- 1Universidade Católica Portuguesa, CBQF—Centro de Biotecnologia e Química Fina – Laboratório Associado, Escola Superior de Biotecnologia, Porto, Portugal
- 2Univ. Bordeaux, INRAE, BIOGECO, Pessac, France
- 3Department of Conservation of Natural Resources, NEIKER-Basque Institute for Agricultural Research and Development, Basque Research and Technology Alliance (BRTA), Derio, Spain
- 4Instituto de Investigacións Agrobiolóxicas de Galicia (IIAG), Consejo Superior de Investigaciones Científicas (CSIC), Santiago de Compostela, Spain
The growing loss of soil functionality due to contamination by metal(loid)s, alone or in combination with organic pollutants, is a global environmental issue that entails major risks to ecosystems and human health. Consequently, the management and restructuring of large metal(loid)-polluted areas through sustainable nature-based solutions is currently a priority in research programs and legislation worldwide. Over the last few years, phytomanagement has emerged as a promising phytotechnology, focused on the use of plants and associated microorganisms, together with ad hoc site management practices, for an economically viable and ecologically sustainable recovery of contaminated sites. It promotes simultaneously the recovery of soil ecological functions and the decrease of pollutant linkages, while providing economic revenues, e.g. by producing non-food crops for biomass-processing technologies (biofuel and bioenergy sector, ecomaterials, biosourced-chemistry, etc.), thus contributing to the international demand for sustainable and renewable sources of energy and raw materials for the bioeconomy. Potential environmental benefits also include the provision of valuable ecosystem services such as water drainage management, soil erosion deterrence, C sequestration, regulation of nutrient cycles, xenobiotic biodegradation, and metal(loid) stabilization. Phytomanagement relies on the proper selection of (i) plants and (ii) microbial inoculants with the capacity to behave as powerful plant allies, e.g., PGPB: plant growth-promoting bacteria and AMF: arbuscular mycorrhizal fungi. This review gives an up-to-date overview of the main annual, perennial, and woody crops, as well as the most adequate cropping systems, presently used to phytomanage metal(loid)-contaminated soils, and the relevant products and ecosystems services provided by the various phytomanagement options. Suitable bioaugmentation practices with PGPB and AMF are also discussed. Furthermore, we identify the potential interest of phytomanagement for stakeholders and end-users and highlight future opportunities boosted by an effective engagement between environmental protection and economic development. We conclude by presenting the legal and regulatory framework of soil remediation and by discussing prospects for phytotechnologies applications in the future.
Introduction
Soil contamination with metal(loid)s, termed also ‘trace elements’ in Biogeochemistry and Life Sciences (hereafter referred as TE) is a global environmental issue that poses serious risks for ecosystem integrity and human health (Joimel et al., 2016; Hou et al., 2017; Pérez and Eugenio, 2018; Bagherifam et al., 2019; Mench et al., 2020; Haller and Jonsson, 2020). Although background and bioavailable TE levels are generally low in soils, except at geochemical anomalies with either deficiency or exceedance, anthropogenic activities such as industry, mining, smelting and metallurgy, intensive agriculture, e-wastes, traffic, use of fossil fuels, etc. have caused an increase in soil TE concentrations reaching hazardous levels (Alloway, 2013; Kumpiene et al., 2017; Petruzzelli et al., 2020). Furthermore, anthropogenically-released TE tend to have higher availability and mobility than those resulting from natural processes (Kabata-Pendias, 2010), raising environmental and human health concerns. In the European Union (EU), there are at least 2.8 million potential contaminated sites and 650,000 registered sites where polluting activities occurred or are occurring (Pérez and Eugenio, 2018). Such high number of contaminated sites, coupled with the threat posed by contaminants to environmental and public health, pushed policymakers and legislators to 1) set soil protection as a strategic priority and 2) encourage the remediation, reclamation, restoration and recovery of those sites (Reinikainen et al., 2016; Castelo-Grande et al., 2018; Ramon and Lull, 2019). Consequently, nowadays, numerous countries have specific legislation and guidelines to deal with contaminated sites and are committed to their remediation, based on either regulatory values or site-specific risk assessment, which further depends on contemplated future land use (Mench et al., 2020). This has boosted the number of initiatives in the EU aimed at recovering contaminated sites (Pérez and Eugenio, 2018). However, despite this positive trend, these numbers fall short given the extent of the problem.
The recovery of TE-polluted land is critical to enhance soil ecosystem services, as well as to decrease the contamination of the soil matrix itself, together with that of recipient waterbodies and food crops, thus ensuring human welfare. Additionally, the reclamation of contaminated sites through phytomanagement creates a set of opportunities to comply with the net-zero carbon emissions targets, by generating areas that act as carbon sinks and by implementing programs for valuing land that commensurate with the current sustainability paradigm (Bardos et al., 2016, 2020; Cundy et al., 2021). Furthermore, the use of contaminated lands for bioenergy production can importantly reduce the clearing of agricultural/fertile areas for this purpose, leading to greenhouse gas emission savings (Mellor et al., 2021). In the last years, stakeholders, including site owners, local population, investors and public authorities, are increasing their environmental awareness and gradually recognizing that recovered sites can provide values, goods and services, and then demanding the management and recovery of contaminated land. In the last 2 decades, environmentally-friendlier, greener technologies have paved the way to become reliable alternatives to previously favored disruptive methodologies (Kidd et al., 2015). Amongst these, phytotechnologies stand out as a cost-effective, sustainable option for the recovery of TE-contaminated areas (Mench et al., 2010, 2018; Herzig et al., 2014; Thijs et al., 2018; Kolbas et al., 2020). Phytotechnologies involve a set of techniques that exploit plants and sustainably manage “soil-plant-microbial” systems to recover polluted sites, especially those with low and medium levels of soil pollution (Vangronsveld et al., 2009; Mench et al., 2010, 2018). Regarding TE-pollution, phytotechnologies can also be an effective choice for highly contaminated sites when the goal is stripping the soil of its bioavailable metal(loid) fractions, or TE-stabilization (thus, decreasing potential TE toxicity). This can be tackled through TE uptake and accumulation in harvestable plant parts (e.g., phytoextraction), or by progressively promoting in situ inactivation by combining the use of TE-excluding plants, soil amendments and/or microbial inoculants (assisted phytostabilization), respectively (Mench et al., 2010, 2018; Epelde et al., 2014; Kidd et al., 2015; Burges et al., 2016, 2017, 2018). Phytoextraction options can also be assisted by soil amendments, chemical agents and soil microorganisms (assisted phytoextraction) (Vangronsveld et al., 2009; Mench et al., 2010; Kidd et al., 2015; Wang et al., 2019; Kolbas et al., 2020). Within this context, microbial bioinoculants and site-tailored cropping systems are most useful tools to help plants cope with soil contamination. Not surprisingly, the research on microbial bioinoculants (e.g., plant growth-promoting bacteria - PGPB and arbuscular mycorrhizal fungi - AMF), soil amendments (organic and/or inorganic), and suitable cropping patterns (e.g., intercropping, winter cropping) escalated in the last decade, yielding very positive and encouraging outcomes.
In the recent past, phytotechnologies were combined with sustainable site management practices, giving birth to a wider approach—phytomanagement—where environmental benefits are allied with financial returns for stakeholders, and/or wider social and economic benefits to the surrounding community (Robinson et al., 2009; Cundy et al., 2016; Burges et al., 2018; Li et al., 2018; Bardos et al., 2020). Beside presenting a plethora of ecological benefits such as the progressive reestablishment of soil health and decreased TE run-off risks, phytomanagement places emphasis on obtaining economic profits by using cash crops to produce biomass for renewable energy and valuable materials (Evangelou et al., 2015; Kidd et al., 2015; Cundy et al., 2016; Šimek et al., 2017; Thijs et al., 2018; Xue et al., 2018). Phytomanagement is in line with the objectives of the European Green Deal roadmap that aims at turning climate and environmental challenges into opportunities, restoring biodiversity and decreasing pollution. Using contaminated sites, for instance, for bioenergy production supports the growing demand for energy sources alternative to fossil fuels, while reducing the prevailing pressure on the use of forests or agricultural productive land for the productions of biofuels (Edrisi and Abhilash, 2016; Sytar and Prasad, 2016).
Under this scope, this review aims to: 1) provide an overview of the main traits and potential economic applications of the most widely used TE-tolerant cash crops that better fit phytomanagement goals; 2) summarize selected field phytomanagement experiments (especially those published in 2010–2020) using these plants; 3) indicate suitable crops that can be coupled with cash crops to improve site management and phytomanagement effectiveness; 4) address the importance of cropping patterns and the use of amendments in the remediation of TE-contaminated sites; and 5) explore the use of microorganisms, namely PGPB and AMF, as probiotics for soil health recovery and plant performance (establishment, survival, growth, and physiological traits). Cash crops were selected based on their ability to provide suficient harvestable biomass that can be processed, especially for the local bioeconomy, and of which there is a significant body of literature based on empirical research, especially from field trials. We conclude by highlighting the current status of phytomanagement and the legal and regulatory frameworks for its implementation across Europe.
The type of amendments, cropping systems and their combination provides a myriad of possible scenarios. This topic is a matter of thorough scrutiny elsewhere (e.g.,Kidd et al., 2015), and therefore it will not be exhaustively addressed here. Genetic-engineered cultivars are also beyond the scope of this review (for information on this topic, see for instance Gunarathne et al., 2019 and Sebastian et al., 2019).
Phytomanagement Benefits and Constraints—Brief Overview
Soil contamination reduces site's economic, ecological and social values. As addressed, a suitable and cost-effective option to remediate such contaminated sites and restore land values is the phytomanagement, a multi-objective management strategy that reconciles economic and social returns with ecological gains (Figure 1) (Nsanganwimana et al., 2014; Kidd et al., 2015; Burges et al., 2018). Phytomanagement can provide financial benefits through planting valuable crops that serve as feedstock for multiple industries and end-products such as furniture, pulp and paper, biochemicals (adhesives and detergents), insulation and building materials, composites and plastic alternatives, food additives, animal feeding and bedding, etc. Some of these crops can also be used as bioenergy crops yielding high-quality biomass (Nsanganwimana et al., 2014, 2015; Burges et al., 2018; Lacalle et al., 2018; Mench et al., 2018; Thijs et al., 2018) to produce renewable energy (electricity, heat and biofuels) (Gonsalvesh et al., 2016; Pandey et al., 2016; Rizwan et al., 2018; Grottola et al., 2019; Pogrzeba et al., 2019; Rusinowski et al., 2019; Sidhu et al., 2020; Tran et al., 2020 ). Most importantly, these crops offer the possibility to combine the production of biomass for energy production and/or other end-products (Grisan et al., 2016; Barla and Kumar, 2019) with TE phytoextraction or phytostabilization (Thijs et al., 2018; Chalot et al., 2020). Likewise, they can simultaneously promote the biodegradation of soil organic contaminants. Economic revenues can also be obtained through phytomining, a phytotechnology focused on the recovery of valuable TE (e.g., Co, Ni, and Re) from the TE-rich biomass of hyperaccumulators (also called bio-ores) (Remigio et al., 2020; Chaney et al., 2018). TE-rich phytomass can be pyrolysed/calcinated and the resulting biochar and/or ashes used as ecocatalysts in biosourced fine chemistry (Clavé et al., 2016; Quintela-Sabarís et al., 2017; Mench et al., 2018; Xue et al., 2018; Bihanic et al., 2020; Kolbas et al., 2020). The production of high-value products fits the Circular Economy (CE) and Bioeconomy paradigms, both highly promoted within the EU and other strong economies such as China, United Kingdom, Canada and Japan (Korhonen et al., 2018). The CE paradigm promotes economic development through a cyclical flow of materials that spill over as direct and indirect benefits to the environmental and social dimension of our society (Korhonen et al., 2018). However, CE requires a shift in the society´s mindset and requires, among other aspects, the design of new business models and robust networking and innovation in production processes and commercial products (Prieto-Sandoval et al., 2018).
Regarding the potential environmental benefits, at the local level, phytomanagement improves soil health and fertility (Herzig et al., 2014; Kidd et al., 2015; Touceda-González et al., 2017; Xue et al., 2018), soil organic matter (OM) quantity and quality (Mench et al., 2018; Risueño et al., 2020; Álvarez-Rogel et al., 2021) and soil biodiversity, both faunal (Chauvat et al., 2014) and microbial (Foulon et al., 2016; Šimek et al., 2017; Durand et al., 2018; Xue et al., 2018; Garbisu et al., 2020; Kidd et al., 2021). At the large scale, phytomanagement can enhance carbon sequestration, mitigate the emission of greenhouse gases, and reduce and/or prevent TE dispersion (Evangelou et al., 2012a; Kidd et al., 2015; Cundy et al., 2016; Šimek et al., 2017; Xue et al., 2018).
As a risk-based approach, prior to its implementation, phytomanagement requires an initial risk assessment to evaluate pollutant linkages (source-pathway-receptor) (Figure 1) (Cundy et al., 2016; Reinikainen et al., 2016). Upon this risk evaluation, an option appraisal must be conducted by weighting several variables such as feasibility, time, economic viability, legal requirements, social approval, etc. to properly outline the best way of handling the abovementioned pollutant linkages. Option appraisal is a baseline cornerstone for the successful design of any restoration plan (Mench et al., 2020). In addition, for phytomanagement to be fully operational at a given site, an optimization stage before full-scale implementation is required. At this stage, issues related to edaphoclimatic conditions are addressed to guide the selection of the most appropriate crops. Potential edaphic constrains typically include physical and chemical characteristics of the soil. Physical constrains typically regard to compaction, reduced water holding capacity and low aeration (Shrestha and Lal, 2011), whereas chemical properties are usually related to low (or high) pH, high TE concentrations, mixtures of TE with other pollutants (e.g., mineral oils, polycyclic aromatic hydrocarbons, pesticides, polychlorinated biphenyls, organochlorines, per- and polyfluoroalkyl substances), low nutrient and OM contents, etc. (Kidd et al., 2015). These soil characteristics depend on ongoing and/or past polluting activities, which in the case of industrial contaminated sites depend on the type of industry and its products (Alloway, 2013). The effects of high TE concentrations depend on their intricate reactions with soil phases, namely through, e.g., dissolution, sorption, complexion, precipitation, which are a function of soil properties (Kabata-Pendias, 2010). For instance, a soil with low pH can increase the ion species of some TE (e.g., metallic cations) dissolved in soil solution, rendering them more bioavailable (Young, 2013). Similarly, climatic conditions also pose critical limitations to phytomanagement success. Temperature shapes plant transpiration, growth and metabolism, as well as water chemistry, thus directly affecting both contaminant uptake and its fate in plant parts and other ecosystem compartments (Bhargava et al., 2012). Likewise, moisture affects plant growth, faunal and microbial activity, and contaminant transport within soil. Prolonged drought induces plant stress, enhancing plant sensitivity to pathogens and herbivory and, notably, reducing plant growth (Kidd et al., 2015). Hence, water management needs to be carefully considered, especially in arid and semi-arid areas that undergo relatively long periods of drought and/or heatwaves, and soils with low water holding capacity (Kidd et al., 2015). Therefore, only plants that successfully withstand all these conditions can be used to phytomanage a contaminated site, especially in the current scenario of climate change. In addition, under such conditions, the selected crops need to achieve economically-viable yields and their products should be non-potentially toxic and fit to the quality standards. A large proportion of the aboveground biomass harvested at phytomanaged sites is from meta(loid)-excluders and therefore free of metal(loid)s excess. Also, root-to-shoot transfer of organic contaminants is frequently low, except for some xenobiotics such as organochlorines. Conversely, some elements (e.g., Ca, Si, Mg, etc.), as well as biomass moisture, may be of concern for the energy sector (Nsanganwimana et al., 2013; Nsanganwimana et al., 2014). Regarding combustion and pyrolysis, both intrinsic alkali metals (e.g., K and Ca) and silica present in the material (which can react to form alkali silicates), and contamination of the harvested biomass by soil, may induce operational problems such as ‘slag’ formation. In particular, for some crops, such as Miscanthus x giganteus and Arundo donax, a delayed harvest can reduce undesirable components (K, Ca, P, S, and N) in the biomass.
According to some studies, TE excess in the biomass can induce changes in heavy hydrocarbons present in tars, bio-oil yield, ash content, and relative evolution of CO2 and H2 in volatiles (reduced CO content) (Edgar et al., 2021). For poplar and willow short rotation coppice (SRC), Zn and Cd concentrations are higher in bark than in wood, decreasing in older branches and trunk. In addition, foliar Zn and Cd concentrations can decrease with growth and successive cuts. Therefore, the selection of the harvested shoot parts and their age are an important factor (Grignet et al., 2020; Grzegórska et al., 2020). Trees growing at brownfield and landfill sites can exhibit higher lignin content than those cultivated in uncontaminated soils due to abiotic stresses, e.g., drought-stress, leading to lower glucose yield (Edgar et al., 2021). In contrast, vetiver plants exposed to Cu excess can display a decrease in lignin and an increase in hemicellulose and cellulose contents, leading to a higher production rate of bioethanol (Geiger et al., 2019).
Metal(loid)-enriched biomass can be processed by torrefaction and pyrolysis for producing biofuels and tars (Bert et al., 2017). Accordingly, the potential emission of volatile TE chemicals at high pyrolysis temperatures, the potential leaching of minerals and organics from chars, and the product quality of the products deserve attention. The TE fate depends on complex and multifactorial processes for all technologies based on thermal conversion (e.g., incineration, pyro-gasification, and pyrolysis) (Edgar et al., 2021). Oxide-forming elements and refractory compounds are often found in ashes and tars. Capture of volatile Cd and As chemicals depends on the filter quality. Ecocatalysts prepared from hyperaccumulators are used in various ways but preparing them with the most essential elements (e.g., Zn, Cu, Co, and Mn) and the least non-essential elements (Cd, As) requires a strong selection of plant species (Clavé et al., 2016). Besides the energy sector (e.g., bioethanol, biodiesel, biogas, and heat), many chemical, physical and biological biomass-processing technologies are reported as pre-treatment and conversion technologies. In the case of anaerobic digestion, some TE excess (e.g., >500 mg Zn kg−1, 20,000 mg Mn kg−1) can decrease the methane content in biogas and daily methane production (Edgar et al., 2021). Essential oils from aromatic plants harvested at phytomanaged sites also did not show TE contamination (Raveau et al., 2020). Similarly, oilseeds from sunflower, hemp, and most Brassicaceae harvested at phytomanaged sites generally do not present TE excess, nor the oil for biodiesel production and other uses.
Several pre-treatments can separate the metal(loid)s from the biomass fraction of interest or on the contrary avoids their release during the process and limit their bioavailability in the biochars produced (Edgar et al., 2021): pre-mixing with chemicals (e.g., MgCO3, FeCl3 and Fe(NO3)3, CaO) before biomass pyrolysis (He et al., 2020); composting (except the methylation of Hg-chemicals); for anaerobic digestion and fermentation, pre-treatment with NaOH enhances the release of biogas and metals from straw; biomass pretreatments with either ethanol organosolv, soda or dilute acid (Asad et al., 2017) and steam explosion (Ziegler-Devin et al., 2019) to release TE before bioethanol production. Post-treatment of conversion products and platform chemicals is also an option (e.g., sorption of arsenicals by Fe hydroxides after solvolysis of Pteris vittata fronds (Carrier et al., 2011). Overall, selection of plant species and cultivars, agricultural practices, harvest timing, etc., can also improve the quality of the harvested biomass compared to the required standards of the biomass-processing technologies.
Phytomanagement of TE-contaminated sites is certainly expanding (Pérez and Eugenio, 2018), but it is still rarely chosen as a remediation technology when compared to conventional physicochemical methods of soil remediation (Kidd et al., 2015; Quintela-Sabarís et al., 2017). As a matter of fact, once a contaminated site is targeted for recovery, the most typical procedure is to engage in faster and more drastic solutions, generally involving the use of physicochemical techniques (e.g., soil replacement and soil washing) and civil engineering works (Ashraf et al., 2019), which often remove the target pollutants at the expense of the destruction of soil integrity and functionality. This is, at least partly, due to technical issues related to the implementation of phyto-based strategies, as well as to the perception of many stakeholders who have a low confidence on the reliability of phytotechnologies (Cundy et al., 2013, 2015; Reinikainen et al., 2016; Ramon and Lull, 2019). Contributing to this is the lack of 1) convincing pilot field applications of plant-based options and 2) specific legal frameworks (Cundy et al., 2016). In any event, phytomanagement can also be handled as a holding-strategy for unused and vacant contaminated sites (Moreira et al., 2021).
In summary, environmental and socioeconomic benefits of phytomanagement options largely depend on specific site requirements, such as the need for amendments and irrigation, specific agronomic techniques, maintenance costs, presence of biomass processing units nearby to decrease costs, etc. Economic benefits obtained from harvested biomass and from other potential end-products can be easily valued, but social and environmental benefits (e.g., ecosystem services) are much more difficult to calculate (Bardos et al., 2016; Kuppens et al., 2018). In any case, different sites with different contamination histories frequently require different technologies for their remediation and recovery, for achieving the desirable goals and end-uses.
Plant Selection
Phytomanagement uses plants that can withstand moderate or high bioavailable levels of TE (and of organic compounds), as well as other abiotic stresses while offering financial returns and environmental gains. The selection of the most suitable plant species for a contaminated site is therefore a critical point and depends on several factors, namely: 1) type, concentration, chemical speciation, bioavailability and location of soil contaminants; 2) physicochemical soil properties (e.g., structure, compaction, fertility, moisture, pH, OM, etc.); 3) water availability; and 4) climatic conditions (e.g., temperature, precipitation, wind, and altitude) (O'Connor et al., 2019); and 5) combined life-cycles of pests and biological auxiliaries. In particular, crops chosen for TE phytostabilization purposes should present a TE-excluder phenotype and avoid TE dispersion by leaching, water and wind erosion (Mench et al., 2007; Ruttens et al., 2006a,b; Mench et al., 2010; Vangronsveld et al., 2009). Plants stabilize TE by root uptake and accumulation, precipitation and adsorption, and by changing their chemical form through pH or redox potential modifications around roots (Mench et al., 2010; Burges et al., 2018; Yan et al., 2020). Conversely, plants meant for TE phytoextraction translocate the TE from roots to shoots and accumulate them in their aboveground tissues (Robinson et al., 2015; Remigio et al., 2020).
Traits such as the level of TE tolerance, growth rate, crop yield, type of life cycle (perennial, annual, and biennial), leaf habit (deciduous, evergreen), growth habit (grasses, shrubs, and trees), water requirements, root depth, susceptibility to diseases/pests, etc. must be taken into account (Kidd et al., 2015). Also, the potential for volatilization (Hg and Se) (Ali et al., 2013) should be carefully anticipated. Importantly, for TE phytostabilization, plants should contain low levels of TE in the harvestable biomass (unlike for phytomining and bioavailable contaminant stripping). In any case, TE uptake and accumulation are TE- and host-specific, can be highly variable within plant species and their populations (different variants or cultivars) (Ruttens et al., 2011), and depends on site specificities. Plants should also be resilient to other abiotic factors often related with contaminated areas, which can include soil nutrient deficiencies, salinity, compaction, etc. (Kidd et al., 2015).
For TE-contaminated sites, local colonizing florae, described as metallophytes (endemic plants found in TE-rich soils) and pseudo-metallophytes (facultative metallophytes, i.e., plants with abilities to grow in both TE and non TE-rich soils) (Favas et al., 2014), should be firstly considered. They present specific traits resulting from the adaption to local harsh conditions that grant them advantages in plant establishment and growth. Furthermore, the use of local colonizing florae prevents ecological site-disturbances that potentially invasive/aggressive species may trigger, by competing with local species and/or acting as ecological disruptors. For instance, Jatropha curcas L., a TE-tolerant bioenergy crop is native in Mexico but in some countries, such as Indonesia, Australia and South Africa, is registered as invasive. This issue can be attenuated by using sterile cultivars, if available, to avoid further colonization, although propagation by stem cuttings is more difficult to prevent. The use of metallophytes in phytomanagement is usually thwarted by their typical low biomass, slow-growing nature and reduced economic value for stakeholders, except when used for phytomining (e.g., Ni by Allyssum species) (Chaney et al., 2007; Remigio et al., 2020). However, they could still be used, for example, as cover crops, intercrops and plant borders in phytomanagement initiatives. Conversely, some pseudo-metallophytes fit the phytomanagement purposes by presenting high biomass and growth rate, while also overcoming constraints posed by abiotic and biotic stresses.
In the past 10–15 years, some energy crops have arisen as most promising in adding value to TE-contaminated areas by generating biomass-based products (gaseous, liquid, and solid) (Grzegórska et al., 2020) that can be converted into different kinds of energy (heat, electricity, and fuel for transportation), while attaining environmental goals. Other industrial crops (e.g., fiber crops), aromatic plants, ornamental plants and biofortified crops are also perfectly suitable for phytomanagement, as one of the commitments of this phytotechnology is to deliver economic benefits for the end-users (Gonsalvesh et al., 2016; Pandey et al., 2016; Rizwan et al., 2018; Grottola et al., 2019; Sidhu et al., 2020).
Greenhouse and mesocosm experiments are often used to test promising plants and favorable clones and cultivars. Besides traditional breeding, mutation and somatic embryogenesis (somaclones) have been used to produce plants with higher tolerance to TE (Herzig et al., 2014; Kolbas et al., 2020). Nonetheless, scarce information still persists regarding the plant performances under natural field conditions. Indeed, TE uptake and accumulations patterns can vary between crops grown in pots and in field. Screening a large range of crop species and cultivars, under different edaphoclimatic conditions, is a prerequisite for assessing robust candidates with ecological restoration capacities for phytomanagement (Zapata-Carbonell et al., 2019). As aforementioned, the plants' life cycle is a factor that has to be taken into account when phytomanaging a contaminated site. Relying on annual plants can entail shortcomings as they have yearly costs related with, e.g., soil ploughing/tillage, irrigation, fertilization and harvest. Additionally, tillage can increase TE dispersion (e.g., through wind erosion and water runoff) and be potentially destructive for soils' surface microbial communities, mainly fungal (Pandey et al., 2015; Burges et al., 2018). Annual plants require rotations or other cropping patterns that, despite bringing benefits to soil health, can further increase the cost of the remediation strategy. Conversely, perennial crops can convey more advantages for remediation. They tend to have deeper roots, which contribute to soil stability, especially when remediating slopes and riverbanks, or when contamination reaches deeper soil layers. Perennial life-cycle plants also tend to be more nutrient-efficient than annual plants, yielding higher biomass and energy, and granting the additional benefit of providing higher carbon sequestration (Burges et al., 2018).
The following sections describe economically valuable crops that can be used for phytomanagement purposes, and their use in selected field trials reported in the period 2010–2020 is summarized in Tables 1 and 2.
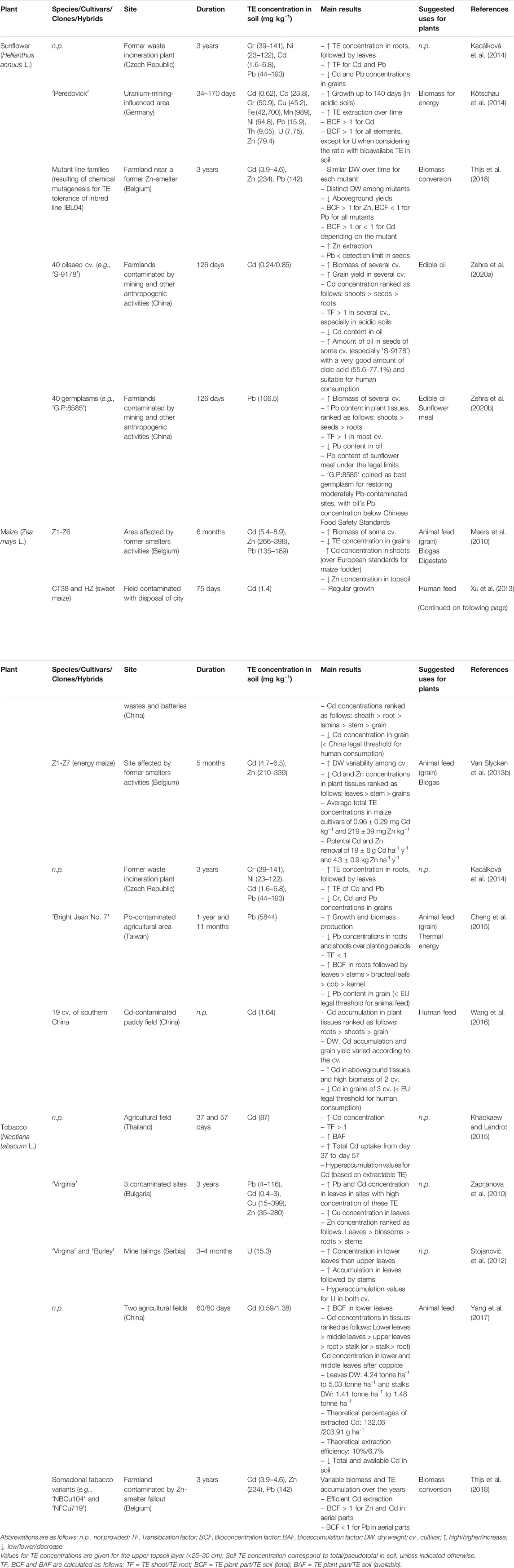
TABLE 1. Examples of field trials with economically valuable annual crops (sunflower, maize, tobacco).
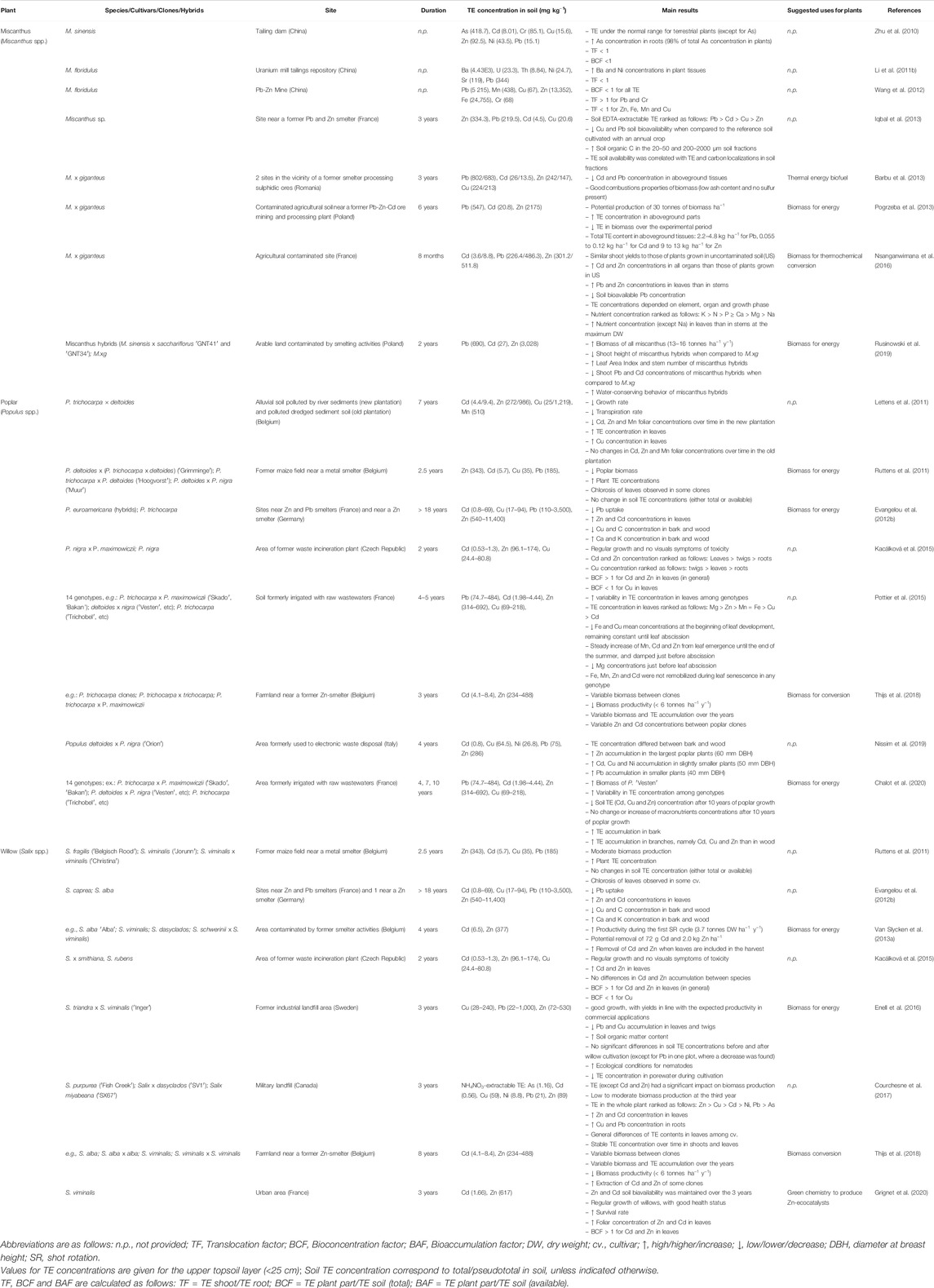
TABLE 2. Examples of field trials with economically valuable perennial and woody crops (miscanthus, poplar and willow).
Annual Crops
Sunflower (Helianthus annuus L.)
Native from northeastern United States (Harter et al., 2004), sunflower is an edible summer annual crop of the Asteraceae family widely cultivated across the globe. It prefers moist and well-drained soils but can maintain a relatively good performance throughout heatwaves and droughts. Alongside its usage for human and livestock consumption (Zehra et al., 2020a,b), sunflower is also a relevant raw feedstock for several technologies depending on the specific plant part under consideration: stalks can be used as insulating materials (Eschenhagen et al., 2019), to produce thermal energy (Wang et al., 2020), bioethanol (Ruiz et al., 2013; Camargo and Sene, 2014), biogas (Hesami et al., 2015), briquettes (Alaru et al., 2013), activated charcoal (Kolbas et al., 2011), and a wide variety of other biocomposites (Mathias et al., 2015; Eschenhagen et al., 2019); seed husks can be employed in the reinforcement of plastic products (Carus and Partanen, 2018); and seed oil can be used for biodiesel production (Kolbas et al., 2011; Ziebell et al., 2013) and medicinal purposes (Bashir et al., 2015). Sunflower can also be used to produce hydrogen fuel (Antonopoulou et al., 2016).
