- 1Pegasus Technical Services, Inc., Cincinnati, OH, United States
- 2Office of Research and Development, United States Environmental Protection Agency, Cincinnati, OH, United States
Opportunistic pathogens (OPs) are natural inhabitants and the predominant disease causative biotic agents in municipal engineered water systems (EWSs). In EWSs, OPs occur at high frequencies and concentrations, cause drinking-water-related disease outbreaks, and are a major factor threatening public health. Therefore, the prevalence of OPs in EWSs represents microbial drinking water quality. Closely or routinely monitoring the dynamics of OPs in municipal EWSs is thus critical to ensuring drinking water quality and protecting public health. Monitoring the dynamics of conventional (fecal) indicators (e.g., total coliforms, fecal coliforms, and Escherichia coli) is the customary or even exclusive means of assessing microbial drinking water quality. However, those indicators infer only fecal contamination due to treatment (e.g., disinfection within water utilities) failure and EWS infrastructure issues (e.g., water main breaks and infiltration), whereas OPs are not contaminants in drinking water. In addition, those indicators appear in EWSs at low concentrations (often absent in well-maintained EWSs) and are uncorrelated with OPs. For instance, conventional indicators decay, while OPs regrow with increasing hydraulic residence time. As a result, conventional indicators are poor indicators of OPs (the major aspect of microbial drinking water quality) in EWSs. An additional or supplementary indicator that can well infer the prevalence of OPs in EWSs is highly needed. This systematic review argues that Legionella as a dominant OP-containing genus and natural inhabitant in EWSs is a promising candidate for such a supplementary indicator. Through comprehensively comparing the behavior (i.e., occurrence, growth and regrowth, spatiotemporal variations in concentrations, resistance to disinfectant residuals, and responses to physicochemical water quality parameters) of major OPs (e.g., Legionella especially L. pneumophila, Mycobacterium, and Pseudomonas especially P. aeruginosa), this review proves that Legionella is a promising supplementary indicator for the prevalence of OPs in EWSs while other OPs lack this indication feature. Legionella as a dominant natural inhabitant in EWSs occurs frequently, has a high concentration, and correlates with more microbial and physicochemical water quality parameters than other common OPs. Legionella and OPs in EWSs share multiple key features such as high disinfectant resistance, biofilm formation, proliferation within amoebae, and significant spatiotemporal variations in concentrations. Therefore, the presence and concentration of Legionella well indicate the presence and concentrations of OPs (especially L. pneumophila) and microbial drinking water quality in EWSs. In addition, Legionella concentration indicates the efficacies of disinfectant residuals in EWSs. Furthermore, with the development of modern Legionella quantification methods (especially quantitative polymerase chain reactions), monitoring Legionella in ESWs is becoming easier, more affordable, and less labor-intensive. Those features make Legionella a proper supplementary indicator for microbial drinking water quality (especially the prevalence of OPs) in EWSs. Water authorities may use Legionella and conventional indicators in combination to more comprehensively assess microbial drinking water quality in municipal EWSs. Future work should further explore the indication role of Legionella in EWSs and propose drinking water Legionella concentration limits that indicate serious public health effects and require enhanced treatment (e.g., booster disinfection).
Introduction
Microbial drinking water quality (i.e., the presence and concentrations of microbes) in municipal engineered water systems (EWSs, including drinking water distribution systems or DWDSs and building premise plumbing systems or PSSs) strongly affects public health (Ashbolt, 2015a; Zhang and Lu, 2021). Pathogenetic microbes in EWSs may infect end consumers and cause life-threatening diseases or disease outbreaks. Microbes enter municipal EWSs with finished water from treatment plants. Drinking water treatment facilities effectively remove and inactivate microbes, especially pathogens, with disinfection (e.g., chlorination, chloramination, and ozonation) and other treatment barriers (e.g., filtration) before discharging finished water to DWDSs (McGuire, 2006; Okpara et al., 2011; Zhang and Liu, 2019). However, residual microbes in finished water could be still active or viable [or viable-but-non-culturable (VBNC)] and enter DWDSs (Haas, 1999; Schoenen, 2002; Liu et al., 2009; Marciano-Cabral et al., 2010; Falkinham et al., 2015a; Lin et al., 2017; Chen et al., 2018; Guo et al., 2021). Microbes also invade EWSs because of deficiencies in design and operations of the systems, installation and maintenance of water mains and distal pipes, and intrusion during cross-connections (actual or potential connections between potable water supplies and non-potable sources), back-pressure (occurs when the supply pressure is lower than the local pipe pressure; a type of backflow), and back-siphonage (due to local negative pressure transients or a vacuum effect; another type of backflow) (Herrick, 1997; Payment, 1999; LeChevallier et al., 2003; Lee and Schwab, 2005; Makris et al., 2014; Beer et al., 2015; Gerba and Pepper, 2015; Lu et al., 2016; Leslie et al., 2021).
Certain microbes after entering or invading municipal EWSs survive, (re)grow, and become natural inhabitants of drinking water. Most natural inhabitants in EWSs are harmless to humans. However, an important group of natural inhabitants in EWSs, water-based opportunistic pathogens (OPs), is the leading cause of drinking-water-based disease outbreaks, significantly threatens public health, and causes serious social-economic losses (Wang et al., 2013; Whiley et al., 2014b; Ashbolt, 2015b; Beer et al., 2015; Falkinham et al., 2015b; Benedict et al., 2017; Liu et al., 2017; El-Chakhtoura et al., 2018; Cullom et al., 2020). For instance, water-based nontuberculous mycobacteria (NTM) infections annually cause over 16,000 hospitalizations and a total hospitalization cost of over 426 million United States (US) dollars in the US (Collier et al., 2012). In addition, OPs survive and (re)grow in EWSs (Hwang et al., 2012; El-Chakhtoura et al., 2018; Douterelo et al., 2019; Zhang and Liu, 2019) even in the presence of disinfectant residuals (Haas, 1999; LeChevallier, 1999; van der Kooij, 2003; Zhang et al., 2019; Zhang et al., 2021a) and under high-shear turbulent flow conditions (Lehtola et al., 2007). Furthermore, conventional water quality indicators (e.g., total and fecal coliforms, thermotolerant coliforms, Escherichia coli, enterococci, and fecal streptococci) and fecal contaminants (i.e., enteric and primary pathogens) that commonly exist in (contaminated) natural waterbodies occur in EWSs at low frequencies and concentrations and are often absent from well-maintained systems (Falkinham, 2020; Zhang and Lu, 2021). Therefore, the presence and concentrations of OPs are the major aspect of microbial water quality in municipal EWSs. Because OPs in drinking water frequently cause disease outbreaks, monitoring the dynamics of OPs in municipal EWSs is an important and urgent task for the water industry and governmental agencies to deeply understand microbial drinking water quality and to better protect public health.
Examining all or dominant (pathogenic) microbes to fully understand microbial drinking water quality in EWSs is technically intense, expensive, time consuming, and often impractical and unnecessary (Gerba, 2015). Therefore, water utilities and public health agencies in the US and many other countries assess microbial drinking water quality mainly or even exclusively through quantifying conventional indicator microbes (i.e., fecal indicators). Fecal indicators infer fecal contamination from warm-blooded animals and the presence of enteric/primary pathogens due to treatment system failure, water pipe breaks, cross-connections, and backflow (Cohen and Shuval, 1973; Edberg et al., 2000; Ashbolt et al., 2001; Lee and Schwab, 2005; Tallon et al., 2005; Gerba, 2015; Lu et al., 2016). Examining fecal indicators using traditional culture-based methods (e.g., the Colilert™ Test for both total coliforms and E. coli) is straightforward and cost effective. However, as discussed above, conventional fecal indicators and fecal contaminants (i.e., enteric pathogens) have low concentrations and are often absent in EWSs. Fecal contaminants and indicators are thus not the major aspect of microbial drinking water quality, especially in (developed) countries where secondary disinfection is mandatory (e.g., the US) and where municipal EWSs are well-maintained and clean (e.g., the Netherlands) (Smeets et al., 2009; Zhang and Liu, 2019; Zhang and Lu, 2021). In addition, fecal indicators behave distinctively from OPs in EWSs (Falkinham et al., 2015b; Falkinham, 2015; Lu et al., 2016; Wang et al., 2017; Perrin et al., 2019a; Zhang and Lu, 2021). For instance, the concentrations of OPs generally increase, while fecal indicators decay with increasing hydraulic residence time (HRT) of distributed water (Falkinham, 2020; Zhang and Lu, 2021). OPs have high resistance to disinfectant residuals (and thus have high concentrations), while fecal indicators are susceptible to disinfectant residuals (and thus have low concentrations or are even absent) in EWSs (Falkinham, 2015). Therefore, conventional fecal indicators do not indicate OPs (the major aspect of microbial drinking water quality) in EWSs (Lehtola et al., 2007; LeChevallier, 2019), and a supplementary or additional indicator that specifically infers the prevalence of OPs is highly needed. To date, a microorganism indicating the presence and concentrations of OPs in municipal EWSs has not been fully developed, evaluated, and validated. To the best of our knowledge, most countries do not require a routine minoring of OPs in municipal EWSs using an indicator microorganism.
This comprehensive literature review aims to purpose an appropriate indicator microorganism that can infer the presence and concentrations of water-based OPs (the major aspect of microbial drinking water quality) in municipal EWSs. We first explain why conventional fecal indicators fail to indicate OPs or microbial drinking water quality in EWSs and why an indicator of OPs is highly needed. We then propose novel critical criteria for selecting an appropriate indicator of the presence and concentrations of OPs in drinking water. We argue that selecting such a supplementary indicator from common OPs in EWSs is appropriate. We finally comprehensively compare the behavior (i.e., occurrence, growth and regrowth, spatiotemporal variations in concentrations, resistance to disinfectant residuals, and responses to physicochemical water quality parameters) of major OPs (i.e., Legionella especially L. pneumophila, Mycobacterium, and Pseudomonas especially P. aeruginosa). The comparison proves that Legionella is a promising supplementary indicator for the presence and concentrations of OPs in EWSs while other OPs do not have this indication feature. This comprehensive literature review for the first time proposes and confirms an appropriate indicator microorganism (Legionella) that well infers water-based OPs and reflects microbial drinking water quality in municipal EWSs, adding new insights into the current literature. This review also systematically summarizes the behavior of Legionella and other common OPs in municipal drinking water, whereas such information is meaningful to developing more effective OP control strategies and promoting public health.
Fecal Indicators Fail to Indicate Opportunistic Pathogens in Municipal Engineered Water Systems
Examining conventional fecal indicators is the most common practice worldwide evaluating drinking water quality in municipal EWSs. However, fecal indicators indicate only the presence of enteric pathogens and/or fecal contaminants (mainly due to treatment failure in water utilities) and cannot infer the presence and concentrations of water-based OPs (the major aspect of microbial drinking water quality). Fecal indicators fail to indicate OPs because of three reasons. First, fecal indicators and OPs in municipal EWSs have distinct microbial community compositions. Fecal indicators mainly include E. coli, Bacteroides (more common than E. coli in the gastrointestinal tract of humans; a good indicator for recent fecal contamination), fecal coliforms, and fecal streptococci (enterococci) (Gerba, 2015). Common water-based OPs in EWSs are Legionella (especially L. pneumophila), Pseudomonas (especially P. aeruginosa), Mycobacterium or NTM (especially M. avium and Mycobacterium avium complex or MAC; MAC includes mainly two closely related species, M. avium and M. intracellulare), Aeromonas, Acinetobacter baumannii, Flavobacterium, Acanthamoeba, Vermamoeba vermiformis (formerly Hartmannella vermiformis), and Naegleria fowleri (Rusin et al., 1997; Ford, 1999; Falkinham et al., 2001; van der Kooij, 2003; Pryor et al., 2004; September et al., 2004; Lau and Ashbolt, 2009; ldi et al., 2010; Wingender and Flemming, 2011; Wang et al., 2012a; van der Wielen and van der Kooij, 2013; Delafont et al., 2014; Wang et al., 2014; Ashbolt, 2015b; Falkinham et al., 2015b; Boyle et al., 2015; Lu et al., 2016; Kim et al., 2017; Delafont et al., 2018; Perrin et al., 2019b; Dai et al., 2019; Isaac and Sherchan, 2019; Zhang et al., 2021b; Zhang and Lu, 2021). The general term “water-based” or “water-related” is more appropriate than “water-borne” to describe OPs in EWSs (Ashbolt, 2015a).
Second, the unique environment (e.g., high surface to volume ratio and low organic matter concentration) of EWSs favors the persistence and (re)growth of water-based OPs over fecal indicators (Falkinham et al., 2015b). Common OPs, such as Legionella, Mycobacterium, and Pseudomonas (re)grow well in such an environment (Ribas et al., 2000; Declerck et al., 2009; van der Wielen and van der Kooij, 2013; Lu et al., 2016; Bédard et al., 2019) (Figure 1). Because of their significant (re)growth, the concentrations of OPs increase with the HRT of distributed water along EWSs (Falkinham, 2015). For instance, the concentrations of Legionella, Mycobacterium, P. aeruginosa, Acanthamoeba, and V. vermiformis downstream in a DWDS in the US were generally greater than those at the entry point of the system (Lu et al., 2016). A recent study similarly found that the concentrations of four common OPs (Legionella, Mycobacterium, Pseudomonas, and V. vermiformis) increased with the HRT of distributed water in four full-scale chloraminated DWDSs in the US (Zhang et al., 2021b). OPs are thus native inhabitants of EWSs and occur more frequently and at greater concentrations downstream in the systems (Falkinham et al., 2015b; Wang et al., 2017). By contrast, the most common fecal indicator E. coli persists but does not substantially regrow in EWSs (Edberg et al., 2000; Abberton et al., 2016; Lu et al., 2016). In general, the concentrations of E. coli and other fecal indicators fall when drinking water moves along EWSs because of dilution and non-growth (Falkinham, 2015) (Figure 1).
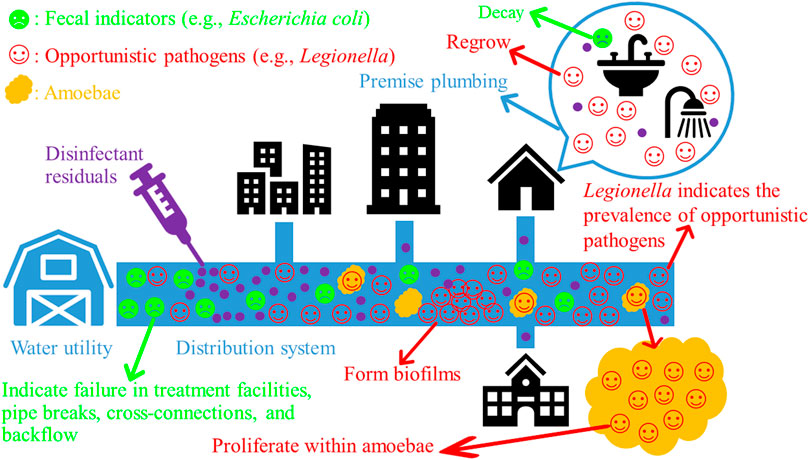
FIGURE 1. The decay of conventional fecal indicators and the (re)growth of opportunistic pathogens (OPs) along municipal engineered water systems (EWSs). Bacterial OPs often proliferate within their amoeba hosts and form biofilms in EWSs. Drawn using PowerPoint (version 2102, Microsoft Office Professional Plus 2019) (Microsoft Corporation, Redmond, Washington, USA).
Third, water-based OPs are generally (much) more resistant to disinfectant residuals (which are commonly dosed to distribution systems) than fecal indicators in EWSs (Sobsey, 1989; Payment, 1999; Falkinham, 2015; Falkinham, 2020; Zhang and Lu, 2021). For instance, the C·t99.9% [the product of disinfectant concentration C (mg·L−1) and contact time t (min) to kill 99.9% of cells] value of free chlorine for medium-grown E. coli was 0.09 mg·min·L−1 at 23 °C (Taylor et al., 2000). The C·t99.9% values of free chlorine for medium-grown (51 to 204 mg·min·L−1 at 23 °C) and drinking-water-grown (551 to 1,552 mg·min·L−1 at 23 °C) M. avium were significantly greater. Similarly, planktonic P. aeruginosa is more resistant than planktonic E. coli to free chlorine, chloramines, and ozone (Ross et al., 1976). Legionella is also more resistant to disinfectants than E. coli and other coliform bacteria (Kim et al., 2002). For example, L. pneumophila was more resistant to ozone than E. coli in a continuous flow reactor (Botzenhart et al., 1993). Residuals of free oxidants (an electrochemical disinfectant) in the range from 4.05 to 19.35 mg·min·L−1 at 21 °C killed 95.9% to 98.6% of L. pneumophila in tap water but eradicated E. coli (Delaedt et al., 2008). In addition, exposure of clinical isolates of A. baumannii to free chlorine (4 mg·L−1) for 2 min in deionized water did not significantly reduce their culturable cell counts (p > 0.05) (Karumathil et al., 2014). On the other hand, certain studies showed that L. pneumophila is more sensitive to disinfectants than E. coli. For instance, a study found that planktonic L. pneumophila serogroup 1 (C·t99% value 15 mg·min·L−1) was more susceptible to monochloramine than planktonic E. coli (C·t99% value 37 mg·min·L−1) in the bulk water of a laboratory-scale plumbing system (Cunliffe, 1990). Another study found that L. pneumophila was more susceptible to chlorine dioxide than E. coli (Botzenhart et al., 1993). Future studies should more comprehensively compare the susceptibilities between OPs and fecal indicators to disinfectants or disinfectant residuals.
Because the environment of EWSs favors the (re)growth of OPs and OPs are resistant to disinfectant residuals, OPs (re)grow better and occur more frequently and at much greater concentrations than conventional fecal indicators in EWSs (Figure 1). For instance, culturable E. coli survived only 5 d in the biofilms and 9 d in the bulk water of a drinking water Propella biofilm reactor, but M. avium (more than 4 weeks) and L. pneumophila (more than 2 weeks) survived much longer in the biofilms and water (Lehtola et al., 2007). Similarly, L. pneumophila and P. aeruginosa spiked to flow-through reactors (continuously perfused with tap water) entered and persisted for weeks in biofilms on rubber/plastic plumbing materials, but spiked Enterobacter nimipressuralis [a coliform species (Kämpfer et al., 2008)] was absent from the biofilms (Moritz et al., 2010). In two full-scale DWDSs in South Australia, E. coli and total coliforms were absent from the bulk water, but Legionella, L. pneumophila, and MAC were present in the water throughout the year (Whiley et al., 2014a). In 10 full-scale DWDSs in the US, L. pneumophila occurred in 14 out of 573 bulk water samples, while total coliforms and E. coli were absent in those 573 samples (LeChevallier, 2019). In hot tap water from six buildings on a university campus in Poland, E. coli had low concentrations [approximately 0 genome copy number per liter (GCN·L−1; GCN also stands for “gene copy number” in this review) or below the limit of detection], whereas Legionella (4.52 × 103 to 1.56 × 104 GCN·L−1 for positive sampling sites) and L. pneumophila (0.98 × 103 to 1.20 × 104 GCN·L−1 for positive sampling sites) frequently occurred at much greater concentrations (Wolf-Baca and Siedlecka, 2019). In four full-scale reclaimed water distribution systems in the US, Legionella, Mycobacterium, Pseudomonas, and Aeromonas occurred more frequently and generally had much greater concentrations than total coliforms, fecal coliforms, E. coli, and enterococci (Jjemba et al., 2010).
