1, half-life = 4.2 days) (Table 2).
This enhanced degradation E2 and EE2 under light conditions was due to photolysis in which the compounds might have degraded directly by absorption of photons or through photosensitization (Zhang and Zhou, 2005; Sornalingam et al., 2016). A review of several publications on the photodegradation of estrogens published recently by Sornalingam et al. reports photodegradation of estrogens under different light sources (sunlight, visible light, UV light-with UV light most effective) and water matrixes (Sornalingam et al., 2016). It was established that the rate of photodegradation of estrogens is influenced by light source and intensity, and solution matrix (Sornalingam et al., 2016). In fact, the same light source was reported to have different effects on individual estrogens, as E1, E2 and EE2 were removed at a similar rate under catalysis, but removal followed the order EE2 > E1 > E2 under UVA light (Coleman et al., 2004). This might explain the observed photodegradation of E2 and EE2, and not for E1 and E3 as individual chemicals can behave differently.
Polybrominated Diphenyls
Due to a limited inventory of PBDE analytical standard and unavailability in Brazilian markets at the time of the study, the degradation experiment for PBDEs was only carried out with WSP facultative inocula. Experiment with activated sludge inocula was not performed.
Biotransformation of Polybrominated Diphenyls With WSP (Facultative) Inocula
Reduction in the concentration of individual PBDE congeners ranged from 40 to 63% and 42–63% under light and dark conditions respectively (Figure 1, Supplementary Figure S13). Furthermore, degradation of the most abundant congener BDE 209 was 40 and 57% under light and dark conditions respectively. Similar results were reported by Stiborova et al. where degradation of BDE 28 to BDE 209 was between 62–78% after 11°months of studies in aerobic reactors inoculated with sewage sludge and placed in the dark (Stiborova et al., 2015). The abiotic control showed no loses, hence, reduction observed for the congeners was due to degradation. However, sorption contributes majorly to the removal of PBDEs in wastewater as our previous studies reported that 20–87% of PBDEs were sorbed onto suspended solids (Komolafe et al., 2019; Komolafe et al., 2021).
The degradation of the PBDE congeners followed first order kinetics with half-lives ranging from 10.4 to 24.3 days and 10–11.5 days under light and dark conditions respectively (Table 2, Supplementary Table S7). The degradation rates were relatively faster in the dark conditions with rate constants between 0.0599–0.0692 d−1 and 0.0285–0.0666 d−1 in dark and light conditions respectively. This was true for all the congeners except BDE 28, where the rate was faster under white light. This is surprising as photodegradation has been suggested as an important abiotic transformation process for PBDEs in the environment, whereby heavily brominated congeners (such as BDE 209, BDE 183) undergo debromination to less brominated ones (such as BDE 28, BDE 47) (Ahn et al., 2006; Davis and Stapleton, 2009; Pan et al., 2016).
A recent review on photodegrdation of PBDEs by Pan et al. showed reductive debromination of PBDEs in different aquatic systems with a range of irradiation sources (xenon or mercury or UV lamps, sunlight (Pan et al., 2016). They also suggested that chemical species such as humic substances and ions (halides and metals) present in the aquatic systems strongly influences photochemical transformations. Furthermore, the source and intensity of light affects photolytic degradation of pollutants (Sornalingam et al., 2016). In this study, the chemical species of the system and the light source (white fluorescent light) might not have facilitated photodegradation, and the observed losses of PBDEs was biodegradation by the microbial community. Furthermore, the increased reaction kinetics in the dark might have been due to the death of autotrophs over time leading to increased availability of nutrients for the heterotrophs to co-metabolically degrade the PBDEs.
Comparing the Degradation Rates of the Different Classes of Chemicals Under Aerobic Condition
With activated sludge inocula, estrogens degraded more rapidly with their average reaction rate 7, 16, 21 and 34 times higher than those for low molecular weight (LWM) PAHs, triclosan, middle molecular weight (MMW) PAHs and high molecular weight (HMW) PAHs respectively (Table 1, Figure 3). LMW PAHs degraded two times faster than triclosan on average. Degradation of triclosan was slightly faster (1.3 times) than MMW PAHs but was 2 times faster than HMW PAHs (Table 1, Figure 3). The difference in chemical structure and functional groups among the chemical classes influenced their biodegradation abilities. Under aerobic conditions, biotransformation of aromatics is initiated by hydroxylation (addition of molecular oxygen to the aromatic ring) followed by cleavage of the aromatic ring and then electrophilic substitution (Pitter and Chudoba, 1990). The presence of strong electron withdrawing substituent groups (such as halogens, nitrogen) decreases the electron density of the aromatic ring and consequently reduces the biodegradation rate in comparison to weaker substituent groups (OH, CH3) (Pitter and Chudoba, 1990). This explains why the degradation of triclosan was slower than estrogens and some PAHs. Vuono et al., reported poor removal of chemicals with strong electron withdrawing functional groups in full scale membrane bioreactors in comparison to other chemicals (Vuono et al., 2016).