Besides these established industrial, commercial and medicinal applications, its high yields, TE-tolerance and capacity to generate a cluster of biomass-based products support the great potential of sunflower as an attractive crop for phytomanagement. Also, the low TE contents usually present in the seeds and oil from sunflower plants grown in contaminated areas points out to a limited risk of contaminating the food chain (Angelova et al., 2016; Mench et al., 2018), and fosters their use for, e.g., feeding livestock (Mench et al., 2018). Several sunflower cultivars show tolerance to As (Piracha et al., 2019), Cd (Thijs et al., 2018; Zehra et al., 2020a; Table 1), Cu (Kolbas et al., 2011, 2014; Mench et al., 2018), Cr (Aslam et al., 2014), Ni (Ahmad et al., 2011), Pb (Kacálková et al., 2014; Zehra et al., 2020b; Table 1), Zn (Herzig et al., 2014; Marques et al., 2013), U (Kötschau et al., 2014 - Table 1; Meng et al., 2018), Cs and Sr (Brooks, 1998). They are also able to successfully grow in multi-metal(loid) contaminated areas, as is the case of mining (Kötschau et al., 2014; Table 1), industrial (Herzig et al., 2014; Mench et al., 2018) and agricultural sites (Thijs et al., 2018; Table 1). Regardless of their tolerance, sunflowers' response to bioavailable TE excess can be highly variable among cultivars, with some showing higher TE extraction efficiency (Nehnevajova et al., 2005), which can limit crop yield. Therefore, efforts have been made to implement breeding programs aimed at developing new cultivars/hybrids with increased TE-tolerance. For instance, Cd and Zn concentrations contrasted by a factor of ≈2 in 14 sunflower cultivars grown in spiked solutions, with differences being mainly due to uptake and translocation patterns (Laporte et al., 2015). Likewise, attempts to develop variants with increased drought and salt tolerance have been performed (Kane et al., 2013), as these abiotic stressors are frequently found in TE-contaminated soils. Sunflower variants with high TE extraction/stabilization capacity can be obtained by chemical mutagenesis (EMS–ethyl-methane-sulfonate), with some of them being more effective in phytoextracting TE (Cd, Cu, Pb and Zn) in field trials, compared to their mother lines (Herzig et al., 2014; Kolbas et al., 2011; 2018; 2020). These variants also showed a higher activity of antioxidant enzymes (Nehnevajova et al., 2012). In any event, cropping systems are critical drivers of sunflower performance (Kolbas et al., 2011; Rizwan et al., 2016; Mench et al., 2018). For instance, crop rotation can reduce allelopathy and the spread of fungal diseases (Markell et al., 2015). Selected sunflower field trials are described in Table 1.
Maize (Zea mays L.)
Maize is an edible annual plant of the Poaceae family native to southwestern Mexico, where it was domesticated from Balsas Teosinte (Van Heerwaarden et al., 2011). Maize plants prefer fertile, well-drained and moisty soils, and are sensitive to frost, water logging and drought (Wuana and Okieimen, 2010). These liabilities, alongside the high water demand, pose limitations for the use of this crop to recover contaminated areas. Several varieties and cultivars of maize are widely cultivated across the globe for food or livestock forage (Wuana and Okieimen, 2010). However, other valuable economic products can be obtained from maize such as bioethanol (Meers et al., 2010), biogas (Thewys et al., 2010; Van Slycken et al., 2013a - Table 1), digestate for soil conditioning (from which TE can also be recovered) (Meers et al., 2010; Table 1), biomass for production of electrical and thermal energy (Schreurs et al., 2011; Witters et al., 2012a,b; Van Slycken et al., 2013a; Cheng et al., 2015; Rizwan et al., 2017), sweeteners (Ranum et al., 2014), and starch for food and industrial applications (De Vasconcelos et al., 2013). Maize can also be used for the production of charcoal/biochar (Břendová et al., 2015).
Maize has been reported to be tolerant to several TE, e.g., Cd (Van Slycken et al., 2013a; Xu et al., 2013; Kacálková et al., 2014 - Table 1; Moreira et al., 2014; Rizwan et al., 2016), Cu (Jarausch-Wehrheim et al., 1996; Karczewska et al., 2009), Zn (Moreira et al., 2016a,b; Van Slycken et al., 2013a - Table 1), Pb (Cheng et al., 2015; Table 1), and Cr and Ni (Kacálková et al., 2014; Table 1). In fact, maize is usually recognized as a root TE accumulator (Li et al., 2009; Meers et al., 2005). However, this root-accumulating phenotype largely depends on soil properties and genetic variability. Its capacity to withstand high TE levels in soil (Wuana and Okieiman, 2010) coupled with its potential for the generation of biomass-based products, makes maize an interesting annual crop for phytomanagement. Kernels tend to have a lower TE content than stems and roots (Putwattana et al., 2015; Wang et al., 2016; Xu et al., 2013 - Table 1), making them potentially suitable for animal feeding if legal TE thresholds are not surpassed (Meers et al., 2010; Van Slycken et al., 2013a; Cheng et al., 2015; Table 1). Maize cultivars (e.g., Bright Jean Number 7) have been bred in the past decades to cope with rising temperatures and low water supply (Cheng et al., 2015; Table 1). Nonetheless, the use of maize for phytomanagement remains a challenge under the current climate change scenario, especially in dry areas, since it can imply higher costs to stakeholders due to its water requirements or lower yields in arid or semi-arid areas (Meers et al., 2010). High biomass producing cultivars have been developed (Meers et al., 2005) to meet the need for renewable energy sources. These high biomass cultivars are most adequate for biomass production in polluted areas, while mitigating the risk of the spread of contaminants to other environmental compartments. For instance, maize grown in a heavily Pb-contaminated area (6,000 mg kg−1) in Taiwan could produce about ≈1545 GJ ha−1 y−1 of thermal energy or the combination of 25 tons of grain for livestock feeding plus the production of 1172 GJ ha−1 y−1 of thermal energy from the remain plant parts (Cheng et al., 2015). Meers et al. (2010) estimated that as much as around 119 to 166 GJ ha−1 y−1 of electrical and thermal energy could be generated from maize grown in a site contaminated with Cd, Zn, and Pb in Flanders (Belgium). This approach can reduce the emission of CO2 by 21 tons ha−1 y−1, when compared to the use of fossil fuel to generate the same amount of energy. Moderately contaminated agricultural areas could also be used to grow energy maize instead of fodder maize, without loss of income to farmers (Meers et al., 2010; Van Slycken et al., 2013a; Table 1). Some selected field trials demonstrate the feasibility of growing of maize in TE-contaminated sites and are summarized in Table 1.
Tobacco (Nicotiana tabacum L.)
Tobacco is an annually grown herbaceous plant of the Solanaceae family native to South America (Goodspeed, 1954), which is mostly known for cigar production (Popova et al., 2018). Tobacco is a fast-growing crop that yields high biomass (Yang et al., 2017), has a relatively deep rooting system, and prefers well-drained and fertile moisty soils to grow. The optimal conditions for tobacco growth involve 12–13 h of light during its vegetative growth and a temperature around 23.5°C (Yang et al., 2018), which is why most of its production is found in tropical climates (Barla and Kumar, 2019). However, tobacco is a widely adaptable crop, with several cultivars planted in over 120 countries under different climatic conditions (Barla and Kumar, 2019). In the past ten years, tobacco has gathered interest as a bioenergy crop, as it has the potential to produce up to 170 tons of biomass per ha (Barla and Kumar, 2019). However, tobacco can have other applications: 1) its seeds yield up to 30–40% of its dry weight in oil, which can then be used for biodiesel production (Grisan et al., 2016; Barla and Kumar, 2019); 2) seed cakes, an oil extraction by-product rich in proteins and other nutrients (Popova et al., 2018), can be used as a source of protein to feed livestock (Rossi et al., 2013; Serrapica et al., 2019), for human dietary supplements and for cosmetic applications; 3) tobacco leaves can be used as source of protein for humans and animals, and as feedstock of amino acids and other for industrial applications (Yang et al., 2017; Table 1 and 4) the biomass of whole aerial parts can be collected for biogas production (González-González and Cuadros, 2014), bioethanol (Asad et al., 2017), pellets (Rossi et al., 2013; Grisan et al., 2016), and the production of thermal energy through combustion. These important economic applications have fostered tobacco and tobacco-hybrid breeding programs, aimed at delivering high-biomass varieties with greater number of seeds with high oil content to improve its profitability.
Tobacco plants can tolerate and accumulate several TE, including Cd (Fässler et al., 2010a, b; Yang et al., 2017; Thijs et al., 2018—Table 1), which can be accumulated in high amounts in its aerial parts (Khaokaew and Landrot, 2015; Table 1), Zn (Herzig et al., 2014; Lyubenova et al., 2009), Cu (Keller et al., 2003; Kolbas et al., 2020); Pb (Yuan et al., 2011; Zaprjanova et al., 2010—Table 1), U (Stojanović et al., 2012; Table 1 (Wu et al., 2020). However, tobacco can volatilize elemental Hg into the atmosphere (Mani and Kumar, 2014), which raises concerns about the environmental implications of using this plant species in areas rich in this non-essential element. TE-resistant somaclonal variants of tobacco have been selected and tested in Switzerland, Belgium, France, and Spain (Lyubenova et al., 2009; Vangronsveld et al., 2009; Herzig et al., 2014; Kidd et al., 2015; Kolbas et al., 2020). The best-performing variants have shown higher shoot TE concentrations, i.e. 5-7-fold increase for Cu, 2-5-fold increase for Cd, and 1.5-fold increase for Zn, in hydroponics when compared to their mother lines, while differing in their antioxidant content (Guadagnini, 2000; Lyubenova et al., 2009). Such tobacco variants are strong candidates for non-food crop rotations in TE-contaminated soils, but their productivity depends on soil properties (Kolbas et al., 2020). Gonsalvesh et al. (2016) suggested that plant biomass with high TE concentrations should be subjected to pyrolysis, instead of direct combustion, when used for energy production, in order to avoid the release of TE into the environment and to reduce plant volume. These authors further suggested that Cd- and Zn-enriched tobacco biomass could be converted through slow pyrolysis and steam activation to biochar and activated carbon, which could then be used as effective adsorbents for Cr (VI) removal or other applications. Pretreatment (soda, organosolvents, and diluted acid) of metal-rich tobacco shoots is another option for producing bioethanol (Asad et al., 2017). Some field studies describing the potential of tobacco for the phytomanagement of TE-contaminated soils are summarized in Table 1.
Perennial Grass Crops
Miscanthus (Miscanthus spp.)
Miscanthus refers to a genus within the Poaceae family, native to eastern and southeastern of Asia and South Pacific, and comprises approximately 11–12 wild rhizomatous species, and several hybrids and cultivars (Hodkinson et al., 2015). This crop prefers well-drained soils and grows worldwide over tropical and moderate cold temperatures. Miscanthus have an excellent ability to adapt to a wide range of environmental conditions and several genotypes can thrive under low temperatures (Clifton-Brown et al., 2001); in saline (Chen et al., 2017) and dry areas (Van der Weijde et al., 2017), marginal lands and TE-contaminated soils (Li G. Y. et al., 2011; Nsanganwimana et al., 2014, 2015; Rusinowski et al., 2019; Tran et al., 2020). However, frost (Clifton-Brown et al., 2001; Zub et al., 2012), and drought can impair Miscanthus establishment and survival, especially during the first year (Arnoult and Brancourt-Humel, 2015). The most important features of this C4 grass are its high biomass production capacity under low nutrient inputs (Lewandowski et al., 2016, 2018), reaching up 7 m height (Hodkinson et al., 2015), and its water-use efficiency (Heaton et al., 2010), both relevant traits for phytomanagement purposes. By the end of the growing season, Miscanthus can accumulate a large amount of nutrients in its rhizomes, which may reduce the use of fertilizers in the next growing season (Cadoux et al., 2012). Having a lifetime of 20–25 years (Lewandowski et al., 2003), this crop has long been used for several purposes, namely, for preventing wind and water erosion of soils due its extensive root system, and for preventing surface and groundwater contamination by controlling TE leaching (McCalmont et al., 2017). From an economic standpoint, Miscanthus can be used: 1) to produce pulp, fiber (Nsanganwimana et al., 2014; Acikel, 2011) and paper (Xue et al., 2015); 2) as thatching material (Stewart et al., 2009); 3) as bedding for animals (Caslin et al., 2010); and 4) as feedstock for fibers (Acikel, 2011), particle composite boards (Park et al., 2012), polyethylene composites (Chupin et al., 2017), concrete and insulation applications (Eschenhagen et al., 2019), biochar (Houben et al., 2014) and activated carbons (Lim et al., 2019). Apart from these uses, Miscanthus is largely known as an important second-generation bioenergy crop (Yan et al., 2012; Pidlisnyuk et al., 2016), used to produce thermoelectric energy (Brosse et al., 2012; Barbu et al., 2013 - Table 2) and biofuels, such as bioethanol (Van der Weijde et al., 2017) and biogas (Thomas et al., 2019). In addition, it is known due to its contribution to increase C stocks and greenhouse gas mitigation (Zang et al., 2018).
Miscanthus plants are known to tolerate and/or accumulate TE such as As (Zhu et al., 2010; Table 2), Pb (Pogrzeba et al., 2013; Nsanganwimana et al., 2016; Table 2), Sb (Wanat et al., 2013), Cr, Al (Stewart et al., 2009), Cu, Sn, Cd, Zn (Galende et al., 2014; Bang et al., 2015), Ba and Ni (Li G. Y. et al., 2011; Table 2). These TEs are usually accumulated in higher concentrations in roots and rhizomes, followed by stems and leaves (Nsanganwimana et al., 2014). By preferentially accumulating TE in their belowground tissues (Bang et al., 2015; Pandey et al., 2016), Miscanthus biomass should a priori be safe for energy production (Pidlisnyuk et al., 2014). Among Miscanthus, M. floridulus (Li G. Y. et al., 2011; Barbu et al., 2013; Table 2), M. sinensis, M. sacchariflorus and M. x giganteus (sterile triploid hybrid from M. sacchariflorus and M. sinensis; M.xg) are well known to grow in contaminated soils (Table 2). Hybrids have been developed in breeding programs specifically for phytoremediation purposes, favoring traits such as resistance to drought and to high TE concentrations. Rusinowski et al. (2019) established two plantations of Miscanthus hybrids and one of M.xg in a TE-contaminated arable area and showed that hybrids had reduced transpiration rates and lower Pb and Cd concentration in tissues under drought stress than M.xg (see Table 2 for more details). These results highlight the potential benefit of plant breeding programs in improving key plant attributes for phytoremediation purposes. However, plant yields can be highly variable among species, hybrids and clones of Miscanthus (Lewandowski et al., 2016). As M.xg usually express the highest biomass production (Pogrzeba et al., 2013; Arnoult and Brancourt-Humel, 2015; Smith et al., 2015), it is one of the most selected candidates for phytomanagement (Burges et al., 2018). Additional advantages of M.xg include its susceptibility to only a few pests and sterility, which limits its invasiveness capacity. Depending on the clone, M.xg and M. sacchariflorus may have high lignin content, making them suitable for thermochemical conversion processes. Positive effects of Miscanthus on contaminated lands include the increase of: 1) soil microbial biomass and activity (Al Souki et al., 2017), 2) density and diversity of soil macroinvertebrates (Hedde et al., 2013), 3) carbon sequestration (Christensen et al., 2016), and 4) long-term TE phytostabilization (Pavel et al., 2014) with few TE inputs from senescent leaves incorporation into the soils (Al Souki et al., 2020). An additional benefit of Miscanthus is the reduction of soil disturbance, as no tillage is needed for its implementation and maintenance (Nsanganwimana et al., 2014). Nonetheless, the entrance of TE into food chains should be carefully considered. For instance, Zhu et al. (2010; Table 2) addressed potential threats involving M. sinensis in restoring TE-contaminated soils, as As can enter the food chain through its consumption by livestock. Further examples of Miscanthus use in phytomanagement are illustrated in Table 2.
Vetiver (Chrysopogon zizanioides (L.) Roberty)
Vetiver is a high-biomass perennial C4 grass of the Poaceae family (Danh et al., 2009) native to India (Lavania, 2000) that can reach 2–3 m height (Truong, 2002). Vetiver has a dense, thick and deep-rooting system (3–4 m depth in the first year of growth) (Truong, 2002; Truong et al., 2008), making it extremely effective for land stabilization, water filtering and erosion control (Truong et al., 2008; Vargas et al., 2016; Gnansounou et al., 2017). It is widely cultivated in tropical areas but can be found worldwide because of its high adaptability to a wide range of temperatures (−14°C to +55°C), low nutrient requirements, and tolerance to extreme soil conditions, including pH (3.3–12.5) (Truong et al., 2008) and salinity (it can survive in soils with an electrical conductivity up to 47.5 dS m−1) (Danh et al., 2012). Furthermore, vetiver tolerates high TE concentrations, especially Pb, Zn and Cr (Antiochia et al., 2007; Danh et al., 2012). However, it is sensitive to shading, which can limit its establishment and survival (Truong et al., 2008). One of the main applications of vetiver relates to the extraction of essential oil (known as Khus) from its roots, which can then be used in medicinal practices, aromatherapy, cosmetics and as food additive (Prakasa Rao et al., 2008; Lal, 2013). Khus has biocide properties, protecting other crops from detrimental fungi such as Rhizoctonia solani (Dubey et al., 2010), and can also act as termite repellent (Zhu et al., 2001). Apart from its ecological and economic benefits, vetiver can further be used as fodder for livestock (Falola et al., 2013), roof covering (Gnansounou et al., 2017), and to produce ropes, several types of handicrafts (Danh et al., 2012; Gnansounou et al., 2017) and pulp and paper (Darajeh et al., 2019). Furthermore, having a potential biomass production in optimal conditions of over 100 tonnes ha−1 yr−1, vetiver can be used for e.g., producing electricity, while providing environmental benefits through soil carbon sequestration (Danh et al., 2012). The combustion of two tonnes of dry vetiver is estimated to be similar to that of one tonne of coal, resulting in a very attractive financial return as vetiver production is cheaper than extracting and processing coal (Danh et al., 2012). Bioethanol and cellulolytic enzymes are other products that can be obtained from vetiver biomass (Subsamran et al., 2019). Generally, only sterile cultivars are used, including for phytomanagement purposes, due to its non-invasive nature (Wilde et al., 2005).
Vetiver is tolerant to Pb (Danh et al., 2012; Pidatala et al., 2016; Bahraminia et al., 2016), Cu and Zn (Hego et al., 2009; Vargas et al., 2016), B (Angin et al., 2008), Cr (Shahandeh and Hossner, 2000; Danh et al., 2012), Al (Truong, 1999; Danh et al., 2009), Ni (Prasad et al., 2014), Fe (Banerjee et al., 2016, 2019), As (Datta et al., 2011), Cd (Ondo Zue Abaga et al., 2014), and Hg (Danh et al., 2009; Lomonte et al., 2014). TE are usually accumulated in vetiver roots (Phusantisampan et al., 2016), although some ecotypes show higher concentrations in shoots (e.g., Zn in the Ratchaburi ecotype) (Roongtanakiat and Sanoh, 2011), depending on site conditions and time. Moreover, vetiver plants showed the ability to accumulate 134Cs, especially in roots, when grown in 134Cs spiked solutions (Roongtanakiat and Akharawutchayanon, 2017). Therefore, the physiological and morphological characteristics of vetiver, coupled with the profitability of its biomass and derived products, make it an excellent candidate for the phytomanagement of TE-contaminated sites (Danh et al., 2012; Banerjee et al., 2016; 2019; Phusantisampan et al., 2016—Table 4; Gnansounou et al., 2017). Actually, vetiver has been advocated useful for revegetating Pb/Zn mine tailings (Wu et al., 2010) and other mine wastes (Danh et al., 2012). Arochas et al. (2010) showed that vetiver plants were able to grow in the tailings and waste rocks of a high-altitude Cu mine (3,500 m above sea level) and some could survive the cold winter for several years. However, these authors cautioned that higher survival rates required plants to be fertilized, irrigated and protected from grazing. Vetiver has shown potential for Cd phytoextraction (Ondo Zue Abaga et al., 2014) and for its use in gold tailings, to prevent dust storm and wind erosion, and mine rehabilitation (Danh et al., 2012). It was efficiently cultivated in Cu/PAH-contaminated soils at a wood preservation site for more than 10 years (Hego et al., 2009).
Woody Crops
Poplar (Populus spp.)
Poplars are typically deciduous trees of the Salicaceae family (Eckenwalder, 1996), native to North America (Dickmann and Kuzovkina, 2014), that comprise ca. 22–45 species (although this range is not consensual), as well as numerous cultivars and hybrids (Dickmann and Kuzovkina, 2014). Despite being mostly found in alluvial and riparian areas of the Northern Hemisphere, their high genetic diversity makes poplars very adaptable to other areas (Pandey et al., 2016). Currently, poplars are disseminated worldwide and can be found from the tropical regions of South America (e.g., Mexico) to central Asia (e.g., India). Nevertheless, poplars prefer temperate areas and moisty, well-drained and aerated alluvial soils (Stanturf and van Oosten, 2014) with a pH ranging from 5.0 to 7.5 (Baker and Broadfoot, 1979). Poplar trees have a deep and extensive rooting system (Guerra et al., 2011) and are one of the fastest growing trees, with some specimens reaching over 40–50 m height. For this reason, they provide an important contribution for global CO2 emissions mitigation (Krzyżaniak et al., 2019) and soil carbon sequestration (Pandey et al., 2016). Poplar trees are usually sensitive to waterlogging (unless temporary), intensive shading and salinity (Stanturf et al., 2001; Stanturf and van Oosten, 2014), although some species and ecotypes can be tolerant to these conditions. For instance, P. euphratica Olivier, which is considered an important species for foresting saline and alkaline arid areas, is able to thrive under salt stress by changing leaf morphology, developing succulence and presenting a plethora of physiological and molecular adaptations (Chen and Polle, 2010). Poplars can be easily propagated by stem cuttings, which benefits its plantation under SRC where poplars are grown over a period of around 2–5 years cycles. As a bioenergy crop, poplar can be grown for the production of bioethanol (Littlewood et al., 2014) and hydrocarbon biofuel (Crawford et al., 2016), and is used to generate heat and electricity through combustion or gasification processes. A good quality gas (syngas) was produced from the gasification of the biomass of the genotype Monviso–P. generosa x P. nigra, grown in a soil contaminated with a mixture of organic and inorganic contaminants (Aghaalikhani et al., 2017; Ancona et al., 2017). These trees can also be grown over a larger period, e.g., 10–30 years, and then be used to produce biomass for other applications (Barontini et al., 2014), including timber, veneer, pulp and paper (Crawford et al., 2016), resin adhesives (Yang S. et al., 2015), biopolymers and phytochemicals (Devappa et al., 2015). The production of biomass is very variable and depends on poplar genotype, climate and soil conditions (Searle and Malins, 2014).
Poplars have the ability to grow in poor and TE-contaminated soils. They can tolerate TE such as As (Vamerali et al., 2009), Cr (Chandra and Kang, 2016), Cd and Pb (Ruttens et al., 2011 - Table 1; Redovniković et al., 2017), B (Robinson et al., 2007), Zn (Lettens et al., 2011; Evangelou et al., 2012b; Thijs et al., 2018; Nissim et al., 2019; Table 2), Cu (Borghi et al., 2008; Kacálková et al., 2015 - Table 2), Ni (Nissim et al., 2019), Fe (Baldantoni et al., 2014) and Hg (Assad et al., 2016). However, poplar species, cultivars and hybrids vary widely in TE tolerance, accumulation and translocation patterns (Migeon et al., 2009; Ruttens et al., 2011). Baldantoni et al. (2014) grew two TE-tolerant clones, P. nigra ′N12′ and P. alba ′AL22′, in a multi-contaminated soil and showed that the former had a significantly higher Cd concentration in all sampled organs, as well as a Pb translocation factor 50-fold higher, than the latter. Conversely, ′AL22′ showed greater Cu accumulation ability. Although certain TEs, such as Cd and Zn, are preferentially accumulated in poplar shoots (notably in the leaves and young bark, making them useful for phytoextraction), poplars tend to follow the pattern observed for other woody crops of retaining TE in roots (useful for phytostabilization), followed by leaves and stems (Shi et al., 2011; Baldantoni et al., 2014). Poplar species, hybrids and clones can differ in their TE tolerance, which can be detected by a reduction in photosynthesis. As an example, Chandra and Kang (2016) showed a reduction in the photosynthetic rates and pigment contents of poplar hybrids grown in Cd, Cu, Zn and Cr contaminated soil. Only one hybrid (′Eco 28′, P. x euroamericana) showed higher photosynthetic rate, chlorophyll and carotenoid contents at the highest TE concentration tested (500 mg kg−1).
Based on field experiments, poplar SRC emerges as a promising option to phytomanage TE-contaminated soils, yielding high biomass production (Mench et al., 2010; Chalot et al., 2020; Zalesny et al., 2019; Padoan et al., 2020). As an example, a SRC of poplar cv. ′Skado′ was able to produce a stem biomass of over 35 tonnes DW ha−1 over a 4-years growth period when grown in a TE-contaminated site (Ciadamidaro et al., 2017). Poplars may also improve organic carbon content and microbial activity in multi-TE contaminated soils (Ancona et al., 2017). Despite all its positive features, the deciduous nature of poplar trees results in TE-rich leaves to spillback into soil. Pottier et al. (2015) reported that although Mg is remobilized in poplar leaves, the concentration of Fe, Mn, Zn and Cd increases until abscission, which can be reintroduced in soil by OM decomposition (see Table 2 for more details). Poplar hybrids are the most used for phytomanagement as these can be easily developed to exhibit the required traits, such as high biomass production, and tolerance to specific TE and local harsh conditions (Lettens et al., 2011; Ruttens et al., 2011; Evangelou et al., 2012b; Kacálková et al., 2015; Thijs et al., 2018). The use of poplar benefits from well-established sylviculture techniques (Zalesny et al., 2016), which can be translated or adapted to TE-contaminated areas. Some examples of poplar uses in TE-contaminated soils are given in Table 2.
Willow (Salix spp.)
Native to eastern Asia, willows belong to the Salicaceae family (Dickmann and Kuzovkina, 2014) and comprise ca. 450 species and over 200 hybrids (Argus, 1986; Newsholme, 1992). This genus is spread worldwide, although mostly found in the Northern Hemisphere (Argus, 1986). The general attributes and growth requirements of willow trees resemble those of poplars, but they are able to tolerate higher soil moisture, colder temperatures and higher salinity (Mirck and Volk, 2010). Besides, some willow species (e.g.,S. repens L. and S. caprea L.) are adapted to thrive in arid areas (Dickmann and Kuzovkina, 2014). Willows have extensive and deep rooting systems (Licht and Isebrands, 2005), prefer a soil pH of 5.5–8.0 (Abrahamson et al., 2002), and can easily establish in floodplains and sandbars. They are regularly used to stabilize stream banks and prevent erosion (Ens et al., 2013; Karp, 2014). The commercial value of willow is often related to the production of several end-products which can be obtained from its biomass, such as timber, fibers for basketry, charcoal (Karp, 2014), biochar (Rasa et al., 2018), fodder (Lira et al., 2008), pellets, whips and wood chips (Stanturf and van Oosten, 2014), pulp and paper and wood flour (Barton-Pudlik and Czaja, 2018). Willow biomass can also be exploited to produce industrial chemicals, such as polymers and resins (Karp, 2014). Willows are easy to propagate by stem cuttings and can rapidly grow in SRC as poplars (Ens et al., 2013). This capacity has fostered plantations of fast-growing willows to provide biomass and lignocellulosic feedstock for renewable energy production (Mleczek et al., 2010), such as for heating and electricity, as well as for bioethanol (Ziegler-Devin et al., 2019) and biosynthetic natural-gas production (Norman and McManus, 2019). Short-rotation coppices can yield up to 30 tonnes ha−1 y−1, depending on genotype, climate and soil conditions (Stolarski et al., 2015). Usually, a cycle of 2–5 years is applied in commercial plantations (Van Slycken et al., 2013b).
Willows are tolerant to a wide range of TE such as Sn (Dagher et al., 2020), Pb (Evangelou et al., 2012b; Table 2), Cd (Witters et al., 2009; Mleczek et al., 2010), Cu, Zn (Witters et al., 2009; Labrecque et al., 2020; Evangelou et al., 2012b - Table 2), Cr (Quaggiotti et al., 2007), Fe, Ni, As (Jha et al., 2017) and Hg (Mleczek et al., 2010). Analogously to poplars, the willow cultivars and clones used in phytoremediation can display considerable differences in terms of biomass production, and TE tolerance and accumulation (Pulford et al., 2002; Migeon et al., 2009; Ruttens et al., 2011; Van Slycken et al., 2013b; Table 2). French et al. (2006) showed that five willow clones grown in TE-contaminated brownfields highly differed in their biomass production and TE (Cu, Cd and Zn) uptake. Two of those clones were particularly efficient at harvesting Cd, showing a 7 to 9- and 9 to 10-fold increase in TE accumulation in stems and leaves, respectively, compared to EDTA-extractable soil concentrations. Clones can also perform differently according to soil characteristics. Puschenreiter et al. (2013) tested a S. x smithiana Willd. clone in seven TE-contaminated soils (mainly with Zn, Cd and Pb) for ca. 2 years and, although this clone revealed to be a relevant Zn/Cd phytoextractor, its biomass and TE uptake decreased in soils with high bioavailable Zn and Cd levels, especially in low pH and carbonate-free soil. Given the wide trait variability among willow clones, it is essential to select the potential best-performing clones based on their TE tolerance, uptake efficiency (TE accumulating clones for phytoextraction vs. TE excluding clones for phytostabilization), TE translocation from roots to shoots, and biomass production (Pulford and Dickinson, 2005; Unterbrunner et al., 2007; Wieshammer et al., 2007). On the other hand, TE accumulation in willow can differ between the plant parts (roots, twigs, leaves, etc.). The ability of willow trees to recover contaminated sites has successfully been tested in field trials (e.g.,Van Ginneken et al., 2007; Vangronsveld et al., 2009; Mench et al., 2010; Kacálková et al., 2015; Kidd et al., 2015; Enell et al., 2016; Courchesne et al., 2017; Thijs et al., 2018; Xue et al., 2018; Grignet et al., 2020) and some examples are given in Table 2.
Other Crops Suitable for Phytomanagement
Beside the above-described crops, other plant species can be used as cash crops for the phytomanagement of TE-polluted soils. Interesting alternatives include: i) industrial hemp (Cannabis sativa L.), ii) kenaf (Hibiscus cannabinus L.), iii) flax (Linum usitatissimum L.), iv) jute (Corchorus capsularis L.), v) switchgrass (Panicum virgatum L.), vi) tall fescue (Festuca arundinaceae Schreb.), vii) giant reed (A. donax L.); vii) colonial bentgrass (Agrostis capillaris L.), viii) perennial ryegrass (Lolium perenne L.) ix) sudangrass (Sorghum bicolor (L.) Moench), x) reed canary grass (Phalaris arundinaceae L.), xi) physic nut (J. curcas L.), xii) castor (Ricinus communis L.), xiii) common nettle (Urtica dioica L.), xiv) virginia fanpetals (Sida hermaphrodita (L.) Rusby), xv) pongamia (Milletia pinnata (L.) Panigrahi), xvi) babul (Acacia nilotica L.), and xvii) sesban (Sesbania sesban (L.) Merr.) (Table 3). Regrettably, most of these species remain poorly investigated regarding their abilities to phytomanage TE-contaminated sites, especially under real field conditions. In spite of limited data to support its wide application in contaminated soils, industrial hemp is seen as one of the most economically and environmentally rewarding bioenergy crops. This plant species offers a range of relevant features for phytomanagement, such as tolerance to high concentrations of TE in soil (e.g., Cu, Cd, Pb, Zn, Cr and Ni) (Rheay et al., 2020) and, depending on the specific cultivars and site conditions, its potential for phytostabilization or phytoextraction. In addition, industrial hemp can be established and grown with very low agrochemical inputs. Remarkably, all hemp tissues can be used for a wide range of applications, including building materials, textiles, animal bedding, organic compost and biofuel production (Alaru et al., 2013; Crini et al., 2020). Finally, its high cellulose content is a key factor for bioethanol production (Crini et al., 2020).
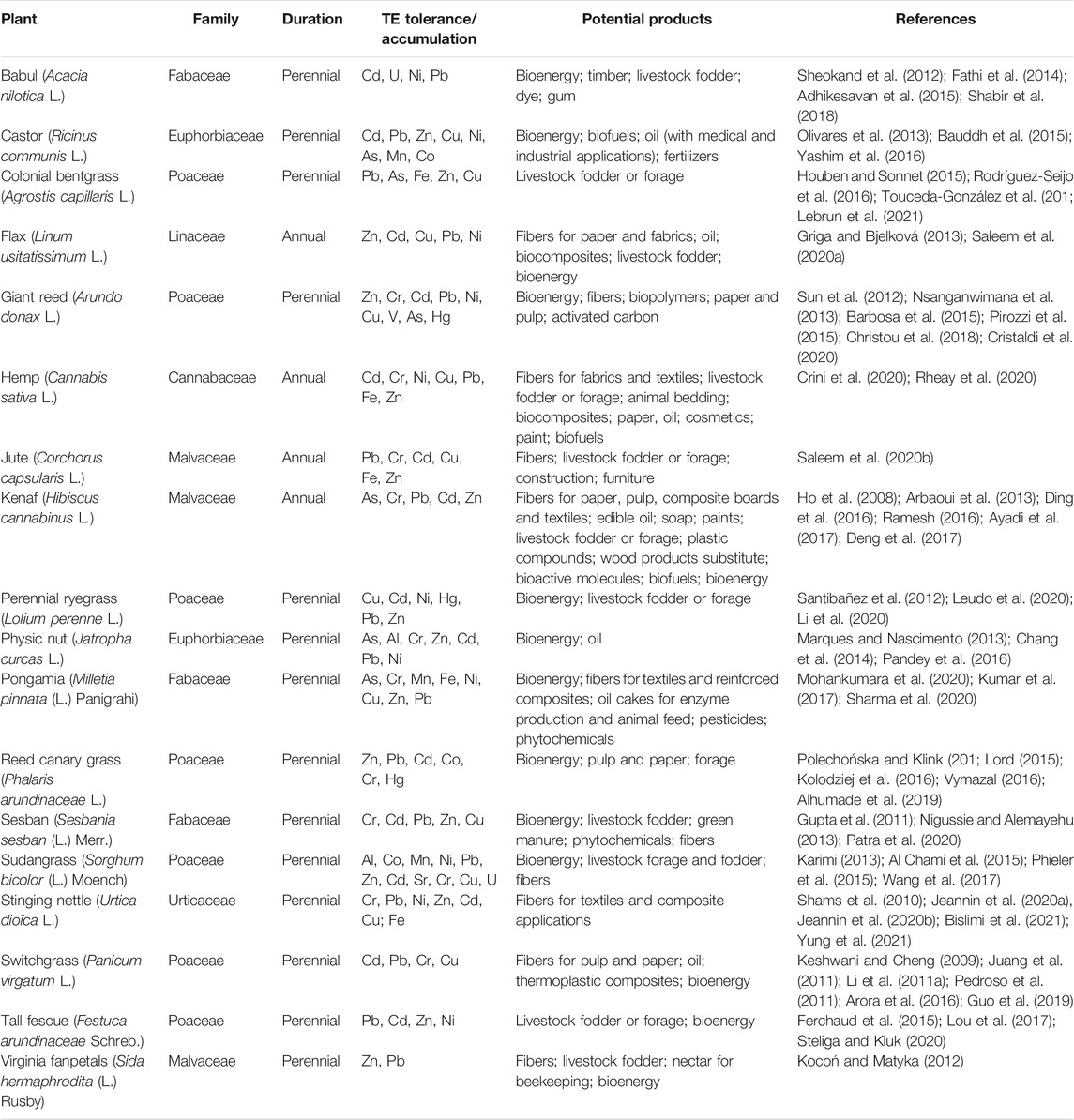
TABLE 3. Other economically valuable crops that can be used in phytomanagement of TE-contaminated sites.
