- Department of Chemistry, Covenant University, Ota, Nigeria
Microplastics, which serve as sources and vector transport of organic contaminants in both terrestrial and marine environments, are emerging micropollutants of increasing concerns due to their potential harmful impacts on the environment, biota and human health. Microplastic particles have a higher affinity for hydrophobic organic contaminants due to their high surface area-to-volume ratio, particularly in aqueous conditions. However, recent findings have shown that the concentrations of organic contaminants adsorbed on microplastic surfaces, as well as their fate through vector distribution and ecological risks, are largely influenced by prevailing environmental factors and physicochemical properties in the aquatic environment. Therefore, this review article draws on scientific literature to discuss inherent polymers typically used in plastics and their affinity for different organic contaminants, as well as the compositions, environmental factors, and polymeric properties that influence their variability in sorption capacities. Some of the specific points discussed are (a) an appraisal of microplastic types, composition and their fate and vector transport in the environment; (b) a critical assessment of sorption mechanisms and major polymeric factors influencing organic contaminants-micro (nano) plastics (MNPs) interactions; (c) an evaluation of the sorption capacities of organic chemical contaminants to MNPs in terms of polymeric sorption characteristics including hydrophobicity, Van der Waals forces, π–π bond, electrostatic, and hydrogen bond interactions; and (d) an overview of the sorption mechanisms and dynamics behind microplastics-organic contaminants interactions using kinetic and isothermal models. Furthermore, insights into future areas of research gaps have been highlighted.
Introduction
Plastic pollution in the terrestrial environment and marine ecosystems is pervasive and has evolved as an emerging global challenge due to exponentially increasing production rates, distribution and consumption of single-use plastics. Although plastics have significantly contributed to improvements in healthcare industry, building construction, electronics and contemporary human life in general, burgeoning build-up of improperly managed plastic wastes has raised concerns over their long-term health and ecotoxicological risks (Fahrenkamp-Uppenbrink, 2018; Ha and Yeo, 2018; Lei et al., 2018; Yu et al., 2018; Barletta et al., 2019; Besseling et al., 2019; Savoca et al., 2019; Adika et al., 2020; Benson and Fred-Ahmadu, 2020; Bakir et al., 2020; Ding et al., 2020; Everaert et al., 2020; Fred-Ahmadu et al., 2020a; Fred-Ahmadu et al., 2020b; Lu et al., 2020; Na et al., 2020; Wilcox et al., 2020; Wong et al., 2020; Yu and Chan, 2020). Microplastics (MPs; 0.1 µm—5 mm) and nanoplastics (NPs; <0.1 µm) are ubiquitous and can accumulate in marine and freshwater ecosystems and exposed organisms, carrying chemical contaminants that are capable of posing considerable threats to human health, environment and aquatic life (de Souza Machado et al., 2018; Hartmann et al., 2019; Benson and Fred-Ahmadu, 2020; Cole et al., 2020; Xu et al., 2020). Micro (nano) plastics (MNPs) are generally speculated to have increased environmental and health threats to marine organisms primarily due to their small size, predicted ubiquitousness, direct and indirect intake of plastic particles, bioavailability and enhanced concentrations of sorbed toxic chemicals (Setälä et al., 2018; Yu et al., 2018; Cole et al., 2020; Fred-Ahmadu et al., 2020a; Fred-Ahmadu et al., 2020b; Xu et al., 2020; Yu and Chan, 2020).
Primary MNPs are directly produced and could get into the environment through various sources like packaging in industrial processes, textiles, and personal care cosmetics, while secondary MNPs originate from the physico-chemical fragmentation of larger plastic particulates (Fred-Ahmadu et al., 2020c; Menéndez-Pedriza and Jaumot, 2020). However, on escape into the environment, they get eroded into lakes, rivers, seas, and the ocean. They are pollutants that do not readily biodegrade, thus causing persistent pollution. Plastics comprise chemical additives that are usually not chemically bonded to the plastic particles molecules and are likely to get leached into the surrounding aqueous medium (Benson and Fred-Ahmadu, 2020; Wright and Kelly, 2017). Several additives, including UV-stabilizers, plasticizers, antioxidants, and flame retardants contained in plastics have been widely reported (Jang et al., 2017; Sun et al., 2019; Tanaka et al., 2019; Fred-Ahmadu et al., 2020a; Fred-Ahmadu et al., 2020b; Jeong and Choi, 2020; Xu et al., 2020). Chemical additives such as esters of phthalic acids, which are commonly known as phthalate esters (PAEs) are ubiquitous, highly toxic, and commercially used as plasticizers for enhancing the durability, flexibility, lightness, and transparency of plastics (Xu et al., 2020; Zhang P. et al., 2019; Hahladakis et al., 2018; Avio et al., 2017; Kang et al., 2017). There are growing concerns that exposure to these chemical additives through direct and indirect ingestion of micro (nano) plastics could have severe reproductive, respiratory, neurotoxicological and stress responses on marine organisms, as well as potential health effects on humans (Xu et al., 2020).
Humans are readily exposed to the health risks of microplastics by daily ingestions through the use of products like toothpaste or skincare products made with plastic microbeads. Studies showing contamination of food suggest the presence of microplastics in the atmosphere (Liebezeit and Liebezeit, 2014). In 2017, 83% of tap water sampled from countries globally were found to be contaminated with microplastics (Carrington, 2017; Tyree and Morrison, 2017). Workers at facilities producing plastics, fish farming industries, wastewater treatment, or aquaculture facilities are at risk as well because they have direct contact with microplastics. Microplastics are small enough to be mistaken for food by fishes, and this results in fishes not feeling the need for food as they have a belly full of these substances that never break down in the digestive system, eventually resulting in starvation. Intestinal blockage, which could increase mortality rate, is also a hazard associated with the ingestion of microplastics (Ashton et al., 2010; Besseling et al., 2013; Cole et al., 2013; Wright et al., 2013; Hämer et al., 2014).
Microplastics vary in sizes and are classified either as primary or secondary microplastics taking into consideration whether they originate from physical or chemical fragmentation of larger plastics (macroplastics) or are micron-sized industrially produced plastics (Wright et al., 2013; Jaikumar et al., 2019). Mostly, primary microplastics (PMPs) are largely manufactured for commercial applications, including plastic pellets, microbeads, personal care products, and microfibers (Andrady, 2011; Cole et al., 2013). Primary microplastics are also known to find use in the healthcare industry as vectors for drugs (Patel et al., 2009). Conversely, secondary microplastics (SMPs) are generated from the degradation of plastics into smaller pieces after plastics have entered the environment (Andrady, 2011). This breakdown could be fueled by biological, geological, physical and chemical degrading agents, including photodegradation caused by sunlight (UV-B) exposure (Eriksen et al., 2014; Ekvall et al., 2019; Jaikumar et al., 2019; Fred-Ahmadu et al., 2020a). Most microplastics in the marine environment are secondary microplastics (UNEP, 2015; da Costa et al., 2016). Microplastics of this type are usually from plastic water bottles, bags, electronic parts, and equipment made of polyethylene (Boucher and Friot, 2017). Moreover, both classes of microplastics are not easily degradable but could persist as organic-vector pollutants in the environment, causing havoc to aquatic life and humans. Microplastics can further degrade into much smaller sizes overtime, the smallest known to exist are 1.6 μm (6.3 × 10−5) in diameter, and report states they are frequently found in oceans (Hasenmueller et al., 2017). This process of breaking down large plastics into smaller ones is known as fragmentation. It is a crucial source of the uneven shape of microplastics (Grossman 2015).
On the other hand, nanoplastics (NPs) are polymer particles within the size range from 1 to 1000 nm that are unintentionally produced as a result of the degradation of microplastics, and are capable of exhibiting a colloidal behavior (Gigault et al., 2017, Gigault et al., 2018; Wagner and Reemtsma, 2019; Wahl et al., 2021). While degradation of aged-plastic or the fragmentation particles of especially macro- and/or microplastics is the principal mechanism of nanoplastics formation in the terrestrial and marine environments, NPs could be released from intentional channels such as manufacturing process, washing fibrous materials, plastic object usage (Bouwmeester et al., 2015; Gilgault et al., 2018; Hartmann et al., 2019; El Hadri et al., 2020). According to Gilgault et al. (2018) and El Hadri et al. (2020), NPs are mono- and polydispersed mix of several polymeric substances that are capable of undergoing heteroaggregation with various natural and man-made species including metal (loids), organic molecules and metal oxides during the process of formation and transformation in the environment. Although there are very limited studies on the sorption potentials and the sparse information associating chemical additives and organic pollutants with NPs, a recent study has suggested that the relatively smaller size, morphology, enhanced surface reactivity and diffusion properties could influence the sorption behavior of NPs (El Hadri et al., 2020; Wahl et al., 2021).
Sorption of organic compounds on MNPs increases the likelihood of these particulates being transported further into the environment and also may portend complex ecological and health effects. The capacity for different plastic polymers to adsorb organic compounds varies with type structures, surface area (Teuten et al., 2007), size (Koelmans et al., 2013), and crystallinity (Rusina et al., 2007). However, the sorption capacity of organic compounds to microplastics varies with chemical and physical conditions of environment like temperature, salinity, hydrophobicity, diffusivity (Rusina et al., 2007; Karapanagioti and Klontza, 2008; Smedes et al., 2009).
This paper provides a detailed overview of the current state of knowledge on the potential environmental impacts of micro (nano) plastics and elucidates their interactions with emerging organic contaminants. The identification of specific sorption mechanisms and environmental factors could help us better understand the possible risks associated with contaminant bioavailability and long-distance transport in aquatic and terrestrial environments. Furthermore, understanding the possibilities of desorption of organic compounds into the marine environment after they have been transported by microplastics and the time it takes could also help weigh the impacts of plastic marine debris on the marine environment and its organisms. In order to achieve this, we conducted a thorough search on Scopus and Google Scholar for the terms “microplastics”, “nanoplastics”, “plastic pollution”, “plastic marine pollution”, “plastic additives”, “marine litters”, “sorption in plastics”, and “plastic interaction with organic contaminants.” A search for sorption mechanisms in connection with flame retardants, polycyclic aromatic hydrocarbons, pharmaceuticals and personal care products, and stabilizers was also conducted. We discovered that between 2007 and 2020, the number of peer-reviewed articles rose year after year (Figure 1). We also observed that about 28 peer-reviewed articles provided a descriptive overview of studies on the interactions of microplastics with organic and toxic inorganic chemicals.
The mechanisms and factors regulating the interactions and sorption of microplastics onto hydrophobic organic contaminants (HOCs) have been widely studied, with many articles documenting the findings. The microplastics-organic contaminants interaction is essential for predicting the fate and vector transport of microplastics in the environment. As a result, the sorbent-sorbent partitioning mechanism provides supporting information for determining the behavior of HOCs on microplastic surfaces, as well as their potential interactions (Atugoda et al., 2020; Fred-Ahmadu et al., 2020c). Organic micropollutant sorption onto micro (nano) plastics has been shown to be a function of plastic type as well as their properties (Guo et al., 2019). For example, the sorption of HOCs such as polycyclic aromatic hydrocarbons onto polyethylene (PE) microplastic pellets have been found to have a proportional relationship with the octanol-water partition coefficients (Kow) (Fries and Zarfl, 2012). Microplastic composition and properties and surface charge have also been shown to play important roles in regulating their ability to sorb organic contaminants (Wang et al., 2015; Zhang et al., 2018b). Furthermore, pH, ionic strength, salinity, sorbate concentration, and dissolved organic matter have been reported to play a significant role in the adsorption of HOCs onto microplastics (Guo et al., 2019; Atugoda et al., 2020).
To date, no literature reviews dedicated entirely to provide a broader analysis of HOCs-microplastic interactions and the evaluation of associated underlying environmental conditions and sorbate-sorbent assumptions in order to explicate the partitioning of organic contaminants based on kinetic and isothermal models. Therefore, this review aims to objectively examine and summarize existing findings and knowledge on the interactions of microplastics and HOCs. The specific objectives of this review were to appraise and synthesize existing scientific information about: a) microplastic types, composition and their fate and vector transport in the environment; b) the sorption mechanisms and major polymeric factors influencing organic contaminants-micro (nano) plastics (MNPs) interactions; c) the sorption capacities of organic chemical contaminants to MNPs in terms of polymeric sorption characteristics including hydrophobicity, van der Waals forces, π–π bond, electrostatic, and hydrogen bond interactions; and d) the sorption mechanisms and dynamics behind microplastics-organic contaminants interactions using kinetic and isothermal models.
Microplastics: Types, Composition and Their Environmental Fate
Plastics are composed of long-chain polymers that are bound together by additives. Microplastics of various major shapes, including fragments, foams, pellets, films, fibers, and microbeads, are ubiquitous in both aquatic and terrestrial environments and are extensively used by humans for a variety of applications. Microfibres and microbeads are mostly secondary microplastics that are formed as a result of clothing washing. Microplastic pollution in aquatic ecosystems is mainly caused by the abundance of fibers, fragments, films, foams, microbeads, and pellets, which marine organisms can ingest and also have the potential for long-distance transport.
Microfibres
Polyesters, polyamides (e.g. nylon), polypropylenes and polyacrylonitrile (e.g. acrylic) are polymers used to produce microfibres. They make up 71% of the total microplastic in the Great Lakes, and they have ranked the third-largest microplastic pollutant (by weight) in the ocean. They are secondary microplastics that enter the environment from clothing, diapers, and cigarette butts. Microfibres enter the environment through a variety of ways, one of which is abrasive action on synthetic fishing gear and marine ropes (Welden and Cowie, 2017). Research funded by Patagonia shows that 2,000 microfibres get into our waterways by washing a fleece jacket just once and synthetic textiles can lose between 0.7 and 1.3 g of microplastic fibers per wash or over 100,000 microplastic fibers per wash (Kalčíková et al., 20117). They also quickly enter water bodies through wastewater discharged from domestic and industrial washing machines or breakdown of plastic-based textiles during production or use. According to the report, about 40% of microfibres are estimated not to be filtered out at wastewater treatment plants, thus they could find their way into ocean and lakes. Sometimes, sewage sludge could entrap microbeads from treatment plants which are often used for fertilizers, thus, causing soil pollution and can eventually be eroded by rain or wind into the marine environment (Kalčíková et al., 2017; Jemec et al., 2016). They are non-biodegradable and usually become persistent pollutants that not only clog sewage drains but can be harmful to aquatic life.
Microbeads
Microbeads are plastic particles that are less than 1 mm in diameter. They are primary microplastics intentionally added to personal care products like facial cleansers, exfoliating soaps, toothpaste, and cosmetics (Leslie, 2014). Polyethylene, polystyrene, polypropylene, polylactic acid and polyethylene terephthalate are synthetic polymers used to make microbeads (Norwegian Environmental Agency, 2014). Microbeads exist as needs to replace natural ingredients in cosmetic increased along the years and is reported by the United Nations Environment Program to first appear in personal products 50 years ago. They are tiny enough to easily pass through water filtration systems (GESAMP, 2015) ending up in the ocean and lakes where they clog the digestive tracts of fishes. A recent study has indicated that up to 94,000 minuscule beads can be flushed down the drain each time someone uses a facial or body wash containing microbeads. Recent work by Napper et al. (2015) showed that microbeads extracted from cosmetics products have the potentials to adsorb organic pollutants. A study by Napper et al. (2015) also revealed a sorption capacity of PE found in microbeads.
Fragments
Fragments are the most common type of microplastics. They originate from the breakdown of larger pieces of plastics into smaller pieces after they are discarded into the environment, thus, secondary microplastics (Eriksen et al., 2014). They are commonly from pieces of cutlery, lids, or single-use product made of polyethylene. Studies that identify the onset of fragmentation are quite little. However, a study shows that fragmentation of polyethylene films occurs through weathering and other processes, but most likely on the subjection of plastic to UV radiation in the presence of atmospheric oxygen (Kalogerakis et al., 2017). Fragments are tiny in size and can be mistaken for food or prey by fishes, and most of the ingested plastics found in the digestive tracts of fishes are in the form of fragments (Lusher et al., 2013).
Nurdles
Nurdles, also known as pellets, are the second most common type of microplastic found in freshwater (Mason et al., 2020). They are the elementary units that make up almost every plastic product. Polyethylene, polypropylene, polystyrene, and polyvinylchloride are synthetic polymers used to produce nurdles. They mostly escape into the environment during shipping or transfer periods, after which they erode into water bodies, before getting washed ashore on a beach. The Environmental Protection Agency, in the early 1990s recognized nurdles as life-threatening products to fishes and wildlife. Nurdles are sponge-like materials that absorb toxins like DDT (dichloro-diphenyl trichloromethane) and PCBs (polychlorinated biphenyls) and can cause further defects upon ingestion by fishes.
Foams
Foams are lightweight microplastics commonly used in food containers, cups, and packing materials. The primary polymer present in foams is polystyrene. They are bonded by chemicals that can be released into food, especially when heated, thus exposing human health to danger. Foams just like other types of microplastics can act as carriers of hydrophilic pollutants such as antibiotics (Zhou et al., 2017), and organophosphorus esters and phthalates due to their porosity (Zhang et al., 2018a).
Physisorption, Chemisorption and Major Factors Governing of Sorption of Organic Contaminants to Micro (Nano) Plastics
It has been reported that MNPs are capable of serving as vectors for transporting hydrophobic organic microcontaminants such as polybrominated diethers (PBDs), polyfluorinated alkyls (PFAS), polychlorinated biphenyls (PCBs), polycyclic aromatic hydrocarbons (PAHs), phthalates surfactants, personal care products (PCPs), pharmaceuticals (tetracycline, ciprofloxacin, sertraline, propranolol, and sulfamethoxazole) in terrestrial and aquatic environments (Guo and Wang, 2019; Atugoda et al., 2020; Benson and Fred-Ahmadu, 2020; Puckowski et al., 2021). The adhesion of these contaminants to microplastics in the environment is a function the high surface area to volume ratio and hydrophobicity of microplastic particles. Furthermore, the degree of adsorption has been shown by various studies to depend on the polymeric properties of microplastics including polymer type, particle size, surface area, charge, crystallinity, compound-specific factors, and environmental factors including pH, salinity, ionic strength, and dissolved organic matter (Li et al., 2018; Guo et al., 2019; Atugoda et al., 2020).
The strong interactions between these contaminants and microplastics are largely associated with the prevailing sorption mechanisms. Sorption is an important process that governs the fate and transport or partitioning of organic pollutants between solid microplastic particles and water. It facilitates the accumulation of molecules of contaminants (adsorbate molecules) in contact with microplastics (adsorbent) in aquatic or terrestrial environments. The degree of interaction by which the adsorbates molecules are accumulated onto the adsorbents defines the nature and type of adsorption. Furthermore, the mechanism can either be classified as physisorption (physical adsorption) or chemisorption (chemical adsorption). Physisorption is the adsorption in which the molecular interactions between the adsorbate molecules and the adsorbent are primarily governed by van der Waals forces whereas chemisorption involves the valence forces of the kind found in the formation of chemical contaminants. Worded differently, chemisorption is the adsorption that takes place if there are chemical bonds between adsorbate molecules and the adsorbent.
Physisorption is non-specific in nature and is generally regarded as a weak, reversible process governed by competitive adsorption and desorption which takes place at different rates at the heterogenous surface of the microplastics (Heinrich et al., 2020). According to a report by Atugoda et al., 2020 on the adsorptive molecular interaction between polyethylene microplastics and ciprofloxacin molecules, it was observed that the adsorbate molecules were accumulated and adsorbed onto the heterogenous polymeric surface of the polyethylene microplastics through weak van der Waal’s forces and electrostatic interactions. This implies that the adsorption mechanism between the molecules of the adorbate and adsorbent were governed primarily by physisorption accompanied by partitioning into the porous monolayer surface of the microplastics (Atugoda et al., 2020). In general, aliphatic polymers including polyethylene and polypropylene microplastics particularly have adsorbate-adsorbent molecular interactions through the van der Waals forces facilitated primarily by the non-specific functional groups (Hüffer et al., 2018; Xu et al., 2018). On the other hand, chemisorption is irreversible and very specific in nature, and depends primarily on the proportionality of the surface area (Zhang et al., 2018b). The following are the major polymeric properties of microplastics affecting the sorption of organic contaminants.
Plastic Polymers: Types and Composition
The composition of plastics includes synthetic polymers which are made of units called monomers and can have average molecular weights up to several million (GESAMP, 2015). Polymers have long chain-like molecules with robust Van der Waal attractive forces between them, and these chain-like molecules contribute to properties such as strength and fracture toughness, which also makes them degradable to a limited extent (Andrady, 2011). Chain length also influences melting point and physical properties like glass transition temperature
In general, it is imperative to note that during plastic sorption (chemisorption and physisorption) of chemical pollutants, the properties of the plastic polymer (micro- and nanoplastics) coupled with various factors of the environmental matrix could play major roles in modulating the sorption rate (Fred-Ahmadu et al., 2020a; Fred-Ahmadu et al., 2020b). The polymer types and properties, environmental factors and sorption characteristics that could assist in furthering our understanding of the mechanisms influencing the sorption processes is presented in Figure 2 below. Overall, sorption depends on the type of organic compound being studied and the weathering process the polymer might have gone through in the environment which affects size of the polymer and influences sorption.
