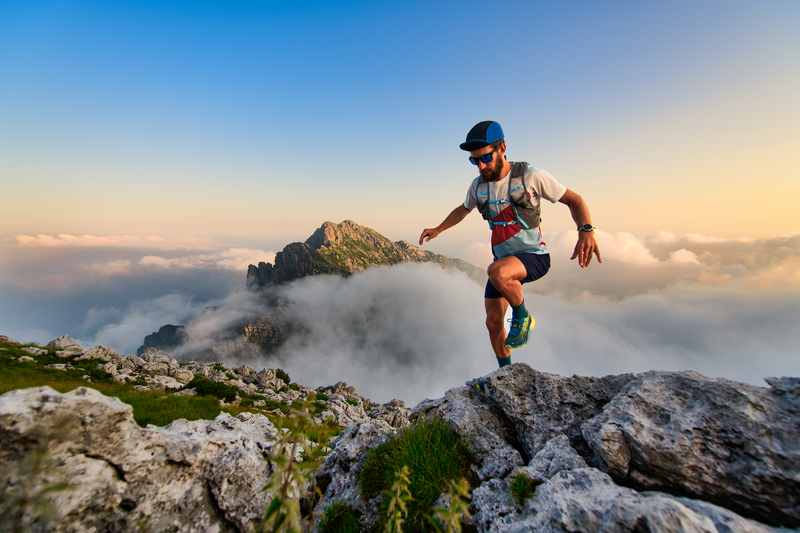
95% of researchers rate our articles as excellent or good
Learn more about the work of our research integrity team to safeguard the quality of each article we publish.
Find out more
ORIGINAL RESEARCH article
Front. Environ. Sci. , 19 March 2021
Sec. Soil Processes
Volume 9 - 2021 | https://doi.org/10.3389/fenvs.2021.631112
This article is part of the Research Topic Observing, Modeling and Understanding Processes in Natural and Managed Peatlands View all 17 articles
A correction has been applied to this article in:
Corrigendum: Methane and Nitrous Oxide Production From Agricultural Peat Soils in Relation to Drainage Level and Abiotic and Biotic Factors
Greenhouse gas emissions from drained agricultural peatlands contribute significantly to global warming. In a laboratory study using intact cores of peat soil from eight different sites in Sweden, factors controlling the emission of the greenhouse gases nitrous oxide (N2O) and methane (CH4) were examined. Soil properties, and the abundance of the total microbial community (16S rRNA gene abundance), and genes encoding for functions controlling N2O emissions (bacterial and archaeal amoA, nirS, nirK, nosZI, and nosZII) were analyzed and compared against measured greenhouse gas emissions. Emissions were measured at different drainage levels, i.e., higher soil water suction values, since drainage is an important factor controlling greenhouse gas emissions from peat soils. The results showed that N2O and CH4 emissions were generally low, except for N2O emissions at near water-saturated conditions, for which three soils displayed high values and large variations in fluxes. Relationships between N2O emissions and soil properties were mainly linked to soil pH, with higher emissions at lower pH. However, specific assemblages of nitrogen cycling guilds that included nosZII, typically present in non-denitrifying N2O reducers, were detected in soils with low N2O emissions. Overall, these results indicate that both pH and biotic controls determine net N2O fluxes.
Emissions of the greenhouse gases carbon dioxide (CO2), nitrous oxide (N2O), and methane (CH4) to the atmosphere have resulted in global warming, while N2O is also involved in destruction of stratospheric ozone (Conrad, 1996). Soils world-wide play an important role in these emissions, with drained agricultural peat soils in particular emitting substantial amounts of CO2 and N2O (Taft et al., 2017). In the 19th century, large peatland areas in Sweden were drained for agricultural purposes, in order to produce food for a growing population. Today, many of these drained peat soils have been abandoned or are under forestry, but the remaining agricultural peat soils contribute 6–8% of total annual anthropogenic greenhouse gas emissions in Sweden (Berglund and Berglund, 2010).
The CO2, CH4, and N2O emitted from soils mainly originate from microbial processes. Emissions of CO2 from drained peat soils occur when the aerated topmost peat layer decomposes, whereas CH4 can be produced in the deeper, water-filled layer by methanogens and potentially oxidized in the aerated upper layer by methane-oxidizing bacteria. Nitrous oxide can be produced during the first step of nitrification, oxidation of ammonia (NH3) to nitrite (NO2–), which is performed by ammonia-oxidizing archaea (AOA) or ammonia-oxidizing bacteria (AOB) (Supplementary Figure 1). Nitrous oxide is also produced by microbial activity during the denitrification process when conditions in the soil are anoxic. Denitrification reduces nitrate (NO3–) to N2O or dinitrogen (N2) in a stepwise process, in the latter case with N2O as an intermediate. There are also non-denitrifying N2O reducers in soil (Jones et al., 2013). The relationship between N2O-producing and N2O-consuming communities regulates net emissions of N2O from the soil (Philippot et al., 2011; Domeignoz-Horta et al., 2016). Drainage and groundwater level have an impact on relative emissions of CO2, N2O, and CH4. For agricultural peatlands, groundwater level is the most important factor regulating emissions of greenhouse gases (Renger et al., 2002; Beyer and Höper, 2015; Regina et al., 2015). Changes in soil moisture due to drying or wetting can influence the availability of dissolved organic carbon and nitrogen species, and therefore alter the microbial community composition and N2O emissions (Banerjee et al., 2016). Furthermore, in field conditions N2O emissions are affected by, e.g., freeze-thaw cycles (Wagner-Riddle et al., 2017), rain events (Kandel et al., 2013), and nitrogen (N) application rates (Bouwman et al., 2002). The presence of living plants can increase both N2O and CH4 emissions, as rhizodeposition is an easily available carbon source (Kuzyakov, 2002). The magnitude of these fluxes are controlled by the gas diffusivity in the soil, which is mainly affected by soil bulk density and water-filled pore space (Smith et al., 2018). However, Taft et al. (2018) showed that total greenhouse gas emissions decline when the groundwater level is at the soil surface, but this is not an option in all agricultural fields, where an optimum groundwater level needs to be found to mitigate greenhouse gas emissions while maintaining traditional crop production (Kløve et al., 2017).