Cropping Systems
Although contaminated soils tend to be strictly interpreted as soils with above background pollutant levels, they often entail other issues that cannot be neglected such as nutrient deficiency, low OM, poor structure, low pH and/or high salinity (Kidd et al., 2015). To tackle these problems and fully grasp the benefits of phytotechnologies to soil remediation and recovery, tailored cropping systems are specifically required. Suitable cropping systems must address the best spatiotemporal organization of cash crops and management practices. Alongside crop selection, cropping systems take into account: 1) crop sequence across years (e.g., monocultures, crop rotation), 2) cropping patterns, i.e. crop spatial arrangement (e.g., intercropping or co-cropping, winter cropping), and 3) management practices (e.g., tillage/no tillage; nutrient management, namely by the use of amendments, fertilizers, foliar sprays; irrigation; weeding) (Bégué et al., 2018). Inoculation of crops with potentially beneficial microorganisms can also be used within cropping systems to optimize remediation processes (see “Bioinoculants” Section). Assessing and weighting the trade-offs among all available cropping systems can critically increase phytomanagement efficiency (Vangronsveld et al., 2009; Kidd et al., 2015). An optimal cropping system should improve crop growth through an efficient use of nutrients and water, increase soil fertility, improve soil ecological functions, and ensure minimal adverse effects of TE on biological recipients (Kidd et al., 2015).
Monocultural options should be avoided during the phytomanagement of contaminated sites. Monocultures of annual crops tend to deplete soil nutrients because they exploit the same nutrient pool across multiple growing seasons. Therefore, monocultural options typically require fertilization (Mench et al., 2010; Herzig et al., 2014; Kidd et al., 2015). Additionally, crops used in monocultural practices are frequently clones, and consequently have low genetic diversity which, when combined with spatial homogeneity, reduces resilience to pests and diseases. For instance, when grown in monoculture, sunflower is commonly affected by white mold caused by the soil-borne fungus Sclerotinia sclerotiorum (Debaeke et al., 2014), while willows and poplars tend to be severely impacted by the insect Chrysomela sp. (Georgi and Müller, 2015). However, the impact of phytopathogens on sunflower can be minimized or even prevented by using crop rotation strategies with non-host crops (Mench et al., 2018). Similarly, using a mixture of cultivars aimed at increasing the genetic pool is a suitable approach for willows and poplars (Reiss and Drinkwater, 2018). This latter approach has the additional advantage of helping plants to cope with adverse edaphoclimatic conditions. Monoculture systems negatively affect biodiversity with concomitant adverse effects for ecosystem recovery and resilience. Crop rotations and/or cropping patterns, including with different cash crops, can improve the recovery of contaminated soils by influencing, for instance, TE bioavailability and crop production (Kidd et al., 2015). Greger and Landberg (2015) showed that growing willow (S. viminalis L.) for 4 years in Cd-contaminated soils led to lower Cd concentrations and increased nutrient content in wheat plants (Triticum aestivum L.) grown later at the same site.
Cover crops are also widely used under rotational and cropping patterns mainly to: 1) increase soil fertility, 2) enhance N and C sequestration (Kaye and Quemada, 2017; García-González et al., 2018); 3) decrease soil erosion (Gómez et al., 2018); 4) improve soil OM (Saleem et al., 2020); 5) improve soil moisture and water quality (García-González et al., 2018); and 6) control weeds and increase the yield of cash crops (Wittwer et al., 2017). Furthermore, cover crops can be used for establishing vegetated borders (Saleem M. et al., 2020). Some of the most used cover crops in contaminated sites are legumes (Fabaceae family), which can endure in N-depleted soils due to their ability to fix atmospheric N2 through a symbiotic relationship with N2-fixing bacteria (rhizobia) on their roots. Nitrogen compounds delivered to the plants are used for growth and development and, in return, rhizobia receive carbohydrates from the plants. At the end of the legume life cycle, N is released to soil. Legumes used in contaminated soils include fava bean (Vicia faba L.), common vetch (V. sativa L.), hairy vetch (V. vilosa L.), alfalfa (Medicago sativa L.), red clover (Trifolium pratense L.), white clover (T. repens L.), birdsfoot trefoil (Locus corniculatus L.) and false indigo (Amorpha fruticosa L). Saad et al. (2018) reported that, when growing in a co-cropping pattern, common vetch was responsible for the observed increase of biomass and Ni accumulation in the hyperaccumulator yellow tuft (Alyssum murale Waldst. & Kit.), as compared to fertilized and non-fertilized monoculture systems. Plants of the Brassicaceae family can also be used as cover crops; interestingly, some of them have potential to be used as cash crops. Examples include mustard (Brassica juncea (L.) Czern.), canola (B. napus L.) and forage radish (Raphanus sativus L.). Brassica species are fast-growers with high TE tolerance, including to Cd (Goswami and Das, 2015; Rizwan et al., 2018), U (Chen et al., 2020), Pb (Bouquet et al., 2017; Gurajala et al., 2019) and Zn (Belouchrani et al., 2016), and its biomass can be used for fuel production (Dhiman et al., 2016). Using a mixture of cover crops is often an efficient option for contaminated sites because they provide complementary benefits. However, the choice of compatible crops and the adjustment of seeding rates are key aspects to prevent overlapping.
Cropping patterns can enhance the recovery of microbial diversity and activity in contaminated soils. Gao et al. (2012) showed that the co-cropping of mustard and tall fescue mitigated the impact of Cd and Pb on soil microbial diversity and enzymatic activities, when compared to unplanted soils. This effect is related not only to the secretion of multiple types of root exudates but also to a decrease in TE availability in soil driven by the accumulating capabilities of mustard (Gao et al., 2012). Likewise, Brereton et al. (2020) showed that the pairwise co-cropping of tall fescue, willow (S. miyabeana Seemen.) and alfalfa promoted higher diversity of rhizosphere bacteria than when each plant was grown in monoculture. Interestingly, this synergistic effect was not detected when all three crops were co-cropped.
Management practices strongly influence the effectiveness of phytotechnologies to recover polluted sites. Despite improving soil aeration and water drainage, tillage practices can affect TE availability. Vamerali et al. (2011) showed that ploughing a TE (As, Cd, Co, Cu, Pb, and Zn) contaminated pyrite waste, capped with non-polluted soil, increased TE availability and improved TE concentrations in sunflower, fodder radish, alfalfa and ryegrass. Tillage practices can also facilitate the proliferation of diseases. For instance, white mold sclerotia can decrease over time in non-till systems but remain constant in till-systems. No-tillage systems can also benefit the diversity and density of soil invertebrates in TE-contaminated soils (Hedde et al., 2013).
The management of TE contaminated sites may include the use of amendments to allow plant establishment and growth, decrease TE bioavailability, and improve soil properties and functions. Alternatively, amendments can be used to increase TE mobility and thus promote phytoextraction. Inorganic amendments (IA) comprise liming agents, phosphates (e.g., H3PO4, KH2PO4, hydroxyapatite, and phosphate rock), Fe/Mn oxyhydroxides and iron grits, natural and synthetic zeolites, alumino-silicates, and cyclonic and fly ashes (Mench et al., 2007; Bolan et al., 2014; Kumpiene et al., 2008, Kumpiene, 2010, Kumpiene et al., 2019). Conversely, organic amendments (OA) include biosolids, green composts, livestock manures, biochar, etc. (Gómez-Sagasti et al., 2018; Kumpiene et al., 2019; Urra et al., 2019a,b). The advantages of OA over IA include their high accessibility and reduced costs. Organic byproducts and residues can be used as OA, although attention must be paid to their content in TEs, organic contaminants, microplastics and antibiotics. The use of OA can promote waste reuse, thus being aligned with the abovementioned CE paradigm. Additional benefits of OA are: 1) provision of macro- and micronutrients, which are commonly limiting factors for plant growth in many TE-contaminated sites; 2) improvement of soil structure and aeration; 3) enhancement of soil OM content; 4) stimulation of soil microorganisms and fauna (Burges et al., 2020); and 5) enhancement of soil moisture and water-holding capacity. Phusantisampan et al. (2016) showed that the application of a pig manure-derived OA to Cd contaminated soil improved its pH and increased its OM and macronutrient content, allowing a better growth of vetiver plants and their phytostabilization potential.
Other bench- and field-scale experiments have also described amendment-induced changes in TE availability and enhanced plant establishment and growth in TE-contaminated soils (Mench et al., 2010; Bolan et al., 2014; Kumpiene et al., 2019). Pavel et al. (2014) added red mud to a soil contaminated with Zn, Cd and Pb to improve the growth of M.xg plants, and observed a significant reduction of soil TE concentration and plant TE uptake which benefited plant biomass (Table 4).
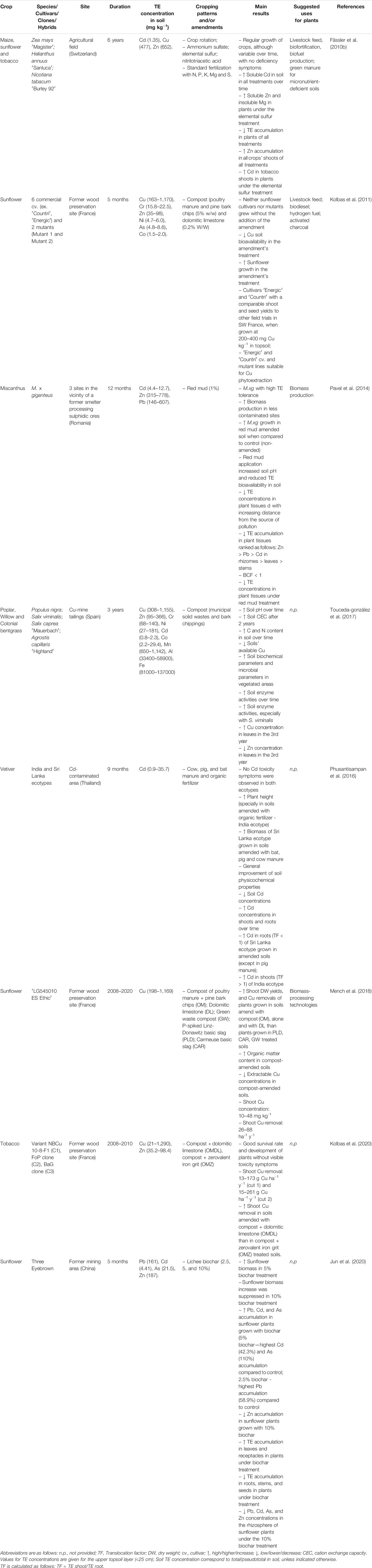
TABLE 4. Examples of field trials with economically valuable crops under different cropping patterns and/or amendments application.
Other management practices to take into account are the adjustment of plant density, irrigation, harvest management, weed and pest control, and the use of pesticides. These practices were thoroughly reviewed in Kidd et al. (2015). However, most of the research has been carried out on the isolated effect of each of these practices, while their combination is only rarely investigated. Finally, the high variability of field site conditions and the wide panel of different amendment types and compositions, cropping systems and plant species complicate the extrapolation and generalization of results. Some examples of field trials with crops under different cropping patterns and/or ammendments application are given in Table 4.
Bioinoculants
As stated, phytoremediation relies on the interactions between soil, plants, and associated microorganisms. Microorganisms are key players for plant growth under suboptimal soil conditions such as those found in soils with TE contamination. Therefore, the inoculation of beneficial microorganisms can help plant survival, establishment, and resilience, and they can also transform contaminants to less toxic forms or change their bioavailability, and consequently, plant uptake (Tiwari and Lata, 2018; Moreira et al., 2019). PGPB, including rhizosphere and endophytic bacteria, and AMF are known to effectively enhance plant growth in contaminated areas, fostering the success of phytomanagement. Some examples on the use of these bioinoculants in phytotechnologies are summarized below.
PGPB
PGPB help plants to cope with high concentrations of TE either by direct or indirect mechanisms acting simultaneously during the different stages of plant cycle (Glick, 2010, 2012; Saharan and Nehra, 2011; Kong and Glick, 2017). Direct mechanisms include 1) the synthesis of phytohormones, such as auxins (e.g., IAA: indole-3-acetic acid), cytokinins, and gibberellins, which are pivotal in regulating plant growth and development (Glick, 2012), and 2) the activity of the enzyme 1-aminocyclopropane-1-carboxylate deaminase (ACC-deaminase). This enzyme hydrolyzes ACC (immediate precursor of ethylene) into α-ketobutyrate and ammonia, thereby reducing the endogenous levels of ethylene in plants, which is often induced by TE excess (Glick, 2014). Several authors have reported the beneficial effects of inoculation with ACC-deaminase and IAA-producing strains on plant growth under TE contamination. Shoot height (+40%) and root length (+100%) of sunflower plants grown on mining wastes increased when inoculated with the IAA and ACC-deaminase producing strain Serratia sp. k120 (Mendoza-Hernández et al., 2016). Likewise, maize plants grown under increasing Pb concentrations and inoculated with bacterial strains having ACC-deaminase activity showed increased growth, photosynthetic pigments and proline contents, and improved antioxidative response (Hassan et al., 2014). Grobelak et al. (2018) also related the higher biomass of B. napus and F. rubra grown in a TE-contaminated soil to the high ACC-deaminase activity of the inoculated bacterial strains. PGPB can also directly favor plant nutrient uptake through the production of siderophores, solubilization of phosphorous, and biological N2 fixation (Glick, 2012). Siderophores have the dual function of Fe chelation, facilitating its root absorption, and phytopathogen inhibition by limiting Fe availability (Ma et al., 2011a). Phosphorus is a key macronutrient for plant growth and development (Hawkesford et al., 2012) and hazardous TE concentrations may hinder P uptake by plants, limiting their growth. Phosphate solubilizing bacteria (PSB) can supply P to plants by converting insoluble inorganic phosphates into available forms through acidification, exchange reactions, and/or production of organic acids (Rodríguez and Fraga, 1999; Sharma et al., 2013). In turn, the mineralization of organic P can also occur by the action of phosphatases excreted by PSB (Richardson et al., 2009). Likewise, some PGPB (e.g., rhizobia, free-living N2-fixing bacteria) are able to transform atmospheric nitrogen into inorganic N forms that can then be taken up by the plants (Kong and Glick, 2017). Indirect plant growth-promoting traits are related to the PGPB ability to decrease or prevent the deleterious effects of phytopathogens on plants. Bacterial strains producing a plethora of compounds, namely antibiotics, hydrogen cyanide (HCN), and lytic enzymes are often used in biocontrol strategies for a broad spectrum of plant pathogens (Saharan and Nehra, 2011). Another positive effect of PGPB inoculation refers to the induction of systemic resistance (ISR) regulated by jasmonate and ethylene signaling pathways (Glick, 2012).
The deployment of efficient bacterial strains can greatly benefit phytomanagement strategies by reducing the harmful effects of hazardous TE levels on the growth of energy plants, including annual (Moreira et al., 2014, 2016a, 2016b, 2019; Gupta et al., 2018; Rosenkranz et al., 2018; Saran et al., 2020), perennial (Babu et al., 2015; Itusha et al., 2019) and woody crops (Wang et al., 2011; Cocozza et al., 2015; Janssen et al., 2015). A non-exhaustive list of the beneficial effects of PGPB, belonging to a large variety of genera (e.g.,Pseudomonas, Bacillus, Psychrobacter, Ralstonia, Chryseobacterium, Klebsiella, Aeromonas, etc.), on energy crops grown in TE-contaminated soils is shown in Table 5. Bioinoculation can increase plant growth in contaminated soils, leading to biomass values similar to those reported for uncontaminated soils (Gupta et al., 2018). However, the effects of bacterial inoculation on plant growth and TE accumulation depend on many factors including strain selection, type and availability of TE, plant species/cultivar, and soil properties (Montalbán et al., 2017; Saran et al., 2020). Moreira et al. (2016a) reported that out of five TE-resistant PGPB inoculated in maize grown in a mine soil containing high Zn and Cd levels, only three efficiently promoted plant growth. Moreover, bacterial inoculation influenced differently TE accumulation in roots, by decreasing Cd and increasing Zn. Likewise, Saran et al. (2020) observed that H. petiolaris plants inoculated with the strain Bacillus proteolyticus ST9 presented higher stem length and lower Pb accumulation than non-inoculated plants, whereas the inoculation of the strain Bacillus paramycoides ST29 decreased the accumulation of Cd and increased Pb content in roots. An alternative strategy to foster plant TE uptake is the combined use of ligands, such as ethylene diaminetetraacetic acid (EDTA), and PGPB. The synergistic use of EDTA and PGPB enhanced maize and sunflower dry biomass, as well as Cu accumulation (Abbaszadeh-Dahaji et al., 2019).
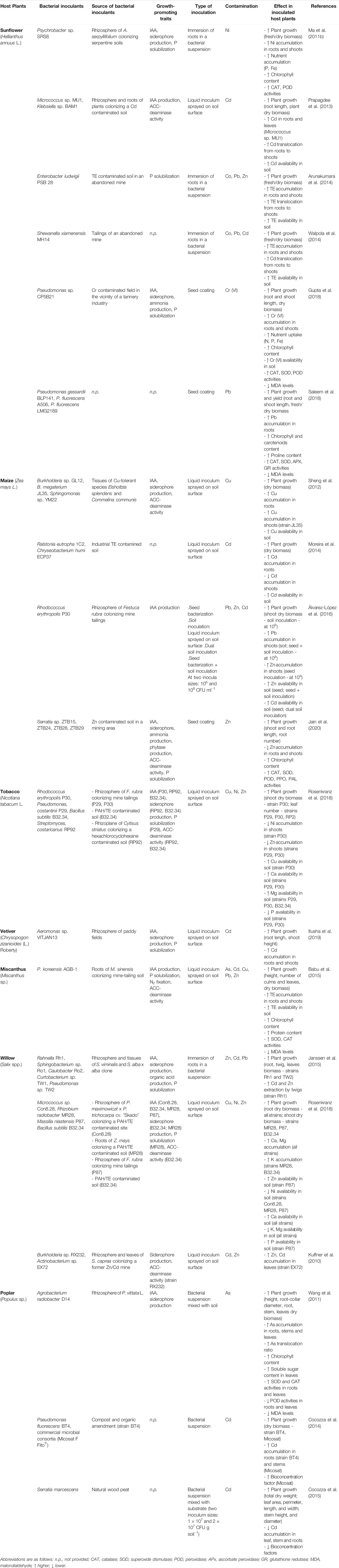
TABLE 5. Effects of PGPB inoculation on growth and development of economically valuable crops grown under TE-contamination.
Some PGPB are effective at decreasing TE availability in soils through the release of extracellular polymeric substances (Guo et al., 2020) that can sequester TE and form stable complexes in soil. Moreira et al. (2016b) reported reduced Zn availability in the rhizosphere of maize inoculated with PGPB. Conversely, bioaugmentation-induced increases of TE availability in rhizosphere soils have also been reported (Sheng et al., 2012; Prapagdee et al., 2013; Arunakumara et al., 2014; Walpola et al., 2014; Babu et al., 2015; Gupta et al., 2018), which can be related to the secretion of siderophores by PGPB that bind TE enhancing their bioavailability and plant uptake (Rajkumar et al., 2010).
The production and accumulation of reactive oxygen species (ROS) in plant tissues triggered by TE hazardous concentrations may also have a negative impact on plant development (Fryzova et al., 2017). Plant responses to ROS lie on the activation of antioxidant defense mechanisms, including enzymatic and non-enzymatic strategies (Apel and Hirt, 2004). Several authors reported enhanced activities of ROS-scavenging enzymes, e.g., superoxide dismutase (SOD), catalase (CAT), and ascorbate peroxidase (APx), when plants are exposed to high levels of TE (Ma et al., 2011b; Wang et al., 2011; Gupta et al., 2018; Saleem et al., 2018). The activity of these enzymes can be further improved by PGPB inoculation, which contributes to reduce oxidative damages in plants, as corroborated by the generalized decrease of malondialdehyde (MDA) contents often observed in inoculated plants (Wang et al., 2011; Gupta et al., 2018; Saleem et al., 2018; Jain et al., 2020).
AMF
AMF are soil-borne fungi that form symbiotic associations with most (80%) terrestrial plants (Smith and Read, 2008). These fungi form specialized structures in the cortical cells of the roots, called arbuscules, vesicles, and hyphae, constituting the intraradical mycelium (IRM). On the other hand, the external network of hyphae, also called extraradical mycelium (ERM) is responsible for translocating nutrients and water to the roots (Smith and Smith, 2011). In TE-polluted soils, AMF have a reinforced importance since they improve soil aggregation, reducing erosion and the risk of TE leaching (He et al., 2020a). Besides, TE can be deposited in the cell wall or the fungal vacuoles, preventing their uptake by plants (Agarwal et al., 2017; Gong and Tian, 2019). Likewise, AMF hyphae produce an insoluble glycoprotein, named glomalin, which forms complexes with TE and, ultimately, reduces their bioavailability (Figure 2) (Miransari, 2010; Gong and Tian, 2019).
AMF can remarkably promote plant growth by improving plant nutrition in TE-contaminated soils. The propagation of ERM in the vicinity of plant roots allows the exploitation of more soil volume, increasing nutrient and water supply to plants (Smith and Smith, 2011). Guo et al. (2013) found increases of 62, 359, and 99% in shoot N, P, and K contents, respectively, in AMF-inoculated maize plants grown in a mixture of mine tailings and topsoil (corresponding increases in the roots were 62, 256, and 141%). Remarkable increases in P accumulation (82% in roots and 300% in shoots) were also observed in AMF-inoculated vetiver plants grown in a substrate containing coal mine wastes (Meyer et al., 2017). Plant growth promotion resulting from AMF inoculation has often been observed in energy crops growing in TE-contaminated soils (Merlos et al., 2016; Sun et al., 2018; He et al., 2020b, Table 6). Nonetheless, the effect of AMF inoculation on TE accumulation in plant tissues is highly variable (Wong et al., 2007; Guo et al., 2013; Meyer et al., 2017; Sun et al., 2018). For instance, the accumulation of TE in roots and shoots of vetiver plants varied according to the AMF species used for bioaugmentation (actually, some species increased TE accumulation while others decreased it) (Meyer et al., 2017). Guo et al. (2013) also reported that although AMF inoculation caused a generalized reduction of TE accumulation in maize tissues, Glomus versiforme was more effective than G. mosseae in this respect. Moreover, according to Sun et al. (2018), AMF inoculation has contrasting effects on TE accumulation in different switchgrass cultivars: inoculated highland cultivars showed a decrease of Cd accumulation, whereas lowland cultivars presented increased concentrations of Cd in their tissues. The effects of AMF on TE accumulation in plants is also highly conditioned by soil TE concentrations. Accordingly, Wong et al. (2007) observed greater accumulation of Pb and Zn in AMF-colonized plants grown under low soil Pb/Zn concentrations (0 and 10 mg kg−1), while inoculated plants grown at higher concentrations (100 and 1,000 mg kg−1) showed lower uptake values for both metals. AMF can also improve plant development under TE contamination by enhancing photosynthetic efficiency (Yang et al., 2015; He et al., 2020a) and antioxidative responses (Sharma et al., 2017).
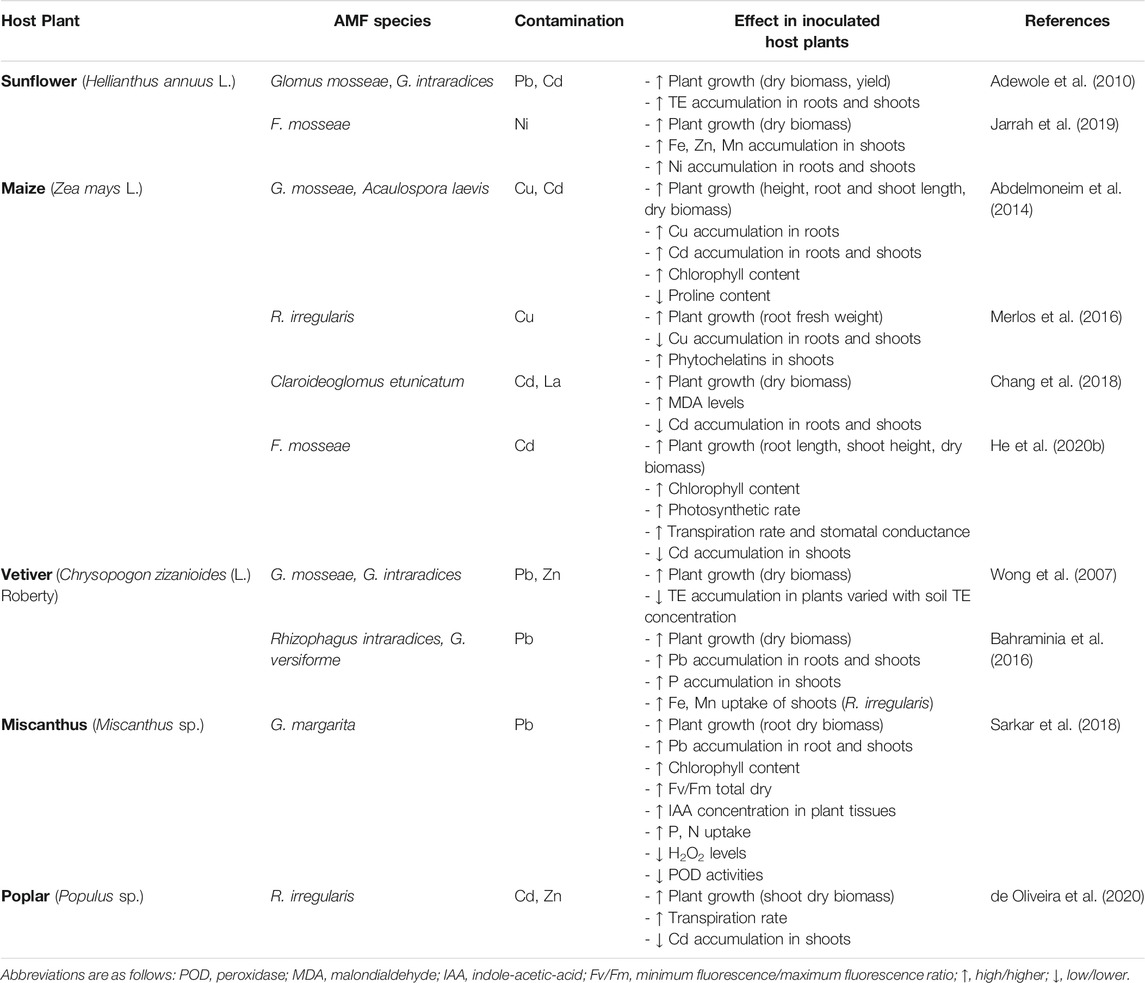
TABLE 6. Effects of AMF inoculation on growth and development of economically valuable crops grown under TE-contamination.
Several studies demonstrate the efficiency of AMF bioaugmentation initiatives in the field. The addition of a commercial mycorrhizal inoculum (SolRize®) induced AMF root colonization and protected Miscanthus × giganteus against the detrimental effects of high concentrations of Cd, Pb, and Zn (Firmin et al., 2015). Mycorrhized plants showed reduced malondialdehyde (MDA) levels in leaves, suggesting that AMF colonization attenuated TE-induced oxidative stress. The same mycorrhizal inoculum (SolRize®) increased Cd and Zn accumulation in M.xg plants grown in TE-contaminated agricultural soils (Nsanganwimana et al., 2015). Likewise, AMF inoculation fostered TE phytostabilization by vetiver (Kafil et al., 2019). Interestingly, mycorrhizal inoculation (commercial inocula with a mixture of ecto- and endomycorrhiza fungi) enhanced, in average, 21% biomass yield of poplars cv. ′Skado′ grown in two Cd, Cu, Pb, and Zn-contaminated fields (Ciadamidaro et al., 2017), and reduced Zn, Cu, Pb, and Cr translocation to leaves (Phanthavongsa et al., 2017). According to Guarino et al. (2018) the combined inoculation (PGPB and mycorrhyzal fungi) of poplar (P. deltoides × P. nigra) and willow (S. purpurea subsp. lambertiana) grown in TE-contaminated field led to a higher TE accumulation in its roots than in non-inoculated plants. Moreover, inoculated plants showed an increased antioxidant enzymatic activity.
Microbial Consortia
The inoculation of plants with a microbial consortia, comprising different groups of microorganisms, on plants exposed to different environmental stresses has deserved special attention in recent years (Oliveira et al., 2005a; Moreira et al., 2016b; Pereira et al., 2016; Mokarram-Kashtiban et al., 2019). Positive interactions can occur between PGPB and AMF, which may synergistically benefit plant growth under TE contamination. The interactive effects of PGPR and AMF on plant growth promotion involve a range of mechanisms (Artursson et al., 2006). AMF can benefit from the metabolites produced by bacterial strains, which increase root cell permeability and consequently root exudation, which in turn promotes hyphal growth and favors root colonization by fungi (Jeffries et al., 2003). Moreover, rhizosphere bacteria can support AMF by enhancing bioavailability of some macronutrients (e.g., N and P), potentiating their role on nutrient acquisition by plants. For instance, N2-fixing bacteria improve the N bioavailability to plants, which may be capitalized by AMF colonization (Barea et al., 2002). Similarly, in soils with low available P levels, PSB may support AMF by releasing phosphate ions from inorganic and organic compounds (Pereira and Castro, 2014), contributing to increase the available P pool in soil, and consequently P acquisition by plants (Smith and Read, 2008). Besides improving plant growth, positive interactions include the increase of their abundance in soil (Marschner and Timonen, 2005). AMF can directly influence the indigenous rhizobacterial community by promoting the production of root exudates, which supply compounds required for bacterial growth (Sood, 2003). On the other hand, AMF survival and root colonization may be improved by PGPB (Hildebrandt et al., 2006; Nadeem et al., 2014).
The application of microbial consortia to energy crops used for the phytomanagement of TE-contaminated soils is still underexplored. However, some encouraging results have been reported. Co-inoculation of the AMF Rhizophagus irregularis and the rhizobacterium Pseudomonas fluorescens in white willow plants exposed to Pb, Cu, and Cd greatly improved plant growth, TE uptake, and the general physiological status (Mokarram-Kashtiban et al., 2019). Likewise, the joint inoculation of two PGPB (Chryseobacterium humi ECP37 and Pseudomonas reactans EDP28) and the AMF R. irregularis increased the biomass of energy maize grown in a mine soil (Moreira et al., 2016b). These authors also found that microbial inoculation led to a general decrease in plant Zn accumulation, which was further evident in those treatments in which the AMF was present. This can be explained by the ability of AMF to adsorb and accumulate TE in their mycelia, limiting their absorption by plants.
Factors Affecting Bioinocula Performance
Once introduced into the soil, bioinocula have to face several constrains that can hinder their survival, persistence, and rhizocompetence (Bashan et al., 2014). Therefore, the selection of microbial strains well-adapted to the local edaphic conditions and their inclusion in an effective carrier are critical points for the success of phytotechnologies in the field (Oliveira et al., 2005b; Ortiz et al., 2015; Malusà et al., 2016). Microorganisms used as bioinoculants for phytomanagement strategies are generally retrieved from contaminated sites and then tested for their in vitro plant growth-promoting (PGP) traits (Pereira et al., 2015; Pires et al., 2017; Tirry et al., 2018). Usually, these PGP traits are evaluated in the absence of stress. However, PGP traits can be disrupted under TE hazardous concentrations, being of utmost importance to perform in vitro tests with relevant exposure to the stressors under consideration. Yet, only a few studies have evaluated the ability of PGPB under TE stressful conditions (Moreira et al., 2016a; Mendoza-Hernández et al., 2016). The selection of PGPB should also have into consideration the TE-induced effects on in vivo seedling growth (Moreira et al., 2016a). Furthermore, the positive effect of PGPB on plant growth observed in TE spiked and/or sterilized soil can be overestimated, being possibly not transferable to real field conditions. In fact, it is recommended to use non-sterilized soil collected at the target site for the assessment of PGPB candidates (Moreira et al., 2019). This approach facilitates the identification of the most effective PGPB under more realistic conditions, including multi-element contamination and competition with indigenous microbial communities.
The inocula size and its application rate are also relevant points. For instance, the application of low cell density inocula often results in low colonization rates, hampering competition with indigenous soil populations (Compant et al., 2010; Shabir et al., 2016). Furthermore, the size of the inoculum can influence TE accumulation in plants and should be considered in phytotechnologies where biomass is the most relevant outcome (Moreira et al., 2016a; Álvarez-López et al., 2016).
To maintain the viability of microbial inoculants and to ensure their efficiency, microbial strains should be incorporated in carriers. Although great progresses have been made in the last decade, the development of stable carrier formulations with ability to provide protection for bioinocula against abiotic and biotic threats is still a challenging task.