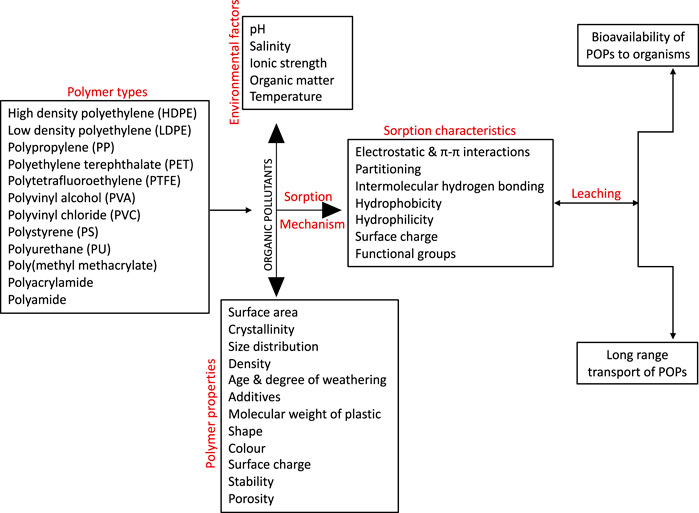
FIGURE 2. The main properties of micro (nano) plastic polymers and factors that govern the sorption of organic pollutants.
Polymer Size and Surface Area
In addition to polymer type, polymer size is a factor that plays a major role in sorption process of organic pollutants unto micro and nanoplastics. Plastic polymer size determines the surface area available for uptake of organic pollutants (Holmes et al., 2012; Wang and Wang, 2018). While sorption on plastic polymers of the same size would depend on the polymer type as shown in study by Rogers (2018), where PE-10 had a higher sorption capacity than PS-10 In contrast, polymer size is an important determining factor of sorption process for plastic polymer with different sizes. Studies have shown that nanoplastics which are smaller in size have a higher sorption capacity than microplastics because of the presence of higher specific surface area. However, further decrease in polymer size could result in aggregation of plastic polymer particles, thus causing an overall decline in specific surface area of available adsorption sites as shown in study by Wang et al., 2019.
Polymer Crystallinity
Earlier X-ray diffraction analysis of polymers showed that plastic polymers have structures that vary between ideally crystalline solids and liquid-like amorphous materials. Synthetic organic polymers are categorized into three major structure types on the basis of the different arrangements of their molecular chains. These are crystalline, semi-crystalline and amorphous polymers (Zhao et al., 2020). They consist of numerous carbon chains randomly distributed through the solid structure and linked by the intermediate amorphous areas (Kavesh and Schultz, 1969; Frost et al., 2009). The degree of crystallinity of a plastic polymer is attributed to these polymer carbon chains and their arrangement. A more ordered and fixed carbon chains suggest a higher degree of crystallinity which hinders movement of organic compound molecules, because of higher energy required for movement, resulting in lower sorption capacity of such polymer (Karapanagioti and Klontza, 2008). A lower degree of crystallinity however, suggests an amorphous structure where carbon chains are disordered, disorderliness in carbon arrangement promotes free movement of organic compounds molecules around the structure of the polymer hence influencing a greater sorption (Hartmann et al., 2017). According to reports, the crystallinity and rubbery domains of micro (nano) plastics are capable of influencing the sorption of organic chemical contaminants to MNPs (Rochman et al., 2013a; Hüffer and Hofmann, 2016; Zhao et al., 2020).
The Sorption Mechanisms of Organic Contaminants to Micro (Nano) Plastics
Hydrophobicity
This is the inability of both microplastics and organic pollutants to dissolve in water, thus having affinity for each other. Hydrophobic interaction is one of the predominant sorption mechanisms influencing the sorption of micropollutants especially undissociated hydrophobic organic contaminants (HOCs) to micro (nano) plastics (Llorca et al., 2018; Liu G. et al., 2019; Tourinho et al., 2019; Wu et al., 2019; Yu et al., 2019). The hydrophobic nature of microplastics contributes significantly to the sorption of organic pollutants on MPs, and hydrophobic interactions are generally associated with the aggregation of nonpolar molecules to nonpolar micro (nano) plastics in an aqueous medium (Hüffer and Hofmann, 2016; Liu F. F. et al., 2019; Tourinho et al., 2019). In general, hydrophobic interaction would usually increase with the organic pollutant-water partition coefficient of the sorbate (Zhoa et al., 2020). The organic pollutants partition between the aqueous phase and the MPs is explained to be characterized by the linear sorption isotherm (Zhu and Chen, 2000). Some studies have reported that the sorption capacity of HOCs such as 17β-estradiol (E2) and antibiotics is largely regulated by the chemical structures (amorphous nature and crystallinity) of the polar and nonpolar MNPs (Zhoa et al., 2020; Liu G. et al., 2019; Li et al., 2018). In broad terms, increased sorption capacity has been generally observed for the polar MNPs in comparison with relatively low capacity indicated for the regular nonpolar MPs (Zhoa et al., 2020; Wang et al., 2018). However, NPs have been shown to have strong hydrophobicity and enhanced sorption capacity to HOCs owing to its increasing surface area ratio associated with fragmentation and other weathering processes (Yu et al., 2019). Thus, chemical contaminants with high hydrophobicity are generally prone to exhibit high adsorption affinity to micro (nano) plastics (Razanajatovo et al., 2018).
Van der Waals Force
This is the relatively weak electric force between the plastic polymers and the organic compounds that allow them adhere to each other. It describes the attraction of intermolecular forces between molecules. Sorption process usually stem from van der Waals interaction when they cannot be explained by either electrostatic interaction or hydrophobic interaction (Wang et al., 2020). The sorption of chemical contaminants by aliphatic polymeric sorbents such as polyethylene microplastics and polyvinyl chloride takes place through van der Waals interactions (Hüffer and Hofmann, 2016; Fred-Ahmadu et al., 2020a; Torres et al., 2021).
The Pi-Pi (π–π) Bond Interaction
This interaction contributes to the sorption capacity of plastic polymers that have benzene rings in their structure (Tourinho et al., 2019). The π–π electron— donor— acceptor interaction is a noncovalent attraction between an electron donor and an electron acceptor either of which could belong to the microplastic or the organic pollutant (Figure 2). In biomedical field and biotechnology, the π–π stacking interactions are widely employed in loading biological and chemical drugs into delivery systems for controlled release in environmental-responsive systems (Zhuang et al., 2019). Hüffer and Hofmann (2016) reported that the enhanced adsorption of pharmaceutical molecules on polystyrene microplastics could be linked to the strong interaction between the aromatic moiety of the polymeric particles (sorbent) and the conjugated π cloud of the aromatic structure of the adsorbate contaminants. Furthermore, it has been reported that the intermolecular binding between Naphthalene (NAP) and naphthalene derivative molecules 1-Methylnaphthalene NAP-CH3, 1-Naphthoic acid NAP-COOH, 1-Naphthol NAP-OH, and 1-Naphthylamine (NAP-NH2) with polystyrene microplastics occurred mainly through the π–π interaction. However, the strength of the π-π interaction was primarily governed by the presence of functional groups in NAP derivatives (Liu L. et al., 2016; Yu et al., 2020). Additionally, the π–π interactions have been reported to play major role in the sorption of diclofenac, ibuprofen, and naproxen on polystyrene microplastics (Elizalde-Velázquez et al., 2020), the sorption of phenanthrene and pyrene on polyurethane microplastics (Zhao et al., 2020), and the sorption of ciprofloxacin, sulfadiazine, and trimethoprim on polystyrene microplastics (Li et al., 2018).
Electrostatic Interaction
Another important and predominant mechanism that governs the sorption of micropollutants to MNPs is the electrostatic interaction, which has been widely reported by many studies (Wang et al., 2015; Guo et al., 2018; Xu et al., 2018; Tourinho et al., 2019; Wu et al., 2019). Electrostatic interaction owes to the electric charges that exist between a plastic polymer and an organic compound. Electrostatic sorption occurs when a plastic polymer comes in contact with an organic compound or attracts molecules with an electric charge opposite that of its own, while an electrostatic repulsion occurs when both plastic polymer and organic compound have the same electric charge (Wang et al., 2015; Guo et al., 2018; Xu et al., 2018). The medium pH and the pH of point of zero charge (pHpzc) are significant contributing factors to electrostatic interaction. In general, the adsorbent is negatively charged when the pH is higher than the pHpzc (Mato et al., 2001; Zhang et al., 2018b; Tourinho et al., 2019; Xu et al., 2019). In contrast, the adsorbent is positively charged when the pH is lower than the pHpzc (Wang et al., 2020). According to a study by Razanajatovo et al. (2018), conducted to evaluate the sorption and desorption of three pharmaceuticals including sertraline, propranolol, and sulfamethoxazole on polyethylene (PE) microplastics in aqueous environment, it was indicated that electrostatic forces played a major role in the sorption process. It was reported that the pHpzc of the PE was lower than the medium pH and accordingly indicated a negatively surface charge on the microplastic particle. Thus, the negatively charged molecule of the surface of the polymeric substance (PE) attracted the oppositely charged of the pharmaceuticals, and therefore increased the rate of absorption of the micropollutants unto the microplastic surface (Razanajatovo et al., 2018; Xu et al., 2018). Conversely, lower sorption capacity is likely associated with electrostatic repulsion between the negative charges of microplastics and contaminants that exhibit anionic characteristics (Razanajatovo et al., 2018; Tourinho et al., 2019; Xu et al., 2019). Generally, the electrostatic repulsion or sorption (attraction) between charged molecules of contaminants and net surface charge on polymers is primarily influenced by three key factors including the medium pH, the pH of point of zero charge of the MPs, and the acid dissociation constant associated with the micropollutant (Razanajatovo et al., 2018; Tourinho et al., 2019; Puckowski et al., 2021).
Hydrogen Bond Interaction
This is another factor that contributes to surface sorption on microplastics. Hydrogen bond interaction is one that involves hydrogen atoms located in microplastics and organic compound. The presence of proton donor and proton acceptor groups influence attraction between organic compound and MPs (Tourinho et al., 2019). It is a bond that is weaker than an ionic or covalent bond, but stronger than van der Waals force (Figure 3). Zhang S. et al. (2019) has reported that specific hydrogen bonding interaction solely influences the sorption of naphthalene on polyuria microplastics.
Polymers Types and Associated Sorption Mechanisms
The six most commonly produced polymers are polyethylene (PE), high-density polyethylene (HDPE), low-density polyethylene (LDPE), polyvinyl chloride (PVC), polystyrene (PS) and polypropylene (PP) (Andrady, 2011; Rochman et al., 2013a).
Polyethylene (PE)
Polyethylene is the most widely used plastic with a global production of about 80 million tons (Merrington, 2011). It comes in low-density polyethylene (LDPE) and high-density polyethylene (HDPE) that differ in their susceptibility to weathering and fragmentation. These differences play a significant role in their fate, behavior and ecological impact in the environment (Andrady, 2011). The LDPE is soft and ductile with a density of 0.910–0.925 g/cm3 and a molecular weight of 40,000 Da (Kurtz and Manley, 2009). On the other hand, HDPE has a density of 0.959–0.965 g/cm3 and a molecular weight of 100,000–250,000 Da (Kurtz and Manley, 2009). PE is a thermoplastic that is reusable, cost-effective, and finds application in films for plastic bags, bottles, milk carton lining, bowls, and buckets, thus widely used and quickly released into the environment. A study has showed that PE has a high sorption capability that stems from its enhanced diffusivity due to their larger free volume, greater flexibility, and mobility that allows organic compounds to diffuse quickly through them (Pascall et al., 2005). Additionally, Razanajatovo et al. (2018) reported that PE made microplastics adsorb compounds with high hydrophobicity readily. A report by Uber et al. (2019) shows that sorption of organic compounds onto HDPE is usually described by both absorption and adsorption process because of the glassy properties of this polymer and amorphous segments of its structure. However, sorption on LDPE, a rubbery polymer is controlled by its density and low crystallinity (van Krevelen et al., 2009). Consequently, more side branches imply higher amount of free volume within its chain network and higher sites for organic compounds to adhere onto (Peacock, 2000).
Polypropylene (PP)
Polypropylene belongs to the polyolefin family and is considered one of the most versatile of all polymers as not only does it find robust applications both as fiber and plastic, but it is also highly recyclable. The utilization of PP includes the production of materials like kitchen utensils, athletic apparel, rugs, and car batteries. PP like PE is a rubbery polymer with higher diffusivity that enhances sorption (Bakir et al., 2012). Polypropylene is the second most widely produced plastic polymer after polyethylene and has a slightly higher chemical resistance than PE (Kenneth et al., 2005). It is created by polymerization of polypropylene gas, obtained from high temperature cracking of petroleum hydrocarbons and propane (Shanmuganathan and Ellison, 2014). Its high flexibility is another property that contributes to its sorption capacity (Koerner and Koerner, 2018). It has a low density and high thermal stability. Furthermore, PP has a lower surface area that influences lower sorption capacity when compared with PE (Fotopoulou and Karapanagioti, 2012). Aging and weathering processes lead to increase of this polymer surface area and effective diffusivity, however, introduction of oxygen-containing groups during weathering process increases polarity of the surface, thus eventually decreasing its sorption capacity (Endo et al., 2005; Zhan et al., 2016).
Polystyrene (PS)
Polystyrene is the most widely used aromatic thermoplastic polymer (Lynwood, 2014), with a global production capacity of 15.5 million metric tons in 2018 and might have a slight increase reaching 15.56 million metric tons by 2023. They are non-polar, like PE (Hüffer and Hofmann, 2016). Naturally transparent and available as foam materials used in packaging and solid plastics used in medical devices. Generally, their uses include protective packaging, containers, lids, bottles, trays, tumblers, and disposable cutlery. The PS just like other polymers are non-biodegradable as they contain carbon to carbon bonds that cannot be broken by microorganisms. However, recent studies show that some organisms can degrade PS, albeit very slowly (Ho et al., 2017). A research in 2015 showed that mealworms, the larvae form of the darkling beetle Tenebrio Molitor could digest expanded PS (Yang et al., 2015). Aumentado also reported that super worms Zophobas Morio in comparison to Tenebrio Molitor consume more significant amounts of EPS over more extended periods. The π-electron system (Nakano, 2010) and amorphous structure (Frick and Stern, 2013) of PS contribute to its high sorption capacity.
Polyesters (PES)
Polyesters are synthetic fiber derived from coal and petroleum. They find the most use in textile industries, food packaging, and the manufacturing of plastic bottles due to their lightweight, durability, and resilience. They make up fiber products that do not significantly get recycled, thus having a higher probability of ending up in the marine environment (Dris et al., 2017). Polyethylene terephthalate (PET) is a plastic polymer that belongs in the polyester group. The presence of positive functional group and crystalline structure increases the sorption capacity of PET (Miandad et al., 2018). The film, intra-particle diffusion, hydrophobic interaction and pH of solution are some of the significant factors that contribute to the sorption capacity of PET (Liu G. et al., 2020). It is commonly used as container for water and packaging products like beverages, food, cosmetic products, and pharmaceutical products. Studies have shown that adsorption on PET plastic polymer is usually accompanied by change in Tg and degree of crystallinity which results in swelling of the polymer. Sorption on PET decreases with increase in organic compound present and is also dependent on the chemical’s microstructure, meaning that sorption also varies with degree of crystallinity which in turn varies with change in temperature (Limam et al., 2005). Data from study by Limam et al. (2005) illustrated that diffusion coefficient increases with increase in temperature, but decreases with increase in degree of crystallinity.
Polyvinyl Chloride (PVC)
Polyvinyl chloride is recorded to be one of the most widely produced polymers. It comes third to polyethylene and polypropylene (Allsopp and Vianello, 2000). It is frequently employed in the production of various materials that are widely used in homes, offices, and schools around the world, thus making them abundant in the environment. They have sponge-like properties that enable them to soak up persistent organic pollutants (POPs). Research shows that PVC has a lower sorption capacity for POPs compared to other polymers; Rodrigues pointed out that the size and shape of PVC polymer particles contribute to its lower sorption capacity. They have glass-like properties that make them more condensed and present higher cohesive force. These properties lead to a reduction in desorption rates as it creates stronger adsorption of organic compounds to the adsorption sites (Rodrigues et al., 2019). However, investigation of sorption process of tylosin; a PPCP on PE, PP, PS and PVC by Guo et al. (2018) revealed that PVC had the highest sorption capacity for tylosin. The high sorption can be attributed to the hydrophilic property of tylosin.
Organic Pollutants and Their Interactions With Micro (Nano) Plastics
Recent studies have shown that microplastics are susceptible to contamination by organic compounds after long term exposure and interaction. Sorption is influenced by not only the properties of plastic polymers but also that of the organic compound getting sorbed. These properties include hydrophobicity and hydrophilicity, the surface charge, and the functional groups (Liu Y. et al., 2020). A research by Hirai et al. (2011) showed that fragments (10 mm) taken from remote and urban beaches contained polychlorinated biphenyls (PCBs), polycyclic aromatic hydrocarbons (PAHs), dichloro-diphenyl-trichloroethane and its metabolites (DDTs), polybrominated diphenyl ethers (PBDEs), alkylphenols and bisphenol. PAH and PCBs were suspected to have been sorbed from seawater and this was owed to the hydrophobicity of the organic pollutants. Microplastics have been suggested by recent studies to act as sinks or sources of organic pollutants. According to regulatory bodies, organic compounds are priority pollutants when they are persistent, accumulate in living organisms, and are toxic. These bodies include the United States Environmental Protection Agency (USEPA), the American Institute for Environment and Health (AIEH), and the International Agency for Research on Cancer (IARC), the European Union (EU). Polycyclic aromatic hydrocarbons (PAHs), organochlorinated pesticides (OCP) polychlorinated biphenyls (PCBs), organophosphorus esters, and phthalate esters (PAEs) are some of these priority pollutants. These persistent organic pollutants (POP) are synthetic organic compounds found in both marine and terrestrial environments (Rios et al., 2007; Benson et al., 2020). They are hydrophobic and lipophilic compounds, that partition firmly to solids in the aquatic system and partition to lipids in organisms. The various types of these chemical additives present in plastics and their interactions with micro- and nanoplastics are depicted in Figure 4.
Polycyclic Aromatic Hydrocarbons (PAHs)
Polycyclic aromatic hydrocarbons (PAHs) are pollutants associated with petroleum and arise from incomplete combustion of organic materials. (Lima et al., 2005). They occur naturally in crude oil, the ingredient for plastic; thus, the plastic being a source of PAHs is expected. PAHs can also act as a sink for PAHs, due to the hydrophobic nature that does not allow them to dissolve in water, but rather associate with particles (Lima et al., 2005). This is why plastics can be used as measures of PAHs in seawater indirectly (Reitsma et al., 2013). It is well known that PAHs are largely sorbed onto polyethylene (Lohmann, 2012), and polyurethane foam (Dmitrienko et al., 2001). PAHs on ingestion cause short terms effects such as eye irritation, nausea, vomiting, diarrhea, and confusion. The long-term effects of exposure to PAHs include jaundice, cataracts, kidney, and liver damage (Illinois Department of Public Health).
For example, Teuten et al. (2007) compared the sorption capacity of PE, PP, and PVC for phenanthrene from seawater and recorded that sorption magnitude that followed the order PE >> PP > PVC. The desorption rate of phenanthrene from these polymers was also studied in order to understand the bioavailability of sorbed phenanthrene, and the result suggested significant desorption rate, thus, confirming the toxicity of phenanthrene sorbed PE. In addition, Rochman et al. (2013b) examined polystyrene (PS) as a source and a sink for polycyclic aromatic hydrocarbons (PAHs) in the marine environment. This research was conducted to study the amount of PAHs associated with virgin PS, sorption of PAHs on PS in the marine environment, and the concentration of PAHs on different types of plastic debris. The results suggested that polystyrene particles are vectors for polycyclic aromatic hydrocarbons, i.e. PAHs are associated with PS debris through absorption and manufacturing. The sorption of PAHs on PS was compared to the sorption on the five most common polymers high-density polyethylene (HDPE), low-density polyethylene (LDPE), polyethylene terephthalate (PET), polypropylene (PP) and polyvinyl chloride (PVC). It was discovered that PS sorbed higher concentrations of PAHs than PP, PET, and PVC, similar to HDPE and LDPE.
Rogers (2018) investigated the sorption of 1,3-dimethyl naphthalene, phenanthrene, and fluoranthene on polyethylene (PE), polystyrene (PS), and polyester (PES). It was observed that 1,3-dimethyl naphthalene has a higher solubility in water than phenanthrene and fluoranthene indicating that a higher amount of 1,3-dimethyl naphthalene remains in the water and lesser quantity is sorbed on the microplastics. Emily stated that sorption of phenanthrene and fluoranthene to microplastics could save marine organisms from the toxicity the PAHs present because where adsorption is possible, there will be a lesser concentration of PAH readily available in the marine environment as they would have been picked up by the microplastics instead. A possible solution will be capturing the microplastics in the ocean, with the PAHs sorbed to them. While this appears to be a good argument, one can add that high solubility of 1,3-dimethyl naphthalene in the aquatic system rather than its sorption on microplastics indicates limited long-range transport of 1,3-dimethyl naphthalene to the marine environment which in turn reduces the bioavailability of this particular PAH to marine organisms.