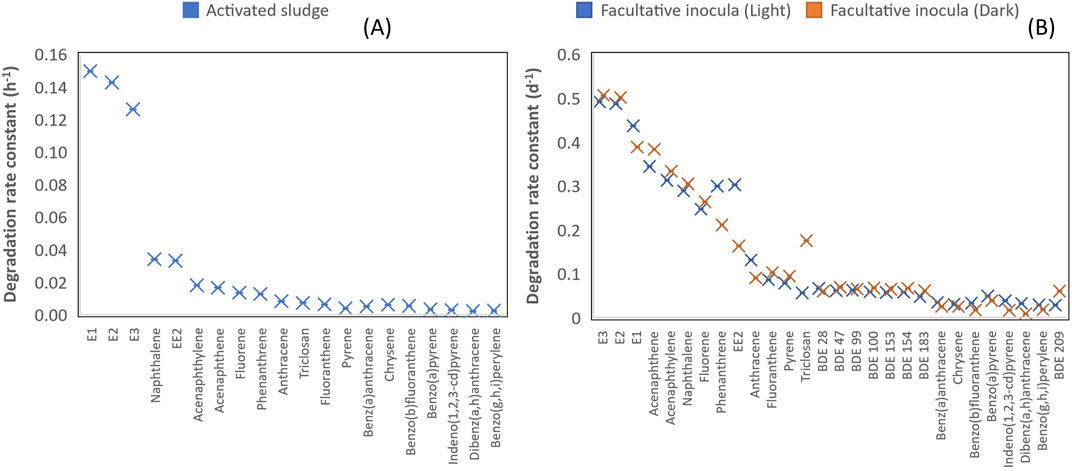
FIGURE 3. (A) Degradation rate constant of different classes of chemicals under aerobic conditions with activated sludge inocula. (B) Degradation rate constant of different classes of chemicals under aerobic condition with facultative inocula (light and dark incubation conditions).
A similar trend was observed with facultative pond inocula where the degradation of estrogens was 2–3, 6–8, 12–20 and 2–8 times faster than LMW PAHs, MMW PAHs, HMW PAHs and triclosan respectively under light and dark incubation conditions (Table 2). Furthermore, the degradation of PAHs was 3–4 times faster than PBDEs under both incubation conditions. In summary, the degradation rate of the chemicals follows this order-estrogens > PAHs > triclosan > PBDEs. The pseudo first-order rates of all the classes of chemicals under aerobic conditions with activated sludge and facultative inocula was also estimated with the suspended solids concentration of the inocula. The pseudo-first order rate ranged from 0.0005–0.0349 L.gSS/h for activated sludge inocula, 0.3800–6.560 L.gSS/d for facultative inocula under light conditions and 0.1200–0.675 L.gSS/d facultative inocula under dark conditions (Supporting Information, Supplementary Table S9).
Biodegradation Under Anaerobic Conditions
After 15 days of incubation, no reduction was observed in the concentration of triclosan (Supplementary Figure S2), PAHs (Supplementary Figure S9), estrogens (Supplementary Figure S12) and PBDEs (Supplementary Figure S14). Also, methylation of triclosan was not observed in the anaerobic batch tests (results not shown). Chen et al. also reported no significant biodegradation of triclosan under anaerobic conditions with mixed culture inocula (Chen et al., 2011). However, 60–90% degradation of triclosan under anaerobic conditions by pure bacteria strains inoculated from anaerobic sludge has been reported (Veetil et al., 2012). Some studies have reported anaerobic degradation of estrogens, especially E1 and E2 (Andersen et al., 2004; Zhang et al., 2015), while other studies reported the resistance of EE2 to anaerobic degradation (Joss et al., 2004; Czajka and Londry, 2006). Furthermore, there has been no previous report on anaerobic degradation of E3. Either activated sludge or lake sediment was used as inoculum in the previous studies that reported anaerobic degradation of estrogens. UASB sludge was used as inoculum in this study, and this might explain the lack of degradation when compared to past studies. There have been several reports of the debromination of PBDEs by dehalogenating bacteria under anaerobic conditions (Gerecke et al., 2005; He et al., 2006; Xia, 2013), hence, our results may seem surprising. However, a study reported less than 20% anaerobic degradation of BDE 47, BDE 99, BDE 100, BDE 153, and BDE 154 after 70 days with mixed culture inocula sourced from river sediment (Yen et al., 2009). Another study also reported 30% debromination and degradation of BDE 209 within 238 days with digested sewage sludge under anaerobic conditions (Gerecke et al., 2005). Both studies reported poor degradation of PBDEs after several months. This explains why degradation of PBDEs was not observed in this study, as the incubation period was comparatively short (15 days).
Predicting Effluent Quality and Associated Risks Using Obtained Degradation Rates
Using the equation given by (Levenspiel, 1999) described in Supplementary Information S5, the obtained biodegradation rates were used to predict effluent concentrations from previously sampled WWTPs (Komolafe et al., 2021) and assess the likelihood of risk when these chemicals are discharged into water bodies accordingly. The assumptions for this assessment are detailed in Supporting Information (S5). This predicted effluent concentration was compared to the measured concentration of these chemicals reported previously (Komolafe et al., 2021).The measured concentrations are combined aqueous and particulate phase concentrations.