The three reasons discussed above confirm that water-based OPs and conventional fecal indicators behave differently in drinking water with distinct community compositions, (re)growth patterns, and susceptibilities to disinfectant residuals. Therefore, fecal indicators are uncorrelated with and fail to indicate OPs in EWSs (ldi et al., 2010; Falkinham, 2015; Wang et al., 2017; Perrin et al., 2019a; Isaac and Sherchan, 2019). Indeed, a human fecal indicator Bacteroides and an enteric pathogen E. coli O157:H7 were absent from the bulk water of a DWDS in a metropolitan area in the US (Lu et al., 2016), the sediments of 18 drinking water storage tanks (DWSTs) across 10 states in the US (Lu et al., 2015), and the tap and shower water from a simulated house premise plumbing in the US (Lu et al., 2017). However, representative OPs including Mycobacterium, Legionella, P. aeruginosa, Acanthamoeba, and V. vermiformis frequently occurred at those sites at relatively high concentrations. In addition, in the hot water from hot water distal outlets in 28 commercial buildings in the US, the concentration of HPC bacteria was uncorrelated with Legionella positivity (p 0.788) and explained only 0.68% of the variation in Legionella positivity (Pierre et al., 2019). In the shower water from a US hospital and cooling water from pilot-scale cooling towers, the concentrations of both HPC bacteria and adenosine triphosphate failed to accurately predict Legionella positivity or colonization (Duda et al., 2015).
The current routine examination of microbial drinking water quality by detecting conventional fecal indicators fails to assess the negative health effects of water-based OPs in EWSs and is thus biased. First, quantifying fecal indicators is the main or even exclusive means of examining microbial drinking water quality in municipal EWSs (Edberg et al., 2000; Gerba, 2015; Wen et al., 2020). To the best of our knowledge, the revised Drinking Water Directive of the European Union (EU) is the only regulation that requires monitoring Legionella in DWDSs for risk assessment [parametric value: < 1,000 colony forming units (CFU) per liter] (The Council of the EU, 2020). Most current drinking water standards and regulations enforce only the quantification of fecal indicators. For instance, through the Safe Drinking Water Act, the US Environmental Protection Agency (US EPA) has established the National Primary Drinking Water Regulations, which require using total coliforms (including fecal coliforms and E. coli) as an indicator of potentially harmful bacteria and set an enforceable Maximum Contaminant Level (MLC) for total coliforms in drinking water to be 5.0% (i.e., total-coliform-positive samples in a month being less than 5.0%) (US EPA, 1989). The same Regulations set a non-enforceable Maximum Contaminant Level Goal (MCLG) for both total coliforms and Legionella to be 0 mg·L−1 [sic] in drinking water. Similarly, the EU sets the maximum number of E. coli, coliform bacteria, and intestinal enterococci to be 0 per 100 mL of tap water (The Council of the EU, 2020). As discussed above, fecal indicators are uncorrelated with OPs in EWSs, and the presence, absence, and/or concentrations of fecal indicators in drinking water cannot reflect the negative public health effects of water-based OPs. Second, despite the critical role of EWSs in maintaining drinking water quality, most standards regulate only the levels of fecal indicators in the water entering DWDSs (i.e., finished water or plant effluent) and leaving PPSs (e.g., tap water). In the US, the Revised Total Coliform Rule is the only regulation that requires quantifying fecal indicators (i.e., total coliforms and E. coli) throughout DWDSs (US EPA, 2013). Third, even though Legionella frequently occurs in municipal EWSs and causes drinking-water-based disease (i.e., Legionnaires’ disease) outbreaks, routine monitoring of Legionella in such systems is rare (Hamilton et al., 2019). Some researchers even inadequately argued for the removal of the requirement for routine monitoring of Legionella in EWSs from all drinking water guidelines (Whiley, 2017). At least, at distal sites of PPSs in hospitals, water authorities or medical personnel need to routinely monitor Legionella to protect the health of patients (Baron et al., 2019). In summary, the routine examination of microbial drinking water quality by detecting fecal indicators unreasonably neglects OPs, which are the major disease causative biotic agent in municipal drinking water. The primary reason for the negligence is that an indicator inferring the presence and concentrations of water-based OPs (the major aspect of microbial drinking water quality) in EWSs is not fully developed, evaluated, and validated. Therefore, we dedicate this literature review to proposing an appropriate indicator (i.e., Legionella) of OPs that can better reflect microbial drinking water quality in municipal EWSs. Water utilities and authorities may use Legionella as a supplementary indicator in conjunction with conventional fecal indicators to more comprehensively evaluate microbial drinking water quality and to better protect public health (Zhang et al., 2021b).
Novel Criteria for Selecting an Appropriate Indicator of Opportunistic Pathogens in Municipal Engineered Water Systems
Scientists have developed multiple criteria for selecting indicator microorganisms to reflect microbial water quality (Gerba, 2015; Wen et al., 2020). Existing criteria are meaningful for selecting indicators of fecal contamination and/or enteric pathogens in drinking water, surface water, recreational water, and wastewater (Gerba, 2015). However, since fecal indicators and OPs in drinking water behave distinctly (Falkinham et al., 2015b; Wang et al., 2017), not all those existing criteria are suitable for selecting an indicator of OPs in municipal EWSs. We need to revise those criteria to select an appropriate indicator microorganism for the presence and concentrations of OPs (the major aspect of microbial drinking water quality) in municipal EWSs. Such revised criteria are missing in the literature. We propose the following novel critical criteria to select an appropriate indicator microorganism of water-based OPs in EWSs:
I) The indicator is one important or dominant member of the natural inhabitants (e.g., OPs) of drinking water. This property of the indicator ensures that the presence and concentration of the indicator suggest the presence and concentration of OPs as a whole group.
II) The indicator occurs in municipal EWSs at a high frequency and concentration and can be feasibly examined through multiple approaches. This property of the indicator makes its detection and quantification easy, flexible (i.e., can be assayed by different methods), and affordable.
III) The presence and concentration of the indicator in EWSs closely correlate with the presence and concentrations of water-based OPs and other critical physicochemical and microbial drinking water quality parameters. This property of the indicator ensures that when the indicator occurs at a high (or low) concentration, the overall concentration of OPs and the public health risks of the drinking water are likely high (or low).
IV) The occurrence and concentration of the indicator indicate the efficacies of disinfectant residuals. This property links the presence and concentration of the indicator to the efficacy of secondary disinfection (a critical treatment barrier). Therefore, a high frequency of occurrence and concentration of the indicator could suggest drinking water quality deterioration (due to disinfectant residual decay and/or consumption) and the need for enhanced or additional treatment (such as booster disinfection and distribution system cleaning/upgrade).
The Potentials of Natural Inhabitants to Indicate Opportunistic Pathogens in Municipal Engineered Water Systems
Conventional fecal indicators behave distinctly from and are uncorrelated with water-based OPs in municipal EWSs (Falkinham, 2020). Therefore, we need to select a novel indicator that can indicate the presence and concentrations of OPs in drinking water for more effective OP control and enhanced public health protection. This novel indicator should be selected from common OPs rather than from non-pathogenic natural inhabitants in municipal EWSs (Table 1). The occurrence and concentrations of non-pathogenic species (which are harmless to humans) do not correlate with the public health risks posed by OPs in drinking water. By contrast, a dominant OP could well indicate the health risks posed by OPs. That dominant OP is itself opportunistically pathogenic and poses public health risks. In addition, the presence and concentration of that dominant OP could well correlate with the presence and concentrations of other OPs because OPs in EWSs share many characteristics such as high resistance to disinfectant residuals, biofilm formation, and proliferation within amebae (Falkinham, 2015; Zhang and Lu, 2021). Therefore, this review selects a novel indicator of OPs from common OPs in municipal EWSs. On the basis of a comprehensive comparison among the behavior of dominant OPs (Legionella, Mycobacterium, and Pseudomonas) in drinking water, we conclude that Legionella is a promising indicator microorganism inferring the public health risks posed by water-based OPs in municipal EWSs while other OPs lack this indication feature (Zhang et al., 2021b).
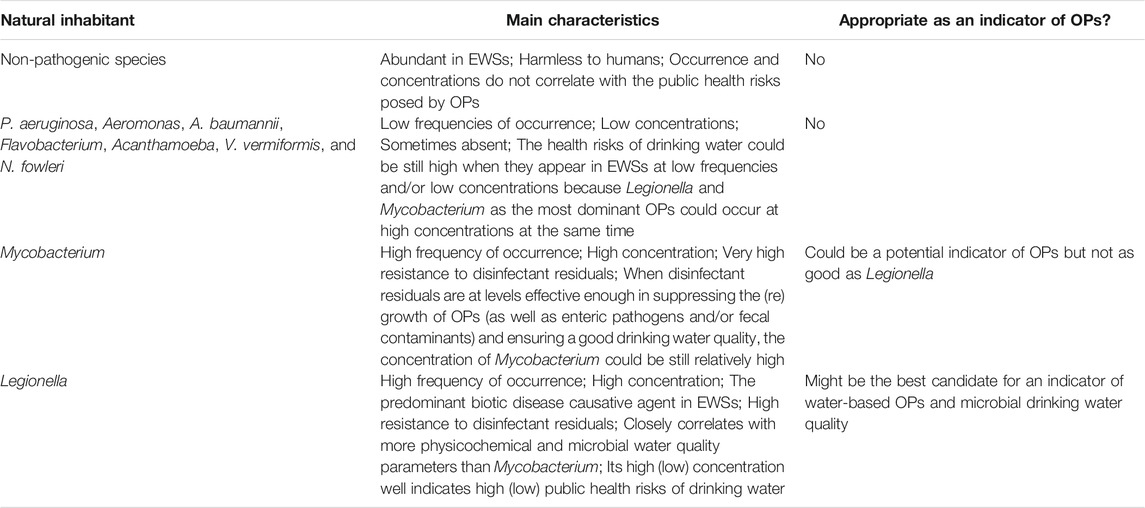
TABLE 1. A brief comparison for the appropriateness of common natural inhabitants in municipal engineered water systems (EWSs) as indicators of opportunistic pathogens (OPs).
Water-based OPs in EWSs have a diverse community with multiple bacterial and amoebal species, while the most common and dominant OPs are Legionella (especially L. pneumophila), Mycobacterium (especially NTM, M. avium, and MAC), and Pseudomonas (especially P. aeruginosa) (Falkinham, 2015; Falkinham, 2020; Lu et al., 2017; Lu et al., 2016; Lu et al., 2015; Zhang and Lu, 2021; Zhang et al., 2021b). Other OPs (such as certain amoebae) occur in drinking water at lower frequencies and/or lower concentrations and thus do not accurately suggest the overall health risks of OPs in municipal EWSs (Table 1). For instance, low concentrations of other OPs in EWSs do not imply that the public health risks of OPs in the drinking water are low, because the three dominant OPs could occur at high concentrations and have a high potential for infection of immunocompromised and immunocompetent populations. Amoebae in drinking water could have strong correlations with bacterial OPs such as Legionella and Mycobacterium (but not always) (Wang et al., 2012a; Wang et al., 2012b; Lu et al., 2016; Valciņa et al., 2019; Zhang et al., 2021b). The association between Legionella and its amoeba hosts mainly exists in pipe biofilms in municipal EWSs (Storey et al., 2004a; Declerck et al., 2007; Lau and Ashbolt, 2009; Thomas and Ashbolt, 2011; Wang et al., 2012a), whereas the detection and monitoring of Legionella are mainly for planktonic Legionella in bulk water. Therefore, the amoeba-Legionella relationships do not warrantee that amoebae are a good indicator of OPs in municipal EWSs. Pseudomonas (especially P. aeruginosa) as a common OP in EWSs have (much) lower concentrations and frequencies of occurrence than Legionella and Mycobacterium (Wang et al., 2012a; Lu et al., 2016; Lu et al., 2017; Zhang et al., 2021b). Therefore, the occurrence and concentration of Pseudomonas (especially P. aeruginosa) also do not fully reflect the health risks of OPs, and Pseudomonas (especially P. aeruginosa) is not a good indicator of OPs in municipal EWSs (Table 1).
By contrast, Legionella (especially L. pneumophila) and Mycobacterium (especially NTM, M. avium, and MAC) are the two most dominant OPs in municipal EWSs with comparably high frequencies of occurrence and concentrations (Wang et al., 2012a; Lu et al., 2016; Lu et al., 2017; Zhang et al., 2021b). Legionella (L. pneumophila) and Mycobacterium (M. avium) both have a sporadic occurrence nature in EWSs (Donohue et al., 2019). When either Legionella or Mycobacterium occurs at a high concentration, the overall concentration of OPs is high, and the corresponding drinking water poses significant public health risks, requiring enhanced treatment (e.g., booster disinfection) and distribution system cleaning or upgrade. Oppositely, when the concentration of either Legionella or Mycobacterium is low, the overall concentration of OPs would be low, and the corresponding drinking water would have a low risk of causing disease outbreaks. Therefore, either Legionella (especially L. pneumophila) or Mycobacterium (especially NTM, M. avium, and MAC) could be a good indicator of water-based OPs and their public health risks in municipal EWSs (Table 1).
Compared with Mycobacterium, Legionella could be a better indicator of water-based OPs in municipal EWSs. First, Legionella (especially L. pneumophila) is the first leading cause of drinking-water-based disease outbreaks (Beer et al., 2015; Benedict et al., 2017) and correlates with more OPs than Mycobacterium. For instance, in four full-scale chloraminated DWDSs in the US, the concentration of Legionella significantly and positively correlated with those of Mycobacterium (p 0.042), Pseudomonas (p < 0.001), and total OPs (i.e., the sum concentration of all four examined OPs; p < 0.001) (Zhang et al., 2021b). Legionella also had a nearly significant positive linear correlation with V. vermiformis (p 0.054). Therefore, the presence of Legionella at a relatively high concentration indicates a well-built growth condition for other bacterial OPs (e.g., Mycobacterium and Pseudomonas especially P. aeruginosa) and their amoeba hosts (e.g., Acanthamoeba, N. fowleri, and V. vermiformis) in municipal EWSs. By contrast, Mycobacterium had a significant positive linear correlation with only Legionella (p 0.042). Similarly, a multiple linear regression reveals that Legionella closely correlated with more physicochemical water quality parameters than Mycobacterium in the four chloraminated DWDSs (Zhang et al., 2021b). Legionella had significant linear correlations with water temperature (p 0.002), total chlorine residual concentration (p 0.012), free ammonia concentration (p 0.037), and total trihalomethane (THM) concentration (p 0.019) (negative correlations except for with free ammonia concentration). Mycobacterium had significant linear correlations with only total chlorine residual concentration (p < 0.001) and total haloacetic acid concentration (p 0.018). Since Legionella correlates with more OPs and more critical physicochemical water quality parameters than Mycobacterium, the presence and concentration of Legionella reflect better the public health risks posed by OPs and even general drinking water quality in municipal EWSs. Second, Mycobacterium (M. avium) has a much higher resistance to disinfectant residuals than Legionella (L. pneumophila) and is thus insensitive to changes in disinfectant residual levels and efficacies (Falkinham, 2015). Therefore, when disinfectant residuals are at levels effective enough in suppressing the (re)growth of OPs (as well as enteric pathogens and/or fecal contaminants) and ensuring a good drinking water quality, the concentration of Mycobacterium (M. avium) could be relatively high, and using Mycobacterium (M. avium) as an indicator of water-based OPs might overestimate the public health risks of OPs in drinking water. Third, among the many OPs in EWSs, Legionella (L. pneumophila) is the most widely known and studied (Falkinham, 2015). Numerous culture-dependent and culture-independent assays are available for Legionella (L. pneumophila) detection and quantification (Wang et al., 2017). With the fast development of modern molecular techniques (especially quantitative polymerase chain reactions or qPCRs), assessing the concentration and diversity of Legionella (L. pneumophila) in drinking water is becoming more straightforward, easier to handle, more affordable, and faster (Girones et al., 2010; Lu et al., 2015; Lu et al., 2016; Wolf-Baca and Siedlecka, 2019; Zhang et al., 2021b). In conclusion, we argue that among the many OPs in municipal EWSs, Legionella is the most appropriate indicator of the presence and concentrations OPs (the major aspect of microbial drinking water quality) (Table 1).
Key Features of Legionella that Make It an Appropriate Indicator of Water-Based Opportunistic Pathogens in Engineered Water Systems
Legionella Is a Major Natural Inhabitant in Engineered Water Systems and Is Listed in Water Quality Regulations
Legionella is a major genus of natural inhabitants in municipal EWSs (Buse et al., 2012; Wang et al., 2012a; Serrano-Suárez et al., 2013; Whiley et al., 2014a; Ashbolt, 2015a; Schwake et al., 2015; Qin et al., 2017). As a dominant OP in municipal EWSs, Legionella has a high concentration and a high frequency of occurrence (Wang et al., 2012a; Whiley et al., 2014a; Lu et al., 2015; Lu et al., 2016; Lu et al., 2017; Qin et al., 2017; Zhang et al., 2021b). Legionella contains both non-pathogenic and pathogenic species. Approximately 50% of the 61 species in Legionella infect humans (Borella et al., 2005a; Lau and Ashbolt, 2009; Springston and Yocavitch, 2017; The International Organization for Standardization, 2017; Gomes et al., 2018; Viasus et al., 2019; Wu et al., 2019) and significantly threaten public health (Buse et al., 2012). In this genus, L. pneumophila (pneumophila means “lung-loving”) is the most common life-threatening species, causing over 80% of Legionella infections (Reingold et al., 1984; Stout and Yu, 1997; Yu et al., 2002; Hornei et al., 2007; Falkinham, 2015; Amemura-Maekawa et al., 2018). However, most or even all species in Legionella are potential OPs to humans (Fields, 1996; Borella et al., 2005a; Mercante and Winchell, 2015; Lesnik et al., 2016; Salinas et al., 2021). Therefore, the presence of Legionella in EWSs indicates the presence of multiple clinically-relevant Legionella species (e.g., L pneumophila, L. bozemanii, L. anisa, L. longbeachae, and L. londiniensis).
Because of its implications for microbial drinking water quality and public health risks, water authorities worldwide have made guidelines, standards, and regulations to monitor and control Legionella in drinking water (generally not for a routine examination, which normally examines only fecal indicators) (Van Kenhove et al., 2019; Wen et al., 2020). In the US, L. pneumophila is on the Drinking Water Contaminant Candidate List 3 (CCL 3) (US EPA, 2009), the CCL 4 (US EPA, 2016), and the draft CCL 5 (US EPA, 2021). In addition, Legionella is the only water-based OP in the National Primary Drinking Water Regulations (US EPA, 1989). In Europe, the Netherlands, France, and Germany enforce regulatory monitoring for Legionella in drinking water (Ashbolt, 2015b). The revised Drinking Water Directive of the EU even requires monitoring Legionella in DWDSs for risk assessment (The Council of the EU, 2020). In addition, Legionella is a must-measure biological parameter in almost all research studies surveying microbial drinking water quality in municipal EWSs. Since Legionella is a major OP in municipal EWSs and is listed in multiple drinking water regulations, Legionella can be developed to be an indicator of microbial drinking water quality reflecting the occurrence, concentration, and behavior of OPs as a whole group in EWSs.
Legionella Shares Many Critical Characteristics With Other Water-Based Opportunistic Pathogens
Water-based OPs in EWSs share several critical characteristics (Falkinham, 2015; 2020). They persist and (re)grow in EWSs, infect humans, tolerate high water temperatures, resist disinfectant residuals, and form biofilms (Figure 1). In addition, bacterial OPs such as Legionella resist the phagocytosis of and proliferate within their amoeba hosts (Figure 1). Because Legionella shares many critical characteristics with other OPs, the occurrence and concentration of Legionella would well indicate the occurrence and concentrations of other OPs, making Legionella an appropriate indicator of the public health risks posed by OPs in municipal EWSs.