The metrics mass and surface area in agreement with other studies showed that PE-10 had a higher sorption capacity than PS-10, irrespective of temperature. This is reflected by PE-10 particle sizes being more massive than PS-10 as shown by Scanning Electron Microscope (SEM), a higher distribution coefficient (Kd), and the possible ability of PE to change form and be more receptive of PAHs. Unfortunately, little could be concluded from the results of the study about the sorption of PAHs to polyesters (PES).
Sørensen et al. (2020), studied the relative bioavailability of MP-sorbed PAHs (fluoranthene and phenanthrene) to two marine copepod species (Acartia Tonsa and Calanus finmarchicus) under co-exposure conditions representing those occurring in the marine environment. The results showed that adsorption occurred more at lower temperatures and for smaller microplastics with sizes 10 μm, while absorption was dominant for microplastics with sizes 100 µm. Results indicated that only free dissolved PAHs were significantly bioavailable to copepods under co-exposure conditions with MP-sorbed PAHs because there was a decrease in the concentration of free PAHs in the water as more MPs were introduced. Research by Teuten et al. (2007) showed that amongst the three polymers investigated which were PE, PP and PVC, PE had the highest sorption capacity for phenanthrene and sorption followed the order PE > PP > PVC. Sorption of PAHs was higher for polyethylene microplastic than for polystyrene microplastic of the same size. This finding is supported by previous studies where the sorption of PAHs to PS and PE particles of comparable sizes was investigated (Wang et al., 2018; Wang and Wang, 2018). The greater segmental mobility and free volume in the molecular segments of polyethylene capable of facilitating solute diffusion into the polymer may be contributing factors to its higher PAH sorption capacity than polystyrene (Pascall et al., 2005; Karapanagioti and Klontza, 2008).
Polyhalogenated Compounds
Organochlorides are organic compounds that have at least one covalently bonded atom of chlorine. They are compounds with numerous useful applications but are of environmental and health concerns (Rossberg et al., 2000). They are naturally occurring in bacteria, plants, and animals from which they could be isolated. They include dioxins produced during burning as well as chloromethane that find their way into the environment by biological decomposition, forest fire, and volcanoes. Organochlorides have been banned in North America and Europe due to their toxicity, but they still find use in the production of Organochlorine pesticides (OCPs) and Polychlorinated biphenyls (PCBs). OCPs are highly mobile, and traces have been found in Antarctica, their toxicity varies according to their molecular size, volatility, and effects on the central nervous system, they also accumulate in food chains, causing reproductive problems. Polybrominated diphenyl ethers (PBDEs) are another example of polyhalogenated compounds, structurally akin to PCBs consisting of two halogenated aromatic rings. The levels at which they are found in households are capable of reducing fertility in humans (Harley et al., 2010) which is why they have attracted scrutiny. Their industrial production is restricted under the Stockholm Convention.
Allen et al. (2018), studied the variations in sorption of organochlorine pesticides (OCPs) and polychlorinated biphenyls (PCBs) across low-density polyethylene (LDPE), high-density polyethylene (HDPE), polypropylene (PP), polyvinyl chloride (PVC), polystyrene (PS), and polyethylene terephthalate (PET). Results showed that sorption rates for each contaminant varied widely. Among the six plastic polymers, LDPE showed the highest sorption for OCPs while both LDPE and PVC showed the highest sorption rate for PCBs. Polyethylene terephthalate (PET) showed the lowest sorption for both OCPs and PCBs. Compared to other similar studies, higher sorption capacity of PVC could be attributed to the increase in its surface area due to the filing of microplastic during preparation and crystallinity as a result of lack of weathering. The glassy feature of PET and PVC could, however, result in their lower sorption capacity for an organic contaminant. In contrast, the rubbery feature of LDPE and HDPE’s contribute to their high sorption capacity.
Except for PVC, these results are similar to experimentally derived plastic-water partition coefficients summarized by other studies (O'Connor et al., 2014; O'Connor et al., 2016) for a variety of PCBs and other chemicals, e.g. LDPE ≈ HDPE ≥ PP > PVC ≈ PS. Field studies in the marine environment by Rochman et al., 2013a also observed relatively low concentrations of PCBs sorbed to PVC and PET, with higher concentrations of PCBs and PAHs found in LDPE, HDPE, and PP. Polyethylene terephthalate (PET) being with the lowest sorption capacity. Scutariu et al. (2019) examined the sorption processes of organochlorine pesticides (OCPs) on PET. Results showed that adsorption followed the order; DDT class (52–58%) > heptachlor class (23%) > endosulfan class (6–15%)> endrin class (4–12%) > HCHs isomers class (2–8%). A study by Rocha-Santos and Duarte, 2017 also showed that DDT, DDD, and DDE compounds exhibited the highest degree of adsorption on PET.
According to (Liu Z. et al., 2020) result in the investigation of chlorophenols on PET showed that the adsorption process by PET is controlled by film and intra-particle diffusion. Hydrophobic interaction was the primary mechanism of adsorption, but solution pH also played a significant factor in adsorption capacity. Xu et al. (2019) sorption study of polybrominated diphenyl ethers on PA, PE, PP, and PS resulted in adsorption that followed the order PS > PA > PP > PE. The sorption process and capacity owed to the different crystallinity, specific surface area, and surface structure of the microplastics.
Phthalate Esters (PAEs)
Phthalate esters (PAEs) are esters of phthalic acids and chemical additives used in the production of plastics, making plastics a source of PAEs in the environment. They are used in enhancing flexibility, durability, longevity, and transparency of plastics. However, they are not chemically bound to the polymers of these plastics and can get leached into the marine environment, especially at relatively high temperatures (Holahan and Smith 2015; Paluselli et al., 2018; Benson and Fred-Ahmadu, 2020; Jiménez-Skrzypek et al., 2020). The PAEs include di (2-n-butoxyethyl) phthalate (DBEP), butylbenzyl phthalate (BBZP), di (2-ethoxyethyl)phthalate (DEEP), di-ethyl phthalate (DEP), di (2-ethylhexyl)phthalate (DEHP), di-cyclohexyl phthalate (DCHP), di-isobutyl phthalate (DiBP), di-methylglycol phthalate (DMGP), di-methyl phthalate (DMP), di (hexyl-2-ethylhexyl)phthalate (HEHP), di-n-amyl phthalate (DnAP), di-n-butyl phthalate (DnBP), di-nhexylphthalate (DnHP), di-n-nonyl phthalate (DnNP), di-n-octyl phthalate (DnOP), and di (4-methyl-2-pentyl)phthalate (DMPP) (Benson and Fred-Ahmadu, 2020). PAEs upon direct or indirect ingestion by humans can cause disruption of the endogenous hormones and result in behavioral and reproductive dysfunction (Diamanti-Kandarakis et al., 2009; Cole et al., 2011; Benjamin et al., 2017; Benson and Fred-Ahmadu, 2020) PAEs are endocrine-disrupting compounds (ECDs) that disrupt natural production, secretion, and metabolic system of the human body (Diamanti-Kandarakis et al., 2009; Benson and Fred-Ahmadu, 2020) Very little research has been done to study the sorption of phthalate esters on microplastics.
Liu F. F. et al. (2019) investigated the sorption of phthalate esters unto three types of microplastic polymers, namely polyvinyl chloride (PVC), polyethylene (PE), and polystyrene (PS). According to the study, polystyrene had the highest sorption capacity for phthalate esters, followed by polyethylene and then polyvinyl chloride. The higher sorption of PAEs to PS was suggested to be as a result of the strong π-π interactions between PS and the two PAEs investigated. The two types of phthalate esters investigated were diethyl phthalate (DEP) and dibutyl phthalate (DBP). DEP had lower
Deng et al. (2020) examined the sorption and bioavailability of four types of PAEs (DEHP, DBP, DEP, and DMP) onto virgin polyethylene. The study addressed how PE could adsorb PAEs, transport, and leach them into the gut of a mouse and cause intestinal accumulation. It was observed that sorption of PAEs onto virgin PE was in the order of DEHP > DBP > DEP > DMP, with maximum adsorption 70.27 ± 7.89, 57.13 ± 6.03, 39.15 ± 5.33, and 31.44 ± 4.13 μg/g respectively. Adsorption of these PAE types on PE is positively correlated with their octanol-water partition coefficient
Pharmaceuticals and Personal Care Products (PPCPs)
In recent years, many synthetic hydrophobic organic chemicals have found far reaching applications in pharmaceuticals and personal care products (PPCPs). The broad utilization of these chemicals has prompted growing concerns over their ecotoxicity and widespread availability in terrestrial and aquatic environments as contaminants (Oberg and Leopold, 2019; Atugoda et al., 2020; Puckowski et al., 2021). Most PPCPs comprise of complex organic compounds of bioactive ingredients including pharmaceuticals such as antibiotics (tetracycline, ciprofloxacin, sertraline, propranolol, and sulfamethoxazole), antiseptics, disinfectants, analgesics, and cytostatic hormones, which when released into the aquatic environment could exert harmful effects on human health, biota and the environment (Yin et al., 2017; Dey et al., 2019; Yang et al., 2021). PPCPs are polar and highly hydrophilic organic contaminant (Caliman and Gavrilescu, 2009; Ebele et al., 2017). The sorption strength of PPCPs is largely influenced by their low volatility and the low octanol–water partition coefficients (Kow) in aquatic medium. They have higher percentage sorption particularly to aged microplastics, because of the increase in surface areas, hydrophilic properties at the surface and oxygen-containing functional groups of aged MPs (Zhang et al., 2018b; Liu G. et al., 2019; Zuo et al., 2019) Partitioning, intermolecular hydrogen bonding, electrostatic and π-π interactions are the mechanisms that govern adsorption of pharmaceuticals on microplastics. The pH of pharmaceuticals in a solution also contributes to their sorption process as there are variations in the speciation of their cation, anion, and zwitterion in a specific pH condition (Guo et al., 2018).
Wu et al. (2016) studied the sorption behavior of pharmaceuticals and personal care products (PPCPs); carbamazepine (CBZ), 4-methyl benzylidene camphor (4MBC), triclosan (TCS), and 17α-Ethinyl estradiol (EE2), to polyethylene (PE) debris (250–280 µm). Hydrophobicity of these selected PPCPs resulted in linear sorption coefficients
Magadini et al. (2020) reported the results of in-situ experiments in New York City waterways to investigate the adsorption of pharmaceuticals; atenolol, sulfamethoxazole, and ibuprofen on to eight types of test materials (pellets from five types of widely-used polymers, small pieces of straws, fragments of bags, and glass beads for control). Adsorption coefficients were calculated based on mass and surface area for each type of material. Greater surface area to mass ratio of straw and bag samples influenced higher values for their mass-based coefficients. In contrast, surface area-based coefficients were predominant among the plastic materials tested as well as glass beads. This result is an indication that surface area is a major determining factor for adsorption of pharmaceutical irrespective of the material type. It was also observed that rapid biofouling and formation of biofilms are factors that control the adsorption of pharmaceuticals onto plastics.
Daugherty et al. (2016) examined the effects of dissolved organic matter (DOM) on the sorption process of tonalide, 4-methyl benzylidene camphor (4-MBC), triclosan, and β-estradiol on aged polypropylene and polyethylene. Results showed that matrix composition and polymer type affected the sorption process, while compound hydrophobicity was the major contributing factor. DOM, however, variedly affected the sorption process based on the examined compounds. For example, sorption of 4-MBC was enhanced, while DOM inhibited that of tonalide. Polypropylene (PP) had a higher sorption capacity for the pollutants than polyethylene. However, sorption on PP microplastics was weaker, due to the presence of DOM that might have competed for the adsorption area. Thus, pollutants on PP microplastics could be more bioavailable to marine life.
Studies by Goedecke et al. (2017) showed sorption of metformin, a type-2 diabetes drug, and difenoconazole, a fungicide, onto virgin polymers polyamide (PA), polypropylene (PP), and polystyrene (PS). The influence of polymer properties, pH, salinity, and agitation on the sorption process was studied. PP was cryo-milled to increase its surface area, and PA was chemically pre-treated to simulate a loss of molecular weight. Sorption capacity followed the order PS > PP > PA, and it was observed that sorption was strongly governed by agitation, the effect of salinity was little, and pH seemed least relevant. Ašmonaitė et al. (2020) compared the ability of two polymers (PE and PS) and naturally occurring silica particles to act as a vector for exposure to 17α-ethinylestradiol, chlorpyrifos, and benzo (α) pyrene in fish. The study showed that while there was a moderate transfer of hydrophobic organic contaminants into fish through silica particles, PS and PE particles facilitated the higher chemical transfer and tissue accumulation of 17α-ethinylestradiol and chlorpyrifos. PS had higher sorption than PE, and this was attributed to its surface area. Guo and Wang (2019) study on the sorption of sulfamethoxazole (SMX), sulfamethazine (SMT), and cephalosporin C (CEP-C) in freshwater and seawater onto the naturally aged microplastics [polystyrene (PS) and polyethylene (PE)], showed that sorption mechanisms were hydrophobicity, van der Waals, and electrostatic. Liu F. F. et al. (2019) investigated the sorption behaviors and mechanism between hydrophilic ciproflaxin (CIP) on pristine and UV-accelerated aged PS and PVC. Sorption capacity of aged microplastics was higher than that of pristine microplastics. Physical interactions, including electrostatic interactions from pH influence that resulted in a different charge of CIP, partitioning, and intermolecular hydrogen bonding, were the dominant mechanism. Table 1 summarizes the previous studies on the characteristics of microplastics and organic chemical pollutants sorbed to degradable polymeric particles.
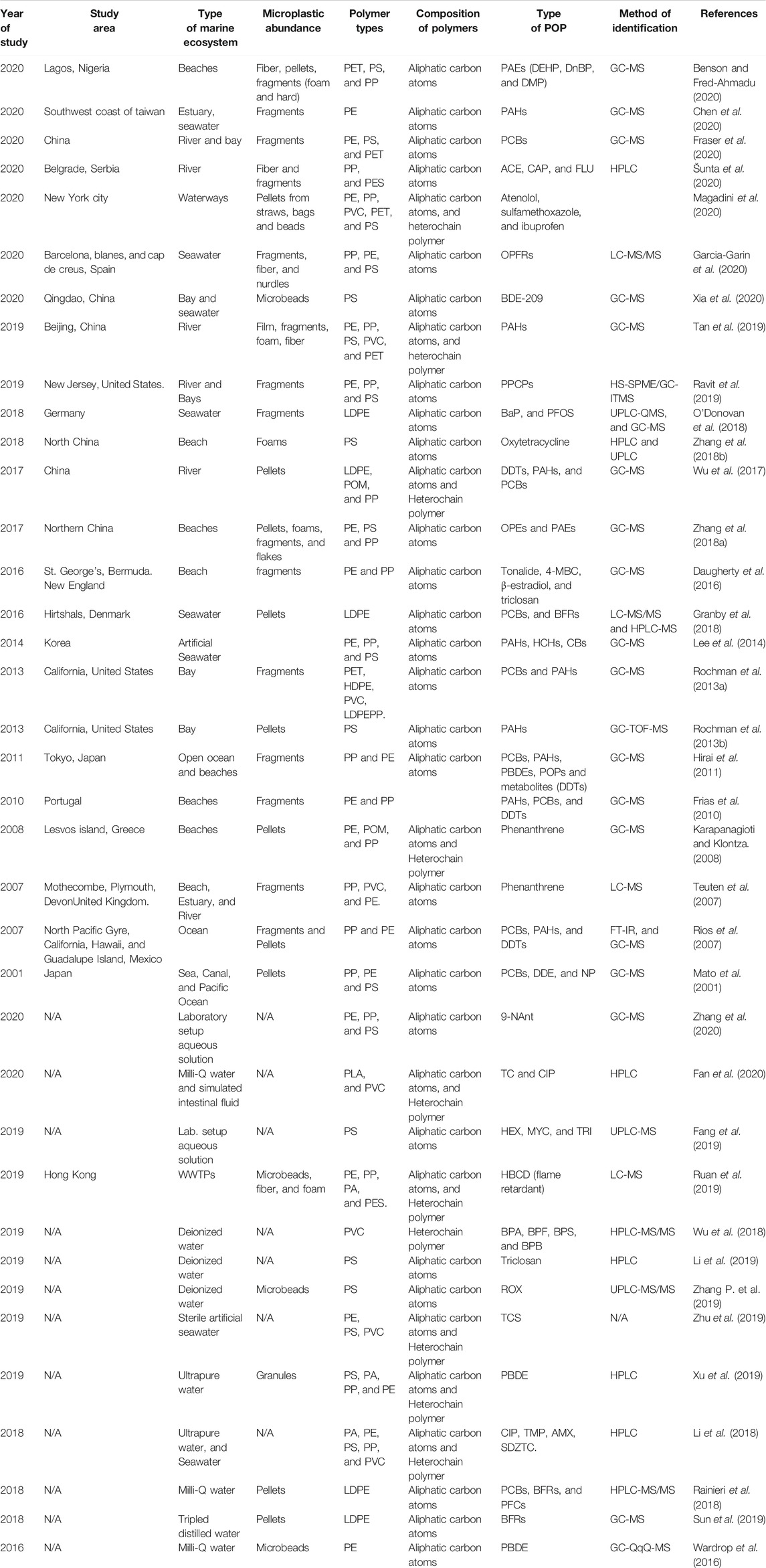
TABLE 1. A summary on characteristics of microplastics and organic pollutants sorbed to microplastics in previous studies.
Modeling Physical and Chemical Adsorption of Contaminants to Micro (Nano) Plastics
The kinetic and isothermal models are valuable tools that have been used in several studies in understanding the desorption and adsorption dynamics of sorbates (mainly chemical contaminants) in terms of order of the rate constant (Cazetta et al., 2011). A variety of kinetic models exists but the most common adsorption kinetics used in micro (nano) plastics-adsorbents studies are the first-order adsorption kinetic model (Skrip et al., 2013; Razanajatovo et al., 2018; Fred-Ahmadu et al., 2020c), pseudo-second-order model (Liu X et al., 2018; Razanajatovo et al., 2018; Wang and Guo, 2020), intraparticle diffusion model (Guo et al., 2019; Qiu et al., 2019), and film diffusion model (Hameed et al., 2008; Hu et al., 2011; Martins et al., 2015; Zhang et al., 2018b; Qiu et al., 2019; Wu et al., 2020). On the other hand, sorption isotherms are valuable tools used in evaluating the distribution of the sorbent molecules between the solid phase and the liquid phase in a system at equilibrium. The isothermal sorption model can effectively identify the adsorption processes and elucidate the interaction mechanisms between the sorbates and sorbent molecules (Guo et al., 2019; Wu et al., 2020). The commonest types of linear isothermal sorption models used include the linear adsorption model (Henry’s law) (Bakir et al., 2014; Hüffer et al., 2018; Razanajatovo et al., 2018; Qiu et al., 2019), Freundlich model (Hamdaoui and Naffrechoux, 2007; Teuten et al., 2007; Razanajatovo et al., 2018; Vieira et al., 2021), Langmuir model (Langmuir, 1916; Hamdaoui and Naffrechoux, 2007; Gonçalves et al., 2020; Yazidi et al., 2020; Vieira et al., 2021), Temkin adsorption model (Temkin, 1941; Hamdaoui and Naffrechoux, 2007; Tan et al., 2009; Ayawei et al., 2017; Wang and Guo, 2020), Elovich adsorption models (Elovich and Larinov, 1962; Hamdaoui and Naffrechoux, 2007), and Dubinine-Radushkevich adsorption model (Yang et al., 2006; Hu and Zhang, 2019; Wang and Guo, 2020).
First-Order Adsorption Kinetic Model
The first-order kinetic model postulated by Lagergren in 1898 (Lagergren, 1898) has been widely used to explore the adsorption kinetic data of chemical micropollutants by polymers (Valderrama et al., 2008; Ocampo-Pérez et al., 2012, Ocampo-Pérez et al., 2015; Razanajatovo et al., 2018). The first-order kinetic model equation is expressed differentially as:
The integration of Eq. 1 using the boundary conditions, t = 0— t and q = 0 yields a nonlinear form of Lagergren’s first order rate equation as follows:
where qt and qe represents the concentrations (µg/g) of chemical pollutants sorbed to the MNPs at time t and at equilibrium, respectively. The rate constant (g μg−1 h−1) of the first-order kinetics is given by K1.
