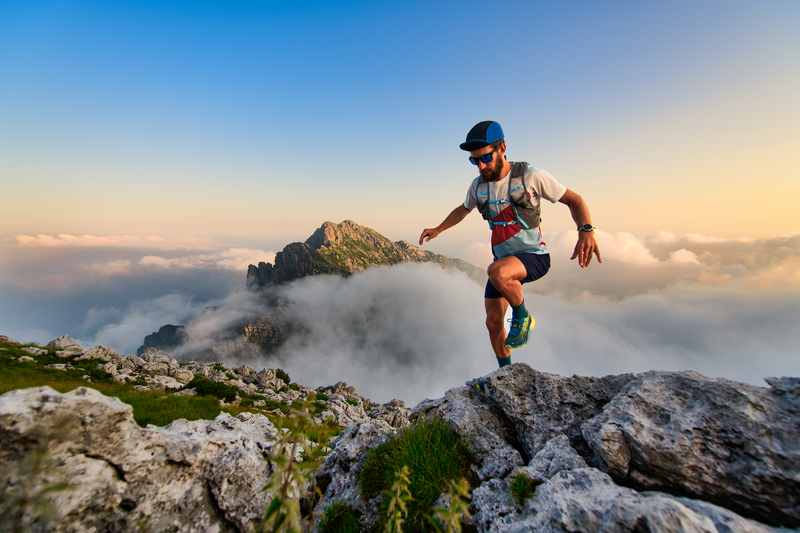
95% of researchers rate our articles as excellent or good
Learn more about the work of our research integrity team to safeguard the quality of each article we publish.
Find out more
ORIGINAL RESEARCH article
Front. Environ. Sci. , 05 August 2021
Sec. Land Use Dynamics
Volume 9 - 2021 | https://doi.org/10.3389/fenvs.2021.683397
Alpine glacial environments and their fluvial systems are among those landscapes most comprehensively affected by climate change. Typically, studies on the consequences of climate change in such environments, e.g., glacier retreat, cover a maximum of 70 years, reflecting the availability of orthophotos or satellite images. This study addresses the long-term transformation processes in a glaciated catchment and highlights the role of human agency in a changing Alpine environment. In order to identify land cover changes between 1820 and 2015 in the Long-Term Ecosystem Research (LTER) site “Jamtal” (Tyrol, Austria) we apply a “regressive-iterative GIS reconstruction method” combining both historical maps and optical remote sensing data. Below 2,100 m a.s.l. the Jamtal experienced a massive 62% decline of unvegetated debris areas and bedrock outcrops (so-called “wasteland”) that was mainly transformed to grassland and sparsely wooded areas. Forests increased by an outstanding 323% and grassland was replaced by sparsely or densely wooded areas. This primarily reflects the abandonment of agricultural uses at unfavourable remote sites. In the higher (formerly) glaciated subbasin, ice-covered areas declined by 55%, which was associated with a major (82%) growth of exposed wasteland. Concurrently, Alpine grassland expanded by 196% and krumholz even by 304%. Approximately half of the new fluvial system that evolved in deglaciated areas between 1870 and 1921 still existed in 2015. Unconsolidated debris buried almost one fifth of the new channels, and almost one third was colonized by vegetation. Recent data show that the deglaciation process is much faster than the colonization process by Alpine vegetation. Accordingly, the extent of wasteland has expanded and potentially amplifies the sediment supply to the fluvial system. Alterations in high Alpine hydrological and sediment/debris regimes significantly affect human use in lower, more favourable areas of the Alpine region. The long-term investigation of the Alpine landscape reveals that the transformation processes have accelerated in recent decades.
Alpine environments are affected by climate change in multiple ways. Compared to other terrestrial ecosystems they experienced a more pronounced increase in air temperature in recent decades (Auer et al., 2007; Gobiet et al., 2014). While the mean temperature rise in Austria amounted to 2°C between 1970 and 2015, the overall increase in the Northern Hemisphere was significantly lower (+1.5°C; Blöschl et al., 2018). Major changes in precipitation patterns are also evident. In Austria, between 1996 and 2014, the mean summer precipitation increased by 14% in relation to the reference period 1976–1995. In contrast, winter precipitation decreased by 7% at the same time. In high altitudes, such as in the Long-Term Ecosystem Research (LTER) site Jamtal valley (Tyrol, Austria), gridded precipitation data even show a winter-time decrease by 10–20% (Hiebl and Frei, 2016). Both the increasing air temperatures and altered precipitation patterns are reflected by the flow and flood regimes of Alpine rivers (Parajka et al., 2010; Hofstätter et al., 2017; Lebiedzinski and Fürst, 2018). Blöschl et al. (2017, 2018) report a more distinct increase of mean annual floods in the period 1976–2014 in smaller (<500 km2) versus larger catchments. Gauge data downstream of the Jamtal valley indicate a (non-significant) trend of increasing mean annual floods by 5–20% per decade (α = 0.05).
Glacier decline most impressively reflects the climatic and hydrological changes (Oerlemans, 1994; Zemp et al., 2015; Fischer et al., 2016a). Glaciers in Austria lost about 20% of their volume in only 10 years between 2006 and 2016 (Helfricht et al., 2019). Fischer et al. (2016b) report a mean annual mass loss of the Jamtalferner, the major glacier in the Jamtal, by 1.0 m water equivalent (w.e.) between 1988 and 2020. The melting glaciers show increasing supraglacial debris on their surface and expose larger areas of unconsolidated and unstable sediments in the proglacial area (i.e., the formerly ice-covered part of the glacier foreland). In such areas, meltwater streams extend their channel system upvalley, following the receding glacier tongue. Newly established fluvial systems fed by meltwater and precipitation rework the freshly deposited glacial sediments in the proglacial zone and partly transport the sediments further downstream (Warburton, 1990; Marren and Toomath, 2014; Lane et al., 2017).
Both increasing temperatures and new exposed areas covered with unconsolidated sediments provide the basis for upward expansion of Alpine vegetation (Dullinger et al., 2003; Dullinger et al., 2004; Fischer et al., 2019). Besides grasses and herbaceous plants, also woody plants (i.e., krumholz) have been colonizing high-altitude ranges since the early 20th century. Leonelli et al. (2011) reported a treeline shift in the Italian Alps by 115 m between 1900 and 2000. According to Walther et al. (2005), within one century, several species of vascular plants shifted upwards on average by 278 m in the Swiss Alps. Newly emerged fluvial systems in proglacial areas are also affected by the upward encroaching vegetation. The roots of these plants help stabilize loose sediments and, thus, the boundaries of fluvial channels (Gurnell et al., 2016). In recent years, several studies have been published on the interaction between fluvial processes and vegetation dynamics [e.g., “fluvial biogeomorphic succession concept” by Corenblit et al. (2007, 2015)]. They primarily focus on braided river stretches in glacially unaffected catchments or further downstream of the glacier forelands (e.g., Gurnell et al., 2001; Egger et al., 2019; Scorpio et al., 2020). Against this background the present study focusses on two research questions involving long-term changes of high Alpine glacial fluvial systems:
1) How has climate warming changed the “landscape template” controlled by topography and declining glaciers?
The physical structure of a river basin (or parts thereof), the “landscape template” or “physical template”, provides the basic conditions for the evolution of fluvial systems (Roni and Beechie, 2013). In similar landscapes (defined by geology, tectonics and climate), similar types of channel patterns will more likely occur. Thus, the “landscape template” sets limits on the range of potential fluvial forms and riverine habitats upon which ecological processes occur (Brierley and Fryirs, 2005; Allen and Castillo, 2007). In human time scales, most landscapes remain comparably stable. Climate change, however, may significantly alter the physical setting of glacial headwater basins and, accordingly, the associated fluvial system (Comiti et al., 2019). Most apparent are declining glaciers, the increase of unvegetated debris and bedrock outcrops (“wasteland”) and the upward shift of the Alpine vegetation belt. Historical glacier extensions have been analysed by Fischer et al. (2018) for several Austrian glaciated mountain ranges and, in particular based on historical maps, for the glacier Jamtalferner in the Jamtal. Historical sources were also used to estimate former glacier extensions in the Central Andes (Le Quesne et al., 2009) and in northern Norway (Weber et al., 2020). Similarly, climate-related shifts of Alpine vegetation have been gaining considerable awareness in recent decades (see review in Verrall and Pickering, 2020). Fischer et al. (2019) analysed the upward shift and transformation of the Alpine vegetation in the foreland of the Jamtalferner using the “Normalized Difference Vegetation Index” (NDVI) calculated from 13 Landsat scenes between 1985 and 2016. Since such studies are bound to available accurate historical sources (orthophotos, maps) they are usually restricted to timeframes of several decades. Owing to the long tradition of military land surveys and cadastre maps in the Habsburg Monarchy, a series of cartographic sources is available for the analysis of the long-term transformation of Alpine land cover. In applying the “regressive-iterative GIS reconstruction method” described in Hohensinner et al. (2013a), we extend the timeframe for the investigation of the land cover changes in the Jamtal back to 1820 CE. The resulting dataset for the entire Jamtal provides the basis for the second research question:
2) Which trajectory of biogeomorphic channel evolution is identifiable over the long term in the proglacial zone?
We hypothesize a two-step process. First, the meltwater streams extend their courses upwards following the retreating glacier and develop new channel patterns (“geomorphic phase” sensu Corenblit et al., 2015). In the following “pioneer phase” and the “biogeomorphic phase”, plant species gradually colonize and stabilize the loose debris (Eichel, 2019).
Methodologically, we combine the more accurate NDVI approach using optical satellite images from the Landsat satellite series for the period 1985–2016 (Fischer et al., 2019) with the long-term, but less accurate “regressive-iterative reconstruction method” based on historical maps (1820–2015; Hohensinner et al., 2013a). The combined approach enables drawing further conclusions compared to using solely one method. It extends our current knowledge about the biogeomorphic interactions in proglacial zones back to times prior to the last major glacial advance of the Little Ice Age (LIA).