The aim of this study was to identify soil factors controlling emissions of N2O and CH4 from drained and cultivated peat soils at different groundwater levels, and since N2O is more important for drained soils further examine the microbial community properties, i.e., abundances if the total bacterial community and genes encoding functions controlling N2O emissions. To obtain controlled conditions, this was done as a laboratory study using intact cores of peat soil from eight sites in southern Sweden, selected to represent a wide range of drained and cultivated peat soils. Soil properties and gene abundances were analyzed in order to determine general and site-specific responses of N2O and CH4 emissions.
In autumn 2011, soil samples were collected at eight different agricultural sites on drained peat soil in southern Sweden (Supplementary Figure 2). Topsoil was sampled at all sites (soils 1–8) and subsoil was sampled at four sites (soils 5–8). All fields except that where soil 8 was sampled were on active farms, with crop production (soils 2 and 4) or combined crop-dairy production (soils 1, 3, 5–7). Soil 8 was taken at a site that was once a dairy farm, but had been abandoned for several years. Three of the farms had vegetables and potatoes in their crop rotation (soils 2, 3, 4). The same eight topsoil and four subsoil samples were used in our previous study Norberg et al. (2018), where they were numbered differently (numbers in brackets); 1–3 (1–3), 4 (5), 5–8 (6–9).
Detailed descriptions of field soil sampling and of the experimental set-up can be found in Norberg et al. (2018). In brief, intact soil cores were sampled in steel cylinders (Ø 7.2 cm, height 10 cm), at approximately 5–15 cm depth for topsoil samples and 20–50 cm depth for subsoil samples. Replicate soil cores to be used in soil analyses and greenhouse gas emissions measurements were taken within a small area (<1 m2). Upon extraction, the cylinders were sealed at both ends with plastic lids and stored in wooden boxes in a cold store (5°C) until the experiment started.
At the start of the experiment, intact soil cores in their cylinders were assigned to plastic boxes (50 cm × 80 cm). Each box contained one sample from each of the 12 soils, with a total of 84 samples distributed across seven boxes. All boxes were treated similarly and were assumed independent in the statistical analysis. The boxes were brought into the experiment one at a time. Before the start of measurements, the caps on the cylinders were removed and the 12 soil samples in the box were kept at room temperature (20°C) for 2 days and then soaked in tap-water for 3 days, until water-saturated. The 12 samples were then placed on a suction sand bed (Romano et al., 2002) for successive adjustment to one of three soil water suction heads: near water-saturated, 0.5 and 1.0 m water column (∼5 and 10 kPa), corresponding to a groundwater level in field conditions of 0.05, 0.5, and 1.0 m below the soil surface, respectively. At all conditions the soil samples were weighed for water content calculations and then greenhouse gas emissions were measured.
When all gas emissions measurements had been completed, the soil cores in three of the seven boxes were divided into two sub-samples, one frozen at −18°C and one refrigerated at +5°C, to be used for subsequent analyses. Soil cores from the four remaining boxes were dried at 105°C for 72 h and weighed for dry weight-based emissions calculations. The mean dry weight (dw) of soil samples in these boxes was used for the corresponding soil samples in the other boxes.
Emissions of N2O and CH4 were measured using a similar approach to that used for determination of CO2 emissions in Norberg et al. (2018). Each soil sample cylinder was placed in a polypropylene jar (Ø 11 cm, height 12 cm) with air-tight screw lids equipped with two injection needles (Ø 0.8 mm, 40 mm long). The jars had thick walls (∼1.5 mm) and potential gas leakage was considered negligible. Gas was sampled by connecting plastic tubing to the injection needles in the lid and circulating the air in the closed jar for 30 s in a 22-mL vial sealed with a rubber septum. During this time, the air in the vial was exchanged seven times, and a representative air sample was thus collected. Fluxes of N2O and CH4 were determined by taking samples at time zero when the lid was closed, and then at 40, 80, and 120 min. The gas samples were analyzed using a gas chromatograph equipped with electron capture and flame ionization detectors (Clarus 500 GC, PerkinElmer, United States).
The N2O and CH4 emission rates from the soils were calculated from the linear increase in gas concentration in the jar headspace during the closure time, as described in Norberg et al. (2018). All measurements of N2O and CH4 were used unless they showed obvious bias upon visual inspection.
Humification degree (H1–H10) of the peat soils was determined according to von Post (1922). The frozen soil samples from the greenhouse gas emissions experiment were used for analysis of mineral nitrogen [nitrate (NO3–) and ammonium (NH4+)] on a TRAACS 800 AutoAnalyzer (Bran & Luebbe, Germany). The refrigerated soil samples were used for different analyses within 30 days of completion of the gas measurements. Total nitrogen (tot-N), total carbon (tot-C), and carbonate carbon (carb-C) content were determined by dry combustion on a LECO CN-2000 analyzer (St. Joseph, MI, United States). Soil pH was measured at a soil-solution ratio of 1:5 with deionized water. Organic matter content (loss on ignition) was measured by dry combustion at 550°C for 24 h, after pre-drying at 105°C for 24 h. Water-extractable organic carbon (WEOC), here presented as total WEOC, was determined by a modified version of the method of Ghani et al. (2003), as described in detail in Norberg et al. (2018). The results were presented as mg WEOC g–1 tot-C in the soil.
Samples for microbiological analyses were taken at a soil water suction head of 0.5 m water column from the soil cylinders in the three boxes used for soil analysis. A small soil drill with inner diameter 3 mm was used to obtain soil cores, with the drill was inserted about 3.5 cm into the soil. The upper 0.5 cm of the core was removed and the remaining 3.0 cm part was placed in a 2 mL microcentrifuge tube and kept frozen until analysis. The drill was disinfected twice between every sample.