The predicted concentrations were in agreement with the measured values (within the same order of magnitude) (Table 3, Table 4). The predicted effluent concentration for triclosan in both the activated sludge WWTP A (7,161 ng/L) and WSP (593 ng/L) was well above the predicted no-effect concentration (PNEC) value (100 ng/L). Also, the predicted concentration of naphthalene and benz(b)fluoranthene were below their EQS values in both WWTPs, while fluoranthene exceeded the EQS in the activated sludge WWTP, and anthracene exceeded in the WSP. For estrogens, the predicted concentration of E3 exceeded the PNEC values in both WWTPs, while that of E1 only exceeded in the activated sludge plant. The summed predicted concentration of EU Water Framework Directive (WFD) PBDEs (BDE 28, 47, 99, 100, 153, 154) was below the EQS value in WWTP C (Table 4). Hence, overall, the effluent from these plants could pose a risk to aquatic organisms when discharged into receiving waters.
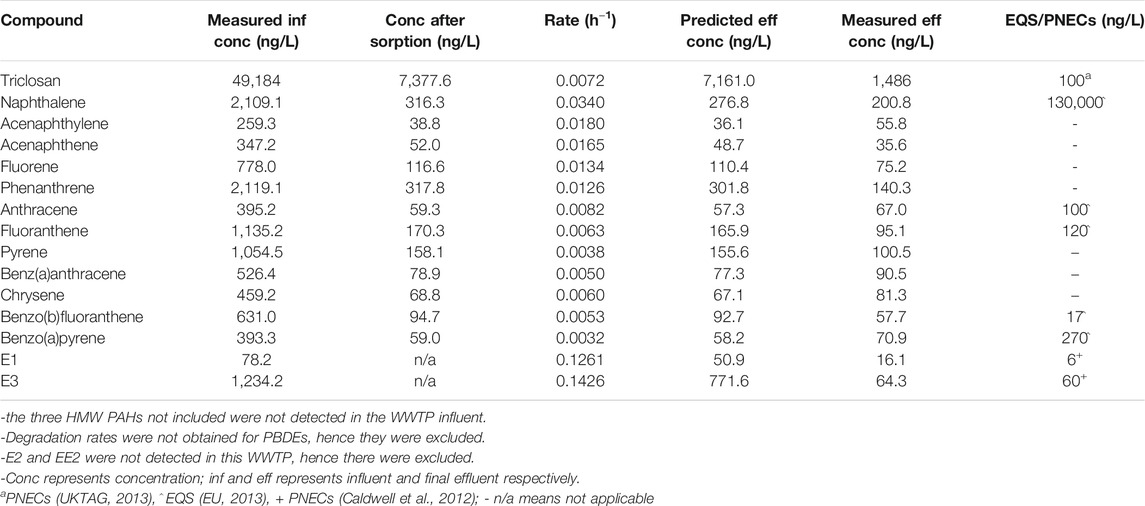
TABLE 3. Predicted effluent concentration of the chemicals after activated sludge treatment (Brazil WWTP A).
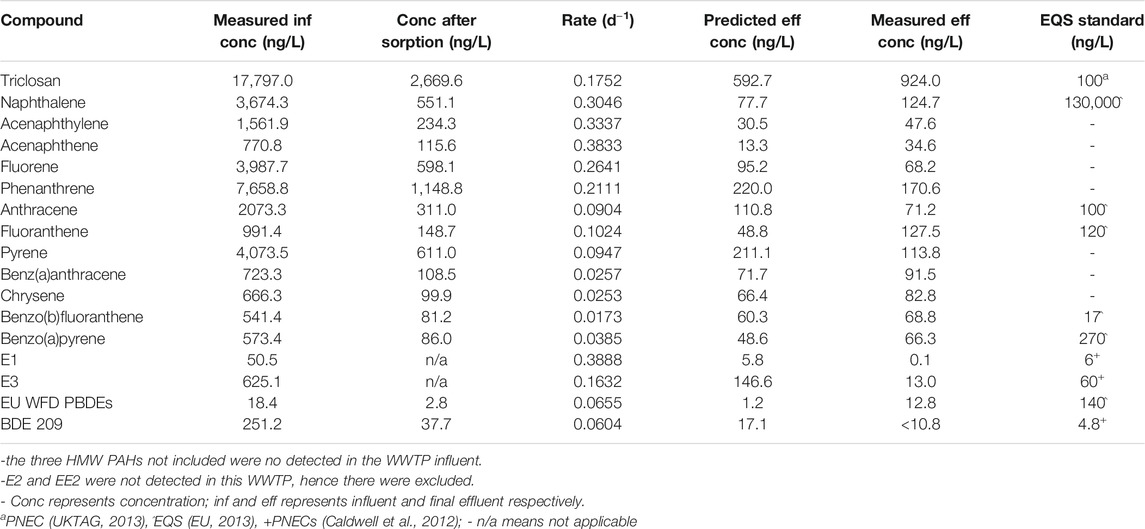
TABLE 4. Predicted effluent concentration of the chemicals after facultative pond treatment (WSP, Brazil WWTP C).
Some of these WWTPs would need unsuitable HRTs or river dilution to meet the EQS or PNEC values for some of the chemicals. For example, an HRT of over 10,000 h or river dilution by 62 times would be required for triclosan to meet PNEC concentrations in the activated sludge WWTP A while E1 will require 96 h or 9 times dilution (Supplementary Table S8). Neither long HRTs nor river dilution are suitable mitigation strategies, however, which would suggest that tertiary treatments are required for effluents of these WWTPs.