The Jamtal represents a typical glacially shaped Alpine valley (catchment) in the Silvretta mountain range in the SW of the Austrian province Tyrol (Figure 1). It stretches across an area of 54.3 km2 and experiences an altitudinal range between 1,564 m a.s.l. at the valley mouth in the village Galtür and 3,397 m a.s.l. at the border to Switzerland. The geological basement consists of metamorphic rocks, i.e. mostly different types of gneiss or amphibolite. The foreland of the glaciers (the Jamtalferner being the largest glacier) is covered by coarse-grained and blocky siliceous glacial material (Fischer et al., 2019). After the glaciers in the Jamtal had reached their LIA maximum in 1864, different types of palaeosoils, such as syrosems and rankers, were exposed in deglaciated areas. Since at least Medieval Times the Alpine pastures in the Jamtal have been used for cattle husbandry during the summer months, but the history of human utilisation in that area extends over several millennia (Reitmaier et al., 2013). Pollen analyses and radiocarbon-datings of fossil trees found close to the Jamtal hut document changes of the Alpine vegetation and altitudinal shifts of the treeline caused by climate change and past human land use (see Jamtalhütte in Figure 1; Dietre et al., 2014; Patzelt, 2019).
FIGURE 1. Study area “Jamtal” valley south of the village Galtür with the glacier Jamtalferner at the border between Tyrol and Switzerland in 2015 (orange boundary: detail study area “Jamtalferner subbasin”; basemaps: tiris maps, Tyrol; Wikimedia Commons, Tschubby 2018).
The Jambach river as the main stem is fed by meltwater of the glacier Jamtalferner and of several smaller glaciers. Thus, the river shows a torrential hydrological regime in summer as opposed to comparably low and stable flow conditions in winter. Numerous tributaries draining the hillslopes of the Jamtal join the Jambach. The largest tributary called Futschölbach drains a smaller subbasin in the SE valley (Figure 1). Due to the retreating glacier, the course of the Jambach has been continuously extending upwards since 1864. In 2019, the total length between the Jamtalferner and the outlet at the Trisanna river in Galtür was 12.98 km. The Jambach features a braided channel in the sander (outwash plain) directly below the terminus of the glacier tongue. Today, the upper third of its course is characterised by alternating flat bar-braided and steep bedrock-confined sections. Further downstream, the Jambach is mostly confined by the steep valley sides, and close to Galtür the valley bottom slightly widens.
One of the largest glaciers in the Silvretta region, the Jamtalferner, is located in the head of the valley. Relicts of several other smaller glaciers are still present in higher areas. Monitoring of the glacier length started already in 1892, and since 1988 the mass balance has been intensively monitored in the framework of a long-term ecological research (LTER) program (Fischer et al., 2016a; Fischer et al., 2018; Fischer et al., 2019). Besides cattle husbandry, direct human interventions include the establishment of the Alpine refuge Jamtalhütte at 2,165 m a.s.l. in 1882 and the construction of a weir in the middle of the valley in 1950, where most of the discharge is deviated for hydro-energetic purposes (Figure 1). Thus, the potential annual mean flow of 3.8 m3/s at the river mouth is considerably reduced (based on HAÖ, 2007). Sediments that are deposited directly upstream of the weir are dredged and then released to the river further downstream. While the riverbed upstream of the weir is largely unaffected by embankments, the downstream course has been repeatedly straightened and narrowed, and longer bank sections are protected.
The present study analyses the reconstructed land cover datasets for three different subregions (Table 1 and Figure 1). The largest study area encompasses the entire Jamtal, for which land cover was analysed in three altitudinal belts (<2,100/2,100–2,800/>2,800 m a.s.l.). Historically, areas below 2,100 m a.s.l. have been potentially favourable for various types of human use such as cattle husbandry, cultivation of crops and the exploitation of (krumholz) wood for fuel or construction wood. The lowest elevation reached by the Jamtalferner between 1820 and 2015 was 2,118 m a.s.l. and maximum elevation of densely forested areas was 2,184 m a.s.l. in 2015 (in the 19th and early 20th century much lower; present study). The area between 2,100 and 2,800 m a.s.l. is mainly formed by wasteland, glaciers and Alpine grassland, which could be partly used for cattle-raising. Until 2015, except for some dispersed smaller patches, notable vegetated areas of any kind reached a maximum elevation of 2,780 m a.s.l. Accordingly, the third altitudinal belt refers to areas above 2,800 m a.s.l.
TABLE 1. Areal extents and altitudes of the three study areas analysed in the present study (compare Figure 1).
The second largest study area refers to the upper part of the Jamtal valley, i.e., the subbasin of the Jamtalferner (Figure 1). Besides the main glacier and adjacent smaller glaciers it also includes lower-lying slopes and the valley floor. The third study area is defined by the deglaciated area located between LIA maximum extents in 1864 and the 2015 glacier margin.
To reconstruct the land cover in the Jamtal at five points in time (1820, 1870, 1921, 1970 and 2015) we georeferenced and vectorized numerous historical maps (Table 2). Several orthophotos and a digital elevation model (DEM) provided a profound data basis for representing the land cover in 2015 and 1970. The GIS reconstruction for the year 1921 is mainly based on three historical maps and plans. For the situation in 1870 the “Third Military Survey” from 1870 to 1872 (scale 1:25,000) was used. The oldest sources, i.e., the “Second Military Survey” 1816–1821 (1:28,800) and the “Franziscean Cadastre” 1855–1857 (1:2,880/5,760), formed the basis for the land cover reconstruction in 1820. The cadastre maps and the land surveys were created by military surveyors for the entire Habsburg Monarchy and followed very elaborate survey instructions that were defined in advance (Fuhrmann, 2007). These maps were made for land taxation and show the land cover/uses of each plot of land. In contrast, the Military Surveys primarily focus on the geographical situation and landscape structures such as water courses and terrain topography. Besides the maps shown in Table 2, additional historical sources in between the five time steps were studied, but not georeferenced in the GIS. The delimitation of the main glacier Jamtalferner between 1870 and 2015 is based on an already published and partly adapted GIS dataset (Fischer et al., 2019).
TABLE 2. Main historical sources and contemporary datasets used for the reconstruction of the historical land cover in the Jamtal (*reconstructed time step; dates in brackets: year of publication).
In agreement with the information shown by the historical sources, we defined 11 land cover classes (Table 3). Settlements including roads and arable land were/are mostly located close to the village Galtür at the valley mouth. The largest areas in the Jamtal refer to grassland (mainly Alpine pastures), sparsely wooded area (krumholz), wasteland (unvegetated moraines, talus fans, exposed bedrock) and former glaciers. Though the orthophotos would allow more accurate classifications and analyses, we applied the same method for all five reconstructed time steps. This helps to guarantee the same level of detail in the resulting GIS datasets.
Each historical map was georeferenced with ESRI ArcGIS 10.6 based on current orthophotos and the DEM. Georeferencing was conducted based on 150–300 reference points per sheet of map. In order to apply topographical landmarks that most probably have not changed in the last 200 years, mountain peaks and ridges that enframe the whole Jamtal were selected. In addition, several smaller ridges and peaks that dissect the valley into smaller subbasins also functioned as reference points. Besides the Alpine refuge Jamtalhütte (built in 1882), several bedrock-confined sections of the Jambach and of the tributary Futschölbach also provided useful landmarks for georeferencing the maps.
After georeferencing, the manual vectorization of the land cover at the five selected time steps followed the “regressive-iterative reconstruction method” described in Hohensinner et al. (2013a). Starting from the best known state in 2015, those in 1970, 1921, 1870 and 1820 were reconstructed step-by-step backwards in time to the least known situation in 1820. This chronological approach enables a significantly more accurate reconstruction of former landscape features compared to the analysis of the state at a single point in time. When one of the time steps was completed (e.g., 1921), the next older situation (1870) was elaborated based on the completed one (1921). This means that every structure (GIS feature) of the 1921-landscape was checked to determine whether it either remained unchanged, changed its appearance or vanished. If any change was detected, the next question was whether it could derive from natural processes, human interventions or points to incorrect mapping. According to these considerations, the specific structure (GIS feature) was modified for the situation in 1870. When the reconstruction of the respective time step was completed, any information on the geographical structures was reviewed to determine the extent to which it affects the interpretation of the structures in the more recent time steps. An important aspect here was whether new conclusions on the states of the landscape in the younger time steps can be drawn and corrections have to be made. In most cases this involved revising not only the next younger situation (1921), but also the proximate ones (=> 1970 => 2015). Finally, when the corrections in all relevant time steps were made, the reconstruction of the next older step (here: 1820) was started. This regressive-iterative approach calls for a permanent critical revision of the already processed time steps and finally ends when the whole time series is reconstructed.
Based on the GIS reconstructions, we analyse changes in historical land cover for the entire Jamtal in three different altitudinal belts (see Study Area Jamtal Valley) for 1820 and 2015. For the Upper Jamtal (subbasin of the Jamtalferner) we analyse the changes in more detail between each of the five time steps (Figure 1). The deglaciated area between the maximum extent of the Jamtalferner in 1864 and in 2015 deserves a closer look. Here, we investigate the biogeomorphic development of the fluvial corridors. For that effort, we merge all running waters that existed between 1820 and 2015, buffered laterally by 50 m, and trace the transformation of the land cover. In addition, we comparatively analyse the reconstructed land cover for the period 1970–2015 with the “Normalized Difference Vegetation Index” (NDVI) that was calculated based on remote-sensing data between 1985 and 2016 (Fischer et al., 2019).
The catchment-wide comparison of the land cover in the entire Jamtal between 1820 and 2015 provides the first input in determining the large-scale alteration of the physical conditions. That first view (Figure 2) illustrates the drastic decline of the glaciated areas within almost 200 years and the significant increase in unvegetated areas (wasteland). It also highlights the upward shift of Alpine grassland. This is also reflected by the transformation analysis in Figure 3 (top). It reveals a decline of the glaciated areas by 73%. In contrast, wasteland increased considerably (37%). The expansion of the latter would have been even greater, but parts of the former unvegetated areas were colonized by plants, leading to a 22% increase of Alpine grassland.