DNA was extracted from the soil plug (40–200 mg soil) using the FastDNA Spin kit for Soil (MP Biomedicals, United States) according to the manufacturer’s instructions. Extract concentrations were determined with the Qubit system (Thermo Fisher Scientific, United States) and the samples were diluted to 1 ng DNA μL–1. Quantitative PCR (qPCR) was used to determine abundances of specific genes, which in turn were used as proxies for the size of microbial communities harboring those genes. For the abundance of the total bacterial community, the bacterial 16S rRNA gene was quantified (Muyzer et al., 1993). For ammonia oxidizers, the archaeal (Tourna et al., 2008) and bacterial (Rotthauwe et al., 1997) amoA genes were quantified, while for denitrifiers, the nitrite reductases encoded by nirS (Throbäck et al., 2004) or nirK (Henry et al., 2004) genes were quantified. For N2O reducers, the genes nosZI (Henry et al., 2006) and nosZII (Jones et al., 2013) coding for nitrous oxide reductases were quantified. The quantifications were performed using gene-specific primers as described in Hellman et al. (2019), with bovine serum albumin (BSA) in all reactions and 10 ng of DNA per reaction for amoA genes and 2 ng of DNA for nir and nos genes. Cycling protocols and primer concentrations used are described in Supplementary Table 1.
Normality was tested with a Ryan-Joiner test. Emissions data on N2O, CH4, and CO2 (CO2 data from Norberg et al., 2018) did not meet the normality requirement and were log10 transformed before statistical analysis. Because of negative data values (consumption of gas), a constant (the smallest possible integer) was added to get a positive value before transformation. Gene abundance data were log10 transformed before statistical analysis to meet the normality requirement. In calculations with gene data per g dw, dry weight data from a soil water suction of 0.5 m were used.
Differences between means of soil properties, greenhouse gas emissions at different suction heads, and gene abundances were tested with one-way ANOVA. When significant effects (p < 0.05) were found, Tukey’s honest significant difference (HSD) test was used to compare mean values. When only two variables were tested, a students’ t-test was used. Relationships between soil properties and greenhouse gas emissions were tested with linear and non-linear regression models (p < 0.05) for data from boxes I, II, and IV. These statistical analyses were carried out using Minitab (Minitab Inc., version 18.1).
To explore the structure of the nitrogen reducing assemblages, principal component analysis (PCA) was performed using ratios between abundances of each functional gene and the 16S rRNA gene. Soil chemical and physical data were correlated to the ordination using the function envfit in the R package vegan (Oksanen et al., 2018) and vectors representing significant (p < 0.05) factors were included in the ordination. Metadata used for overlaying vectors were the variables in Table 1 (excluding humification degree), tot-N, air-filled pore space (AFPS) at the three soil water suction heads, pore volume (square root-transformed), and fluxes of N2O, CH4, and CO2 (tot-N, AFPS, pore volume, bulk density, and CO2 data from Norberg et al., 2018, gas data transformed as described). Permutation MANOVA (PERMANOVA, 999 permutations) on a Bray dissimilarity matrix based on the gene ratios was used to evaluate differences in overall nitrogen cycling assemblages between soils, using the function adonis in R package vegan. The multivariate analyses were performed in R, version 3.6.1 (R Core Team, 2016).
Topsoil content of tot-C ranged from 26 to 43% and the tot-N content from 1.6 to 3.2%. Soils 3, 4, and 6 had a higher carb-C content (2.9–5.3%) than the other soils (0.2–0.5%). Therefore, these soils also had high pH (6.9–7.4), while pH in the other soils was between 5.9 and 6.7. Soils 5–7 were sampled on the same farm, but soil characteristics showed large variation (Table 1). On average, the subsoil differed from the corresponding topsoil, with lower C/N ratio, porosity, and WEOC, but higher bulk density and NH4+, while no difference in pH, org-C, carb-C, and NO3– was found between topsoil and subsoil (p < 0.05).
The N2O emissions from the soil cores were significantly higher (p < 0.05) at near water-saturated conditions than at the two drainage steps, which had similar N2O emissions (Figures 1A–C). At near water-saturated conditions, the highest N2O emissions were recorded from soils 1, 2, and 8, but with a wide range, while for the other soils the N2O emissions were lower and the range was smaller (Figure 1A). At a suction head of 0.5 and 1.0 m water column, the variation between the soils was small and soil 5 displayed the highest N2O emissions.
Figure 1. Soil nitrous oxide (N2O) fluxes (ng kg–1 dry soil min–1) at (A) near water-saturated conditions, (B) 0.5 m water column, and (C) 1.0 m water column; and (D–F) methane (CH4) fluxes at the respective soil water suction heads. Different letters denote significantly different values of the mean (open circle, n = 7, p < 0.05). Note the different scales.
Similarly to the N2O fluxes, the CH4 fluxes were significantly higher under near water-saturated conditions than at the two drainage steps, which had similar CH4 fluxes (Figures 1D–F). In general, soils 5 and 7 displayed a larger range of CH4 fluxes, with more high values, and soil 8 showed negative values at all suction heads. The N2O, CH4, and CO2 fluxes (CO2 data from Norberg et al., 2018) showed no relationship at any of the three soil water suction heads tested (p > 0.05).
Subsoil samples had lower N2O emissions than topsoil samples at a soil water suction head of 0.5 and 1.0 m water column (p < 0.05), but no difference at near water-saturated conditions (Figure 2). However, subsoil samples had lower emissions of CH4 than topsoil samples at near water-saturated conditions and at a soil water suction head of 0.5 m, but not at 1.0 m water column (Figure 2). At near water-saturated conditions, N2O fluxes from topsoils correlated positively with NO3– and negatively with NH4+ (p < 0.05) (Table 2). Other factors correlating with N2O fluxes at different soil water suction heads were pH and carb-C, with increasing N2O emissions with decreasing carb-C and pH (p < 0.05) (Table 2). Several carbon fractions (org-C, tot-C, C/N ratio, WEOC) showed a significant positive relationship with N2O emissions (p < 0.05) (Table 2). Fluxes of CH4 displayed a negative relationship with NO3– and a positive relationship with pH (p < 0.05) (Table 2).
Figure 2. Soil nitrous oxide (N2O) fluxes (ng kg–1 dry soil min–1) from topsoil and subsoil samples of soils 5–8 at (A) near water-saturated conditions, (B) 0.5 m water column, and (C) 1.0 m water column; and (D–F) methane (CH4) fluxes at the respective soil water suction heads. Different subscript letters denote significantly different values of the mean (open circle, n = 28, p < 0.05). Note the different scales.
Table 2. Linear relationships (p-values) between nitrous oxide (N2O) and methane (CH4) fluxes and soil properties at the three soil water suction heads: −0.05 m (near water-saturated), 0.5 m, and 1.0 m water column.