Legal and Regulatory Framework
The legislation aimed at protecting soil from contamination mainly covers agricultural practices, e.g., the use of sewage sludge and other organic wastes as amendments (Urra et al., 2019b, c), wastewater irrigation, application of pesticides and fertilizers, as well as industrial and commercial activities. At least 13 pieces of EU legislation apply to contaminated soils (Pérez and Sánchez, 2017). However, legislation/regulations for soil protection and contamination are frequently tailored to each country's views and needs (Ramon and Lull, 2019). As expected, many environmental, economic and social aspects influence the process of decision-making on contaminated land management (CLM) (Reinikainen et al., 2016; Castelo-Grande et al., 2018). These aspects must be rightly balanced in risk management strategies in order to properly define the sustainability of the outcome in policy frameworks. Policy frameworks and functional policy instruments (e.g., inventories, surveys, regulations, guidelines, and funding mechanisms) are requested to reach sustainable practices and must involve environmental administrations and key stakeholders, e.g., authorities, landowners, consultants, industries, communities and academics (Cundy et al., 2016; Reinikainen et al., 2016).
Many international policies have replaced the initial CLM option of a multifunctional land use (i.e., low risk tolerance, exhaustive remediation) by the concepts of risk-based and sustainable land management (SLM) (e.g.,Vegter et al., 2002; Paleari, 2017; MTES, 2018; Ramon and Lull, 2019). Risk-based land management is worldwide addressed in guidance documents, policy papers and case-studies (U. S. EPA, 2008; Ellis and Hadley, 2009; CL: AIRE, 2010; USACE, 2010; ITRC, 2011; Smith and Nadebaum, 2016). Sustainable land management is adopted in the CLM policy frameworks of many countries (e.g., Europe, North-America, Australia and New Zealand) (AEP (Alberta Environment and Parks), 2017), targeted in the EU 7th Environment Action Program (Pérez and Sánchez, 2017), and present in international standards and guidance of ISO and ASTM International (Bardos et al., 2016). Several European countries (e.g., Belgium, Estonia, Austria, Denmark, Sweden, Hungary, Switzerland, France, and Finland) have set up long-term policy targets concerning the management and remediation of contaminated sites (Castelo-Grande et al., 2018; MTES, 2018; Pérez and Eugenio, 2018; Ramon and Lull, 2019). Legislation and issues involved in creating a contaminated site strategy for low- and middle-income countries were reviewed by Kovalick and Montgomery (2014, 2017). Most risk-oriented policies focus on the abandonment of those policies aimed at restoring soils to their original reference state, and consider the acceptable residual risks. Some national trigger values classifying soils as contaminated or requiring remediation have included the bioavailability concept explicitly (e.g., United Kingdom, Belgium, and Switzerland) or implicitly (trigger values set according to the main influencing soil physicochemical properties, e.g. soil pH, granulometry, and OM content). Some phytomanagement options are aimed at removing the bioavailable contaminant fraction (“bioavailable contaminant stripping”), a target which significantly reduces the length of time required for remediation and addresses the pollutant linkages. Some European projects (e.g., Rejuvenate, Greenland, and Hombre) developed decision support frameworks as a holistic approach to return marginal damaged/contaminated land to functional and economic use for producing biomass in line with the bioeconomy paradigm (Bardos et al., 2011; Cundy et al., 2013, 2015, 2016).
The Working Group on Contaminated Sites and Brownfields under the EIONET NRCs Soil set a Land and Soil Indicator (LSI003) for presenting the progress made in the management of contaminated sites by EU Member States and cooperating countries (Pérez and Sánchez, 2017). Relevant current barriers to SLM are not related to sustainability appraisal but the lack of regulatory requirements, client demand and economic considerations (Hou et al., 2014; Rizzo et al., 2016; Bardos et al., 2018). The lack of specific policy instruments and clear guidelines, e.g. on the reuse of contaminated soil, can impede sustainable remediation even in countries with well-developed policies and regulatory frameworks (Reinikainen et al., 2016). One important issue for risk-based land management is the perception of perpetual management of the hazard (source) when the chemical substances are not removed (Bagherifam et al., 2019). Consequently, questions of ongoing liability and transfer of information remain. This requires, from the start of the risk assessment process to 1) educate stakeholders, 2) allow communities to become contributors to scientific knowledge and 3) maintain collaboration with environmental authorities (Cundy et al., 2013). Prospects and threats regarding future land use (e.g., options of site redevelopment and potential long-term liabilities), in line with economic and social uncertainties, influence the risk management strategy and the desired remediation level. Progress toward sustainable remediation is driven by three generic elements: 1) increased recognition of secondary environmental impacts of remediation, 2) stakeholder demand for more sustainable practices, and 3) institutional pressure that promotes sustainable practices (Hou et al., 2014).
Discussion
Total cost of soil remediation in Europe is estimated up to 119 billion euro (Huysegoms et al., 2019). In addition, site remediation is often affected by substantial cost overruns, due to misguided land use and wasteful delays in its reconversion (Barrieu et al., 2017). As outlined in this review, the commercial success of many phytotechnologies much relies on the subsequent processing of biomass for the production of valuable products. Long-term economic studies on the implementation and maintenance of phytomanagement strategies are highly needed to gather data and develop indicators on their economic sustainability, which could then be included in policy frameworks (Reinikainen et al., 2016). These indicators must include the economic benefits derived from the variety of ecosystem services provided by a vegetation cover, that are far from being easy to identify and quantify (Boerema et al., 2017), including avoiding pollutant dispersion and, consequently, reducing potential risks to human health (Burges et al., 2020). Currently, leading practitioners of restoration projects carry out investment analyses in order to estimate total financial costs, so that they can then choose the most convenient project (usually, by means of the application of conventional remediation technologies) from an economical point of view (Gerhardt et al., 2017). On the other hand, those focused only on contaminant remediation per se are usually more concerned with the values of specific environmental and technical indicators. A way to gather consensus is to firstly assess pollutant linkages and associated risks for conventional vs. nature-based remediation solutions, possibly integrating both of them in the management of polluted sites. Importantly, legislation on soil protection has to respect the principle of subsidiarity and proportionality, be in line with advanced research findings, and take into consideration energy expenditure and related issues such as climate change.
Life Cycle Assessment (LCA) has been proposed as an important tool to assess the sustainability of nature-based solutions (O'Connor et al., 2019; Visentin et al., 2019), and has provided valuable insights about positive and adverse impacts of land remediation. LCA studies clearly attested that the sustainable management of contaminated sites can provide positive environmental and socioeconomic outputs (Vigil et al., 2015; O'Connor et al., 2019), bringing meaningful benefits for a country’s development. A central and up-to-date environmental benefit of soil rehabilitation encompasses climate change mitigation, through the increase of soil C sequestration (Paustian et al., 2019; Kasanke et al., 2021; Souki et al., 2021), which can help tackling the net-zero carbon targets in urban and sub-urban areas (Olsson et al., 2019; Bardos et al., 2020). Carbon neutrality is a multinational pledge that is gaining momentum and is supporting efforts toward including degraded land conversion actions in decarbonation strategies (Lask et al., 2021). Additionally, the worldwide scaling up of the demand for renewable energy can also help leveraging the recovery of degraded areas through phytomanagement, with the support and engagement of multiple stakeholders and investors. Finally, it would assist the production of energy for local use, in addition to contributing to the economic development of the region where phytomanagement takes place, through the creation of employment opportunities and the provision of a cheaper supply of bioenergy and biomass.
Several aspects still have to be addressed and improved for pushing forward the phytomanagement of contaminated soils. The technical skills for implementing phytotechnologies are scattered and require an articulation of various disciplines. Priorities are to 1) guide the collection of case study data, and 2) identify and evaluate methods/tools to assess the recovery of soil functions and ecosystem services, without ignoring groundwater pollution during soil remediation. Relevant feasible phytomanagement solutions, focused on contaminant transfer and exposure pathways, must be assessed in the long-term under real field conditions, taking into account at least the site zoning, the conceptual remediation model, the targeted pollutant linkages and the future land use (before full deployment of solutions). Overall, such information will provide experience feedback on plant species (cash crops), plant communities, associated microbes, soil amendments and cultural management, in order to drive the trajectory toward a desired sustainable and resilient novel ecosystem. As many different case studies as possible, e.g., rural and urban land, brownfields, natural ecosystems including marshes, etc. must be evaluated. Methods currently used in Ecology and Ecotoxicology (including bio-monitoring techniques, guides to assess soil functions and ecosystem services, quantification of residual risks) must be adapted to assess those sites under and after phytomanagement. Besides, monitoring of control cards and archiving of samples is highly recommended. The recognition of secondary environmental impacts of remediation initiatives demands for a full assessment of traits over a range of conditions. Long-term economic studies that include revenues derived from biomass produced compared to the costs of inaction or remediation with alternative technologies, are a priority for the implementation and maintenance of phytomanagement. This calls for the multi-actor approach required under the circular economy paradigm where a chain of players from different sectors must be connected. In fact, the success of phytomanagement greatly relies on the feed of the biomass produced into industrial processes for a range of applications (Puschenreiter et al., 2014; Evangelou et al., 2015; Cundy et al., 2016; Jiang et al., 2019). Nevertheless, the use of contaminated biomass for several purposes has been proven at the bench scale but its full-scale use has been yet less clearly demonstrated and is still constrained by a series of regulatory issues. Progress in phytomanagement acceptance across authorities, legislators and the industry sector depend, to a great extent, on the publication and outreach of successful case studies and the identification of reasons for failure. In this respect, scientists, consultants, authorities and landowners must work together to boost, and benefit from, the full potential of phytotechnologies, such as phytomanagement, for an efficient, economically viable and sustainable recovery of contaminated sites.
Conclusion
Managing contaminated land through phytomanagement holds great promise but it is still far from broad and large-scale implementation. Still, the application of nature-based solutions for the recovery of polluted sites is progressively increasing. Long-term field trials will shed light on the environmental, social and economic benefits, which will contribute to gain the trust of stakeholders and investors. The pursuit of soil restoration through a cost-effective and sustainable solution requires a shift in cultural and policies paradigms. Fortunately, halting and reversing land degradation, while ensuring restoration of ecosystem services, is nowadays strongly supported by multiple national and international organizations. In fact, in March 2019, the United Nations declared 2021–2030 the “Decade on Ecosystem Restoration”. Phytomanagement strategies are intrinsically aligned with many of the UN’s 2030 Sustainable Development Goals, but particularly with SDG #15, which aims to “protect, restore and promote sustainable use of terrestrial ecosystems, sustainably manage forests, combat desertification and halt and reverse land degradation and halt biodiversity loss.” Furthermore, The European Parliament has just adopted a resolution for soil protection and the remediation of contaminated soils (European Parliament, 2021).
In this review, we summarized the benefits and drawbacks of phytomanaging metal (loid)-contaminated soils, discussed guidelines for selecting the most suitable crops, and described those used in phytomanagement (encompassing phytoremediation) studies with noteworthy benefits for soil functions. We have also reviewed different cropping patterns that could assist this practice, and addressed the use of bioinoculants, namely stress-tolerant PGPB and/or AMF that can fuel crop establishment and growth in TE-contaminated soils. Although holding great potential, there are still conflicts underlying the use of bioinoculants, which mainly come from unforeseen outcomes concerning the role they should fulfill. Nonetheless, we have identified positive outcomes brought by the bound of bioinoculants and crops. We have analyzed the legal and regulatory framework of soil management and discussed the constraints in the application of phytomanagement across borders, which mainly falls on a lack of common strategies and legislation. More field research is required for understanding the myriad of poorly understood links and mechanisms that still challenge the large-scale employment of phytomanagement. Finally, education and public awareness about soil health and climate change mitigation are of crucial importance to boost the demand for acute and appropriate actions from governments and societal organizations.
Author Contributions
HM and SP conceptualized the review. HM, SP, PK, CG, and MM performed literature review and the critical analysis of the data. HM, SP, CG, and MM wrote sections of the manuscript. CG and PC revised the first draft. All authors contributed to manuscript revision, read, and approved the submitted version.
Funding
Phy2Sudoe - Advancing in the application of innovative phytomanagement strategies in contaminated areas of the SUDOE space (SOE4/P5/E1021) funded by FEDER-Fundo Europeu de Desenvolvimento Regional under Programa INTERREG. The authors would like to thank the scientific collaboration of Fundação Ciência e Tecnologia (FCT) project UIDB/50016/2020.
Conflict of Interest
The authors declare that the research was conducted in the absence of any commercial or financial relationships that could be construed as a potential conflict of interest.
Publisher’s Note
All claims expressed in this article are solely those of the authors and do not necessarily represent those of their affiliated organizations, or those of the publisher, the editors and the reviewers. Any product that may be evaluated in this article, or claim that may be made by its manufacturer, is not guaranteed or endorsed by the publisher.
References
Abbaszadeh-Dahaji, P., Baniasad-Asgari, A., and Hamidpour, M. (2019). The Effect of Cu-Resistant Plant Growth-Promoting Rhizobacteria and EDTA on Phytoremediation Efficiency of Plants in a Cu-Contaminated Soil. Environ. Sci. Pollut. Res. 26, 31822–31833. doi:10.1007/s11356-019-06334-0
Abdelmoneim, T. S., Moussa, T. A. A., Almaghrabi, O. A., and Abdelbagi, I. (2014). Investigation the Effect of Arbuscular Mycorrhizal Fungi on the Tolerance of maize Plant to Heavy Metals Stress. Life Sci. J. 11, 255–263. doi:10.7537/marslsj110414.37
Abrahamson, L. P., Volk, T. A., Kopp, R. F., White, E. H., and Ballard, J. L. (2002). Willow Biomass Producer’s Handbook (Revised). Syracuse: SUNY-ESF.
Acikel, H. (2011). The Use of Miscanthus (Giganteus) as a Plant Fiber in concrete Production. Sci. Res. Essays 6, 2660–2667. doi:10.5897/SRE10.1139
Adewole, M. B., Awotoye, O. O., Ohiembor, M. O., and Salami, A. O. (2010). Influence of Mycorrhizal Fungi on Phytoremediating Potential and Yield of sunflower in Cd and Pb Polluted Soils. J. Agr Sci. Belgrade 55, 17–28. doi:10.2298/jas1001017a
Adhikesavan, C., Rajagopal, K., and Rajaduari, J. S. (2015). Production and Characterization of Biodiesel from Acacia nilotica Seeds. Int. J. Chem.Tech. Res. 8, 854–859.
AEP (Alberta Environment and Parks) (2017). Alberta Risk Management Plan Guide. Available at: https://open.alberta.ca/dataset/ffa8dc73-d464-4aa4-a391 f03428ae728f/resource/b363b5e3-f897-4a29-9630-7534bfbd0056/download/ABRiskManagementGuide-Oct31-2017A-FINAL.pdf (Accessed July 10, 2020).
Agarwal, A., Singh, J., and Singh, A. P. (2017). Arbuscular Mycorrhizal Fungi and its Role in Sequestration of Heavy Metals. Trends Biosci. 10, 4068–4077.
Aghaalikhani, A., Savuto, E., Di Carlo, A., and Borello, D. (2017). Poplar from Phytoremediation as a Renewable Energy Source: Gasification Properties and Pollution Analysis. Energ. Proced. 142, 924–931. doi:10.1016/j.egypro.2017.12.148
Ahmad, M. S. A., Ashraf, M., and Hussain, M. (2011). Phytotoxic Effects of Nickel on Yield and Concentration of Macro- and Micro-nutrients in sunflower (Helianthus annuus L.) Achenes. J. Hazard. Mater. 185, 1295–1303. doi:10.1016/j.jhazmat.2010.10.045
Al Chami, Z., Amer, N., Al Bitar, L., and Cavoski, I. (2015). Potential Use of Sorghum bicolor and Carthamus tinctorius in Phytoremediation of Nickel, lead and Zinc. Int. J. Environ. Sci. Technol. 12 (12), 3957–3970. doi:10.1007/s13762-015-0823-0
Al Souki, K. S., Liné, C., Louvel, B., Waterlot, C., Douay, F., and Pourrut, B. (2020). Miscanthus x giganteus Culture on Soils Highly Contaminated by Metals: Modelling Leaf Decomposition Impact on Metal Mobility and Bioavailability in the Soil–Plant System. Ecotoxicol. Environ. Saf. 199, 110654. doi:10.1016/j.ecoenv.2020.110654
Al Souki, K. S., Louvel, B., Douay, F., and Pourrut, B. (2017). Assessment of Miscanthus x giganteus Capacity to Restore the Functionality of Metal-Contaminated Soils: Ex Situ experiment. Appl. Soil Ecol. 115, 44–52. doi:10.1016/j.apsoil.2017.03.002
Alaru, M., Kukk, L., Astover, A., Lauk, R., Shanskiy, M., and Loit, E. (2013). An Agro-Economic Analysis of Briquette Production from Fibre Hemp and Energy sunflower. Ind. Crops Prod. 51, 186–193. doi:10.1016/j.indcrop.2013.08.066
Alhumade, H., da Silva, J. C. G., Ahmad, M. S., Çakman, G., Yıldız, A., Ceylan, S., et al. (2019). Investigation of Pyrolysis Kinetics and thermal Behavior of Invasive Reed Canary (Phalaris arundinacea) for Bioenergy Potential. J. Anal. Appl. Pyrolysis 140, 385–392. doi:10.1016/j.jaap.2019.04.018
Ali, H., Khan, E., and Sajad, M. A. (2013). Phytoremediation of Heavy Metals-Concepts and Applications. Chemosphere 91 (7), 869–881. doi:10.1016/j.chemosphere.2013.01.075
Alloway, B. J. (2013). Heavy Metals in Soils: Trace Metals and Metalloids in Soils and Their Bioavailability. Environ. Pollut. Book Series 22. Dordrecht: Springer. doi:10.1007/978-94-007-4470-7
Álvarez-López, V., Prieto-Fernández, A., Janssen, J., Herzig, R., Vangronsveld, J., and Kidd, P. S. (2016). Inoculation Methods using Rhodococcus erythropolis strain P30 Affects Bacterial Assisted Phytoextraction Capacity of Nicotiana tabacum. Int. J. Phytoremediation 18, 406–415. doi:10.1080/15226514.2015.1109600
Álvarez-Rogel, J., Peñalver-Alcalá, A., Jiménez-Cárceles, F. J., Carmen Tercero, M., and Nazaret González-Alcaraz, M. (2021). Evidence Supporting the Value of Spontaneous Vegetation for Phytomanagement of Soil Ecosystem Functions in Abandoned Metal(loid) Mine Tailings. Catena 201, 105191. doi:10.1016/j.catena.2021.105191
Ancona, V., Barra Caracciolo, A., Grenni, P., Di Lenola, M., Campanale, C., Calabrese, A., et al. (2017). Plant-assisted Bioremediation of a Historically PCB and Heavy Metal-Contaminated Area in Southern Italy. New Biotechnol. 38, 65–73. doi:10.1016/j.nbt.2016.09.006
Angelova, V. R., Ivanova, R. V., Ivanov, K. I., Perifanova-Nemska, M. N., and Uzunova, G. I. (2016). Potential of sunflower (Helianthus annuus L.) for Phytoremediation of Soils Contaminated with Heavy Metals. World J. Sci. Eng. Technol. 10 (9), 1–8. doi:10.22620/agrisci.2016.20.033
Angin, I., Turan, M., Ketterings, Q. M., and Cakici, A. (2008). Humic Acid Addition Enhances B and Pb Phytoextraction by Vetiver Grass (Vetiveria zizanioides (L.) Nash). Water Air Soil Pollut. 188, 335–343. doi:10.1007/s11270-007-9548-0
Antiochia, R., Campanella, L., Ghezzi, P., and Movassaghi, K. (2007). The Use of Vetiver for Remediation of Heavy Metal Soil Contamination. Anal. Bioanal. Chem. 388 (4), 947–956. doi:10.1007/s00216-007-1268-1
Antonopoulou, G., Vayenas, D., and Lyberatos, G. (2016). Ethanol and Hydrogen Production from sunflower Straw: The Effect of Pretreatment on the Whole Slurry Fermentation. Biochem. Eng. J. 116, 65–74. doi:10.1016/j.bej.2016.06.014
Apel, K., and Hirt, H. (2004). Reactive Oxygen Species: Metabolism, Oxidative Stress, and Signal Transduction. Annu. Rev. Plant Biol. 55, 373–399. doi:10.1146/annurev.arplant.55.031903.141701
Arbaoui, S., Evlard, A., Mhamdi, M. E. W., Campanella, B., Paul, R., and Bettaieb, T. (2013). Potential of Kenaf (Hibiscus cannabinus L.) and Corn (Zea mays L.) for Phytoremediation of Dredging Sludge Contaminated by Trace Metals. Biodegradation 24 (4), 563–567. doi:10.1007/s10532-013-9626-5
Argus, G. W. (1986). The Genus Salix (Salicaceae) in the southeastern United States. Syst. Bot. Monogr. 9, 1–170. doi:10.2307/25027618
Arnoult, S., and Brancourt-Hulmel, M. (2015). A Review on Miscanthus Biomass Production and Composition for Bioenergy Use: Genotypic and Environmental Variability and Implications for Breeding. Bioenergy Res. 8, 502–526. doi:10.1007/s12155-014-9524-7
Arochas, A., Volker, K., and Fonceca, R. (2010). “Application of of Vetiver Grass for Mine Sites Rehabilitation in Chile,” in Latin American Vetiver Conference, Santiago, Chile, Oct. 2010. Santiago.
Arora, K., Sharma, S., and Monti, A. (2016). Bio-remediation of Pb and Cd Polluted Soils by Switchgrass: A Case Study in India. Int. J. Phytorem. 18 (7), 704–709. doi:10.1080/15226514.2015.1131232
Artursson, V., Finlay, R. D., and Jansson, J. K. (2006). Interactions between Arbuscular Mycorrhizal Fungi and Bacteria and Their Potential for Stimulating Plant Growth. Environ. Microbiol. 8, 1–10. doi:10.1111/j.1462-2920.2005.00942.x
Arunakumara, K., Walpola, B. C., Song, J.-S., Shin, M.-J., Lee, C.-J., and Yoon, M.-H. (2014). Phytoextraction of Heavy Metals Induced by Bioaugmentation of a Phosphate Solubilizing Bacterium. Korean J. Environ. Agric. 33, 220–230. doi:10.5338/kjea.2014.33.3.220
Asad, M., Menana, Z., Ziegler-Devin, I., Bert, V., Chalot, M., Herzig, R., et al. (2017). Pretreatment of Trace Element-Enriched Biomasses Grown on Phytomanaged Soils for Bioethanol Production. Ind. Crops Prod. 107, 63–72. doi:10.1016/j.indcrop.2017.05.028
Ashraf, S., Ali, Q., Zahir, Z. A., Ashraf, S., and Asghar, H. N. (2019). Phytoremediation: Environmentally Sustainable Way for Reclamation of Heavy Metal Polluted Soils. Ecotoxicol. Environ. Saf. 174, 714–727. doi:10.1016/j.ecoenv.2019.02.068
Aslam, U., Ahmad, I., Hussain, M., Khan, A., Ghani, A., Mustafa, I., et al. (2014). Effect of Heavy Metal Pollution on Mineral Absorption in Sunflower (Helianthus annuus L.) Hybrids. Acta Physiol. Plant 36, 101–108. doi:10.1007/s11738-013-1390-y
Assad, M., Parelle, J., Cazaux, D., Gimbert, F., Chalot, M., and Tatin-Froux, F. (2016). Mercury Uptake into poplar Leaves. Chemosphere 146, 1–7. doi:10.1016/j.chemosphere.2015.11.103
Ayadi, R., Hanana, M., Mzid, R., Hamrouni, L., Khouja, M. L., and Salhi Hanachi, A. (2017). Hibiscus cannabinus L. - Kenaf: a Review Paper. J. Nat. Fibers 14 (4), 466–484. doi:10.1080/15440478.2016.1240639
Babu, A. G., Shea, P. J., Sudhakar, D., Jung, I.-B., and Oh, B.-T. (2015). Potential Use of Pseudomonas koreensis AGB-1 in Association with Miscanthus sinensis to Remediate Heavy Metal(loid)-Contaminated Mining Site Soil. J. Environ. Manage. 151, 160–166. doi:10.1016/j.jenvman.2014.12.045
Bagherifam, S., Brown, T. C., Fellows, C. M., and Naidu, R. (2019). Derivation Methods of Soils, Water and Sediments Toxicity Guidelines: A Brief Review with a Focus on Antimony. J. Geochem. Explor. 205, 106348. doi:10.1016/j.gexplo.2019.106348
Bahraminia, M., Zarei, M., Ronaghi, A., and Ghasemi-Fasaei, R. (2016). Effectiveness of Arbuscular Mycorrhizal Fungi in Phytoremediation of lead- Contaminated Soil by Vetiver Grass. Int. J. Phytorem. 18 (7), 730–737. doi:10.1080/15226514.2015.1131242
Baker, J. B., and Broadfoot, W. M. (1979). A Practical Field Method of Site Evaluation for Commercially Important Hardwoods. General Technical Report SO-36. New Orleans, Louisiana: USDA Forest Service Southern Forest and Range Experiment Station.
Baldantoni, D., Cicatelli, A., Bellino, A., and Castiglione, S. (2014). Different Behaviours in Phytoremediation Capacity of Two Heavy Metal Tolerant poplar Clones in Relation to Iron and Other Trace Elements. J. Environ. Manage. 146, 94–99. doi:10.1016/j.jenvman.2014.07.045
Banerjee, R., Goswami, P., Lavania, S., Mukherjee, A., and Lavania, U. C. (2019). Vetiver Grass Is a Potential Candidate for Phytoremediation of Iron Ore Mine Spoil Dumps. Ecol. Eng. 132, 120–136. doi:10.1016/j.ecoleng.2018.10.012
Banerjee, R., Goswami, P., Pathak, K., and Mukherjee, A. (2016). Vetiver Grass: an Environment Clean-Up Tool for Heavy Metal Contaminated Iron Ore Mine-Soil. Ecol. Eng. 90, 25–34. doi:10.1016/j.ecoleng.2016.01.027
Bang, J., Kamala-Kannan, S., Lee, K.-J., Cho, M., Kim, C.-H., Kim, Y.-J., et al. (2015). Phytoremediation of Heavy Metals in Contaminated Water and Soil Using Miscanthus sp. Goedae-Uksae 1. Int. J. Phytorem. 17 (6), 515–520. doi:10.1080/15226514.2013.862209
Barbosa, B., Boléo, S., Sidella, S., Costa, J., Duarte, M. P., Mendes, B., et al. (2015). Phytoremediation of Heavy Metal-Contaminated Soils Using the Perennial Energy Crops Miscanthus spp. and Arundo donax L. Bioenerg. Res. 8, 1500–1511. doi:10.1007/s12155-015-9688-9
Barbu, C. H., Pavel, P. B., Sand, C., and Pop, M. R. (2013). Reduced Uptake of Cd and Pb by Miscanthus sinensis x giganteus Cultivated on Polluted Soil and its Use as Biofuel. Environ. Eng. Manag. J. 12 (2), 233–236. doi:10.30638/eemj.2013.026
Bardos, P., Spencer, K. L., Ward, R. D., Maco, B. H., and Cundy, A. B. (2020). Integrated and Sustainable Management of post-industrial Coasts. Front. Environ. Sci. 8.
Bardos, R. P., Bone, B., Andersson-Sköld, Y., Suer, P., Track, T., and Wagelmans, M. (2011). Crop-based Systems for Sustainable Risk-Based Land Management for Economically Marginal Damaged Land. Remediation 21, 11–33. doi:10.1002/rem.20297
Bardos, R. P., Bone, B. D., Boyle, R., Evans, F., Harries, N. D., Howard, T., et al. (2016). The Rationale for Simple Approaches for Sustainability Assessment and Management in Contaminated Land Practice. Sci. Total Environ. 563-564, 755–768. doi:10.1016/j.scitotenv.2015.12.001
Bardos, R., Thomas, H., Smith, J., Harries, N., Evans, F., Boyle, R., et al. (2018). The Development and Use of Sustainability Criteria in SuRF-UK's Sustainable Remediation Framework. Sustainability 10, 1781. doi:10.3390/su10061781
Barea, J.-M., Azcón, R., and Azcón-Aguilar, C. (2002). Mycorrhizosphere Interactions to Improve Plant Fitness and Soil Quality. Antonie Van Leeuwenhoek 81, 343–351. doi:10.1023/a:1020588701325
Barla, F. G., and Kumar, S. (2019). Tobacco Biomass as a Source of Advanced Biofuels. Biofuels 10 (3), 335–346. doi:10.1080/17597269.2016.1242684
Barontini, M., Scarfone, A., Spinelli, R., Gallucci, F., Santangelo, E., Acampora, A., et al. (2014). Storage Dynamics and Fuel Quality of poplar Chips. Biomass Bioenergy 62, 17–25. doi:10.1016/j.biombioe.2014.01.022
Barrieu, P., Bellamy, N., and Sinclair-Desgagné, B. (2017). Assessing Contaminated Land Cleanup Costs and Strategies. Appl. Math. Model. 42, 478–492. doi:10.1016/j.apm.2016.10.015
Barton-Pudlik, J., and Czaja, K. (2018). Fast-growing Willow (Salix viminalis) as a Filler in Polyethylene Composites. Composites B: Eng. 143, 68–74. doi:10.1016/j.compositesb.2018.01.031
Bashan, Y., de-Bashan, L. E., Prabhu, S. R., and Hernandez, J.-P. (2014). Advances in Plant Growth-Promoting Bacterial Inoculant Technology: Formulations and Practical Perspectives (1998-2013). Plant Soil 378, 1–33. doi:10.1007/s11104-013-1956-x
Bashir, T., Zahara, K., Haider, S., and Tabassum, S. (2015). Chemistry, Pharmacology and Ethnomedicinal Uses of Helianthus annuus (sunflower): a Review. Pab 4 (2), 226–235. doi:10.19045/bspab.2015.42011
Bauddh, K., Singh, K., Singh, B., and Singh, R. P. (2015). Ricinus communis: A Robust Plant for Bio-Energy and Phytoremediation of Toxic Metals from Contaminated Soil. Ecol. Eng. 84, 640–652. doi:10.1016/j.ecoleng.2015.09.038
Bégué, A., Arvor, D., Bellon, B., Betbeder, J., De Abelleyra, D., Ferraz, R. P. D., et al. (2018). Remote Sensing and Cropping Practices: A Review. Remote Sensing 10 (1), 99. doi:10.3390/rs10010099
Belouchrani, A. S., Mameri, N., Abdi, N., Grib, H., Lounici, H., and Drouiche, N. (2016). Phytoremediation of Soil Contaminated with Zn Using Canola (Brassica napus L). Ecol. Eng. 95, 43–49. doi:10.1016/j.ecoleng.2016.06.064
Bert, V., Allemon, J., Sajet, P., Dieu, S., Papin, A., Collet, S., et al. (2017). Torrefaction and Pyrolysis of Metal-Enriched Poplars from Phytotechnologies: Effect of Temperature and Biomass Chlorine Content on Metal Distribution in End-Products and Valorization Options. Biomass Bioenergy 96, 1–11. doi:10.1016/j.biombioe.2016.11.003
Bhargava, A., Carmona, F. F., Bhargava, M., and Srivastava, S. (2012). Approaches for Enhanced Phytoextraction of Heavy Metals. J. Environ. Manage. 105, 103–120. doi:10.1016/j.jenvman.2012.04.002
Bihanic, C., Richards, K., Olszewski, T. K., and Grison, C. (2020). Eco-Mn Ecocatalysts: Toolbox for Sustainable and Green Lewis Acid Catalysis and Oxidation Reactions. Chemcatchem. 12, 1529–1545. doi:10.1002/cctc.201901845
Bislimi, K., Halili, J., Sahiti, H., Bici, M., Mazreku, I., Al Tawaha, A. R., et al. (2021). Effect of Mining Activity in Accumulation of Heavy Metals in Soil and Plant (Urtica dioica L.). J. Ecol. Eng. 22 (1), 1–7. doi:10.12911/22998993/128691
Boerema, A., Rebelo, A. J., Bodi, M., Esler, K. J., and Meire, P. (2017). Are Ecosystem Services Adequately Quantified? J. Appli. Ecol. 54 (2), 358–370.
Bolan, N., Kunhikrishnan, A., Thangarajan, R., Kumpiene, J., Park, J., Makino, T., et al. (2014). Remediation of Heavy Metal(loid)s Contaminated Soils - to Mobilize or to Immobilize? J. Hazard. Mater. 266, 141–166. doi:10.1016/j.jhazmat.2013.12.018
Borghi, M., Tognetti, R., Monteforti, G., and Sebastiani, L. (2008). Responses of Two poplar Species (Populus alba and Populus x canadensis) to High Copper Concentrations. Environ. Exp. Bot. 62 (3), 290–299. doi:10.1016/j.envexpbot.2007.10.001
Bouquet, D., Braud, A., and Lebeau, T. (2017). Brassica juncea Tested on Urban Soils Moderately Contaminated by lead: Origin of Contamination and Effect of Chelates. Int. J. Phytorem. 19 (5), 425–430. doi:10.1080/15226514.2016.1244160
Brereton, N. J. B., Gonzalez, E., Desjardins, D., Labrecque, M., and Pitre, F. E. (2020). Co-cropping with Three Phytoremediation Crops Influences Rhizosphere Microbiome Community in Contaminated Soil. Sci. Total Environ. 711, 135067. doi:10.1016/j.scitotenv.2019.135067
Brooks, R. R. (1998). Plants that Hyperaccumulate Heavy Metals: Their Role in Phytoremediation, Microbiology, Archaeology, mineral Exploration, and Phytomining. New York: CAB International Press, 380.