FIGURE 2. Reconstructed land cover patterns in the Jamtal in 1820 and 2015 (width of running waters is magnified).
FIGURE 3. Land cover transformation in the entire Jamtal between 1820 and 2015 (top) and for different altitudinal belts in the same period (bottom). Percent values at right margins: relative changes up to 2015 compared to initial situation in 1820. Example bottom left: At altitudes between 1,550 and 2,100 m a.s.l. a great proportion of wasteland (WL) transformed to grassland (GR) and some smaller proportions to sparsely wooded areas (SP) and forests (FO). Only land cover types with a significant proportion are labelled in the bottom plots (SE, settlement; RW, running water; FS, fluvial sediment; SW, stagnant water; WE, wetland; AL, arable land; GL, glacier).
More closely examining the three separately analysed altitudinal zones yields further insights. The zone below 2,100 m a.s.l. that was favourable for various types of human use (compare Study Area Jamtal Valley) experienced a massive decline of wasteland by 62%. It mainly transformed to grassland, sparsely wooded areas and to a minor extent to forests (Figure 3 bottom left). The latter increased by an outstanding 323%—overtopped only by the expansion of settlement areas (+774%). Grassland decreased markedly and was replaced by sparsely or densely wooded areas (forests). Beyond the wasteland loss, unvegetated fluvial sediment areas and running waters also declined by 60 and 27%, respectively. Fluvial sediments of the Jambach and—even more so—of several tributaries at the valley slopes were consolidated partly by natural colonization processes but also by torrent control measures and other human interventions. The data reflect a general trend of amplified human land reclamation on the one hand and the abandonment of more intensive forms of land use at unfavourable sites on the other.
Glacier shrinkage is most evident in the middle altitudinal belt, whose upper boundary is defined by the vegetation limit (Figure 3 centre. They lost 82% of their former extent in 1820 and were largely replaced by wasteland (+35%). The increase of Alpine grassland by 47% reflects the colonization of former debris areas and the upward shift of the vegetation limit. Starting from a small coverage, sparsely wooded areas (krumholz) expanded by a considerable 248%. A 57% glacier decline is also evident in the highest elevation zone (Figure 3 right). In 2015, formerly glaciated areas were represented by unvegetated debris. Alpine grassland was restricted to smaller patches and showed a comparably negligible contribution.
Tracing the development of the Jamtalferner subbasin for each time period between the reconstructed time steps yields more detailed insights into the evolution of this glacially shaped landscape. Figure 4 illustrates the comprehensive changes in land cover over 195 years. The reconstruction of the situation in 1820 enables tracing the last decades of glacier growth. Until 1870, glacier coverage increased from 64 to 70 percent cover (p.c.) related to the extent of the subbasin (Figure 5 bottom left). Associated with the advancing glacier, unvegetated wasteland diminished by 5 p.c. The data suggest that grassland had remained stable at 4% of the study area. Glacier growth, however, might have had wiped out some patches of Alpine grassland, which is not reflected in the historical sources. The reconstruction for 1870 is very close to the maximum extent of the Jamtalferner in the LIA, which occurred in the Jamtal in 1864 (Fischer et al., 2019). Accordingly, after 1870 up to 1921, glacier extents decreased by 14 p.c., while wasteland expanded by only 7 p.c. (Figure 5 bottom). This is due to the colonization by vegetation that led to a doubling of Alpine grassland from 4 to 8 p.c. Minor shares of formerly ice-covered areas were replaced by fluvial sediments, i.e. sander and sediment bars along the upwards extending meltwater streams. To a minor extent, deglaciation and expansion of vegetation are also evident for the period between 1921 and 1970. Up to 2015 these processes accelerated. Though the period 1970–2015 spans only 45 years, ice-covered areas decreased by 18 p.c., wasteland expanded by 14 p.c. and grassland by 4 p.c. In 2015, for the first time, krumholz reached a notable area in the subbasin.
FIGURE 4. Reconstructed land cover patterns in the Upper Jamtal (Jamtalferner subbasin: Jamtalferner glacier and subjacent valley floor) between 1820 and 2015 (width of running waters is magnified).
FIGURE 5. Land cover transformation in the Jamtalferner subbasin between 1820 and 2015 (top) and for separate time periods (bottom). Top: percent values on right margin refer to relative changes from 1820 to 2015. Bottom: percent values refer to percent cover (p.c.) of the Jamtalferner subbasin. Example bottom right: In 1970, 40% of the subbasin featured wasteland (WL, black/grey). Thereof, until 2015 a marked proportion transformed to grassland (GR, green), while large formerly glaciated areas (GL, blue) developed to wasteland (WL). Finally, in 2015, wasteland encompassed 54% of the entire subbasin. Only land cover types with a significant proportion are labelled (RW, running water; FS, fluvial sediment; SP, sparsely wooded).
Over the long term, since 1820, glaciers declined by 55%, which went hand in hand with an outstanding increase of wasteland by +82% (Figure 5 top). Fluvially shaped sediment areas enlarged by 126%, with even larger increases in Alpine grassland (196%) and krumholz (304%).
The evolution of the fluvial corridors in the area that had been exposed by the retreating glacier between the maximum glacier extent in 1864 and 2015 (proglacial zone) merits a closer look. We therefore analyse all the water courses present in that area between 1820 and 2015, including a lateral buffer of 50 m. Glacier advance after 1820 not only wiped out parts of the main Jambach stream but also several unvegetated sediment bars (compare 1820 and 1870 in Figures 4, 6 bottom left). Wasteland, i.e., areas covered with glacial debris, almost vanished. Until 1870, 97% of the fluvial corridors were gradually covered with ice. This state can be interpreted as a “reset” of the fluvial system in the analysed proglacial zone.
FIGURE 6. Land cover transformation of the fluvial corridors 1820–2015 in the proglacial area that deglaciated between the maximum extents of the glaciers in 1864 and in 2015 (compare Figure 1). Fluvial corridors refer to all running waters that existed in that area between 1820 and 2015 including lateral buffers of 50 m. Top: percent values on right margin refer to relative changes from 1820 to 2015. Bottom: percent values refer to percent cover (p.c.) of the fluvial corridors. Example bottom middle: In 1921, 12% of the fluvial corridors featured fluvial sediments (FS, orange). Thereof, until 1970 a marked proportion transformed to wasteland (WL, black/grey). In turn, smaller proportions of former glaciers (GL, blue) and wasteland (WL) developed to fluvial sediment areas (FS). In 1970, fluvial sediments made up 14% of the analysed fluvial corridors. Only land cover types with a significant proportion are labelled (RW, running water; GR, grassland; SP, sparsely wooded).
The drastic decline of the glaciers between 1870 and 1921 is reflected by the enlargement of exposed debris and rock areas (wasteland) to 23 p.c. of the overall fluvial corridor extent. Areas covered by fluvial sediments, primarily outwash plains (sander), significantly increased to 12 p.c. and running waters to ca. 1 p.c. Vegetated areas, almost inexistent in 1870, within 51 years already covered 4 p.c. of the corridors. Ongoing deglaciation between 1921 and 1970 exposed new debris areas, which enlarged to a total of 40 p.c. (Figure 6 bottom). Fluvial sediments expanded only slightly, although some formerly glaciated areas transformed to outwash plains. This is because other types of unconsolidated debris (wasteland) replaced some older fluvially shaped areas. One potential explanation is that the terrain becomes steeper as the glacier retreats towards higher altitudes. Here, debris from the valley sides and lateral moraines may have buried older fluvial landforms. Nevertheless, running waters approximately doubled and vegetated sites expanded by 2 p.c. up to 1970.
Finally, until 2015 almost all of the formerly ice-covered sites transformed to wasteland or fluvial sediment areas (outwash plains). Wasteland then constituted the dominant type of land cover (59 p.c.). Due to the upwards lengthening of the fluvial system the running waters doubled once more. Alpine vegetation significantly profited from the changing physical conditions and expanded from 6 p.c. in 1970 to 20 p.c. in 2015.
Over the long term, the entire system of fluvial corridors that had evolved between 1820 and 2015 from ice-covered areas is characterized by a 491% expansion of running waters; fluvially shaped areas grew by 605%. Moreover, vegetated sites—originally almost inexistent—expanded by almost 12,000% (Figure 6 top).
As noted above, terrain slope might play a role in the evolution of the fluvial corridors. Figure 7 displays certain forms of land cover transformation in relation to terrain slope after the maximum glacier expansion between 1870 and 2015. It shows that fluvial sediments such as outwash plains predominantly replaced formerly glaciated areas in flatter terrain with a median slope of only 11° (GL-FS in Figure 7). Alpine grassland could develop at steeper, but not too steep formerly ice-covered sites (GL-GR, median slope = 17°), and glaciers in steeper terrain were replaced largely by unvegetated wasteland (GL-WL).
FIGURE 7. Terrain slope versus land cover transformation of the fluvial corridors 1870–2015 in the proglacial area that deglaciated between 1864 and 2015 (for abbreviations see Table 3; compare Figure 1; n = number of 5 m raster cells).
Fluvially shaped areas remained stable over the long term in very flat terrain (FS-FS, median slope = 4°). In steeper terrain with a median slope of 13° such sites underwent colonization by vegetation (FS-GR). In much steeper terrain, however, most were soon covered by debris from lateral moraines or from the valley sides or were washed out, exposing the bedrock (FS-WL, median slope = 19°). In turn, wasteland that was colonized by vegetation showed a median slope of 30° (WL-GR). Slightly steeper wasteland often remained devoid of vegetation (WL-WL). Because the latter two cases show very similar distributions, vegetation coverage of former wasteland might also be affected by factors other than those analysed in the present study.