Conclusion
Degradation of all the chemicals was only observed under aerobic conditions with a faster reaction kinetic with activated sludge inocula over the facultative inocula from the WSP. The degradation kinetics of the chemicals was in the following order: estrogens > LMW PAHs > triclosan > PBDEs > MMW PAHs > HMW PAHs. The results suggested that biodegradation is a major removal mechanism during biological treatment in the activated sludge and WSP WWTPs surveyed. However, volatilization was also observed to contribute to the removal of some of the chemicals within these two treatment systems. Furthermore, the susceptibility of chemicals to photodegradation differed between and within classes of chemicals- PAHs and estrogens degraded faster under light conditions (white fluorescent light) while triclosan and PBDEs degraded faster in the dark. Degradation of all test chemicals was not observed under anaerobic conditions with the UASB sludge inocula, suggesting that their removal - if any - observed during UASB treatment was most likely due to sorption to sludge and not to biodegradation. Sorption is an important removal pathway of these chemicals in treatment systems that should be investigated thoroughly in a future study to understand the overall fate of the chemicals.
The first order degradation rates obtained in this study for different classes of chemicals including triclosan, PAHs, estrogens and PBDEs was successfully used to predict effluent concentrations and estimate required HRTs for removal of these chemicals to concentrations below current EQS/PNEC values. Importantly, there was a good match between the predicted and measured effluent concentration for all the chemicals investigated. These concentrations were above the EQS/PNEC values for some of the chemicals in all classes, and the required HRT for adequate removal is mostly impractical. However, river dilution might ensure compliance for some of the chemicals under certain circumstances, although this is not really a desirable or suitable mitigation strategy. Furthermore, the synergistic effect of the cocktail of different classes of compounds on aquatic organisms remains unknown. This work suggests that improvements in these technologies and/or other tertiary treatment technologies are required to ensure such systems can achieve environmentally safe levels of some micropollutants in receiving water courses.
Data Availability Statement
The original contributions presented in the study are included in the article/Supplementary Material, further inquiries can be directed to the corresponding author.
Author Contributions
OK: Conceptualization, Methodology, Data acquisition, Formal analysis, Investigation, Writing- original draft. WM: Conceptualization, Supervision, Analytical Methodology, Writing-review and editing. KA: Investigation, Writing-review and editing. LV: Resources, Writing-review and editing. CM: Supervision, Writing-review and editing. RD: Funding acquisition, Conceptualization, Supervision, Writing-review and editing.
Funding
This project was funded by a Challenging Engineering award from the Engineering and Physical Science Research Council (EPSRC; EP/I025782/1).
Conflict of Interest
Author OK was employed by GFL Environmental Inc.
The remaining authors declare that the research was conducted in the absence of any commercial or financial relationships that could be construed as a potential conflict of interest.
Acknowledgments
We thank Dan Curtis, Paul Donohoe and Bernie Bowler for their assistance in the instrumental analysis of these chemicals. We also thank Thiago Bressani, and Fernanda Espinosa (UFMG) for their assistance during sampling and laboratory work in Brazil. The authors would like to acknowledge the National Council for Scientific and Technological Development from Brazilian Ministry of Education–CNPq-and to the Institute of Sustainable Sewage Treatment Plants - INCT ETEs; Sustentáveis and their funders.
Supplementary Material
The Supplementary Material for this article can be found online at: https://www.frontiersin.org/articles/10.3389/fenvs.2021.700245/full#supplementary-material
References
Ahn, M.-Y., Filley, T. R., Jafvert, C. T., Nies, L., Hua, I., and Bezares-Cruz, J. (2006). Photodegradation of Decabromodiphenyl Ether Adsorbed onto clay Minerals, Metal Oxides, and Sediment. Environ. Sci. Technol. 40, 215–220. doi:10.1021/es051415t
Andersen, H. R., Kjølholt, J., Hansen, M., Stuer-Lauridsen, F., Blicher, T. D., Ingerslev, F., et al. (2004). Degradation of Estrogens in Sewage Treatment Processes. Environ. Project 899.
Aranami, K., and Readman, J. W. (2007). Photolytic Degradation of Triclosan in Freshwater and Seawater. Chemosphere 66, 1052–1056. doi:10.1016/j.chemosphere.2006.07.010
Armstrong, D. L., Lozano, N., Rice, C. P., Ramirez, M., and Torrents, A. (2018). Degradation of Triclosan and Triclocarban and Formation of Transformation Products in Activated Sludge Using Benchtop Bioreactors. Environ. Res. 161, 17–25. doi:10.1016/j.envres.2017.10.048
Balmer, M. E., Poiger, T., Droz, C., Romanin, K., Bergqvist, P.-A., Müller, M. D., et al. (2004). Occurrence of Methyl Triclosan, a Transformation Product of the Bactericide Triclosan, in Fish from Various Lakes in Switzerland. Environ. Sci. Technol. 38, 390–395. doi:10.1021/es030068p
Bertilsson, S., and Widenfalk, A. (2002). Photochemical Degradation of PAHs in Freshwaters and Their Impact on Bacterial Growth–Influence of Water Chemistry. Hydrobiologia 469, 23–32. doi:10.1023/a:1015579628189
Bester, K. (2005). Fate of Triclosan and Triclosan-Methyl in Sewage Treatmentplants and Surface Waters. Arch. Environ. Contam. Toxicol. 49, 9–17. doi:10.1007/s00244-004-0155-4
Brasil Bernardelli, J. K., Liz, M. V., Belli, T. J., Lobo-Recio, M. A., and Lapolli, F. R. (2015). Removal of Estrogens by Activated Sludge Under Different Conditions Using Batch Experiments. Brazili. J. Chem. Enginee. 32 (2), 421–432.