Pseudo-Second-Order Kinetic Model
The second-order kinetic model is expressed using the following differential equation:
Thus, integrating Eq. 3 and using the initial conditions as q = 0, t = 0— t would yield the following nonlinear form of the pseudo-second-order equation as indicated below (Blanchard et al., 1984):
where qt is the adsorbed amount (µg g−1) at time t, qe is the adsorbed amount (µg g−1) at equilibrium, and
Intraparticle Diffusion Model
The adsorption kinetic data can be evaluated using the intraparticle diffusion model which is represented by the following equation (Weber and Morris, 1963):
where qt = the concentration of the chemical contaminant adsorbed to MNPs at time t,
Film Diffusion Model
The film diffusion kinetic model (FDM) can also be used to describe the possible adsorption interactions between MNPs and sorbate molecules (Martins et al., 2015; Zhang et al., 2018b). The FDM kinetics basically describes the “diffusion across liquid film” following the characteristic movement of sorbates across concentration gradients of solid subsurface regions, and the existence of adsorption by external film mass transfer followed by intraparticle pore diffusion to the surface of adsorbates (Boyd et al., 1947; McKay and Allen, 1980; Quek and Al-Duri, 2007; Plazinski, 2010). The nonlinear form of the film diffusion model equation is expressed as:
where Bt is the Boyd constant, qt is the adsorbed amount at time t, and qe is the adsorbed amount at equilibrium. However, the Boyd equation can be employed in predicting the rate-limiting step associated with either the intraparticle diffusion or film diffusion adsorption processes (Rocha et al., 2012; Martins et al., 2015). Using kinetic data from a study, a plot of Bt against time t would yield the Boyd plot and this could be used in identifying if the rate-limiting step is governed by film diffusion adsorption or intraparticle diffusion. The interpretation follows that if the experimental datapoints fit linearly and the line passes through the origin, then it could be deduced that the intraparticle diffusion involves the rate-limiting step of adsorption process, or else, the film diffusion governs the adsorption mechanism if the line of best fit passes further away from the origin (Hameed et al., 2008; Hu et al., 2011; Qiu et al., 2019; Wu et al., 2020).
Linear Adsorption Model
The linear isothermal model otherwise known as Henry’s law represents the fundamental adsorbate-adsorbent isotherm in which the sorbed amount at equilibrium relates linearly with the equilibrium concentration of the adsorbate on the adsorbent (Ayawei et al., 2017; Qiu et al., 2019). The linear model is an empirical isothermal equation which describes appropriate fitting of the adsorbate adsorption capacity when the initial adsorbate concentration is relatively low (Ruthven, 1984). The model has been widely used in understanding the partitioning of chemical pollutants between the solid and liquid phases (Bakir et al., 2014; Hüffer et al., 2018; Razanajatovo et al., 2018; Qiu et al., 2019; Wang and Guo, 2020). The linear model equation is as follows:
where qe (µg g−1) and Ce (µg L−1) = the adsorbed amount and adsorbate concentrations at equilibrium, and KH (L µg−1) = the Henry’s constant (partition coefficient).
Freundlich Adsorption Model
The Freundlich model is an empirical adsorption model that can be used to explore the adsorption or equilibria data into heterogenous surfaces, which is applicable to mono- and multilayer sorption and relates to the occupation of high-energy sites first (Yang, 1998; Hamdaoui and Naffrechoux, 2007; Teuten et al., 2007; Vieira et al., 2021). The Freundlich isothermal model is considered as an empirical equation that relates the adsorbate-adsorbent interactions on an heterogenous surface with adsorbing particles having variable adsorption affinities (Hameed et al., 2008; Foo and Hameed, 2010; Martins et al., 2015). The model is given by (Freundlich, 1906):
where qe (µg g−1) and Ce = (µg L−1) the amount of the chemical contaminant adsorbed to MNPs in the solid phase and in the aqueous phase at equilibrium, kf is the Freundlich sorption coefficient, which is corresponds to the relative sorption capacity of the polymer adsorbent, n is the Freundlich isotherm exponent (dimensionless), which determines the nonlinearity (Tolls, 2001; Hamdaoui and Naffrechoux, 2007). The Freundlich model could basically be used in describing the nonlinear sorption between the adsorbates and adsorbents. In general, the higher the concentration of the adsorbates, the higher the affinity of the adsorbates on the surface of the adsorbents. Moreover, the linear form of this model is expressed by the following equation:
However, it is important to explore the sorption isotherm by evaluating the optimum adsorption capacity between the MNPs and sorbates using variable weights of the adsorbent with constant initial amount Co of the absorbates. Consequently, ln qmax is equivalent to the extrapolated of ln q for C = Co. Therefore, following Halsey (1952), Eq. 6 can be expressed as follows:
where qmax = the Freundlich maximum adsorption capacity (µg L−1), and C0 = the initial concentration of the absorbates in the bulk solution (µg L−1). The Freundlich isothermal model has been relates the porosity and heterogeneity of micro (nano) plastic surface in understanding the sorption interactions between organic pollutants and MNPs. Several studies have reported that the Freundlich model could fit the sorption isotherms of organic chemical contaminants (sorbates) such antibiotics (propranolol, sertraline, sulfamethoxazole) (Razanajatovo et al., 2018), persistent organic pollutants (dichlorodiphenyltrichloroethane, phenanthrene and perfluorooctanoic acid) (Bakir et al., 2014), 2,2′,4,4′-tetrabromodiphenyl ether (Wu et al., 2020), polyhalogenated carbazoles (3-bromocarbazole, 2,7-dibromocarbazole, 3,6-dibromocarbazole, 3,6-dichlorocarbazole, 3,6-diiodocarbazole) (Qui et al., 2019) to MPs surfaces.
Langmuir Adsorption Model
The Langmuir adsorption model postulated by Langmuir (1916) describes the sorption relating to homogeneous surfaces on which the micropollutants will express high sorption affinity and form monolayers with specific sorption sites of the MNPs (Hamdaoui and Naffrechoux, 2007; Gonçalves et al., 2020; Yazidi et al., 2020; Vieira et al., 2021). The nonlinear equation of the Langmuir model can be described as:
where qe (µg g−1) = the concentration of the chemical contaminant adsorbed to MNPs in the solid phase at equilibrium, Ce (µg L−1) = the concentration adsorbed in the aqueous phase at equilibrium, qmax = the maximum adsorption capacity (µg g−1), and b = the Langmuir constant associated with the free energy of absorption (L µg−1). Moreover, the Langmuir isotherm can be transformed into other linear equations as follows:
Temkin Adsorption Model
The Temkin isotherm equation formulated by Temkin (1941) as an adsorption model assumes that adsorption is a multi-layer process characterized by uniform aggregation of binding energies, up to maximum binding energy (Temkin, 1941). It presumes that the heat of adsorption of aggregate molecules of adsorbate in the layer decreases linearly with increase in surface coverage on account of the adsorbate-adsorbent interactions on the adsorption process (Hamdaoui and Naffrechoux, 2007; Tan et al., 2009; Ayawei et al., 2017; Wang and Guo, 2020). Additionally, the model ignores the relatively high and low concentrations of the adsorbate in the liquid phase during the adsorption process (Hamdaoui and Naffrechoux, 2007). Temkin model is expressed as:
However, the linearized form of the Temkin isotherm model can be expressed as follows:
where R = the universal gas constant (kJ mol−1 K−1), T = the temperature (K), b = Temkin constant associated with the energy of adsorption, and KT = the Temkin equilibrium constant (L µg−1).
Elovich Adsorption Model
The Elovich isotherm model is widely used to describe the multilayer adsorption process of substances on heterogeneous surfaces (Elovich and Larinov, 1962). It is based on the kinetic principle which presumes that adsorption sites increase exponentially with adsorption (Hamdaoui and Naffrechoux, 2007). The Elovich isotherm can be expressed as follows:
However, the linear form of the Elovich isotherm is expressed as:
where qe is the adsorbed amount (µg g−1) at equilibrium, Ce (µg L−1) = the concentration adsorbed in the aqueous phase at equilibrium, KE = the Elovich equilibrium constant (L µg−1), and qmax = the Elovich maximum adsorption capacity (µg g−1). The KE and qmax can be calculated from the slope and intercept of the plot of
Dubinine-Radushkevich Adsorption Model
The Dubinine-Radushkevich (D-R) adsorption model plays an important role in explaining the physicochemical properties of the sorption process through the estimation of the free energy of sorption on heterogeneous surfaces (Ayawei et al., 2017; Wu et al., 2020). The D-R isotherm model is widely used to explain single-solute adsorption and to account for the effect of the porosity of heterogenous adsorbent surface (Alberti et al., 2012; Hu and Zhang, 2019). The isotherm model is based on the adsorption potential theory and the assumption that the adsorption process is comparable to the micropore volume filling and the equilibrium of the adsorption in a particular adsorbent-adsorbate interaction follows a Gaussian energy distribution (Dubinin and Radushkevich, 1947; Dąbrowski, 2001; Inglezakis, 2007; Wang and Guo, 2020). The nonlinear Dubinine-Radushkevich model can be expressed as follows:
Moreover, the linear form of the D-R isothermal model is expressed as:
Therefore, the adsorption potential,
where qmax (µg g−1) = the maximum adsorption capacity, qe (µg g−1) = the amount of adsorbate adsorbed per unit mass of adsorbent at equilibrium, ε (kJ mol−1) = the adsorption potential based on Polanyi’s potential theory, β (mol2 kJ−2) = a constant related to the adsorption energy, T (K) = absolute temperature, R (8.314 J mol−1 K−1) = universal gas constant, Cs (µg L−1) = the solubility of adsorbates, and Ce (µg g−1) = concentration of adsorbate at equilibrium (Yang et al., 2006; Hu and Zhang, 2019; Wang and Guo, 2020). The Dubinine-Radushkevich adsorption isotherm is an important model employed to differentiate physisorption from chemisorption (Atugoda et al., 2020).
Classically, the factors influencing the sorption potential of micropollutants onto plastic particles is a function of the polymeric properties of micro (nano) plastics, micropollutant’s characteristics, and solution chemistry of the environmental matrix including the pH, salinity, and dissolved organic matter (DOM) (Daugherty et al., 2016). Worded differently, the sorption capacity of chemical contaminants onto micro (nano) plastics will vary as the environmental matrix conditions changes. The maximum adsorption efficiency of 3-dimensional reduced graphene oxide onto PS microplastics has also been shown to be influenced by the concentration of ionic species, pH, matrix temperature, initial concentration of sorbate, and adsorption time (Yuan et al., 2020). According to this report, the Langmuir adsorption isotherm model and the pseudo-second-order kinetic model indicated a chemical adsorption mechanism influenced primarily by strong π–π interaction through electrostatic attraction and physical retention. The influence of DOM on the adsorption of oxytetracycline to weathered microplastic polystyrene foams using humic acid (HA) and fulvic acids (FA) as the two representative dissolved organic materials have been reported by Zhang et al. (2018b).
The report investigated and reported the influence of dissolved organic matter (DOM) on the adsorption of oxytetracycline to virgin as well as weathered microplastic PS foams using fulvic acids (FA) and humic acid (HA) as DOM representatives. The studies report that the oxytetracycline sorption to weathered PS foams significant varied with change in pH, ionic strength and the concentration of HA. Based on the Freundlich isotherm model, the sorption capacity coefficient Kf value of oxytetracycline increased considerably from 2420 (mg kg−1) (mg L−1)1/n (10 mg L−1 HA) to 3640 (mg kg−1) (mg L−1) 1/n (30 mg L−1 HA) and 5340 (mg kg−1) (mg L-1)1/n (50 mg L−1 HA) over an increasing concentration of HA for weathered microplastic PS foams. However, the sorption capacity decreased to 3940 (mg kg−1) (mg L−1)1/n when the concentration of the organic matter HA was raised to 100 mg L−1. On the contrary, with increase in the concentration of fulvic acid from 10 to 100 mg L−1, the sorption capacity coefficient slightly decreased from 1580 (mg kg−1) (mg L−1)1/n (10 mg L−1 FA) to 1450 (mg kg−1) (mg L−1)1/n (30 mg L−1 HA), and later increased slightly from 1670 (mg kg−1) (mg L−1)1/n (50 mg L−1 HA) to 1980 (mg kg−1) (mg L−1)1/n (100 mg L−1 HA) (Zhang et al., 2018b). Li et al. (2018) recently reported the sorption of multiple antibiotics, including amoxicillin, ciprofloxacin, tetracycline, sulfadiazine, and trimethoprim, by polyethylene, polyamide, polypropylene, polystyrene, and polyvinyl chloride microplastics, indicating that the sorption processes involved various MPS-antibiotics interaction mechanisms, such as van der Waals force, electrostatic and hydrophobic interactions, and hydrogen bonding. On the other hand, π-π interactions, electrostatic and hydrophobic interactions have been identified as the primary partitioning mechanisms regulating the sorption capacity of tetracycline by polypropylene, polystyrene, and polyethylene microplastics (Xu et al., 2018).
Several studies have also shown that rising temperature improves sorbates-MPs sorption, implying that the number of active surface sites on microplastics may increase as the temperature of the environmental matrix increases (Choi et al., 2020; Lin et al., 2021). For example, Lin et al. (2021) showed that with an increase in temperature to 313 K, the Langmuir partition coefficient (Qmax) and Freundlich partition coefficient (Kf) increased slightly from 1.01 to 1.58 μmol g−1 and 0.01–0.03 (µmol g-1) (µmol L−1)1/n, respectively, for virgin polystyrene microplastics.
In general, most isotherm models such as the Langmuir model is technically constrained by many environmental conditions and sorbate-sorbent assumptions, including homogeneous surfaces on which the micropollutants will show high affinity and form monolayer sorption with specific sites on microplastics (Chen et al., 2017). Besides, the Freundlich model presumes adsorbate-adsorbent interactions on heterogeneous adsorbent surfaces and extend to both mono- and multi-layer sorption on MPs (Saadi et al., 2015). For example, Lin et al. (2021) used several kinetic models to investigate the mechanisms and factors governing the sorption of tetracycline by virgin polystyrene microplastics and polystyrene-hexabromocyclododecane composite microplastics. According to the findings, intra-particle diffusion and film diffusion were the key influencing factors for tetracycline sorption into polystyrene-hexabromocyclododecane composite and polystyrene, respectively. However, to further provide a broader analysis of the sorption process and elucidate the sorption mechanisms and governing factors linking microplastics-tetracycline interactions, statistical physics models were employed. The findings explicated that the sorption mechanism was linked with the formation of monolayer through multi-molecular and non-parallel processes. In general, multiple mechanisms, including hydrophobic interaction and π-π interactions, collectively govern the sorption of tetracycline onto polystyrene-hexabromocyclododecane composite and polystyrene microplastics.
Prevalence and Impacts of Micro (Nano) Plastics in the Environment
Micro (nano) plastic particles and hydrophobic waterborne contaminants are two types of pervasive micropollutants found in marine ecosystems and terrestrial environments, mostly in water columns, sediments, beach coastlines, coastal seawaters, and within mid-abysmal ocean gyres, and their abundances, fate and vector transports and interactions have piqued the attention of researchers around the globe (Schwarzenbach et al., 2006; Cole et al., 2011; Brach et al., 2018; Benson and Fred-Ahmadu, 2020; Benson et al., 2020; Fred-Ahmadu et al., 2020b; Pereira et al., 2020; Li et al., 2021). Microplastic ingestion by marine animals has been widely documented, a phenomenon that may allow the transmission of hydrophobic organic compounds or toxic chemical additives to humans via the marine food web.
Plastic particles in aquatic environments could be found as fragments of 1 μm in diameter and 15 μm in length, rendering them increasingly ingestible by several smaller planktonic marine animals present in pelagic and benthic ecosystems (Frias et al., 2010; Cole et al., 2011). Although these microfibers could be potentially harmful to aquatic life because they can cluster, knot and interfere with animal activity, these plastics can typically prevent egestion once swallowed (Murray and Cowie, 2011). In both of these scenarios, the probability of voluntary ingestion of microplastics by aquatic animals, mistaking them for prey, is high. Microplastic uptake by higher trophic animals may also occur as a result of consuming lower trophic organisms that have ingested microplastic particles (Fendall and Sewell, 2009; Farrell and Nelson, 2013; Setälä et al., 2014; Brennecke et al., 2015; Pannetier et al., 2020).
The potential risk that could also be associated with ingestion of microplastics by aquatic organisms may be attributed to the leaching of inherent contaminants from the microplastics, as well as the release of the extraneous toxic chemicals compounds that may have adhered to the microplastic debris. Evidence of the availability and contamination impacts of such chemicals has been highlighted by many researchers. The adverse effects of microplastics on fishes and large aquatic animals, zooplankton, phytoplankton, microalgae, crustaceans, and seabirds have been widely reported (Boerger et al., 2010; Kögel et al., 2019; Ma et al., 2020; Corinaldesi et al., 2021; Wang et al., 2021), at the population levels (e.g., fertility, mortality, growth and organismal development, feeding activity) (Zarfl et al., 2011; Sussarellu et al., 2016; Heindler et al., 2017; Mouchi et al., 2019; Chapron et al., 2020; Liu G. et al., 2020; Issac and Kandasubramanian, 2021), cellular (e.g., motility; cell fragmentation, membrane stability, apoptosis) (Von Moos et al., 2012; Sun et al., 2019; Han et al., 2020; Tallec et al., 2020), and molecular levels (e.g., mortality, gene expression, stress defense, and oxidative stress effects) (Balbi et al., 2017; Liu Z et al., 2018; Yu et al., 2018; Sendra et al., 2020; Capolupo et al., 2021). Corals readily ingest polypropylene microplastics upon exposure to plastic particles, resulting in a variety of biological implications ranging from feeding dysfunction to mucus formation and distorted gene expression (Corinaldesi et al., 2021). These multiple effects mean that microplastics, at the concentrations seen in some marine areas and expected for most oceans in the coming decades, could lead to the death and eventual extinction of corals. Other larger kinds of plastics including meso- and macroplastics have been reported to cause the entanglement of marine organisms which could result in suffocation, starvation, exhaustion, injuries, decreased reproduction rates, and death (UNEP and NOAA, 2015).
Moreover, human consumption of microplastic particles via ingestion of contaminated drinking water, unsafe seafood, or trophic transfer, inhalation and dermal exposure routes is now well documented (Barboza et al., 2018; Atugoda et al., 2020). However, the fate and impacts of microplastics after they enter the human body are still under investigation.
Concluding Remarks and Outlook
The mechanisms controlling the sorption of organic compounds on plastic polymers are not fully understood yet as most studies are carried out indoors under conditions that represent the real environment. However, hydrophobicity of POPs, the surface area of plastic polymer, and polymer makeup are three significant factors that influence the sorption of POPs on plastic polymers. Hydrophobicity of organic compounds increases the likelihood of OCs getting sorbed onto plastic polymers rather than dissolving in water, a surface area, especially that of aging MPs contributes to higher sorption capacity of plastic polymer. Plastic polymers have different affinities for different organic compounds Additives in MPs pose threats on marine life as studies show they are capable of leaching into organs of marine life like fishes after they have been picked up as food, there is a possibility of some of these additives increasing concentrations of toxic chemicals in the environment. There is no fixed order of sorption capacity for plastic polymers as sorption for each polymer varies depending on the interaction between the polymers and that of hydrophobic organic contaminants. However, PE from recent studies has the highest sorption capacity for organic compounds. In contrast, PVC has the lowest sorption capacity due to their glassy make up and possesses substantial adsorption sites that result in lower desorption rates. Many kinetic and isothermal models including first-order adsorption kinetic model, pseudo-second-order model, intraparticle diffusion model, film diffusion model, linear model, Freundlich model, Langmuir model, Temkin model and Elovich adsorption model have been used in understanding the sorbate-sorbent adsorption mechanisms, more studies are still required to investigate the most suitable models for different microplastic and nanoplastic types in order to fully appreciate their adsorption peculiarities.
Mathematical models that relate the real aquatic conditions to that of the laboratory set up should be developed to help for better prediction of sorption processes that can be fully trusted as it is only then that feasible solutions can be proposed. Since aging of microplastics contributes significantly to their sorption capacity, more sorption studies on naturally aged plastics should be carried out as all microplastics ultimately age. Studies should be carried out on bioavailability of organic compounds making up microplastics like microbeads found in toothpaste and how long it takes for leaching of these OCs into the human organs to occur if these compounds are not passed out as feces soon enough. More adsorption and desorption studies of organic compounds found in microplastics and nanoplastics are needed to understand their fate, bioavailability, and sorption distinctions. The non-biodegradability of most plastics is due carbon to carbon bonds which microorganisms have not evolved enough to break. Since plastics are essential, more studies that generate substances that serve as weak links between these bonds that could help to biodegrade by microorganisms should be carried out.
Author Contributions
NB conceptualized and supervised the study, OA and NB led the writing of the manuscript, NB reviewed and edited the submitted manuscript, which was approved by all authors.
Funding
This research was supported by the Covenant University Center for Research and Innovation (CUCRID), through the Covenant University Seed Grant.
Conflict of Interest
The authors declare that the research was conducted in the absence of any commercial or financial relationships that could be construed as a potential conflict of interest.
Acknowledgments
The authors acknowledge the invaluable contributions of reviewers.