The total bacterial community size, i.e., abundance of 16S rRNA gene copies g–1 dw soil, differed between the topsoils (p < 0.05) (Figure 3A). Soil 3 had a lower abundance than most other soils, while soils 6–8 displayed the highest abundances. The abundance of AOB communities reflected the pattern observed for the total community (Figure 3B). In contrast, the AOA could only be properly quantified in soils 2, 4, 5, and 6 and displayed low abundances, 1.7 × 107–5.1 × 108 copies g–1 dw soil. Nevertheless, soil 6 had significantly higher AOA abundance than soils 2, 4, and 5 (p < 0.05). Because AOA were not detected in half of the soil samples, they were excluded from other statistical analyses.
Figure 3. Abundances of (A) 16S rRNA gene, (B) ammonia-oxidizing bacteria (AOB), (C) nirS, (D) nirK, (E) nosZI, and (F) nosZII (copies g–1 dry soil) for the eight topsoils. Different letters denote significantly different values of the mean (open circle, n = 2–3, p < 0.05). Note the different scales.
Across samples, nirS was more abundant than nirK (p < 0.05) and the variation between soils was higher (Figures 3C,D). The abundance of nosZI was higher than that of nosZII across the soils (p < 0.05), with the highest in soil 7 and 6, respectively. The abundance was lowest in soils 3 and 4 for nosZI and in soil 3 for nosZII (Figures 3E,F). All soils had Σnos/Σnir ratio <1 (range 0.04–0.22), indicating genetic potential for net production of N2O (Supplementary Table 2). The lowest ratio was found in soil 6 and the highest in soil 8 (p < 0.05), while for the other soils there were no significant differences. No relationship was found between Σnos/Σnir ratio and N2O emissions from the eight topsoils at any of the three soil water suction heads tested (p > 0.05).
There were differences in gene abundances between topsoil and subsoil at the four sites where this comparison could be made (soils 5–8). AOB and nosZI were more abundant in topsoils than in subsoils, but for the 16S rRNA gene, nirS, nirK, and nosZII there were no differences (p > 0.05, data not shown).
The concatenated functional gene abundances normalized to total bacterial community size, as visualized in the PCA plots, indicated different nitrogen cycling guild assemblages in each of the eight topsoils (PERMANOVA: R2 = 0.925, p = 0.001) (Figure 4). Soil NH4+ content and C/N ratio were the strongest drivers shaping the guild assemblages in the topsoils, but soil pH was also important. The relative abundance of nirS-type denitrifiers coincided with AOB and nosZII N2O reducers, resulting in soils 4 and 6, which were dominated by these assemblages, having lower N2O emissions than the other soils at near water saturation and at a suction head of 0.5 m water column (Figures 1, 4). The relative abundance of nirK-type denitrifiers and nosZI co-varied, with soils 5 and 7 and some of the replicates of soils 1 and 8 having higher emissions (depending on drainage level) (Figures 1, 4). The wide range of soil characteristics exhibited by soils 5–7, which originated from the same farm and were collected within 1 km2 (Table 1), was reflected in the nitrogen cycling guild assemblages (Figure 4).
Figure 4. Nitrogen cycling assemblages in topsoils. Principal component analysis of the respective ratios between abundances of nirS, nirK, nosZI, nosZII, and bacterial amoA and 16S rRNA gene abundances in the eight soils at a water suction head of 0.5 m. Solid arrows show direction of soil factors that correlate significantly (p < 0.05), with the ordination and arrow length proportional to the strength of correlation. Dashed arrows indicate increasing relative abundance of each functional group in relation to soil sample. The nitrous oxide (N2O) values represent fluxes at near water-saturated conditions, WEOC, water-extractable organic C; carbC, carbonate carbon; orgC, organic carbon.
Overall, in this laboratory study N2O emissions from the peat soil samples were higher and more variable at near water-saturated conditions than under more aerated soil conditions, indicating that a fluctuating water level at near saturated soil conditions triggers N2O emissions. Similarly, a previous field study on agricultural peat soil reported that N2O emissions increased after several days of rainfall and that a decline in N2O emissions could be seen after drainage of the topsoil, due to lowering of the groundwater level (Taghizadeh-Toosi et al., 2019). Those authors concluded that a stable groundwater level could potentially control N2O emissions from soil. In laboratory studies on peat soil columns, pulses of N2O emissions have been recorded during draining and wetting events (Dinsmore et al., 2009; Taft et al., 2018). In field studies, episodic N2O fluxes correlated with rain events have been reported (Maljanen et al., 2004; Elder and Lal, 2008; Kandel et al., 2013). Tiemeyer et al. (2016) suggested that this may be due to N2O production by nitrification rather than denitrification. This was confirmed by Liimatainen et al. (2018), who concluded that nitrification is the main process for N2O production in peat soils. However, others have recorded reduced N2O emissions when the groundwater level is at the soil surface, but elevated emissions when the groundwater level is lowered (Regina et al., 1999; van Beek et al., 2011; Taft et al., 2018). Optimal drainage levels for reduced N2O emissions have been discussed in several studies and a suction head of 0.1–0.5 m is suggested (Regina et al., 2015; Susilawati et al., 2016; Taft et al., 2018; Wen et al., 2020). However, lowering of the groundwater level as an agricultural mitigation option for N2O emissions will instead increase soil CO2 emissions (Norberg et al., 2018). Likely, an optimal drainage level is soil-dependent, due to local abiotic or biotic factors, as is the case in our study. Here, soils 1, 2, and 8 appears to be more likely to emit large amounts of N2O in near water-saturated conditions while soils 5 and 7 shows higher probability of CH4 production in more aerated conditions than the other soils. In the present laboratory study, the greenhouse gas emissions measurements probably display higher fluxes than would be the case under field conditions. As was concluded by Norberg et al. (2018), the moisture of the topsoil in the soil cores never reached levels as low as it can be under field conditions and the constant temperature of 20°C in the laboratory was much higher than average field temperature during the growing season in Sweden. On the other hand, the absence of living plants probably reduced the N2O emissions compared to field conditions since rhizodeposition stimulates N2O production (Ai et al., 2020), although the supply of N may be limited during the growing season due to plant uptake.