Brosse, N., Dufour, A., Meng, X., Sun, Q., and Ragauskas, A. (2012). Miscanthus: a Fast-Growing Crop for Biofuels and Chemicals Production. Biofuels, Bioprod. Bioref. 6 (5), 580–598. doi:10.1002/bbb.1353
Burges, A., Alkorta, I., Epelde, L., and Garbisu, C. (2018). From Phytoremediation of Soil Contaminants to Phytomanagement of Ecosystem Services in Metal Contaminated Sites. Int. J. Phytorem. 20, 384–397. doi:10.1080/15226514.2017.1365340
Burges, A., Epelde, L., Benito, G., Artetxe, U., Becerril, J. M., and Garbisu, C. (2016). Enhancement of Ecosystem Services during Endophyte-Assisted Aided Phytostabilization of Metal Contaminated Mine Soil. Sci. Total Environ. 562, 480–492. doi:10.1016/j.scitotenv.2016.04.080
Burges, A., Epelde, L., Blanco, F., Becerril, J. M., and Garbisu, C. (2017). Ecosystem Services and Plant Physiological Status during Endophyte-Assisted Phytoremediation of Metal Contaminated Soil. Sci. Total Environ. 584-585, 329–338. doi:10.1016/j.scitotenv.2016.12.146
Burges, A., Fievet, V., Oustriere, N., Epelde, L., Garbisu, C., Becerril, J. M., et al. (2020). Long-term Phytomanagement with Compost and a sunflower - Tobacco Rotation Influences the Structural Microbial Diversity of a Cu-Contaminated Soil. Sci. Total Environ. 700, 134529. doi:10.1016/j.scitotenv.2019.134529
Břendová, K., Tlustoš, P., and Száková, J. (2015). Can Biochar from Contaminated Biomass Be Applied into Soil for Remediation Purposes? Water Air Soil Pollut. 226 (6), 193. doi:10.1007/s11270-015-2456-9
Cocozza, C., Trupiano, D., Lustrato, G., Alfano, G., Vitullo, D., Falasca, A., et al. (2015). Challenging Synergistic Activity of poplar-bacteria Association for the Cd Phytostabilization. Environ. Sci. Pollut. Res. 22, 19546–19561. doi:10.1007/s11356-015-5097-z
Cadoux, S., Riche, A. B., Yates, N. E., and Machet, J. M. (2012). Nutrient Requirements of Miscanthus x giganteus: Conclusions from a Review of Published Studies. Biomass Bioenergy 38, 14–22. doi:10.1016/j.biombioe.2011.01.015
Camargo, D., and Sene, L. (2014). Production of Ethanol from the Hemicellulosic Fraction of sunflower Meal Biomass. Biomass Conv. Bioref. 4, 87–93. doi:10.1007/s13399-013-0096-0
Carrier, M., Loppinet-Serani, A., Absalon, C., Marias, F., Aymonier, C., and Mench, M. (2011). Conversion of Fern (Pteris vittata L.) Biomass from a Phytoremediation Trial in Sub- and Supercritical Water Conditions. Biomass Bioenergy 35, 872–883. doi:10.1016/j.biombioe.2010.11.007
Carus, M., and Partanen, A. (2018). Natural Fibre-Reinforced Plastics: Establishment and Growth in Niche Markets. Hürth: Nova InstituteGmbH.
Caslin, B., Finnan, J., and McCracken, A. (2010). Miscanthus: Best Practice Guidelines. Hillsborough, Ireland: Teagasc, P. 46.
Castelo-Grande, T., Augusto, P. A., Fiúza, A., and Barbosa, D. (2018). Strengths and Weaknesses of European Soil Legislations: The Case Study of Portugal. Environ. Sci. Pol. 79, 66–93. doi:10.1016/j.envsci.2017.10.010
Chalot, M., Girardclos, O., Ciadamidaro, L., Zappelini, C., Yung, L., Durand, A., et al. (2020). Poplar Rotation Coppice at a Trace Element-Contaminated Phytomanagement Site: A 10-year Study Revealing Biomass Production, Element export and Impact on Extractable Elements. Sci. Total Environ. 699, 134260. doi:10.1016/j.scitotenv.2019.134260
Chandra, R., and Kang, H. (2016). Mixed Heavy Metal Stress on Photosynthesis, Transpiration Rate, and Chlorophyll Content in poplar Hybrids. For. Sci. Technol. 12 (2), 55–61. doi:10.1080/21580103.2015.1044024
Chaney, R. L., Angle, J. S., Broadhurst, C. L., Peters, C. A., Tappero, R. V., and Sparks, D. L. (2007). Improved Understanding of Hyperaccumulation Yields Commercial Phytoextraction and Phytomining Technologies. J. Environ. Qual. 36, 1429–1443. doi:10.2134/jeq2006.0514
Chaney, R. L., Baker, A. J. M., and Morel, J. L. (2018). “The Long Road to Developing Agromining/phytomining,” in Agromining: Farming for Metals: Extracting Unconventional Resources Using Plants. Editors A. Van der Ent, G. Echevarria, A.J.M. Baker, and J.L. Morel (Cham: Springer), 1–17. doi:10.1007/978-3-030-58904-2_1
Chang, F.-C., Ko, C.-H., Tsai, M.-J., Wang, Y.-N., and Chung, C.-Y. (2014). Phytoremediation of Heavy Metal Contaminated Soil by Jatropha Curcas. Ecotoxicology 23 (10), 1969–1978. doi:10.1007/s10646-014-1343-2
Chang, Q., Diao, F.-w., Wang, Q.-f., Pan, L., Dang, Z.-h., and Guo, W. (2018). Effects of Arbuscular Mycorrhizal Symbiosis on Growth, Nutrient and Metal Uptake by maize Seedlings (Zea mays L.) Grown in Soils Spiked with Lanthanum and Cadmium. Environ. Pollut. 241, 607–615. doi:10.1016/j.envpol.2018.06.003
Chauvat, M., Perez, G., Hedde, M., and Lamy, I. (2014). Establishment of Bioenergy Crops on Metal Contaminated Soils Stimulates Belowground Fauna. Biomass Bioenerg. 62, 207–211.
Chen, C. L., van der Schoot, H., Dehghan, S., Kamei, A., Schwarz, K. U., et al. (2017). Genetic Diversity of Salt Tolerance in Miscanthus. Front. Plant Sci. 8, 187. doi:10.3389/fpls.2017.00187
Chen, L., Long, C., Wang, D., and Yang, J. (2020). Phytoremediation of Cadmium (Cd) and Uranium (U) Contaminated Soils by Brassica juncea L. Enhanced with Exogenous Application of Plant Growth Regulators. Chemosphere 242, 125112. doi:10.1016/j.chemosphere.2019.125112
Chen, S., and Polle, A. (2010). Salinity Tolerance of Populus. Plant Biol. (Stuttg) 12, 317–333. doi:10.1111/j.1438-8677.2009.00301.x
Cheng, S. F., Huang, C. Y., Lin, Y. C., Lin, S. C., and Chen, K. L. (2015). Phytoremediation of lead Using Corn in Contaminated Agricultural Land-An In Situ Study and Benefit Assessment. Ecotoxicol. Environ. Saf. 111, 72–77. doi:10.1016/j.ecoenv.2014.09.024
Christensen, B. T., Lærke, P. E., Jørgensen, U., Kandel, T. P., and Thomsen, I. K. (2016). Storage of Miscanthus-Derived Carbon in Rhizomes, Roots, and Soil. Can. J. Soil Sci. 96 (4), 354–360. doi:10.1139/cjss-2015-0135
Christou, M., Alexopoulou, E., Cosentino, S. L., Copani, V., Nogues, S., Sanchez, E., et al. (2018). “Giant Reed,” in Perennial Grasses for Bioenergy and Bioproducts. Editors E. Alexopoulou (Cambridge: Academic Press), 107–151. doi:10.1016/b978-0-12-812900-5.00004-7
Chupin, L., Arnoult, S., Brancourt-Hulmel, M., Lapierre, C., Gineau, E., and Navard, P. (2017). Polyethylene Composites Made from Below-Ground Miscanthus Biomass. Ind. Crops Prod. 109, 523–528. doi:10.1016/j.indcrop.2017.09.007
Ciadamidaro, L., Girardclos, O., Bert, V., Zappelini, C., Yung, L., Foulon, J., et al. (2017). Poplar Biomass Production at Phytomanagement Sites is Significantly Enhanced by Mycorrhizal Inoculation. Environ. Exp. Bot. 139, 48–56. doi:10.1016/j.envexpbot.2017.04.004
CL: AIRE (2010). A Framework for Assessing the Sustainability of Soil and Groundwater Remediation (SuRF-UK). Available at www.claire.co.uk/surfuk (Accessed May 30, 2020).
Clavé, G., Garoux, L., Boulanger, C., Hesemann, P., and Grison, C. (2016). Ecological Recycling of a Bio-Based Catalyst for Cu Click Reaction: a New Strategy for a Greener Sustainable Catalysis. ChemistrySelect 1, 1410–1416. doi:10.1002/slct.201600430
Clifton-Brown, J. C., Lewandowski, I., Andersson, B., Basch, G., Christian, D. G., Kjeldsen, J. B., et al. (2001). Performance of 15 Miscanthus Genotypes at Five Sites in Europe. Agron. J. 93, 1013–1019. doi:10.2134/agronj2001.9351013x
Cocozza, C., Vitullo, D., Lima, G., Maiuro, L., Marchetti, M., and Tognetti, R. (2014). Enhancing Phytoextraction of Cd by Combining poplar (Clone "I-214") with Pseudomonas fluorescens and Microbial Consortia. Environ. Sci. Pollut. Res. 21, 1796–1808. doi:10.1007/s11356-013-2073-3
Compant, S., Clément, C., and Sessitsch, A. (2010). Plant Growth-Promoting Bacteria in the Rhizo- and Endosphere of Plants: Their Role, Colonization, Mechanisms Involved and Prospects for Utilization. Soil Biol. Biochem. 42, 669–678. doi:10.1016/j.soilbio.2009.11.024
Courchesne, F., Turmel, M. C., Cloutier-Hurteau, B., Constantineau, S., Munro, L., and Labrecque, M. (2017). Phytoextraction of Soil Trace Elements by Willow during a Phytoremediation Trial in Southern Québec, Canada. Int. J. Phytorem. 19 (6), 545–554. doi:10.1080/15226514.2016.1267700
Crawford, J. T., Shan, C. W., Budsberg, E., Morgan, H., Bura, R., and Gustafson, R. (2016). Hydrocarbon Bio-Jet Fuel from Bioconversion of poplar Biomass: Techno-Economic Assessment. Biotechnol. Biofuels 9 (1), 141. doi:10.1186/s13068-016-0545-7
Crini, G., Lichtfouse, E., Chanet, G., and Morin-Crini, N. (2020). Applications of Hemp in Textiles, Paper Industry, Insulation and Building Materials, Horticulture, Animal Nutrition, Food and Beverages, Nutraceuticals, Cosmetics and hygiene, Medicine, Agrochemistry, Energy Production and Environment: a Review. Environ. Chem. Lett. 18, 1451–1476. doi:10.1007/s10311-020-01029-2
Cristaldi, A., Oliveri Conti, G., Cosentino, S. L., Mauromicale, G., Copat, C., Grasso, A., et al. (2020). Phytoremediation Potential of Arundo donax (Giant Reed) in Contaminated Soil by Heavy Metals. Environ. Res. 185, 109427. doi:10.1016/j.envres.2020.109427
Cundy, A., Bardos, P., Puschenreiter, M., Witters, N., Mench, M., Bert, V., et al. (2015). Developing Effective Decision Support for the Application of “Gentle” Remediation Options: The GREENLAND Project. Remediation 25, 101–114. doi:10.1002/rem.21435
Cundy, A. B., Bardos, R. P., Church, A., Puschenreiter, M., Friesl-Hanl, W., Müller, I., et al. (2013). Developing Principles of Sustainability and Stakeholder Engagement for "gentle" Remediation Approaches: the European Context. J. Environ. Manage. 129, 283–291. doi:10.1016/j.jenvman.2013.07.032
Cundy, A. B., Bardos, R. P., Puschenreiter, M., Mench, M., Bert, V., Friesl-Hanl, W., et al. (2016). Brownfields to green fields: Realising Wider Benefits from Practical Contaminant Phytomanagement Strategies. J. Environ. Manage. 184, 67–77. doi:10.1016/j.jenvman.2016.03.028
Cundy, A. B., LaFreniere, L., Bardos, R. P., Yan, E., Sedivy, R., and Roe, C. (2021). Integrated Phytomanagement of a Carbon Tetrachloride-Contaminated Site in Murdock, Nebraska (USA). J. Clean. Prod. 290, 125190. doi:10.1016/j.jclepro.2020.125190
Dagher, D. J., Pitre, F. E., and Hijri, M. (2020). Ectomycorrhizal Fungal Inoculation of Sphaerosporella brunnea Significantly Increased Stem Biomass of Salix miyabeana and Decreased lead, Tin, and Zinc, Soil Concentrations during the Phytoremediation of an Industrial Landfill. JoF 6 (2), 87. doi:10.3390/jof6020087
Danh, L. T., Truong, P., Mammucari, R., Pu, Y., and Foster, N. R. (2012). Phytoremediation of Soils Contaminated by Heavy Metals, Metalloids, and Radioactive Materials Using Vetiver Grass. Phytotechnologies: Remediation of Environmental Contaminants. New Hamshire, USA: CRC Press, 255–280.
Danh, L. T., Truong, P., Mammucari, R., Tran, T., and Foster, N. (2009). Vetiver Grass,vetiveria Zizanioides: a Choice Plant for Phytoremediation of Heavy Metals and Organic Wastes. Int. J. Phytorem. 11 (8), 664–691. doi:10.1080/15226510902787302
Darajeh, N., Truong, P., Rezania, S., Alizadeh, H., and Leung, D. W. (2019). Effectiveness of Vetiver Grass versus Other Plants for Phytoremediation of Contaminated Water. J. Environ. Treat. Tech. 7, 485–500.
Datta, R., Quispe, M. A., and Sarkar, D. (2011). Greenhouse Study on the Phytoremediation Potential of Vetiver Grass, Chrysopogon zizanioides L., in Arsenic-Contaminated Soils. Bull. Environ. Contam. Toxicol. 86 (1), 124–128. doi:10.1007/s00128-010-0185-8
De Oliveira, V. H., Ullah, I., Dunwell, J. M., and Tibbett, M. (2020). Mycorrhizal Symbiosis Induces Divergent Patterns of Transport and Partitioning of Cd and Zn in. Populus trichocarpa. Environ. Exp. Bot. 171, 103925. doi:10.1016/j.envexpbot.2019.103925
De Vasconcelos, M. C. B. M., Bennett, R., Castro, C., Cardoso, P., Saavedra, M. J., and Rosa, E. A. (2013). Study of Composition, Stabilization and Processing of Wheat Germ and maize Industrial By-Products. Ind. Crops Prod. 42, 292–298. doi:10.1016/j.indcrop.2012.06.007
Debaeke, P., Mestries, E., Desanlis, M., and Seassau, C. (2014). Effects of Crop Management on the Incidence and Severity of Fungal Diseases in Sunflower, in Sunflowers: Growth and Development, Environmental Influences and Pests/Diseases. Editors J.E. Arribas New York, United States: Nova Science Publishers. 201–226.
Deng, Y., Li, D., Huang, Y., and Huang, S. (2017). Physiological Response to Cadmium Stress in Kenaf (Hibiscus cannabinus L.) Seedlings. Ind. Crops Prod. 107, 453–457. doi:10.1016/j.indcrop.2017.06.008
Devappa, R. K., Rakshit, S. K., and Dekker, R. F. H. (2015). Forest Biorefinery: Potential of poplar Phytochemicals as Value-Added Co-products. Biotechnol. Adv. 33 (6), 681–716. doi:10.1016/j.biotechadv.2015.02.012
Dhiman, S. S., Selvaraj, C., Li, J., Singh, R., Zhao, X., Kim, D., et al. (2016). Phytoremediation of Metal-Contaminated Soils by the Hyperaccumulator Canola (Brassica napus L.) and the Use of its Biomass for Ethanol Production. Fuel 183, 107–114. doi:10.1016/j.fuel.2016.06.025
Dickmann, D. I., and Kuzovkina, Y. (2014). “Poplars and willows of the World with Emphasis on Silviculturally Important Species,” in Poplars and willows: Trees for Society and the Environment. Editors J.G. Isebrands, and J. Richardson (Croydon: FAO and CABI), 8–91.
Ding, H., Wang, G., Lou, L., and Lv, J. (2016). Physiological Responses and Tolerance of Kenaf (Hibiscus cannabinus L.) Exposed to Chromium. Ecotoxicol. Environ. Saf. 133, 509–518. doi:10.1016/j.ecoenv.2016.08.007
Dubey, N., Raghav, C. S., Gupta, R. L., and Chhonkar, S. S. (2010). Chemical Composition and Antifungal Activity of Vetiver Oil of North and South India against Rhizoctonia solani. Pest Res. J. 22, 63–67.
Durand, A., Maillard, F., Alvarez-Lopez, V., Guinchard, S., Bertheau, C., Valot, B., et al. (2018). Bacterial Diversity Associated with poplar Trees Grown on a Hg-Contaminated Site: Community Characterization and Isolation of Hg-Resistant Plant Growth-Promoting Bacteria. Sci. Total Environ. 622-623, 1165–1177. doi:10.1016/j.scitotenv.2017.12.069
Eckenwalder, J. E. (1996). “Systematics and Evolution of Populus,” in Biology of Populus and its Implications for Management and Conservation. Editors RF Stettler, HD Bradshaw, PE Heilman, and TM Hinckley (Ottawa, ON, Canada: NRC Research Press, National Research Council of Canada), Chap. 1, 7–32.
Edgar, V. N., Fabián, F. L., Julián Mario, P. C., and Ileana, V. R. (2021). Coupling Plant Biomass Derived from Phytoremediation of Potential Toxic-Metal-Polluted Soils to Bioenergy Production and High-Value By-Products - A Review. Appl. Sci. 11, 2982. doi:10.3390/app11072982
Edrisi, S. A., and Abhilash, P. C. (2016). Exploring Marginal and Degraded Lands for Biomass and Bioenergy Production: An Indian Scenario. Renew. Sustain. Energ. Rev. 54, 1537–1551. doi:10.1016/j.rser.2015.10.050
Ellis, D. E., and Hadley, P. W. (2009). Sustainable Remediation white Paper – Integrating Sustainable Principles, Practices, and Metrics into Remediation Projects. Remediation 19, 5–114. doi:10.1002/rem.20210
Enell, A., Andersson-Sköld, Y., Vestin, J., and Wagelmans, M. (2016). Risk Management and Regeneration of Brownfields Using Bioenergy Crops. J. Soils Sediments 16, 987–1000. doi:10.1007/s11368-015-1264-6
Ens, J., Farrell, R. E., and Bélanger, N. (2013). Effects of Edaphic Conditions on Site Quality for Salix purpurea ‘Hotel' Plantations across a Large Climatic Gradient in Canada. New Forests 44 (6), 899–918. doi:10.1007/s11056-013-9384-6
Epelde, L., Burges, A., Mijangos, I., and Garbisu, C. (2014). Microbial Properties and Attributes of Ecological Relevance for Soil Quality Monitoring during a Chemical Stabilization Field Study. Appl. Soil Ecol. 75, 1–12. doi:10.1016/j.apsoil.2013.10.003
Eschenhagen, A., Raj, M., Rodrigo, N., Zamora, A., Labonne, L., Evon, P., et al. (2019). Investigation of Miscanthus and Sunflower Stalk Fiber-Reinforced Composites for Insulation Applications. Adv. Civ. Eng. 2019, 1–7. doi:10.1155/2019/9328087
European Parliament (2021). European Parliament Resolution of 28 April 2021 on Soil protection (2021/2548(RSP). Available at: https://www.europarl.europa.eu/doceo/document/TA-9-2021-0143_EN.html (Access on May 4, 2021).
Evangelou, M. W. H., Conesa, H. M., Robinson, B. H., and Schulin, R. (2012a). Biomass Production on Trace Element-Contaminated Land: A Review. Environ. Eng. Sci. 29, 823–839. doi:10.1089/ees.2011.0428
Evangelou, M. W. H., Deram, A., Gogos, A., Studer, B., and Schulin, R. (2012b). Assessment of Suitability of Tree Species for the Production of Biomass on Trace Element Contaminated Soils. J. Hazard. Mater. 209-210, 233–239. doi:10.1016/j.jhazmat.2012.01.008
Evangelou, M. W. H., Papazoglou, E. G., Robinson, B. H., and Schulin, R. (2015). “Phytomanagement: Phytoremediation and the Production of Biomass for Economic Revenue on Contaminated Land,” in Phytoremediation. Editors A. Ansari, S. Gill, R. Gill, G. Lanza, and L Newman (Cham: Springer). doi:10.1007/978-3-319-10395-2_9
Falola, O. O., Alasa, M. C., Amuda, A. J., and Babayemi, O. J. (2013). Nutritional and Antinutritional Components of Vetiver Grass (Chrysopogon zizanioides L. Roberty) at Different Stages of Growth. Pakistan J. Nutr. 12 (11), 957–959. doi:10.3923/pjn.2013.957.959
Fässler, E., Robinson, B. H., Gupta, S. K., and Schulin, R. (2010a). Uptake and Allocation of Plant Nutrients and Cd in maize, sunflower and Tobacco Growing on Contaminated Soil and the Effect of Soil Conditioners under Field Conditions. Nutr. Cycl Agroecosyst 87, 339–352. doi:10.1007/s10705-009-9342-z
Fässler, E., Robinson, B. H., Stauffer, W., Gupta, S. K., Papritz, A., and Schulin, R. (2010b). Phytomanagement of Metal-Contaminated Agricultural Land Using sunflower, maize and Tobacco. Agric. Ecosyst. Environ. 136, 49–58. doi:10.1016/j.agee.2009.11.007
Fathi, R. A., Godbold, D. L., Al-Salih, H. S., and Jones, D. (2014). Potential of Phytoremediation to Clean up Uranium-Contaminated Soil with Acacia Species. J. Environ. Earth Sci. 4 (4), 81–91.
Favas, P. J., Pratas, J., Varun, M., D’Souza, R., and Paul, M. S. (2014). Phytoremediation of Soils Contaminated with Metals and Metalloids at Mining Areas: Potential of Native flora. Environ. Risk Assess. Soil Contam. 3, 485–516. doi:10.5772/57469
Ferchaud, F., Vitte, G., Bornet, F., Strullu, L., and Mary, B. (2015). Soil Water Uptake and Root Distribution of Different Perennial and Annual Bioenergy Crops. Plant Soil 388 (1), 307–322. doi:10.1007/s11104-014-2335-y
Firmin, S., Labidi, S., Fontaine, J., Laruelle, F., Tisserant, B., Nsanganwimana, F., et al. (2015). Arbuscular Mycorrhizal Fungal Inoculation Protects Miscanthus×giganteus against Trace Element Toxicity in a Highly Metal-Contaminated Site. Sci. Total Environ. 527-528, 91–99. doi:10.1016/j.scitotenv.2015.04.116
Foulon, J., Zappelini, C., Durand, A., Valot, B., Blaudez, D., and Chalot, M. (2016). Impact of poplar-based Phytomanagement on Soil Properties and Microbial Communities in a Metal-Contaminated Site. FEMS Microbiol. Ecol. 92 (10), fiw163. doi:10.1093/femsec/fiw163
French, C. J., Dickinson, N. M., and Putwain, P. D. (2006). Woody Biomass Phytoremediation of Contaminated brownfield Land. Environ. Pollut. 141 (3), 387–395. doi:10.1016/j.envpol.2005.08.065
Fryzova, R., Pohanka, M., Martinkova, P., Cihlarova, H., Brtnicky, M., Hladky, J., et al. (2017). Oxidative Stress and Heavy Metals in Plants. Rev. Environ. Contam. T. 245, 129–156. doi:10.1007/398_2017_7
Galende, M. A., Kolbas, A., Marchand, L., Oustrière, N., Nsanganwimana, F., Douay, F., and Mench, M. (2014). “Miscanthus giganteus as a Candidate for Phytomanaging Cu-Contaminated Soils,” in Int. Congress Phytoremediation of Polluted Soils, Vigo, Spain, July 29th-30th Vigo, 44.
Gao, Y., Miao, C., Xia, J., Mao, L., Wang, Y., and Zhou, P. (2012). Plant Diversity Reduces the Effect of Multiple Heavy Metal Pollution on Soil Enzyme Activities and Microbial Community Structure. Front. Environ. Sci. Eng. 6 (2), 213–223. doi:10.1007/s11783-011-0345-z
Garbisu, C., Alkorta, I., Kidd, P., Epelde, L., and Mench, M. (2020). Keep and Promote Biodiversity at Polluted Sites under Phytomanagement. Environ. Sci. Pollut. Res. 27 (36), 44820–44834. doi:10.1007/s11356-020-10854-5
García-González, I., Hontoria, C., Gabriel, J. L., Alonso-Ayuso, M., and Quemada, M. (2018). Cover Crops to Mitigate Soil Degradation and Enhance Soil Functionality in Irrigated Land. Geoderma 322, 81–88. doi:10.1016/j.geoderma.2018.02.024
Geiger, E. M., Sarkar, D., and Datta, R. (2019). Evaluation of Copper-Contaminated Marginal Land for the Cultivation of Vetiver Grass (Chrysopogon zizanioides) as a Lignocellulosic Feedstock and its Impact on Downstream Bioethanol Production. Appl. Sci. 9, 2685. doi:10.3390/app9132685
Georgi, R., and Müller, M. (2015). Biotic Risk Factors in Short Rotation Coppice in Germany: Current Situation, New Findings and Future Perspectives. Bioenergy Dendromass Sustain. Dev. Rural Areas, 199–216. doi:10.1002/9783527682973.ch16
Gerhardt, K. E., Gerwing, P. D., and Greenberg, B. M. (2017). Opinion: Taking Phytoremediation from Proven Technology to Accepted Practice. Plant Sci. 256, 170–185.
Ginneken, L. V., Meers, E., Guisson, R., Ruttens, A., Elst, K., Tack, F. M. G., et al. (2007). Phytoremediation for Heavy Metal-contaminated Soils Combined with Bioenergy Production. J. Environ. Eng. Landsc. 15 (4), 227–236. doi:10.3846/16486897.2007.9636935
Glick, B. R. (2010). Using Soil Bacteria to Facilitate Phytoremediation. Biotechnol. Adv. 28, 367–374. doi:10.1016/j.biotechadv.2010.02.001
Glick, B. R. (2012). Plant Growth-Promoting Bacteria: Mechanisms and Applications. Scientifica 2012, 1–15. doi:10.6064/2012/963401
Glick, B. R. (2014). Bacteria with ACC Deaminase Can Promote Plant Growth and Help to Feed the World. Microbiol. Res. 169, 30–39. doi:10.1016/j.micres.2013.09.009
Gnansounou, E., Alves, C. M., and Raman, J. K. (2017). Multiple Applications of Vetiver Grass–A Review. Int. J. Envi. Sci. 2, 125–141.
Gómez, J. A., Campos, M., Guzmán, G., Castillo-Llanque, F., Vanwalleghem, T., Lora, Á., et al. (2018). Soil Erosion Control, Plant Diversity, and Arthropod Communities under Heterogeneous Cover Crops in an Olive Orchard. Environ. Sci. Pollut. Res. 25 (2), 977–989. doi:10.1007/s11356-016-8339-9
Gómez-Sagasti, M. T., Hernández, A., Artetxe, U., Garbisu, C., and Becerril, J. M. (2018). How Valuable Are Organic Amendments as Tools for the Phytomanagement of Degraded Soils? The Knowns, Known Unknowns, and Unknowns. Front. Sustain. Food Syst. 2, 68. doi:10.3389/fsufs.2018.00068
Gong, X., and Tian, D. Q. (2019). Study on the Effect Mechanism of Arbuscular Mycorrhiza on the Absorption of Heavy Metal Elements in Soil by Plants. IOP Conf. Ser. Earth Environ. Sci. 267, 052064–052067. doi:10.1088/1755-1315/267/5/052064
Gonsalvesh, L., Yperman, J., Carleer, R., Mench, M., Herzig, R., and Vangronsveld, J. (2016). Valorisation of Heavy Metals Enriched Tobacco Biomass through Slow Pyrolysis and Steam Activation. J. Chem. Technol. Biotechnol. 91, 1585–1595. doi:10.1002/jctb.4889
González-González, A., and Cuadros, F. (2014). Optimal and Cost-Effective Industrial Biomethanation of Tobacco. Renew. Energ. 63 (0), 280–285. doi:10.1016/j.renene.2013.09.027
Goodspeed, T. H. (1954). The Genus Nicotiana: Origin, Relationships and Evolution of its Species in the Light of Their Distribution, Morphology and Cytogenetics. Waltham, MA: Chronica Botanica.
Goswami, S., and Das, S. (2015). A Study on Cadmium Phytoremediation Potential of Indian Mustard, Brassica juncea. Int. J. Phytorem. 17 (6), 583–588. doi:10.1080/15226514.2014.935289
Greger, M., and Landberg, T. (2015). Novel Field Data on Phytoextraction: Pre-Cultivation WithSalixReduces Cadmium in Wheat Grains. Int. J. Phytorem. 17 (10), 917–924. doi:10.1080/15226514.2014.1003785
Griga, M., and Bjelková, M. (2013). “Flax (Linum usitatissimum L.) and Hemp (Cannabis sativa L.) as Fibre Crops for Phytoextraction of Heavy Metals: Biological, Agro-Technological and Economical point of View,” in Plant-based Remediation Processes. Editor KD Gupta (Berlin/Heidelberg: Springer), 199–237. doi:10.1007/978-3-642-35564-6_11
Grignet, A., de Vaufleury, A., Papin, A., and Bert, V. (2020). Urban Soil Phytomanagement for Zn and Cd In Situ Removal, Greening, and Zn-Rich Biomass Production Taking Care of Snail Exposure. Environ. Sci. Pollut. Res. 27 (3), 3187–3201. doi:10.1007/s11356-019-06796-2
Grisan, S., Polizzotto, R., Raiola, P., Cristiani, S., Ventura, F., Di Lucia, F., et al. (2016). Alternative Use of Tobacco as a Sustainable Crop for Seed Oil, Biofuel, and Biomass. Agron. Sustain. Dev. 36 (4), 55. doi:10.1007/s13593-016-0395-5
Grobelak, A., Kokot, P., Świątek, J., Jaskulak, M., and Rorat, A. (2018). Bacterial ACC Deaminase Activity in Promoting Plant Growth on Areas Contaminated with Heavy Metals. J. Ecol. Eng. 19, 150–157. doi:10.12911/22998993/89818
Grottola, C. M., Giudicianni, P., Pindozzi, S., Stanzione, F., Faugno, S., Fagnano, M., et al. (2019). Steam Assisted Slow Pyrolysis of Contaminated Biomasses: Effect of Plant Parts and Process Temperature on Heavy Metals Fate. Waste Manage. 85, 232–241. doi:10.1016/j.wasman.2018.12.028
Grzegórska, A., Rybarczyk, P., Rogala, A., and Zabrocki, D. (2020). Phytoremediation-From Environment Cleaning to Energy Generation-Current Status and Future Perspectives. Energies 13, 2905. doi:10.3390/en13112905
Guadagnini, M. (2000). In Vitro-Breeding for Metal-Accumulation in Two Tobacco (Nicotiana tabacum) Cultivars. [PhD Dissertation]. Nr. 1288, Switzerland: University of Freiburg.
Guarino, C., Paura, B., and Sciarrillo, R. (2018). Enhancing Phytoextraction of HMs at Real Scale, by Combining Salicaceae Trees with Microbial Consortia. Front. Environ. Sci. 6, 1–11. doi:10.3389/fenvs.2018.00137
Guerra, F., Gainza, F., Perez, R., and Zamudio, F. (2011). “Phytoremediation of Heavy Metals Using Poplars (Populus spp.): A Glimpse of the Plant Responses to Copper, Cadmium and Zinc Stress,” in Handbook of Phytoremediation. Editors I.A. Golubev New York: Nova Science Publishers, 387–413.
Gunarathne, V., Mayakaduwa, S., Ashiq, A., Weerakoon, S. R., Biswas, J. K., and Vithanage, M. (2019). “Transgenic Plants: Benefits, Applications, and Potential Risks in Phytoremediation,” in Transgenic Plant Technology for Remediation of Toxic Metals and Metalloids. Editors M.N.V. Prasad (London: Academic Press), 89–102. doi:10.1016/b978-0-12-814389-6.00005-5
Guo, J., Muhammad, H., Lv, X., Wei, T., Ren, X., Jia, H., et al. (2020). Prospects and Applications of Plant Growth Promoting Rhizobacteria to Mitigate Soil Metal Contamination: A Review. Chemosphere 246, 125823. doi:10.1016/j.chemosphere.2020.125823
Guo, W., Zhao, R., Zhao, W., Fu, R., Guo, J., Bi, N., et al. (2013). Effects of Arbuscular Mycorrhizal Fungi on maize (Zea mays L.) and Sorghum (Sorghum bicolor L. Moench) Grown in Rare Earth Elements of Mine Tailings. Appl. Soil Ecol. 72, 85–92. doi:10.1016/j.apsoil.2013.06.001
Guo, Z., Gao, Y., Cao, X., Jiang, W., Liu, X., Liu, Q., et al. (2019). Phytoremediation of Cd and Pb Interactive Polluted Soils by Switchgrass (Panicum virgatum L.). Int. J. Phytorem. 21 (14), 1486–1496. doi:10.1080/15226514.2019.1644285
Gupta, A. K., Su, S.-W., and Chen, Z.-S. (2011). Heavy-Metal Bioavailability and Chelate Mobilization Efficiency in an Assisted Phytoextraction Process by Sesbania sesban (L.) Merr. Commun. Soil Sci. Plant Anal. 42 (2), 231–245. doi:10.1080/00103624.2011.535073
Gupta, P., Rani, R., Chandra, A., and Kumar, V. (2018). Potential Applications of Pseudomonas Sp. (Strain CPSB21) to Ameliorate Cr6+ Stress and Phytoremediation of Tannery Effluent Contaminated Agricultural Soils. Sci. Rep. 8, 1–10. doi:10.1038/s41598-018-23322-5
Gurajala, H. K., Cao, X., Tang, L., Ramesh, T. M., Lu, M., and Yang, X. (2019). Comparative Assessment of Indian Mustard (Brassica juncea L.) Genotypes for Phytoremediation of Cd and Pb Contaminated Soils. Environ. Pollut. 254, 113085. doi:10.1016/j.envpol.2019.113085
Haller, H., and Jonsson, A. (2020). Growing Food in Polluted Soils: A Review of Risks and Opportunities Associated with Combined Phytoremediation and Food Production (CPFP). Chemosphere 254, 126826. doi:10.1016/j.chemosphere.2020.126826
Harter, A. V., Gardner, K. A., Falush, D., Lentz, D. L., Bye, R. A., and Rieseberg, L. H. (2004). Origin of Extant Domesticated Sunflowers in Eastern North America. Nature 430, 201–205. doi:10.1038/nature02710
Hassan, W., Bano, R., Bashir, F., and David, J. (2014). Comparative Effectiveness of ACC-Deaminase And/or Nitrogen-Fixing Rhizobacteria in Promotion of maize (Zea mays L.) Growth under lead Pollution. Environ. Sci. Pollut. Res. 21, 10983–10996. doi:10.1007/s11356-014-3083-5
Hawkesford, M., Horst, W., Kichey, T., Lambers, H., Schjoerring, J., Møller, I. S., et al. (2012). “Functions of Macronutrients,” in Mineral Nutrition of Higher Plants. Editor P. Marschner (Academic Press), 135–189.doi:10.1016/b978-0-12-384905-2.00006-6
He, J., Strezov, V., Zhou, X., Kumar, R., and Kan, T. (2020). Pyrolysis of Heavy Metal Contaminated Biomass Pre-treated with Ferric Salts: Product Characterisation and Heavy Metal Deportment. Bioresour. Technol. 313 (313), 123641. doi:10.1016/j.biortech.2020.123641
He, Y. M., Yang, R., Lei, G., Li, B., Jiang, M., Yan, K., et al. (2020a). Arbuscular Mycorrhizal Fungi Reduce Cadmium Leaching from Polluted Soils under Simulated Heavy Rainfall. Environ. Pollut. 263, 114406. doi:10.1016/j.envpol.2020.114406
He, Y. M., Fan, X. M., Zhang, G. Q., Li, B., Li, T. G., Zu, Y. Q., et al. (2020b). Effects of Arbuscular Mycorrhizal Fungi and Dark Septate Endophytes on maize Performance and Root Traits under a High Cadmium Stress. South Afr. J. Bot. 134, 415–423. doi:10.1016/j.sajb.2019.09.018
Heaton, E. A., Dohleman, F. G., Miguez, A. F., Juvik, J. A., Lozovaya, V., Widholm, J., et al. (2010). Miscanthus. A Promising Biomass Crop. Adv. Bot. Res. 56, 76–137. doi:10.1016/B978-0-12-381518-7.00003-0
Hedde, M., van Oort, F., Boudon, E., Abonnel, F., and Lamy, I. (2013). Responses of Soil Macroinvertebrate Communities to Miscanthus Cropping in Different Trace Metal Contaminated Soils. Biomass Bioenergy 55, 122–129. doi:10.1016/j.biombioe.2013.01.016
Hego, E., Mench, M., and Bes, C. (2009). “Potential Use of Vetiveria zizanioides for the Phytostabilisation of Cu-Contaminated Soils at a wood Treatment Site,” in COST action 859 Working Groups 1 and 2 and MC Meeting, Szeged, Hungary, 16-17 April, 2009 Szeged, Hungary, 53–54.
