- 1Faculty of Environmental Sciences and Natural Resource Management, Norwegian University of Life Sciences (NMBU), Ås, Norway
- 2Faculty of Chemistry, Biotechnology and Food Science, Norwegian University of Life Sciences (NMBU), Ås, Norway
Liming acidic soils is often found to reduce their N2O emission due to lowered N2O/(N2O + N2) product ratio of denitrification. Some field experiments have shown the opposite effect, however, and the reason for this could be that liming stimulates nitrification-driven N2O production by enhancing nitrification rates, and by favoring ammonia oxidizing bacteria (AOB) over ammonia oxidizing archaea (AOA). AOB produce more N2O than AOA, and high nitrification rates induce transient/local hypoxia, thereby stimulating heterotrophic denitrification. To study these phenomena, we investigated nitrification and denitrification kinetics and the abundance of AOB and AOA in soils sampled from a field experiment 2–3 years after liming. The field trial compared traditional liming (carbonates) with powdered siliceous rocks. As expected, the N2O/(N2O + N2) product ratio of heterotrophic denitrification declined with increasing pH, and the potential nitrification rate and its N2O yield (YN2O: N2O-N/NO3–-N), as measured in fully oxic soil slurries, increased with pH, and both correlated strongly with the AOB/AOA gene abundance ratio. Soil microcosm experiments were monitored for nitrification, its O2-consumption and N2O emissions, as induced by ammonium fertilization. Here we observed a conspicuous dependency on water filled pore space (WFPS): at 60 and 70% WFPS, YN2O was 0.03-0.06% and 0.06–0.15%, respectively, increasing with increasing pH, as in the aerobic soil slurries. At 85% WFPS, however, YN2O was more than two orders of magnitude higher, and decreased with increasing pH. A plausible interpretation is that O2 consumption by fertilizer-induced nitrification cause hypoxia in wet soils, hence induce heterotrophic nitrification, whose YN2O decline with increasing pH. We conclude that while low emissions from nitrification in well-drained soils may be enhanced by liming, the spikes of high N2O emission induced by ammonium fertilization at high soil moisture may be reduced by liming, because the heterotrophic N2O reduction is enhanced by high pH.
Introduction
Although soils are buffered by ion exchange reactions and weathering of minerals (Chadwick and Chorover, 2001), they become gradually acidified by cultivation, primarily due to ammoniacal nitrogen fertilization and loss of base cations (von Uexküll and Mutert, 1995; Guo et al., 2010). Soil acidification is commonly counteracted by carbonate addition, either as frequent low doses or as infrequent heavy dressings (Shaaban et al., 2014; Goulding, 2016). Crop plants display substantial variation in acid tolerance (Goulding, 2016), and even cultivars within one species may vary in their tolerance to soil acidity (Kochian et al., 2004). Thus, the target pH for sustaining high yields will depend on the crop, with the minimum given by its tolerance to acidity and the maximum by the micronutrient availability of the soil at elevated pH (White and Robson, 1989). Liming may also serve other purposes than securing an agronomic minimum pH for crop production. Heavy dressings of CaO (or Ca(OH)2) reduce soil erosion by improving the structure of heavy clay soils (Ulén and Etana, 2014), while the effect on crop growth appears variable, possibly due to reduced manganese availability at high pH in some soils (Blomquist et al., 2018).
Liming beyond the minimum for crops has also been proposed as a means of reducing N2O emissions, since soil acidification leads to high N2O/N2 product ratios of denitrification under standardized anoxic incubations. This was first observed by Wijler and Delwiche (1954), and corroborated by subsequent investigations, although the reason remained obscure (Čuhel and Šimek, 2011). Recent studies of transcription and enzyme kinetics have provided compelling evidence that this is a post-transcriptional phenomenon: the making of functional N2O-reductase is increasingly impaired with increasing proton concentrations (Liu et al., 2014).