There was a correlation between increasing N2O emissions and decreasing pH of the soil cores tested in this study, as also found in some previous studies (Weslien et al., 2009; Andert et al., 2012; Norberg et al., 2016). However, this is not always the case (Maljanen et al., 2010; Taft et al., 2017). Correlation analysis in the present study showed that N2O emissions increased with increasing carbon content (tot-C, org-C, WEOC, C/N ratio; p < 0.05) at different soil water suction heads. In mineral soils, N2O emissions have been shown to increase with increasing carbon availability (Petersen et al., 2008), probably due to the limitation in easily available carbon sources, which is not the case in carbon-rich peat soils. Instead, the relationship between N2O emissions and soil carbon content in peat soils in the present study may be due to the significant correlation between decreasing C content (tot-C, org-C, WEOC, C/N ratio) and increasing carb-C content, i.e., increasing pH. Lower N2O emissions at high pH indicate that liming could be an agricultural mitigation option but this will most likely be counteracted by increased CO2 emissions (Ivarson, 1977).
At near water-saturated conditions, N2O emissions increased with increasing NO3– content and with decreasing NH4+ content. Liimatainen et al. (2018) observed a positive correlation between N2O emissions and NO3–, but concluded that increased soil phosphorus and copper concentrations were the most important factor regulating N2O emissions from peat soils with low C/N ratio (15–27). Copper is an essential part of nos activity and lack of copper can prevent the last step in the denitrification process, thus promoting N2O release, while copper is also essential for the ammonium oxidizers. Findings by Liimatainen et al. (2018) that higher copper content in peat soils gives higher N2O emissions indicate that nitrification is a more important process than denitrification for N2O production in peat soils.
The eight soils analyzed had different assemblages of nitrogen cycling guilds, with soil C/N ratio, NH4+ content, and pH appearing to be the strongest drivers shaping the different assemblages. Soils 4 and 6, which were dominated by AOB, nirS-type denitrifiers, and nosZ clade II N2O reducers, correlated negatively with N2O emissions. This agrees with findings that nosZ clade II microbes, which dominate the non-denitrifying N2O reducers (Graf et al., 2014), can be important N2O sinks (Jones et al., 2014; Domeignoz-Horta et al., 2016). Further, nirS-type denitrifiers are more often complete denitrifiers, with N2O reduction capacity, than nirK types (Graf et al., 2014). However, the soils with lower N2O emissions in the present study also had neutral pH. Thus, it is not possible to separate the effects of pH and biotic factors, although both could possibly explain the emissions patterns observed in this study. The abundance ratio of nir and nos genes was low for all eight soils (0.04–0.22), which indicates higher genetic potential for production of N2O than for reduction of N2O to N2. However, since gene abundance ratios were not related to the emissions patterns observed, this potential was not realized or synchronized during the experiment.
Anoxic conditions, optimal for CH4 production, probably take a longer time to achieve than the approximately 3 days allowed in this study. Therefore, no high CH4 emissions were recorded, as also found in other similar studies, where CH4 emissions are generally low or negligible and CH4 may instead be consumed (Blodau and Moore, 2003; Karki et al., 2014; Musarika et al., 2017; Taft et al., 2017; Matysek et al., 2019). Nevertheless, CH4 emissions were higher at near water-saturated conditions than at the two drainage steps, where the CH4 fluxes were similar. This confirms previous findings in two studies on peat soil monoliths of no difference in fluxes of CH4 between groundwater levels of 0.15 and 0.55 m depth (Susilawati et al., 2016; Wen et al., 2020). In contrast, Tiemeyer et al. (2016) found a threshold at a groundwater depth of 0.2 m, where CH4 fluxes increased and showed greater variability than at lower groundwater levels. In the present study, CH4 fluxes increased with increasing pH, which contradicts findings in Maljanen et al. (2010). The negative relationship between CH4 fluxes and NO3– content was mainly due to the high NO3– values in combination with low CH4 fluxes in soil 8.
Under drained conditions, topsoils emitted more N2O than subsoil samples, confirming findings by Säurich et al. (2019) and Berglund and Berglund (2011). Possible reasons for the higher N2O emissions, are higher nutrient availability, higher pH, narrower C/N ratio, and higher bulk density (Säurich et al., 2019). In the present study, there was no difference in pH between topsoil and subsoil samples, while C/N ratio was lower and bulk density and NH4+ content were higher for topsoils compared with the corresponding subsoils.
Biotic factors also differed between topsoils and subsoils. The abundance of AOB was significantly lower in subsoils than in topsoils (on average for the four soils for which this comparison could be made). Jia and Conrad (2009) reported a three-fold decrease in AOB from topsoil to subsoil in a mineral soil, while Andert et al. (2011) observed no differences in abundance of ammonia oxidizers between depths in a peat soil. The nosZI gene, coding for N2O reductase as the last step in the denitrification pathway, was also significantly more abundant in the topsoil samples. Koops et al. (1996) showed that the topsoil (0–20 cm) contributes most (over 70%) to total denitrification in drained peat soil, while the subsoil (20–40 cm) contributes up to 30% of the total N losses by denitrification. This indicates that peat soils can have favorable conditions for denitrification in both topsoil and subsoil. Andert et al. (2012) found that the community composition for denitrifiers did not change with soil depth, but that potential denitrification rate decreased rapidly with depth.
Measurements of N2O emissions from different peat soils revealed wide variations at near water-saturated conditions and lower levels at two experimental drainage intensities (suction head 0.5 and 1.0 m water column). This confirms that high and fluctuating groundwater level can increase N2O emissions more than deeper drainage with higher air-filled porosity. The N2O emissions were primarily correlated with peat soil pH and carbonate-carbon content, with increasing N2O emissions with decreasing pH. No strong correlation between N2O emissions and individual gene abundances was detected, but specific assemblages of N cycling guilds were indicative of soils with lower emissions. Subsoils emitted less N2O than topsoils under drained conditions and, as expected, CH4 fluxes from both topsoil and subsoil were low at all soil water levels. Lower N2O emissions at high pH and lower groundwater levels indicate that liming and increased drainage intensity could be agricultural mitigation options, but will most likely increase CO2 emissions. Finding an optimal drainage level to minimize greenhouse gas emissions in agricultural peatlands is a challenge since it is soil-dependent, due to local abiotic and biotic factors.