Abbreviations
MPs, microplastics; MNPs, micro (nano) plastics; PMPs, primary microplastics; SMPs, secondary microplastics; PE, polyethylene; PP, polypropylene; PVC, polyvinyl chloride; PA, polyamide; PS, polystyrene; PU, polyurethane; PET, polyethylene terephthalate; LDPE, low density polyethylene; HDPE, high density polyethylene; POM, polyoxymethylene; POM, polyoxymethylene; PLA, polylactic acid; DOM, dissolved organic matter; HOCs, hydrophobic organic contaminants; PPCPs, pharmaceuticals and personal care products; MS, Mass spectroscopy; GC-MS, Gas chromatography—Mass spectroscopy; GC-TOF-MS, Gas chromatography coupled to time-of-flight mass spectrometry; UPLC—QMS, Ultra-performance liquid chromatography—Quadrupole mass spectrometry; HS-SPME/GC-ITMS, Headspace solid phase micro extraction coupled with gas chromatography/ion trap mass spectrometry; HPLC, high-performance liquid chromatography; GC-QqQ-MS, Gas chromatograph triple-quadrupole mass spectrometer; PCB, polychlorinated biphenyl; DEHP, di(2-ethylhexyl) phthalate; DnBP, dibutyl phthalate; DMP, dimethyl phthalatePAHs, polycyclic aromatic hydrocarbons; PBDEs, polybrominated diphenyl ethers; DDT, dichlorodiphenyltrichloroethane; PBDEs, polybrominated diphenyl ethers; DDE, dichlorodiphenyl dichloroethene; NP, nitrophenol; 4-MBC, 4-methylbenzylidene camphor; PAEs, phthalate Esters; BaP, benzo[a]pyrene; PFOS, perfluorooctane sulfonic acid; OPEs, organophosphorus esters; TH, tetracycline hydrochloride; 9-NAnt, 9-Nitroanthracene; HEX, hexaconazole; MYC, myclobutanil; TRI, triadimenol; ACE, acetamiprid; CAP, chlorantraniliprole; FLU, flubendiamide; HCHs, 4 hexachlorocyclohexanes; CBs, 2 chlorinated benzenes; EVA, ethylene vinyl acetate; ABS, acrylonitrile butadiene styrene; SDZ, sulfadiazine; AMX, amoxicillin; TC, tetracycline; CIP, ciprofloxacin; TMP, trimethoprim; BPS, bisphenol S; 4.4′-sulfonyldiphenol; BPF, bisphenol F; 4.4′-dihydroxydiphenylmethane); BPAF, bisphenol AF; 4.4′-(hexafluoroisopropylidene) diphenol]; bisphenol B [BPB; 2,2-bis(4-hydroxyphenyl) butane]; ROX, roxithromycin; TCS, triclosan; OPFRs, organophosphate flame retardants; PFCs, perfluorinated compounds; BFRs, brominated flame retardants; BDE-209, decabromodiphenyl ether; WWTPs, wastewater treatment plants; HBCD, hexabromocyclododecane; Kf, Freundlich partition coefficient; Kow, octanol-water partition coefficient; Kd, Langmuir partition coefficient; FA, fulvic acid; HA, humic acid; FDM, film diffusion kinetic model.
References
Adika, S. A., Mahu, E., Crane, R., Marchant, R., Montford, J., Folorunsho, R., et al. (2020). Microplastic Ingestion by Pelagic and Demersal Fish Species from the Eastern Central Atlantic Ocean, off the Coast of Ghana. Mar. Pollut. Bull. 153, 110998. doi:10.1016/j.marpolbul.2020.110998
Alberti, G., Amendola, V., Pesavento, M., and Biesuz, R. (2012). Beyond the Synthesis of Novel Solid Phases: Review on Modelling of Sorption Phenomena. Coord. Chem. Rev. 256, 28–45. doi:10.1016/j.ccr.2011.08.022
Allen, T., Farley, S., Draper, J., Clement, C., and Polidoro, B. (2018). Variations in Sorption of Organochlorine Pesticides and PCBs across Six Different Plastic Polymers. J. Environ. Toxicol. Stud. 2, 1–6. doi:10.16966/2576-6430.109
Allsopp, M. W., and Vianello, G. (2000). Poly (Vinyl Chloride). Ullmann's Encyclopedia of Industrial Chemistry. Weinheim, Germany:Wiley Online Library. doi:10.1002/14356007.a21_717
Andrady, A. L. (2011). Microplastics in the marine Environment. Mar. Pollut. Bull. 62 (8), 1596–1605. doi:10.1016/j.marpolbul.2011.05.030
Ashton, K., Holmes, L., and Turner, A. (2010). Association of Metals with Plastic Production Pellets in the marine Environment. Mar. Pollut. Bull. 60 (11), 2050–2055. doi:10.1016/j.marpolbul.2010.07.014
Ašmonaitė, G., Tivefälth, M., Westberg, E., Magnér, J., Backhaus, T., and Almroth, B. C. (2020). Microplastics as a Vector for Exposure to Hydrophobic Organic Chemicals in Fish: a Comparison of Two Polymers and Silica Particles Spiked with Three Model Compounds. Front. Environ. Sci. 8, 87. doi:10.3389/fenvs.2020.00087
Atugoda, T., Wijesekara, H., Werellagama, D. R. I. B., Jinadasa, K. B. S. N., Bolan, N. S., and Vithanage, M. (2020). Adsorptive Interaction of Antibiotic Ciprofloxacin on Polyethylene Microplastics: Implications for Vector Transport in Water. Environ. Technol. Innovation 19, 100971. doi:10.1016/j.eti.2020.100971
Avio, C. G., Gorbi, S., and Regoli, F. (2017). Plastics and microplastics in the oceans: From emerging pollutants to emerged threat. Marine Environ. Res. 128, 2–11.
Ayawei, N., Ebelegi, A. N., and Wankasi, D. (2017). Modelling and Interpretation of Adsorption Isotherms. J. Chem. 2017, 11. doi:10.1155/2017/3039817
Bakir, A., Desender, M., Wilkinson, T., Van Hoytema, N., Amos, R., Airahui, S., et al. (2020). Occurrence and Abundance of Meso and Microplastics in Sediment, Surface Waters, and marine Biota from the South Pacific Region. Mar. Pollut. Bull. 160, 111572. doi:10.1016/j.marpolbul.2020.111572
Bakir, A., Rowland, S. J., and Thompson, R. C. (2012). Competitive Sorption of Persistent Organic Pollutants onto Microplastics in the marine Environment. Mar. Pollut. Bull. 64 (12), 2782–2789. doi:10.1016/j.marpolbul.2012.09.010
Bakir, A., Rowland, S. J., and Thompson, R. C. (2014). Enhanced Desorption of Persistent Organic Pollutants from Microplastics under Simulated Physiological Conditions. Environ. Pollut. 185, 16–23. doi:10.1016/j.envpol.2013.10.007
Balbi, T., Camisassi, G., Montagna, M., Fabbri, R., Franzellitti, S., Carbone, C., et al. (2017). Impact of Cationic Polystyrene Nanoparticles (PS-NH2) on Early Embryo Development of Mytilus galloprovincialis: Effects on Shell Formation. Chemosphere 186, 1–9. doi:10.1016/j.chemosphere.2017.07.120
Barboza, L. G. A., Dick Vethaak, A., Lavorante, B. R. B. O., Lundebye, A.-K., and Guilhermino, L. (2018). Marine Microplastic Debris: An Emerging Issue for Food Security, Food Safety and Human Health. Mar. Pollut. Bull. 133, 336–348. doi:10.1016/j.marpolbul.2018.05.047
Barletta, M., Lima, A. R. A., and Costa, M. F. (2019). Distribution, Sources and Consequences of Nutrients, Persistent Organic Pollutants, Metals and Microplastics in South American Estuaries. Sci. Total Environ. 651, 1199–1218. doi:10.1016/j.scitotenv.2018.09.276
Benjamin, S., Masai, E., Kamimura, N., Takahashi, K., Anderson, R. C., and Faisal, P. A. (2017). Phthalates impact human health: epidemiological evidences and plausible mechanism of action. J. Hazard. Mater. 340, 360–383.
Benson, N. U., and Fred-Ahmadu, O. H. (2020). Occurrence and Distribution of Microplastics-Sorbed Phthalic Acid Esters (PAEs) in Coastal Psammitic Sediments of Tropical Atlantic Ocean, Gulf of Guinea. Sci. Total Environ. 730, 139013. doi:10.1016/j.scitotenv.2020.139013
Benson, N. U., Fred-Ahmadu, O. H., Ekett, S. I., Basil, M. O., Adebowale, A. D., Adewale, A. G., et al. (2020). Occurrence, Depth Distribution and Risk Assessment of PAHs and PCBs in Sediment Cores of Lagos Lagoon, Nigeria. Reg. Stud. Mar. Sci. 37, 101335. doi:10.1016/j.rsma.2020.101335
Besseling, E., Foekema, E. M., Van Franeker, J. A., Leopold, M. F., Kühn, S., Bravo Rebolledo, E. L., et al. (2015). Microplastic in a Macro Filter Feeder: Humpback Whale Megaptera Novaeangliae. Mar. Pollut. Bull. 95 (1), 248–252. doi:10.1016/j.marpolbul.2015.04.007
Besseling, E., Redondo-Hasselerharm, P., Foekema, E. M., and Koelmans, A. A. (2019). Quantifying Ecological Risks of Aquatic Micro- and Nanoplastic. Crit. Rev. Environ. Sci. Technol. 49, 32–80. doi:10.1080/10643389.2018.1531688
Besseling, E., Wegner, A., Foekema, E. M., Van Den Heuvel-Greve, M. J., and Koelmans, A. A. (2013). Effects of Microplastic on Fitness and PCB Bioaccumulation by the Lugworm Arenicola marina (L.). Environ. Sci. Technol. 47 (1), 593–600. doi:10.1021/es302763x
Blanchard, G., Maunaye, M., and Martin, G. (1984). Removal of Heavy Metals from Waters by Means of Natural Zeolites. Water Res. 18, 1501–1507. doi:10.1016/0043-1354(84)90124-6
Boerger, C. M., Lattin, G. L., Moore, S. L., and Moore, C. J. (2010). Plastic Ingestion by Planktivorous Fishes in the North Pacific Central Gyre. Mar. Pollut. Bull. 60, 2275–2278. doi:10.1016/j.marpolbul.2010.08.007
Boucher, J., and Friot, D. (2017). Primary Microplastics in the Oceans: A Global Evaluation of Sources (Pp. 2017–002). Gland, Switzerland: IUCN.
Bouwmeester, H., Hollman, P. C. H., and Peters, R. J. B. (2015). Potential Health Impact of Environmentally Released Micro- and Nanoplastics in the Human Food Production Chain: Experiences from Nanotoxicology. Environ. Sci. Technol. 49 (2015), 8932–8947. doi:10.1021/acs.est.5b01090
Boyd, G. E., Adamson, A. W., and Myers, L. S. (1947). The Exchange Adsorption of Ions from Aqueous Solutions by Organic Zeolites. II. Kinetics. J. Am. Chem. Soc. 69, 2836. doi:10.1021/ja01203a066
Brach, L., Deixonne, P., Bernard, M.-F., Durand, E., Desjean, M.-C., Perez, E., et al. (2018). Anticyclonic Eddies Increase Accumulation of Microplastic in the North Atlantic Subtropical Gyre. Mar. Pollut. Bull. 126, 191–196. doi:10.1016/j.marpolbul.2017.10.077
Brennecke, D., Ferreira, E. C., Costa, T. M. M., Appel, D., da Gama, B. A. P., and Lenz, M. (2015). Ingested Microplastics (>100μm) Are Translocated to Organs of the Tropical Fiddler Crab Uca Rapax. Mar. Pollut. Bull. 96 (1–2), 491–495. doi:10.1016/j.marpolbul.2015.05.001
C. Lynwood (Editor) (2014). Polystyrene: Synthesis, Characteristics, and Applications (Nova Science Publishers, Inc).
Caliman, F. A., and Gavrilescu, M. (2009). Pharmaceuticals, Personal Care Products and Endocrine Disrupting Agents in the Environment - A Review. Clean. - Soil Air Water 37, 277–303. doi:10.1002/clen.200900038
Capolupo, M., Valbonesi, P., and Fabbri, E. (2021). A Comparative Assessment of the Chronic Effects of Micro- and Nano-Plastics on the Physiology of the Mediterranean Mussel Mytilus galloprovincialis. Nanomaterials (Basel, Switzerland) 11 (3), 649. doi:10.3390/nano11030649
Carrington, D. (2017). Plastic Fibres Found in Tap Water Around the World, Study Reveals. Available at: https://www.theguardian.com/environment/2017/sep/06/plastic-fibres-found-tap-water-around-world-study-reveals (Accessed August 20, 2020).
Cazetta, A. L., Vargas, A. M. M., Nogami, E. M., Kunita, M. H., Guilherme, M. R., Martins, A. C., et al. (2011). NaOH-activated Carbon of High Surface Area Produced from Coconut Shell: Kinetics and Equilibrium Studies from the Methylene Blue Adsorption. Chem. Eng. J. 174, 117–125. doi:10.1016/j.cej.2011.08.058
Chapron, L., Lartaud, F., Le Bris, N., Peru, E., and Galand, P. E. (2020). Local Variability in Microbiome Composition and Growth Suggests Habitat Preferences for Two Reef-Building Cold-Water Coral Species. Front. Microbiol. 11, 275. doi:10.3389/fmicb.2020.00275
Chen, C. F., Ju, Y. R., Lim, Y. C., Hsu, N. H., Lu, K. T., Hsieh, S. L., et al. (2020). Microplastics and Their Affiliated PAHs in the Sea Surface Connected to the Southwest Coast of Taiwan. Chemosphere 254, 126818. doi:10.1016/j.chemosphere.2020.126818 &
Chen, Z., Wang, J., Pu, Z., Zhao, Y., Jia, D., Chen, H., et al. (2017). Synthesis of Magnetic Fe3O4/CFA Composites for the Efficient Removal of U(VI) from Wastewater. Chem. Eng. J. 320 (2017), 448–457. doi:10.1016/j.cej.2017.03.074
Choi, Y. K., Choi, T. R., Gurav, R., Bhatia, S. K., Park, Y. L., Kim, H. J., et al. (2020). Adsorption Behavior of Tetracycline onto Spirulina Sp. (Microalgae)-derived Biochars Produced at Different Temperatures. Sci. Total Environ. 710, 136282. doi:10.1016/j.scitotenv.2019.136282
Cole, M., Liddle, C., Consolandi, G., Drago, C., Hird, C., Lindeque, P. K., et al. (2020). Microplastics, Microfibres and Nanoplastics Cause Variable Sub-lethal Responses in Mussels (Mytilus spp.). Mar. Pollut. Bull. 160, 111552. doi:10.1016/j.marpolbul.2020.111552
Cole, M., Lindeque, P., Fileman, E., Halsband, C., Goodhead, R., Moger, J., et al. (2013). Microplastic Ingestion by Zooplankton. Environ. Sci. Technol. 47 (12), 6646–6655. doi:10.1021/es400663f
Cole, M., Lindeque, P., Halsband, C., and Galloway, T. S. (2011). Microplastics as Contaminants in the marine Environment: a Review. Mar. Pollut. Bull. 62 (12), 2588–2597. doi:10.1016/j.marpolbul.2011.09.025
Corinaldesi, C., Canensi, S., Dell’Anno, A., Tangherlini, M., Di Capua, I., Varrella, A., et al. (2021). Multiple Impacts of Microplastics Can Threaten marine Habitat-Forming Species. Commun. Biol. 4, 43. doi:10.1038/s42003-021-01961-1
da Costa, J. P., Santos, P. S., Duarte, A. C., and Rocha-Santos, T. (2016). (Nano) plastics in the environment-sources, fates and effects. Sci. Total Environ. 566, 15–26.
Daugherty, M., Conte, M., and Weber, J. C. (2016). “Adsorption of Organic Pollutants to Microplastics: the Effects of Dissolved Organic Matter,” in Semester in Environmental Science (Nahant, MA: Northwestern University), 1–27.
de Souza Machado, A. A., Kloas, W., Zarfl, C., Hempel, S., and Rillig, M. C. (2018). Microplastics as an Emerging Threat to Terrestrial Ecosystems. Glob. Change Biol. 24 (4), 1405–1416. doi:10.1111/gcb.14020
Deng, Y., Yan, Z., Shen, R., Wang, M., Huang, Y., Ren, H., et al. (2020). Microplastics Release Phthalate Esters and Cause Aggravated Adverse Effects in the Mouse Gut. Environ. Int. 143, 105916. doi:10.1016/j.envint.2020.105916
Deng, Y., Zhang, Y., Lemos, B., and Ren, H. (2017). Tissue Accumulation of Microplastics in Mice and Biomarker Responses Suggest Widespread Health Risks of Exposure. Scientific Rep. 7, 46687. doi:10.1038/srep46687
Dey, S., Bano, F., and Malik, A. (2019). “Pharmaceuticals and Personal Care Product (PPCP) Contamination—A Global Discharge Inventory,” in Pharmaceuticals and Personal Care Products: Waste Management and Treatment Technology. Editors M. N. V. Prasad, M. Vithanage, and A. Kapley, (Butterworth-Heinemann), 1–26.
Diamanti-Kandarakis, E., Bourguignon, J. P., Giudice, L. C., Hauser, R., Prins, G. S., Soto, A. M., et al. (2009). Endocrine-disrupting Chemicals: An Endocrine Society Scientific Statement. Endocr. Rev. 30 (4), 293–342. doi:10.1210/er.2009-0002
Ding, J., Huang, Y., Liu, S., Zhang, S., Zou, H., Wang, Z., et al. (2020). Toxicological Effects of Nano- and Micro-polystyrene Plastics on Red tilapia: Are Larger Plastic Particles More Harmless? J. Hazard. Mater. 396, 122693. doi:10.1016/j.jhazmat.2020.122693
Dmitrienko, S. G., Gurariy, E. Y., Nosov, R. E., and Zolotov, Y. A. (2001). Solid-phase Extraction of Polycyclic Aromatic Hydrocarbons from Aqueous Samples Using Polyurethane Foams in Connection with Solid-Matrix Spectrofluorimetry. Anal. Lett. 34 (3), 425–438. doi:10.1081/al-100102584
Dris, R., Gasperi, J., Mirande, C., Mandin, C., Guerrouache, M., Langlois, V., et al. (2017). A First Overview of Textile Fibers, Including Microplastics, in Indoor and Outdoor Environments. Environ. Pollut. 221, 453–458. doi:10.1016/j.envpol.2016.12.013
Dubinin, M. M., and Radushkevich, L. V. (1947). The Equation of the Characteristic Curve of the Activated Charcoal. Proc. Acad. Sci. USSR Phys. Chem. Sect. 55, 331–337.
Dąbrowski, A. (2001). Adsorption–from Theory to Practice. Adv. Colloid Interf. Sci. 93, 135–224. doi:10.1016/s0001-8686(00)00082-8
Ebele, A. J., Abdallah, M. A. E., and Harrad, S. (2017). Pharmaceuticals and Personal Care Products (PPCPs) in the Freshwater Aquatic Environment. Emerg. Contam. 3, 1–16. doi:10.1016/j.emcon.2016.12.004
Ekvall, M. T., Lundqvist, M., Kelpsiene, E., Šileikis, E., Gunnarsson, S. B., and Cedervall, T. (2019). Nanoplastics Formed during the Mechanical Breakdown of Daily-Use Polystyrene Products. Nanoscale Adv. 1, 1055–1061. doi:10.1039/C8NA00210J
El Hadri, H., Lisa, J. M., Gigault, J., Reynaud, S., and Grassl, B. (2020). Fate of Nanoplastics in the Environment: Implication of the Cigarette Butts. Environ. Pollut. 268, 115170. doi:10.1016/j.envpol.2020.115170
Elizalde-Velázquez, A., Subbiah, S., Anderson, T. A., Green, M. J., Zhao, X., and Cañas-Carrell, J. E. (2020). Sorption of Three Common Nonsteroidal Anti-inflammatory Drugs (NSAIDs) to Microplastics. Sci. Total Environ. 715, 136974. doi:10.1016/j.scitotenv.2020.136974
Elovich, S. Y., and Larinov, O. G. (1962). Theory of Adsorption from Solutions of Nonelectrolytes on Solid (I) Equation Adsorption from Solutions and the Anal- Ysis of its Simplest Form, (II) Verification of the Equation of Adsorption Isotherm from Solutions, Izv. Akad. Nauk. SSSR. Otd. Khim. Nauk 2, 209–216.