Numerous field and microcosm experiments have been conducted to determine if N2O emission can be reduced by increasing soil pH, be it by carbonates (Shaaban et al., 2014, 2015, 2018; Oo et al., 2018), or biochar (reviewed by Cayuela et al., 2014). Although the investigations generally corroborate the hypothesis, there are also cases where N2O emissions were either unaffected or even increased in response to elevated soil pH (see review by Qu et al., 2014). One reason for the variable effect of liming on N2O emissions from soils could be the production of N2O by ammonia oxidizing organisms. The three known groups of ammonia oxidizing organisms are the ammonia oxidizing bacteria (AOB), the ammonia oxidizing archaea (AOA) and the recently discovered comammox group (Daims et al., 2015), which oxidizes NH3 all the way to NO3– While little is known about the ecology and N2O production of comammox, the biochemistry and ecophysiology of AOB and AOA and their N2O production have been subject to intense studies. AOA are frequently found to outnumber AOB in soils with low availability of NH4+ and low pH, while AOB appear to thrive in response to high pH and fertilization with NH4+ (Nicol et al., 2008). Thus, liming acid soils should favor AOB over AOA, which would enhance N2O production by nitrification because AOB produce much more N2O per mole N oxidized than AOA, as pointed out by Hink et al. (2017a).
Next to the prevalence of AOB and AOA, the amount of N2O produced per mole N oxidized could also be affected by the rate of nitrification: nitrification consumes oxygen and produces NO2– and NO3–, hence potentially inducing denitrification, either by heterotrophic or nitrifying organisms (Kool et al., 2011a, b; Huang et al., 2014). Isotope tracing data have been taken to suggest that nitrifier denitrification is more significant than heterotrophic denitrification in soils (Zhu et al., 2013; Wrage-Mönning et al., 2018), but the validity of this has been challenged recently by Bakken and Frostegård (2017), who claim that the isotope tracing method cannot be used to distinguish between the two pathways. This distinction is important in the context of pH management because the net production of N2O by heterotrophic denitrification is strongly dependent on pH, while that by nitrifier denitrification is not: denitrification by nitrifying organisms produces pure N2O regardless of pH, because they lack the gene for N2O reductase (nosZ) (Klotz and Stein, 2011).
While increasing soil pH was expected to reduce the N2O emission from denitrification by enhancing the synthesis of functional N2O reductase, we hypothesized that it would have the opposite effect on N2O emission from nitrification, both by increasing its N2O yield (mol N2O per mol NO3– produced) and by enhancing the potential nitrification rate. The latter would lead to high oxygen consumption after fertilization with ammonium, hence increasing the risk for hypoxia and denitrification if the soil moisture content is high. Such nitrification induced denitrification would be a strong N2O source, hypothetically enhanced by increasing the soil pH, unless effectively counteracted by enhanced synthesis of N2O reductase at high pH. To shed light on the potential stimulation of N2O emission from nitrification after liming, we conducted a series of laboratory incubations with soils sampled over a period of 2 years from a field trial, in which carbonates were compared with siliceous rock powders as agents to increase the soil pH (Nadeem et al., 2015). The motive for testing powdered siliceous rocks was to explore the possibility of using carbonate-free minerals, thus avoiding the emission of carbonate-CO2. In addition, we describe the effect of the rock powders on the AOB/AOA ratio as a function of increasing soil pH.
While corroborating the well-known effect of pH-increase on the synthesis of functional N2O reductase (hence reducing N2O emission from denitrification), the strictly oxic incubations demonstrated that the rate of nitrification and its N2O yield increased with soil pH. We further demonstrate that nitrification-induced denitrification increases with soil moisture, and provide evidence that oxygen consumption by nitrification induces heterotrophic denitrification, rather than nitrifier-denitrification. The results are important because they explain why the effect of liming on the N2O emission induced by ammonium fertilization depends on the soil moisture content: at very high soil moisture content, ammonium fertilization may cause high N2O emission by inducing heterotrophic denitrification, and this emission can be reduced by liming. At modest/low moisture content, ammonium fertilization induces modest N2O emission from nitrification alone, and this is enhanced by liming.