Legionella Is a Major Disease Causative Biotic Agent in Municipal Engineered Water Systems
Legionella persists and (re)grows in EWSs (Falkinham et al., 2015b; Lu et al., 2016; Proctor et al., 2017; Wang et al., 2017; Salinas et al., 2021) and infects immunocompromised and immunocompetent populations, posing significant public health risks. Legionella infections are legionellosis (Borella et al., 2005a; Hornei et al., 2007), which contains community-acquired Legionnaires’ disease, outbreak-acquired Legionnaires’ disease, and Pontiac fever (Falkinham et al., 2015a). Legionnaires’ disease is a severe, life-threatening, multisystem disease involving pneumonia, while Pontiac fever is a self-limited, non-lethal, influenza-like disease (Fields et al., 2002; Lau and Ashbolt, 2009; Ashbolt, 2015a; Principe et al., 2017; Alexander et al., 2019). Legionella inhabits most natural and engineered aquatic environments and appears along its major reservoir, EWSs (from finished water or plant effluent to distal sites in buildings/houses) (Fields et al., 2002; Borella et al., 2005a; Whiley et al., 2014b; Falkinham et al., 2015a; Lu et al., 2015; Lu et al., 2016; Lu et al., 2017; Zhang et al., 2021b). Legionella in natural aquatic ecosystems (e.g., freshwater) has a low concentration and rarely infects humans (Lau and Ashbolt, 2009), while drinking water in engineered aquatic environments, especially EWSs, is the major source for legionellosis. Specifically, cooling towers, building PPSs (i.e., cold and hot water systems), household humidifiers, and pools/spas are the main contributors to legionellosis (Shands et al., 1985; Lin et al., 1998b; Hines et al., 2014; Demirjian et al., 2015; Springston and Yocavitch, 2017; Hamilton et al., 2018; Orkis et al., 2018).
Legionella in drinking water from municipal EWSs directly infects humans, whereas a direct transmission of legionellosis from infected persons or animals to non-infected persons is rare (US EPA, 2000; Correia et al., 2016; Beauté, 2017; Prussin et al., 2017; CDC, 2021). Drinking-water-based Legionella infects humans mainly through two pathways. First, inhalation of Legionella-containing aerosols (i.e., small droplets of drinking water in the air that contain Legionella) is the most common pathway (Hornei et al., 2007; Beauté, 2017; Prussin et al., 2017; CDC, 2021). For instance, immunocompromised and immunosuppressed people might get legionellosis by inhaling Legionella-containing aerosols generated from showerheads while showering (Brady, 1989; Schoen and Ashbolt, 2011). Therefore, showerheads are an important source of Legionella-containing aerosols and could be an important source of legionellosis (Cordes et al., 1981; Bollin et al., 1985; Muder et al., 1986; Collins et al., 2017; Hayes-Phillips et al., 2019). Second, aspiration or micro-aspiration of drinking water with Legionella (i.e., drinking water with Legionella accidentally goes into the lungs while drinking) is a less common pathway (Blatt et al., 1993; US EPA, 2000; Sabria and Yu, 2002; CDC, 2021). Legionella could also directly enter damaged skin (wound infection), but wound infection does not cause pulmonary diseases (Hornei et al., 2007).
From 2007 to 2008, 36 drinking-water-based disease outbreaks occurred in 24 states in the US and Puerto Rico, and 12 (33%) of the outbreaks were due to acute respiratory illness caused by Legionella (Brunkard et al., 2011). From 2009 to 2010, Legionella caused more outbreaks: Thirty three drinking-water-based disease outbreaks and 1,040 illnesses occurred in 17 states in the US, and Legionella caused 19 (58%) outbreaks and 72 (7%) illnesses (Hilborn et al., 2013). From 2011 to 2014, more than one dozen drinking-water-based disease outbreaks occurred annually in the US, resulting in hundreds of illnesses, more than 50 hospitalizations, and 6 to 7 deaths per year (Beer et al., 2015; Benedict et al., 2017). Legionella caused more than 50% of those outbreaks, nearly 90% of those hospitalizations, and all those deaths. The annual hospitalizations caused by community-acquired Legionnaires' disease are from 8,000 to 18,000 in the US (Marston et al., 1997), leading to an annual hospitalization cost for water-based Legionnaires’ disease in the US to be over 433 million US dollars (Collier et al., 2012; Falkinham et al., 2015b).
Legionella Survives in a Wide Water Temperature Range in Municipal Engineered Water Systems
Legionella survives in a relatively wide water temperature range, and certain Legionella species or strains are thermotolerant or thermophilic. Legionella (mainly L. pneumophila) survives and/or (re)grows in a water temperature range from approximately 20 °C to approximately 50 °C (Kusnetsov et al., 1996; Leoni et al., 2001; Fields et al., 2002; Kim et al., 2002; Huang et al., 2011; Springston and Yocavitch, 2017) with an optimal (re)growth temperature range from approximately 35 °C to approximately 45 °C (Katz and Hammel, 1987; Rogers et al., 1994; Fields et al., 2002; Mathys et al., 2008; Buse and Ashbolt, 2011; Springston and Yocavitch, 2017). Certain thermophilic/thermotolerant Legionella species or strains, including some L. pneumophila strains, even (re)grow in hot tap water at a temperature greater than 50 °C (Mathys et al., 2008; Lesnik et al., 2016). Therefore, municipal EWSs provide a suitable water temperature range for Legionella (re)growth and are the major reservoir for this genus.
Legionella Has a High Resistance to Disinfectant Residuals in Municipal Engineered Water Systems
Legionella inherently has a high resistance to disinfectant residuals. In the US and many other countries, water utilities dose disinfectant residuals such as free chlorine, combined chlorine (mainly monochloramine), and chlorine dioxide residuals to DWDSs as a treatment barrier to suppress microbial (re)growth and prevent distribution system upsets (Olivieri et al., 1986; Norton and LeChevallier, 1997; Gagnon et al., 2004; Hwang et al., 2012; Gillespie et al., 2014; Zhang et al., 2021b). The relatively high resistance of Legionella to disinfectant residuals provides it with ecological advantages in municipal EWSs. Therefore, Legionella has a relatively high frequency of occurrence and a high concentration in EWSs, especially in seasons and/or at locations where that treatment barrier is ineffective because of disinfectant residual decay and/or consumption.
Legionella Proliferates Within Amoebae in Municipal Engineered Water Systems
Legionella proliferates within its amoeba hosts in municipal EWSs (Figure 1). The proliferation significantly enhances its resistance to disinfectant residuals, persistence in drinking water, and pathogenicity (Kim et al., 2002; Campos et al., 2003; Borella et al., 2005a; Lau and Ashbolt, 2009; Marciano-Cabral et al., 2010; Berjeaud et al., 2016). For instance, L. pneumophila within A. polyphage cysts survived free chlorine at a high dose (50 mg·L−1) for 18 h at 25 °C (Kilvington and Price, 1990). A pure planktonic L. pneumophila culture was significantly more sensitive (p < 0.005) to free chlorine (initial concentration 2 to 3 mg·L−1, contact time up to 1 h, 30 °C) and chlorine dioxide (initial concentration 0.4 mg·L−1, contact time up to 1 h, 30 °C) than L. pneumophila co-cultured with Acanthamoeba (Dupuy et al., 2011). Similarly, the C·t99.99% value of free chlorine for pure planktonic L. pneumophila serogroup 1 was only 2.5 mg·min·L−1 (initial free chlorine concentration 0.5 mg·L−1, room temperature) (Cervero-Aragó et al., 2015). The same strain associated with Acanthamoeba was significantly more resistant to free chlorine (C·t99.99% value from 19 to 245 mg·min·L−1; initial free chlorine concentration 0.5, 1.2, or 2.5 mg·L−1; room temperature). In addition, L. pneumophila concentration (log10CFU per coupon) decreased by 1.47 to 2.07 units after exposure to free chlorine (initial concentration 0.5 mg·L−1, contact time 15 min to 24 h, 21 °C) in mixed-culture biofilms without V. vermiformis (Donlan et al., 2005). When V. vermiformis was present in the biofilms, L. pneumophila concentration decreased by only 0.30 to 0.90 units under the same chlorination condition. Compared with free chlorine, monochloramine (initial concentration 0.5 mg·L−1, contact time 15 min to 24 h, 21 °C) killed more L. pneumophila (concentration decreased by 1.77 to 2.29 units) in the biofilms without V. vermiformis. When V. vermiformis was present in the biofilms, monochloramine was less effective in killing L. pneumophila (concentration decreased by only 0.08 to 1.59 units under the same chloramination condition). Furthermore, compared with axenic planktonic L. gormanii, the resistance of Tetrahymena pyriformis (a ciliated protozoan) ingested L. gormanii to free chlorine (pH 7.0, 25 °C) was at least 50 folds greater (King et al., 1988). Legionella within amoebae is also more resistant to heavy metal ions such as copper and silver ions (Unger and Lück, 2012). Copper ions may appear in EWSs because of the use of copper or copper-containing pipes, whereas copper-silver ionization is effective in controlling Legionella in hospital water systems because of the strong antimicrobial activities of silver and copper ions (Liu et al., 1994; Lin et al., 1998a; Stout et al., 1998; Zhang et al., 2014; Zhang et al., 2016a; Zhang et al., 2016b). L. pneumophila in an endosymbiosis phase within A. polyphage was significantly more resistant to silver ions (0.1 mg·L−1), copper ions (1.0 mg·L−1), or the combination of silver (0.1 mg·L−1) and copper (1.0 mg·L−1) ions than a pure planktonic culture of L. pneumophila (Hwang et al., 2006).
The proliferation of Legionella within amoebae also enhances its tolerance to thermal treatment (Storey et al., 2004b). For instance, thermal treatment at 50 and 55 °C killed 99.99% of pure planktonic L. pneumophila serogroup 1 after 46 and 8 min, respectively (Cervero-Aragó et al., 2015). The contact time to kill 99.99% of L. pneumophila serogroup 1 associated with Acanthamoeba was significantly longer (745 min at 50 °C and 48 min at 55 °C). However, at 60 and 70 °C, L. pneumophila serogroup 1 associated with Acanthamoeba had the same tolerance to thermal treatment as the pure planktonic culture.
Legionella Forms Biofilms in Municipal Engineered Water Systems
In addition to the proliferation of Legionella within amoebae, biofilm formation (Figure 1) enhances the resistance of Legionella to disinfectant residuals (Momba et al., 2000; Wright, 2000; Donlan and Costerton, 2002; Kim et al., 2002; Loret et al., 2005; Bridier et al., 2011). For instance, the C·t99% value of iodine for well-water-grown L. pneumophila serotype 1 biofilm cells (> 960 mg·min·L−1) was significantly greater than that for the planktonic cells of well-water-grown L. pneumophila (200 mg·min·L−1) (Cargill et al., 1992). Planktonic L. pneumophila took a long time (28 d) to recover in tap water amended with 0.5 mg·L−1 of free chlorine, whereas free chlorine at 200 mg·L−1 (chlorination conducted in water, contact time 1 h) inhibited only moderately the growth of one- and two-month-old L. pneumophila biofilms on stainless steel (Cooper and Hanlon, 2010). In heterotrophic drinking water biofilms, the absolute concentration of naturally occurring L. pneumophila was stable (p > 0.05) when free chlorine residual concentration increased from 0 to 0.2 and to 1.2 mg·L−1, and the relative concentration of L. pneumophila against total cell numbers even slightly increased when free chlorine residual concentration increased from 0 to 0.2 and to 1.2 mg·L−1 (Gião et al., 2009).
Legionella Frequently Occurs in Municipal Engineered Water Systems at a Relatively High Concentration
Because Legionella survives and (re)grows in a wide water temperature range, resists disinfectant residuals, proliferates within amebae, and forms biofilms, it has a high frequency of occurrence (greater than approximately 40%) and concentration in municipal EWSs (Borella et al., 2005b; Leoni et al., 2005; Wang et al., 2012a; Lu et al., 2017; Qin et al., 2017; Isaac and Sherchan, 2019; Zhang et al., 2021b). Legionella concentration in the bulk water in the mains of DWDSs could reach 107 GCN·L−1 level or be even greater (109 GCN·L−1 level at dead-ends) (Whiley et al., 2014a). In the bulk water in DWSTs, Legionella concentration could reach 104 GCN·L−1 level (Qin et al., 2017). In the bulk water of PPSs, Legionella concentration could be greater than 106 GCN·L−1 (Wang et al., 2012a). In the sediments of DWSTs, Legionella concentration could reach 104 GCN·g−1 or 104 cell equivalents·g−1 level (Lu et al., 2015; Qin et al., 2017). At the end-points of PPSs (i.e., the inner surfaces of taps and showerheads), the highest concentration of Legionella in biofilms could be at 106 GCN·swab−1 level (Wang et al., 2012a).
Legionella Closely Correlates With Drinking Water Quality Parameters in Municipal Engineered Water Systems
The Close Correlations Between Legionella and Physicochemical Drinking Water Quality Parameters
Legionella often correlates with physicochemical drinking water quality parameters in municipal EWSs. For instance, in four full-scale chloraminated DWDSs in the US, Legionella had significant correlations with water temperature (p 0.002), total chlorine residual concentration (p 0.012), free ammonia concentration (p 0.037), and total THM concentration (p 0.019) (Zhang et al., 2021b). In a research center in Germany, Legionella concentration in the hot drinking water from a showerhead positively correlated with water temperature (49.2 to 57.9 °C, R2 0.730) although Legionella was uncorrelated with water temperature (7.6 to 16.9 °C) in the cold drinking water from the center (R2 0.097) (Lesnik et al., 2016). In addition, Legionella (L. pneumophila) commonly has a negative correlation with copper ion concentration in building hot water systems (HWSs) (p < 0.05) (Zacheus and Martikainen, 1994; Leoni et al., 2005) probably because copper ions have strong antimicrobial activities (Rogers et al., 1994; Borella et al., 2004; Cooper and Hanlon, 2010; Bargellini et al., 2011; Serrano-Suárez et al., 2013). Legionella also commonly has a negative correlation with disinfectant residual concentrations in EWSs (Zhang et al., 2021b). For instance, the concentration of Legionella in the tap biofilms from three laboratories in northern China was negatively associated with free and total chlorine residual concentrations in the bulk water (Liu et al., 2012). In 11 hospitals in San Antonio (Texas, USA) where Legionella appeared in the PPSs, average free chlorine residual concentration in patient-room tap water and the proportion of Legionella-positive sites negatively correlated (linear regression, R2 0.52, p 0.01) (Kool et al., 1999a). Therefore, a high frequency of occurrence and a high concentration of Legionella in drinking water indicate that disinfectant residuals as a treatment barrier at the current levels are ineffective in suppressing OP (re)growth and the public health risks of the drinking water could be high, requiring enhanced treatment or EWS upgrade. In other words, Legionella could indicate the public health risks of drinking water (mainly posed by water-based OPs) in municipal EWSs.
The Close Correlations Between Legionella and Microbial Drinking Water Quality Parameters
Legionella in EWSs closely correlates with microbial drinking water quality parameters. In general, Legionella positively correlates with major OPs such as Mycobacterium, Pseudomonas (especially P. aeruginosa), V. vermiformis, and Acanthamoeba in EWSs (Wang et al., 2012a; Serrano-Suárez et al., 2013; Lu et al., 2016). For instance, in the bulk water of four full-scale chloraminated DWDSs in the US, the concentration of Legionella significantly and positively correlated with those of Mycobacterium (p 0.042), Pseudomonas (p < 0.001), and total OPs (p < 0.001) (Zhang et al., 2021b).
In conclusion, Legionella closely correlates with multiple physicochemical and microbial drinking water quality parameters in municipal EWSs. The close correlations between Legionella and other OPs suggest that the presence and concentration of Legionella could infer the presence and concentrations of other major OPs. The close correlations between Legionella and physicochemical drinking water quality parameters (such as disinfectant residuals) further suggest that Legionella could indicate the overall drinking water quality and the potential public health risks posed by OPs in EWSs.
Legionella Indicates the Efficacies of Disinfectant Residuals and Explains Their Modes of Action in Engineered Water Systems
Legionella Indicates the Efficacies of Disinfectant Residuals in Engineered Water Systems
Disinfectant residuals at relatively high levels are effective in preventing the (re)growth of OPs in EWSs (Olivieri et al., 1986; Norton and LeChevallier, 1997; Gagnon et al., 2004; Hwang et al., 2012; Gillespie et al., 2014; Zhang and Lu, 2021). For instance, Legionella (L. pneumophila) was absent from the bulk water (finished water, DWSTs, water mains entering hospitals, and taps) of an EWS (Pennsylvania, USA) because free chlorine residual of approximately 0.2 mg·L−1 appeared throughout the system (States et al., 1987). The frequencies of occurrence of Legionella in tap water (26%) and water-main biofilms (0%) in a chloraminated EWS in the US were significantly lower than those (64% for tap water and 43% for biofilms) in a no-residual EWS in Norway (Waak et al., 2018). In the effluents of simulated household water heaters, Legionella concentration negatively correlated with the concentrations of disinfectant residuals (free chlorine or chloramines) in the influents of the heaters (Spearman’s rank correlation coefficients between −0.752 and −0.019) (Wang et al., 2015). Similarly, the concentration of Legionella in the bulk water of simulated DWDSs (sampled 14 months after the start-up) negatively correlated with chloramine residual concentrations (Spearman’s rank correlation coefficients between −0.908 and −0.693, p < 0.01) (Wang et al., 2012b). In the hot water from 67 buildings in Finland, chlorine residual inhibited the (re)growth of Legionella (Spearman’s rank correlation coefficient between chlorine residual level and Legionella concentration −0.19, p < 0.01) (Zacheus and Martikainen, 1994). A recent study found that the distal site/outlet (hot water) positivity of Legionella for 28 buildings in the US was uncorrelated with free chlorine residual level in the incoming cold water of the buildings (Pierre et al., 2019). However, free chlorine residual still prevented the (re)growth of Legionella in those buildings: Cold water from water mains entering the buildings (free chlorine residual 0.34 mg·L−1, Legionella concentration 1.4 × 104 CFU·L−1, Legionella positivity 3.7%) and cold water storage tanks (free chlorine residual 0.23 mg·L−1, Legionella concentration 3.8 × 103 CFU·L−1, Legionella positivity 10.3%) had much greater free chlorine residual concentration than hot water from hot water return lines (free chlorine residual 0.07 mg·L−1, Legionella concentration 3.6 × 105 CFU·L−1, Legionella positivity 26.7%) and hot water distal outlets (free chlorine 0.05 mg·L−1, Legionella concentration 4.2 × 105 CFU·L−1, Legionella positivity 30.4%) and thus much lower Legionella concentration and positivity.
Since disinfectant residuals are effective in inhibiting the (re)growth of OPs in EWSs, injecting disinfectants into building water systems reduces the frequencies of occurrence and concentrations of OPs. For instance, the positivity of Legionella at the cold and hot water distal outlets significantly decreased from approximately 60% to less than 10% after two hospitals in the US injected chlorine dioxide into the incoming water mains (Zhang et al., 2009). Likewise, after a hospital (Pennsylvania, USA) injected monochloramine into the HWS of a building, the distal site/outlet positivity of culturable Legionella was significantly lower compared with the control building (no monochloramine dosing) or the same building before the chloramination (Baron et al., 2014; Duda et al., 2014; Baron et al., 2015). In a hospital building (Modena, Italy), injecting monochloramine into a HWS (target concentration 1.5 to 3.0 mg·L−1, continuous application) significantly reduced the frequencies of occurrence of L. pneumophila in the hot water from heaters, return loops, and distal outlets from 97.0% (of 32 samples) to 13.3% (of 60 samples) (first-year application) (Marchesi et al., 2011; Marchesi et al., 2012). Meanwhile, the geometric means of L. pneumophila concentration for the positive hot water samples dramatically dropped from 1.7 × 104 to 3.4 × 102 CFU·L−1 after the dosing of monochloramine (first-year application). Over three years after the dosing of monochloramine, Legionella appeared in only 8 hot water samples (9.5% of 84 samples) with a low concentration (3.3 × 102 CFU·L−1, geometric mean for the 8 positive samples) (Marchesi et al., 2013).