Endo, S., Takizawa, R., Okuda, K., Takada, H., Chiba, K., Kanehiro, H., et al. (2005). Concentration of Polychlorinated Biphenyls (PCBs) in Beached Resin Pellets: Variability Among Individual Particles and Regional Differences. Mar. Pollut. Bull. 50 (10), 1103–1114. doi:10.1016/j.marpolbul.2005.04.030
Eriksen, M., Lebreton, L. C., Carson, H. S., Thiel, M., Moore, C. J., Borerro, J. C., et al. (2014). Plastic Pollution in the World's Oceans: More Than 5 Trillion Plastic Pieces Weighing over 250,000 Tons Afloat at Sea. PLoS One 9 (12), e111913. doi:10.1371/journal.pone.0111913
Everaert, G., De Rijcke, M., Lonneville, B., Janssen, C. R., Backhaus, T., Mees, J., et al. (2020). Risks of Floating Microplastic in the Global Ocean. Environ. Pollut. 267, 115499. doi:10.1016/j.envpol.2020.115499
Fahrenkamp-Uppenbrink, J. (2018). Microplastics Everywhere. Science 360 (44), 17–46. doi:10.1126/science.360.6384.44-q
Fan, X., Zou, Y., Geng, N., Liu, J., Hou, J., Li, D., et al. (2020). Investigation on the Adsorption and Desorption Behaviors of Antibiotics by Degradable MPs with or without UV Ageing Process. J. Hazard. Mater. 401, 123363. doi:10.1016/j.jhazmat.2020.123363
Fang, S., Yu, W., Li, C., Liu, Y., Qiu, J., and Kong, F. (2019). Adsorption Behavior of Three Triazole Fungicides on Polystyrene Microplastics. Sci. Total Environ. 691, 1119–1126. doi:10.1016/j.scitotenv.2019.07.176
Farrell, P., and Nelson, K. (2013). Trophic Level Transfer of Microplastic: Mytilus edulis (L.) to Carcinus maenas (L.). Environ. Pollut. 177, 1–3. doi:10.1016/j.envpol.2013.01.046
Fendall, L. S., and Sewell, M. A. (2009). Contributing to marine pollution by washing your face: microplastics in facial cleansers. Mar. Pollut. Bull. 58, 1225–1228.
Foo, K. Y., and Hameed, B. H. (2010). Insights into the Modeling of Adsorption Isotherm Systems. Chem. Eng. J. 156, 2–10. doi:10.1016/j.cej.2009.09.013
Fotopoulou, K. N., and Karapanagioti, H. K. (2012). Surface Properties of Beached Plastic Pellets. Mar. Environ. Res. 81, 70–77. doi:10.1016/j.marenvres.2012.08.010
Fraser, M. A., Chen, L., Ashar, M., Huang, W., Zeng, J., Zhang, C., et al. (2020). Occurrence and Distribution of Microplastics and Polychlorinated Biphenyls in Sediments from the Qiantang River and Hangzhou Bay, China. Ecotoxicology Environ. Saf. 196, 110536. doi:10.1016/j.ecoenv.2020.110536
Fred-Ahmadu, O. H., Ayejuyo, O. O., and Benson, N. U. (2020a). Dataset on Microplastics and Associated Trace Metals and Phthalate Esters in sandy Beaches of Coastal Atlantic Ecosystem of Lagos, Nigeria. Data in Brief 31, 105755. doi:10.1016/j.dib.2020.105755
Fred-Ahmadu, O. H., Ayejuyo, O. O., and Benson, N. U. (2020b). Microplastics Distribution and Characterization in Epipsammic Sediments of Tropical Atlantic Ocean, Nigeria. Reg. Stud. Mar. Sci. 38, 101365. doi:10.1016/j.rsma.2020.101365
Fred-Ahmadu, O. H., Bhagwat, G., Oluyoye, I., Benson, N. U., Ayejuyo, O. O., and Palanisami, T. (2020c). Interaction of Chemical Contaminants with Microplastics: Principles and Perspectives. Sci. Total Environ. 706, 135978. doi:10.1016/j.scitotenv.2019.135978
Frias, J. P. G. L., Sobral, P., and Ferreira, A. M. (2010). Organic Pollutants in Microplastics from Two Beaches of the Portuguese Coast. Mar. Pollut. Bull. 60 (11), 1988–1992. doi:10.1016/j.marpolbul.2010.07.030
Frick, A., and Stern, C. (2013). DSC-Prüfung in der Anwendung. Carl Hanser Verlag GmbH Co KG. doi:10.3139/9783446436923
Fries, E., and Zarfl, C. (2012). Sorption of Polycyclic Aromatic Hydrocarbons (PAHs) to Low and High Density Polyethylene (PE). Environ. Sci. Pollut. Res. 19 (2012), 1296–1304. doi:10.1007/s11356-011-0655-5
Frost, K., Kaminski, D., Kirwan, G., Lascaris, E., and Shanks, R. (2009). Crystallinity and Structure of Starch Using Wide Angle X-ray Scattering. Carbohydr. Polym. 78 (3), 543–548. doi:10.1016/j.carbpol.2009.05.018
Garcia-Garin, O., Vighi, M., Sala, B., Aguilar, A., Tsangaris, C., Digka, N., et al. (2020). Assessment of Organophosphate Flame Retardants in Mediterranean Boops boops and Their Relationship to Anthropization Levels and Microplastic Ingestion. Chemosphere 252, 126569. doi:10.1016/j.chemosphere.2020.126569
GESAMP, S. (2015). Fate and effects of microplastics in the marine environment: a global assessment. IMO/FAO/UNESCO-IOC/UNIDO/WMO/IAEA/UN/UNEP/UNDP Joint Group of Experts on the Scientific Aspects of Marine Environmental Protection), Rep. Stud. GESAMP, 96.
Gigault, J., El Hadri, H., Reynaud, S., Deniau, E., and Grassl, B. (2017). Asymmetrical Flow Field Flow Fractionation Methods to Characterize Submicron Particles: Application to Carbon-Based Aggregates and Nanoplastics. Anal. Bioanal. Chem. 409 (29), 6761–6769. doi:10.1007/s00216-017-0629-7
Gigault, J., ter Halle, A., Baudrimont, M., Pascal, P-Y., Gauffre, F., Phi, T-L., et al. (2018). Current Opinion: What Is a Nanoplastic? Environ. Pollut. 235, 1030–1034. doi:10.1016/j.envpol.2018.01.024
Goedecke, C., Mülow-Stollin, U., Hering, S., Richter, J., Piechotta, C., Paul, A., et al. (2017). A First Pilot Study on the Sorption of Environmental Pollutants on Various Microplastic Materials,. J. Environ. Anal. Chem. 4, 191. doi:10.41722380/2391.1000191
Gonçalves, J. O., da Silva, K. A., Rios, E. C., Crispim, M. M., Dotto, G. L., and Pinto, L. A. A. (2020). Chitosan Hydrogel Scaffold Modified with Carbon Nanotubes and its Application for Food Dyes Removal in Single and Binary Aqueous Systems. Int. J. Biol. Macromol. 142, 85–93. doi:10.1016/j.ijbiomac.2019.09.074
Granby, K., Rainieri, S., Rasmussen, R. R., Kotterman, M. J., Sloth, J. J., Cederberg, T. L., et al. (2018). The Influence of Microplastics and Halogenated Contaminants in Feed on Toxicokinetics and Gene Expression in European Seabass (Dicentrarchus labrax). Environ. Res. 164, 430–443. doi:10.1016/j.envres.2018.02.035
Grossman, E. (2015). How plastics from your clothes can end up in your fish. TIME, USA 15 Jan. 2015, http://time.com/3669084/plastics-pollution-fish/.
Guo, X., Liu, Y., and Wang, J. (2019). Sorption of Sulfamethazine onto Different Types of Microplastics: A Combined Experimental and Molecular Dynamics Simulation Study,. Mar. Pollut. Bull. 145, 547–554. doi:10.1016/j.marpolbul.2019.06.063
Guo, X., Pang, J., Chen, S., and Jia, H. (2018). Sorption Properties of Tylosin on Four Different Microplastics. Chemosphere 209, 240–245. doi:10.1016/j.chemosphere.2018.06.100
Guo, X., and Wang, J. (2019). Sorption of Antibiotics onto Aged Microplastics in Freshwater and Seawater. Mar. Pollut. Bull. 149, 110511. doi:10.1016/j.marpolbul.2019.110511
Ha, J., and Yeo, M. (2018). The Environmental Effects of Microplastics on Aquatic Ecosystems. Mol. Cel. Toxicol. 14, 353–359. doi:10.1007/s13273-018-0039-8
Hahladakis, J. N., Velis, C. A., Weber, R., Iacovidou, E., and Purnell, P. (2018). An Overview of Chemical Additives Present in Plastics: Migration, Release, Fate and Environmental Impact during Their Use, Disposal and Recycling. J. Hazard. Mater. 344, 179–199. doi:10.1016/j.jhazmat.2017.10.014
Halsey, G. D. (1952). , 4, 259–269. doi:10.1016/S0360-0564(08)60616-1 The Role of Surface Heterogeneity in AdsorptionAdv. Catal.
Hamdaoui, O., and Naffrechoux, E. (2007). Modeling of Adsorption Isotherms of Phenol and Chlorophenols onto Granular Activated Carbon: Part I. Two-Parameter Models and Equations Allowing Determination of Thermodynamic Parameters. J. Hazard. Mater. 147 (1–2), 381–394. doi:10.1016/j.jhazmat.2007.01.021
Hameed, B. H., Tan, I. A. W., and Ahmad, A. L. (2008). Adsorption Isotherm, Kinetic Modeling and Mechanism of 2,4,6-trichlorophenol on Coconut Husk-Based Activated Carbon. Chem. Eng. J. 144, 235–244. doi:10.1016/j.cej.2008.01.028
Hämer, J., Gutow, L., Köhler, A., and Saborowski, R. (2014). Fate of Microplastics in the marine Isopod Idotea Emarginata. Environ. Sci. Technol. 48 (22), 13451–13458. doi:10.1021/es501385y
Han, S., Bang, J., Choi, D., Hwang, J., Kim, T., Oh, Y., et al. (2020). Surface Pattern Analysis of Microplastics and Their Impact on Human-Derived Cells. ACS Appl. Polym. Mater. 2 (11), 4541–4550. doi:10.1021/acsapm.0c00645
Harley, K. G., Marks, A. R., Chevrier, J., Bradman, A., Sjödin, A., and Eskenazi, B. (2010). PBDE Concentrations in Women’s Serum and Fecundability. Environ. Health Perspect. 118 (5), 699–704. doi:10.1289/ehp.0901450
Hartmann, N. B., Hüffer, T., Thompson, R. C., Hassellöv, M., Verschoor, A., Daugaard, A. E., et al. (2019). Are We Speaking the Same Language? Recommendations for a Definition and Categorization Framework for Plastic Debris. Environ. Sci. Technol. 53, 1039–1047. doi:10.1021/acs.est.8b05297
Hartmann, N., Rist, S., Bodin, J., Jensen, L., Schmidt, S., Mayer, P., et al. (2017). Microplastics as Vectors for Environmental Contaminants: Exploring Sorption, Desorption, and Transfer to Biota. Integrated Environ. Assess. Manage. 13, 488–493. doi:10.1002/ieam.1904
Hasenmueller, E. A., Martin, K. M., Conkle, J. L., and White, J. R. (2017). Assessing Microplastic Loads in the Mississippi River and its Major Tributaries. AGUFM 2017, H31H–H1603.
Heindler, F. M., Alajmi, F., Huerlimann, R., Zeng, C., Newman, S. J., Vamvounis, G., et al. (2017). Toxic Effects of Polyethylene Terephthalate Microparticles and Di(2-Ethylhexyl)phthalate on the Calanoid Copepod, Parvocalanus Crassirostris. Ecotoxicology Environ. Saf. 141, 298–305. doi:10.1016/j.ecoenv.2017.03.029
Heinrich, P., Hanslik, L., Kämmer, N., and Braunbeck, P. (2020). The tox is in the detail: technical fundamentals for designing, performing, and interpreting experiments on toxicity of microplastics and associated substances. Environ. Sci. Pollut. Res. 27, 22292–22318.
Hirai, H., Takada, H., Ogata, Y., Yamashita, R., Mizukawa, K., Saha, M., et al. (2011). Organic Micropollutants in marine Plastics Debris from the Open Ocean and Remote and Urban Beaches. Mar. Pollut. Bull. 62 (8), 1683–1692. doi:10.1016/j.marpolbul.2011.06.004
Ho, B. T., Roberts, T. K., and Lucas, S. (2017). An overview on biodegradation of polystyrene and modified polystyrene: the microbial approach. Critic. Rev. Biotechnol. 38, 308–320.
Holahan, M. R., and Smith, C. A. (2015). Phthalates and Neurotoxic Effects on Hippocampal Network Plasticity. Neurotoxicology 48, 21–34. doi:10.1016/j.neuro.2015.02.008
Holmes, L. A., Turner, A., and Thompson, R. C. (2012). Adsorption of Trace Metals to Plastic Resin Pellets in the marine Environment. Environ. Pollut. 160, 42–48. doi:10.1016/j.envpol.2011.08.052
Hu, Q., and Zhang, Z. (2019). Application of Dubinin–Radushkevich Isotherm Model at the Solid/solution Interface: A Theoretical Analysis,. J. Mol. Liquids 277, 646–648. doi:10.1016/j.molliq.2019.01.005
Hu, X.-J., Wang, J.-S., Liu, Y.-G., Li, X., Zeng, G.-M., Bao, Z.-L., et al. (2011). Adsorption of Chromium (VI) by Ethylenediamine-Modified Cross- Linked Magnetic Chitosan Resin: Isotherms, Kinetics and Thermodynamics. J. Hazard. Mater. 185, 306–314. doi:10.1016/j.jhazmat.2010.09.034
Hüffer, T., and Hofmann, T. (2016). Sorption of Non-polar Organic Compounds by Micro-sized Plastic Particles in Aqueous Solution. Environ. Pollut. 214, 194–201. doi:10.1016/j.envpol.2016.04.018
Hüffer, T., Weniger, A-K., and Hofmann, T. (2018). Sorption of Organic Compounds by Aged Polystyrene Microplastic Particles. Environ. Pollut. 236, 218–225. doi:10.1016/j.envpol.2018.01.022
Inglezakis, V. J. (2007). Solubility-normalized Dubinin–Astakhov Adsorption Isotherm for Ion-Exchange Systems. Microporous Mesoporous Mater. 103, 72–81. doi:10.1016/j.micromeso.2007.01.039
Issac, M. N., and Kandasubramanian, B. (2021). Effect of Microplastics in Water and Aquatic Systems. Environ. Sci. Pollut. Res. 28, 19544. doi:10.1007/s11356-021-13184-2
Jaikumar, G., Brun, N. R., Vijver, M. G., and Bosker, T. (2019). Reproductive Toxicity of Primary and Secondary Microplastics to Three Cladocerans during Chronic Exposure. Environ. Pollut. 249, 638–646. doi:10.1016/j.envpol.2019.03.085
Jang, M., Shim, W. J., Han, G. M., Rani, M., Song, Y. K., and Hong, S. H. (2017). Widespread Detection of a Brominated Flame Retardant, Hexabromocyclododecane, in Expanded Polystyrene marine Debris and Microplastics from South Korea and the Asia-Pacific Coastal Region. Environ. Pollut. 231, 785–794. doi:10.1016/j.envpol.2017.08.066
Jemec, A., Horvat, P., Kunej, U., Bele, M., and Kržan, A. (2016). Uptake and effects of microplastic textile fibers on freshwater crustacean Daphnia magna. Environ. Pollut. 219, 201–209. doi:10.1016/j.envpol.2016.10.037
Jeong, J., and Choi, J. (2020). Development of AOP Relevant to Microplastics Based on Toxicity Mechanisms of Chemical Additives Using ToxCast™ and Deep Learning Models Combined Approach. Environ. Int. 137, 105557. doi:10.1016/j.envint.2020.105557
Jiménez-Skrzypek, G., González-Sálamo, J., Varela-Martínez, D. A., González-Curbelo, M. Á., and Hernández-Borges, J. (2020). Analysis of Phthalic Acid Esters in Sea Water and Sea Sand Using Polymer-Coated Magnetic Nanoparticles as Extraction Sorbent. J. Chromatogr. A 1611, 460620. doi:10.1016/j.chroma.2019.460620
Kalčíková, G., Alič, B., Skalar, T., Bundschuh, M., and Gotvajn, A. Ž. (2017). Wastewater Treatment Plant Effluents as Source of Cosmetic Polyethylene Microbeads to Freshwater. Chemosphere 188, 25–31. doi:10.1016/j.chemosphere.2017.08.131
Kalogerakis, N., Karkanorachaki, K., Kalogerakis, G., Triantafyllidi, E. I., Gotsis, A. D., Partsinevelos, P., et al. (2017). Microplastics Generation: Onset of Fragmentation of Polyethylene Films in marine Environment Mesocosms. Front. Mar. Sci. 4, 84. doi:10.3389/fmars.2017.00084
Kang, M., Yang, F., Ren, H., Zhao, W., Zhao, Y., Li, L., et al. (2017). Influence of continental organic aerosols to the marine atmosphere over the East China Sea: Insights from lipids, PAHs and phthalates. Sci. Total Environ. 607, 339–350.
Karapanagioti, H. K., and Klontza, I. (2008). Testing Phenanthrene Distribution Properties of virgin Plastic Pellets and Plastic Eroded Pellets Found on Lesvos Island Beaches (Greece). Mar. Environ. Res. 65 (4), 283–290. doi:10.1016/j.marenvres.2007.11.005
Kavesh, S., and Schultz, J. M. (1969). Meaning and Measurement of Crystallinity in Polymers: A Review. Polym. Eng. Sci. 9 (5), 331–338. doi:10.1002/pen.760090504
Kenneth, S., Whiteley, T., Geoffrey, H., Hartmut, K., Ralph, L. M., and Wolfgang, I. (2005). “Polyolefins,” in Ullmann's Encyclopedia of Industrial Chemistry (Weinheim: Wiley VCH).
Koelmans, A. A., Besseling, E., Wegner, A., and Foekema, E. M. (2013). Plastic as a Carrier of POPs to Aquatic Organisms: a Model Analysis. Environ. Sci. Technol. 47 (14), 7812–7820. doi:10.1021/es401169n
Koerner, G. R., and Koerner, R. M. (2018). Polymeric Geomembrane Components in Landfill Liners. Solid Waste Landfilling: Concepts, Processes, Technology. Amsterdam, The Netherlands: Elsevier, 313.
Kögel, T., Bjorøy, Ø., Toto, B., Bienfait, A. M., and Sanden, M. (2019). Micro-and Nanoplastic Toxicity on Aquatic Life: Determining Factors. Sci. Tot. Environ. 709, 136050. doi:10.1016/j.scitotenv.2019.136050
Kurtz, S., and Manley, M. (2009). “Cross-Linked Polyethylene,” in Surgical Treatment of Hip Arthritis (WB Saunders), 456–467.
Lagergren, S. (1898). About the Theory of So-Called Adsorption of Solution Substances. K. Sven. Vetenskapsakad. Handl. 24, 1–39.