Materials and Methods
Experimental Site and Soil Sampling
Soils were sampled from a field trial established in autumn 2014, to test the effect of finely ground siliceous minerals as alternatives to liming. The trial is situated on the research farm of the Norwegian University of Life Sciences (NMBU) at Ås (59° 49′ N, 10° 47′ E, 75 m a.s.l) in southeast Norway. Mean annual temperature is 7.7°C and mean annual precipitation is 1,083 mm (Hansen and Grimenes, 2015). The field had been under crop rotation including leys since 1953, and the soil (clay loam) had not been limed since 1970. The field trial consists of six treatments: two types of calcareous lime (calcite and dolomite), and three types of siliceous rock powders (olivine, norite, and larvikite) and an untreated control. Each treatment was replicated four times and randomly distributed in two blocks (same blocks as the previous crop rotation experiment). Soil pH and content of soil organic matter measured prior to liming is given in Supplementary Table 1. Minerals were applied during autumn 2014 at a rate of 30 t ha–1 (siliceous rock powders and calcite) and 23 t ha–1 (dolomite). The siliceous minerals were Olivine: Blueguard 63, particle size <63 μm, from the company Sibelco Nordic AS Norway; Larvikite: rock cutting dust from Lundhs AS Norway, which was further ball milled and wet sieved to achieve a particle size <63 μm; Norite: waste material from titanium enrichment process (cyclone removal of particles), from Titania AS Norway, particle size <300 μm (∼50% W/W <63 μm); Calcite obtained as a suspension of colloidal particles (0.4–1.5 μm) from OMYA-Hustadmarmor AS; and Dolomite, obtained as an agricultural lime from Franzefoss A/S Norway. The mineral materials were mixed evenly into the upper 20 cm of the soil by plowing after adding half of the material, then harrowing after adding the second half. Winter wheat was sown in late autumn 2014, but failed to establish, and the field was plowed again in the late spring of 2015. Barley (Hordeum vulgar, cultivar Sunita) was sown on June 5 as a cover crop together with a grass mixture (20% Phleum pratense, 25% Lolium perenne, 25% Festuca pratensis, 20% Festuca arundinacea schreb, 10% Poa pratense L), fertilized with 100 kg N ha–1 (mineral fertilizer, NPK 22:3:10). The cover crop was harvested after 8 weeks. Ley growth was poor in the year of establishment, but improved in subsequent years. The ley was fertilized with 270 kg N ha–1 y–1 as NPK or CAN, split into 120 kg N in spring, 90 kg N after the 1st harvest and 60 kg N after the 2nd harvest. Soil samples were collected in 2015 and 2016 (during summer and late autumn), at 15, 21, and 27 months after liming. A composite sample (2–10 cm) was taken from each replicate plot by pooling at least 6 auger cores per plot. The soil samples were immediately transferred to the laboratory, sieved (2 mm), and stored in plastic bags at 4°C, until used for analyses and laboratory incubations (1–5 days after collection). To determine soil pH, 10 g soil were thoroughly dispersed in 25 mL 0.01 M CaCl2 (hand shaken). The slurry was left to settle overnight, then shaken again, and finally left to settle for 15 min before measuring the pH.
Nitrification and Denitrification Kinetics
Soil Slurry Experiments
Soil slurries were used for potential nitrification and denitrification experiments. Equivalents of 4 g dry weight soil were transferred to 120 ml serum flasks and dispersed in 40 ml 1 mM NH4Cl (for nitrification) or KNO3 solution (for denitrification). All flasks were equipped with Teflon coated magnetic stirrers, and crimp-sealed with Teflon-coated silicone septa for nitrification experiments (to avoid inhibition of nitrification), and butyl rubber septa for denitrification experiments.
To ensure low initial NO3– concentrations in the nitrification assay, the soils were first flooded with deionized water and drained by vacuum in 500 ml filter funnels (Millipore) with 0.45 μm filters, then transferred to the vials (4 g dry weight equivalents). The nitrification assay was performed at 23°C, while shaking the flasks horizontally on a reciprocal shaker (125 rpm) to ensure that fully oxic condition were maintained throughout the incubation. To measure N2O, the bottles were removed from the shaker once or twice per day and placed for a short period in a temperature-controlled water bath (23°C) of the incubation robot (Molstad et al., 2007). The robot sampled the headspace (∼1 mL) with a hypodermic needle, to determine O2 and N2O concentrations. After each sampling, an equal volume of He was pumped back to the headspace to keep the flask pressure at ∼1 atm. We used the new version of the robot, described by Molstad et al. (2016), equipped with an Agilent GC-7890A gas chromatograph with three detectors (FID, TCD, ECD) for determining the concentrations of O2, N2, N2O, and CO2, and with a chemiluminescence detector (Teledyne NO/NOx analyzer mod 200E) for detection of NO. After each gas analysis, the flasks were returned to the reciprocal shaker. Oxygen concentrations in the headspace were monitored throughout, and pure oxygen was added when O2 concentration fell below 16 vol% in the headspace, thus maintaining O2 concentration between 15 and 20 vol%.