As discussed above, because Legionella concentration has a general negative correlation with disinfectant residual levels, Legionella well indicates the efficacy of an important treatment barrier in municipal EWSs, secondary disinfection (i.e., dosing and maintaining disinfectant residuals) (Zhang et al., 2021b). When Legionella occurs in EWSs at a high frequency and/or a high concentration, the treatment barrier is ineffective in preventing the (re)growth of Legionella and other OPs, where the OPs might have a high potential causing disease (such as legionellosis) outbreaks. Therefore, Legionella occurring at a high frequency and/or concentration indicates drinking water quality deterioration and requires enhanced treatment (such as booster disinfection) and/or EWS cleaning/upgrade. Oppositely, drinking water from an EWS where Legionella occurs at a low frequency and/or a low concentration generally has a high quality and will less likely cause disease outbreaks due to the presence of OPs.
Monochloramine Is More Effective Than Free Chlorine in Controlling Legionella in Engineered Water Systems
Monochloramine has a long-lasting bactericidal effect and is more effective than free chlorine in controlling Legionella in municipal EWSs (Kool, 2001; Lin et al., 2011; Buse et al., 2012; Springston and Yocavitch, 2017). Therefore, the prevalence of Legionella in chloraminated EWSs is lower than that in chlorinated EWSs, suggesting that drinking water from chloraminated EWSs is less likely to cause outbreaks of legionellosis (Kool, 2001; Kim et al., 2002; Lin et al., 2011; Buse et al., 2012). Among 32 hospitals in the US where drinking-water-associated, hospital-acquired Legionnaires’ disease outbreaks occurred at least once per hospital from 1979 to 1997, free chlorine was the disinfectant residual in the drinking water for 31 hospitals, and monochloramine was the disinfectant residual in the drinking water for only one (Kool et al., 1999b; Kool et al., 2000). By contrast, for 48 hospitals in the US without a drinking-water-associated, hospital-acquired Legionnaires’ disease outbreak from 1979 to 1997, free chlorine was the disinfectant residual for 36 hospitals, and monochloramine was the disinfectant residual for 12. Therefore, hospitals supplied with drinking water with monochloramine residual (adjusted odds ratio for outbreaks of Legionnaires’ disease 1.0) are significantly less likely to have drinking-water-associated, hospital-acquired Legionnaires’ disease outbreaks than hospitals with free chlorine residual in drinking water (adjusted odds ratio for outbreaks of Legionnaires’ disease 10.2). Similarly, 16% of 38 hospitals in the US that had outbreaks/cases of hospital-acquired Legionnaires’ disease used drinking water with monochloramine residual, while 46% of 114 hospitals in the US without an outbreak/case of hospital-acquired Legionnaires’ disease used drinking water with monochloramine residual (Heffelfinger et al., 2003). As a result, the adjusted odds ratio (0.20) for definite cases or outbreaks of hospital-acquired Legionnaires’ disease in a hospital using drinking water with monochloramine residual is low.
Legionella Explains the Modes of Action of Free Chlorine and Monochloramine Residuals in Engineered Water Systems
Free chlorine has a higher oxidation-reduction (redox) potential than monochloramine and is thus a stronger oxidant and biocide (Table 2) (Akin et al., 1982; Wolfe et al., 1984; Kouame and Haas, 1991; Gagnon et al., 2004; Lee et al., 2011b; Chiao et al., 2014; Zhang et al., 2018). Therefore, free chlorine is more effective than monochloramine in killing planktonic Legionella. For instance, the C·t99.98% to 99.99% values of free chlorine (1 to 5 mg·min·L−1, room temperature or 30 °C) against planktonic L. pneumophila (Dupuy et al., 2011; Cervero-Aragó et al., 2015) were significantly lower than the C·t99.9% to 99.99% values of monochloramine (16 to 65 mg·min·L−1, 25 to 35 °C) against planktonic L. pneumophila (Dupuy et al., 2011; Jakubek et al., 2013).
However, monochloramine residual is more effective than free chlorine residual in controlling Legionella in municipal EWSs. The greater effectiveness of monochloramine residual in preventing Legionella (re)growth in EWSs is due to three reasons. First, the majority of Legionella cells in EWSs are in biofilms/sediments and within protozoans (e.g., amoebae) (Stout et al., 1985; Lin et al., 1998b; Fields et al., 2002; Batté et al., 2003; Lau and Ashbolt, 2009; Abdel-Nour et al., 2013; Ashbolt, 2015a; Falkinham, 2015). Approximately 95% of the biomass in EWSs is in biofilms and sediments on pipe walls, and only 5% of the biomass is in bulk water (Block, 1992; Flemming et al., 2002; Wingender and Flemming, 2011). For instance, in a pilot-scale HWS, more than 98.5% of Legionella cells lived in the pipe biofilms (Saby et al., 2005). Monochloramine well penetrates biofilms and controls biofilm contamination (LeChevallier et al., 1988; Norton and LeChevallier, 1997; Lee et al., 2011b; Pressman et al., 2012). By contrast, the rapid reaction between free chlorine and organic matter of biofilm origin greatly prevents free chlorine from penetrating biofilms (Chen and Stewart, 1996; Grobe et al., 2002; Bridier et al., 2011). For example, exposing a 526 µm thick P. aeruginosa biofilm to a flowing free chlorine solution (18.6 mg·L−1) for 3 h only raised free chlorine concentration at the substratum to 1.8 mg·L−1 (i.e., approximately 10% of the bulk free chlorine level) (Chen and Stewart, 1996). Similarly, free chlorine level in dual-species biofilms of P. aeruginosa and K. pneumoniae was only 20% or less of the free chlorine level in the bulk liquid (De Beer et al., 1994). Therefore, monochloramine residual penetrates biofilms faster and more completely than free chlorine residual in municipal EWSs (Lee et al., 2018). Indeed, the maximum initial penetration rate of monochloramine to nitrifying biofilms was approximately 170 times that of free chlorine (equivalent chlorine levels) (Lee et al., 2011b). Because monochloramine penetrates biofilms faster and more completely but free chlorine only inactivates cells on biofilm surfaces contacting bulk water (Kelsey, 2014; Lee et al., 2018), monochloramine is more effective in killing biofilm cells of Legionella. For instance, monochloramine and free chlorine at the same C·t value of 270 mg·min·L−1 (initial concentration 1.5 mg·L−1 for both disinfectants) reduced L. pneumophila concentration in mixed-culture bacterial biofilms by approximately 99.7% and approximately 87.4%, respectively (Donlan et al., 2001). In addition, monochloramine is more effective than free chlorine in killing biofilm cells of other bacterial species: The C·t> 99.9% value of monochloramine (3 mg·min·L−1) against K. pneumoniae attached to glass slides was significantly lower than the C·t99.6% value of free chlorine (150 mg·min·L−1) (LeChevallier et al., 1988). The slow and incomplete biofilm penetration of free chlorine is an important reason for the relatively strong resistance of biofilm cells to free chlorine (Grobe et al., 2002).
Second, the association between Legionella and its amoeba hosts significantly enhances the resistance of Legionella to free chlorine (Cervero-Aragó et al., 2015) rather than monochloramine probably because monochloramine penetrates amoebae more completely. For instance, free chlorine with a C·t value of 5 mg·min·L−1 (30 °C) killed 99.98% of pure planktonic L. pneumophila but only 98.74% of L. pneumophila co-cultured with Acanthamoeba (p < 0.005) (Dupuy et al., 2011). However, monochloramine (C·t value 2 mg·min·L−1, 30 °C) was comparably effective (p > 0.005) in killing pure planktonic L. pneumophila and L. pneumophila co-cultured with Acanthamoeba. In addition, monochloramine is more effective in killing free-living amoebae (i.e., Acanthamoeba, Naegleria, and Vermamoeba) than free chlorine (Dupuy et al., 2014) although opposite evidence does exist (De Jonckheere and Van de Voorde, 1976; Ercken et al., 2003; Loret and Greub, 2010; Mogoa et al., 2011). Therefore, monochloramine is more effective than free chlorine in killing amoeba-associated Legionella. For instance, monochloramine at 0.5 mg·L−1 killed 84.51% (contact time 3 h) or 97.43% (contact time 24 h) of biofilm-associated L. pneumophila in the presence of V. vermiformis (Donlan et al., 2005). Free chlorine at 0.5 mg·L−1 killed much less biofilm-associated L. pneumophila in the presence of V. vermiformis at a contact time of 3 h (78.62%) or 24 h (87.41%).
Third, since free chlorine is more reactive and has a higher redox potential (Table 2) than monochloramine, monochloramine residual is more stable and easier to maintain in EWSs (Wolfe et al., 1984; Cunliffe, 1990; Vikesland et al., 1998; Vikesland and Valentine, 2000; Kim et al., 2002; Dupuy et al., 2011; Gall et al., 2015; Zhang et al., 2018). Moreover, monochloramine has a wider working pH range than free chlorine in water (Ercken et al., 2003; Lin et al., 2011; Springston and Yocavitch, 2017; Baron et al., 2019).
Numerous Methods Are Available to Detect and Quantify Legionella in Engineered Water Systems
Numerous methods are available to recover, identify, and/or quantify Legionella in drinking water from EWSs. Those methods include the “gold standard” culture-based assays (e.g., with special media or amoebal co-culture), molecular assays through analyzing DNA/RNA/proteins (e.g., qPCRs, high-throughput DNA sequencing, and flow cytometry), and phenotypic assays (e.g., immunoassays) (Lee et al., 2011a; Aw and Rose, 2012; Wang et al., 2017; Zhang et al., 2021a).
Among the various available Legionella detection, recovery, and quantification assays, standard culture-based methods and (regular or traditional) qPCRs are the most widely used (Whiley and Taylor, 2016). Standard culture-based assays as the gold standard detect only culturable Legionella in drinking water (Marinelli et al., 2017). However, Legionella and other bacteria, including other OPs, in (chloraminated/chlorinated) EWSs could and often enter a VBNC state (Alleron et al., 2008; Kirschner, 2016; Casini et al., 2018; Cullom et al., 2020; Sciuto et al., 2021). VBNC Legionella is alive and infective and produces virulence proteins (or, at least retains its virulence) (Oliver, 2010; Alleron et al., 2013; Dietersdorfer et al., 2018). However, a standard culture-based approach cannot detect or recover VBNC Legionella (Marinelli et al., 2017; Whiley, 2017; Párraga-Niño et al., 2018). Therefore, standard or traditional culture-based assays underestimate the prevalence and public health risks of Legionella in municipal drinking water. On the other hand, traditional or regular qPCRs as a very common method for Legionella detection and quantification in EWSs detect total DNA or genetic markers of Legionella, including those from both live (culturable and VBNC) and dead Legionella cells (Dusserre et al., 2008; Wang et al., 2012a; Whiley et al., 2014a; Taylor et al., 2014; Lu et al., 2015; Lu et al., 2016; Lu et al., 2017; Qin et al., 2017; Waak et al., 2018; Isaac and Sherchan, 2019; Zhang et al., 2021b). Therefore, traditional qPCRs (significantly) overestimate the health risks of Legionella in EWSs because dead Legionella cells no longer infect humans (Fleischmann et al., 2021). One promising approach to resolving this overestimation issue is propidium monoazide qPCRs (PMA-qPCRs). PMA is a DNA intercalating molecule that penetrates only membrane-damaged or membrane-compromised cells (i.e., dead cells), binds to DNA within the dead cells, and inhibits DNA amplification during subsequent qPCRs (Nocker et al., 2007; Li and Chen, 2013; Golpayegani et al., 2019; Guo et al., 2021). The DNA or genetic markers of Legionella within membrane-intact (i.e., live, including culturable and VBNC) cells are not bound to PMA and are normally amplified during qPCRs. Therefore, PMA-qPCRs detect only live (culturable and VBNC) Legionella cells and more accurately estimate the public health risks of Legionella. Researchers have successfully applied PMA-qPCRs to detect live Legionella (L. pneumophila) cells in various settings including drinking water (Yáñez et al., 2011; Slimani et al., 2012; Li et al., 2015; Bonetta et al., 2017; Bonetta et al., 2018; Kontchou and Nocker, 2019). Using PMA-qPCRs to detect Legionella (and other microbes) has certain limitations such as incomplete reduction/suppression of the qPCR signals of DNA from dead cells, especially when qPCR amplicons are short or when the abundance of dead cells is high (Pan and Breidt, 2007; Kralik et al., 2010; Luo et al., 2010; Yáñez et al., 2011; Taylor et al., 2014; Ditommaso et al., 2015; Codony et al., 2020). In addition to PMA-qPCRs, reverse transcription qPCRs (RT-qPCRs) are a useful tool for detecting viable Legionella in (drinking) water (Bej et al., 1991; Boss et al., 2018).
A comprehensive summary of the traditional and novel Legionella recovery, identification, and quantification methods is beyond the scope of this literature review. Interested readers may consult a few publications for the details of those methods (Marre et al., 2001; Fields, 2007; Buse et al., 2012; Kirschner, 2016; Whiley and Taylor, 2016; Wang et al., 2017; Párraga-Niño et al., 2018; Baume et al., 2019; Ezenarro et al., 2020; Walker and McDermott, 2021). Because of its frequent occurrence, relatively high concentration, and various quantification methods, Legionella is a promising indicator of water-based OPs (the major aspect of microbial drinking water quality) in municipal EWSs.
Discussion and Future Work
Our early work preliminarily proposed that Legionella could be an indicator of non-fecal pathogens in municipal DWDSs (Lu et al., 2016). Our recent study examined the dynamics of dominant OPs in four full-scale DWDSs and confirmed that Legionella is a promising indicator of water-based OPs and microbial drinking water quality in municipal EWSs (Zhang et al., 2021b). The current systematic review greatly expands our previous argument and provides convincing reasons for the appropriateness of Legionella as an indicator of water-based OPs (the major aspect of microbial drinking water quality) in municipal EWSs. However, this review does not imply that Legionella should be used as a sole indicator to infer microbial drinking water quality (Huang et al., 2021). First, Mycobacterium is highly resistant to disinfectant residuals (Falkinham, 2015) and could be more resistant to chloramines than Legionella (Lin et al., 2011). Therefore, in a chloraminated EWS, a low frequency of occurrence and a low concentration of Legionella do not necessarily indicate a low health risk from Mycobacterium. In this case, water utilities might use Mycobacterium and Legionella in combination to better examine microbial drinking water quality (Lu et al., 2017; Huang et al., 2021). Future work needs to comprehensively compare the appropriateness of Legionella and Mycobacterium as indicators of water-based OPs and microbial drinking water quality. Evaluating the combined use of Legionella and Mycobacterium as indicators of microbial water quality is also necessary. Second, this review does not imply that we shall abandon the routine monitoring of conventional fecal indicators in municipal EWSs. Even though conventional fecal indicators do not indicate the presence and concentrations of OPs in drinking water, they do well indicate issues in treatment utilities (e.g., failure of disinfection processes), EWS problems (i.e., infiltration, backflow, and water main breaks), and the presence of enteric pathogens and/or fecal contaminants in drinking water. Therefore, this systematic review does not argue substituting Legionella for conventional fecal indicators as the sole indicator of microbial drinking water quality. Instead, this review argues that Legionella as an additional or supplementary indicator for microbial water quality should be used in conjunction with conventional fecal indicators. The combined use of Legionella and fecal indicators as microbial drinking water quality indicators would help more comprehensively understand the health risks of drinking water in municipal EWSs. Future work needs to explore how to use Legionella and conventional fecal indicators in combination to better monitor microbial drinking water quality and better protect public health.
This review focuses on convincing the water industry that Legionella is an appropriate supplementary indicator microorganism inferring the prevalence of water-based OPs (the major aspect of microbial drinking water quality) in municipal EWSs. When an indicator microorganism is proposed, carefully selecting a threshold (i.e., a numerical permissible limit) of the indicator that indicates a health risk or requires an action to be taken is important. For instance, when the concentration of the indicator exceeds a certain threshold, a boil-water-advisory should be issued, and enhanced treatment (i.e., booster disinfection) is required. Future work should propose Legionella limits (i.e., concentration limits and positivity limits) for drinking water that indicate serious public health risks and require enhanced or additional treatment.
This review grouped municipal DWDSs and building PPSs to EWSs for the ease of discussion. However, microbial growth conditions between DWDSs and PPSs are significantly different (Zhang et al., 2021b). In addition, the legal landscapes of water responsibility between DWDSs and PPSs are distinct. For instance, general microbial water quality monitoring is typically done only in DWDSs rather than in PPSs. Legionella as an indicator of microbial water quality in those two systems thus has distinct roles. Future work needs to clarify how to appropriately use Legionella as an indicator of water-based OPs and microbial water quality in these two different systems.
Conclusion
Legionella as an important natural inhabitant frequently occurs in municipal EWSs with a relatively high concentration. Opportunistically pathogenetic species in the genus Legionella (mainly L. pneumophila but include many other species) in EWSs frequently cause drinking-water-based disease (e.g., Legionnaires’ disease) outbreaks and pose significant public health risks. Quantification of Legionella in EWSs with modern molecular-based assays (especially qPCRs) is affordable and fast. Legionella in EWSs closely correlates with major physicochemical and microbial water quality parameters, especially the occurrence and concentrations of water-based OPs. Biofilms/sediments and amoebae are the main niches of Legionella in EWSs, and the association of Legionella with biofilms/sediments and amoebae significantly enhances the already high resistance of Legionella to disinfectant residuals. Legionella indicates the efficacies and explains the modes of action of disinfectant residuals in EWSs. For instance, monochloramine is more effective than free chlorine in controlling Legionella in EWSs because monochloramine penetrates biofilms/sediments/amoebae faster and more completely and has a long-lasting antimicrobial/bactericidal effect (i.e., more stable than free chlorine). Those features of Legionella make it a promising indicator of the prevalence of water-based OPs and microbial water quality in municipal EWSs.
Author Contributions
CZ performed the literature search, analyzed previous results, and drafted and revised the article. JL was the principal investigator who designed the study and finalized the article. All authors contributed to the article and approved the submitted version.
Author’s Disclaimer
The views in this paper belong to the authors and do not represent the opinions and policies of the US EPA. Mention of trade names or commercial products does not constitute endorsement or recommendation by the US EPA for use.
Conflict of Interest
CZ was employed by Pegasus Technical Services, Inc. (Cincinnati, Ohio, United States). The remaining author declares that the research was conducted in the absence of any commercial or financial relationships that could be construed as a potential conflict of interest.
Publisher’s Note
All claims expressed in this article are solely those of the authors and do not necessarily represent those of their affiliated organizations, or those of the publisher, the editors and the reviewers. Any product that may be evaluated in this article, or claim that may be made by its manufacturer, is not guaranteed or endorsed by the publisher.
Acknowledgments
The Safe and Sustainable Water Resources (SSWR) Program’s Pathogen Detection Project (SSWR 2.2B), Distribution System Indicators and Plumbing Water Project (SSWR 6.01C), and Water Treatment and Infrastructure Project (SSWR 7.2.1) (Office of Research and Development, United States Environmental Protection Agency or US EPA) supported this work. The Office of Research and Development (US EPA) reviewed this work and approved its publication.