Historical data sources, i.e., maps and plans, display the physical environment in a generalized manner using discrete symbology classes for different types of landforms. They therefore differ fundamentally from present datasets such as optical satellite or orthophotos that render the complex nature in radiometric measurements at several discrete spectral bands. Beyond potential inaccuracies in the qualitative information shown by historical data, errors in geographical position are also main sources of potential misinterpretations. This applies in particular to the historical maps prior to 1900 CE. The map series of the “Franciscean Cadastre” and the “Military Land Surveys” were produced by military cartographers working with very elaborate survey instructions (Fuhrmann, 2007). Nevertheless, more intensively human-used areas in the valleys were mapped very accurately, whereas those in higher altitudes at the transition to the wasteland or glaciers lack such accuracy. According to Ulbrich (1961), in the cadastre the mapped distances of 100 m showed average errors in length of 1.3 and 4.9 m at distances around 2,000 m. The assumption is that the “Military Land Surveys” show significantly greater errors of geographical position. The “regressive-iterative reconstruction method” applied in the present study partly helps to adjust potential errors in location (Hohensinner et al., 2013a). In addition, the use of 150–300 reference points (mountain peaks, ridges, refuge hut etc.) per sheet of map also helps to mitigate potential inaccuracies. For example, the extents of the glacier shown by the “Third Military Survey” 1870–1872 matches well with the terminal and lateral moraines of the LIA maximum in 1864 that are still visible in current orthophotos and in the DEM. This justified resorting to an already published GIS dataset (Fischer et al., 2019) in reconstructing the extents of the main glacier Jamtalferner between 1870 and 2015.
Undoubtedly, the reconstruction of the land cover in 1820 based on the “Second Military Survey” 1816–1821 entails the greatest inaccuracies. In the lower section of the Jamtal the cadastre maps helped to rectify potential imprecisions. In higher terrain, however, the cadastre partially also lacks information. Moreover, the glacier advance until 1864 wiped out older landscape features such as moraines. Unfortunately, no other accurately surveyed map is available to more profoundly validate the quality of the maps from around 1820. A sensitivity analysis performed by applying varying reference points in the georeferencing process indicates a potential position error of the glacier terminus of ±70 m. Accordingly, the Jamtalferner would have had advanced by ca. 440 ± 70 m between 1820 and 1870. Historical surveys of the Danube River in Vienna that were discovered after termination of the GIS reconstructions enabled an unintentional validation of the compiled dataset. It showed that the “regressive-iterative” approach yielded robust results: certain details of the landscape reconstruction had to be revised but did not significantly change the overall result (Hohensinner et al., 2013b).
Alpine grassland or shrubs tend to fade out as the terrain gets higher, which is reflected by the orthophotos from 1970 to 2015. Smaller vegetation patches in higher terrain, however, are usually not accurately displayed by historical maps. In order to obtain comparable data for all analysed time steps, we generally omitted such smaller patches in the reconstructions based on orthophotos. Thus, vascular plants may have reached higher altitudes than suggested by the data of the present study (Lamprecht et al., 2018; Rogora et al., 2018). Another point of interest is the differentiation between wasteland (moraines, talus fans, rocks etc.) and fluvial sediments. Fortunately, larger patches of the latter are commonly separately depicted in the historical maps.
Climate change affects the physical framework conditions of Alpine environments in manifold and complex ways. Beyond glacier decline it alters the erosion processes, the sediment supply and the flow/flood regime of running waters along with the spatial distribution of plant species (Bronstert et al., 2002; Leonelli et al., 2011; Blöschl et al., 2017; Blöschl et al., 2018; Comiti et al., 2019). In lower Alpine areas that are more favourable for agricultural uses, humans modify the Alpine environment even more intensively (Bätzing, 2003; Andric et al., 2010). In many cases it is not possible to clearly distinguish between the consequences of human agency and of climate change (Cannone et al., 2007). For example, humans have significantly modified Alpine vegetation by lowering the treeline due to livestock farming and wood extraction over millennia (Ellenberg, 1996; Dietre et al., 2014). In the 20th century, farmland was abandoned in many unfavourable sites that are too steep, too high or too remote to cultivate economically (Tappeiner et al., 2006; Tasser et al., 2009; Mayer and Erschbamer, 2017). After such abandonment the Alpine vegetation has the potential to recover and a gradual upward shift of the treeline is expected (Carnelli et al., 2004; Gehrig-Fasel et al., 2007; Remy, 2012). Although global warming may boost the upward shift of the vegetation belt, in lower altitudes such vegetation dynamics might primarily reflect human interferences rather than climate change (Leibundgut and Kohn, 2014).
According to Reitmaier et al. (2013) the Silvretta mountain range, in which the Jamtal is located, was also subject to human land uses over millennia. The land cover changes identified for the Jamtal below 2,100 m a.s.l. should therefore be interpreted in this context. In 1774, Alpine forests covered significantly larger areas around Galtür compared to the mid-19th century (Fromme, 1957, based on “Wälderausschätzungsprotokoll” of the saltworks in Hall; Provincial Archive Tyrol, Cod. 3693). According to the map “Atlas Tyrolensis” created by Peter Anich also in 1774, Fromme (1957) argued that forested areas probably reached altitudes up to the Jambach tributary “Futschölbach” at approximately 2,200 m a.s.l. (see Figure 1). However, given the accuracy of the “Atlas Tyrolensis”, this finding must be critically assessed. Our reconstruction of the land cover in 1820 does not reflect the occurrence of larger wooded areas in such a high altitude (Figure 2). Besides potential inaccuracies in the 1774 atlas and also in our reconstruction, two processes—natural and socio-economic—probably resulted in a significant decline of wooded areas until the mid-19th century. Between 1810 and 1820, climate models indicate the lowest mean annual temperatures for the study area within the overall time period 1780–2013 (Chimani et al., 2013). In the following decades until 1864, the Jamtalferner glacier significantly expanded down toward the valley (Figure 2). Harsher climatic conditions and glacier growth probably physiologically weakened the woody vegetation at higher altitudes.
Beyond the changes of the physical environment, the socio-economic framework conditions have also changed. Population growth and the associated divided inheritance of land gradually diminished peasant land property (Böhm, 1970). Consequently, in the valleys around Galtür, land uses such as woodland pasture, extraction of leaf fodder and cattle husbandry in Alpine pastures had been intensified and led to large-scale devastation of Alpine forests and pastures. According to Böhm (1970), the all-embracing exploitation of the Alpine resources culminated in the mid-19th century, going hand in hand with amplified soil denudation, frequent debris flows and avalanches. Then, mountain farmers were forced to change their land use practices, to abandon subsistence economy or to migrate and search for other forms of livelihood. Lorenz (1945-1974) reported that the cultivation of hay in the mountains had significantly declined and had been substituted by meadows closer to the village since 1850. Summerly cattle husbandry in the Jamtal also dropped by 69% and some Alpine pastures had been abandoned by 1952 (Fromme, 1957).
The reconstructions of the land cover in 1820 and 1870 reflect the reported state of overexploitation in times of adverse climate and glacier growth (Figures 2, 4). The extraordinary increase of forests by 323% (mostly former grassland and krumholz) between 1820 and 2015 points to at least partially planned afforestation after some areas had been taken out of operation (Figure 3 bottom left). Afforestation plans, amongst others for the Jamtal, were already published by Fromme (1957) in order to reduce damage by avalanches and torrents. Rockslides and debris flows repeatedly buried meadows and pastures (Vogt, 1993). Maps from the 19th century show large alluvial fans (fluvial sediments) along several tributaries in the valley sides that largely vanished and were replaced by grassland or krumholz by the late 20th century. This points to efforts to stabilize the alluvial fans. Böhm (1970) reported afforestation measures for the valley sides around Galtür. According to the municipality of Galtür, historically peasants have meliorated such areas by applying humus and manure. Moreover, the lower section of the Jambach has been significantly human modified by repeated channel straightening and narrowing, partial bank stabilization and water abstraction.
Despite the numerous forms of human interventions, climate change probably also played a role in altitudes up to 2,100 m a.s.l. because larger proportions of wasteland have transformed to grassland or krumholz (Figure 3 bottom left). Such colonization processes are even more evident in altitudes up to 2,800 m a.s.l. Nevertheless, above 2,100 m a.s.l., the predominating process was the transition from formerly glaciated areas to wasteland. The closer analysis shows an annual 0.11 p.c. glacier growth related to the area of the Jamtalferner subbasin between 1820 and 1870 (roughly corresponding to the LIA maximum in 1864; Figure 5). This corresponds to the final decades of a long period of glacier growth that had already started in late Medieval Times (Böhm, 1970). Until the early 18th century, the Jamtalferner could still be crossed with horses. After 1870, the annual rate of areal glacier losses was 0.26 p.c. related to the subbasin area, followed by a phase of minor losses of 0.19 p.c. between 1921 and 1970. In contrast, the final period (1970–2015) featured extraordinary 0.40 p.c. annual losses. These results reflect the general trend of accelerating warming in the recent decades, but do not necessarily correlate with measured or gridded mean annual/summer/winter temperatures and precipitation sums before 1970 (Chimani et al., 2011; Chimani et al., 2013) due to glacier response times and adjustments in glacier dynamics (Jóhannesson et al., 1989; Zekollari et al., 2020).
Besides glacier retreat, the most obvious process of land cover change is the expansion of Alpine grassland and sparsely wooded areas (krumholz) into wasteland in higher altitudes. After the LIA maximum, the extent of grassland doubled from 4 to 8 p.c. of the Jamtalferner subbasin until 1921 (Figure 5 bottom). In 2015, that extent already reached 13 p.c., and the value of sparsely wooded areas reached 1 p.c. Within 145 years, this expansion was accompanied by a significant upward shift of the uppermost vegetated site by 460 m from 2,320 m a.s.l. in 1870 to 2,780 m a.s.l. in 2015 (excluding some smaller high-altitude vegetation patches in 2015 that probably would not have been mapped in historical surveys). For comparison, in the Italian Alps the treeline shifted by 115 m between 1900 and 2000 (Leonelli et al., 2011). In the Swiss Alps, vascular plants expanded upwards on average by 278 m within one century (Walther et al., 2005). These values seem to be rather low compared to 460 m close to the Jamtalferner. For the Swiss Alps, however, the proportion of actually upward-shifting woody vegetation is greater in higher altitudes, while forest ingrowth in (abandoned) grassland prevails in lower altitudes (Gehrig-Fasel et al., 2007). Within only 12 years a median upward shift by 28 m was recorded below 2,350 m a.s.l., and a few vegetation patches in higher terrain shifted by a median of 50 m. Indeed, the reconstructions for the entire Jamtal in 1820 and 2015 yield a smaller upward shift of the uppermost vegetation limit compared to the formerly largely glaciated subbasin of the Jamtalferner. In the entire valley it amounted to only 243 m (maximum 307 m when taking smaller high-altitude vegetation patches into account in 2015).