Buth, J. M., Steen, P. O., Sueper, C., Blumentritt, D., Vikesland, P. J., Arnold, W. A., et al. (2010). Dioxin Photoproducts of Triclosan and its Chlorinated Derivatives in Sediment Cores. Environ. Sci. Technol. 44, 4545–4551. doi:10.1021/es1001105
Caldwell, D. J., Mastrocco, F., Anderson, P. D., Länge, R., and Sumpter, J. P. (2012). Predicted-no-effect Concentrations for the Steroid Estrogens Estrone, 17β-Estradiol, Estriol, and 17α-Ethinylestradiol. Environ. Toxicol. Chem. 31, 1396–1406. doi:10.1002/etc.1825
Cea-Barcia, G., Carrère, H., Steyer, J. P., and Patureau, D. (2013). Evidence for PAH Removal Coupled to the First Steps of Anaerobic Digestion in Sewage Sludge. Int. J. Chem. Eng. 2013. doi:10.1155/2013/450542
Chen, X., Nielsen, J. L., Furgal, K., Liu, Y., Lolas, I. B., and Bester, K. (2011). Biodegradation of Triclosan and Formation of Methyl-Triclosan in Activated Sludge under Aerobic Conditions. Chemosphere 84, 452–456. doi:10.1016/j.chemosphere.2011.03.042
Chen, Z., Song, Q., Cao, G., and Chen, Y. (2008). Photolytic Degradation of Triclosan in the Presence of Surfactants. Chem. Pap. 62, 608–615. doi:10.2478/s11696-008-0077-0
Coello-Garcia, T., Curtis, T. P., Mrozik, W., and Davenport, R. J. (2019). Enhanced Estrogen Removal in Activated Sludge Processes through the Optimization of the Hydraulic Flow Pattern. Water Res. 164, 114905. doi:10.1016/j.watres.2019.114905
Coleman, H. M., Routledge, E. J., Sumpter, J. P., Eggins, B. R., and Byrne, J. A. (2004). Rapid Loss of Estrogenicity of Steroid Estrogens by UVA Photolysis and Photocatalysis over an Immobilised Titanium Dioxide Catalyst. Water Res. 38, 3233–3240. doi:10.1016/j.watres.2004.04.021
Czajka, C. P., and Londry, K. L. (2006). Anaerobic Biotransformation of Estrogens. Sci. Total Environ. 367, 932–941. doi:10.1016/j.scitotenv.2006.01.021
Davis, E. F., and Stapleton, H. M. (2009). Photodegradation Pathways of Nonabrominated Diphenyl Ethers, 2-Ethylhexyltetrabromobenzoate and Di(2-Ethylhexyl)tetrabromophthalate: Identifying Potential Markers of Photodegradation. Environ. Sci. Technol. 43, 5739–5746. doi:10.1021/es901019w
Dytczak, M. A., Londry, K. L., and Oleszkiewicz, J. A. (2008). Biotransformation of Estrogens in Nitrifying Activated Sludge under Aerobic and Alternating Anoxic/aerobic Conditions. Water Environ. Res. 80, 47–52. doi:10.1002/j.1554-7531.2008.tb00348.x
EU (2013). DIRECTIVE 2013/39/EU of the EUROPEAN PARLIAMENT and of the COUNCIL of 12 August 2013 Amending Directives 2000/60/EC and 2008/105/EC as Regards Priority Substances in the Field of Water PolicyEuropean Union. https://eur-lex.europa.eu/legal-content/EN/ALL/?uri=CELEX:32013L0039.