References
Abberton, C. L., Bereschenko, L., van der Wielen, P. W., and Smith, C. J. (2016). Survival, biofilm formation, and growth potential of environmental and enteric Escherichia coli strains in drinking water microcosms. Appl. Environ. Microbiol. 82 (17), 5320–5331. doi:10.1128/aem.01569-16
Abdel-Nour, M., Duncan, C., Low, D., and Guyard, C. (2013). Biofilms: The stronghold of Legionella pneumophila. Int. J. Mol. Sci. 14 (11), 21660–21675. doi:10.3390/ijms141121660
Akin, E. W., Hoff, J. C., and Lippy, E. C. (1982). Waterborne outbreak control: which disinfectant? Environ. Health Perspect. 46, 7–12. doi:10.1289/ehp.82467
Alexander, T.Y., Kamali, A., and Vugia, D.J. (2019). Legionella epidemiologic and environmental risks. Curr. Epidemiol. Rep. 6 (3), 310–320. doi:10.1007/s40471-019-00207-3
Alleron, L., Khemiri, A., Koubar, M., Lacombe, C., Coquet, L., Cosette, P., Jouenne, T., and Frere, J. (2013). VBNC Legionella pneumophila cells are still able to produce virulence proteins. Water Res. 47 (17), 6606–6617. doi:10.1016/j.watres.2013.08.032
Alleron, L., Merlet, N., Lacombe, C., and Frère, J. (2008). Long-term survival of Legionella pneumophila in the viable but nonculturable state after monochloramine treatment. Curr. Microbiol. 57 (5), 497–502. doi:10.1007/s00284-008-9275-9
Amemura-Maekawa, J., Kura, F., Chida, K., Ohya, H., Kanatani, J. I., Isobe, J., Tanaka, S., Nakajima, H., Hiratsuka, T., Yoshino, S., Sakata, M., Murai, M., and Ohnishi, M. (2018). Legionella pneumophila and other Legionella species isolated from legionellosis patients in Japan between 2008 and 2016. Appl. Environ. Microbiol. 84 (18), e00721–18. doi:10.1128/AEM.00721-18
Ashbolt, N. J. (2015a). Environmental (saprozoic) pathogens of engineered water systems: understanding their Ecology for Risk Assessment and Management. Pathogens 4 (2), 390–405. doi:10.3390/pathogens4020390
Ashbolt, N. J. (2015b). Microbial contamination of drinking water and human health from community water systems. Curr. Environ. Health Rep. 2 (1), 95–106. doi:10.1007/s40572-014-0037-5
Ashbolt, N.J., Grabow, W.O.K., and Snozzi, M. (2001). “Indicators of microbial water quality.” in Water Quality: Guidelines, Standards and Health: Assessment of Risk and Risk Management for Water-Related Infectious Disease. Editors L. Fewtrell, and J. Bartram (London, United Kingdom, IWA Publishing), pp. 289–316.
Aw, T. G., and Rose, J. B. (2012). Detection of pathogens in water: from phylochips to qPCR to pyrosequencing. Curr. Opin. Biotechnol. 23 (3), 422–430. doi:10.1016/j.copbio.2011.11.016
Bargellini, A., Marchesi, I., Righi, E., Ferrari, A., Cencetti, S., Borella, P., and Rovesti, S. (2011). Parameters predictive of Legionella contamination in hot water systems: Association with trace elements and heterotrophic plate counts. Water Res. 45 (6), 2315–2321. doi:10.1016/j.watres.2011.01.009
Baron, J., Morris, L., and Stout, J. (2019). “Control of Legionella in hospital potable water systems.” in Decontamination in Hospitals and Healthcare. 2nd Edn, Editor J. T. Walker (United Kingdom: Elsevier Ltd.) (Woodhead Publishing), pp. 71–100.
Baron, J. L., Harris, J. K., Holinger, E. P., Duda, S., Stevens, M. J., Robertson, C. E., Ross, K. A., Pace, N. R., and Stout, J. E. (2015). Effect of monochloramine treatment on the microbial ecology of Legionella and associated bacterial populations in a hospital hot water system. Syst. Appl. Microbiol. 38 (3), 198–205. doi:10.1016/j.syapm.2015.02.006
Baron, J. L., Vikram, A., Duda, S., Stout, J. E., and Bibby, K. (2014). Shift in the microbial ecology of a hospital hot water system following the introduction of an on-site monochloramine disinfection system. PLOS One 9 (7), e102679. doi:10.1371/journal.pone.0102679
Batté, M., Appenzeller, B., Grandjean, D., Fass, S., Gauthier, V., Jorand, F., Mathieu, L., Boualam, M., Saby, S., and Block, J. (2003). Biofilms in drinking water distribution systems. Rev. Environ. Sci. Biotechnol. 2 (2-4), 147–168. doi:10.1023/b:resb.0000040456.71537.29
Baume, M., Cariou, A., Leveau, A., Fessy, N., Pastori, F., Jarraud, S., and Pierre, S. (2019). Quantification of Legionella DNA certified reference material by digital droplet PCR. J. Microbiol. Methods 157, 50–53. doi:10.1016/j.mimet.2018.12.019
Beauté, J. (2017). Legionnaires’ disease in Europe, 2011 to 2015. Eurosurveillance 22 (27), 30566. doi:10.2807/1560-7917.es.2017.22.27.30566
Bédard, E., Paranjape, K., Lalancette, C., Villion, M., Quach, C., Laferrière, C., Faucher, S. P., and Prévost, M. (2019). Legionella pneumophila levels and sequence-type distribution in hospital hot water samples from faucets to connecting pipes. Water Res. 156, 277–286. doi:10.1016/j.watres.2019.03.019
Beer, K. D., Gargano, J. W., Roberts, V. A., Hill, V. R., Garrison, L. E., Kutty, P. K., Hilborn, E. D., Wade, T. J., Fullerton, K. E., and Yoder, J. S. (2015). Surveillance for waterborne disease outbreaks associated with drinking water—United States, 2011-2012. Morb. Mortal. Wkly. Rep. 64 (31), 842–848. doi:10.15585/mmwr.mm6431a2
Bej, A., Mahbubani, M., and Atlas, R. (1991). Detection of viable Legionella pneumophila in water by polymerase chain reaction and gene probe methods. Appl. Environ. Microbiol. 57 (2), 597–600. doi:10.1128/aem.57.2.597-600.1991
Benedict, K. M., Reses, H., Vigar, M., Roth, D. M., Roberts, V. A., Mattioli, M., Cooley, L. A., Hilborn, E. D., Wade, T. J., and Fullerton, K. E. (2017). Surveillance for waterborne disease outbreaks associated with drinking water—United States, 2013-2014. Morb. Mortal. Wkly. Rep. 66 (44), 1216–1221. doi:10.15585/mmwr.mm6644a3
Berjeaud, J.-M., Chevalier, S., Schlusselhuber, M., Portier, E., Loiseau, C., Aucher, W., Lesouhaitier, O., and Verdon, J. (2016). Legionella pneumophila: the paradox of a highly sensitive opportunistic waterborne pathogen able to persist in the environment. Front. Microbiol. 7, 486. doi:10.3389/fmicb.2016.00486
Blatt, S. P., Parkinson, M. D., Pace, E., Hoffman, P., Dolan, D., Lauderdale, P., Zajac, R. A., and Melcher, G. P. (1993). Nosocomial Legionnaires’ disease: aspiration as a primary mode of disease acquisition. Am. J. Med. 95 (1), 16–22. doi:10.1016/0002-9343(93)90227-g
Block, J. C. (1992). “Biofilms in drinking water distribution systems.” in Biofilms—Science and Technology. Editors L.F. Melo, T. R. Bott, M. Fletcher, and B. Capdeville (Dordrecht, the Netherlands: Springer-Science+Business Media, B.V.; Kluwer Academic Publishers), pp. 469–485. doi:10.1007/978-94-011-1824-8_42
Bollin, G., Plouffe, J., Para, M. F., and Hackman, B. (1985). Aerosols containing Legionella pneumophila generated by shower heads and hot-water faucets. Appl. Environ. Microbiol. 50 (5), 1128–1131. doi:10.1128/aem.50.5.1128-1131.1985
Bonetta, S., Pignata, C., Bonetta, S., Meucci, L., Giacosa, D., Marino, E., Gilli, G., and Carraro, E. (2017). Viability of Legionella pneumophila in water samples: A comparison of propidium monoazide (PMA) treatment on membrane filters and in liquid. Int. J. Environ. Res. Public. Health 14 (5), 467. doi:10.3390/ijerph14050467
Bonetta, S., Pignata, C., Bonetta, S., Meucci, L., Giacosa, D., Marino, E., Gorrasi, I., Gilli, G., and Carraro, E. (2018). Effectiveness of a neutral electrolysed oxidising water (NEOW) device in reducing Legionella pneumophila in a water distribution system: A comparison between culture, qPCR and PMA-qPCR detection methods. Chemosphere 210, 550–556. doi:10.1016/j.chemosphere.2018.07.053
Borella, P., Guerrieri, E., Marchesi, I., Bondi, M., and Messi, P. (2005a). Water ecology of Legionella and protozoan: environmental and public health perspectives. Biotechnol. Annu. Rev. 11, 355–380. doi:10.1016/s1387-2656(05)11011-4
Borella, P., Montagna, M. T., Romano-Spica, V., Stampi, S., Stancanelli, G., Triassi, M., Neglia, R., Marchesi, I., Fantuzzi, G., and Tatò, D. (2004). Legionella infection risk from domestic hot water. Emerg. Infect. Dis. 10 (3), 457. doi:10.3201/eid1003.020707
Borella, P., Montagna, M. T., Stampi, S., Stancanelli, G., Romano-Spica, V., Triassi, M., Marchesi, I., Bargellini, A., Tatò, D., and Napoli, C. (2005b). Legionella contamination in hot water of Italian hotels. Appl. Environ. Microbiol. 71 (10), 5805–5813. doi:10.1128/aem.71.10.5805-5813.2005
Boss, R., Baumgartner, A., Kroos, S., Blattner, M., Fretz, R., and Moor, D. (2018). Rapid detection of viable Legionella pneumophilain tap water by a qPCR and RT-PCR-based method. J. Appl. Microbiol. 125 (4), 1216–1225. doi:10.1111/jam.13932
Botzenhart, K., Tarcson, G., and Ostruschka, M. (1993). Inactivation of bacteria and coliphages by ozone and chlorine dioxide in a continuous flow reactor. Water Sci. Technol. 27 (3-4), 363–370. doi:10.2166/wst.1993.0376
Boyle, D. P., Zembower, T. R., Reddy, S., and Qi, C. (2015). Comparison of clinical features, virulence, and relapse among Mycobacterium avium complex species. Am. J. Respir. Crit. Care Med. 191 (11), 1310–1317. doi:10.1164/rccm.201501-0067oc
Brady, M. T. (1989). Nosocomial Legionnaires disease in a children’s hospital. J. Pediatr. 115 (1), 46–50. doi:10.1016/s0022-3476(89)80327-0
Bridier, A., Briandet, R., Thomas, V., and Dubois-Brissonnet, F. (2011). Resistance of bacterial biofilms to disinfectants: A review. Biofouling 27 (9), 1017–1032. doi:10.1080/08927014.2011.626899
Brunkard, J. M., Ailes, E., Roberts, V. A., Hill, V., Hilborn, E. D., Craun, G. F., Rajasingham, A., Kahler, A., Garrison, L., and Hicks, L. (2011). Surveillance for waterborne disease outbreaks associated with drinking water—United States, 2007–2008. MMWR Morb. Mortal. Wkly. Rep. 60 (SS12), 38–68.
Buse, H., and Ashbolt, N. (2011). Differential growth of Legionella pneumophila strains within a range of amoebae at various temperatures associated with in-premise plumbing. Lett. Appl. Microbiol. 53 (2), 217–224. doi:10.1111/j.1472-765x.2011.03094.x
Buse, H. Y., Schoen, M. E., and Ashbolt, N. J. (2012). Legionellae in engineered systems and use of quantitative microbial risk assessment to predict exposure. Water Res. 46 (4), 921–933. doi:10.1016/j.watres.2011.12.022
Campos, C., Loret, J., Cooper, A., and Kelly, R. (2003). Disinfection of domestic water systems for Legionella pneumophila. J. Water Supply: Res. Technol.-AQUA 52 (5), 341–354. doi:10.2166/aqua.2003.0032
Cargill, K. L., Pyle, B. H., Sauer, R. L., and McFeters, G. A. (1992). Effects of culture conditions and biofilm formation on the iodine susceptibility of Legionella pneumophila. Can. J. Microbiol. 38 (5), 423–429. doi:10.1139/m92-071
Casini, B., Baggiani, A., Totaro, M., Mansi, A., Costa, A. L., Aquino, F., Miccoli, M., Valentini, P., Bruschi, F., and Lopalco, P. L. (2018). Detection of viable but non-culturable Legionella in hospital water network following monochloramine disinfection. J. Hospital Infect. 98 (1), 46–52. doi:10.1016/j.jhin.2017.09.006
CDC (2021). Legionella (Legionnaires' disease and pontiac fever): Causes, how it spreads, and people at increased risk. Available at: https://www.cdc.gov/legionella/about/causes-transmission.html (Accessed August 2021).
Cervero-Aragó, S., Rodríguez-Martínez, S., Puertas-Bennasar, A., and Araujo, R. M. (2015). Effect of common drinking water disinfectants, chlorine and heat, on free Legionella and amoebae-associated Legionella. PLOS One 10 (8), e0134726. doi:10.1371/journal.pone.0134726
Chen, S., Li, X., Wang, Y., Zeng, J., Ye, C., Li, X., Guo, L., Zhang, S., and Yu, X. (2018). Induction of Escherichia coli into a VBNC state through chlorination/chloramination and differences in characteristics of the bacterium between states. Water Res. 142, 279–288. doi:10.1016/j.watres.2018.05.055
Chen, X., and Stewart, P. S. (1996). Chlorine penetration into artificial biofilm is limited by a reaction−diffusion interaction. Environ. Sci. Technol. 30 (6), 2078–2083. doi:10.1021/es9509184
Chiao, T.-H., Clancy, T. M., Pinto, A., Xi, C., and Raskin, L. (2014). Differential resistance of drinking water bacterial populations to monochloramine disinfection. Environ. Sci. Technol. 48 (7), 4038–4047. doi:10.1021/es4055725
Codony, F., Dinh-Thanh, M., and Agustí, G. (2020). Key factors for removing bias in viability PCR-based methods: a review. Curr. Microbiol. 77 (4), 682–687. doi:10.1007/s00284-019-01829-y
Cohen, J., and Shuval, H. I. (1973). Coliforms, fecal coliforms, and fecal streptococci as indicators of water pollution. Water Air Soil Pollut. 2 (1), 85–95. doi:10.1007/bf00572392
Collier, S. A., Stockman, L. J., Hicks, L. A., Garrison, L. E., Zhou, F. J., and Beach, M. J. (2012). Direct healthcare costs of selected diseases primarily or partially transmitted by water. Epidemiol. Infect. 140 (11), 2003–2013. doi:10.1017/s0950268811002858
Collins, S., Stevenson, D., Bennett, A., and Walker, J. (2017). Occurrence of Legionella in UK household showers. Int. J. Hyg. Environ. Health 220 (2), 401–406. doi:10.1016/j.ijheh.2016.12.001
Cooper, I., and Hanlon, G. (2010). Resistance of Legionella pneumophila serotype 1 biofilms to chlorine-based disinfection. J. Hosp. Infect. 74 (2), 152–159. doi:10.1016/j.jhin.2009.07.005
Cordes, L. G., Wiesenthal, A. M., Gorman, G. W., Phair, J. P., Sommers, H. M., Brown, A., Yu, V. L., Magnussen, M. H., Meyer, R. D., and Wolf, J. S. (1981). Isolation of Legionella pneumophila from hospital shower heads. Ann. Intern. Med. 94 (2), 195–197. doi:10.7326/0003-4819-94-2-195
Correia, A. M., Ferreira, J. S., Borges, V., Nunes, A., Gomes, B., Capucho, R., Gonçalves, J., Antunes, D. M., Almeida, S., and Mendes, A. (2016). Probable person-to-person transmission of Legionnaires’ disease. N. Engl. J. Med. 374 (5), 497–498. doi:10.1056/nejmc1505356
Cullom, A. C., Martin, R. L., Song, Y., Williams, K., Williams, A., Pruden, A., and Edwards, M. A. (2020). Critical review: Propensity of premise plumbing pipe materials to enhance or diminish growth of Legionella and other opportunistic pathogens. Pathogens 9 (11), 957. doi:10.3390/pathogens9110957
Cunliffe, D. A. (1990). Inactivation of Legionella pneumophila by monochloramine. J. Appl. Bacteriol. 68 (5), 453–459. doi:10.1111/j.1365-2672.1990.tb02896.x
Dai, D., Rhoads, W. J., Katner, A., Strom, L., Edwards, M. A., Pruden, A., and Pieper, K. J. (2019). Molecular survey of Legionella and Naegleria fowleriin private well water and premise plumbing following the 2016 Louisiana flood. Environ. Sci.: Water Res. Technol. 5 (8), 1464–1477. doi:10.1039/c9ew00109c
De Beer, D., Srinivasan, R., and Stewart, P. S. (1994). Direct measurement of chlorine penetration into biofilms during disinfection. Appl. Environ. Microbiol. 60 (12), 4339–4344. doi:10.1128/aem.60.12.4339-4344.1994
De Jonckheere, J., and Van de Voorde, H. (1976). Differences in destruction of cysts of pathogenic and nonpathogenic Naegleria and Acanthamoeba by chlorine. Appl. Environ. Microbiol. 31 (2), 294–297. doi:10.1128/aem.31.2.294-297.1976
Declerck, P., Behets, J., Margineanu, A., van Hoef, V., De Keersmaecker, B., and Ollevier, F. (2009). Replication of Legionella pneumophila in biofilms of water distribution pipes. Microbiol. Res. 164 (6), 593–603. doi:10.1016/j.micres.2007.06.001
Declerck, P., Behets, J., van Hoef, V., and Ollevier, F. (2007). Detection of Legionella spp. and some of their amoeba hosts in floating biofilms from anthropogenic and natural aquatic environments. Water Res. 41 (14), 3159–3167. doi:10.1016/j.watres.2007.04.011
Delaedt, Y., Daneels, A., Declerck, P., Behets, J., Ryckeboer, J., Peters, E., and Ollevier, F. (2008). The impact of electrochemical disinfection on Escherichia coli and Legionella pneumophila in tap water. Microbiol. Res. 163 (2), 192–199. doi:10.1016/j.micres.2006.05.002
Delafont, V., Mougari, F., Cambau, E., Joyeux, M., Bouchon, D., Héchard, Y., and Moulin, L. (2014). First evidence of amoebae-mycobacteria association in drinking water network. Environ. Sci. Technol. 48 (20), 11872–11882. doi:10.1021/es5036255
Delafont, V., Rodier, M.-H., Maisonneuve, E., and Cateau, E. (2018). Vermamoeba vermiformis: A free-living amoeba of interest. Microb. Ecol. 76 (4), 991–1001. doi:10.1007/s00248-018-1199-8
Demirjian, A., Lucas, C. E., Garrison, L. E., Kozak-Muiznieks, N. A., States, S., Brown, E. W., Wortham, J. M., Beaudoin, A., Casey, M. L., and Marriott, C. (2015). The importance of clinical surveillance in detecting Legionnaires’ disease outbreaks: A large outbreak in a hospital with a Legionella disinfection system-Pennsylvania, 2011-2012. Clin. Infect. Dis. 60 (11), 1596–1602. doi:10.1093/cid/civ153
Dietersdorfer, E., Kirschner, A., Schrammel, B., Ohradanova-Repic, A., Stockinger, H., Sommer, R., Walochnik, J., and Cervero-Aragó, S. (2018). Starved viable but non-culturable (VBNC) Legionella strains can infect and replicate in amoebae and human macrophages. Water Res. 141, 428–438. doi:10.1016/j.watres.2018.01.058
Ditommaso, S., Ricciardi, E., Giacomuzzi, M., Arauco Rivera, S. R., and Zotti, C. M. (2015). Legionella in water samples: How can you interpret the results obtained by quantitative PCR? Mol. Cell. Probes 29 (1), 7–12. doi:10.1016/j.mcp.2014.09.002
Donlan, R., Forster, T., Murga, R., Brown, E., Lucas, C., Carpenter, J., and Fields, B. (2005). Legionella pneumophila associated with the protozoan Hartmannella vermiformisin a model multi-species biofilm has reduced susceptibility to disinfectants. Biofouling 21 (1), 1–7. doi:10.1080/08927010500044286
Donlan, R., Murga, R., Carpenter, J., Brown, E., Besser, R., and Fields, B. (2001). “Monochloramine disinfection of biofilm-associated Legionella pneumophila in a potable water model system.” in Legionella. Editors R. Marre, Y. Abu Kwaik, C. Bartlett, N. P. Cianciotto, B. S. Fields, M. Frosch, J. Hacker, and P.C . Lück (Washington, D.C., USA; ASM Press), pp. 406–410.