Herzig, R., Nehnevajova, E., Pfistner, C., Schwitzguébel, J.-P., Ricci, A., and Keller, C. (2014). Feasibility of Labile Zn Phytoextraction Using Enhanced Tobacco and sunflower: Results of Five- and One-Year Field-Scale Experiments in Switzerland. Int. J. Phytorem. 16, 735–754. doi:10.1080/15226514.2013.856846
Hesami, S. M., Zilouei, H., Karimi, K., and Asadinezhad, A. (2015). Enhanced Biogas Production from sunflower Stalks Using Hydrothermal and Organosolv Pretreatment. Ind. Crops Prod. 76, 449–455. doi:10.1016/j.indcrop.2015.07.018
Hildebrandt, U., Ouziad, F., Marner, F.-J., and Bothe, H. (2006). The bacterium Paenibacillus validus stimulates Growth of the Arbuscular Mycorrhizal fungus Glomus intraradices up to the Formation of fertile Spores. FEMS Microbiol. Lett. 254, 258–267. doi:10.1111/j.1574-6968.2005.00027.x
Ho, W. M., Ang, L. H., and Lee, D. K. (2008). Assessment of Pb Uptake, Translocation and Immobilization in Kenaf (Hibiscus cannabinus L.) for Phytoremediation of Sand Tailings. J. Environ. Sci. 20 (11), 1341–1347. doi:10.1016/s1001-0742(08)62231-7
Hodkinson, T. R., Klaas, M., Jones, M. B., Prickett, R., and Barth, S. (2015). Miscanthus: a Case Study for the Utilization of Natural Genetic Variation. Plant Genet. Resour. 13 (3), 219–237. doi:10.1017/s147926211400094x
Hou, D., Al-Tabbaa, A., and Guthrie, P. (2014). The Adoption of Sustainable Remediation Behaviour in the US and UK: a Cross Country Comparison and Determinant Analysis. Sci. Total Environ. 490, 905–913. doi:10.1016/j.scitotenv.2014.05.059
Hou, D., Ding, Z., Li, G., Wu, L., Hu, P., Guo, G., et al. (2017). A Sustainability Assessment Framework for Agricultural Land Remediation in China. Land Degrad. Dev. 29, 1005–1018. doi:10.1002/ldr.2748
Houben, D., Sonnet, P., and Cornelis, J.-T. (2014). Biochar from Miscanthus: a Potential Silicon Fertilizer. Plant Soil 374 (1), 871–882. doi:10.1007/s11104-013-1885-8
Houben, D., and Sonnet, P. (2015). Impact of Biochar and Root-Induced Changes on Metal Dynamics in the Rhizosphere of Agrostis capillaris and Lupinus albus. Chemosphere 139, 644–651. doi:10.1016/j.chemosphere.2014.12.036
Huysegoms, L., Rousseau, S., and Cappuyns, V. (2019). Indicator Use in Soil Remediation Investments: Views from Policy, Research and Practice. Ecol. Indicators 103, 70–82. doi:10.1016/j.ecolind.2019.03.048
Iqbal, M., Bermond, A., and Lamy, I. (2013). Impact of Miscanthus Cultivation on Trace Metal Availability in Contaminated Agricultural Soils: Complementary Insights from Kinetic Extraction and Physical Fractionation. Chemosphere 91 (3), 287–294. doi:10.1016/j.chemosphere.2012.11.032
ITRC (2011). Green and Sustainable Remediation: State of the Science and Practice. GSR-1. Washington D.C.: Interstate Technology and Regulatory Council (ITRC)Available at: http://www.itrcweb.org/GuidanceDocuments/GSR-1.pdf.
Itusha, A., Osborne, W. J., and Vaithilingam, M. (2019). Enhanced Uptake of Cd by Biofilm Forming Cd Resistant Plant Growth Promoting Bacteria Bioaugmented to the Rhizosphere of Vetiveria zizanioides. Int. J. Phytorem. 21, 487–495. doi:10.1080/15226514.2018.1537245
Jain, D., Kour, R., Bhojiya, A. A., Meena, R. H., Singh, A., Mohanty, S. R., et al. (2020). Zinc Tolerant Plant Growth Promoting Bacteria Alleviates Phytotoxic Effects of Zinc on maize through Zinc Immobilization. Sci. Rep. 10, 1–13. doi:10.1038/s41598-020-70846-w
Janssen, J., Weyens, N., Croes, S., Beckers, B., Meiresonne, L., Van Peteghem, P., et al. (2015). Phytoremediation of Metal Contaminated Soil Using Willow: Exploiting Plant-Associated Bacteria to Improve Biomass Production and Metal Uptake. Int. J. Phytorem. 17, 1123–1136. doi:10.1080/15226514.2015.1045129
Jarausch-Wehrheim, B., Mocquot, B., and Mench, M. (1996). Uptake and Partitioning of Sludge-Borne Copper in Field-Grown maize (Zea mays L.). Eur. J. Agron. 5 (3-4), 259–271. doi:10.1016/s1161-0301(96)02017-5
Jarrah, M., Ghasemi-fasaei, R., Ronaghi, A., Zarei, M., and Mayel, S. (2019). Enhanced Ni Phytoextraction by Effectiveness of Chemical and Biological Amendments in sunflower Plant Grown in Ni-Polluted Soils. Chem. Ecol. 35, 732–745. doi:10.1080/02757540.2019.1644325
Jeannin, T., Yung, L., Evon, P., Labonne, L., Ouagne, P., Lecourt, M., et al. (2020a). Are Nettle Fibers Produced on Metal-Contaminated Lands Suitable for Composite Applications? Mater. Today Proc. 31, S291–S295. doi:10.1016/j.matpr.2020.01.365
Jeannin, T., Yung, L., Evon, P., Labonne, L., Ouagne, P., Lecourt, M., et al. (2020b). Native Stinging Nettle (Urtica dioica L.) Growing Spontaneously under Short Rotation Coppice for Phytomanagement of Trace Element Contaminated Soils: Fibre Yield, Processability and Quality. Ind. Crops Prod. 145, 111997. doi:10.1016/j.indcrop.2019.111997
Jeffries, P., Gianinazzi, S., Perotto, S., Turnau, K., and Barea, J.-M. (2003). The Contribution of Arbuscular Mycorrhizal Fungi in Sustainable Maintenance of Plant Health and Soil Fertility. Biol. Fertil. Soils 37, 1–16. doi:10.1007/s00374-002-0546-5
Jha, A. B., Misra, A. N., and Sharma, P. (2017). “Phytoremediation of Heavy Metal-Contaminated Soil Using Bioenergy Crops,” in Phytoremediation Potential of Bioenergy Plants. Editors K. Bauddh, B. Sing, and J. Korstad (Singapore: Springer), 63–96. doi:10.1007/978-981-10-3084-0_3
Jiang, W., Jacobson, M. G., and Langholtz, M. H. (2019). A Sustainability Framework for Assessing Studies about Marginal Lands for Planting Perennial Energy Crops. Biofuels, Bioprod. Bioref. 13 (1), 228–240. doi:10.1002/bbb.1948
Joimel, S., Cortet, J., Jolivet, C. C., Saby, N. P. A., Chenot, E. D., Branchu, P., et al. (2016). Physico-chemical Characteristics of Topsoil for Contrasted forest, Agricultural, Urban and Industrial Land Uses in France. Sci. Total Environ. 545-546, 40–47. doi:10.1016/j.scitotenv.2015.12.035
Juang, K.-W., Lai, H.-Y., and Chen, B.-C. (2011). Coupling Bioaccumulation and Phytotoxicity to Predict Copper Removal by Switchgrass Grown Hydroponically. Ecotoxicology 20 (4), 827–835. doi:10.1007/s10646-011-0635-z
Jun, L., Wei, H., Aili, M., Juan, N., Hongyan, X., Jingsong, H., et al. (2020). Effect of Lychee Biochar on the Remediation of Heavy Metal-Contaminated Soil Using sunflower: A Field experiment. Environ. Res. 188, 109886. doi:10.1016/j.envres.2020.109886
Kabata-Pendias, A. (2010). Trace Elements in Soils and Plants. 4th ed. Boca Raton: CRC Press. doi:10.1201/b10158
Kacálková, L., Tlustoš, P., and Száková, J. (2014). Chromium, Nickel, Cadmium, and Lead Accumulation in Maize, Sunflower, Willow, and Poplar. Pol. J. Environ. Stud. 23 (3), 753–761.
Kacálková, L., Tlustoš, P., and Száková, J. (2015). Phytoextraction of Risk Elements by Willow and Poplar Trees. Int. J. Phytorem. 17 (5), 414–421. doi:10.1080/15226514.2014.910171
Kafil, M., Boroomand Nasab, S., Moazed, H., and Bhatnagar, A. (2019). Phytoremediation Potential of Vetiver Grass Irrigated with Wastewater for Treatment of Metal Contaminated Soil. Int. J. Phytorem. 21, 92–100. doi:10.1080/15226514.2018.1474443
Kane, N. C., Burke, J. M., Marek, L., Seiler, G., Vear, F., Baute, G., et al. (2013). Sunflower Genetic, Genomic and Ecological Resources. Mol. Ecol. Resour. 13 (1), 10–20. doi:10.1111/1755-0998.12023
Karczewska, A., Gałka, B., Kabała, C., Szopka, K., Kocan, K., and Dziamba, K. (2009). Effects of Various Chelators on the Uptake of Cu, Pb, Zn and Fe by maize and Indian Mustard from Silty Loam Soil Polluted by the Emissions from Copper Smelter. Fresenius Environ. Bull. 18 (10), 1967–1974.
Karimi, N. (2013). Comparative Phytoremediation of Chromium-Contaminated Soils by Alfalfa (Medicago sativa) and Sorghum bicolor (L) Moench. Ijsres 1 (3), 44–49. doi:10.12983/ijsres-2013-p044-049
Karp, A. (2014). “Willows as a Source of Renewable Fuels and Diverse Products,” in Challenges and Opportunities for the World's Forests in the 21st Century. Editors T. Fenning (Dordrecht: Springer), 617–641. doi:10.1007/978-94-007-7076-8_27
Kasanke, C. P., Zhao, Q., Bell, S., Thompson, A. M., and Hofmockel, K. S. (2021). Can Switchgrass Increase Carbon Accrual in Marginal Soils? The Importance of Site Selection. GCB Bioenergy 13 (2), 320–335. doi:10.1111/gcbb.12777
Kaye, J. P., and Quemada, M. (2017). Using Cover Crops to Mitigate and Adapt to Climate Change. A Review. Agron. Sustain. Dev. 37 (1), 4. doi:10.1007/s13593-016-0410-x
Keller, C., Hammer, D., Kayser, A., Richner, W., Brodbeck, M., and Sennhauser, M. (2003). Root Development and Heavy Metal Phytoextraction Efficiency: Comparison of Different Plant Species in the Field. Plant Soil 249, 67–81. doi:10.1023/a:1022590609042
Keshwani, D. R., and Cheng, J. J. (2009). Switchgrass for Bioethanol and Other Value-Added Applications: a Review. Bioresour. Technol. 100 (4), 1515–1523. doi:10.1016/j.biortech.2008.09.035
Khaokaew, S., and Landrot, G. (2015). A Field-Scale Study of Cadmium Phytoremediation in a Contaminated Agricultural Soil at Mae Sot District, Tak Province, Thailand: (1) Determination of Cd-Hyperaccumulating Plants. Chemosphere 138, 883–887. doi:10.1016/j.chemosphere.2014.09.108
Kidd, P., Mench, M., Álvarez-López, V., Bert, V., Dimitriou, I., Friesl-Hanl, W., et al. (2015). Agronomic Practices for Improving Gentle Remediation of Trace Element-Contaminated Soils. Int. J. Phytorem. 17, 1005–1037. doi:10.1080/15226514.2014.1003788
Kidd, P. S., Álvarez, A., Álvarez-López, V., Cerdeira-Pérez, A., Rodríguez-Garrido, B., Prieto-Fernández, Á., et al. (2021). Beneficial Traits of Root Endophytes and Rhizobacteria Associated with Plants Growing in Phytomanaged Soils with Mixed Trace Metal-Polycyclic Aromatic Hydrocarbon Contamination. Chemosphere 277, 130272. doi:10.1016/j.chemosphere.2021.130272
Kocoń, A., and Matyka, M. (2012). Phytoextractive Potential of Miscanthus X giganteus and Sida hermaphrodita Growing under Moderate Pollution of Soil with Zn and Pb. J. Food Agric. Environ. 10, 1253–1256.
Kolbas, A., Herzig, R., Marchand, L., Maalouf, J.-P., Kolbas, N., and Mench, M. (2020). Field Evaluation of One Cu-Resistant Somaclonal Variant and Two Clones of Tobacco for Copper Phytoextraction at a wood Preservation Site. Environ. Sci. Pollut. Res. 27, 27831–27848. doi:10.1007/s11356-020-09151-y
Kolbas, A., Kolbas, N., Marchand, L., Herzig, R., and Mench, M. (2018). Morphological and Functional Responses of a Metal-Tolerant sunflower Mutant Line to a Copper-Contaminated Soil Series. Environ. Sci. Pollut. Res. 25 (17), 16686–16701. doi:10.1007/s11356-018-1837-1
Kolbas, A., Marchand, L., Herzig, R., Nehnevajova, E., and Mench, M. (2014). Phenotypic Seedling Responses of a Metal-Tolerant Mutant Line of sunflower Growing on a Cu-Contaminated Soil Series: Potential Uses for Biomonitoring of Cu Exposure and Phytoremediation. Plant Soil 376 (1), 377–397. doi:10.1007/s11104-013-1974-8
Kolbas, A., Mench, M., Herzig, R., Nehnevajova, E., and Bes, C. M. (2011). Copper Phytoextraction in Tandem with Oilseed Production Using Commercial Cultivars and Mutant Lines of sunflower. Int. J. Phytorem. 13, 55–76. doi:10.1080/15226514.2011.568536
Kolodziej, B., Stachyra, M., Antonkiewicz, J., Bielińska, E., and Wiśniewski, J. (2016). The Effect of Harvest Frequency on Yielding and Quality of Energy Raw Material of Reed Canary Grass Grown on Municipal Sewage Sludge. Biomass Bioenergy 85, 363–370.
Kong, Z., and Glick, B. R. (2017). The Role of Plant Growth-Promoting Bacteria in Metal Phytoremediation. Adv. Microbial Physiol. 71, 97–132. doi:10.1016/bs.ampbs.2017.04.001
Korhonen, J., Honkasalo, A., and Seppälä, J. (2018). Circular Economy: the Concept and its Limitations. Ecol. Econ. 143, 37–46. doi:10.1016/j.ecolecon.2017.06.041
Kötschau, A., Büchel, G., Einax, J. W., von Tümpling, W., and Merten, D. (2014). Sunflower (Helianthus annuus): Phytoextraction Capacity for Heavy Metals on a Mining-Influenced Area in Thuringia, Germany. Environ. Earth Sci. 72 (6), 2023–2031. doi:10.1007/s12665-014-3111-2
Kovalick, W. W., and Montgomery, R. H. (2014). Developing a Program for Contaminated Site Management in Low and Middle Income Countries. Washington, D.C.: World BankAvailable at: https://openknowledge.worldbank.org/handle/10986/18631.
Kovalick, W. W., and Montgomery, R. H. (2017). Models and Lessons for Developing a Contaminated Site Program: an International Review. Environ. Technol. Innovation 7, 77–86. doi:10.1016/j.eti.2016.12.005
Krzyżaniak, M., Stolarski, M. J., and Warmiński, K. (2019). Life Cycle Assessment of poplar Production: Environmental Impact of Different Soil Enrichment Methods. J. Clean. Prod. 206, 785–796. doi:10.1016/j.jclepro.2018.09.180
Kuffner, M., De Maria, S., Puschenreiter, M., Fallmann, K., Wieshammer, G., Gorfer, M., et al. (2010). Culturable Bacteria from Zn- and Cd-accumulatingSalix Capreawith Differential Effects on Plant Growth and Heavy Metal Availability. J. Appl. Microbiol. 108, 1471–1484. doi:10.1111/j.1365-2672.2010.04670.x
Kumar, D., Tripathi, D. K., Liu, S., Singh, V. K., Sharma, S., Dubey, N. K., et al. (2017). Pongamia pinnata (L.) Pierre Tree Seedlings Offer a Model Species for Arsenic Phytoremediation. Plant Gene 11, 238–246. doi:10.1016/j.plgene.2017.06.002
Kumpiene, J., Antelo, J., Brännvall, E., Carabante, I., Ek, K., Komárek, M., et al. (2019). In Situ chemical Stabilization of Trace Element-Contaminated Soil - Field Demonstrations and Barriers to Transition from Laboratory to the Field - A Review. Appl. Geochem. 100, 335–351. doi:10.1016/j.apgeochem.2018.12.003
Kumpiene, J., Giagnoni, L., Marschner, B., Denys, S., Mench, M., Adriaensen, K., et al. (2017). Assessment of Methods for Determining Bioavailability of Trace Elements in Soils: A Review. Pedosphere 27, 389–406. doi:10.1016/S1002-0160(17)60337-0
Kumpiene, J., Lagerkvist, A., and Maurice, C. (2008). Stabilization of As, Cr, Cu, Pb and Zn in Soil Using Amendments - A Review. Waste Manage. 28 (1), 215–225. doi:10.1016/j.wasman.2006.12.012
Kumpiene, J. (2010). “Trace Element Immobilization in Soil Using Amendments,” in Trace Element in Soils. Editors P. Hooda (Chichester, UK: Wiley-Blackwell), 353–379. doi:10.1002/9781444319477.ch15
Kuppens, T., Rafiaani, P., Vanreppelen, K., Yperman, J., Carleer, R., Schreurs, S., et al. (2018). Combining Monte Carlo Simulations and Experimental Design for Incorporating Risk and Uncertainty in Investment Decisions for Cleantech: a Fast Pyrolysis Case Study. Clean. Techn Environ. Pol. 20 (6), 1195–1206. doi:10.1007/s10098-018-1543-1
Labrecque, M., Hu, Y., Vincent, G., and Shang, K. (2020). The Use of Willow Microcuttings for Phytoremediation in a Copper, Zinc and lead Contaminated Field Trial in Shanghai. China. Int. J. Phytorem. 1–7.
Lacalle, R. G., Gómez-Sagasti, M. T., Artetxe, U., Garbisu, C., and Becerril, J. M. (2018). Brassica napus Has a Key Role in the Recovery of the Health of Soils Contaminated with Metals and Diesel by Rhizoremediation. Sci. Total Environ. 618, 347–356. doi:10.1016/j.scitotenv.2017.10.334
Lal, R. K. (2013). On Genetic Diversity in Germplasm of Vetiver ‘Veteveria zizanioides (L.) Nash'. Ind. Crops Prod. 43, 93–98. doi:10.1016/j.indcrop.2012.07.005
Laporte, M.-A., Sterckeman, T., Dauguet, S., Denaix, L., and Nguyen, C. (2015). Variability in Cadmium and Zinc Shoot Concentration in 14 Cultivars of sunflower (Helianthus annuus L.) as Related to Metal Uptake and Partitioning. Environ. Exp. Bot. 109, 45–53. doi:10.1016/j.envexpbot.2014.07.020
Lask, J., Kam, J., Weik, J., Kiesel, A., Wagner, M., and Lewandowski, I. (2021). A Parsimonious Model for Calculating the Greenhouse Gas Emissions of Miscanthus Cultivation Using Current Commercial Practice in the UK. Glob. Change Biol. Bioenergy.
Lavania, U. C. (2000). “Primary and Secondary Centers of Origin of Vetiver and its Dispersion,” in Proceedings of the IInd International Conference on Vetiver: Vetiver and Environment, Cha Am, Phetchaburi Province, Thailand, January, 2000. Editors N. Chomachalow, and M Barang (Bangkok, Thailand: Office of Royal Development Projects Board), 424–427.
Lebrun, M., Nandillon, R., Miard, F., Le Forestier, L., Morabito, D., and Bourgerie, S. (2021). Effects of Biochar, Ochre and Manure Amendments Associated with a Metallicolous Ecotype of Agrostis capillaris on As and Pb Stabilization of a Former Mine Technosol. Environ. Geochem. Health 43, 1–15. doi:10.1007/s10653-020-00592-5
Lettens, S., Vandecasteele, B., De Vos, B., Vansteenkiste, D., and Verschelde, P. (2011). Intra- and Inter-annual Variation of Cd, Zn, Mn and Cu in Foliage of Poplars on Contaminated Soil. Sci. Total Environ. 409 (11), 2306–2316. doi:10.1016/j.scitotenv.2011.02.029
Leudo, A. M., Cruz, Y., Montoya-Ruiz, C., Delgado, M. D. P., and Saldarriaga, J. F. (2020). Mercury Phytoremediation with Lolium perenne-Mycorrhizae in Contaminated Soils. Sustainability 12 (9), 3795. doi:10.3390/su12093795
Lewandowski, I., Clifton-Brown, J., Kiesel, A., Hastings, A., and Iqbal, Y. (2018). “Miscanthus,” in Perennial Grasses for Bioenergy and Bioproducts. Editors E. Alexopoulou (London: Academic Press), 35–59. doi:10.1016/b978-0-12-812900-5.00002-3
Lewandowski, I., Clifton-Brown, J., Trindade, L. M., van der Linden, G. C., Schwarz, K. U., Müller-Sämann, K., et al. (2016). Progress on Optimizing Miscanthus Biomass Production for the European Bioeconomy: Results of the EU FP7 Project OPTIMISC. Front. Plant Sci. 7, 1620. doi:10.3389/fpls.2016.01620
Lewandowski, I., Scurlock, J. M. O., Lindvall, E., and Christou, M. (2003). The Development and Current Status of Perennial Rhizomatous Grasses as Energy Crops in the US and Europe. Biomass Bioenergy 25, 335–361. doi:10.1016/s0961-9534(03)00030-8
Li, C., Wang, Q. H., Xiao, B., and Li, Y. F. (2011a). “Phytoremediation Potential of Switchgrass (Panicum virgatum L.) for Cr-Polluted Soil,” in Proceedings of International Symposium on Water Resource and Environmental Protection (ISWREP), Vol. 3, 1731–1734. doi:10.1109/ISWREP.2011.5893582
Li, F.-L., Qiu, Y., Xu, X., Yang, F., Wang, Z., Feng, J., et al. (2020). EDTA-enhanced Phytoremediation of Heavy Metals from Sludge Soil by Italian Ryegrass (Lolium perenne L.). Ecotoxicol. Environ. Saf. 191, 110185. doi:10.1016/j.ecoenv.2020.110185
Li, G.-Y., Hu, N., Ding, D.-x., Zheng, J.-f., Liu, Y.-l., Wang, Y.-d., et al. (2011b). Screening of Plant Species for Phytoremediation of Uranium, Thorium, Barium, Nickel, Strontium and Lead Contaminated Soils from a Uranium Mill Tailings Repository in South China. Bull. Environ. Contam. Toxicol. 86, 646–652. doi:10.1007/s00128-011-0291-2
Li, N. Y., Li, Z. A., Zhuang, P., Zou, B., and McBride, M. (2009). Cadmium Uptake from Soil by Maize with Intercrops. Water Air Soil Pollut. 199, 45–56. doi:10.1007/s11270-008-9858-x
Li, X., Cundy, A. B., and Chen, W. (2018). Fuzzy Synthetic Evaluation of Contaminated Site Management Policy from the Perspective of Stakeholders: a Case Study from China. J. Clean. Prod. 198, 1593–1601. doi:10.1016/j.jclepro.2018.07.036
Licht, L. A., and Isebrands, J. G. (2005). Linking Phytoremediated Pollutant Removal to Biomass Economic Opportunities. Biomass Bioenergy 28, 203–218. doi:10.1016/j.biombioe.2004.08.015
Lim, G. H., Chae, J. S., Cha, Y. L., Kang, Y. C., and Roh, K. C. (2019). Giant-miscanthus-derived Activated Carbon and its Application to Lithium Sulfur Batteries. Carbon Lett. 30, 477–484. doi:10.1007/s42823-019-00117-w
Lira, C. D., Barry, T. N., Pomroy, W. E., McWilliam, E. L., and Lopez-Villalobos, N. (2008). Willow (Salix spp.) Fodder Blocks for Growth and Sustainable Management of Internal Parasites in Grazing Lambs. Anim. Feed Sci. Tech. 141 (1-2), 61–81. doi:10.1016/j.anifeedsci.2007.05.030
Littlewood, J., Guo, M., Boerjan, W., and Murphy, R. J. (2014). Bioethanol from poplar: a Commercially Viable Alternative to Fossil Fuel in the European Union. Biotechnol. Biofuels 7 (1), 1–12. doi:10.1186/1754-6834-7-113
Lomonte, C., Wang, Y., Doronila, A., Gregory, D., Baker, A. J. M., Siegele, R., et al. (2014). Study of the Spatial Distribution of Mercury in Roots of Vetiver Grass (Chrysopogon zizanioides) by Micro-PIXE Spectrometry. Int. J. Phytorem. 16 (11), 1170–1182. doi:10.1080/15226514.2013.821453
Lord, R. (2015). Reed Canarygrass (Phalaris arundinacea), Outperforms Miscanthus or Willow on Marginal Soils, brownfield and Non-agricultural Sites for Local, Sustainable Energy Crop Production. Biomass Bioenergy 78, 110–125. doi:10.1016/j.biombioe.2015.04.015
Lou, Y., Zhao, P., Wang, D., Amombo, E., Sun, X., Wang, H., et al. (2017). Germination, Physiological Responses and Gene Expression of Tall Fescue (Festuca arundinacea Schreb.) Growing under Pb and Cd. PLoS One 12 (1), e0169495. doi:10.1371/journal.pone.0169495
Lyubenova, L., Nehnevajova, E., Herzig, R., and Schröder, P. (2009). Response of Antioxidant Enzymes in Nicotiana tabacum Clones during Phytoextraction of Heavy Metals. Environ. Sci. Pollut. Res. 16 (5), 573–581. doi:10.1007/s11356-009-0175-8
Ma, Y., Prasad, M. N. V., Rajkumar, M., and Freitas, H. (2011a). Plant Growth Promoting Rhizobacteria and Endophytes Accelerate Phytoremediation of Metalliferous Soils. Biotechnol. Adv. 29, 248–258. doi:10.1016/j.biotechadv.2010.12.001
Ma, Y., Rajkumar, M., Vicente, J. A. F., and Freitas, H. (2011b). Inoculation of Ni-Resistant Plant Growth Promoting Bacterium Psychrobacter sp. Strain SRS8 for the Improvement of Nickel Phytoextraction by Energy Crops. Int. J. Phytorem. 13, 126–139. doi:10.1080/15226511003671403
Malusà, E., Pinzari, F., and Canfora, L. (2016). “Efficacy of Biofertilizers: Challenges to Improve Crop Production,” in Microbial Inoculants in Sustainable Agricultural Productivity. Editors D. P. Singh, H. B. Singh, and R. Prabha (India: Springer), 17–40. doi:10.1007/978-81-322-2644-410.1007/978-81-322-2644-4_2
Mani, D., and Kumar, C. (2014). Biotechnological Advances in Bioremediation of Heavy Metals Contaminated Ecosystems: an Overview with Special Reference to Phytoremediation. Int. J. Environ. Sci. Technol. 11 (3), 843–872. doi:10.1007/s13762-013-0299-8
Markell, S. G., Harveson, R. M., Block, C. C., and Gulya, T. J. (2015). “Sunflower Diseases,” in Sunflower: Chemistry, Production, Processing and Utilization. Editors E. Martínez-Force, N.T. Dunford, and J.J. Salas (Urbana, IL: AOCS Press), 93–128. doi:10.1016/b978-1-893997-94-3.50010-6
Marques, A. P. G. C., Moreira, H., Franco, A. R., Rangel, A. O. S. S., and Castro, P. M. L. (2013). Inoculating Helianthus annuus (sunflower) Grown in Zinc and Cadmium Contaminated Soils with Plant Growth Promoting Bacteria - Effects on Phytoremediation Strategies. Chemosphere 92 (1), 74–83. doi:10.1016/j.chemosphere.2013.02.055
Marques, M. C., and do Nascimento, C. W. A. (2013). Analysis of Chlorophyll Fluorescence Spectra for the Monitoring of Cd Toxicity in a Bio-Energy Crop (Jatropha curcas). J. Photochem. Photobiol. B: Biol. 127, 88–93. doi:10.1016/j.jphotobiol.2013.07.016
Marschner, P., and Timonen, S. (2005). Interactions between Plant Species and Mycorrhizal Colonization on the Bacterial Community Composition in the Rhizosphere. Appl. Soil Ecol. 28, 23–36. doi:10.1016/j.apsoil.2004.06.007
Mathias, J. D., Alzina, A., Grédiac, M., Michaud, P., Roux, P., Baynast, De., et al. (2015). Upcycling sunflower Stems as Natural Fibers for Biocomposite Applications. BioRes. 10 (4), 8076–8088. doi:10.15376/biores.10.4.8076-8088
McCalmont, J. P., Hastings, A., McNamara, N. P., Richter, G. M., Robson, P., Donnison, I. S., et al. (2017). Environmental Costs and Benefits of growing Miscanthus for Bioenergy in the UK. Gcb Bioenergy 9 (3), 489–507. doi:10.1111/gcbb.12294
Meers, E., Ruttens, A., Hopgood, M., Lesage, E., and Tack, F. M. G. (2005). Potential of Brassica rapa, Cannabis sativa, Helianthus annuus and Zea mays for Phytoextraction of Heavy Metals from Calcareous Dredged Sediment Derived Soils. Chemosphere 61, 561–572. doi:10.1016/j.chemosphere.2005.02.026
Meers, E., Van Slycken, S., Adriaensen, K., Ruttens, A., Vangronsveld, J., Du Laing, G., et al. (2010). The Use of Bio-Energy Crops (Zea mays) for ‘phytoattenuation' of Heavy Metals on Moderately Contaminated Soils: A Field experiment. Chemosphere 78, 35–41. doi:10.1016/j.chemosphere.2009.08.015
Mellor, P., Lord, R. A., João, E., Thomas, R., and Hursthouse, A. (2021). Identifying Non-agricultural Marginal Lands as a Route to Sustainable Bioenergy Provision - A Review and Holistic Definition. Renew. Sustain. Energ. Rev. 135, 110220. doi:10.1016/j.rser.2020.110220
Mench, M. J., Dellise, M., Bes, C. M., Marchand, L., Kolbas, A., Le Coustumer, Ph., et al. (2018). Phytomanagement and Remediation of Cu-Contaminated Soils by High Yielding Crops at a Former wood Preservation Site: sunflower Biomass and Ionome. Front. Ecol. Evol. 6, 123. doi:10.3389/fevo.2018.00123
Mench, M., Lepp, N., Bert, V., Schwitzguébel, J.-P., Gawronski, S. W., Schröder, P., et al. (2010). Successes and Limitations of Phytotechnologies at Field Scale: Outcomes, Assessment and Outlook from COST Action 859. J. Soils Sediments 10, 1039–1070. doi:10.1007/s11368-010-0190-x (Accessed on 15th September, 2020).
Mench, M., Vangronsveld, J., Lepp, N., Ruttens, A., Bleeker, P., and Geebelen, W. (2007). “Use of Soil Amendments to Attenuate Trace Element Exposure: Sustainability, Side Effects, and Failures,” in Natural Attenuation of Trace Element Availability in Soils. Editors R. E. Hamon, M. McLaughlin, and E. Lombi (Pensacola, FL: SETAC Press), 197–228.
Mench, M., Vilela, J., Pereira, S., Moreira, H., Castro, P., Ávila, P., et al. (2020). Guide of Best Practices for Phytomanaging Metal(loid)-Contaminated Soils: GT1 Characterization and Risk Assessment of Contaminated/Degraded Sites and Implementation of Suitable Phytomanagement Options. EU PhytoSUDOE Project (SOE1/P5/E0189), Deliverable E1.2.1. 66 P. Available at http://www.phytosudoe.eu/wp-content/uploads/2020/05/E_1_2_guide_best_practices_GT1_final.pdf (Accessed September 15, 2020).