Falås, P., Wick, A., Castronovo, S., Habermacher, J., Ternes, T. A., and Joss, A. (2016). Tracing the Limits of Organic Micropollutant Removal in Biological Wastewater Treatment. Water Res. 95, 240–249. doi:10.1016/j.watres.2016.03.009
Fernandez-Fontaina, E., Omil, F., Lema, J. M., and Carballa, M. (2012). Influence of Nitrifying Conditions on the Biodegradation and Sorption of Emerging Micropollutants. Water Res. 46, 5434–5444. doi:10.1016/j.watres.2012.07.037
Gaulke, L. S., Strand, S. E., Kalhorn, T. F., and Stensel, H. D. (2008). 17α-ethinylestradiol Transformation via Abiotic Nitration in the Presence of Ammonia Oxidizing Bacteria. Environ. Sci. Technol. 42, 7622–7627. doi:10.1021/es801503u
Gerecke, A. C., Hartmann, P. C., Heeb, N. V., Kohler, H.-P. E., Giger, W., Schmid, P., et al. (2005). Anaerobic Degradation of Decabromodiphenyl Ether. Environ. Sci. Technol. 39, 1078–1083. doi:10.1021/es048634j
Ghosal, D., Ghosh, S., Dutta, T. K., and Ahn, Y. (2016). Current State of Knowledge in Microbial Degradation of Polycyclic Aromatic Hydrocarbons (PAHs): a Review. Front. Microbiol. 7. doi:10.3389/fmicb.2016.01369
Gomez, E., Wang, X., Dagnino, S., Leclercq, M., Escande, A., Casellas, C., et al. (2007). Fate of Endocrine Disrupters in Waste Stabilization Pond Systems. Water Sci. Tech. 55, 157–163. doi:10.2166/wst.2007.350
González, G. M. E. (2007). Enzymatic Degradation Of Polycyclic Aromatic Hydrocarbons (PAHs) by Manganese Peroxidase in Reactors Containing Organic Solvents. Univ Santiago Compostela.
Haiyan, R., Shulan, J., ud din Ahmad, N., Dao, W., and Chengwu, C. (2007). Degradation Characteristics and Metabolic Pathway of 17α-Ethynylestradiol by Sphingobacterium Sp. JCR5. Chemosphere 66, 340–346. doi:10.1016/j.chemosphere.2006.04.064
Haritash, A. K., and Kaushik, C. P. (2009). Biodegradation Aspects of Polycyclic Aromatic Hydrocarbons (PAHs): a Review. J. Hazard. Mater. 169, 1–15. doi:10.1016/j.jhazmat.2009.03.137
He, J., Robrock, K. R., and Alvarez-Cohen, L. (2006). Microbial Reductive Debromination of Polybrominated Diphenyl Ethers (PBDEs). Environ. Sci. Technol. 40, 4429–4434. doi:10.1021/es052508d
Heidler, J., and Halden, R. U. (2007). Mass Balance Assessment of Triclosan Removal during Conventional Sewage Treatment. Chemosphere 66, 362–369. doi:10.1016/j.chemosphere.2006.04.066
Helbling, D. E., Johnson, D. R., Honti, M., and Fenner, K. (2012). Micropollutant Biotransformation Kinetics Associate with WWTP Process Parameters and Microbial Community Characteristics. Environ. Sci. Technol. 46, 10579–10588. doi:10.1021/es3019012
Jones, V., Gardner, M., and Ellor, B. (2014). Concentrations of Trace Substances in Sewage Sludge from 28 Wastewater Treatment Works in the UK. Chemosphere 111, 478–484. doi:10.1016/j.chemosphere.2014.04.025
Joss, A., Andersen, H., Ternes, T., Richle, P. R., and Siegrist, H. (2004). Removal of Estrogens in Municipal Wastewater Treatment under Aerobic and Anaerobic Conditions: Consequences for Plant Optimization. Environ. Sci. Technol. 38, 3047–3055. doi:10.1021/es0351488
Kochany, J., and Maguire, R. J. (1994). Abiotic Transformations of Polynuclear Aromatic Hydrocarbons and Polynuclear Aromatic Nitrogen Heterocycles in Aquatic Environments. Sci. Total Environ. 144, 17–31. doi:10.1016/0048-9697(94)90424-3
Komolafe, O., Bowler, B., Dolfing, J., Mrozik, W., and Davenport, R. J. (2019). Quantification of Polybrominated Diphenyl Ether (PBDE) Congeners in Wastewater by Gas Chromatography with Electron Capture Detector (GC-ECD). Anal. Methods 11, 3474–3482. doi:10.1039/c9ay00266a
Komolafe, O., Mrozik, W., Dolfing, J., Acharya, K., Vassalle, L., Mota, C. R., et al. (2021). Occurrence and Removal of Micropollutants in Full-Scale Aerobic, Anaerobic and Facultative Wastewater Treatment Plants in Brazil. J. Environ. Manage. 287, 112286. doi:10.1016/j.jenvman.2021.112286
Levenspiel, O. (1999). Chemical Reaction Engineering. Ind. Eng. Chem. Res. 38, 4140–4143. doi:10.1021/ie990488g
Lindström, A., Buerge, I. J., Poiger, T., Bergqvist, P.-A., Müller, M. D., and Buser, H.-R. (2002). Occurrence and Environmental Behavior of the Bactericide Triclosan and its Methyl Derivative in Surface Waters and in Wastewater. Environ. Sci. Technol. 36, 2322–2329. doi:10.1021/es0114254
Liu, Z.-h., Kanjo, Y., and Mizutani, S. (2009). Removal Mechanisms for Endocrine Disrupting Compounds (EDCs) in Wastewater Treatment - Physical Means, Biodegradation, and Chemical Advanced Oxidation: a Review. Sci. Total Environ. 407, 731–748. doi:10.1016/j.scitotenv.2008.08.039
Liu, Z.-h., Lu, G.-n., Yin, H., Dang, Z., and Rittmann, B. (2015). Removal of Natural Estrogens and Their Conjugates in Municipal Wastewater Treatment Plants: a Critical Review. Environ. Sci. Technol. 49, 5288–5300. doi:10.1021/acs.est.5b00399
Luo, Y., Guo, W., Ngo, H. H., Nghiem, L. D., Hai, F. I., Zhang, J., et al. (2014). A Review on the Occurrence of Micropollutants in the Aquatic Environment and Their Fate and Removal during Wastewater Treatment. Sci. Total Environ. 473-474, 619–641. doi:10.1016/j.scitotenv.2013.12.065
Novak, J. T., Goldsmith, C. D., Benoit, R. E., and O'Brien, O. H. (1985). Biodegradation of Methanol and Tertiary Butyl Alcohol in Subsurface Systems. Water Sci. Technol. 17 (9), 71–85.