Donlan, R. M., and Costerton, J. W. (2002). Biofilms: Survival mechanisms of clinically relevant microorganisms. Clin. Microbiol. Rev. 15 (2), 167–193. doi:10.1128/cmr.15.2.167-193.2002
Donohue, M. J., King, D., Pfaller, S., and Mistry, J. H. (2019). The sporadic nature of Legionella pneumophila, Legionella pneumophila Sg1 and Mycobacterium avium occurrence within residences and office buildings across 36 states in the United States. J. Appl. Microbiol. 126 (5), 1568–1579. doi:10.1111/jam.14196
Douterelo, I., Sharpe, R. L., Husband, S., Fish, K. E., and Boxall, J. B. (2019). Understanding microbial ecology to improve management of drinking water distribution systems. Wiley Interdiscip. Rev. Water 6 (1), e01325. doi:10.1002/wat2.1325
Duda, S., Baron, J. L., Wagener, M. M., Vidic, R. D., and Stout, J. E. (2015). Lack of correlation between Legionella colonization and microbial population quantification using heterotrophic plate count and adenosine triphosphate bioluminescence measurement. Environ. Monit. Assess 187 (7), 393. doi:10.1007/s10661-015-4612-5
Duda, S., Kandiah, S., Stout, J. E., Baron, J. L., Yassin, M., Fabrizio, M., Ferrelli, J., Hariri, R., Wagener, M. M., and Goepfert, J. (2014). Evaluation of a new monochloramine generation system for controlling Legionella in building hot water systems. Infect. Control Hosp. Epidemiol. 35 (11), 1356–1363. doi:10.1086/678418
Dupuy, M., Berne, F., Herbelin, P., Binet, M., Berthelot, N., Rodier, M. H., Soreau, S., and Héchard, Y. (2014). Sensitivity of free-living amoeba trophozoites and cysts to water disinfectants. Int. J. Hyg. Environ. Health 217 (2-3), 335–339. doi:10.1016/j.ijheh.2013.07.007
Dupuy, M., Mazoua, S., Berne, F., Bodet, C., Garrec, N., Herbelin, P., Ménard-Szczebara, F., Oberti, S., Rodier, M.-H., and Soreau, S. (2011). Efficiency of water disinfectants against Legionella pneumophila and Acanthamoeba. Water Res. 45 (3), 1087–1094. doi:10.1016/j.watres.2010.10.025
Dusserre, E., Ginevra, C., Hallier-Soulier, S., Vandenesch, F., Festoc, G., Etienne, J., Jarraud, S., and Molmeret, M. (2008). A PCR-based method for monitoring Legionella pneumophila in water samples detects viable but noncultivable legionellae that can recover their cultivability. Appl. Environ. Microbiol. 74 (15), 4817–4824. doi:10.1128/aem.02899-07
Edberg, S., Rice, E., Karlin, R., and Allen, M. (2000). Escherichia coli: the best biological drinking water indicator for public health protection. J. Appl. Microbiol. 88 (S1), 106S–116S. doi:10.1111/j.1365-2672.2000.tb05338.x
El-Chakhtoura, J., Saikaly, P. E., van Loosdrecht, M., and Vrouwenvelder, J. S. (2018). Impact of distribution and network flushing on the drinking water microbiome. Front. Microbiol. 9, 2205. doi:10.3389/fmicb.2018.02205
Ercken, D., Verelst, L., Declerck, P., Duvivier, L., Van Damme, A., and Ollevier, F. (2003). Effects of peracetic acid and monochloramine on the inactivation of Naegleria lovaniensis. Water Sci. Technol. 47 (3), 167–171. doi:10.2166/wst.2003.0190
Ezenarro, J. J., Párraga-Niño, N., Sabrià, M., Del Campo, F. J., Muñoz-Pascual, F.-X., Mas, J., and Uria, N. (2020). Rapid detection of Legionella pneumophila in drinking water, based on filter immunoassay and chronoamperometric measurement. Biosensors 10 (9), 102. doi:10.3390/bios10090102
Falkinham, J. O. (2020). Living with Legionella and other waterborne pathogens. Microorganisms 8 (12), 2026. doi:10.3390/microorganisms8122026
Falkinham, J. (2015). Common features of opportunistic premise plumbing pathogens. Int. J. Environ. Res. Public. Health 12 (5), 4533–4545. doi:10.3390/ijerph120504533
Falkinham, J. O., Norton, C. D., and LeChevallier, M. W. (2001). Factors influencing numbers of Mycobacterium avium, Mycobacterium intracellulare, and other mycobacteria in drinking water distribution systems. Appl. Environ. Microbiol. 67 (3), 1225–1231. doi:10.1128/aem.67.3.1225-1231.2001
Falkinham, J. O., Hilborn, E. D., Arduino, M. J., Pruden, A., and Edwards, M. A. (2015a). Epidemiology and ecology of opportunistic premise plumbing pathogens: Legionella pneumophila, Mycobacterium avium, and Pseudomonas aeruginosa. Environ. Health Perspect. 123 (8), 749–758. doi:10.1289/ehp.1408692
Falkinham, J., Pruden, A., and Edwards, M. (2015b). Opportunistic premise plumbing pathogens: Increasingly important pathogens in drinking water. Pathogens 4 (2), 373–386. doi:10.3390/pathogens4020373
Felfoldi, T., Heéger, Z., Vargha, M., and Márialigeti, K. (2010). Detection of potentially pathogenic bacteria in the drinking water distribution system of a hospital in Hungary. Clin. Microbiol. Infect. 16 (1), 89–92. doi:10.1111/j.1469-0691.2009.02795.x
Fields, B. S. (1996). The molecular ecology of legionellae. Trends Microbiol. 4 (7), 286–290. doi:10.1016/0966-842x(96)10041-x
Fields, B.S. (2007). “Legionellae and Legionnaires’ disease.” in Manual of Environmental Microbiology. 3rd edn. Editors C. J. Hurst, R. L. Crawford, J. L. Garland, D. A. Lipson, A. L. Mills, and L. D. Stetzenbach (Washington, D.C., USA: ASM Press), pp. 1005–1015.
Fields, B. S., Benson, R. F., and Besser, R. E. (2002). Legionella and Legionnaires’ disease: 25 years of investigation. Clin. Microbiol. Rev. 15 (3), 506–526. doi:10.1128/cmr.15.3.506-526.2002
Fleischmann, S., Robben, C., Alter, T., Rossmanith, P., and Mester, P. (2021). How to evaluate non-growing cells-current strategies for determining antimicrobial resistance of VBNC bacteria. Antibiotics 10 (2), 115. doi:10.3390/antibiotics10020115
Flemming, H.-C., Percival, S., and Walker, J. (2002). Contamination potential of biofilms in water distribution systems. Water Sci. Technol.: Water Supply 2 (1), 271–280. doi:10.2166/ws.2002.0032
Ford, T. E. (1999). Microbiological safety of drinking water: United States and global perspectives. Environ. Health Perspect. 107 (Suppl. 1), 191–206. doi:10.2307/3434483
Gagnon, G. A., O’Leary, K. C., Volk, C. J., Chauret, C., Stover, L., and Andrews, R. C. (2004). Comparative analysis of chlorine dioxide, free chlorine and chloramines on bacterial water quality in model distribution systems. J. Environ. Eng. 130 (11), 1269–1279. doi:10.1061/(asce)0733-9372(2004)130:11(1269)
Gall, A. M., Mariñas, B. J., Lu, Y., and Shisler, J. L. (2015) Waterborne viruses: A barrier to safe drinking water. PLoS Pathog. 11 (6), e1004867. doi:10.1371/journal.ppat.1004867
Gerba, C. P. (2015). “Indicator microorganisms.” in Environmental Microbiology, 3rd Edn. Editors I. L. Pepper, C. P. Gerba, and T. J. Gentry (San Diego, California, USA: Elsevier Inc. (Academic Press)), pp. 551–564.
Gerba, C. P., and Pepper, I. L. (2015). “Drinking water treatment and distribution.” in Environmental Microbiology, 3rd Edn. Editors I. L. Pepper, C. P. Gerba, and T. J. Gentry (San Diego, California, USA: Elsevier Inc. (Academic Press)), pp. 633–643.
Gião, M., Wilks, S., Azevedo, N., Vieira, M., and Keevil, C. (2009). Incorporation of natural uncultivable Legionella pneumophila into potable water biofilms provides a protective niche against chlorination stress. Biofouling 25 (4), 345–351. doi:10.1080/08927010902803305
Gillespie, S., Lipphaus, P., Green, J., Parsons, S., Weir, P., Juskowiak, K., Jefferson, B., Jarvis, P., and Nocker, A. (2014). Assessing microbiological water quality in drinking water distribution systems with disinfectant residual using flow cytometry. Water Res. 65, 224–234. doi:10.1016/j.watres.2014.07.029
Girones, R., Ferrús, M. A., Alonso, J. L., Rodriguez-Manzano, J., Calgua, B., de Abreu Corrêa, A., Hundesa, A., Carratala, A., and Bofill-Mas, S. (2010). Molecular detection of pathogens in water–The pros and cons of molecular techniques. Water Res. 44 (15), 4325–4339. doi:10.1016/j.watres.2010.06.030
Golpayegani, A., Douraghi, M., Rezaei, F., Alimohammadi, M., and Nodehi, R. N. (2019). Propidium monoazide-quantitative polymerase chain reaction (PMA-qPCR) assay for rapid detection of viable and viable but non-culturable (VBNC) Pseudomonas aeruginosa in swimming pools. J. Environ. Health Sci. Eng. 17 (1), 407–416. doi:10.1007/s40201-019-00359-w
Gomes, T. S., Gjiknuri, J., Magnet, A., Vaccaro, L., Ollero, D., Izquierdo, F., Fenoy, S., Hurtado, C., and del Águila, C. (2018). The influence of Acanthamoeba-Legionella interaction in the virulence of two different Legionella species. Front. Microbiol. 9, 2962. doi:10.3389/fmicb.2018.02962
Grobe, K. J., Zahller, J., and Stewart, P. S. (2002). Role of dose concentration in biocide efficacy against Pseudomonas aeruginosa biofilms. J. Indust. Microbiol. Biotechnol. 29 (1), 10–15. doi:10.1038/sj.jim.7000256
Guo, L., Wan, K., Zhu, J., Ye, C., Chabi, K., and Yu, X. (2021). Detection and distribution of vbnc/viable pathogenic bacteria in full-scale drinking water treatment plants. J. Hazard. Mater. 406, 124335. doi:10.1016/j.jhazmat.2020.124335
Haas, C. N. (1999). Benefits of using a disinfectant residual. J. Am. Water Works Assoc. 91 (1), 65–69. doi:10.1002/j.1551-8833.1999.tb08569.x
Hamilton, K., Prussin, A., Ahmed, W., and Haas, C. (2018). Outbreaks of Legionnaires' disease and pontiac fever 2006-2017. Curr. Environ. Health Rep. 5 (2), 263–271. doi:10.1007/s40572-018-0201-4
Hamilton, K. A., Hamilton, M. T., Johnson, W., Jjemba, P., Bukhari, Z., LeChevallier, M., Haas, C. N., and Gurian, P. (2019). Risk-based critical concentrations of Legionella pneumophila for indoor residential water uses. Environ. Sci. Technol. 53 (8), 4528–4541. doi:10.1021/acs.est.8b03000
Hayes-Phillips, D., Bentham, R., Ross, K., and Whiley, H. (2019). Factors influencing Legionella contamination of domestic household showers. Pathogens 8 (1), 27. doi:10.3390/pathogens8010027
Heffelfinger, J. D., Kool, J. L., Fridkin, S., Fraser, V. J., Hageman, J., Carpenter, J., Whitney, C. G., and Society for Healthcare Epidemiology of America, (2003). Risk of hospital-acquired Legionnaires' disease in cities using monochloramine versus other water disinfectants. Infect. Control Hosp. Epidemiol. 24 (8), 569–574. doi:10.1086/502256
Hilborn, E. D., Wade, T. J., Hicks, L., Garrison, L., Carpenter, J., Adam, E., et al. (2013). Surveillance for waterborne disease outbreaks associated with drinking water and other nonrecreational water - United States, 2009-2010. MMWR Morb. Mortal. Wkly. Rep. 62 (35), 714–720.
Hines, S. A., Chappie, D. J., Lordo, R. A., Miller, B. D., Janke, R. J., Lindquist, H. A., Fox, K. R., Ernst, H. S., and Taft, S. C. (2014). Assessment of relative potential for Legionella species or surrogates inhalation exposure from common water uses. Water Res. 56, 203–213. doi:10.1016/j.watres.2014.02.013
Hornei, B., Ewig, S., Exner, M., Tartakovsky, I., Lajoie, L., Dangendorf, F., Surman-Lee, S., and Fields, B. (2007). “Legionellosis.” in Legionella and the Prevention of Legionellosis. Editors J. Bartram, Y. Chartier, J. V. Lee, K. Pond, and S. Surman-Lee (Geneva, Switzerland: World Health Organization), pp. 1–28.
Huang, C. K., Weerasekara, A., Bond, P. L., Weynberg, K. D., and Guo, J. (2021). Characterizing the premise plumbing microbiome in both water and biofilms of a 50-year-old building. Sci. Total Environ. 798, 149225. doi:10.1016/j.scitotenv.2021.149225
Huang, S. W., Hsu, B. M., Huang, C. C., and Chen, J. S. (2011). Utilization of polymerase chain reaction and selective media cultivation to identify Legionella in Taiwan spring water samples. Environ. Monit. Assess. 174 (1-4), 427–37. doi:10.1007/s10661-010-1467-7
Hwang, C., Ling, F., Andersen, G. L., LeChevallier, M. W., and Liu, W.-T. (2012). Microbial community dynamics of an urban drinking water distribution system subjected to phases of chloramination and chlorination treatments. Appl. Environ. Microbiol. 78 (22), 7856–7865. doi:10.1128/aem.01892-12
Hwang, M. G., Katayama, H., and Ohgaki, S. (2006). Effect of intracellular resuscitation of Legionella pneumophila in Acanthamoeba polyphage cells on the antimicrobial properties of silver and copper. Environ. Sci. Technol. 40 (23), 7434–7439. doi:10.1021/es060412t
Isaac, T.S., and Sherchan, S.P. (2019). Molecular detection of opportunistic premise plumbing pathogens in rural Louisiana's drinking water distribution system. Environ. Res. 181, 108847. doi:10.1016/j.envres.2019.108847
Jakubek, D., Guillaume, C., Binet, M., Leblon, G., DuBow, M., and Le Brun, M. (2013). Susceptibility of Legionella strains to the chlorinated biocide, monochloramine. Microb. Environ. 28 (3), 336–345. doi:10.1264/jsme2.me12205
Jjemba, P. K., Weinrich, L. A., Cheng, W., Giraldo, E., and LeChevallier, M. W. (2010). Regrowth of potential opportunistic pathogens and algae in reclaimed-water distribution systems. Appl. Environ. Microbiol. 76 (13), 4169–4178. doi:10.1128/aem.03147-09
Kämpfer, P, Nienhüser, A, Packroff, G, Wernicke, F, Mehling, A, Nixdorf, K, Fiedler, S, Kolauch, C, and Esser, M (2008). Molecular identification of coliform bacteria isolated from drinking water reservoirs with traditional methods and the Colilert-18 system. Int. J. Hyg. Environ. Health 211 (3-4), 374–384. doi:10.1016/j.ijheh.2007.07.021
Karumathil, D., Yin, H.-B., Kollanoor-Johny, A., and Venkitanarayanan, K. (2014). Effect of chlorine exposure on the survival and antibiotic gene expression of multidrug resistant Acinetobacter baumannii in water. Int. J. Environ. Res. Public. Health 11 (2), 1844–1854. doi:10.3390/ijerph110201844
Katz, S. M., and Hammel, J. M. (1987). The effect of drying, heat, and pH on the survival of Legionella pneumophila. Ann. Clin. Lab. Sci. 17 (3), 150–156.
Kelsey, M. C. (2014). “Control of waterborne microorganisms and reducing the threat from Legionella and Pseudomonas.” in Decontamination in Hospitals and Healthcare. Editor J. T. Walker (Cambridge, United Kingdom: Elsevier B.V. (Woodhead Publishing Limited)), pp. 208–231.
Kilvington, S., and Price, J. (1990). Survival of Legionella pneumophila within cysts of Acanthamoeba polyphaga following chlorine exposure. J. Appl. Bacteriol. 68 (5), 519–525. doi:10.1111/j.1365-2672.1990.tb02904.x
Kim, B., Anderson, J., Mueller, S., Gaines, W., and Kendall, A. (2002). Literature review-efficacy of various disinfectants against Legionella in water systems. Water Res. 36 (18), 4433–4444. doi:10.1016/s0043-1354(02)00188-4
Kim, S.-Y., Shin, S. H., Moon, S. M., Yang, B., Kim, H., Kwon, O. J., Huh, H. J., Ki, C.-S., Lee, N. Y., and Shin, S. J. (2017). Distribution and clinical significance of Mycobacterium avium complex species isolated from respiratory specimens. Diagn. Microbiol. Infect. Dis. 88 (2), 125–137. doi:10.1016/j.diagmicrobio.2017.02.017
King, C. H., Shotts, E. B., Wooley, R. E., and Porter, K. G. (1988). Survival of coliforms and bacterial pathogens within protozoa during chlorination. Appl. Environ. Microbiol. 54 (12), 3023–3033. doi:10.1128/aem.54.12.3023-3033.1988
Kirschner, A. K. (2016). Determination of viable legionellae in engineered water systems: Do we find what we are looking for? Water Res. 93, 276–288. doi:10.1016/j.watres.2016.02.016
Kontchou, J. A., and Nocker, A. (2019). Optimization of viability qPCR for selective detection of membrane-intact Legionella pneumophila. J. Microbiol. Methods 156, 68–76. doi:10.1016/j.mimet.2018.12.003
Kool, J. L. (2001). “Control of Legionella in drinking water systems: Impact of monochloramine.” in Legionella. Editors R. Marre, Y. Abu Kwaik, C. Bartlett, N. P. Cianciotto, B. S. Fields, M. Frosch, J. Hacker, and P. C. Lück (Washington, D.C., USA: ASM Press), pp. 411–418.