Denitrification kinetics were measured by frequent sampling from the headspace of 120 ml serum flasks containing 4 g dry weight soil and 40 ml of 1 mM KNO3. The flasks were placed in the water bath of the incubation robot, and the slurries were stirred continuously (400 rpm). Before the incubation, all flasks were washed with He by repeated evacuation and filling. The final He overpressure was released by using a syringe without plunger containing water. The observed gas kinetics were corrected for dilution and leakage as outlined by Molstad et al. (2007). Soil pH for each replicate sample was measured at the beginning and end of the incubation, and the average of these two values was used for further calculations.
NO2– and NO3– Measurements
Nitrification rates were determined from the accumulation of NO3– and NO2– in the soil slurries over time. Once per day, 0.5 ml of the soil slurry was removed with a syringe and centrifuged at 10,000 g for 15 min at 4°C. NO3– and NO2– concentrations in the supernatant were determined immediately by colorimetry using a microplate reader (Infinite F50, TECAN Austria GmbH) at 540 nm. Both NO3– and NO2– were measured by Griess reaction (Keeney and Nelson, 1982), with NO3– being converted to NO2– by vanadium chloride (Doane and Horwath, 2003).
Calculations
Nitrification rates were calculated from NO2– + NO3– accumulation, while denitrification rates were calculated as the sum of NO, N2O and N2 accumulation. N2O emission potentials from each process were estimated by calculating the N2O yield as N2O/(NO2– + NO3–) for nitrification and the N2O production index (IN2O) for denitrification. The N2O index of denitrification was calculated as described in Liu et al. (2010).
where N2O (t) is the accumulated flux of N2O at any time t, N2 (t) is the accumulated flux of N2 at any time, and T is the time when a certain amount of NO3–-N kg–1 soil was recovered as gaseous N (NO2–, NO, N2O, and N2). IN2O was calculated for two time periods, T10 and T25; i.e., the time point when 10 and 25 μmol NO3–, respectively, had been denitrified to gaseous N.
Incubation Experiments With Remolded Soil
To study nitrification and denitrification under more realistic conditions than in slurries, we conducted a series of incubations with remolded soils.
Aerobic incubation was used to study nitrification (and its N2O emission): 10 g of sieved soil from each plot (15 months after liming) was added to 120 ml serum flasks, packed to a bulk density of 1 g cm–3 and adjusted to 60% water filled pore space (WFPS). To induce nitrification, the soil was amended with a concentrated (NH4)2SO4 solution equivalent to 110 mg N kg–1 soil by carefully distributing the solution throughout the soil volume, by using a syringe with a long needle. The flasks were incubated with air in the headspace at 20°C for 150 h, and O2 consumption and N2O production were monitored by the robotized incubation system described above. Nitrification was estimated from NO3– and NO2– accumulation in parallel offline incubation flasks covered with aluminum foil, which were subsampled periodically for determination of NO3– and NO2– concentrations (0.5 g soil was transferred to Eppendorf tubes with 1 ml deionized water, shaken, and centrifuged). The experiment was repeated at higher WFPS values (70 and 85%) with soils collected 27 months after liming to quantify the net N2O emissions under conditions supporting coupled nitrification-denitrification, i.e., the reduction of NO3– or NO2– from nitrification by denitrification.
Anaerobic incubation of remolded soil was used to study denitrification: Prior to incubation, the soils were flooded with 2 mM KNO3 solution and then drained, as described by Liu et al. (2010). After drainage an equivalent of 20 g dry weight soil was loosely packed into 120 ml serum flasks. Headspace gas was replaced with He. Headspace gasses were monitored as described above.