Pan, Y., Tsang, D. C. W., Wang, Y., Li, Y., and Yang, X. (2016). The Photodegradation of Polybrominated Diphenyl Ethers (PBDEs) in Various Environmental Matrices: Kinetics and Mechanisms. Chem. Eng. J. 297, 74–96. doi:10.1016/j.cej.2016.03.122
Pérez, S., Guillamón, M., and Barceló, D. (2001). Quantitative Analysis of Polycyclic Aromatic Hydrocarbons in Sewage Sludge from Wastewater Treatment Plants. J. Chromatogr. A 938, 57–65. doi:10.1016/s0021-9673(01)01338-3
Pessoa, G. P., de Souza, N. C., Vidal, C. B., Alves, J. A. C., Firmino, P. I. M., Nascimento, R. F., et al. (2014). Occurrence and Removal of Estrogens in Brazilian Wastewater Treatment Plants. Sci. Total Environ. 490, 288–295. doi:10.1016/j.scitotenv.2014.05.008
Petrie, B., McAdam, E. J., Hassard, F., Stephenson, T., Lester, J. N., and Cartmell, E. (2014). Diagnostic Investigation of Steroid Estrogen Removal by Activated Sludge at Varying Solids Retention Time. Chemosphere 113, 101–108. doi:10.1016/j.chemosphere.2014.04.051
Pitter, P., and Chudoba, J. (1990). Biodegradability of Organic Substance in the Aquatic Environment. American Chemical Society. https://agris.fao.org/agris-search/search.do?recordID=US19910113373.
Racz, L., and Goel, R. K. (2010). Fate and Removal of Estrogens in Municipal Wastewater. J. Environ. Monit. 12, 58–70. doi:10.1039/b917298j
Ren, Y.-X., Nakano, K., Nomura, M., Chiba, N., and Nishimura, O. (2007). Effects of Bacterial Activity on Estrogen Removal in Nitrifying Activated Sludge. Water Res. 41, 3089–3096. doi:10.1016/j.watres.2007.04.028
Roh, H., Subramanya, N., Zhao, F., Yu, C.-P., Sandt, J., and Chu, K.-H. (2009). Biodegradation Potential of Wastewater Micropollutants by Ammonia-Oxidizing Bacteria. Chemosphere 77, 1084–1089. doi:10.1016/j.chemosphere.2009.08.049
Saeed, T., Ali, L. N., Al-Bloushi, A., Al-Hashash, H., Al-Bahloul, M., Al-Khabbaz, A., et al. (2011). Effect of Environmental Factors on Photodegradation of Polycyclic Aromatic Hydrocarbons (PAHs) in the Water-Soluble Fraction of Kuwait Crude Oil in Seawater. Mar. Environ. Res. 72, 143–150. doi:10.1016/j.marenvres.2011.07.004
Salgado, R., Noronha, J. P., Oehmen, A., Carvalho, G., and Reis, M. A. M. (2010). Analysis of 65 Pharmaceuticals and Personal Care Products in 5 Wastewater Treatment Plants in Portugal Using a Simplified Analytical Methodology. Water Sci. Tech. 62, 2862–2871. doi:10.2166/wst.2010.985
Shi, J., Fujisawa, S., Nakai, S., and Hosomi, M. (2004). Biodegradation of Natural and Synthetic Estrogens by Nitrifying Activated Sludge and Ammonia-Oxidizing Bacterium Nitrosomonas Europaea. Water Res. 38, 2323–2330. doi:10.1016/j.watres.2004.02.022
Sornalingam, K., McDonagh, A., and Zhou, J. L. (2016). Photodegradation of Estrogenic Endocrine Disrupting Steroidal Hormones in Aqueous Systems: Progress and Future Challenges. Sci. Total Environ. 550, 209–224. doi:10.1016/j.scitotenv.2016.01.086
Stiborova, H., Vrkoslavova, J., Lovecka, P., Pulkrabova, J., Hradkova, P., Hajslova, J., et al. (2015). Aerobic Biodegradation of Selected Polybrominated Diphenyl Ethers (PBDEs) in Wastewater Sewage Sludge. Chemosphere 118, 315–321. doi:10.1016/j.chemosphere.2014.09.048
Tamura, I., and Yamamoto, H. (2012). Biodegradation and Photodegradation of Selected Antimicrobial/Antifungal Agents in the Aquatic Environment and Their Sorption onto Sediments. Kankyou Kagaku 22, 113–119. doi:10.5985/jec.22.113
Trably, E., Patureau, D., and Delgenes, J. P. (2005). “Polycyclic Aromatic Hydrocarbons (PAHs) Removal during Anaerobic and Aerobic Sludge Treatments,” in Environmental Chemistry (Berlin: Springer), 431–440.