Kool, J. L., Bergmire-Sweat, D., Butler, J. C., Brown, E. W., Peabody, D. J., Massi, D. S., Carpenter, J. C., Pruckler, J. M., Benson, R. F., and Fields, B. S. (1999a). Hospital characteristics associated with colonization of water systems by Legionella and risk of nosocomial Legionnaires' disease: A cohort study of 15 hospitals. Infect. Control Hosp. Epidemiol. 20 (12), 798–805. doi:10.1086/501587
Kool, J. L., Carpenter, J. C., and Fields, B. S. (1999b). Effect of monochloramine disinfection of municipal drinking water on risk of nosocomial Legionnaires’ disease. The Lancet 353 (9149), 272–277. doi:10.1016/s0140-6736(98)06394-6
Kool, J. L., Carpenter, J. C., and Fields, B. S. (2000). Monochloramine and Legionnaires’ disease. J. Am. Water Works Assoc. 92 (9), 88–96. doi:10.1002/j.1551-8833.2000.tb09007.x
Kouame, Y., and Haas, C. N. (1991). Inactivation of E. coli by combined action of free chlorine and monochloramine. Water Res. 25 (9), 1027–1032. doi:10.1016/0043-1354(91)90195-v
Kralik, P., Nocker, A., and Pavlik, I. (2010). Mycobacterium avium subsp. paratuberculosis viability determination using F57 quantitative PCR in combination with propidium monoazide treatment. Int. J. Food Microbiol. 141 (Supplement), S80–S86. doi:10.1016/j.ijfoodmicro.2010.03.018
Kusnetsov, J., Ottoila, E., and Martikainen, P. (1996). Growth, respiration and survival of Legionella pneumophila at high temperatures. J. Appl. Bacteriol. 81 (4), 341–347. doi:10.1111/j.1365-2672.1996.tb03517.x
Lau, H., and Ashbolt, N. (2009). The role of biofilms and protozoa in Legionella pathogenesis: Implications for drinking water. J. Appl. Microbiol. 107 (2), 368–378. doi:10.1111/j.1365-2672.2009.04208.x
LeChevallier, M. W. (1999). The case for maintaining a disinfectant residual. J. Am. Water Works Assoc. 91 (1), 86–94. doi:10.1002/j.1551-8833.1999.tb08573.x
LeChevallier, M. W. (2019). Occurrence of culturable Legionella pneumophila in drinking water distribution systems. AWWA Water Sci. 1 (3), e1139. doi:10.1002/aws2.1139
LeChevallier, M. W., Cawthon, C. D., and Lee, R. G. (1988). Inactivation of biofilm bacteria. Appl. Environ. Microbiol. 54 (10), 2492–2499. doi:10.1128/aem.54.10.2492-2499.1988
LeChevallier, M. W., Gullick, R. W., Karim, M. R., Friedman, M., and Funk, J. E. (2003). The potential for health risks from intrusion of contaminants into the distribution system from pressure transients. J. Water Health 1 (1), 3–14. doi:10.2166/wh.2003.0002
Lee, E. J., and Schwab, K. J. (2005). Deficiencies in drinking water distribution systems in developing countries. J Water Health 3 (2), 109–127. doi:10.2166/wh.2005.0012
Lee, J., Lai, S., Exner, M., Lenz, J., Gaia, V., Casati, S., Hartemann, P., Lück, C., Pangon, B., and Ricci, M. (2011a). An international trial of quantitative PCR for monitoring Legionella in artificial water systems. J. Appl. Microbiol. 110 (4), 1032–1044. doi:10.1111/j.1365-2672.2011.04957.x
Lee, W. H., Pressman, J. G., and Wahman, D. G. (2018). Three-dimensional free chlorine and monochloramine biofilm penetration: Correlating penetration with biofilm activity and viability. Environ. Sci. Technol. 52 (4), 1889–1898. doi:10.1021/acs.est.7b05215
Lee, W. H., Wahman, D. G., Bishop, P. L., and Pressman, J. G. (2011b). Free chlorine and monochloramine application to nitrifying biofilm: Comparison of biofilm penetration, activity, and viability. Environ. Sci. Technol. 45 (4), 1412–1419. doi:10.1021/es1035305
Lehtola, M. J., Torvinen, E., Kusnetsov, J., Pitkänen, T., Maunula, L., von Bonsdorff, C.-H., Martikainen, P. J., Wilks, S. A., Keevil, C. W., and Miettinen, I. T. (2007). Survival of Mycobacterium avium, Legionella pneumophila, Escherichia coli, and caliciviruses in drinking water-associated biofilms grown under high-shear turbulent flow. Appl. Environ. Microbiol. 73 (9), 2854–2859. doi:10.1128/aem.02916-06
Leoni, E., De Luca, G., Legnani, P., Sacchetti, R., Stampi, S., and Zanetti, F. (2005). Legionella waterline colonization: Detection of Legionella species in domestic, hotel and hospital hot water systems. J. Appl. Microbiol. 98 (2), 373–379. doi:10.1111/j.1365-2672.2004.02458.x
Leoni, E., Legnani, P., Sabattini, M.B., and Righi, F. (2001). Prevalence of Legionella spp. in swimming pool environment. Water Res. 35 (15), 3749–3753. doi:10.1016/s0043-1354(01)00075-6
Leslie, E., Hinds, J., and Hai, F. I. (2021). Causes, factors, and control measures of opportunistic premise plumbing pathogens-a critical review. Appl. Sci. 11 (10), 4474. doi:10.3390/app11104474
Lesnik, R., Brettar, I., and Höfle, M. G. (2016). Legionella species diversity and dynamics from surface reservoir to tap water: From cold adaptation to thermophily. ISME J. 10 (5), 1064–1080. doi:10.1038/ismej.2015.199
Li, B., and Chen, J.-Q. (2013). Development of a sensitive and specific qPCR assay in conjunction with propidium monoazide for enhanced detection of live Salmonella spp. in food. BMC Microbiol. 13, 273. doi:10.1186/1471-2180-13-273
Li, H., Xin, H., and Li, S. F. Y. (2015). Multiplex PMA-qPCR assay with internal amplification control for simultaneous detection of viable Legionella pneumophila, Salmonella typhimurium, and Staphylococcus aureus in environmental waters. Environ. Sci. Technol. 49 (24), 14249–14256. doi:10.1021/acs.est.5b03583
Lin, H., Ye, C., Chen, S., Zhang, S., and Yu, X. (2017). Viable but non-culturable E. coli induced by low level chlorination have higher persistence to antibiotics than their culturable counterparts. Environ. Pollut. 230, 242–249. doi:10.1016/j.envpol.2017.06.047
Lin, Y. E., Stout, J. E., and Victor, L. Y. (2011). Controlling Legionellain hospital drinking water: An evidence-based review of disinfection methods. Infect. Control Hosp. Epidemiol. 32 (2), 166–173. doi:10.1086/657934
Lin, Y. E., Vidic, R. D., Stout, J. E., McCartney, C. A., and Yu, V. L. (1998a). Inactivation of Mycobacterium avium by copper and silver ions. Water Res. 32 (7), 1997–2000. doi:10.1016/s0043-1354(97)00460-0
Lin, Y. E., Vidic, R. D., Stout, J. E., and Yu, V. L. (1998b). Legionella in water distribution systems. J. Am. Water Works Assoc. 90 (9), 112–122. doi:10.1002/j.1551-8833.1998.tb08503.x
Liu, G., Zhang, Y., Knibbe, W.-J., Feng, C., Liu, W., Medema, G., and van der Meer, W. (2017). Potential impacts of changing supply-water quality on drinking water distribution: A review. Water Res. 116, 135–148. doi:10.1016/j.watres.2017.03.031
Liu, R., Yu, Z., Guo, H., Liu, M., Zhang, H., and Yang, M. (2012). Pyrosequencing analysis of eukaryotic and bacterial communities in faucet biofilms. Sci. Total Environ. 435-436, 124–131. doi:10.1016/j.scitotenv.2012.07.022
Liu, Y., Wang, C., Tyrrell, G., Hrudey, S. E., and Li, X.F. (2009). Induction of Escherichia coli O157:H7 into the viable but non-culturable state by chloraminated water and river water, and subsequent resuscitation. Environ. Microbiol. Rep. 1 (2), 155–161. doi:10.1111/j.1758-2229.2009.00024.x
Liu, Z., Stout, J. E., Tedesco, L., Boldin, M., Hwang, C., Diven, W. F., and Yu, V. L. (1994). Controlled evaluation of copper-silver ionization in eradicating Legionella pneumophila from a hospital water distribution system. J. Infect. Dis. 169 (4), 919–922. doi:10.1093/infdis/169.4.919
Loret, J.-F., and Greub, G. (2010). Free-living amoebae: Biological by-passes in water treatment. Int. J. Hyg. Environ. Health 213 (3), 167–175. doi:10.1016/j.ijheh.2010.03.004
Loret, J., Robert, S., Thomas, V., Lévi, Y., Cooper, A., and McCoy, W. (2005). Comparison of disinfectants for biofilm, protozoa and Legionella control. J. Water Health 3 (4), 423–433. doi:10.2166/wh.2005.047
Lu, J., Buse, H., Struewing, I., Zhao, A., Lytle, D., and Ashbolt, N. (2017). Annual variations and effects of temperature on Legionella spp. and other potential opportunistic pathogens in a bathroom. Environ. Sci. Pollut. Res. 24 (3), 2326–2336. doi:10.1007/s11356-016-7921-5
Lu, J., Struewing, I., Vereen, E., Kirby, A., Levy, K., Moe, C., and Ashbolt, N. (2016). Molecular detection of Legionella spp. and their associations with Mycobacterium spp., Pseudomonas aeruginosa and amoeba hosts in a drinking water distribution system. J. Appl. Microbiol. 120 (2), 509–521. doi:10.1111/jam.12996
Lu, J., Struewing, I., Yelton, S., and Ashbolt, N. (2015). Molecular survey of occurrence and quantity of Legionella spp., Mycobacterium spp., Pseudomonas aeruginosa and amoeba hosts in municipal drinking water storage tank sediments. J. Appl. Microbiol. 119 (1), 278–288. doi:10.1111/jam.12831
Luo, J.-F., Lin, W.-T., and Guo, Y. (2010). Method to detect only viable cells in microbial ecology. Appl. Microbiol. Biotechnol. 86, 377–384. doi:10.1007/s00253-009-2373-1
Makris, K. C., Andra, S. S., and Botsaris, G. (2014). Pipe scales and biofilms in drinking-water distribution systems: undermining finished water quality. Crit. Rev. Environ. Sci. Technol. 44 (13), 1477–1523. doi:10.1080/10643389.2013.790746
Marchesi, I., Cencetti, S., Marchegiano, P., Frezza, G., Borella, P., and Bargellini, A. (2012). Control of Legionella contamination in a hospital water distribution system by monochloramine. Am. J. Infect. Control 40 (3), 279–281. doi:10.1016/j.ajic.2011.03.008
Marchesi, I., Ferranti, G., Bargellini, A., Marchegiano, P., Predieri, G., Stout, J. E., and Borella, P. (2013). Monochloramine and chlorine dioxide for controlling Legionella pneumophila contamination: biocide levels and disinfection by-product formation in hospital water networks. J. Water Health 11 (4), 738–747. doi:10.2166/wh.2013.079
Marchesi, I., Marchegiano, P., Bargellini, A., Cencetti, S., Frezza, G., Miselli, M., and Borella, P. (2011). Effectiveness of different methods to control Legionella in the water supply: Ten-year experience in an Italian university hospital. J. Hosp. Infect. 77 (1), 47–51. doi:10.1016/j.jhin.2010.09.012
Marciano-Cabral, F., Jamerson, M., and Kaneshiro, E. S. (2010). Free-living amoebae, Legionella and Mycobacterium in tap water supplied by a municipal drinking water utility in the USA. J. Water Health 8 (1), 71–82. doi:10.2166/wh.2009.129
Marinelli, L., Cottarelli, A., Solimini, A., Del Cimmuto, A., and De Giusti, M. (2017). Evaluation of timing of re-appearance of VBNC Legionella for risk assessment in hospital water distribution systems, Ann. Ig. 29 (5), 431–439. doi:10.7416/ai.2017.2175
Marre, R., Abu Kwaik, Y., Bartlett, C., Cianciotto, N. P., Fields, B. S., Frosch, M., Hacker, J., and Lück, P. C. (2001). Legionella. Washington, D.C., USA: ASM Press.
Marston, B. J., Plouffe, J.F., File, T.M., Hackman, B.A., Salstrom, S.-J., Lipman, H.B., Kolczak, M.S., and Breiman, R.F. (1997). Incidence of community-acquired pneumonia requiring hospitalization. Results of a population-based active surveillance Study in Ohio. The Community-Based Pneumonia Incidence Study Group. Arch. Intern. Med. 157 (15), 1709–1718. doi:10.1001/archinte.157.15.1709
Mathys, W., Stanke, J., Harmuth, M., and Junge-Mathys, E. (2008). Occurrence of Legionella in hot water systems of single-family residences in suburbs of two German cities with special reference to solar and district heating. Int. J. Hyg. Environ. Health 211 (1-2), 179–85. doi:10.1016/j.ijheh.2007.02.004
McGuire, M. J. (2006). Eight revolutions in the history of US drinking water disinfection. J. Am. Water Works Assoc. 98 (3), 123–149. doi:10.1002/j.1551-8833.2006.tb07612.x
Mercante, J. W., and Winchell, J. M. (2015). Current and emerging Legionella diagnostics for laboratory and outbreak investigations. Clin. Microbiol. Rev. 28 (1), 95–133. doi:10.1128/cmr.00029-14
Mogoa, E., Bodet, C., Morel, F., Rodier, M.-H., Legube, B., and Héchard, Y. (2011). Cellular response of the amoeba Acanthamoeba castellanii to chlorine, chlorine dioxide, and monochloramine treatments. Appl. Environ. Microbiol. 77 (14), 4974–4980. doi:10.1128/aem.00234-11
Momba, M., Kfir, R., Venter, S. N., and Cloete, T. E. (2000). An overview of biofilm formation in distribution systems and its impact on the deterioration of water quality. Water SA 26 (1), 59–66.
Moritz, M. M., Flemming, H.-C., and Wingender, J. (2010). Integration of Pseudomonas aeruginosa and Legionella pneumophila in drinking water biofilms grown on domestic plumbing materials. Int. J. Hyg. Environ. Health 213 (3), 190–197. doi:10.1016/j.ijheh.2010.05.003
Muder, R. R., Victor, L.Y., and Woo, A.H. (1986). Mode of transmission of Legionella pneumophila. A critical review. Arch. Intern. Med. 146 (8), 1607–1612. doi:10.1001/archinte.146.8.1607
Nocker, A., Sossa-Fernandez, P., Burr, M. D., and Camper, A. K. (2007). Use of propidium monoazide for live/dead distinction in microbial ecology. Appl. Environ. Microbiol. 73 (16), 5111–5117. doi:10.1128/aem.02987-06
Norton, C. D., and LeChevallier, M. W. (1997). Chloramination: Its effect on distribution system water quality. J. Am. Water Works Assoc. 89 (7), 66–77. doi:10.1002/j.1551-8833.1997.tb08260.x
Okpara, C., Oparaku, N., and Ibeto, C. (2011). An overview of water disinfection in developing countries and potentials of renewable energy. J. Environ. Sci. Technol. 4 (1), 18–30. doi:10.3923/jest.2011.18.30
Oliver, J. D. (2010). Recent findings on the viable but nonculturable state in pathogenic bacteria. FEMS Microbiol. Rev. 34 (4), 415–425. doi:10.1111/j.1574-6976.2009.00200.x
Olivieri, V. P., Snead, M. C., Krusé, C. W., and Kawata, K. (1986). Stability and effectiveness of chlorine disinfectants in water distribution systems. Environ. Health Perspect. 69, 15–29. doi:10.1289/ehp.866915
Orkis, L. T., Harrison, L. H., Mertz, K. J., Brooks, M. M., Bibby, K. J., and Stout, J. E. (2018). Environmental sources of community-acquired legionnaires' disease: A review. Int. J. Hyg. Environ. Health 221 (5), 764–774. doi:10.1016/j.ijheh.2018.04.013
Pan, Y., and Breidt, F. (2007). Enumeration of viable Listeria monocytogenes cells by real-time PCR with propidium monoazide and ethidium monoazide in the presence of dead cells. Appl. Environ. Microbiol. 73 (24), 8028–8031. doi:10.1128/aem.01198-07
Párraga-Niño, N., Quero, S., Ventós-Alfonso, A., Uria, N., Castillo-Fernandez, O., Ezenarro, J. J., Muñoz, F.-X., Garcia-Nuñez, M., and Sabrià, M. (2018). New system for the detection of Legionella pneumophila in water samples. Talanta 189, 324–331. doi:10.1016/j.talanta.2018.07.013
Payment, P. (1999). Poor efficacy of residual chlorine disinfectant in drinking water to inactivate waterborne pathogens in distribution systems. Can. J. Microbiol. 45 (8), 709–715. doi:10.1139/w99-063
Perrin, Y., Bouchon, D., Delafont, V., Moulin, L., and Héchard, Y. (2019a). Microbiome of drinking water: A full-scale spatio-temporal study to monitor water quality in the Paris distribution system. Water Res. 149, 375–385. doi:10.1016/j.watres.2018.11.013
Perrin, Y., Bouchon, D., Héchard, Y., and Moulin, L. (2019b). Spatio-temporal survey of opportunistic premise plumbing pathogens in the Paris drinking water distribution system. Int. J. Hyg. Environ. Health 222 (4), 687–694. doi:10.1016/j.ijheh.2019.04.010
Pierre, D., Baron, J. L., Ma, X., Sidari, F. P., Wagener, M. M., and Stout, J. E. (2019). Water quality as a predictor of Legionella positivity of building water systems. Pathogens 8 (4), 295. doi:10.3390/pathogens8040295
Pressman, J. G., Lee, W. H., Bishop, P. L., and Wahman, D. G. (2012). Effect of free ammonia concentration on monochloramine penetration within a nitrifying biofilm and its effect on activity, viability, and recovery. Water Res. 46 (3), 882–894. doi:10.1016/j.watres.2011.11.071
Principe, L., Tomao, P., and Visca, P. (2017). Legionellosis in the occupational setting. Environ. Res. 152, 485–495. doi:10.1016/j.envres.2016.09.018
Proctor, C. R., Dai, D., Edwards, M. A., and Pruden, A. (2017). Interactive effects of temperature, organic carbon, and pipe material on microbiota composition and Legionella pneumophila in hot water plumbing systems. Microbiome 5, 130. doi:10.1186/s40168-017-0348-5
Prussin, A. J., II, Schwake, D. O., and Marr, L. C. (2017). Ten questions concerning the aerosolization and transmission of Legionella in the built environment. Build. Environ. 123, 684–695. doi:10.1016/j.buildenv.2017.06.024
Pryor, M., Springthorpe, S., Riffard, S., Brooks, T., Huo, Y., Davis, G., and Sattar, S. A. (2004). Investigation of opportunistic pathogens in municipal drinking water under different supply and treatment regimes. Water Sci. Technol. 50 (1), 83–90. doi:10.2166/wst.2004.0025
Qin, K., Struewing, I., Domingo, J., Lytle, D., and Lu, J. (2017). Opportunistic pathogens and microbial communities and their associations with sediment physical parameters in drinking water storage tank sediments. Pathogens 6 (4), 54. doi:10.3390/pathogens6040054
Reingold, A. L., Thomason, B. M., Brake, B. J., Thacker, L., Wilkinson, H. W., and Kuritsky, J. N. (1984). Legionella pneumonia in the United States: The distribution of serogroups and species causing human illness. J. Infect. Dis. 149 (5), 819–819. doi:10.1093/infdis/149.5.819
Ribas, F., Perramon, J., Terradillos, A., Frias, J., and Lucena, F. (2000). The Pseudomonas group as an indicator of potential regrowth in water distribution systems. J. Appl. Microbiol. 88 (4), 704–710. doi:10.1046/j.1365-2672.2000.01021.x
Rogers, J., Dowsett, A., Dennis, P., Lee, J., and Keevil, C. (1994). Influence of temperature and plumbing material selection on biofilm formation and growth of Legionella pneumophila in a model potable water system containing complex microbial flora. Appl. Environ. Microbiol. 60 (5), 1585–1592. doi:10.1128/aem.60.5.1585-1592.1994
Ross, W., van Leeuwen, J., and Grabow, W. (1976). Studies on disinfection and chemical oxidation with ozone and chlorine in water reclamation. Water SA 2 (l), 25–32.
Rusin, P. A., Rose, J. B., Haas, C. N., and Gerba, C. P. (1997). “Risk assessment of opportunistic bacterial pathogens in drinking water.” in Reviews of Environmental Contamination and Toxicology: Continuation of Residue Reviews (Volume 152). Editor G. W. Ware (New York, New York, USA: Springer-Verlag (Springer Science+Business Media)), pp. 57–83.