Langmuir, I. (1916). The Constitution and Fundamental Properties of Solids and Liquids. J. Am. Chem. Soc. 38, 2221–2295. doi:10.1021/ja02268a002
Lee, H., Shim, W. J., and Kwon, J. H. (2014). Sorption Capacity of Plastic Debris for Hydrophobic Organic Chemicals. Sci. Total Environ. 470, 1545–1552. doi:10.1016/j.scitotenv.2013.08.023
Lei, L., Wu, S., Lu, S., Liu, M., Song, Y., Fu, Z., et al. (2018). Microplastic Particles Cause Intestinal Damage and Other Adverse Effects in Zebrafish Danio rerio and Nematode Caenorhabditis elegans. Sci. Total Environ. 619–620, 1–8. doi:10.1016/j.scitotenv.2017.11.103
Li, C., Wang, X., Liu, K., Zhu, L., Wei, N., Zong, C., et al. (2021). Pelagic Microplastics in Surface Water of the Eastern Indian Ocean during Monsoon Transition Period: Abundance, Distribution, and Characteristics. Sci. Total Environ. 755 (2), 142629. doi:10.1016/j.scitotenv.2020.142629
Li, J., Zhang, K., and Zhang, H. (2018). Adsorption of Antibiotics on Microplastics. Environ. Pollut. 237, 460–467. doi:10.1016/j.envpol.2018.02.050
Li, Y., Li, M., Li, Z., Yang, L., and Liu, X. (2019). Effects of Particle Size and Solution Chemistry on Triclosan Sorption on Polystyrene Microplastic. Chemosphere 231, 308–314. doi:10.1016/j.chemosphere.2019.05.116
Liebezeit, G., and Liebezeit, E. (2014). Synthetic Particles as Contaminants in German Beers. Food Additives & Contaminants: A 31 (9), 1574–1578. doi:10.1080/19440049.2014.945099
Lima, A. L. C., Farrington, J. W., and Reddy, C. M. (2005). Combustion-derived Polycyclic Aromatic Hydrocarbons in the Environment—A Review. Environ. Forensics 6 (2), 109–131. doi:10.1080/15275920590952739
Limam, M., Tighzert, L., Fricoteaux, F., and Bureau, G. (2005). Sorption of Organic Solvents by Packaging Materials: Polyethylene Terephthalate and TOPAS. Polym. Test. 24 (3), 395–402. doi:10.1016/j.polymertesting.2004.09.004
Lin, L., Tang, S., Wang, X., Sun, X., and Liu, Y. (2021). Sorption of tetracycline onto hexabromocyclododecane/polystyrene composite and polystyrene microplastics: Statistical physics models, influencing factors, and interaction mechanisms. Environ. Pollut. 284, 117164.doi:10.1016/j.envpol.2021.117164
Liu, F. F., Liu, G. Z., Zhu, Z. L., Wang, S. C., and Zhao, F. F. (2019). Interactions between Microplastics and Phthalate Esters as Affected by Microplastics Characteristics and Solution Chemistry. Chemosphere 214, 688–694. doi:10.1016/j.chemosphere.2018.09.174
Liu, G., Jiang, R., You, J., Muir, D. C., and Zeng, E. Y. (2020). Microplastic Impacts on Microalgae Growth: Effects of Size and Humic Acid. Environ. Sci. Technol. 54 (3), 1782–1789. doi:10.1021/acs.est.9b06187
Liu, G., Zhu, Z., Yang, Y., Sun, Y., Yu, F., and Ma, J. (2019). Sorption Behavior and Mechanism of Hydrophilic Organic Chemicals to virgin and Aged Microplastics in Freshwater and Seawater. Environ. Pollut. 246, 26–33. doi:10.1016/j.envpol.2018.11.100
Liu, L., Fokkink, R., and Koelmans, A. A. (2016). Sorption of Polycyclic Aromatic Hydrocarbons to Polystyrene Nanoplastic. Environ. Toxicol. Chem. 35, 1650–1655. doi:10.1002/etc.3311
Liu, X., Zheng, M., Wang, L., Ke, R., Lou, Y., Zhang, X., et al. (2018). Sorption Behaviors of Tris-(2,3-Dibromopropyl) Isocyanurate and Hexabromocyclododecanes on Polypropylene Microplastics. Mar. Pollut. Bull. 135, 581–586. doi:10.1016/j.marpolbul.2018.07.061
Liu, Y., Li, Z., Jalón-Rojas, I., Wang, X. H., Fredj, E., Zhang, D., et al. (2020). Assessing the Potential Risk and Relationship between Microplastics and Phthalates in Surface Seawater of a Heavily Human-Impacted Metropolitan bay in Northern China. Ecotoxicology Environ. Saf. 204, 111067. doi:10.1016/j.ecoenv.2020.111067
Liu, Z., Cai, M., Yu, P., Chen, M., Wu, D., Zhang, M., et al. (2018). Age-dependent Survival, Stress Defense, and AMPK in Daphnia pulex after Short-Term Exposure to a Polystyrene Nanoplastic. Aquat. Toxicol. 204, 1–8. doi:10.1016/j.aquatox.2018.08.017
Liu, Z., Qin, Q., Hu, Z., Yan, L., Ieong, U. I., and Xu, Y. (2020). Adsorption of Chlorophenols on Polyethylene Terephthalate Microplastics from Aqueous Environments: Kinetics, Mechanisms and Influencing Factors. Environ. Pollut. 265, 114926. doi:10.1016/j.envpol.2020.114926
Llorca, M., Schirinzi, G., Martínez, M., Barceló, D., and Farré, M. (2018). Adsorption of Perfluoroalkyl Substances on Microplastics under Environmental Conditions. Environ. Pollut. 235, 680–691. doi:10.1016/j.envpol.2017.12.075
Lohmann, R. (2012). Critical Review of Low-Density Polyethylene’s Partitioning and Diffusion Coefficients for Trace Organic Contaminants and Implications for its Use as a Passive Sampler. Environ. Sci. Technol. 46 (2), 606–618. doi:10.1021/es202702y
Lu, S., Qiu, R., Hu, J., Li, X., Chen, Y., Zhang, X., et al. (2020). Prevalence of Microplastics in Animal-Based Traditional Medicinal Materials: Widespread Pollution in Terrestrial Environments. Sci. Total Environ. 709, 136214. doi:10.1016/j.scitotenv.2019.136214
Lusher, A. L., Mchugh, M., and Thompson, R. C. (2013). Occurrence of Microplastics in the Gastrointestinal Tract of Pelagic and Demersal Fish from the English Channel. Mar. Pollut. Bull. 67 (1-2), 94–99. doi:10.1016/j.marpolbul.2012.11.028
C. Lynwood (Editor) (2014). Polystyrene: Synthesis, Characteristics, and Applications (New York, NY: Nova Science Publishers, Inc).
Ma, H., Pu, S., Liu, S., Bai, Y., Mandal, S., and Xing, B. (2020). Microplastics in Aquatic Environments: Toxicity to Trigger Ecological Consequences. Environ. Pollut. 261, 114089. doi:10.1016/j.envpol.2020.114089
Magadini, D. L., Goes, J. I., Ortiz, S., Lipscomb, J., Pitiranggon, M., and Yan, B. (2020). Assessing the Sorption of Pharmaceuticals to Microplastics through In-Situ Experiments in New York City Waterways. Sci. Total Environ. 729, 138766. doi:10.1016/j.scitotenv.2020.138766
Martins, A. C., Pezoti, O., Cazetta, A. L., Bedin, K. C., Yamazaki, D. A., Bandoch, G. F., et al. (2015). Removal of tetracycline by NaOH-activated carbon produced from macadamia nut shells: kinetic and equilibrium studies. Chem. Eng. J. 260, 291–299.
Mason, S. A., Daily, J., Aleid, G., Ricotta, R., Smith, M., Donnelly, K., et al. (2020). High Levels of Pelagic Plastic Pollution within the Surface Waters of Lakes Erie and Ontario. J. Great Lakes Res. 46, 277. doi:10.1016/j.jglr.2019.12.012
Mato, Y., Isobe, T., Takada, H., Kanehiro, H., Ohtake, C., and Kaminuma, T. (2001). Plastic Resin Pellets as a Transport Medium for Toxic Chemicals in the marine Environment. Environ. Sci. Technol. 35 (2), 318–324. doi:10.1021/es0010498
McKay, G., and Allen, S. J. (1980). Surface Mass Transfer Processes Using Peat as an Adsorbent for Dyestuffs. Can. J. Chem. Eng. 58, 521. doi:10.1002/cjce.5450580416
Menéndez-Pedriza, A., and Jaumot, J. (2020). Interaction of Environmental Pollutants with Microplastics: A Critical Review of Sorption Factors, Bioaccumulation and Ecotoxicological Effects. Toxics 8 (2), 40. doi:10.3390/toxics8020040
Merrington, A. (2011). Applied Plastics Engineering Handbook: Processing and Materials. Midland, MI 48640, USA: Michigan Molecular Institute, 1910 W. St. Andrews Road, 177.
Miandad, R., Kumar, R., Barakat, M. A., Basheer, C., Aburiazaiza, A. S., Nizami, A. S., et al. (2018). Untapped Conversion of Plastic Waste Char into Carbon-Metal LDOs for the Adsorption of Congo Red. J. Colloid Interf. Sci. 511, 402–410. doi:10.1016/j.jcis.2017.10.029
Mouchi, V., Chapron, L., Peru, E., Pruski, A. M., Meistertzheim, A. L., Vétion, G., et al. (2019). Long-term Aquaria Study Suggests Species-specific Responses of Two Cold-Water Corals to Macro-And Microplastics Exposure. Environ. Pollut. 253, 322–329. doi:10.1016/j.envpol.2019.07.024
Murray, F., and Cowie, P. R. (2011). Plastic Contamination in the Decapod Crustacean Nephrops norvegicus (Linnaeus, 1758). Mar. Pollut. Bull. 62, 1207–1217. doi:10.1016/j.marpolbul.2011.03.032
Na, J., Song, J., Achar, J. C., and Jung, J. (2020). Synergistic Effect of Microplastic Fragments and Benzophenone-3 Additives on Lethal and Sublethal Daphnia magna Toxicity. J. Hazard. Mater. 402, 123845. doi:10.1016/j.jhazmat.2020.123845
Nakano, T. (2010). Synthesis, Structure and Function of π-stacked Polymers. Polym. J. 42 (2), 103–123. doi:10.1038/pj.2009.332
Napper, I. E., Bakir, A., Rowland, S. J., and Thompson, R. C. (2015). Characterisation, Quantity and Sorptive Properties of Microplastics Extracted from Cosmetics. Mar. Pollut. Bull. 99 (1-2), 178–185. doi:10.1016/j.marpolbul.2015.07.029
Norwegian Environment Agency, Norway (2014). News page 19.09.2014. Retrieved from: http://www.miljodirektoratet.no/no/Nyheter/Nyheter/2014/September-2014/Krepsepest-pavist-i-Rodenessjoen/(In Norwegian).
Oberg, G., and Leopold, A. (2019). On the role of review papers in the face of escalating publication rates-a case study of research on contaminants of emerging concern (CECs). Environ. Int. 131, 104960.
O'Connor, I. A., Golsteijn, L., and Hendriks, A. J. (2016). Review of the Partitioning of Chemicals into Different Plastics: Consequences for the Risk Assessment of marine Plastic Debris. Mar. Pollut. Bull. 113 (1-2), 17–24. doi:10.1016/j.marpolbul.2016.07.021
O'Donovan, S., Mestre, N. C., Abel, S., Fonseca, T. G., Carteny, C. C., Cormier, B., et al. (2018). Ecotoxicological Effects of Chemical Contaminants Adsorbed to Microplastics in the Clam. Scrobicularia Plana. Front. Mar. Sci. 5, 143. doi:10.3389/fmars.2018.00143
Ocampo-Pérez, R., Abdel daiem, M. M., Rivera-Utrilla, J., Méndez-Díaz, J. D., and Sánchez-Polo, M. (2012). Modeling Adsorption Rate of Organic Micropollutants Present in Landfill Leachates onto Granular Activated Carbon. J. Colloid Interf. Sci. 385, 174–182. doi:10.1016/j.jcis.2012.07.004
Ocampo-Pérez, R., Leyua-Ramos, R., Rivera-Utrilla, J., Flores-Cano, J. V., and Sánchez-Polo, M. (2015). Modeling Adsorption Rate of Tetracyclines on Activated Carbons from Aqueous Phase. Chem. Eng. Res. Des. 104, 579–588. doi:10.1016/j.cherd.2015.09.011
O’Connor, I. A., Golsteijn, L., and Hendriks, A. J. (2014). Meta-analysis of Plastic Water Partition Coefficients and Consequences for the Risk Assessment of Plastic marine Debris. Modelling the oral uptake of chemicals. Available at: https://hdl.handle.net/2066/133632.
Paluselli, A., Fauvelle, V., Galgani, F., and Sempéré, R. (2018). Phthalate Release from Plastic Fragments and Degradation in Seawater. Environ. Sci. Technol. 53 (1), 166–175. doi:10.1021/acs.est.8b05083
Pannetier, P., MorinLe Bihanic, B. F., Dubreil, L., Clérandeau, C., Chouvellon, F., Van Arkel, K., et al. (2020). Environmental Samples of Microplastics Induce Significant Toxic Effects in Fish Larvae. Environ. Int. 134, 105047. doi:10.1016/j.envint.2019.105047
Pascall, M. A., Zabik, M. E., Zabik, M. J., and Hernandez, R. J. (2005). Uptake of Polychlorinated Biphenyls (PCBs) from an Aqueous Medium by Polyethylene, Polyvinyl Chloride, and Polystyrene Films. J. Agric. Food Chem. 53 (1), 164–169. doi:10.1021/jf048978t
Patel, M. M., Goyal, B. R., Bhadada, S. V., Bhatt, J. S., and Amin, A. F. (2009). Getting into the brain. CNS Drugs 23, 35–58.
Peacock, A. (2000). Handbook of polyethylene: structures: properties, and applications. New York, NY: CRC press. Marcel Dekker, Inc.
Pereira, J. M., Rodríguez, Y., Blasco-Monleon, S., Porter, A., Lewis, C., and Pham, C. K. (2020). Microplastic in the Stomachs of Open-Ocean and Deep-Sea Fishes of the North-East Atlantic. Environ. Pollut. 265, 115060. doi:10.1016/j.envpol.2020.115060
Plazinski, W. (2010). Applicability of the Film-Diffusion Model for Description of the Adsorption Kinetics at the Solid/solution Interfaces. Appl. Surf. Sci. 256 (17), 5157–5163. doi:10.1016/j.apsusc.2009.12.083
Puckowski, A., Cwięk, W., Mioduszewska, K., Stepnowski, P., and Białk-Bielińska, A. (2021). Sorption of Pharmaceuticals on the Surface of Microplastics. Chemosphere 263, 127976. doi:10.1016/j.chemosphere.2020.127976
Qiu, Y., Zheng, M., Wang, L., Zhao, Q., Lou, Y., Shi, L., et al. (2019). Sorption of Polyhalogenated Carbazoles (PHCs) to Microplastics. Mar. Pollut. Bull. 146, 718–728. doi:10.1016/j.marpolbul.2019.07.034
Quek, S. Y., and Al-Duri, B. (2007). Application of Film-Pore Diffusion Model for the Adsorption of Metal Ions on Coir in a Fixed-Bed Column. Chem. Eng. Process. Process Intensification 46 (5), 477–485. doi:10.1016/j.cep.2006.06.019
Rainieri, S., Conlledo, N., Larsen, B. K., Granby, K., and Barranco, A. (2018). Combined Effects of Microplastics and Chemical Contaminants on the Organ Toxicity of Zebrafish (Danio rerio). Environ. Res. 162, 135–143. doi:10.1016/j.envres.2017.12.019
Ravit, B., Cooper, K., Buckley, B., Yang, I., and Deshpande, A. (2019). Organic Compounds Associated with Microplastic Pollutants in New Jersey, USA Surface Waters. AIMS Environ. Sci. 6 (6), 445. doi:10.3934/environsci.2019.6.445
Razanajatovo, R. M., Ding, J., Zhang, S., Jiang, H., and Zou, H. (2018). Sorption and Desorption of Selected Pharmaceuticals by Polyethylene Microplastics. Mar. Pollut. Bull. 136, 516–523. doi:10.1016/j.marpolbul.2018.09.048
Reitsma, P. J., Adelman, D., and Lohmann, R. (2013). Challenges of Using Polyethylene Passive Samplers to Determine Dissolved Concentrations of Parent and Alkylated PAHs under Cold and saline Conditions. Environ. Sci. Technol. 47 (18), 10429–10437. doi:10.1021/es402528q
Rios, L. M., Moore, C., and Jones, P. R. (2007). Persistent Organic Pollutants Carried by Synthetic Polymers in the Ocean Environment. Mar. Pollut. Bull. 54 (8), 1230–1237. doi:10.1016/j.marpolbul.2007.03.022
Rocha, S. D. F., Ribeiro, M. V., Viana, P. R. M., and Mansur, M. B. (2012). Bone char: an alternative for removal of diverse organic and inorganic compounds from industrial wastewater. Application of Adsorbents for Water Pollution. Bentham Science Publishers Ltd, 502-522.
Rocha-Santos, T. A., and Duarte, A. C. (2017). Characterization and Analysis of Microplastics. Elsevier.
Rochman, C. M., Hoh, E., Hentschel, B. T., and Kaye, S. (2013a). Long-term Field Measurement of Sorption of Organic Contaminants to Five Types of Plastic Pellets: Implications for Plastic marine Debris. Environ. Sci. Technol. 47 (3), 1646–1654. doi:10.1021/es403605f
Rochman, C. M., Manzano, C., Hentschel, B. T., Simonich, S. L. M., and Hoh, E. (2013b). Polystyrene Plastic: a Source and Sink for Polycyclic Aromatic Hydrocarbons in the marine Environment. Environ. Sci. Technol. 47 (24), 13976–13984. doi:10.1021/es403605f
Rodrigues, J. P., Duarte, A. C., Santos-Echeandía, J., and Rocha-Santos, T. (2019). Significance of Interactions between Microplastics and POPs in the marine Environment: a Critical Overview. Trac Trends Anal. Chem. 111, 252–260. doi:10.1016/j.trac.2018.11.038
Rogers, E. (2018). Investigation of Polycyclic Aromatic Hydrocarbons (PAH) Absorption from Seawater to Model Microplastic Particles. Master's thesis, (Trondheim, Norway:Norwegian University of Science and Technology).
Rossberg, M., Lendle, W., Pfleiderer, G., Tögel, A., Dreher, E. L., Langer, E., et al. (2000). Chlorinated Hydrocarbons. Ullmann's Encyclopedia Ind. Chem. Weinheim, Germany:Wiley Online Library. doi:10.1002/14356007.a06_233
Ruan, Y., Zhang, K., Wu, C., Wu, R., and Lam, P. K. (2019). A Preliminary Screening of HBCD Enantiomers Transported by Microplastics in Wastewater Treatment Plants. Sci. Total Environ. 674, 171–178. doi:10.1016/j.scitotenv.2019.04.007
Rusina, T. P., Smedes, F., Klanova, J., Booij, K., and Holoubek, I. (2007). Polymer Selection for Passive Sampling: A Comparison of Critical Properties. Chemosphere 68 (7), 1344–1351. doi:10.1016/j.chemosphere.2007.01.025
Ruthven, D. M. (1984). Principle of Adsorption and Adsorption Processes. New Jersey, NJ, USA: John Willey and Sons.
Saadi, R., Saadi, Z., Fazaeli, R., and Fard, N. E. (2015). Monolayer and Multilayer Adsorption Isotherm Models for Sorption from Aqueous media. Kor. J. Chem. Eng. 32, 787–799. doi:10.1007/s11814-015-0053-7
Savoca, S., Capillo, G., Mancuso, M., Bottari, T., Crupi, R., Branca, C., et al. (2019). Microplastics Occurrence in the Tyrrhenian Waters and in the Gastrointestinal Tract of Two Congener Species of Seabreams. Environ. Toxicol. Pharmacol. 67, 35–41. doi:10.1016/j.etap.2019.01.011
Schwarzenbach, R. P., Escher, B. I., Fenner, K., Hofstetter, T. B., Johnson, C. A., Von Gunten, U., et al. (2006). The challenge of micropollutants in aquatic systems. Science 313(5790), 1072–1077.
Scutariu, R. E., Puiu, D., Nechifor, G., Niculescu, M., Pascu, L. F., and Galaon, T. (2019). In Vitro sorption Study of Some Organochlorine Pesticides on Polyethylene Terephthalate Microplastics. Revista de Chim. 70 (12), 4620–4626. doi:10.37358/RC.19.12.7803
Sendra, M., Carrasco-Braganza, M. I., Yeste, P. M., Vila, M., and Blasco, J. (2020). Immunotoxicity of Polystyrene Nanoplastics in Different Hemocyte Subpopulations of Mytilus galloprovincialis. Scientific Rep. 10 (1), 8637. doi:10.1038/s41598-020-65596-8
Setälä, O., Fleming-Lehtinen, V., and Lehtiniemi, M. (2014). Ingestion and Transfer of Microplastics in the Planktonic Food Web. Environ. Pollut. 185, 77–83. doi:10.1016/j.envpol.2013.10.013
Setälä, O., Lehtiniemi, M., Coppock, R., and Cole, M. (2018). Microplastics in marine Food Webs. Microplastic Contam. Aquat. Environments 2018, 339–363. doi:10.1016/B978-0-12-813747-5.00011-4
Shanmuganathan, K., and Ellison, C. J. (2014). “Layered Double Hydroxides: an Emerging Class of Flame Retardants,” in Polymer Green Flame Retardants (Elsevier), 675–707. doi:10.1016/b978-0-444-53808-6.00020-2
Skrip, S., Das, G. K., and Chatterjee, S. G. (2013). Analytical expressions for the adsorbate breakthrough curve from a fixed bed of adsorbent with first-order and second-order kinetic models. Indian Chem. Eng. 55, 87–103.