Mendoza-Hernández, J., Perea-Vélez, Y. S., Arriola-Morales, J., Martínez-Simón, S. M., and Pérez-Osorio, G. (2016). Assessing the Effects of Heavy Metals in ACC Deaminase and IAA Production on Plant Growth-Promoting Bacteria. Microbiol. Res. 188–189, 53–61. doi:10.1016/j.micres.2016.05.001
Meng, F., Jin, D., Guo, K., Larson, S. L., Ballard, J. H., Chen, L., et al. (2018). Influences of U Sources and Forms on its Bioaccumulation in Indian Mustard and Sunflower. Water Air Soil Poll. 229 (11369), 2018. doi:10.1007/s11270-018-4023-7
Merlos, M. A., Zitka, O., Vojtech, A., Azcón-Aguilar, C., and Ferrol, N. (2016). The Arbuscular Mycorrhizal Fungus Rhizophagus irregularis Differentially Regulates the Copper Response of Two maize Cultivars Differing in Copper Tolerance. Plant Sci. 253, 68–76. doi:10.1016/j.plantsci.2016.09.010
Meyer, E., Londoño, D. M. M., de Armas, R. D., Rossi, M. J., Stoffel, S. C. G., et al. (2017). Arbuscular Mycorrhizal Fungi in the Growth and Extraction of Trace Elements by Chrysopogon zizanioides (Vetiver) in a Substrate Containing Coal Mine Wastes. Int. J. Phytorem. 19, 113–120. doi:10.1080/15226514.2016.1207596
Migeon, A., Richaud, P., Guinet, F., Chalot, M., and Blaudez, D. (2009). Metal Accumulation by Woody Species on Contaminated Sites in the north of France. Water Air Soil Poll. 204 (1-4), 89. doi:10.1007/s11270-009-0029-5
Miransari, M. (2010). Contribution of Arbuscular Mycorrhizal Symbiosis to Plant Growth under Different Types of Soil Stress. Plant Biol. 12, 563–569. doi:10.1111/j.1438-8677.2009.00308.x
Mirck, J., and Volk, T. A. (2010). Response of Three Shrub Willow Varieties (Salix spp.) to Storm Water Treatments with Different Concentrations of Salts. Bioresour. Technol. 101, 3484–3492. doi:10.1016/j.biortech.2009.12.128
Mleczek, M., Rutkowski, P., Rissmann, I., Kaczmarek, Z., Golinski, P., Szentner, K., et al. (2010). Biomass Productivity and Phytoremediation Potential of Salix alba and Salix viminalis. Biomass Bioenergy 34 (9), 1410–1418. doi:10.1016/j.biombioe.2010.04.012
Mohankumara, P. B., Thakare, S. P., Guna, V., and Arpitha, G. R. (2020). Millettia pinnata: a Study on the Extraction of Fibers and Reinforced Composites. Bioresour. Bioproc. 7 (1), 1–6. doi:10.1186/s40643-019-0292-2
Mokarram-Kashtiban, S., Hosseini, S. M., Tabari Kouchaksaraei, M., and Younesi, H. (2019). The Impact of Nanoparticles Zero-Valent Iron (nZVI) and Rhizosphere Microorganisms on the Phytoremediation Ability of white Willow and its Response. Environ. Sci. Pollut. Res. 26, 10776–10789. doi:10.1007/s11356-019-04411-y
Montalbán, B., Thijs, S., Lobo, M. C., Weyens, N., Ameloot, M., Vangronsveld, J., et al. (2017). Cultivar and Metal-specific Effects of Endophytic Bacteria in Helianthus tuberosus Exposed to Cd and Zn. Int. J. Mol. Sci. 18, 2026. doi:10.3390/ijms18102026
Moreira, H., Marques, A. P. G. C., Franco, A. R., Rangel, A. O. S. S., and Castro, P. M. L. (2014). Phytomanagement of Cd-Contaminated Soils Using maize (Zea mays L.) Assisted by Plant Growth-Promoting Rhizobacteria. Environ. Sci. Pollut. Res. 21, 9742–9753. doi:10.1007/s11356-014-2848-1
Moreira, H., Pereira, S. I. A., Marques, A. P. G. C., Rangel, A. O. S. S., and Castro, P. M. L. (2019). Effects of Soil Sterilization and Metal Spiking in Plant Growth Promoting Rhizobacteria Selection for Phytotechnology Purposes. Geoderma 334, 72–81. doi:10.1016/j.geoderma.2018.07.025
Moreira, H., Pereira, S. I. A., Marques, A. P. G. C., Rangel, A. O. S. S., and Castro, P. M. L. (2016a). Mine Land Valorization through Energy maize Production Enhanced by the Application of Plant Growth-Promoting Rhizobacteria and Arbuscular Mycorrhizal Fungi. Environ. Sci. Pollut. Res. 23, 6940–6950. doi:10.1007/s11356-015-5914-4
Moreira, H., Pereira, S. I. A., Marques, A. P. G. C., Rangel, A. O. S. S., and Castro, P. M. L. (2016b). Selection of Metal Resistant Plant Growth Promoting Rhizobacteria for the Growth and Metal Accumulation of Energy maize in a Mine Soil - Effect of the Inoculum Size. Geoderma 278, 1–11. doi:10.1016/j.geoderma.2016.05.003
Moreira, H., Pereira, S. I. A., Mench, M., Cardoso, E., Garbisu, C., Kidd, P., et al. (2021). Technical Guide on Strategies to Enhance Phytomanagement Efficiency at Metal(loid)-Polluted/degraded Sites: Planting Patterns, Bioinoculation and Soil Organic Amendments. Available at: http://old.phytosudoe.eu/wp-content/uploads/2020/07/Technical-Guide-GT3_Draft_baja2.pdf (Accessed January 23, 2021).
MTES (Ministère de la transition écologique et solidaire) (2018). French National methodology for managing contaminated land. Direction générale de la Prévention des Risques, Bureau du Sol et du Sous-Sol. Available at: http://ssp-infoterre.brgm.fr/sites/default/files/upload/documents/leaflet_french_methodology_2018_12_19.pdf and full documents at http://ssp-infoterre.brgm.fr/methodologie-nationale-gestion-sites-sols-pollues (Access on June 21th, 2020).
Nadeem, S. M., Ahmad, M., Zahir, Z. A., Javaid, A., and Ashraf, M. (2014). The Role of Mycorrhizae and Plant Growth Promoting Rhizobacteria (PGPR) in Improving Crop Productivity under Stressful Environments. Biotechnol. Adv. 32, 429–448. doi:10.1016/j.biotechadv.2013.12.005
Nehnevajova, E., Herzig, R., Federer, G., Erismann, K.-H., and Schwitzguébel, J.-P. (2005). Screening of sunflower Cultivars for Metal Phytoextraction in a Contaminated Field Prior to Mutagenesis. Int. J. Phytorem. 7, 337–349. doi:10.1080/16226510500327210
Nehnevajova, E., Lyubenova, L., Herzig, R., Schröder, P., Schwitzguébel, J.-P., and Schmülling, T. (2012). Metal Accumulation and Response of Antioxidant Enzymes in Seedlings and Adult sunflower Mutants with Improved Metal Removal Traits on a Metal-Contaminated Soil. Environ. Exp. Bot. 76, 39–48. doi:10.1016/j.envexpbot.2011.10.005
Nigussie, Z., and Alemayehu, G. (2013). Sesbania sesban (L.) Merrill: Potential Uses of an Underutilized Multipurpose Tree in Ethiopia. Afr. J. Plant Sci. 7 (10), 468–475. doi:10.5897/ajps2012.0716
Nissim, W. G., Palm, E., Mancuso, S., and Azzarello, E. (2019). Trace Element Partitioning in a poplar Phytoextraction Stand in Relation to Stem Size. J. Environ. Manage. 247, 688–697. doi:10.1016/j.jenvman.2019.06.105
Norman, J. B., and McManus, M. (2019). The Techno-Economic Viability of Bio-Synthetic Natural Gas Production Utilising Willow Grown on Contaminated Land. AIMS Energy 7 (3), 285–312. doi:10.3934/energy.2019.3.285
Nsanganwimana, F., Marchand, L., Douay, F., and Mench, M. (2013). Arundo donax L., a Candidate for Phytomanaging Water and Soils Contaminated by Trace Elements and Producing Plant-Based Feedstock. Int. J. Phytorem. 16, 982–1017. doi:10.1080/15226514.2013.810580
Nsanganwimana, F., Pourrut, B., Mench, M., and Douay, F. (2014). Suitability of Miscanthus Species for Managing Inorganic and Organic Contaminated Land and Restoring Ecosystem Services. A Review. J. Environ. Manage. 143, 123–134. doi:10.1016/j.jenvman.2014.04.027
Nsanganwimana, F., Pourrut, B., Waterlot, C., Louvel, B., Bidar, G., Labidi, S., et al. (2015). Metal Accumulation and Shoot Yield of Miscanthus×giganteus Growing in Contaminated Agricultural Soils: Insights into Agronomic Practices. Agric. Ecosyst. Environ. 213, 61–71. doi:10.1016/j.agee.2015.07.023
Nsanganwimana, F., Waterlot, C., Louvel, B., Pourrut, B., and Douay, F. (2016). Metal, Nutrient and Biomass Accumulation during the Growing Cycle of Miscanthus established on Metal-Contaminated Soils. J. Plant Nutr. Soil Sci. 179 (2), 257–269. doi:10.1002/jpln.201500163
O'Connor, D., Zheng, X., Hou, D., Shen, Z., Li, G., Miao, G., et al. (2019). Phytoremediation: Climate Change Resilience and Sustainability Assessment at a Coastal brownfield Redevelopment. Environ. Int. 130, 104945. doi:10.1016/j.envint.2019.104945
Olivares, A. R., Carrillo-González, R., González-Chávez, M. D. C. A., and Hernández, R. M. S. (2013). Potential of castor Bean (Ricinus communis L.) for Phytoremediation of Mine Tailings and Oil Production. J. Environ. Manage. 114, 316–323.
Oliveira, R. S., Castro, P. M. L., Dodd, J. C., and Vosátka, M. (2005a). Synergistic Effect of Glomus intraradices and Frankia spp. On the Growth and Stress Recovery of Alnus glutinosa in an Alkaline Anthropogenic Sediment. Chemosphere 60, 1462–1470. doi:10.1016/j.chemosphere.2005.01.038
Oliveira, R. S., Vosátka, M., Dodd, J. C., and Castro, P. M. L. (2005b). Studies on the Diversity of Arbuscular Mycorrhizal Fungi and the Efficacy of Two Native Isolates in a Highly Alkaline Anthropogenic Sediment. Mycorrhiza 16, 23–31. doi:10.1007/s00572-005-0010-0
Olsson, L., Barbosa, H., Bhadwal, S., Cowie, A., Delusca, K., Flores-Renteria, D., et al. (2019). “Land Degradation,” in Climate Change and Land: An IPCC Special Report on Climate Change, Desertification, Land Degradation, Sustainable Land Management, Food Security, and Greenhouse Gas Fluxes in Terrestrial Ecosystems. Editor P.R. Shukla, J. Skea, E. Calvo Buendia, V. Masson-Delmotte, H.-O. Pörtner, D. C. Robertset al. (Geneva, Switzerland: IPCC)
Ondo Zue Abaga, N., Dousset, S., Mbengue, S., and Munier-Lamy, C. (2014). Is Vetiver Grass of Interest for the Remediation of Cu and Cd to Protect Marketing Gardens in Burkina Faso? Chemosphere 113, 42–47. doi:10.1016/j.chemosphere.2014.04.010
Ortiz, N., Armada, E., Duque, E., Roldán, A., and Azcón, R. (2015). Contribution of Arbuscular Mycorrhizal Fungi And/or Bacteria to Enhancing Plant Drought Tolerance under Natural Soil Conditions: Effectiveness of Autochthonous or Allochthonous Strains. J. Plant Physiol. 174, 87–96. doi:10.1016/j.jplph.2014.08.019
Padoan, E., Passarella, I., Prati, M., Bergante, S., Facciotto, G., and Ajmone-Marsan, F. (2020). The Suitability of Short Rotation Coppice Crops for Phytoremediation of Urban Soils. Appl. Sci. 10 (1), 307. doi:10.3390/app10010307
Paleari, S. (2017). Is the European Union Protecting Soil? A Critical Analysis of Community Environmental Policy and Law. Land Use Policy 64, 163–173. doi:10.1016/j.landusepol.2017.02.007
Pandey, V. C., Bajpai, O., Pandey, D. N., and Singh, N. (2015). Saccharum spontaneum: an Underutilized Tall Grass for Revegetation and Restoration Programs. Genet. Resour. Crop Evol. 62 (3), 443–450. doi:10.1007/s10722-014-0208-0
Pandey, V. C., Bajpai, O., and Singh, N. (2016). Energy Crops in Sustainable Phytoremediation. Renew. Sustain. Energ. Rev. 54, 58–73. doi:10.1016/j.rser.2015.09.078
Park, H.-J., Oh, S.-W., and Wen, M.-Y. (2012). Manufacture and Properties of Miscanthus-wood Particle Composite boards. J. Wood Sci. 58, 459–464. doi:10.1007/s10086-012-1262-x
Patra, D. K., Pradhan, C., Kumar, J., and Patra, H. K. (2020). Assessment of Chromium Phytotoxicity, Phytoremediation and Tolerance Potential of Sesbania sesban and Brachiaria mutica Grown on Chromite Mine Overburden Dumps and Garden Soil. Chemosphere 252, 126553. doi:10.1016/j.chemosphere.2020.126553
Paustian, K., Larson, E., Kent, J., Marx, E., and Swan, A. (2019). Soil C Sequestration as a Biological Negative Emission Strategy. Front. Clim. 1, 8. doi:10.3389/fclim.2019.00008
Pavel, P.-B., Puschenreiter, M., Wenzel, W. W., Diacu, E., and Barbu, C. H. (2014). Aided Phytostabilization Using Miscanthus sinensis×giganteus on Heavy Metal-Contaminated Soils. Sci. Total Environ. 479-480, 125–131. doi:10.1016/j.scitotenv.2014.01.097
Pedroso, G. M., De Ben, C., Hutmacher, R. B., Orloff, S., Putnam, D., Six, J., et al. (2011). Switchgrass Is a Promising, High-Yielding Crop for California Biofuel. Cal Ag 65 (3), 168–173. doi:10.3733/ca.e.v065n03p168
Pereira, S. I. A., and Castro, P. M. L. (2014). Phosphate-solubilizing Rhizobacteria Enhance Zea mays Growth in Agricultural P-Deficient Soils. Ecol. Eng. 73, 526–535. doi:10.1016/j.ecoleng.2014.09.060
Pereira, S. I. A., Moreira, H., Argyras, K., Castro, P. M. L., and Marques, A. P. G. C. (2016). Promotion of sunflower Growth under saline Water Irrigation by the Inoculation of Beneficial Microorganisms. Appl. Soil Ecol. 105, 36–47. doi:10.1016/j.apsoil.2016.03.015
Pereira, S. I. A., Pires, C., Henriques, I., Correia, A., Magan, N., and Castro, P. M. L. (2015). Assessment of Rhizospheric Culturable Bacteria of Phragmites australis and Juncus Effusus from Polluted Sites. J. Basic Microbiol. 55, 1179–1190. doi:10.1002/jobm.201500010
Pérez, A. P., and Sánchez, S. P. (2017). European Achievements in Soil Remediation and brownfield Redevelopment. Brussels: JRC. doi:10.2760/818120
Petruzzelli, G., Pedron, F., Pedron, F., and Rosellini, I. (2020). Bioavailability and Bioaccessibility in Soil: a Short Review and a Case Study. Aims Environ. Sci. 7, 208–225. doi:10.3934/environsci.2020013
Pérez, A. P., and Eugenio, N. R. (2018). Status of Local Soil Contamination in Europe: Revision of the Indicator “Progress in the Management Contaminated Sites in Europe. EUR 29124 EN. Luxembourg: Publications Office of the European Union. doi:10.2760/093804
Phanthavongsa, P., Chalot, M., Papin, A., Lacercat-Didier, L., Roy, S., Blaudez, D., et al. (2017). Effect of Mycorrhizal Inoculation on Metal Accumulation by poplar Leaves at Phytomanaged Sites. Environ. Exp. Bot. 143, 72–81. doi:10.1016/j.envexpbot.2017.08.012
Phieler, R., Merten, D., Roth, M., Büchel, G., and Kothe, E. (2015). Phytoremediation Using Microbially Mediated Metal Accumulation in Sorghum Bicolor. Environ. Sci. Pollut. Res. 22 (24), 19408–19416. doi:10.1007/s11356-015-4471-1
Phusantisampan, T., Meeinkuirt, W., Saengwilai, P., Pichtel, J., and Chaiyarat, R. (2016). Phytostabilization Potential of Two Ecotypes of Vetiveria zizanioides in Cadmium-Contaminated Soils: Greenhouse and Field Experiments. Environ. Sci. Pollut. Res. 23 (19), 20027–20038. doi:10.1007/s11356-016-7229-5
Pidatala, V. R., Li, K., Sarkar, D., Ramakrishna, W., and Datta, R. (2016). Identification of Biochemical Pathways Associated with lead Tolerance and Detoxification in Chrysopogon zizanioides L. Nash (Vetiver) by Metabolic Profiling. Environ. Sci. Technol. 50 (5), 2530–2537. doi:10.1021/acs.est.5b04725
Pidlisnyuk, V., Stefanovska, T., Lewis, E. E., Erickson, L. E., and Davis, L. C. (2014). Miscanthus as a Productive Biofuel Crop for Phytoremediation. Crit. Rev. Plant Sci. 33 (1), 1–19. doi:10.1080/07352689.2014.847616
Pidlisnyuk, V., Trögl, J., Stefanovska, T., Shapoval, P., and Erickson, L. (2016). Preliminary Results on Growing Second Generation Biofuel Crop Miscanthus x giganteus at the Polluted Military Site in Ukraine. Nova Biotechnol. Chim. 15 (1), 77–84. doi:10.1515/nbec-2016-0008
Piracha, M. A., Ashraf, M., and Niaz, A. (2019). Arsenic Fractionation and its Impact on Physiological Behavior of sunflower (Helianthus annuus L.) in Three Texturally Different Soils under Alkaline Calcareous Conditions. Environ. Sci. Pollut. Res. 26 (17), 17438–17449. doi:10.1007/s11356-019-05141-x
Pires, C., Franco, A. R., Pereira, S. I. A., Henriques, I., Correia, A., Magan, N., et al. (2017). Metal(loid)-Contaminated Soils as a Source of Culturable Heterotrophic Aerobic Bacteria for Remediation Applications. Geomicrobiol. J. 34, 760–768. doi:10.1080/01490451.2016.1261968
Pirozzi, D., Fiorentino, N., Impagliazzo, A., Sannino, F., Yousuf, A., Zuccaro, G., et al. (2015). Lipid Production from Arundo donax Grown under Different Agronomical Conditions. Renew. Energ. 77, 456–462. doi:10.1016/j.renene.2014.12.046
Pogrzeba, M., Krzyżak, J., Rusinowski, S., McCalmont, J. P., and Jensen, E. (2019). “Energy Crop at Heavy Metal-Contaminated Arable Land as an Alternative for Food and Feed Production: Biomass Quantity and Quality,” in Plant Metallomics and Functional Omics. Editors S. Gaurav (Cham: Springer), 1–21. doi:10.1007/978-3-030-19103-0_1
Pogrzeba, M., Krzyżak, J., and Sas-Nowosielska, A. (2013). Environmental Hazards Related toMiscanthusxgiganteuscultivation on Heavy Metal Contaminated Soil. E3S Web of Conferences 1, 29006. EDP Sciences. doi:10.1051/e3sconf/20130129006
Polechońska, L., and Klink, A. (2014). Trace Metal Bioindication and Phytoremediation Potentialities of Phalaris arundinacea L. (Reed Canary Grass). J. Geochem. Explor. 146, 27–33. doi:10.1016/j.gexplo.2014.07.012
Popova, V., Petkova, Z., Ivanova, T., Stoyanova, M., Lazarov, L., Stoyanova, A., et al. (2018). Biologically Active Components in Seeds of Three Nicotiana Species. Ind. Crops Prod. 117, 375–381. doi:10.1016/j.indcrop.2018.03.020
Pottier, M., García de la Torre, V. S., Victor, C., David, L. C., Chalot, M., and Thomine, S. (2015). Genotypic Variations in the Dynamics of Metal Concentrations in poplar Leaves: a Field Study with a Perspective on Phytoremediation. Environ. Pollut. 199, 73–82. doi:10.1016/j.envpol.2015.01.010
Prakasa Rao, E. V. S., Gopinath, C. T., and Khanuja, S. P. S. (2008). Environmental, Economic and Equity Aspects of Vetiver in South India. In First National Indian Vetiver Workshop. The Vetiver International. Kochi. India, 21–23.
Prapagdee, B., Chanprasert, M., and Mongkolsuk, S. (2013). Bioaugmentation with Cadmium-Resistant Plant Growth-Promoting Rhizobacteria to Assist Cadmium Phytoextraction by Helianthus annuus. Chemosphere 92, 659–666. doi:10.1016/j.chemosphere.2013.01.082
Prasad, A., Chand, S., Kumar, S., Chattopadhyay, A., and Patra, D. D. (2014). Heavy Metals Affect Yield, Essential Oil Compound, and Rhizosphere Microflora of Vetiver (Vetiveria zizanioides Linn. Nash) Grass. Commun. Soil Sci. Plant Anal. 45 (11), 1511–1522. doi:10.1080/00103624.2014.904334
Prieto-Sandoval, V., Jaca, C., and Ormazabal, M. (2018). Towards a Consensus on the Circular Economy. J. Clean. Prod. 179, 605–615. doi:10.1016/j.jclepro.2017.12.224
Pulford, I., and Dickinson, N. (2005). Phytoremediation Technologies Using Trees. Biotechnol. Bioremed 14, 383–403. doi:10.1201/9781420032048.sec4
Pulford, I. D., Riddell-Black, D., and Stewart, C. (2002). Heavy Metal Uptake by Willow Clones from Sewage Sludge-Treated Soil: the Potential for Phytoremediation. Int. J. Phytorem. 4, 59–72. doi:10.1080/15226510208500073
Puschenreiter, M., Mench, M., Bert, V., Kumpiene, J., Kidd, P., et al. (2014). “Increasing the Applicability of Gentle Soil Remediation Methods: Lessons from the Greenland Project. 3,” in Rencontres nationales de la recherche sur les sites et sols pollués, Paris, France, Nov 2014 Paris, France. ffineris-01862484f Available at: https://hal-ineris.archives-ouvertes.fr/ineris-01862484/document.
Puschenreiter, M., Wittstock, F., Friesl-Hanl, W., and Wenzel, W. W. (2013). Predictability of the Zn and Cd Phytoextraction Efficiency of a Salix smithiana Clone by DGT and Conventional Bioavailability Assays. Plant Soil 369 (1-2), 531–541. doi:10.1007/s11104-013-1597-0
Putwattana, N., Kruatrachue, M., Kumsopa, A., and Pokethitiyook, P. (2015). Evaluation of Organic and Inorganic Amendments on maize Growth and Uptake of Cd and Zn from Contaminated Paddy Soils. Int. J. Phytorem. 17 (2), 165–174. doi:10.1080/15226514.2013.876962
Quaggiotti, S., Barcaccia, G., Schiavon, M., Nicolé, S., Galla, G., Rossignolo, V., et al. (2007). Phytoremediation of Chromium Using Salix Species: Cloning ESTs and Candidate Genes Involved in the Cr Response. Gene 402 (1-2), 68–80. doi:10.1016/j.gene.2007.07.021
Quintela-Sabarís, C., Marchand, L., Kidd, P. S., Friesl-Hanl, W., Puschenreiter, M., Kumpiene, J., et al. (2017). Assessing Phytotoxicity of Trace Element-Contaminated Soils Phytomanaged with Gentle Remediation Options at Ten European Field Trials. Sci. Total Environ. 599-600, 1388–1398. doi:10.1016/j.scitotenv.2017.04.187
Rajkumar, M., Ae, N., Prasad, M. N. V., and Freitas, H. (2010). Potential of Siderophore-Producing Bacteria for Improving Heavy Metal Phytoextraction. Trends Biotechnol. 28, 142–149. doi:10.1016/j.tibtech.2009.12.002
Ramesh, M. (2016). Kenaf (Hibiscus cannabinus L.) Fibre Based Bio-Materials: A Review on Processing and Properties. Prog. Mater. Sci. 78-79, 1–92. doi:10.1016/j.pmatsci.2015.11.001
Ramón, F., and Lull, C. (2019). Legal Measures to Prevent and Manage Soil Contamination and to Increase Food Safety for Consumer Health: The Case of Spain. Environ. Pollut. 250, 883–891. doi:10.1016/j.envpol.2019.04.074
Ranum, P., Peña-Rosas, J. P., and Garcia-Casal, M. N. (2014). Global maize Production, Utilization, and Consumption. Ann. N.Y. Acad. Sci. 1312 (1), 105–112. doi:10.1111/nyas.12396
Rasa, K., Heikkinen, J., Hannula, M., Arstila, K., Kulju, S., and Hyväluoma, J. (2018). How and Why Does Willow Biochar Increase a clay Soil Water Retention Capacity? Biomass and Bioenergy 119, 346–353. doi:10.1016/j.biombioe.2018.10.004
Raveau, R., Fontaine, J., Hijri, M., and Sahraoui, A. L. H. (2020). The Aromatic Plant Clary Sage Shaped Bacterial Communities in the Roots and in the Trace Element-Contaminated Soil More Than Mycorrhizal Inoculation–A Two-Year Monitoring Field Trial. Front. Microbiol. 11, 1–18. doi:10.3389/fmicb.2020.586050
Redovniković, I. R., De Marco, A., Proietti, C., Hanousek, K., Sedak, M., Bilandžić, N., et al. (2017). Poplar Response to Cadmium and Lead Soil Contamination. Ecotoxicol. Environ. Saf. 144, 482–489. doi:10.1016/j.ecoenv.2017.06.011
Reinikainen, J., Sorvari, J., and Tikkanen, S. (2016). Finnish Policy Approach and Measures for the Promotion of Sustainability in Contaminated Land Management. J. Environ. Manage. 184, 108–119. doi:10.1016/j.jenvman.2016.08.046
Reiss, E. R., and Drinkwater, L. E. (2018). Cultivar Mixtures: a Meta-analysis of the Effect of Intraspecific Diversity on Crop Yield. Ecol. Appl. 28 (1), 62–77. doi:10.1002/eap.1629
Remigio, A. C., Chaney, R. L., Baker, A. J. M., Edraki, M., Erskine, P. D., Echevarria, G., et al. (2020). Phytoextraction of High Value Elements and Contaminants from Mining and mineral Wastes: Opportunities and Limitations. Plant Soil 449, 11–37. doi:10.1007/s11104-020-04487-3
Rheay, H. T., Omondi, E. C., and Brewer, C. E. (2020). Potential of Hemp (Cannabis sativa L.) for Paired Phytoremediation and Bioenergy Production. Glob. Change Biol. Bioenergy 13 (4), 525–536. doi:10.1111/gcbb.12782
Richardson, A. E., Barea, J.-M., McNeill, A. M., and Prigent-Combaret, C. (2009). Acquisition of Phosphorus and Nitrogen in the Rhizosphere and Plant Growth Promotion by Microorganisms. Plant Soil 321, 305–339. doi:10.1007/s11104-009-9895-2
Risueño, Y., Petri, C., and Conesa, H. M. (2020). The Importance of Edaphic Niches Functionality for the Sustainability of Phytomanagement in Semiarid Mining Impacted Ecosystems. J. Environ. Manage. 266, 110613. doi:10.1016/j.jenvman.2020.110613
Rizwan, M., Ali, S., Qayyum, M. F., Ok, Y. S., Zia-ur-Rehman, M., Abbas, Z., et al. (2017). Use of maize (Zea mays L.) for Phytomanagement of Cd-Contaminated Soils: a Critical Review. Environ. Geochem. Health 39 (2), 259–277. doi:10.1007/s10653-016-9826-0
Rizwan, M., Ali, S., Rizvi, H., Rinklebe, J., Tsang, D. C. W., Meers, E., et al. (2016). Phytomanagement of Heavy Metals in Contaminated Soils Using sunflower: A Review. Crit. Rev. Environ. Sci. Technol. 46, 1498–1528. doi:10.1080/10643389.2016.1248199
Rizwan, M., Ali, S., Zia ur Rehman, M., Tsang, D. C. W., Bashir, A., et al. (2018). Cadmium Phytoremediation Potential of Brassica Crop Species: a Review. Sci. Total Environ. 631-632, 1175–1191. doi:10.1016/j.scitotenv.2018.03.104
Rizzo, E., Bardos, P., Pizzol, L., Critto, A., Giubilato, E., Marcomini, A., et al. (2016). Comparison of International Approaches to Sustainable Remediation. J. Environ. Manage. 184, 4–17. doi:10.1016/j.jenvman.2016.07.062
Robinson, B. H., Anderson, C. W. N., and Dickinson, N. M. (2015). Phytoextraction: Where's the Action? J. Geochem. Explor.n 151, 34–40. doi:10.1016/j.gexplo.2015.01.001
Robinson, B. H., Bañuelos, G., Conesa, H. M., Evangelou, M. W. H., and Schulin, R. (2009). The Phytomanagement of Trace Elements in Soil. Crit. Rev. Plant Sci. 28, 240–266. doi:10.1080/07352680903035424
Robinson, B. H., Green, S. R., Chancerel, B., Mills, T. M., and Clothier, B. E. (2007). Poplar for the Phytomanagement of boron Contaminated Sites. Environ. Pollut. 150 (2), 225–233. doi:10.1016/j.envpol.2007.01.017
Rodríguez, H., and Fraga, R. (1999). Phosphate Solubilizing Bacteria and Their Role in Plant Growth Promotion. Biotechnol. Adv. 17, 319–339. doi:10.1016/S0734-9750(99)00014-2
Rodríguez-Seijo, A., Lago-Vila, M., Andrade, M. L., and Vega, F. A. (2016). Pb Pollution in Soils from a Trap Shooting Range and the Phytoremediation Ability of Agrostis capillaris L. Environ. Sci. Pollut. Res. 23 (2), 1312–1323. doi:10.1007/s11356-015-5340-7
Roongtanakiat, N., and Akharawutchayanon, T. (2017). Evaluation of Vetiver Grass for Radiocesium Absorption Ability. Agric. Nat. Resour. 51 (3), 173–180. doi:10.1016/j.anres.2017.01.002
Roongtanakiat, N., and Sanoh, S. (2011). Phytoextraction of Zinc, Cadmium and lead from Contaminated Soil by Vetiver Grass. Kasetsart J. (Nat Sci. 45, 603–612.
Rosenkranz, T., Kidd, P., and Puschenreiter, M. (2018). Effect of Bacterial Inoculants on Phytomining of Metals from Waste Incineration Bottom Ash. Waste Manage. 73, 351–359. doi:10.1016/j.wasman.2017.12.006
Rossi, L., Fusi, E., Baldi, G., Fogher, C., Cheli, F., Baldi, A., et al. (2013). Tobacco Seeds By-Product as Protein Source for Piglets. Ojvm 03, 73–78. doi:10.4236/ojvm.2013.31012
Ruiz, E., Romero, I., Moya, M., Cara, C., Vidal, J. D., and Castro, E. (2013). Dilute Sulfuric Acid Pretreatment of sunflower Stalks for Sugar Production. Bioresour. Technol. 140, 292–298. doi:10.1016/j.biortech.2013.04.104
Rusinowski, S., Krzyżak, J., Clifton-Brown, J., Jensen, E., Mos, M., Webster, R., et al. (2019). New Miscanthus Hybrids Cultivated at a Polish Metal-Contaminated Site Demonstrate High Stomatal Regulation and Reduced Shoot Pb and Cd Concentrations. Environ. Pollut. 252, 1377–1387. doi:10.1016/j.envpol.2019.06.062
Ruttens, A., Boulet, J., Weyens, N., Smeets, K., Adriaensen, K., Meers, E., et al. (2011). Short Rotation Coppice Culture of willows and Poplars as Energy Crops on Metal Contaminated Agricultural Soils. Int. J. Phytorem. 13, 194–207. doi:10.1080/15226514.2011.568543
Ruttens, A., Colpaert, J. V., Mench, M., Boisson, J., Carleer, R., and Vangronsveld, J. (2006a). Phytostabilization of a Metal Contaminated sandy Soil. II: Influence of Compost And/or Inorganic Metal Immobilizing Soil Amendments on Metal Leaching. Environ. Pollut. 144 (2), 533–539. doi:10.1016/j.envpol.2006.01.021
Ruttens, A., Mench, M., Colpaert, J. V., Boisson, J., Carleer, R., and Vangronsveld, J. (2006b). Phytostabilization of a Metal Contaminated sandy Soil. I: Influence of Compost And/or Inorganic Metal Immobilizing Soil Amendments on Phytotoxicity and Plant Availability of Metals. Environ. Pollut. 144 (2), 524–532. doi:10.1016/j.envpol.2006.01.038
Saad, R. F., Kobaissi, A., Amiaud, B., Ruelle, J., and Benizri, E. (2018). Changes in Physicochemical Characteristics of a Serpentine Soil and in Root Architecture of a Hyperaccumulating Plant Cropped with a Legume. J. Soils Sediments 18 (5), 1994–2007. doi:10.1007/s11368-017-1903-1
Saharan, B. S., and Nehra, V. (2011). Plant Growth Promoting Rhizobacteria: A Critical Review. Life Sci. Med. Res. 2011, 1–30.