Sabria, M., and Yu, V. L. (2002). Hospital-acquired legionellosis: solutions for a preventable infection. The Lancet Infect. Dis. 2 (6), 368–373. doi:10.1016/s1473-3099(02)00291-8
Saby, S., Vidal, A., and Suty, H. (2005). Resistance of Legionella to disinfection in hot water distribution systems. Water Sci. Technol. 52 (8), 15–28. doi:10.2166/wst.2005.0216
Salinas, M. B., Fenoy, S., Magnet, A., Vaccaro, L., Gomes, T. D., Angulo, S., Hurtado, C., Ollero, D., Valdivieso, E., and del Águila, C. (2021). Are pathogenic Legionella non-pneumophila a common bacteria in water distribution networks? Water Res. 196, 117013. doi:10.1016/j.watres.2021.117013
Schoen, M. E., and Ashbolt, N. J. (2011). An in-premise model for Legionella exposure during showering events. Water Res. 45 (18), 5826–5836. doi:10.1016/j.watres.2011.08.031
Schoenen, D. (2002). Role of disinfection in suppressing the spread of pathogens with drinking water: possibilities and limitations. Water Res. 36 (15), 3874–3888. doi:10.1016/s0043-1354(02)00076-3
Schwake, D. O., Alum, A., and Abbaszadegan, M. (2015). Impact of environmental factors on Legionella populations in drinking water. Pathogens 4 (2), 269–282. doi:10.3390/pathogens4020269
Sciuto, E. L., Laganà, P., Filice, S., Scalese, S., Libertino, S., Corso, D., Faro, G., and Coniglio, M. A. (2021). Environmental management of Legionella in domestic water systems: consolidated and innovative approaches for disinfection methods and risk assessment. Microorganisms 9 (3), 577. doi:10.3390/microorganisms9030577
September, S., Brözel, V., and Venter, S. (2004). Diversity of nontuberculoid Mycobacterium species in biofilms of urban and semi urban drinking water distribution systems. Appl. Environ. Microbiol. 70 (12), 7571–7573. doi:10.1128/aem.70.12.7571-7573.2004
Serrano-Suárez, A., Dellundé, J., Salvadó, H., Cervero-Aragó, S., Méndez, J., Canals, O., Blanco, S., Arcas, A., and Araujo, R. (2013). Microbial and physicochemical parameters associated with Legionella contamination in hot water recirculation systems. Environ. Sci. Pollut. Res. 20 (8), 5534–5544. doi:10.1007/s11356-013-1557-5
Shands, K. N., Ho, J. L., Meyer, R. D., Gorman, G. W., Edelstein, P. H., Mallison, G. F., Finegold, S. M., and Fraser, D. W. (1985). Potable water as a source of legionnaires’ disease. JAMA 253 (10), 1412–1416. doi:10.1001/jama.253.10.1412
Slimani, S., Robyns, A., Jarraud, S., Molmeret, M., Dusserre, E., Mazure, C., Facon, J. P., Lina, G., Etienne, J., and Ginevra, C. (2012). Evaluation of propidium monoazide (PMA) treatment directly on membrane filter for the enumeration of viable but non cultivable Legionella by qPCR. J. Microbiol. Methods 88 (2), 319–321. doi:10.1016/j.mimet.2011.12.010
Smeets, P. W. M. H., Medema, G. J., and Van Dijk, J. C. (2009). The Dutch secret: how to provide safe drinking water without chlorine in the Netherlands. Drink. Water Eng. Sci. 2 (1), 1–14. doi:10.5194/dwes-2-1-2009
Sobsey, M. D. (1989). Inactivation of health-related microorganisms in water by disinfection processes. Water Sci. Technol. 21 (3), 179–195. doi:10.2166/wst.1989.0098
Springston, J. P., and Yocavitch, L. (2017). Existence and control of Legionella bacteria in building water systems: A review. J. Occup. Environ. Hyg. 14 (2), 124–134. doi:10.1080/15459624.2016.1229481
States, S. J., Conley, L. F., Kuchta, J. M., Oleck, B. M., Lipovich, M. J., Wolford, R. S., Wadowsky, R. M., McNamara, A. M., Sykora, J. L., Keleti, G., and Yee, R.B. (1987). Survival and multiplication of Legionella pneumophila in municipal drinking water systems. Appl. Environ. Microbiol. 53 (5), 979–986. doi:10.1128/aem.53.5.979-986.1987
Storey, M. V., Ashbolt, N. J., and Stenström, T. A. (2004a). Biofilms, thermophilic amoebae and Legionella pneumophila—a quantitative risk assessment for distributed water. Water Sci. Technol. 50 (1), 77–82. doi:10.2166/wst.2004.0023
Storey, M. V., Winiecka-Krusnell, J., Ashbolt, N. J., and Stenström, T.-A. (2004b). The efficacy of heat and chlorine treatment against thermotolerant Acanthamoebae and Legionellae. Scand. J. Infect. Dis. 36 (9), 656–662. doi:10.1080/00365540410020785
Stout, J. E., Lin, Y. E., Goetz, A. M., and Muder, R. R. (1998). Controlling Legionella in hospital water systems: Experience with the superheat-and-flush method and copper-silver ionization. Infect. Control Hosp. Epidemiol. 19 (12), 911–914. doi:10.2307/30142016
Stout, J. E., and Yu, V. L. (1997). Legionellosis. N. Engl. J. Med. 337 (10), 682–687. doi:10.1056/nejm199709043371006
Stout, J. E., Yu, V. L., and Best, M. G. (1985). Ecology of Legionella pneumophila within water distribution systems. Appl. Environ. Microbiol. 49 (1), 221–228. doi:10.1128/aem.49.1.221-228.1985
Tallon, P., Magajna, B., Lofranco, C., and Leung, K.T. (2005). Microbial indicators of faecal contamination in water: A current perspective. Water, Air, Soil Pollut. 166 (1-4), 139–166. doi:10.1007/s11270-005-7905-4
Taylor, M. J., Bentham, R. H., and Ross, K. E. (2014). Limitations of using propidium monoazide with qPCR to discriminate between live and dead Legionella in biofilm samples. Microbiol. Insights 7, 15–24. doi:10.4137/MBI.S17723
Taylor, R. H., Falkinham, J. O., Norton, C. D., and LeChevallier, M. W. (2000). Chlorine, chloramine, chlorine dioxide, and ozone susceptibility of Mycobacterium avium. Appl. Environ. Microbiol. 66 (4), 1702–1705. doi:10.1128/aem.66.4.1702-1705.2000
The Council of the EU, (2020). Directive (EU) 2020/2184 of the European parliament and of the council of 16 December 2020 on the quality of water intended for human consumption (Recast). Official J. Eur. Union L435, 1–62.
The International Organization for Standardization (2017). Water Quality—Enumeration of Legionella (ISO 11731:2017). Geneva, Switzerland: The International Organization for Standardization.
Thomas, J. M., and Ashbolt, N. J. (2011). Do free-living amoebae in treated drinking water systems present an emerging health risk? Environ. Sci. Technol. 45 (3), 860–869. doi:10.1021/es102876y
Unger, C., and Lück, C. (2012). Inhibitory effects of silver ions on Legionella pneumophila grown on agar, intracellular in Acanthamoeba castellanii and in artificial biofilms. J. Appl. Microbiol. 112 (6), 1212–1219. doi:10.1111/j.1365-2672.2012.05285.x
US EPA (1989). National primary drinking water regulations (40 CFR Part 141). Federal Register 54 (124), 27526–27537.
US EPA (2000). Legionella: Drinking Water Fact Sheet. Available at: https://www.epa.gov/sites/default/files/2015-10/documents/legionella-factsheet.pdf (Accessed August 2021).
US EPA (2009). Drinking water contaminant candidate list 3-final (74 FR 51850). Federal Register 74 (194), 51850–51862.
US EPA (2013). National primary drinking water regulations: Revisions to the total coliform rule: 40 CFR Parts 141 and 142 (78 FR 10269). Federal Register 78 (30), 10269–10365.
US EPA (2016). Drinking water contaminant candidate list 4-Final (81 FR 81099). Federal Register 81 (222), 81099–81114.
US EPA (2021). Drinking water contaminant candidate list 5—Draft: 40 CFR Part 141 (86 FR 37948). Federal Register 86 (135), 37948–37972.
Valciņa, O., Pūle, D., Mališevs, A., Trofimova, J., Makarova, S., Konvisers, G., Bērziņš, A., and Krūmiņa, A. (2019). Co-occurrence of free-living amoeba and Legionella in drinking water supply systems. Medicina 55 (8), 492.
van der Kooij, D. (2003). “Managing regrowth in drinking water distribution systems.” in Heterotrophic Plate Counts and Drinking-Water Safety: The Significance of HPCs for Water Quality and Human Health. Editors J. Bartram, J. Cotruvo, M. Exner, C. Fricker, and A. Glasmacher (London, United Kingdom: IWA Publishing), pp. 199–232.
van der Wielen, P. W. J. J., and van der Kooij, D. (2013). Nontuberculous mycobacteria, fungi, and opportunistic pathogens in unchlorinated drinking water in the Netherlands. Appl. Environ. Microbiol. 79 (3), 825–834. doi:10.1128/aem.02748-12
Van Kenhove, E., Dinne, K., Janssens, A., and Laverge, J. (2019). Overview and comparison of Legionella regulations worldwide. Am. J. Infect. Control 47 (8), 968–978. doi:10.1016/j.ajic.2018.10.006
Viasus, D., Calatayud, L., McBrown, M. V., Ardanuy, C., and Carratalà, J. (2019). Urinary antigen testing in community-acquired pneumonia in adults: An update. Expert Rev. Anti-Infect. Ther. 17 (2), 107–115. doi:10.1080/14787210.2019.1565994
Vikesland, P. J., Ozekin, K., and Valentine, R. L. (1998). Effect of natural organic matter on monochloramine decomposition: Pathway elucidation through the use of mass and redox balances. Environ. Sci. Technol. 32 (10), 1409–1416. doi:10.1021/es970589a
Vikesland, P. J., and Valentine, R. L. (2000). Reaction pathways involved in the reduction of monochloramine by ferrous iron. Environ. Sci. Technol. 34 (1), 83–90. doi:10.1021/es990511p
Waak, M. B., LaPara, T. M., Hallé, C., and Hozalski, R. M. (2018). Occurrence of Legionella spp. in water-main biofilms from two drinking water distribution systems. Environ. Sci. Technol. 52 (14), 7630–7639. doi:10.1021/acs.est.8b01170
Walker, J. T., and McDermott, P. J. (2021). Confirming the presence of Legionella pneumophila in your water system: A review of current Legionella testing methods. J. AOAC Int. 104 (4), 135–1147. doi:10.1093/jaoacint/qsab003
Wang, H., Bédard, E., Prévost, M., Camper, A. K., Hill, V. R., and Pruden, A. (2017). Methodological approaches for monitoring opportunistic pathogens in premise plumbing: A review. Water Res. 117, 68–86. doi:10.1016/j.watres.2017.03.046
Wang, H., Edwards, M., Falkinham, J. O., and Pruden, A. (2012a). Molecular survey of the occurrence of Legionella spp., Mycobacterium spp., Pseudomonas aeruginosa, and amoeba hosts in two chloraminated drinking water distribution systems. Appl. Environ. Microbiol. 78 (17), 6285–6294. doi:10.1128/aem.01492-12
Wang, H., Edwards, M. A., Falkinham, J. O., and Pruden, A. (2013). Probiotic approach to pathogen control in premise plumbing systems? A review. Environ. Sci. Technol. 47 (18), 10117–10128. doi:10.1021/es402455r
Wang, H., Masters, S., Edwards, M. A., Falkinham, J. O., and Pruden, A. (2014). Effect of disinfectant, water age, and pipe materials on bacterial and eukaryotic community structure in drinking water biofilm. Environ. Sci. Technol. 48 (3), 1426–1435. doi:10.1021/es402636u
Wang, H., Masters, S., Falkinham, J. O., Edwards, M. A., and Pruden, A. (2015). Distribution system water quality affects responses of opportunistic pathogen gene markers in household water heaters. Environ. Sci. Technol. 49 (14), 8416–8424. doi:10.1021/acs.est.5b01538
Wang, H., Masters, S., Hong, Y., Stallings, J., Falkinham, J. O., Edwards, M. A., and Pruden, A. (2012b). Effect of disinfectant, water age, and pipe material on occurrence and persistence of Legionella, mycobacteria, Pseudomonas aeruginosa, and two amoebas. Environ. Sci. Technol. 46 (21), 11566–11574. doi:10.1021/es303212a
Wen, X., Chen, F., Lin, Y., Zhu, H., Yuan, F., Kuang, D., Jia, Z., and Yuan, Z. (2020). Microbial indicators and their use for monitoring drinking water quality-A review. Sustainability 12 (6), 2249. doi:10.3390/su12062249
Whiley, H. (2017). Response to comments on Whiley Legionella risk management and control in potable water systems: Argument for the abolishment of routine testing. Int. J. Environ. Res. Public Health 14 (1), 12. doi:10.3390/ijerph14010103
Whiley, H., Keegan, A., Fallowfield, H., and Bentham, R. (2014a). Detection of Legionella, L. pneumophila and Mycobacterium avium complex (MAC) along potable water distribution pipelines. Int. J. Environ. Res. Public. Health 11 (7), 7393–7405. doi:10.3390/ijerph110707393
Whiley, H., Keegan, A., Fallowfield, H., and Ross, K. (2014b). Uncertainties associated with assessing the public health risk from Legionella. Front. Microbiol. 5, 501. doi:10.3389/fmicb.2014.00501
Whiley, H., and Taylor, M. (2016). Legionella detection by culture and qPCR: comparing apples and oranges. Crit. Rev. Microbiol. 42 (1), 65–74. doi:10.3109/1040841x.2014.885930
Wingender, J., and Flemming, H.-C. (2011). Biofilms in drinking water and their role as reservoir for pathogens. Int. J. Hyg. Environ. Health 214 (6), 417–423. doi:10.1016/j.ijheh.2011.05.009
Wolf-Baca, M., and Siedlecka, A. (2019). Detection of pathogenic bacteria in hot tap water using the qPCR method: preliminary research. SN Appl. Sci. 1 (8), 840. doi:10.1007/s42452-019-0533-1
Wolfe, R. L., Ward, N. R., and Olson, B. H. (1984). Inorganic chloramines as drinking water disinfectants: A review. J. Am. Water Works Assoc. 76 (5), 74–88. doi:10.1002/j.1551-8833.1984.tb05337.x
Wright, J. B. (2000). “Legionella biofilms: Their implications, study and control.” in Biofilms: Recent Advances in Their Study and Control. Editor L.V. Evans (The Netherlands: Harwood Academic Publishers (Taylor & Francis)), pp. 301–321.
Wu, H.-Y., Yan, H., Zheng, M.-L., Sun, M.-M., Wang, Q., Hu, C.-M., Zhan, X.-Y., Yuan, M.-G., Qu, P.-H., and Hu, C.-H. (2019). Legionella qingyii sp. nov., isolated from water samples in China. Int. J. Syst. Evol. Microbiol. 69 (7), 2017–2022. doi:10.1099/ijsem.0.003421
Yáñez, M. A., Nocker, A., Soria-Soria, E., Múrtula, R., Martínez, L., and Catalán, V. (2011). Quantification of viable Legionella pneumophila cells using propidium monoazide combined with quantitative PCR. J. Microbiol. Methods 85 (2), 124–130. doi:10.1016/j.mimet.2011.02.004
Yu, V. L., Plouffe, J. F., Pastoris, M. C., Stout, J. E., Schousboe, M., Widmer, A., Summersgill, J., File, T., Heath, C. M., Paterson, D. L., and Chereshsky, A. (2002). Distribution of Legionella species and serogroups isolated by culture in patients with sporadic community‐acquired legionellosis: An international collaborative survey. J. Infect. Dis. 186 (1), 127-128. doi:10.1086/341087
Zacheus, O. M., and Martikainen, P. J. (1994). Occurrence of legionellae in hot water distribution systems of finnish apartment buildings. Can. J. Microbiol. 40 (12), 993-999. doi:10.1139/m94-159
Zhang, C., Brown, P. J. B., and Hu, Z. (2018). Thermodynamic properties of an emerging chemical disinfectant, peracetic acid. Sci. Total Environ. 621, 948–959. doi:10.1016/j.scitotenv.2017.10.195
Zhang, C., Brown, P. J. B., Miles, R. J., White, T. A., Grant, D. G., Stalla, D., and Hu, Z. (2019). Inhibition of regrowth of planktonic and biofilm bacteria after peracetic acid disinfection. Water Res. 149, 640–649. doi:10.1016/j.watres.2018.10.062
Zhang, C., Hu, Z., and Deng, B. (2016a). Silver nanoparticles in aquatic environments: Physiochemical behavior and antimicrobial mechanisms. Water Res. 88, 403–427. doi:10.1016/j.watres.2015.10.025
Zhang, C., Hu, Z., Li, P., and Gajaraj, S. (2016b). Governing factors affecting the impacts of silver nanoparticles on wastewater treatment. Sci. Total Environ. 572, 852–873. doi:10.1016/j.scitotenv.2016.07.145
Zhang, C., Liang, Z., and Hu, Z. (2014). Bacterial response to a continuous long-term exposure of silver nanoparticles at sub-ppm silver concentrations in a membrane bioreactor activated sludge system. Water Res. 50, 350–358. doi:10.1016/j.watres.2013.10.047
Zhang, C., and Lu, J. (2021). Optimizing disinfectant residual dosage in engineered water systems to minimize the overall health risks of opportunistic pathogens and disinfection by-products. Sci. Total Environ. 770, 145356. doi:10.1016/j.scitotenv.2021.145356
Zhang, C., Qin, K., Struewing, I., Buse, H.Y., Santo Domingo, J., and Lu, J. (2021a). The bacterial community diversity of bathroom hot tap water was significantly lower than that of cold tap and shower water. Front. Microbiol. 12, 433. doi:10.3389/fmicb.2021.625324
Zhang, C., Struewing, I., Mistry, J. H., Wahman, D. G., Pressman, J., and Lu, J. (2021b). Legionella and other opportunistic pathogens in full-scale chloraminated municipal drinking water distribution systems. Water Res. 205, 117571. doi:10.1016/j.watres.2021.117571
Zhang, Y., and Liu, W.-T. (2019). The application of molecular tools to study the drinking water microbiome—Current understanding and future needs. Crit. Rev. Environ. Sci. Technol. 49 (13), 1188–1235. doi:10.1080/10643389.2019.1571351
Keywords: Legionella pneumophila, Mycobacterium, Pseudomonas, drinking water distribution systems, premise plumbing, public health
Citation: Zhang C and Lu J (2021) Legionella: A Promising Supplementary Indicator of Microbial Drinking Water Quality in Municipal Engineered Water Systems. Front. Environ. Sci. 9:684319. doi: 10.3389/fenvs.2021.684319
Received: 15 April 2021; Accepted: 24 August 2021;
Published: 10 November 2021.
Edited by:
Prosun Bhattacharya, Royal Institute of Technology, SwedenReviewed by:
Andrea Turolla, Politecnico di Milano, ItalyXiao-Yong Zhan, Sun Yat-sen University, China
Copyright © 2021 Zhang and Lu. This is an open-access article distributed under the terms of the Creative Commons Attribution License (CC BY). The use, distribution or reproduction in other forums is permitted, provided the original author(s) and the copyright owner(s) are credited and that the original publication in this journal is cited, in accordance with accepted academic practice. No use, distribution or reproduction is permitted which does not comply with these terms.
*Correspondence: Jingrang Lu, bHUuamluZ3JhbmdAZXBhLmdvdg==