The raw data supporting the conclusions of this article will be made available by the authors, without undue reservation.
KB, SH, and ÖB designed the research. LN and KB planned the research activities. LN and MH performed the research, collected, and analyzed the data. LN wrote the manuscript. SH, MH, KB, and ÖB adjusted the manuscript. All authors contributed to the article and approved the submitted version.
This work was supported by the Swedish Farmers Foundation for Agricultural Research (grant number H0733481) and the Nordic Joint Committee for Agricultural and Food Research (NKJ) through the Swedish Research Council (Formas) (grant number 220-2010-13).
The authors declare that the research was conducted in the absence of any commercial or financial relationships that could be construed as a potential conflict of interest.
We wish to thank the farmers at the sampling sites for letting us access their land and the staff in the laboratory at the Department of Soil and Environment for performing all the soil analyses. Special thanks to Christina Öhman for excellent ideas and help with carrying out the experiment.
The Supplementary Material for this article can be found online at: https://www.frontiersin.org/articles/10.3389/fenvs.2021.631112/full#supplementary-material
Ai, C., Zhang, M., Sun, Y., Zhang, L., Zeng, L., Liu, Y., et al. (2020). Wheat rhizodeposition stimulates soil nitrous oxide emission and denitrifiers harboring the nosZ clade I gene. Soil Biol. Biochem. 143:107738. doi: 10.1016/j.soilbio.2020.107738
Andert, J., Börjesson, G., and Hallin, S. (2012). Temporal changes in methane oxidizing and denitrifying communities and their activities in a drained peat soil. Wetlands 32, 1047–1055. doi: 10.1007/s13157-012-0335-3
Andert, J., Wessen, E., Börjesson, G., and Hallin, S. (2011). Temporal changes in abundance and composition of ammonia-oxidizing bacterial and archaeal communities in a drained peat soil in relation to N2O emissions. J. Soils Sediments 11, 1399–1407. doi: 10.1007/s11368-011-0413-9
Banerjee, S., Helgason, B., Wang, L., Winsley, T., Ferrari, B. C., and Siciliano, S. D. (2016). Legacy effects of soil moisture on microbial community structure and N2O emissions. Soil Biol. Biochem. 95, 40–50. doi: 10.1016/j.soilbio.2015.12.004
Berglund, Ö, and Berglund, K. (2010). Distribution and cultivation intensity of agricultural peat and gyttja soils in Sweden and estimation of greenhouse gas emissions from cultivated peat soils. Geoderma 154, 173–180. doi: 10.1016/j.geoderma.2008.11.035
Berglund, Ö, and Berglund, K. (2011). Influence of water table level and soil properties on emissions of greenhouse gases from cultivated peat soil. Soil Biol. Biochem. 43, 923–931. doi: 10.1016/j.soilbio.2011.01.002
Beyer, C., and Höper, H. (2015). Greenhouse gas exchange of rewetted bog peat extraction sites and a Sphagnum cultivation site in northwest Germany. Biogeosciences 12, 2101–2117. doi: 10.5194/bg-12-2101-2015
Blodau, C., and Moore, T. R. (2003). Experimental response of peatland carbon dynamics to a water table fluctuation. Aquat. Sci. 65, 47–62. doi: 10.1007/s000270300004
Bouwman, A. F., Boumans, L. J. M., and Batjes, N. H. (2002). Emissions of N2O and NO from fertilized fields: summary of available measurement data. Glob. Biogeochem. Cycles 16:1058.
Conrad, R. (1996). Soil microorganisms as controllers of atmospheric trace gases (H-2, CO, CH4, OCS, N2O, and NO). Microbiol. Rev. 60, 609–640. doi: 10.1128/mr.60.4.609-640.1996
R Core Team (2016). A Language and Environment for Statistical Computing. Vienna: R Foundation for Statistical Computing.
Dinsmore, K. J., Skiba, U. M., Billett, M. F., and Rees, R. M. (2009). Effect of water table on greenhouse gas emissions from peatland mesocosms. Plant Soil 318, 229–242. doi: 10.1007/s11104-008-9832-9
Domeignoz-Horta, L. A., Putz, M., Spor, A., Bru, D., Breuil, M. C., Hallin, S., et al. (2016). Non-denitrifying nitrous oxide-reducing bacteria – an effective N2O sink in soil. Soil Biol. Biochem. 103, 376–379. doi: 10.1016/j.soilbio.2016.09.010
Elder, J. W., and Lal, R. (2008). Tillage effects on gaseous emissions from an intensively farmed organic soil in North Central Ohio. Soil Tillage Res. 98, 45–55. doi: 10.1016/j.still.2007.10.003
Ghani, A., Dexter, M., and Perrott, K. W. (2003). Hot-water extractable carbon in soils: a sensitive measurement for determining impacts of fertilisation, grazing and cultivation. Soil Biol. Biochem. 35, 1231–1243. doi: 10.1016/s0038-0717(03)00186-x
Graf, D. R. H., Jones, C. M., and Hallin, S. (2014). Intergenomic comparisons highlight modularity of the denitrification pathway and underpin the importance of community structure for N2O emissions. PLoS One 9:e114118. doi: 10.1371/journal.pone.0114118
Hellman, M., Bonilla-Rosso, G., Widerlund, A., Juhanson, J., and Hallin, S. (2019). External carbon addition for enhancing denitrification modifies bacterial community composition and affects CH4 and N2O production in sub-arctic mining pond sediments. Water Res. 158, 22–33. doi: 10.1016/j.watres.2019.04.007
Henry, S., Baudoin, E., Lopez-Gutierrez, J. C., Martin-Laurent, F., Brauman, A., and Philippot, L. (2004). Quantification of denitrifying bacteria in soils by nirK gene targeted real-time PCR. J. Microbiol. Methods 59, 327–335. doi: 10.1016/j.mimet.2004.07.002
Henry, S., Bru, D., Stres, B., Hallet, S., and Philippot, L. (2006). Quantitative detection of the nosZ gene, encoding nitrous oxide reductase, and comparison of the abundances of 16S rRNA, narG, nirK, and nosZ genes in soils. Appl. Environ. Microbiol. 72, 5181–5189. doi: 10.1128/aem.00231-06
Ivarson, K. C. (1977). Changes in decomposition rate, microbial population and carbohydrate content of an acid peat bog after liming and reclamation. Can. J. Soil Sci. 7, 129–137. doi: 10.4141/cjss77-017
Jia, Z. J., and Conrad, R. (2009). Bacteria rather than Archaea dominate microbial ammonia oxidation in an agricultural soil. Environ. Microbiol. 11, 1658–1671.