Fischer et al. (2019) calculated the density of ground cover using the “Normalized Difference Vegetation Index” (NDVI; Rouse et al., 1974). In the proglacial zone of the Jamtalferner, the overall NDVI increased with the time of exposure from a mean of 0.11 in 1985 to 0.27 in 2016. Combining the reconstructed dataset for 1970–2015 with the NDVI 1985–2016 shows that almost all the areas that had remained grassland between 1970 and 2015 feature an increasing NDVI (see GR-GR in Figure 8 bottom). This means that the ground cover in such areas had increased (= vegetation ingrowth). Interestingly, sites that had developed from unvegetated fluvial sediments or wasteland to grassland (FS-GR and WL-GR) started with a higher NDVI in 1985 than unvegetated sites that had remained devoid of vegetation (FS-FS and WL-WL, Figure 8 top). This suggests that the transformation to grassland must have already started prior to 1985. Moreover, ground cover in such newly colonized areas increased more than at sites that remained grassland between 1970 and 2015 (Figure 8 bottom). Areas that were identified as fluvial sediments in both 1970 and 2015 did not exhibit any significant increase in ground cover (Fs-FS, bottom). Persistent wasteland, however, shows a slight increase (WL-WL, bottom). Finally, glaciated sites that had developed to fluvially shaped areas between 1970 and 2015 (GL-FS) show a stronger increase in ground cover than those that had turned to wasteland (GL-WL, bottom).
FIGURE 8. “Normalized Difference Vegetation Index” (NDVI) calculated for the proglacial zone of the Jamtalferner in 1985 and 2016 based on Fischer et al. (2019). Bottom graph: changes (difference) of the NDVI between 1985 and 2016. X-axes: raster cell transformations from land cover type in 1970 to type in 2015 (for abbreviations see Table 3; compare study area in Figure 1; n = number of 5 m raster cells).
The analyses of the historical land cover changes both in the entire Jamtal and in the subbasin of the Jamtalferner reveal that the “physical” factors that control channel-forming processes have markedly changed within almost 200 years. In the lower section of the valley, primarily direct and indirect forms of human interventions (water deviation, partial channel straightening, stabilization of unconsolidated debris/sediments by vegetation) modified the basic conditions for channel evolution (reduced fluvio-dynamics and bedload supply). The increasing human pressure on the lower sections of Alpine rivers has been recently addressed for the entire Alpine region by Hohensinner et al. (2021). In higher altitudes, however, climate change has led (and still leads) to the modification of the “landscape template” in which certain types of running waters may evolve (compare Introduction; Roni and Beechie, 2013). The “landscape template” (or “physical template”) defined by geology, tectonics and climate constrains the potential range of channel evolution and riverine habitats and, consequently, the thereof dependent ecological processes (Brierley and Fryirs, 2005; Allen and Castillo, 2007). Commonly, the physical framework conditions only change over the very long-term. Extreme floods in alluvial landscapes or volcanic eruptions, for example, may alter “landscape templates” abruptly. Likewise, climate change alters the ice coverage and terrain topography of glacial headwater basins also over the short term. Glacier decline has extended Alpine river systems upwards and has exposed large areas covered by unconsolidated debris. Thus, the physical structure and basic conditions of the upper Jambach basin have comprehensively changed in respect of catchment configuration, topography, river length and sediment supply. In such locations, new fluvial systems arise almost from “scratch”, adopting the newly available space along with its glacio-fluvial processes as a “template” for channel evolution.
For fluvial systems, the most obvious consequence of climate change, i.e., of glacier decline, is the gradual lengthening of the river courses. After the maximum extent of the Jamtalferner in 1864, the meltwater stream rapidly extended its course upwards between 1870 and 1921 by ca. 26 m per year (measured along the main arm to the terminus of the glacier tongue; Figure 4). Until 1970 the annual lengthening was only 12.5 m, followed by a phase of slightly accelerating lengthening by 16 m per year until 2015. In the recent years until 2019, annual rates once more increased to 22.5 m. In total, since 1870, the main arm has extended upwards by approximately 2,740 m (ca. 19 m/year), traversing an altitude of 370 m. Between 1921 and 1970 the glacier receded to a higher terrain level and the new water course had to traverse an escarpment of exposed bedrock. That might help explain the slower lengthening rates between 1921 and 1970. The recent accelerated channel lengthening is well reflected by surveys of the annual geodetic mass loss of the glaciers in the Silvretta mountain range, where the study site is located. Between 1969 and 2002 the glaciers annually lost 0.2 ± 0.1 m water equivalent (w.e.). The value peaked between 2002 and 2004/06 at 1.5 ± 0.7 m w.e./year. Since then until 2017/18, the mean mass loss amounted to 0.8 ± 0.1 m w.e./year (Fischer et al., submitted). The Jamtalferner in particular lost on average 0.6 m w.e./year between 1988/89 and 2001/02. Up to 2020 this has accelerated to 1.3 m w.e./year (Fischer et al., 2016b).
Today, the fluvial system in the Jambachferner subbasin features three alluvial braided sections. Longitudinally, these are separated by two sections that are confined by bedrock or by lateral moraines. The upper braided section flows through the current sander (outwash-plain) directly below the glacier terminus. Further glacier disintegration can be expected at Jamtalferner because the main glacier stream is already fed by two branches in the glacier foreland. The harsh physical conditions at such high altitudes, with high meltwater discharges and extreme precipitation events in summer, are reflected by a highly dynamic evolution of the new channel system (Lane et al., 2017; Carrivick and Heckmann, 2017). The dominant geomorphic processes include sudden increases in sediment transport rates, debris flows from lateral moraines or valley sides on the one hand, and large volumes of debris deposited in proglacial areas on the other hand (Baewert and Morche, 2014; Comiti et al., 2019). Applying the “fluvial biogeomorphic succession concept” according to Corenblit et al. (2007, 2015), the newly emerged meltwater streams of the Jambach river system have largely remained in the “geomorphic phase” in which flow and sediment fluxes are the dominant channel controls. Despite the extraordinary, dynamic nature of such environments, our data show that Alpine plants gradually colonized glacial debris or (glacio-)fluvial sediments. We traced the active channels (water surfaces and unvegetated fluvial sediments) in the fluvial corridors that had evolved in former glaciated areas between 1870 and 1921. This revealed that Alpine vegetation (grassland or krumholz) played only a very minor role in the first 49–100 years (depending on the point in time when the respective part of the active channel originated between 1870 and 1921; Figure 9 left). Until 1970, only 1% of the newly emerged active channel area had undergone terrestrialization and developed to grassland. The largest proportion (76%) remained an active channel, while 23% re-transformed to wasteland, possibly due to debris flows from adjacent higher terrain. Gully erosion in steep lateral moraines and re-deposition of the mobilized material at slope foots are typical paraglacial processes in former glaciated areas (Eichel et al., 2018). Thus, fluvial channels and sediment bars are prone to be buried by re-deposited debris. Forty-five years later, by 2015, approximately half of the original active channel area still remained and 7% were covered by debris (wasteland). Over time, colonization by vegetation accelerated, and, between 1970 and 2015, 21% of the active channel had already become grassland. This reflects the “pioneer phase” according to Corenblit et al. (2015) in which larger parts of the former channel are already vegetated, but the plants cannot yet significantly influence the channel-forming processes or the channel pattern. Finally, after 145 years, 30% of the original active channel were covered by grass or herbaceous plants and only 1% featured some kind of woody vegetation. This represents the onset of the “biogeomorphic phase” in which woody vegetation can significantly influence bank resistance, and, consequently, channel geometry.
FIGURE 9. Biogeomorphic trajectories of the fluvial corridors in the proglacial area (deglaciated area between 1864 and 2015): glaciated areas and wasteland that developed into active channels between 1870 and 2015 (left) and those that started later in/after 1921 (right). Area proportions (percent cover) < 0.1% are not labelled (act. channel = active channel (water surface + unvegetated fluvial sediments), sparsely w. = sparsely wooded (krumholz); blue arrows: changes as part of the geomorphic phase of channel evolution; green arrows: pioneer/biogeomorphic phase; grey arrows: terrestrial processes).
Channel dynamics and colonization by plants may vary substantially spatially and temporally. Of the active channels that emerged later, i.e., between 1921 and 1970, more than the half re-transformed to wasteland by 2015 (Figure 9 right). Only 0.3% of such channels were colonized by grass or herbs (compared to 1% in the section that arose already between 1870 and 1921; Figure 9 left). The channel section that emerged between 1921 and 1970 is located in higher terrain than in the first 50 years after the glacier began to recede. Here, the physical conditions, the “landscape template”, differs from that earlier developed section. The terrain topography is generally steeper due to nearby exposed bedrock and lateral moraines. This probably explains the high proportion of former active channel areas that transformed to wasteland between 1970 and 2015 (56.7% in Figure 9). Figure 7 shows that fluvial areas in steeper terrain (median slope: 19°) were prone to be buried by unconsolidated debris (see FS-WL). In comparison, Eichel et al. (2018), who analyzed paraglacial processes at lateral moraines, reported lower geomorphic activity and initial colonization by “Alpine ecosystem engineering species” already at slope gradients of 19°. Our data on the slope-dependent land cover transformation, however, refer to the fluvial corridors, i.e., active channels and directly adjacent landforms (see Land Cover Classification and GIS Reconstruction). Here, both fluvial and paraglacial processes interfere and potentially amplify morphodynamic disturbances. In contrast, 50% of the unvegetated areas (wasteland) untouched by fluvial dynamics transformed to grassland even at slope gradients up to 30° (Figure 7, WL-GR). According to Eichel et al. (2018), gullying processes and landforms require gradients above 30°, which conforms well with the present findings.