UKTAG (2013). Updated Recommendations on Environmental Standards; River Basin Management. http://www.wfduk.org/sites/default/files/Media/Environmental%20standards/UKTAG%20Environmental%20Standards%20Phase%203%20Final%20Report%2004112013.pdf.
Urase, T., and Kikuta, T. (2005). Separate Estimation of Adsorption and Degradation of Pharmaceutical Substances and Estrogens in the Activated Sludge Process. Water Res. 39, 1289–1300. doi:10.1016/j.watres.2005.01.015
Veetil, P. G. P., Nadaraja, A. V., Bhasi, A., Khan, S., and Bhaskaran, K. (2012). Degradation of Triclosan under Aerobic, Anoxic, and Anaerobic Conditions. Appl. Biochem. Biotechnol. 167, 1603–1612. doi:10.1007/s12010-012-9573-3
Verlicchi, P., Al Aukidy, M., and Zambello, E. (2012). Occurrence of Pharmaceutical Compounds in Urban Wastewater: Removal, Mass Load and Environmental Risk after a Secondary Treatment-A Review. Sci. Total Environ. 429, 123–155. doi:10.1016/j.scitotenv.2012.04.028
Villar-Navarro, E., Baena-Nogueras, R. M., Paniw, M., Perales, J. A., and Lara-Martín, P. A. (2018). Removal of Pharmaceuticals in Urban Wastewater: High Rate Algae Pond (HRAP) Based Technologies as an Alternative to Activated Sludge Based Processes. Water Res. 139, 19–29. doi:10.1016/j.watres.2018.03.072
Vuono, D. C., Regnery, J., Li, D., Jones, Z. L., Holloway, R. W., and Drewes, J. E. (2016). rRNA Gene Expression of Abundant and Rare Activated-Sludge Microorganisms and Growth Rate Induced Micropollutant Removal. Environ. Sci. Technol. 50, 6299–6309. doi:10.1021/acs.est.6b00247
Wang, L., Zhang, X., Tao, Y., and Hu, J. (2013). Adsorption and Biodegradation of Typical Estrogens in Activated Sludge under Aerobic Conditions. Harbin.
Xia, X. (2013). Microbial Degradation of Polybrominated Diphenyl Ethers: Current and Future. J. Bioremed Biodeg 4, e130. doi:10.4172/2155-6199.1000e130
Xue, W., Wu, C., Xiao, K., Huang, X., Zhou, H., Tsuno, H., et al. (2010). Elimination and Fate of Selected Micro-organic Pollutants in a Full-Scale Anaerobic/anoxic/aerobic Process Combined with Membrane Bioreactor for Municipal Wastewater Reclamation. Water Res. 44, 5999–6010. doi:10.1016/j.watres.2010.07.052
Yen, J. H., Liao, W. C., Chen, W. C., and Wang, Y. S. (2009). Interaction of Polybrominated Diphenyl Ethers (PBDEs) with Anaerobic Mixed Bacterial Cultures Isolated from River Sediment. J. Hazard. Mater. 165, 518–524. doi:10.1016/j.jhazmat.2008.10.007
Yi, T., Harper, W. F., Holbrook, R. D., and Love, N. G. (2006). Role of Particle Size and Ammonium Oxidation in Removal of 17α-Ethinyl Estradiol in Bioreactors. J. Environ. Eng. 132, 1527–1529. doi:10.1061/(asce)0733-9372(2006)132:11(1527)
Zhang, Y., and Zhou, J. L. (2005). Removal of Estrone and 17β-Estradiol from Water by Adsorption. Water Res. 39, 3991–4003. doi:10.1016/j.watres.2005.07.019
Keywords: environmental fate, biodegradation, micropollutants, wastewater treatment, effluent quality prediction
Citation: Komolafe O, Mrozik W, Dolfing J, Acharya K, Vassalle L, Mota CR and Davenport R (2021) Fate of four Different Classes of Chemicals Under Aerobic and Anaerobic Conditions in Biological Wastewater Treatment. Front. Environ. Sci. 9:700245. doi: 10.3389/fenvs.2021.700245
Received: 25 April 2021; Accepted: 07 June 2021;
Published: 21 June 2021.
Edited by:
Zhi Wang, Innovation Academy for Precision Measurement Science and Technology (CAS), ChinaReviewed by:
Hyeong-Moo Shin, University of Texas at Arlington, United StatesShuying Li, Zhejiang University, China
Copyright © 2021 Komolafe, Mrozik, Dolfing, Acharya, Vassalle, Mota and Davenport. This is an open-access article distributed under the terms of the Creative Commons Attribution License (CC BY). The use, distribution or reproduction in other forums is permitted, provided the original author(s) and the copyright owner(s) are credited and that the original publication in this journal is cited, in accordance with accepted academic practice. No use, distribution or reproduction is permitted which does not comply with these terms.
*Correspondence: Oladapo Komolafe, b2tvbW9sYWZlQGdmbGVudi5jb20=