Jones, C. M., Graf, D. R. H., Bru, D., Philippot, L., and Hallin, S. (2013). The unaccounted yet abundant nitrous oxide-reducing microbial community: a potential nitrous oxide sink. ISME J 7, 417–426. doi: 10.1038/ismej.2012.125
Jones, C. M., Spor, A., Brennan, F. P., Breuil, M. C., Bru, D., Lemanceau, P., et al. (2014). Recently identified microbial guild mediates soil N2O sink capacity. Nat. Clim. Chang. 4, 801–805. doi: 10.1038/nclimate2301
Kandel, T. P., Elsgaard, L., Karki, S., and Laerke, P. E. (2013). Biomass yield and greenhouse gas emissions from a drained fen peatland cultivated with reed canary grass under different harvest and fertilizer regimes. Bioenergy Res. 6, 883–895. doi: 10.1007/s12155-013-9316-5
Karki, S., Elsgaard, L., Audet, J., and Laerke, P. E. (2014). Mitigation of greenhouse gas emissions from reed canary grass in paludiculture: effect of groundwater level. Plant Soil 383, 217–230. doi: 10.1007/s11104-014-2164-z
Kløve, B., Berglund, K., Berglund, Ö, Weldon, S., and Maljanen, M. (2017). Future options for cultivated Nordic peat soils: can land management and rewetting control greenhouse gas emissions? Environ. Sci. Policy 69, 85–93. doi: 10.1016/j.envsci.2016.12.017
Koops, J. G., Oenema, O., and Van Beusichem, M. L. (1996). Denitrification in the top and sub soil of grassland on peat soils. Plant Soil 184, 1–10. doi: 10.1007/bf00029269
Kuzyakov, Y. (2002). Review: factors affecting rhizosphere priming effects. J. Plant Nutri. Soil 165, 382–396. doi: 10.1002/1522-2624(200208)165:4<382::aid-jpln382>3.0.co;2-#
Liimatainen, M., Voigt, C., Martikainen, P. J., Hytonen, J., Regina, K., Oskarsson, H., et al. (2018). Factors controlling nitrous oxide emissions from managed northern peat soils with low carbon to nitrogen ratio. Soil Biol. Biochem. 122, 186–195. doi: 10.1016/j.soilbio.2018.04.006
Maljanen, M., Komulainen, V. M., Hytönen, J., Martikainen, P., and Laine, J. (2004). Carbon dioxide, nitrous oxide and methane dynamics in boreal organic agricultural soils with different soil characteristics. Soil Biol. Biochem. 36, 1801–1808. doi: 10.1016/j.soilbio.2004.05.003
Maljanen, M., Sigurdsson, B. D., Guomundsson, J., Oskarsson, H., Huttunen, J. T., and Martikainen, P. J. (2010). Greenhouse gas balances of managed peatlands in the Nordic countries – present knowledge and gaps. Biogeosciences 7, 2711–2738. doi: 10.5194/bg-7-2711-2010
Matysek, M., Leake, J., Banwart, S., Johnson, I., Page, S., Kaduk, J., et al. (2019). Impact of fertiliser, water table, and warming on celery yield and CO2 and CH4 emissions from fenland agricultural peat. Sci. Total Environ. 667, 179–190. doi: 10.1016/j.scitotenv.2019.02.360
Musarika, S., Atherton, C. E., Gomersall, T., Wells, M. J., Kaduk, J., Cumming, A. M. J., et al. (2017). Effect of water table management and elevated CO2 on radish productivity and on CH4 and CO2 fluxes from peatlands converted to agriculture. Sci. Total Environ. 58, 665–672. doi: 10.1016/j.scitotenv.2017.01.094
Muyzer, G., Dewaal, E. C., and Uitterlinden, A. G. (1993). Profiling of complex microbial-populations by denaturing gradient gel-electrophoresis analysis of polymerase chain reaction-amplified genes-coding for 16s ribosomal-RNA. Appl. Environm. Microbiol. 59, 695–700. doi: 10.1128/aem.59.3.695-700.1993
Norberg, L., and Berglund, Ö, and Berglund, K. (2016). Nitrous oxide and methane fluxes during the growing season from cultivated peat soils, peaty marl and gyttja clay under different cropping systems. Acta Agric. Scand. B Soil Plant Sci. 66, 602–612. doi: 10.1080/09064710.2016.1205126
Norberg, L., and Berglund, Ö, and Berglund, K. (2018). Impact of drainage and soil properties on carbon dioxide emissions from intact cores of cultivated peat soils. Mires Peat 21, 1–14.
Oksanen, J., Blanchet, F. G., Friendly, M., Kindt, R., Legendre, P., Mcglinn, D., et al. (2018). vegan: Community Ecology Package. Available online at: https://CRAN.R-project.org/package=vegan. (accessed April 6, 2020).
Petersen, S. O., Schjonning, P., Thomsen, I. K., and Christensen, B. T. (2008). Nitrous oxide evolution from structurally intact soil as influenced by tillage and soil water content. Soil Biol. Biochem. 40, 967–977. doi: 10.1016/j.soilbio.2007.11.017
Philippot, L., Andert, J., Jones, C. M., Bru, D., and Hallin, S. (2011). Importance of denitrifiers lacking the genes encoding the nitrous oxide reductase for N2O emissions from soil. Glob. Chang. Biol. 17, 1497–1504. doi: 10.1111/j.1365-2486.2010.02334.x
Regina, K., Sheehy, J., and Myllys, M. (2015). Mitigating greenhouse gas fluxes from cultivated organic soils with raised water table. Mitig. Adapt. Strateg. Glob. Chang. 20, 1529–1544. doi: 10.1007/s11027-014-9559-2
Regina, K., Silvola, J., and Martikainen, P. J. (1999). Short-term effects of changing water table on N2O fluxes from peat monoliths from natural and drained boreal peatlands. Glob. Chang. Biol. 5, 183–189. doi: 10.1046/j.1365-2486.1999.00217.x
Renger, M., Wessolek, G., Schwarzel, K., Sauerbrey, R., and Siewert, C. (2002). Aspects of peat conservation and water management. J. Plant Nutr. Soil Sci. 165, 487–493. doi: 10.1002/1522-2624(200208)165:4<487::aid-jpln487>3.0.co;2-c
Romano, N., Hopmans, J. W., and Dane, J. H. (2002). “Suction table,” in Methods of Soil Analysis, Part 4-Physical Methods, eds J. H. Dane and C. G. Topp (Madison, WI: Soil Science Society of America), 692–698.