Recent optical remote sensing data from 2019 covering a part of the proglacial zone reveal that the deglaciation process is much faster than the colonization process by Alpine vegetation. Accelerating glacier decline is reflected by increasing proportions of wasteland compared to other forms of land cover (see Figures 5, 6 bottom right). Consequently, growing areas of unconsolidated debris increasingly characterize the headwater sections of (formerly) glaciated basins. The results show that climate change, i.e., glacier retreat, not only affects the hydrological regime of Alpine fluvial systems, but also boosts the sediment supply and bedload transport (Blöschl et al., 2007; Baewert and Morche, 2014; Marren and Toomath, 2014). Such glacio-fluvial and paraglacial transformation processes of the physical environment can be expected so far for most glaciers in the European Alps and other glaciated mountain regions on earth under recent climate scenarios (Curry et al., 2006; Hock et al., 2019; Zekollari et al., 2019; Zekollari et al., 2020).
The combination of high-resolution remote sensing data such as orthophotos and optical satellite images (1970–2016) and less detailed historical maps (1820–1921) enabled the analysis of a longer time series on land cover changes in the past. It also yielded new interpretations of the “Normalized Difference Vegetation Index” (NDVI) based on optical remote sensing data (Figure 8). The reconstruction of the long-term land cover changes in the Tyrolean Jamtal valley (main glacier Jamtalferner) reveals the “hybrid” nature of the Alpine environment, governed by both direct and indirect human modifications as well as natural processes. The main findings of the compiled datasets, with a special focus on the primarily glacially controlled fluvial system in the Jamtal between 1820 and 2015/19, are:
1) Below an altitude of 2,100 m a.s.l. the Jamtal experienced a massive decline of wasteland (unvegetated debris and outcropping rocks) that had mainly transformed to grassland and sparsely wooded areas. Forests significantly expanded, while grassland decreased markedly, becoming sparsely or densely wooded areas (forests). Running waters and fluvial sediment areas experienced major areal losses due to channel straightening and land reclamation. Although climate change also affects the vegetation in the lower section of the valley, the data primarily reflect the long-term practices and legacy effects of human land uses in Alpine environments. After the overexploitation in the Jamtal had peaked in the mid-19th century, the mountain farmers were forced to abandon their practices. Since then a general trend of amplified land reclamation in favourable areas closer to settlements on the one hand and the abandonment of more intensive forms of land uses in unfavourable, remote sites on the other hand is evident. In this respect, the Jamtal represents a prototype of a “socio-ecological system” as conceptualized in Long-Term Socio-Ecological Research (LTSER). Such systems operate on short and long time scales and are shaped by a multiplicity of legacies—social, ecological and institutional (Haberl et al., 2006; Singh et al., 2012).
In the higher subbasin of the Jamtalferner, climate change more directly influences the land cover changes. Between 1820 and 2015, glaciated areas declined by 55%, associated with a tremendous growth of exposed wasteland (Figure 5 top). Fluvially shaped sediment areas enlarged by 126%. In parallel, Alpine grassland expanded by 196% and krumholz by even 304%. A detailed analysis of the fluvial corridors in the proglacial zone (area that deglaciated after the LIA maximum in 1864) reveals that the physical structure of the subbasin, the “landscape template” that defines the basic conditions for the evolution of certain types of channel patterns, has undergone an all-embracing alteration. New fluvial systems have emerged that extend upwards, thereby following the receding glacier tongue.
2) Since the maximum extent of the glacier in 1864/70, up until 2019 the main stream of the river system has extended upwards by approximately 2,740 m (ca. 19 m/year), traversing an altitude of 370 m. Today, the newly emerged river section shows three bar-braided sections in outwash plains (sander) separated by laterally confined river sections. High meltwater discharges and extreme precipitation events in summer as well as sufficient sediment supply in the foreland of the glacier (proglacial area) primarily control the new, highly dynamic channel system. Approximately half of the new channel system that evolved in formerly glaciated areas between 1870 and 1921 still existed in 2015. Unconsolidated debris buried almost one fifth of the new channels and almost one third was colonized by vegetation.
Recent data show that the deglaciation process proceeds much faster than the colonization process by Alpine vegetation. Accordingly, the extent of wasteland (unvegetated debris, rocks) is expanding and potentially amplifying the sediment supply of the fluvial system. Climate change boosts the transformation of the Alpine glacier landscape along with its fluvial systems, both hydrologically and in respect of sediment delivery. New fluvial systems arise in former glaciated areas, which also affects human use in lower, more favourable regions of the Alpine landscape. The long-term investigation of such landscapes reveals that the transformation processes have accelerated in recent decades. Moreover, it highlights the fundamental geomorphological and ecological transformation processes proceeding in higher glaciated catchments under recent climate change scenarios globally.
The historical evolution of the high Alpine terrain topography, i.e. slopes of deglaciated moraines and of the valley bottom, is crucial for understanding potential fluvial sediment supply, debris flow and colonization processes of vegetation. Thus, 3D reconstruction of past topographical conditions based on historical photos and orthophotos by digital mono-photogrammetry would significantly improve long-term research in Alpine environments.
The raw data supporting the conclusions of this article will be made available by the authors, without undue reservation.
SH: Conceptualization, methodology, investigation, validation, writing; UA: Investigation; AF: Investigation, review; GS: Investigation, review; KH: Project funding, project administration, investigation, writing.
This work was funded by the Austrian Academy of Sciences (ÖAW) within the Earth System Sciences (ESS) research program, i.e., the project “Changing debris cover on Eastern Alpine glaciers: quantification and hydrological impacts” (Hidden.Ice). Open access funding was provided by the Austrian Academy of Sciences (ÖAW).
Author GS was employed by the company ENVEO – Environmental Earth Observation IT GmbH.
The remaining authors declare that the research was conducted in the absence of any commercial or financial relationships that could be construed as a potential conflict of interest.
All claims expressed in this article are solely those of the authors and do not necessarily represent those of their affiliated organizations, or those of the publisher, the editors and the reviewers. Any product that may be evaluated in this article, or claim that may be made by its manufacturer, is not guaranteed or endorsed by the publisher.
The authors thank the two reviewers for the considerable time spent to improve the paper and Michael Stachowitsch for professional scientific English proofreading.
Allan, J. D., and Castillo, M. M. (2007). Stream Ecology. Structure and Function of Running Waters. Second Edition. Dordrecht, Netherlands: Springer.
Andric, M., Martincic, A., Stular, B., Petek, F., and Goslar, T. (2010). Land-Use Changes in the Alps (Slovenia) in the Fifteenth, Nineteenth and Twentieth Centuries Ad: A Comparative Study of the Pollen Record and Historical Data. The Holocene 20 (7), 1023–1037. doi:10.1177/0959683610369506
Auer, I., Böhm, R., Jurkovic, A., Lipa, W., Orlik, A., Potzmann, R., et al. (2007). HISTALP-Historical Instrumental Climatological Surface Time Series of the Greater Alpine Region. Int. J. Climatol. 27, 17–46. doi:10.1002/joc.1377
Baewert, H., and Morche, D. (2014). Coarse Sediment Dynamics in a Proglacial Fluvial System (Fagge River, Tyrol). Geomorphology 218, 88–97. doi:10.1016/j.geomorph.2013.10.021
Bätzing, W. (2003). Die Alpen. Geschichte und Zukunft einer europäischen Kulturlandschaft. Munich: Ch. Beck.
Blöschl, G., Ardoin-Bardin, S., Bonell, M., Dorninger, M., Goodrich, D., Gutknecht, D., et al. (2007). At what Scales Do Climate Variability and Land Cover Change Impact on Flooding and Low Flows? Hydrol. Process. 21, 1241–1247. doi:10.1002/hyp.6669
Blöschl, G., Blaschke, A. P., Haslinger, K., Hofstätter, M., Parajka, J., Salinas, J., et al. (2018). Auswirkungen der Klimaänderung auf Österreichs Wasserwirtschaft - ein aktualisierter Statusbericht. Österr Wasser- und Abfallw 70, 462–473. doi:10.1007/s00506-018-0498-0
Blöschl, G., Parajka, J., Blaschke, A. P., Hofstätter, M., Haslinger, K., and Schöner, W. (2017). Klimawandel in der Wasserwirtschaft – Schwerpunkt Hochwasser, Dürre und Trockenheit. Report. Vienna: Federal Ministry of Agriculture, Regions and Tourism.
Böhm, H. (1970). “Das Paznauntal. Die Bodennutzung eines alpinen Tales auf geländeklimatischer, agrarökologischer und sozialgeographischer Grundlage,” in Forschungen zur deutschen Landeskunde. Bonn-Bad Godesberg: Bundesforschungsanstalt für Landeskunde und Raumordnung.
Brierley, G. J., and Fryirs, K. A. (2005). Geomorphology and River Management. Applications of the River Styles Framework. Chichester, UK: John Wiley & Sons.