Smedes, F., Geertsma, R. W., Zande, T. V. D., and Booij, K. (2009). Polymer− Water Partition Coefficients of Hydrophobic Compounds for Passive Sampling: Application of Cosolvent Models for Validation. Environ. Sci. Technol. 43 (18), 7047–7054. doi:10.1021/es9009376
Sørensen, L., Rogers, E., Altin, D., Salaberria, I., and Booth, A. M. (2020). Sorption of PAHs to Microplastic and Their Bioavailability and Toxicity to marine Copepods under Co-exposure Conditions. Environ. Pollut. 258, 113844. doi:10.1016/j.envpol.2019.113844
Sun, B., Hu, Y., Cheng, H., and Tao, S. (2019). Releases of Brominated Flame Retardants (BFRs) from Microplastics in Aqueous Medium: Kinetics and Molecular-Size Dependence of Diffusion. Water Res. 151, 215–225. doi:10.1016/j.watres.2018.12.017
Sun, J., Dai, X., Wang, Q., van Loosdrecht, M. C., and Ni, B. J. (2019). Microplastics in Wastewater Treatment Plants: Detection, Occurrence and Removal. Water Res. 152, 21–37. doi:10.1016/j.watres.2018.12.050
Sun, Y., Xu, W., Gu, Q., Chen, Y., Zhou, Q., Zhang, L., et al. (2019). Small-sized Microplastics Gegatively Affect Rotifers: Changes in the Key Life-History Traits and Rotifer–Phaeocystis Population Dynamics. Environ. Sci. Technol. 53 (15), 9241–9251. doi:10.1021/acs.est.9b02893
Šunta, U., Prosenc, F., Trebše, P., Bulc, T. G., and Kralj, M. B. (2020). Adsorption of Acetamiprid, Chlorantraniliprole and Flubendiamide on Different Type of Microplastics Present in Alluvial Soil. Chemosphere 261, 127762. doi:10.1016/j.chemosphere.2020.127762
Sussarellu, R., Suquet, M., Thomas, Y., Lambert, C., Fabioux, C., Pernet, M. E., et al. (2016). Oyster Reproduction Is Affected by Exposure to Polystyrene Microplastics. Proc. Natl. Acad. Sci. United States America 113 (9), 2430–2435. doi:10.1073/pnas.1519019113
Tallec, K., Paul-Pont, I., Boulais, M., Le Goïc, N., González-Fernández, C., Le Grand, F., et al. (2020). Nanopolystyrene Beads Affect Motility and Reproductive success of Oyster Spermatozoa (Crassostrea gigas). Nanotoxicology 14 (8), 1039–1057. doi:10.1080/17435390.2020.1808104
Tan, I. A. W., Ahmad, A. L., and Hameed, B. H. (2009). Adsorption Isotherms, Kinetics, Thermodynamics and Desorption Studies of 2,4,6-trichlorophenol on Oil palm Empty Fruit bunch-based Activated Carbon. J. Hazard. Mater. 164, 473–482. doi:10.1016/j.jhazmat.2008.08.025
Tan, X., Yu, X., Cai, L., Wang, J., and Peng, J. (2019). Microplastics and Associated PAHs in Surface Water from the Feilaixia Reservoir in the Beijiang River, China. Chemosphere 221, 834–840. doi:10.1016/j.chemosphere.2019.01.022
Tanaka, K., Takada, H., Ikenaka, Y., Nakayama, S. M. M., and Ishizuka, M. (2019). Occurrence and Concentrations of Chemical Additives in Plastic Fragments on a beach on the Island of Kauai, Hawaii. Mar. Pollut. Bull. 150, 110732. doi:10.1016/j.marpolbul.2019.110732
Temkin, M. I. (1941). Adsorption Equilibrium and the Kinetics of Processes on Nonhomogeneous Surfaces and in the Interaction between Adsorbed Molecules. Zh. Fiz. Chim. 15, 296–332.
Ten Hulscher, Th. E. M., and Cornelissen, G. (1996). Effect of Temperature on Sorption Equilibrium and Sorption Kinetics of Organic Micropollutants—A Review. Chemosphere 32, 609–626. doi:10.1016/0045-6535(95)00345-2
Teuten, E. L., Rowland, S. J., Galloway, T. S., and Thompson, R. C. (2007). Potential for Plastics to Transport Hydrophobic Contaminants. Environ. Sci. Technol. 41 (22), 7759–7764. doi:10.1021/es071737s
Tolls, J. (2001). Sorption of Veterinary Pharmaceuticals in Soils: A Review. Environ. Sci. Technol. 35, 3397–3406. doi:10.1021/es0003021
Torres, F. G., Dioses-Salinas, D. C., Pizarro-Ortega, C. I., and De-la-Torre, G. E. (2021). Sorption of Chemical Contaminants on Degradable and Non-degradable Microplastics: Recent Progress and Research Trends. Sci. Total Environ. 757, 143875. doi:10.1016/j.scitotenv.2020.143875
Tourinho, P. S., Kocí, V., Loureiro, S., and van Gestel, C. A. (2019). Partitioning of Chemical Contaminants to Microplastics: Sorption Mechanisms, Environmental Distribution and Effects on Toxicity and Bioaccumulation. Environ. Pollut. 252, 1246–1256. doi:10.1016/j.envpol.2019.06.030
Tyree, C., and Morrison, D. (2017). Invisibles: Plastic Invasion. Available at: https://orbmedia.org/stories/Invisibles_plastics/ (Accessed August 20, 2020).
Uber, T. H., Hüffer, T., Planitz, S., and Schmidt, T. C. (2019). Characterization of Sorption Properties of High-Density Polyethylene Using the Poly-Parameter Linearfree-Energy Relationships. Environ. Pollut. 248, 312–319. doi:10.1016/j.envpol.2019.02.024
UNEP and NOAA (2015). The Honolulu Strategy: a Global Framework for Prevention and Management of marine Debris. Available at: http://unep.org/gpa/documents/publications/honolulustrategy.pdf.
UNEPUnited Nations Environment Programme (UNEP) (2015). Plastic in Cosmetics (Fact Sheet). Nairobi, 1.24, 1–3.
Valderrama, C., Gamisans, X., de las Heras, X., Farrán, A., and Cortina, J. L. (2008). Sorption Kinetics of Polycyclic Aromatic Hydrocarbons Removal Using Granular Activated Carbon: Intraparticle Diffusion Coefficients. J. Hazard. Mater. 157, 386–396. doi:10.1016/j.jhazmat.2007.12.119
van Krevelen, D. W., and Te Nijenhuis, K. (2009). Properties of Polymers: Their Correlation with Chemical Structure; Their Numerical Estimation and Prediction from Additive Group Contributions. Elsevier Science, 1030.
Vieira, Y., Lima, E. C., Foletto, E. L., and Dotto, G. L. (2021). Microplastics Physicochemical Properties, Specific Adsorption Modeling and Their Interaction with Pharmaceuticals and Other Emerging Contaminants. Sci. Total Environ. 753, 141981. doi:10.1016/j.scitotenv.2020.141981
Von Moos, N., Burkhardt-Holm, P., and Köhler, A. (2012). Uptake and Effects of Microplastics on Cells and Tissue of the Blue Mussel Mytilus edulis L. After an Experimental Exposure. Envir. Sci. Tech. 46, 11327–11335. doi:10.1021/es302332w
Wagner, S., and Reemtsma, T. (2019). Things We Know and Don't Know about Nanoplastic in the Environment. Nat. Nanotechnol. 14, 300–301. doi:10.1038/s41565-019-0424-z
Wahl, A., Le Juge, C., Davranche, M., El Hadri, H., Grassl, B., Reynaud, S., et al. (2021). Nanoplastic Occurrence in a Soil Amended with Plastic Debris. Chemosphere 262, 127784. doi:10.1016/j.chemosphere.2020.127784
Wang, F., Shih, K. M., and Li, X. Y. (2015). The Partition Behavior of Perfluorooctanesulfonate (PFOS) and Perfluorooctanesulfonamide (FOSA) on Microplastics. Chemosphere 119, 841–847. doi:10.1016/j.chemosphere.2014.08.047
Wang, F., Zhang, M., Sha, W., Wang, Y., Hao, H., Dou, Y., et al. (2020). Sorption Behaviour and Mechanisms of Organic Contaminants to Nano and Microplastics. Molecules 25, 1827. doi:10.3390/molecules25081827
Wang, J., and Guo, X. (2020). Adsorption Isotherm Models: Classification, Physical Meaning, Application and Solving Method. Chemosphere 258, 127279. doi:10.1016/j.chemosphere.2020.127279
Wang, J., Liu, X., Liu, G., Zhang, Z., Wu, H., Cui, B., et al. (2019). Size Effect of Polystyrene Microplastics on Sorption of Phenanthrene and Nitrobenzene. Ecotoxicology Environ. Saf. 173, 331–338. doi:10.1016/j.ecoenv.2019.02.037
Wang, L., Wu, W. M., Bolan, N. S., Tsang, D., Li, Y., Qin, M., et al. (2021). Environmental Fate, Toxicity and Risk Management Strategies of Nanoplastics in the Environment: Current Status and Future Perspectives. J. Hazard. Mater. 401, 123415. doi:10.1016/j.jhazmat.2020.123415
Wang, W., and Wang, J. (2018). Different Partition of Polycyclic Aromatic Hydrocarbon on Environmental Particulates in Freshwater: Microplastics in Comparison to Natural Sediment. Ecotoxicology Environ. Saf. 147, 648–655. doi:10.1016/j.ecoenv.2017.09.029
Wang, Z., Chen, M., Zhang, L., Wang, K., Yu, X., Zheng, Z., et al. (2018). Sorption Behaviors of Phenanthrene on the Microplastics Identified in a Mariculture Farm in Xiangshan Bay, southeastern China. Sci. Total Environ. 628-629, 1617–1626. doi:10.1016/j.scitotenv.2018.02.146
Wardrop, P., Shimeta, J., Nugegoda, D., Morrison, P. D., Miranda, A., Tang, M., et al. (2016). Chemical Pollutants Sorbed to Ingested Microbeads from Personal Care Products Accumulate in Fish. Environ. Sci. Technol. 50 (7), 4037–4044. doi:10.1021/acs.est.5b06280
Weber, W. J., and Morris, J. C. (1963). Kinetics of Adsorption on Carbon from Solution. J. Sanit. Eng. Div. 1, 1–2.
Welden, N. A., and Cowie, P. R. (2017). Degradation of Common Polymer Ropes in a Sublittoral marine Environment. Mar. Pollut. Bull. 118 (1-2), 248–253. doi:10.1016/j.marpolbul.2017.02.072
Wilcox, C., Hardesty, B. D., and Law, K. L. (2020). Abundance of Floating Plastic Particles Is Increasing in the Western North Atlantic Ocean. Environ. Sci. Technol. 54, 790–796. doi:10.1021/acs.est.9b04812
Wong, S. L., Nyakuma, B. B., Wong, K. Y., Lee, C. T., Lee, T. H., and Lee, C. H. (2020). Microplastics and Nanoplastics in Global Food Webs: A Bibliometric Analysis (2009–2019),. Mar. Pollut. Bull. 158, 111432. doi:10.1016/j.marpolbul.2020.111432
Wright, S. L., and Kelly, F. J. (2017). Plastic and Human Health: a Micro Issue? Environ. Sci. Technol. 51 (12), 6634–6647. doi:10.1021/acs.est.7b00423
Wright, S. L., Thompson, R. C., and Galloway, T. S. (2013). The Physical Impacts of Microplastics on marine Organisms: a Review. Environ. Pollut. 178, 483–492. doi:10.1016/j.envpol.2013.02.031
Wu, C. C., Bao, L. J., Liu, L. Y., Shi, L., Tao, S., and Zeng, E. Y. (2017). Impact of Polymer Colonization on the Fate of Organic Contaminants in Sediment. Environ. Sci. Technol. 51 (18), 10555–10561. doi:10.1021/acs.est.7b03310
Wu, C., Zhang, K., Huang, X., and Liu, J. (2016). Sorption of Pharmaceuticals and Personal Care Products to Polyethylene Debris. Environ. Sci. Pollut. Res. 23 (9), 8819–8826. doi:10.1007/s11356-016-6121-7
Wu, J., Xu, P., Chen, Q., Ma, D., Ge, W., Jiang, T., et al. (2020). Effects of Polymer Aging on Sorption of 2,2′,4,4′-tetrabromodiphenyl Ether by Polystyrene Microplastics. Chemosphere 253, 126706. doi:10.1016/j.chemosphere.2020.126706
Wu, P., Cai, Z., Jin, H., and Tang, Y. (2019). Adsorption Mechanisms of Five Bisphenol Analogues on PVC Microplastics. Sci. Total Environ. 650, 671–678. doi:10.1016/j.scitotenv.2018.09.049
Xia, B., Zhang, J., Zhao, X., Feng, J., Teng, Y., Chen, B., et al. (2020). Polystyrene Microplastics Increase Uptake, Elimination and Cytotoxicity of Decabromodiphenyl Ether (BDE-209) in the marine Scallop Chlamys Farreri. Environ. Pollut. 258, 113657. doi:10.1016/j.envpol.2019.113657
Xu, B., Liu, F., Brookes, P. C., and Xu, J. (2018). Microplastics Play a Minor Role in Tetracycline Sorption in the Presence of Dissolved Organic Matter. Environ. Pollut. 240, 87–94. doi:10.1016/j.envpol.2018.04.113
Xu, E. G., Cheong, R. S., Liu, L., Hernandez, L. M., Azimzada, A., Bayen, S., et al. (2020). Primary and Secondary Plastic Particles Exhibit Limited Acute Toxicity but Chronic Effects on Daphnia magna. Environ. Sci. Technol. 54, 6859. doi:10.1021/acs.est.0c00245
Xu, P., Ge, W., Chai, C., Zhang, Y., Jiang, T., and Xia, B. (2019). Sorption of Polybrominated Diphenyl Ethers by Microplastics. Mar. Pollut. Bull. 145, 260–269. doi:10.1016/j.marpolbul.2019.05.050
Yang, C. (1998). Statistical Mechanical Study on the Freundlich Isotherm Equation. Colloid Interf. Sci. 208, 379–387. doi:10.1006/jcis.1998.5843
Yang, K., Zhu, L., and Xing, B. (2006). Adsorption of Polycyclic Aromatic Hydrocarbons by Carbon Nanomaterials. Environ. Sci. Technol. 40, 1855–1861. doi:10.1021/es052208w
Yang, L., Wang, T., Zhou, Y., Shi, B., Bi, R., and Meng, J. (2021). Contamination, Source and Potential Risks of Pharmaceuticals and Personal Products (PPCPs) in Baiyangdian Basin, an Intensive Human Intervention Area, China. Sci. Total Environ. 760, 144080. doi:10.1016/j.scitotenv.2020.144080
Yang, Y., Yang, J., Wu, W. M., Zhao, J., Song, Y., Gao, L., et al. (2015). Biodegradation and Mineralization of Polystyrene by Plastic-Eating Mealworms: Part 1. Chemical and Physical Characterization and Isotopic Tests. Environ. Sci. Technol. 49 (20), 12080–12086. doi:10.1021/acs.est.5b02661
Yazidi, A., Atrous, M., Soetaredjo, F. E., Sellaoui, L., Ismadji, S., Erto, A., et al. (2020). Adsorption of Amoxicillin and Tetracycline on Activated Carbon Prepared from Durian Shell in Single and Binary Systems: Experimental Study and Modeling Analysis. Chem. Eng. J. 379, 122320. doi:10.1016/j.cej.2019.122320
Yin, L., Wang, B., Yuan, H., Deng, S., Huang, J., Wang, Y., et al. (2017). Pay Special Attention to the Transformation Products of PPCPs in Environment. Emerging Contaminants 3 (2), 69–75. doi:10.1016/j.emcon.2017.04.001
Yu, F., Yang, C. F., Zhu, Z. L., Bai, X. T., and Ma, J. (2019). Adsorption Behavior of Organic Pollutants and Metals on Micro/nanoplastics in the Aquatic Environment. Sci. Total Environ. 694, 133643. doi:10.1016/j.scitotenv.2019.133643
Yu, H., Yang, B., Waigi, M. G., Peng, F., Li, Z., and Hu, X. (2020). The Effects of Functional Groups on the Sorption of Naphthalene on Microplastics,. Chemosphere 261, 127592. doi:10.1016/j.chemosphere.2020.127592
Yu, P., Liu, Z., Wu, D., Chen, M., Lv, W., and Zhao, Y. (2018). Accumulation of Polystyrene Microplastics in Juvenile Eriocheir Sinensis and Oxidative Stress Effects in the Liver. Aquat. Toxicol. 200, 28–36. doi:10.1016/j.aquatox.2018.04.015
Yu, S.-P., and Chan, B. K. K. (2020). Effects of Polystyrene Microplastics on Larval Development, Settlement, and Metamorphosis of the Intertidal Barnacle. Amphibalanus Amphitrite. Ecotoxicol. Environ. Saf. 194, 110362. doi:10.1016/j.ecoenv.2020.110362
Yuan, F., Yue, L., Zhao, H., and Wu, H. (2020). Study on the Adsorption of Polystyrene Microplastics by Three-Dimensional Reduced Graphene Oxide. Water Sci. Technol. 81 (10), 2163–2175. doi:10.2166/wst.2020.269
Zarfl, C., Fleet, D., Fries, E., Galgani, F., Gerdts, G., Hanke, G., et al. (2011). Microplastics in Oceans. Mar. Pollut. Bull. 62, 1589–1591. doi:10.1016/j.marpolbul.2011.02.040
Zhan, Z., Wang, J., Peng, J., Xie, Q., Huang, Y., and Gao, Y. (2016). Sorption of 3, 3′, 4, 4′-tetrachlorobiphenyl by Microplastics: a Case Study of Polypropylene. Mar. Pollut. Bull. 110 (1), 559–563. doi:10.1016/j.marpolbul.2016.05.036
Zhang, H., Wang, J., Zhou, B., Zhou, Y., Dai, Z., Zhou, Q., et al. (2018b). Enhanced Adsorption of Oxytetracycline to Weathered Microplastic Polystyrene: Kinetics, Isotherms and Influencing Factors. Environ. Pollut. 243, 1550–1557. doi:10.1016/j.envpol.2018.09.122
Zhang, H., Zhou, Q., Xie, Z., Zhou, Y., Tu, C., Fu, C., et al. (2018a). Occurrences of Organophosphorus Esters and Phthalates in the Microplastics from the Coastal Beaches in north China. Sci. Total Environ. 616, 1505–1512. doi:10.1016/j.scitotenv.2017.10.163
Zhang, J., Chen, H., He, H., Cheng, X., Ma, T., Hu, J., et al. (2020). Adsorption Behavior and Mechanism of 9-Nitroanthracene on Typical Microplastics in Aqueous Solutions. Chemosphere 245, 125628. doi:10.1016/j.chemosphere.2019.125628
Zhang, P., Huang, P., Sun, H., Ma, J., and Li, B. (2019). The Structure of Agricultural Microplastics (PT, PU and UF) and Their Sorption Capacities for PAHs and PHE Derivates under Various Salinity and Oxidation Treatments. Environ. Pollut. 257, 113525. doi:10.1016/j.envpol.2019.113525
Zhang, S., Ding, J., Razanajatovo, R. M., Jiang, H., Zou, H., and Zhu, W. (2019). Interactive Effects of Polystyrene Microplastics and Roxithromycin on Bioaccumulation and Biochemical Status in the Freshwater Fish Red tilapia (Oreochromis niloticus). Sci. Total Environ. 648, 1431–1439. doi:10.1016/j.scitotenv.2018.08.266
Zhao, L., Rong, L., Xu, J., Lian, J., Wang, L., and Sun, H. (2020). Sorption of Five Organic Compounds by Polar and Nonpolar Microplastics. Chemosphere 257, 127206. doi:10.1016/j.chemosphere.2020.127206
Zhou, Q., Zhang, H., Zhou, Y., Dai, Z., Li, Y., Fu, C., et al. (2017). Surface weathering and changes in components of microplastics from estuarine beaches. Chin. Sci. Bull. 63, 214–223.
Zhu, L., and Chen, B. (2000). Sorption Behavior of P-Nitrophenol on the Interface between Anion-Cation Organobentonite and Water. Environ. Sci. Technol. 34, 2997–3002. doi:10.1021/es991460z
Zhu, Z. L., Wang, S. C., Zhao, F. F., Wang, S. G., Liu, F. F., and Liu, G. Z. (2019). Joint Toxicity of Microplastics with Triclosan to marine Microalgae. Skeletonema Costatum. Environ. Pollut. 246, 509–517. doi:10.1016/j.envpol.2018.12.044
Zhuang, W. R., Wang, Y., Cui, P. F., Xing, L., Lee, J., Kim, D., et al. (2019). Applications of π-π Stacking Interactions in the Design of Drug-Delivery Systems. J. Controlled Release 294, 311–326. doi:10.1016/j.jconrel.2018.12.014
Keywords: microplastics (MPs), nanoplastics (NPs), persistent organic pollutants (POPs), plastic additives, sorption—desorption isotherms, kinetic sorption model, emerging micropollutants
Citation: Agboola OD and Benson NU (2021) Physisorption and Chemisorption Mechanisms Influencing Micro (Nano) Plastics-Organic Chemical Contaminants Interactions: A Review. Front. Environ. Sci. 9:678574. doi: 10.3389/fenvs.2021.678574
Received: 09 March 2021; Accepted: 05 May 2021;
Published: 28 May 2021.
Edited by:
Jastin Samuel, Lovely Professional University, IndiaReviewed by:
Vineet Kumar, Guru Ghasidas Vishwavidyalaya, IndiaPriyanka Jha, University of KwaZulu-Natal, South Africa
Copyright © 2021 Agboola and Benson. This is an open-access article distributed under the terms of the Creative Commons Attribution License (CC BY). The use, distribution or reproduction in other forums is permitted, provided the original author(s) and the copyright owner(s) are credited and that the original publication in this journal is cited, in accordance with accepted academic practice. No use, distribution or reproduction is permitted which does not comply with these terms.
*Correspondence: Nsikak U. Benson, nsikak.benson@covenantuniversity.edu.ng