Saleem, M., Asghar, H. N., Zahir, Z. A., and Shahid, M. (2018). Impact of lead Tolerant Plant Growth Promoting Rhizobacteria on Growth, Physiology, Antioxidant Activities, Yield and lead Content in sunflower in lead Contaminated Soil. Chemosphere 195, 606–614. doi:10.1016/j.chemosphere.2017.12.117
Saleem, M. H., Ali, S., Hussain, S., Kamran, M., Chattha, M. S., Ahmad, S., et al. (2020a). Flax (Linum usitatissimum L.): A Potential Candidate for Phytoremediation? Biological and Economical Points of View. Plants 9 (4), 496. doi:10.3390/plants9040496
Saleem, M. H., Ali, S., Rehman, M., Hasanuzzaman, M., Rizwan, M., Irshad, S., et al. (2020b). Jute: A Potential Candidate for Phytoremediation of Metals-A Review. Plants 9 (2), 258. doi:10.3390/plants9020258
Saleem, M., Pervaiz, Z. H., Contreras, J., Lindenberger, J. H., Hupp, B. M., Chen, D., et al. (2020). Cover Crop Diversity Improves Multiple Soil Properties via Altering Root Architectural Traits. Rhizosphere 16, 100248. doi:10.1016/j.rhisph.2020.100248
Santibañez, C., de la Fuente, L. M., Bustamante, E., Silva, S., Leon-Lobos, P., and Ginocchio, R. (2012). Potential Use of Organic-And Hard-Rock Mine Wastes on Aided Phytostabilization of Large-Scale Mine Tailings under Semiarid Mediterranean Climatic Conditions: Short-Term Field Study. Appl. Environ. Soil Sci. 2012, 1–15. doi:10.1155/2012/895817
Saran, A., Imperato, V., Fernandez, L., Gkorezis, P., d’Haen, J., Merini, L. J., et al. (2020). Phytostabilization of Polluted Military Soil Supported by Bioaugmentation with PGP-Trace Element Tolerant Bacteria Isolated from Helianthus petiolaris. Agronomy 10, 204. doi:10.3390/agronomy10020204
Sarkar, A., Asaeda, T., Wang, Q., Kaneko, Y., and Rashid, M. H. (2018). Arbuscular Mycorrhiza Confers lead Tolerance and Uptake in Miscanthus sacchariflorus. Chem. Ecol. 34, 454–469. doi:10.1080/02757540.2018.1437150
Schreurs, E., Voets, T., and Thewys, T. (2011). GIS-based Assessment of the Biomass Potential from Phytoremediation of Contaminated Agricultural Land in the Campine Region in Belgium. Biomass Bioenergy 35 (10), 4469–4480. doi:10.1016/j.biombioe.2011.09.005
Searle, S. Y., and Malins, C. J. (2014). Will Energy Crop Yields Meet Expectations? Biomass and Bioenergy 65, 3–12. doi:10.1016/j.biombioe.2014.01.001
Sebastian, A., Shukla, P., Nangia, A. K., and Prasad, M. N. V. (2019). “Transgenics in Phytoremediation of Metals and Metalloids,” in Transgenic Plant Technology for Remediation of Toxic Metals and Metalloids. Editors M.N.V. Prasad (Cambridge: Academic Press), 3–22. doi:10.1016/b978-0-12-814389-6.00001-8
Serrapica, F., Masucci, F., Raffrenato, E., Sannino, M., Vastolo, A., Barone, C. M. A., et al. (2019). High Fiber Cakes from Mediterranean Multipurpose Oilseeds as Protein Sources for Ruminants. Animals 9 (11), 918. doi:10.3390/ani9110918
Shabir, G., Arslan, M., Fatima, K., Amin, I., Khan, Q. M., and Afzal, M. (2016). Effects of Inoculum Density on Plant Growth and Hydrocarbon Degradation. Pedosphere 26, 774–778. doi:10.1016/S1002-0160(15)60084-4
Shabir, R., Abbas, G., Saqib, M., Shahid, M., Shah, G. M., Akram, M., et al. (2018). Cadmium Tolerance and Phytoremediation Potential of acacia (Acacia nilotica L.) under Salinity Stress. Int. J. Phytorem. 20 (7), 739–746. doi:10.1080/15226514.2017.1413339
Shahandeh, H., and Hossner, L. R. (2000). Plant Screening for Chromium Phytoremediation. Int. J. Phytorem. 2, 31–51. doi:10.1080/15226510008500029
Shams, K. M., Tichy, G., Fischer, A., Sager, M., Peer, T., Bashar, A., et al. (2010). Aspects of Phytoremediation for Chromium Contaminated Sites Using Common Plants Urtica dioica, Brassica napus and Zea mays. Plant Soil 328 (1), 175–189. doi:10.1007/s11104-009-0095-x
Sharma, A., Kaushik, N., and Rathore, H. (2020). Karanja (Milletia pinnata (L.) Panigrahi): a Tropical Tree with Varied Applications. Phytochem. Rev. 19 (3), 643–658. doi:10.1007/s11101-020-09670-z
Sharma, S., Anand, G., Singh, N., and Kapoor, R. (2017). Arbuscular Mycorrhiza Augments Arsenic Tolerance in Wheat (Triticum aestivum L.) by Strengthening Antioxidant Defense System and Thiol Metabolism. Front. Plant Sci. 8, 1–21. doi:10.3389/fpls.2017.00906
Sharma, S. B., Sayyed, R. Z., Trivedi, M. H., and Gobi, T. A. (2013). Phosphate Solubilizing Microbes: Sustainable Approach for Managing Phosphorus Deficiency in Agricultural Soils. Springerplus 2, 587. doi:10.1128/jcm.35.12.3305-3307.199710.1186/2193-1801-2-587
Sheng, X., Sun, L., Huang, Z., He, L., Zhang, W., and Chen, Z. (2012). Promotion of Growth and Cu Accumulation of Bio-Energy Crop (Zea mays) by Bacteria: Implications for Energy Plant Biomass Production and Phytoremediation. J. Environ. Manage. 103, 58–64. doi:10.1016/j.jenvman.2012.02.030
Sheokand, S., Sarita, D., Ravi, K., and Anita, K. (2012). Studies on Phytoremediation Potential of Azadirachta indica and Acacia nilotica. Int. J. Plant Sci. 7 (2), 351–355.
Shi, X., Zhang, X., Chen, G., Chen, Y., Wang, L., and Shan, X. (2011). Seedling Growth and Metal Accumulation of Selected Woody Species in Copper and lead/zinc Mine Tailings. J. Environ. Sci. 23 (2), 266–274. doi:10.1016/s1001-0742(10)60402-0
Shrestha, R. K., and Lal, R. (2011). Changes in Physical and Chemical Properties of Soil after Surface Mining and Reclamation. Geoderma 161, 168–176. doi:10.1016/j.geoderma.2010.12.015
Sidhu, V., Sarkar, D., and Datta, R. (2020). Growing Biofuel Feedstocks in Copper-Contaminated Soils of a Former Superfund Site. Appl. Sci. 10 (4), 1499. doi:10.3390/app10041499
Šimek, M., Elhottova, D., Mench, M., Giagnoni, L., Nannipieri, P., and Renella, G. (2017). Greenhouse Gas Emissions from a Cu-Contaminated Soil Remediated by In Situ Stabilization and Phytomanaged by a Mixed Stand of poplar, willows and False Indigo-bush. Int. J. Phytorem. 19, 976–984. doi:10.1080/15226514.2016.1267706
Smith, G., and Nadebaum, P. (2016). The Evolution of Sustainable Remediation in Australia and New Zealand: A Storyline. J. Environ. Manage. 184, 27–35. doi:10.1016/j.jenvman.2016.05.010
Smith, L. L., Allen, D. J., and Barney, J. N. (2015). Yield Potential and Stand Establishment for 20 Candidate Bioenergy Feedstocks. Biomass Bioenergy 73, 145–154. doi:10.1016/j.biombioe.2014.12.015
Smith, S. E., and Smith, F. A. (2011). Roles of Arbuscular Mycorrhizas in Plant Nutrition and Growth: New Paradigms from Cellular to Ecosystem Scales. Annu. Rev. Plant Biol. 62, 227–250. doi:10.1146/annurev-arplant-042110-103846
Sood, G. S. (2003). Chemotactic Response of Plant-Growth-Promoting Bacteria Towards Roots of Vesicular-Arbuscular Mycorrhizal Tomato Plants. FEMS Microbiol. Ecol. 45, 219–227. doi:10.1016/S0168-6496(03)00155-7
Souki, Al., Burdová, H., Trubač, J., Štojdl, J., Kuráň, P., et al. (2021). Sequestration in Marginal Land upon Shift towards Perennial C4Miscanthus × giganteus: A Case Study in North-Western Czechia. Agronomy 11 (2), 293. doi:10.3390/agronomy11020293
Stanturf, J. A., and van Oosten, C. (2014). “Operational poplar and Willow Culture,” in Poplars and willows: Trees for Society and the Environment. Editors J.G. Isebrands, and J. Richardson (Rome and Boston: FAO and CABI), 200–257.
Stanturf, J. A., van Oosten, C., Netzer, D. A., Coleman, M. D., and Portwood, C. J. (2001). “Ecology and Silviculture of poplar Plantations,” in Poplar Culture in North America, (Part A). Editors D.I. Dickmann, J.G. Isebrands, J.E. Eckenwalder, and J. Richardson (Ottawa, Canada: NRC Research Press), 153–206.
Steliga, T., and Kluk, D. (2020). Application of Festuca arundinacea in Phytoremediation of Soils Contaminated with Pb, Ni, Cd and Petroleum Hydrocarbons. Ecotoxicol. Environ. Saf. 194, 110409. doi:10.1016/j.ecoenv.2020.110409
Stewart, J. R., Toma, Y., Fernández, F. G., Nishiwaki, A., Yamada, T., and Bollero, G. (2009). The Ecology and Agronomy of Miscanthus sinensis, a Species Important to Bioenergy Crop Development, in its Native Range in Japan: a Review. Glob. Change Biol. Bioenergy 1, 126–153. doi:10.1111/j.1757-1707.2009.01010.x
Stojanović, M. D., Mihajlović, M. L., Milojković, J. V., Lopičić, Z. R., Adamović, M., and Stanković, S. (2012). Efficient Phytoremediation of Uranium Mine Tailings by Tobacco. Environ. Chem. Lett. 10 (4), 377–381. doi:10.1007/s10311-012-0362-6
Stolarski, M. J., Rosenqvist, H., Krzyżaniak, M., Szczukowski, S., Tworkowski, J., Gołaszewski, J., et al. (2015). Economic Comparison of Growing Different Willow Cultivars. Biomass Bioenergy 81, 210–215. doi:10.1016/j.biombioe.2015.07.002
Subsamran, K., Mahakhan, P., Vichitphan, K., Vichitphan, S., and Sawaengkaew, J. (2019). Potential Use of Vetiver Grass for Cellulolytic Enzyme Production and Bioethanol Production. Biocatal. Agric. Biotechnol. 17, 261–268. doi:10.1016/j.bcab.2018.11.023
Sun, H., Xie, Y., Zheng, Y., Lin, Y., and Yang, F. (2018). The Enhancement by Arbuscular Mycorrhizal Fungi of the Cd Remediation Ability and Bioenergy Quality-Related Factors of Five Switchgrass Cultivars in Cd-Contaminated Soil. Peer J. 6, e4425. doi:10.7717/peerj.4425
Sun, Y., Yue, Q., Gao, B., Wang, B., Li, Q., Huang, L., et al. (2012). Comparison of Activated Carbons from Arundo donax Linn with H4P2O7 Activation by Conventional and Microwave Heating Methods. Chem. Eng. J. 192, 308–314. doi:10.1016/j.cej.2012.04.007
Sytar, O., and Prasad, M. N. V. (2016). “Production of Biodiesel Feedstock from the Trace Element Contaminated Lands in Ukraine,” in Bioremediation and Bioeconomy. Editors M.N.V. Prasad (Amsterdam, Netherlands: Elsevier), 3–28. doi:10.1016/B978-0-12-802830-8.00001-0
Thewys, T., Witters, N., Van Slycken, S., Ruttens, A., Meers, E., Tack, F. M. G., et al. (2010). Economic Viability of Phytoremediation of a Cadmium Contaminated Agricultural Area Using Energy maize. Part I: Effect on the Farmer's Income. Int. J. Phytorem. 12, 650–662. doi:10.1080/15226514.2010.493187
Thijs, S., Witters, N., Janssen, J., Ruttens, A., Weyens, N., Herzig, R., et al. (2018). Tobacco, sunflower and High Biomass SRC Clones Show Potential for Trace Metal Phytoextraction on a Moderately Contaminated Field Site in Belgium. Front. Plant Sci. 9, 1879. doi:10.3389/fpls.2018.01879
Thomas, H. L., Arnoult, S., Brancourt-Hulmel, M., and Carrère, H. (2019). Methane Production Variability According to Miscanthus Genotype and Alkaline Pretreatments at High Solid Content. BioEnergy Res. 12 (2), 325–337. doi:10.1007/s12155-018-9957-5
Tirry, N., Tahri Joutey, N., Sayel, H., Kouchou, A., Bahafid, W., Asri, M., et al. (2018). Screening of Plant Growth Promoting Traits in Heavy Metals Resistant Bacteria: Prospects in Phytoremediation. J. Genet. Eng. Biotechnol. 16, 613–619. doi:10.1016/j.jgeb.2018.06.004
Tiwari, S., and Lata, C. (2018). Heavy Metal Stress, Signaling, and Tolerance Due to Plant-Associated Microbes: an Overview. Front. Plant Sci. 9, 1–12. doi:10.3389/fpls.2018.00452
Touceda-González, M., Álvarez-López, V., Prieto-Fernández, Á., Rodríguez-Garrido, B., Trasar-Cepeda, C., Mench, M., et al. (2017). Aided Phytostabilisation Reduces Metal Toxicity, Improves Soil Fertility and Enhances Microbial Activity in Cu-Rich Mine Tailings. J. Environ. Manage. 186, 301–313. doi:10.1016/j.jenvman.2016.09.019
Tran, K. Q., Werle, S., Trinh, T. T., Magdziarz, A., Sobek, S., and Pogrzeba, M. (2020). Fuel Characterization and Thermal Degradation Kinetics of Biomass from Phytoremediation Plants. Biomass Bioenerg. 134, 105469.
Truong, P. (2002). “Vetiver Grass Technology,” in Vetiveria the Genus Vetiveria. Editor M. Maffei (New York: Taylor & Francis), 114–132.
Truong, P. (1999). Vetiver Grass Technology for Mine Rehabilitation. Bangkok: Office of the Royal Development Projects Board.
Truong, P., Van, T. T., and Pinners, E. (2008). Vetiver System Applications Technical Reference Manual. Vetiver Netw. Int. 89, 1–89.
Unterbrunner, R., Puschenreiter, M., Sommer, P., Wieshammer, G., Tlustoš, P., Zupan, M., et al. (2007). Heavy Metal Accumulation in Trees Growing on Contaminated Sites in Central Europe. Environ. Pollut. 148 (1), 107–114. doi:10.1016/j.envpol.2006.10.035
Urra, J., Alkorta, I., and Garbisu, C. (2019a). Potential Benefits and Risks for Soil Health Derived from the Use of Organic Amendments in Agriculture. Agronomy 9 (9), 542. doi:10.3390/agronomy9090542
Urra, J., Alkorta, I., Lanzén, A., Mijangos, I., and Garbisu, C. (2019b). The Application of Fresh and Composted Horse and Chicken Manure Affects Soil Quality, Microbial Composition and Antibiotic Resistance. Appl. Soil Ecol. 135, 73–84. doi:10.1016/j.apsoil.2018.11.005
Urra, J., Alkorta, I., Mijangos, I., Epelde, L., and Garbisu, C. (2019c). Application of Sewage Sludge to Agricultural Soil Increases the Abundance of Antibiotic Resistance Genes without Altering the Composition of Prokaryotic Communities. Sci. Total Environ. 647, 1410–1420. doi:10.1016/j.scitotenv.2018.08.092
USACE (2010). Decision Framework for Incorporation of Green and Sustainable Practices into Environmental Remediation Projects. Interim Guidance 10–01. Washington, D.C.: United States Army Corps of Engineers (USACE)Available at: https://www.hnc.usace.army.mil/Portals/65/docs/Directorates/EMCX/DECISION%20FRAMEWORK%20FOR%20INCORPORATION.pdf (Access on June 14, 2020).
U.S. EPA (2008). Green Remediation: Incorporating Sustainable Environmental Practices into Remediation of Contaminated Sites. EPA 542-R-08–002. Washington D.C: United States Environmental Protection Agency (U.S. EPA).
Vamerali, T., Bandiera, M., Coletto, L., Zanetti, F., Dickinson, N. M., and Mosca, G. (2009). Phytoremediation Trials on Metal- and Arsenic-Contaminated Pyrite Wastes (Torviscosa, Italy). Environ. Pollut. 157 (3), 887–894. doi:10.1016/j.envpol.2008.11.003
Vamerali, T., Bandiera, M., and Mosca, G. (2011). In situ Phytoremediation of Arsenic-and Metal-Polluted Pyrite Waste with Field Crops: Effects of Soil Management. Chemosphere 83 (9), 1241–1248.
Van der Weijde, T., Huxley, L. M., Hawkins, S., Sembiring, E. H., Farrar, K., Dolstra, O., et al. (2017). Impact of Drought Stress on Growth and Quality of Miscanthus for Biofuel Production. GCB Bioenergy 9 (4), 770–782. doi:10.1111/gcbb.12382
Van Heerwaarden, J., Doebley, J., Briggs, W. H., Glaubitz, J. C., Goodman, M. M., de Jesus Sanchez Gonzalez, J., et al. (2011). Genetic Signals of Origin, Spread, and Introgression in a Large Sample of maize Landraces. Proc. Natl. Acad. Sci. 108 (3), 1088–1092. doi:10.1073/pnas.1013011108
Van Slycken, S., Witters, N., Meers, E., Peene, A., Michels, E., Adriaensen, K., et al. (2013a). Safe Use of Metal-Contaminated Agricultural Land by Cultivation of Energy maize (Zea mays). Environ. Pollut. 178, 375–380. doi:10.1016/j.envpol.2013.03.032
Van Slycken, S., Witters, N., Meiresonne, L., Meers, E., Ruttens, A., Van Peteghem, P., et al. (2013b). Field Evaluation of Willow under Short Rotation Coppice for Phytomanagement of Metal-Polluted Agricultural Soils. Int. J. Phytoremediation 15 (7), 677–689. doi:10.1080/15226514.2012.723070
Vangronsveld, J., Herzig, R., Weyens, N., Boulet, J., Adriaensen, K., Ruttens, A., et al. (2009). Phytoremediation of Contaminated Soils and Groundwater: Lessons from the Field. Environ. Sci. Pollut. Res. 16, 765–794. doi:10.1007/s11356-009-0213-6
Vargas, C., Pérez-Esteban, J., Escolástico, C., Masaguer, A., and Moliner, A. (2016). Phytoremediation of Cu and Zn by Vetiver Grass in Mine Soils Amended with Humic Acids. Environ. Sci. Pollut. Res. 23 (13), 13521–13530. doi:10.1007/s11356-016-6430-x
Vegter, J., Lowe, J., and Kasamas, H. (2002). Sustainable Management of Contaminated Land: An Overview. A Report from the Contaminated Land Rehabilitation Network for Environmental Technologies. Vienna: UmweltbundesamtAvailable at: https://www.researchgate.net/publication/326803897_Sustainable_management_of_contaminated_land_an_overview (Accessed on June 11th, 2020).
Vigil, M., Marey-Pérez, M. F., Martinez Huerta, G., and Álvarez Cabal, V. Á. (2015). Is Phytoremediation without Biomass Valorization Sustainable? - Comparative LCA of Landfilling vs. Anaerobic Co-digestion. Sci. Total Environ. 505, 844–850. doi:10.1016/j.scitotenv.2014.10.047
Visentin, C., da Silva Trentin, A. W., Braun, A. B., and Thomé, A. (2019). Application of Life Cycle Assessment as a Tool for Evaluating the Sustainability of Contaminated Sites Remediation: a Systematic and Bibliographic Analysis. Sci. Total Environ. 672, 893–905. doi:10.1016/j.scitotenv.2019.04.034
Vymazal, J. (2016). Concentration Is Not Enough to Evaluate Accumulation of Heavy Metals and Nutrients in Plants. Sci. Total Environ. 544, 495–498. doi:10.1016/j.scitotenv.2015.12.011
Walpola, B. C., Arunakumara, K. K. I. U., Song, J.-S., Lee, C.-J., and Yoon, M.-H. (2014). Mobilization of Heavy Metals in Contaminated Soils Induced by Bioaugmentation of Shewanella xiamenensis HM14. Korean J. Soil Sci. Fertilizer 47, 290–298. doi:10.7745/kjssf.2014.47.4.290
Wanat, N., Austruy, A., Joussein, E., Soubrand, M., Hitmi, A., Gauthier-Moussard, C., et al. (2013). Potentials of Miscanthus×giganteus Grown on Highly Contaminated Technosols. J. Geochem. Explor. 126-127, 78–84. doi:10.1016/j.gexplo.2013.01.001
Wang, A., Wang, M., Liao, Q., and He, X. (2016). Characterization of Cd Translocation and Accumulation in 19 maize Cultivars Grown on Cd-Contaminated Soil: Implication of maize Cultivar Selection for Minimal Risk to Human Health and for Phytoremediation. Environ. Sci. Pollut. Res. 23 (6), 5410–5419. doi:10.1007/s11356-015-5781-z
Wang, C., Liang, W., Yang, Y., Liu, F., Sun, H., Zhu, Z., et al. (2020). Biomass Carbon Aerogels Based Shape-Stable Phase Change Composites with High Light-To-thermal Efficiency for Energy Storage. Renew. Energ. 153, 182–192. doi:10.1016/j.renene.2020.02.008
Wang, L., Hou, D., Shen, Z., Zhu, J., Jia, X., Ok, Y. S., et al. (2019). Field Trials of Phytomining and Phytoremediation: A Critical Review of Influencing Factors and Effects of Additives. Crit. Rev. Environ. Sci. Technol., 1–51.
Wang, Q., Xiong, D., Zhao, P., Yu, X., Tu, B., and Wang, G. (2011). Effect of Applying an Arsenic-Resistant and Plant Growth-Promoting Rhizobacterium to Enhance Soil Arsenic Phytoremediation by Populus deltoides LH05-17. J. Appl. Microbiol. 111, 1065–1074. doi:10.1111/j.1365-2672.2011.05142.x
Wang, X., Chen, C., and Wang, J. (2017). Phytoremediation of Strontium Contaminated Soil by Sorghum bicolor (L.) Moench and Soil Microbial Community-Level Physiological Profiles (CLPPs). Environ. Sci. Pollut. Res. 24 (8), 7668–7678. doi:10.1007/s11356-017-8432-8
Wang, Y., Zhan, M., Zhu, H., Guo, S., Wang, W., and Xue, B. (2012). Distribution and Accumulation of Metals in Soils and Plant from a Lead-Zinc Mineland in Guangxi, South China. Bull. Environ. Contam. Toxicol. 88 (2), 198–203. doi:10.1007/s00128-011-0473-y
Wieshammer, G., Unterbrunner, R., García, T. B., Zivkovic, M. F., Puschenreiter, M., and Wenzel, W. W. (2007). Phytoextraction of Cd and Zn from Agricultural Soils by Salix ssp. and Intercropping of Salix caprea and Arabidopsis halleri. Plant Soil 298 (1-2), 255–264. doi:10.1007/s11104-007-9363-9
Wilde, E. W., Brigmon, R. L., Dunn, D. L., Heitkamp, M. A., and Dagnan, D. C. (2005). Phytoextraction of lead from Firing Range Soil by Vetiver Grass. Chemosphere 61 (10), 1451–1457. doi:10.1016/j.chemosphere.2005.04.059
Witters, N., Mendelsohn, R. O., Van Slycken, S., Weyens, N., Schreurs, E., Meers, E., et al. (2012a). Phytoremediation, a Sustainable Remediation Technology? Conclusions from a Case Study. I: Energy Production and Carbon Dioxide Abatement. Biomass Bioenergy 39, 454–469. doi:10.1016/j.biombioe.2011.08.016
Witters, N., Mendelsohn, R., Van Passel, S., Van Slycken, S., Weyens, N., Schreurs, E., et al. (2012b). Phytoremediation, a Sustainable Remediation Technology? II: Economic Assessment of CO2 Abatement through the Use of Phytoremediation Crops for Renewable Energy Production. Biomass Bioenergy 39, 470–477. doi:10.1016/j.biombioe.2011.11.017
Witters, N., Van Slycken, S., Ruttens, A., Adriaensen, K., Meers, E., Meiresonne, L., et al. (2009). Short-rotation Coppice of Willow for Phytoremediation of a Metal-Contaminated Agricultural Area: a Sustainability Assessment. Bioenerg. Res. 2, 144–152. doi:10.1007/s12155-009-9042-1
Wittwer, R. A., Dorn, B., Jossi, W., and Van Der Heijden, M. G. (2017). Cover Crops Support Ecological Intensification of Arable Cropping Systems. Sci. Rep. 7 (1), 1–12. doi:10.1038/srep41911
Wong, C. C., Wu, S. C., Kuek, C., Khan, A. G., and Wong, M. H. (2007). The Role of Mycorrhizae Associated with Vetiver Grown in Pb-/Zn-Contaminated Soils: Greenhouse Study. Restor Ecol. 15 (1), 60–67. doi:10.1111/j.1526-100x.2006.00190.x
Wu, S. C., Wong, C. C., Shu, W. S., Khan, A. G., and Wong, M. H. (2010). Mycorrhizo-remediation of lead/zinc Mine Tailings Using Vetiver: a Field Study. Int. J. Phytoremed. 13 (1), 61–74. doi:10.1080/15226511003671353
Wu, Z. Z., Yang, J. Y., Zhang, Y. X., Wang, C. Q., Guo, S. S., and Yu, Y. Q. (2021). Growth Responses, Accumulation, Translocation and Distribution of Vanadium in Tobacco and its Potential in Phytoremediation. Ecotoxicol. Environ. Saf. 207, 111297. doi:10.1016/j.ecoenv.2020.111297
Wuana, R. A., and Okieimen, F. E. (2010). Phytoremediation Potential of maize (Zea mays L.). A Review. Afr. J. Gen. Agric. 6 (4), 275–287.
Xu, W., Lu, G., Dang, Z., Liao, C., Chen, Q., and Yi, X. (2013). Uptake and Distribution of Cd in Sweet maize Grown on Contaminated Soils: a Field-Scale Study. Bioinorg. Chem. Appl. 2013, 1–8. doi:10.1155/2013/959764
Xue, K., Zhou, J., Van Nostrand, J., Bes, C., Giagnoni, L., et al. (2018). Functional Activity and Functional Gene Diversity of a Cu-Contaminated Soil Remediated by Aided Phytostabilization Using Compost, Dolomitic limestone and a Mixed Tree Stand. Environ. Pollut. 242, 229–238. doi:10.1016/j.envpol.2018.06.057
Xue, S., Xiao, L., and Yi, Z. (2015). “Miscanthus Production and Utilization in Dongting lake Region, China,” in Abstracts of the Conference on Perennial Biomass Crops for a Resource-Constrained World, Stuttgart-Hohenheim, Germany, 7th–10th September Hohenheim, Germany, 75. Available at www.biomass2015.eu.
Yan, A., Wang, Y., Tan, S. N., Yusof, M. L. M., Ghosh, S., and Chen, Z. (2020). Phytoremediation: a Promising Approach for Revegetation of Heavy Metal-Polluted Land. Front. Plant Sci. 11, 1–15. doi:10.3389/fpls.2020.00359
Yan, J., Chen, W., Luo, F., Ma, H., Meng, A., Li, X., et al. (2012). Variability and Adaptability of Miscanthus Species Evaluated for Energy Crop Domestication. Glob. Change Biol. Bioenergy 4 (1), 49–60. doi:10.1111/j.1757-1707.2011.01108.x
Yang, L. Y., Yang, S. L., Li, J. Y., Ma, J. H., Pang, T., Zou, C. M., et al. (2018). Effects of Different Growth Temperatures on Growth, Development, and Plastid Pigments Metabolism of Tobacco (Nicotiana tabacum L.) Plants. Bot. Stud. 59 (1), 5. doi:10.1186/s40529-018-0221-2
Yang, S., Zhang, Y., Yuan, T. Q., and Sun, R. C. (2015). Lignin–phenol–formaldehyde Resin Adhesives Prepared with Biorefinery Technical Lignins. J. Appl. Polym. Sci. 132 (42493), 1–8. doi:10.1002/app.42493
Yang, Y., Ge, Y., Zeng, H., Zhou, X., Peng, L., and Zeng, Q. (2017). Phytoextraction of Cadmium-Contaminated Soil and Potential of Regenerated Tobacco Biomass for Recovery of Cadmium. Sci. Rep. 7 (1), 1–10. doi:10.1038/s41598-017-05834-8
Yang, Y., Han, X., Liang, Y., Ghosh, A., Chen, J., and Tang, M. (2015). The Combined Effects of Arbuscular Mycorrhizal Fungi (AMF) and lead (Pb) Stress on Pb Accumulation, Plant Growth Parameters, Photosynthesis, and Antioxidant Enzymes in Robinia pseudoacacia L. PLoS One 10, e0145726–24. doi:10.1371/journal.pone.0145726
Yashim, Z., Agbaji, E., Gimba, C., and Idris, S. (2016). Phytoremediation Potential of Ricinus communis L. (Castor Oil Plant) in Northern Nigeria. Ijpss 10 (5), 1–8. doi:10.9734/ijpss/2016/21680
Young, S. D. (2013). “Chemistry of Heavy Metals and Metalloids in Soils,” in Heavy Metals in Soils. Editors J.B. Alloway (Dordrecht: Springer), 51–95. doi:10.1007/978-94-007-4470-7_3
Yuan, Z. L., Xiong, S. P., Li, C.-M., and Ma, X.-M. (2011). Effects of Chronic Stress of Cadmium and lead on Anatomical Structure of Tobacco Roots. Agric. Sci. China 10, 1941–1948. doi:10.1016/s1671-2927(11)60195-8
Yung, L., Bertheau, C., Tafforeau, F., Zappelini, C., Valot, B., Maillard, F., et al. (2021). Partial Overlap of Fungal Communities Associated with Nettle and poplar Roots when Co-occurring at a Trace Metal Contaminated Site. Sci. Total Environ. 782, 146692. doi:10.1016/j.scitotenv.2021.146692
Zalesny, R. S., Headlee, W. L., Gopalakrishnan, G., Bauer, E. O., Hall, R. B., Hazel, D. W., et al. (2019). Ecosystem Services of poplar at Long-term Phytoremediation Sites in the Midwest and Southeast, United States. Wires Energ. Environ. 8 (6), e349. doi:10.1002/wene.349
Zalesny, R. S., Stanturf, J. A., Gardiner, E. S., Bañuelos, G. S., Hallett, R. A., Hass, A., et al. (2016). Environmental Technologies of Woody Crop Production Systems. Bioenergy Res. 9 (2), 492–506. doi:10.1007/s12155-016-9738-y
Zang, H., Blagodatskaya, E., Wen, Y., Xu, X., Dyckmans, J., and Kuzyakov, Y. (2018). Carbon Sequestration and Turnover in Soil under the Energy Crop Miscanthus : Repeated 13 C Natural Abundance Approach and Literature Synthesis. GCB Bioenergy 10 (4), 262–271. doi:10.1111/gcbb.12485
Zapata-Carbonell, J., Bégeot, C., Carry, N., Choulet, F., Delhautal, P., Gillet, F., et al. (2019). Spontaneous Ecological Recovery of Vegetation in a Red gypsum Landfill: Betula pendula Dominates after 10 Years of Inactivity. Ecol. Eng. 132, 31–40. doi:10.1016/j.ecoleng.2019.03.013
Zaprjanova, P., Ivanov, K., Angelova, V., and Dospatliev, L. (2010). “Relation between Soil Characteristics and Heavy Metal Content in Virginia Tobacco,” in 19th World Congress of Soil Science, Soil Solutions for a Changing World, Brisbane, Australia, August 1–6, 2010. Editors R. Gilkes, and N. Prakongkep (Warragul: Australian Society of Soil Science Incorporated), 205–208.
Zehra, A., Sahito, Z. A., Tong, W. B., Tang, L., Hamid, Y., Wang, Q., et al. (2020a). Identification of High Cadmium-Accumulating Oilseed sunflower (Helianthus annuus) Cultivars for Phytoremediation of an Oxisol and an Inceptisol. Ecotoxicol. Environ. Saf. 187, 109857. doi:10.1016/j.ecoenv.2019.109857
Zehra, A., Sahito, Z. A., Tong, W., Tang, L., Hamid, Y., Khan, M. B., et al. (2020b). Assessment of sunflower Germplasm for Phytoremediation of lead-polluted Soil and Production of Seed Oil and Seed Meal for Human and Animal Consumption. J. Environ. Sci. 87, 24–38. doi:10.1016/j.jes.2019.05.031
Zhu, B. C. R., Henderson, G., Chen, F., Fei, H., and Laine, R. A. (2001). Evaluation of Vetiver Oil and Seven Insect-Active Essential Oils against the Formosan Subterranean Termite. J. Chem. Ecol. 27 (8), 1617–1625. doi:10.1023/a:1010410325174
Zhu, Y.-M., Wei, C.-Y., and Yang, L.-S. (2010). Rehabilitation of a Tailing Dam at Shimen County, Hunan Province: Effectiveness Assessment. Acta Ecologica Sin. 30 (3), 178–183. doi:10.1016/j.chnaes.2010.04.009
Ziebell, A. L., Barb, J. G., Sandhu, S., Moyers, B. T., Sykes, R. W., Doeppke, C., et al. (2013). Sunflower as a Biofuels Crop: An Analysis of Lignocellulosic Chemical Properties. Biomass Bioenergy 59, 208–217. doi:10.1016/j.biombioe.2013.06.009
Ziegler-Devin, I., Menana, Z., Chrusciel, L., Chalot, M., Bert, V., and Brosse, N. (2019). Steam Explosion Pretreatment of Willow Grown on Phytomanaged Soils for Bioethanol Production. Ind. Crops Prod. 140, 111722. doi:10.1016/j.indcrop.2019.111722
Keywords: phytoremediation, cash crops, cropping systems, PGPB, bioeconomy, bioinoculants, AMF
Citation: Moreira H, Pereira SIA, Mench M, Garbisu C, Kidd P and Castro PML (2021) Phytomanagement of Metal(loid)-Contaminated Soils: Options, Efficiency and Value. Front. Environ. Sci. 9:661423. doi: 10.3389/fenvs.2021.661423
Received: 30 January 2021; Accepted: 21 June 2021;
Published: 04 August 2021.
Edited by:
Jane A Entwistle, Northumbria University, United KingdomReviewed by:
Richard Alastair Lord, University of Strathclyde, United KingdomAndrew Brian Cundy, University of Southampton, United Kingdom
Copyright © 2021 Moreira, Pereira, Mench, Garbisu, Kidd and Castro. This is an open-access article distributed under the terms of the Creative Commons Attribution License (CC BY). The use, distribution or reproduction in other forums is permitted, provided the original author(s) and the copyright owner(s) are credited and that the original publication in this journal is cited, in accordance with accepted academic practice. No use, distribution or reproduction is permitted which does not comply with these terms.
*Correspondence: Helena Moreira, aGdtb3JlaXJhQHVjcC5wdA==
†Deceased