Bronstert, A., Niehoff, D., and Bürger, G. (2002). Effects of Climate and Land-Use Change on Storm Runoff Generation: Present Knowledge and Modelling Capabilities. Hydrol. Process. 16, 509–529. doi:10.1002/hyp.326
Cannone, N., Sgorbati, S., and Guglielmin, M. (2007). Unexpected Impacts of Climate Change on alpine Vegetation. Front. Ecol. Environ. 5 (7), 360–364. doi:10.1890/1540-9295(2007)5[360:uiocco]2.0.co;2
Carnelli, A. L., Theurillat, J.-P., Thinon, M., Vadi, G., and Talon, B. (2004). Past Uppermost Tree Limit in the Central European Alps (Switzerland) Based on Soil and Soil Charcoal. The Holocene 14 (3), 393–405. doi:10.1191/0959683604hl715rp
Carrivick, J. L., and Heckmann, T. (2017). Short-term Geomorphological Evolution of Proglacial Systems. Geomorphology 287, 3–28. doi:10.1016/j.geomorph.2017.01.037
Chimani, B., Böhm, R., Matulla, C., and Ganekind, M. (2011). Development of a Longterm Dataset of Solid/liquid Precipitation. Adv. Sci. Res. 6, 39–43. doi:10.5194/asr-6-39-2011
Chimani, B., Matulla, C., Böhm, R., and Hofstätter, M. (2013). A New High Resolution Absolute Temperature Grid for the Greater Alpine Region Back to 1780. Int. J. Climatol. 33, 2129–2141. doi:10.1002/joc.3574
Comiti, F., Mao, L., Penna, D., Dell'Agnese, A., Engel, M., Rathburn, S., et al. (2019). Glacier Melt Runoff Controls Bedload Transport in Alpine Catchments. Earth Planet. Sci. Lett. 520, 77–86. doi:10.1016/j.epsl.2019.05.031
Corenblit, D., Baas, A., Balke, T., Bouma, T., Fromard, F., Garófano-Gómez, V., et al. (2015). Engineer Pioneer Plants Respond to and Affect Geomorphic Constraints Similarly Along Water-Terrestrial Interfaces World-Wide. Glob. Ecol. Biogeogr. 24, 1363–1376. doi:10.1111/geb.12373
Corenblit, D., Tabacchi, E., Steiger, J., and Gurnell, A. M. (2007). Reciprocal Interactions and Adjustments Between Fluvial Landforms and Vegetation Dynamics in River Corridors: A Review of Complementary Approaches. Earth-Science Rev. 84 (1-2), 56–86. doi:10.1016/j.earscirev.2007.05.004
Curry, A. M., Cleasby, V., and Zukowskyj, P. (2006). Paraglacial Response of Steep, Sediment-Mantled Slopes to post-'Little Ice Age' Glacier Recession in the Central Swiss Alps. J. Quat. Sci. 21, 211–225. doi:10.1002/jqs.954
Dietre, B., Walser, C., Lambers, K., Reitmaier, T., Hajdas, I., and Haas, J. N. (2014). Palaeoecological Evidence for Mesolithic to Medieval Climatic Change and Anthropogenic Impact on the Alpine flora and Vegetation of the Silvretta Massif (Switzerland/Austria). Quat. Int. 353, 3–16. doi:10.1016/j.quaint.2014.05.001
Dirnböck, T., Bezák, P., Dullinger, S., Haberl, H., Lotze-Campen, H., Mirtl, M., et al. (2012). “Critical Scales for Long-Term Socio-Ecological Biodiversity Research,” in Long Term Socio-Ecological Research: Studies in Society-Nature Interactions Across Spatial and Temporal Scales. Human-Environment Interactions Series (Dordrecht/Heidelberg/New York/London: Springer), Vol. 2, 123–138. doi:10.1007/978-94-007-1177-8_6
Dullinger, S., Dirnböck, T., and Grabherr, G. (2004). Modelling Climate Change-Driven Treeline Shifts: Relative Effects of Temperature Increase, Dispersal and Invasibility. J. Ecol. 92, 241–252. doi:10.1111/j.0022-0477.2004.00872.x
Dullinger, S., Dirnböck, T., and Grabherr, G. (2003). Patterns of Shrub Invasion into High Mountain Grasslands of the Northern Calcareous Alps, Austria. Arctic, Antarctic, Alpine Res. 35, 434–441. doi:10.1657/1523-0430(2003)035[0434:posiih]2.0.co;2
Egger, G., Drescher, A., PrunierGräßer, P. L., Juszczyk, I., Kudrnovsky, H., Blasel, L., et al. (2019a). “Riparian Vegetation - Surviving in an Ever-Changing Environment,” in Rivers of the Alps. Diversity in Nature and Culture. Editors S. Muhar, M. Muhar, G. Egger, and D. Siegrist (Bern: Haupt-Verlag), 182–201.
Eichel, J., Draebing, D., and Meyer, N. (2018). From Active to Stable: Paraglacial Transition of Alpine Lateral Moraine Slopes. Land Degrad. Dev. 29, 4158–4172. doi:10.1002/ldr.3140
Eichel, J. (2019). “Vegetation Succession and Biogeomorphic Interactions in Glacier Forelands,” in Geomorphology of Proglacial Systems. Geography of the Physical Environment. Editors T. Heckmann, and D. Morche (Cham: Springer), 327–349. doi:10.1007/978-3-319-94184-4_19
Ellenberg, H. (1996). Vegetation Mitteleuropas mit den Alpen in ökologischer, dynamischer und historischer Sicht. 5th edition. Stuttgart: Ulmer.
Fischer, A., Fickert, T., Schwaizer, G., Patzelt, G., and Groß, G. (2019). Vegetation Dynamics in Alpine Glacier Forelands Tackled from Space. Sci. Rep. 9 (1), 13918. doi:10.1038/s41598-019-50273-2
Fischer, A., Helfricht, K., Wiesenegger, H., Hartl, L., Seiser, B., and Stocker-Waldhuber, M. (2016a). “What Future for Mountain Glaciers? Insights and Implications from Long-Term Monitoring in the Austrian Alps,” in Mountain Ice and Water: Investigations of the Hydrologic Cycle in Alpine Environments. Developments in Earth Surface Processes. Editors G. B. Greenwood, and J. H. Shroder (Amsterdam: Elsevier), 21, 315–382. doi:10.1016/B978-0-444-63787-1.00009-3
Fischer, A., Markl, G., and Kuhn, M. (2016b). Glacier Mass Balances and Elevation Zones of Jamtalferner. Silvretta, Austria: PANGAEA. doi:10.1594/PANGAEA.818772
Fischer, A., Patzelt, G., Achrainer, M., Groß, G., Lieb, G. K., Kellerer-Pirklbauer, A., et al. (2018). Gletscher im Wandel. 125 Jahre Gletschermessdienst des Alpenvereins. Berlin: Springer Spectrum, 139.
Fischer, A., Seiser, B., Helfricht, K., and Stocker-Waldhuber, M. (2021). (submitted): High-Resolution Inventory to Capture Glacier Disintegration in the Austrian Silvretta. Cryosphere Discuss. preprint. doi:10.5194/tc-2020-376
Fromme, G. (1957). der Waldrückgang im Oberinntal (Tirol). Mitteilungen der forstlichen Bundes-Versuchsanstalt Mariabrunn 54, 5–221.
Fuhrmann, S. (2007). Digitale Historische Geobasisdaten im Bundesamt für Eich- und Vermessungswesen (BEV) – Die Urmappe des Franziszeischen Katasters. Österreichische Zeitschrift für Vermessung und Geoinformation 1, 24–35.
Gehrig-Fasel, J., Guisan, A., and Zimmermann, N. E. (2007). Three Line Shifts in the Swiss Alps: Climate Change or Land Abandonment? J. vegetation Sci. 18, 571–582. doi:10.1111/j.1654-1103.2007.tb02571.x
Gobiet, A., Kotlarski, S., Beniston, M., Heinrich, G., Rajczak, J., and Stoffel, M. (2014). 21st century Climate Change in the European Alps-Aa Review. Sci. Total Environ. 493, 1138–1151. doi:10.1016/j.scitotenv.2013.07.050
Gurnell, A. M., Corenblit, D., García de Jalón, D., González del Tánago, M., Grabowski, R. C., O'Hare, M. T., et al. (2016). A Conceptual Model of Vegetation-Hydrogeomorphology Interactions within River Corridors. River Res. Applic. 32 (2), 142–163. doi:10.1002/rra.2928
Gurnell, A. M., Petts, G. E., Hannah, D. M., Smith, B. P. G., Edwards, P. J., Kollmann, J., et al. (2001). Riparian Vegetation and Island Formation along the Gravel-Bed Fiume Tagliamento, Italy. Earth Surf. Process. Landforms 26, 31–62. doi:10.1002/1096-9837(200101)26:1<31::aid-esp155>3.0.co;2-y
Haberl, H., Winiwarter, V., Andersson, K., Ayres, R. U., Boone, C., Castillo, A., et al. (2006). From LTER to LTSER: Conceptualizing the Socioeconomic Dimension of Long-Term Socioecological Research. Ecol. Soc. 11 (2), 13, Available at: http://www.ecologyandsociety.org/vol11/iss2/art13/. doi:10.5751/es-01786-110213
HAÖ (2007). Hydrological Atlas of Austria. Vienna: Federal Ministry of Agriculture, Regions and Tourism. doi:10.1109/wicom.2007.851
Helfricht, K., Huss, M., Fischer, A., and Otto, J.-C. (2019). Calibrated Ice Thickness Estimate for All Glaciers in Austria. Front. Earth Sci. 7, 1–15. doi:10.3389/feart.2019.00068
Hiebl, J., and Frei, C. (2016). Daily Temperature Grids for Austria since 1961 – Concept, Creation and Applicability. Theor. Appl. Climatology 124 (1-2), 161–178. doi:10.1007/s00704-015-1411-4
Hock, R., Rasul, G., Adler, C., Cáceres, B., Gruber, S., Hirabayashi, Y., et al. (2019). “High Mountain Areas,” in IPCC Special Report on the Ocean and Cryosphere in a Changing Climate. Editors H.-O. Pörtner, D. C. Roberts, V. Masson‐Delmotte, and P. Zhai (Geneva: IPCC), 131–202.