Rotthauwe, J. H., Witzel, K. P., and Liesack, W. (1997). The ammonia monooxygenase structural gene amoA as a functional marker: molecular fine-scale analysis of natural ammonia-oxidizing populations. Appl. Environ. Microbiol. 63, 4704–4712. doi: 10.1128/aem.63.12.4704-4712.1997
Säurich, A., Tiemeyer, B., Dettmann, U., and Don, A. (2019). How do sand addition, soil moisture and nutrient status influence greenhouse gas fluxes from drained organic soils? Soil Biol. Biochem. 135, 71–84. doi: 10.1016/j.soilbio.2019.04.013
Smith, K. A., Ball, T., Conen, F., Dobbie, K. E., Massheder, J., and Rey, A. (2018). Exchange of greenhouse gases between soil and atmosphere: interactions of soil physical factors and biological processes. Eur. J. Soil Sci. 69, 10–20. doi: 10.1111/ejss.12539
Susilawati, H. L., Setyanto, P., Ariani, M., Hervani, A., and Inubushi, K. (2016). Influence of water depth and soil amelioration on greenhouse gas emissions from peat soil columns. Soil Sci. Plant Nutr. 62, 57–68. doi: 10.1080/00380768.2015.1107459
Taft, H. E., Cross, P. A., Edwards-Jones, G., Moorhouse, E. R., and Jones, D. L. (2017). Greenhouse gas emissions from intensively managed peat soils in an arable production system. Agric. Ecosyst. Environ. 237, 162–172. doi: 10.1016/j.agee.2016.11.015
Taft, H. E., Cross, P. A., and Jones, D. L. (2018). Efficacy of mitigation measures for reducing greenhouse gas emissions from intensively cultivated peatlands. Soil Biol. Biochem. 127, 10–21. doi: 10.1016/j.soilbio.2018.08.020
Taghizadeh-Toosi, A., Elsgaard, L., Clough, T. J., Labouriau, R., Ernstsen, V., and Petersen, S. O. (2019). Regulation of N2O emissions from acid organic soil drained for agriculture. Biogeosciences 16, 4555–4575. doi: 10.5194/bg-16-4555-2019
Throbäck, I. N., Enwall, K., and Jarvis, Å, and Hallin, S. (2004). Reassessing PCR primers targeting nirS, nirK and nosZ genes for community surveys of denitrifying bacteria with DGGE. FEMS Microbiol. Ecol. 49, 401–417. doi: 10.1016/j.femsec.2004.04.011
Tiemeyer, B., Albiac Borraz, E., Augustin, J., Bechtold, M., Beetz, S., Beyer, C., et al. (2016). High emissions of greenhouse gases from grasslands on peat and other organic soils. Glob. Change Biol. 22, 4134–4149. doi: 10.1111/gcb.13303
Tourna, M., Freitag, T. E., Nicol, G. W., and Prosser, J. I. (2008). Growth, activity and temperature responses of ammonia-oxidizing archaea and bacteria in soil microcosms. Environ. Microbiol. 10, 1357–1364. doi: 10.1111/j.1462-2920.2007.01563.x
van Beek, C. L., Pleijter, M., and Kuikman, P. J. (2011). Nitrous oxide emissions from fertilized and unfertilized grasslands on peat soil. Nutr. Cycl. Agroecosyst. 89, 453–461. doi: 10.1007/s10705-010-9408-y
von Post, L. (1922). Sveriges geologiska undersöknings torvinventering och några av dess hittills vunna resultat (in swedish). Sven. Mosskulturföreningens Tidskr. 36, 1–27.
Wagner-Riddle, C., Congreves, K., Abalos, D., Berg, A. A., Brown, S. E., Ambadan, J. T., et al. (2017). Globally important nitrous oxide emissions from croplands induced by freeze–thaw cycles. Nat. Geosci. 10, 279–283. doi: 10.1038/ngeo2907
Wen, Y., Zang, H. D., Ma, Q. X., Freeman, B., Chadwick, D. R., Evans, C. D., et al. (2020). Impact of water table levels and winter cover crops on greenhouse gas emissions from cultivated peat soils. Sci. Total Environ. 719:135130. doi: 10.1016/j.scitotenv.2019.135130
Keywords: histosols, methane, nitrous oxide, functional genes, suction head, groundwater level
Citation: Norberg L, Hellman M, Berglund K, Hallin S and Berglund Ö (2021) Methane and Nitrous Oxide Production From Agricultural Peat Soils in Relation to Drainage Level and Abiotic and Biotic Factors. Front. Environ. Sci. 9:631112. doi: 10.3389/fenvs.2021.631112
Received: 19 November 2020; Accepted: 01 March 2021;
Published: 19 March 2021.
Edited by:
Annalea Lohila, University of Helsinki, FinlandCopyright © 2021 Norberg, Hellman, Berglund, Hallin and Berglund. This is an open-access article distributed under the terms of the Creative Commons Attribution License (CC BY). The use, distribution or reproduction in other forums is permitted, provided the original author(s) and the copyright owner(s) are credited and that the original publication in this journal is cited, in accordance with accepted academic practice. No use, distribution or reproduction is permitted which does not comply with these terms.
*Correspondence: Lisbet Norberg, bGlzYmV0Lm5vcmJlcmdAc2x1LnNl
Disclaimer: All claims expressed in this article are solely those of the authors and do not necessarily represent those of their affiliated organizations, or those of the publisher, the editors and the reviewers. Any product that may be evaluated in this article or claim that may be made by its manufacturer is not guaranteed or endorsed by the publisher.
Research integrity at Frontiers
Learn more about the work of our research integrity team to safeguard the quality of each article we publish.