Hofstätter, M., Lexer, A., Homann, M., and Blöschl, G. (2017). Large-scale Heavy Precipitation over central Europe and the Role of Atmospheric Cyclone Track Types. Int. J. Climatol 38 (51), e497–e517. doi:10.1002/joc.5386
Hohensinner, S., Egger, G., Muhar, S., Vaudor, L., and Piégay, H. (2021). What Remains Today of Pre‐industrial Alpine Rivers? Census of Historical and Current Channel Patterns in the Alps. River Res. Applic 37, 128–149. doi:10.1002/rra.3751
Hohensinner, S., Lager, B., Sonnlechner, C., Haidvogl, G., Gierlinger, S., Schmid, M., et al. (2013b). Changes in Water and Land: the Reconstructed Viennese Riverscape from 1500 to the Present. Water Hist. 5 (2), 145–172. doi:10.1007/s12685-013-0074-2
Hohensinner, S., Sonnlechner, C., Schmid, M., and Winiwarter, V. (2013a). Two Steps Back, One Step Forward: Reconstructing the Dynamic Danube Riverscape under Human Influence in Vienna. Water Hist. 5 (2), 121–143. doi:10.1007/s12685-013-0076-0
Jóhannesson, T., Raymond, C., and Waddington, E. (1989). Time-Scale for Adjustment of Glaciers to Changes in Mass Balance. J. Glaciol. 35 (121), 355–369. doi:10.3189/s002214300000928x
Lamprecht, A., Semenchuk, P. R., Steinbauer, K., Winkler, M., and Pauli, H. (2018). Climate Change Leads to Accelerated Transformation of High-Elevation Vegetation in the central Alps. New Phytol. 220 (2), 447–459. doi:10.1111/nph.15290
Lane, S. N., Bakker, M., Gabbud, C., Micheletti, N., and Saugy, J.-N. (2017). Sediment export, Transient Landscape Response and Catchment-Scale Connectivity Following Rapid Climate Warming and Alpine Glacier Recession. Geomorphology 277, 210–227. doi:10.1016/j.geomorph.2016.02.015
Le Quesne, C., Acuña, C., Boninsegna, J. A., Rivera, A., and Barichivich, J. (2009). Long-term Glacier Variations in the Central Andes of Argentina and Chile, Inferred from Historical Records and Tree-Ring Reconstructed Precipitation. Palaeography, Palaeoclimatol. Palaeoecol. 281 (3-4), 334–344. doi:10.1016/j.palaeo.2008.01.039
Lebiedzinski, K., and Fürst, J. (2018). Entwicklung der alpinen Abflussregime in Österreich im Zeitraum 1961-2010. Österr Wasser- und Abfallw 70, 474–484. doi:10.1007/s00506-018-0499-z
Leibundgut, C., and Kohn, I. (2014). European Traditional Irrigation in Transition Part I: Irrigation in Times Past-A Historic Land Use Practice across Europe. Irrig. Drain. 63, 273–293. doi:10.1002/ird.1826
Leonelli, G., Pelfini, M., Morra di Cella, U., and Garavaglia, V. (2011). Climate Warming and the Recent Treeline Shift in the European Alps: The Role of Geomorphological Factors in High-Altitude Sites. AMBIO 40, 264–273. doi:10.1007/s13280-010-0096-2
Marren, P. M., and Toomath, S. C. (2014). Channel Pattern of Proglacial Rivers: Topographic Forcing Due to Glacier Retreat. Earth Surf. Process. Landforms 39, 943–951. doi:10.1002/esp.3545
Mayer, R., and Erschbamer, B. (2017). Long-term Effects of Grazing on Subalpine and alpine Grasslands in the Central Alps, Austria. Basic Appl. Ecol. 24, 9–18. doi:10.1016/j.baae.2017.07.005
Oerlemans, J. (1994). Quantifying Global Warming from the Retreat of Glaciers. Science 264 (5156), 243–245. doi:10.1126/science.264.5156.243
Parajka, J., Kohnová, S., Bálint, G., Barbuc, M., Borga, M., Claps, P., et al. (2010). Seasonal Characteristics of Flood Regimes Across the Alpine–Carpathian Range. J. Hydrol. 394 (1-2), 78–89. doi:10.1016/j.jhydrol.2010.05.015
Patzelt, G. (2019). Gletscher – Klimazeugen von der Eiszeit bis zur Gegenwart. Berlin: Hatje-Cantz Verlag.
Reitmaier, T., Lambers, K., Walser, C., Zingman, I., Haas, J. N., Dietre, B., et al. (2013). Alpine Archäologie in der Silvretta. Archäologie der Schweiz 36 (1), 4–14.
Remy, D. (2012). Waldgrenze und Waldgrenzschwankungen in der Silvretta/Zentralalpen – Funde von Pinus cembra oberhalb der potentiellen Waldgrenze im Oberen Fimbertal. Berichte der Reinhold-Tüxen-Gesellschaft 24, 61–75.
Rogora, M., Frate, L., Carranza, M. L., Freppaz, M., Stanisci, A., Bertani, I., et al. (2018). Assessment of Climate Change Effects on Mountain Ecosystems through a Cross-Site Analysis in the Alps and Apennines. Sci. Total Environ. 624, 1429–1442. doi:10.1016/j.scitotenv.2017.12.155
Roni, P., and Beechie, T. (2013). Stream and Watershed Restoration – A Guide to Restoring Riverine Processes and Habitats. Chichester, UK: Wiley-Blackwell.
Rouse, J. W., Haas, R. H., Scheel, J. A., and Deering, D. W. (1974). Monitoring Vegetation Systems in the Great Plains with ERTS. Proc. 3rd Earth Resource Technol. Satellite (Erts) Symp. 1, 48–62.
Scorpio, V., Andreoli, A., Zaramella, M., Moritsch, S., Theule, J., Dell'Agnese, A., et al. (2020). Restoring a Glacier‐Fed River: Past and Present Morphodynamics of a Degraded Channel in the Italian Alps. Earth Surf. Process. Landforms 45, 2804–2823. doi:10.1002/esp.4931
Tappeiner, U., Tasser, E., Leitinger, E., and Tappeiner, G. (2006). “Alpine space – man & environment: Die Alpen im Jahr 2020,” in Landnutzung in den Alpen: historische Entwicklung und zukünftige Szenarien (Innsbruck: Innsbruck University Press), Vol. 1, 23–39.
Tasser, E., Ruffini, F. V., and Tappeiner, U. (2009). An Integrative Approach for Analysing Landscape Dynamics in Diverse Cultivated and Natural Mountain Areas. Landscape Ecol. 24, 611–628. doi:10.1007/s10980-009-9337-9
Ulbrich, K. (1961). Genauigkeit der ersten Meßtisch-Katastralvermessung in Österreich. Österreichische Zeitschrift für Vermessungswesen 49 (2), 44–53.
Verrall, B., and Pickering, C. M. (2020). Alpine Vegetation in the Context of Climate Change: A Global Review of Past Research and Future Directions. Sci. Total Environ. 748, 141344. doi:10.1016/j.scitotenv.2020.141344
Vogt, W. (1993). Die Alpe Jam. Seine Geschichte oder die wundersamen Beziehungen zwischen Engadin, Tarasp - Tirol, Galtür - und Göfis. Göfis: Agrargemeinschaft Jamalpe.
Walther, G. R., Beißner, S., and Burga, C. A. (2005). Trends in the Upward Shift of Alpine Plants. J. Vegetation Sci. 16, 541–548. doi:10.1111/j.1654-1103.2005.tb02394.x
Warburton, J. (1990). Observations of Bedload Transport Can Channel Bed Changes in a Proglacial Mountain Stream. Arctic Alpine Res. 24, 195–203.
Weber, P., Andreassen, L. M., Boston, C. M., Lovell, H., and Kvarteig, S. (2020). An ∼1899 Glacier Inventory for Nordland, Northern Norway, Produced from Historical Maps. J. Glaciol. 66 (256), 259–277. doi:10.1017/jog.2020.3
Zekollari, H., Huss, M., and Farinotti, D. (2019). Modelling the Future Evolution of Glaciers in the European Alps Under the EURO-CORDEX RCM Ensemble. The Cryosphere 13, 1125–1146. doi:10.5194/tc-13-1125-2019
Zekollari, H., Huss, M., and Farinotti, D. (2020). On the Imbalance and Response Time of Glaciers in the European Alps. Geophys. Res. Lett. 47, e2019GL085578. doi:10.1029/2019gl085578
Keywords: land use, glacier, meltwater stream, land cover change, historical environmental change, European Alps, long-term ecosystem research
Citation: Hohensinner S, Atzler U, Fischer A, Schwaizer G and Helfricht K (2021) Tracing the Long-Term Evolution of Land Cover in an Alpine Valley 1820–2015 in the Light of Climate, Glacier and Land Use Changes. Front. Environ. Sci. 9:683397. doi: 10.3389/fenvs.2021.683397
Received: 20 March 2021; Accepted: 26 July 2021;
Published: 05 August 2021.
Edited by:
André Mascarenhas, Humboldt University of Berlin, GermanyReviewed by:
Athanasios Votsis, University of Twente, NetherlandsCopyright © 2021 Hohensinner, Atzler, Fischer, Schwaizer and Helfricht. This is an open-access article distributed under the terms of the Creative Commons Attribution License (CC BY). The use, distribution or reproduction in other forums is permitted, provided the original author(s) and the copyright owner(s) are credited and that the original publication in this journal is cited, in accordance with accepted academic practice. No use, distribution or reproduction is permitted which does not comply with these terms.
*Correspondence: Severin Hohensinner, c2V2ZXJpbi5ob2hlbnNpbm5lckBib2t1LmFjLmF0; Andrea Fischer, YW5kcmVhLmZpc2NoZXJAb2Vhdy5hYy5hdA==
Disclaimer: All claims expressed in this article are solely those of the authors and do not necessarily represent those of their affiliated organizations, or those of the publisher, the editors and the reviewers. Any product that may be evaluated in this article or claim that may be made by its manufacturer is not guaranteed or endorsed by the publisher.
Research integrity at Frontiers
Learn more about the work of our research integrity team to safeguard the quality of each article we publish.