SSU rRNA Gene Sequence Analysis
DNA was extracted according to the protocol of Lim et al. (2016). To determine the relative proportion of AOB vs. AOA, we conducted amplicon sequencing of the SSU rRNA gene from the microbial communities in the different field plots as sampled December, 2016. Briefly, the V4 region of the 16S rRNA gene was amplified with the 515f (5′-GTGYCAGCMGCCGCGGTAA-3′) and 806rB (5′-GGACTACNVGGGTWTCTAAT-3′) primers (Apprill et al., 2015; Parada et al., 2016) by following the Earth Microbiome Project protocol1, and amplicons were sequenced on an Illumina MiSeq instrument using a 600 cycle kit, v3 (2 × 300 bp paired-end reads). A total of 36 samples were sequenced, which includes field plots that were sequenced in triplicate (Supplementary File 1) as technical replicates. The number of sequence reads per sample ranged from 81,276 to 457,377 with a mean of 299,296 and standard deviation of 99,835. The demultiplexed FASTQ files were obtained from the sequencer and analyzed in the statistical software R2 using the packages DADA2 (Callahan et al., 2016) and Phyloseq (McMurdie and Holmes, 2013). Taxonomy of the resultant Amplicon Sequence Variants (ASVs) was established by reference to the Silva SSU rRNA database release 132 (Yilmaz et al., 2014). We then identified ammonia-oxidizers by searching for the term “nitroso” among all levels of the taxonomic hierarchy, whereby we determined that the ammonia-oxidizing Archaea consisted solely 42 ASVs in 2 classes of the phylum Thaumarchaeota, Nitrososphaeria and “Group_1.1c”. Similarly, we identified ammonia-oxidizing Bacteria as consisting of 77 ASVs within the family Nitrosomonadaceae within the phylum Gammaproteobacteria. The abundances of these ASVs in the different samples were tallied to obtain the relative abundance of AOB and AOA and thus to determine the AOB/AOA ratio. To identify nitrite oxidizing bacteria, we similarly searched for their taxonomic descriptors and identified Nitrospira, Nitrobacter, and Candidatus Nitrotoga as present in the dataset. A total of 20 Nitrospira ASVs were detected while only one sample contained one Nitrobacter ASV and another sample contained a single Candidatus Nitrotoga ASV.
Accession Numbers
The sequence data analyzed in this study is available for download at the Sequence Read Archive (SRA) under BioProject accession PRJNA541961, which corresponds to BioSample accessions SAMN12414160 to SAMN12414195 and SRA accessions SRS5197145 to SRS5197180.
Results
Soil pH
Average soil pH measured in the field plots throughout the 26 months after application of lime and siliceous minerals are shown in Table 1. The pH increased 1.57 units in the calcite treatment, 0.58 in the dolomite, 0.16 in the olivine and 0.11 in the norite treatment. Larvikite had no significant effect on the soil pH (p = 0.12 for pairwise t-test of larvikite versus control). The calcite effect decreased over time by 0.5 units (Supplementary Figure 1).
Denitrification Kinetics in Anoxic Slurry Incubations
The gas kinetics in anoxic slurries of soils sampled 27 months after incorporation of lime and rock powder (Figure 1) showed transient accumulation of both NO and N2O which clearly declined in magnitude with increasing soil pH, more dramatically so for N2O than for NO. Stable plateaus of N2, reached after ∼60 h indicated depletion of N oxides since the levels largely accounted for the initial amount of NO3–-N present in the flasks (∼14 mmol N kg–1; the soil contained ∼4 mmol NO3– kg–1). Table 2 summarizes the maximum denitrification rates, maximum NO and N2O accumulation, and the N2O index (IN2O) for incubation experiments carried out with soils sampled 15, 21, and 27 months after liming. Denitrification rates were lowest in samples taken during winter 2015 (the year of ley establishment), and largest in summer of the first production year, after which they decreased again in the following winter. Denitrification rates exhibited a slight but statistically significant increase with soil pH (Supplementary Figure 3), while the transient accumulation of NO declined with pH. The influence of soil pH on the N2O/(N2O + N2) ratio of denitrification was examined by plotting the N2O index (IN2O) across pH, revealing a marked decrease with increasing pH (Supplementary Figure 4).
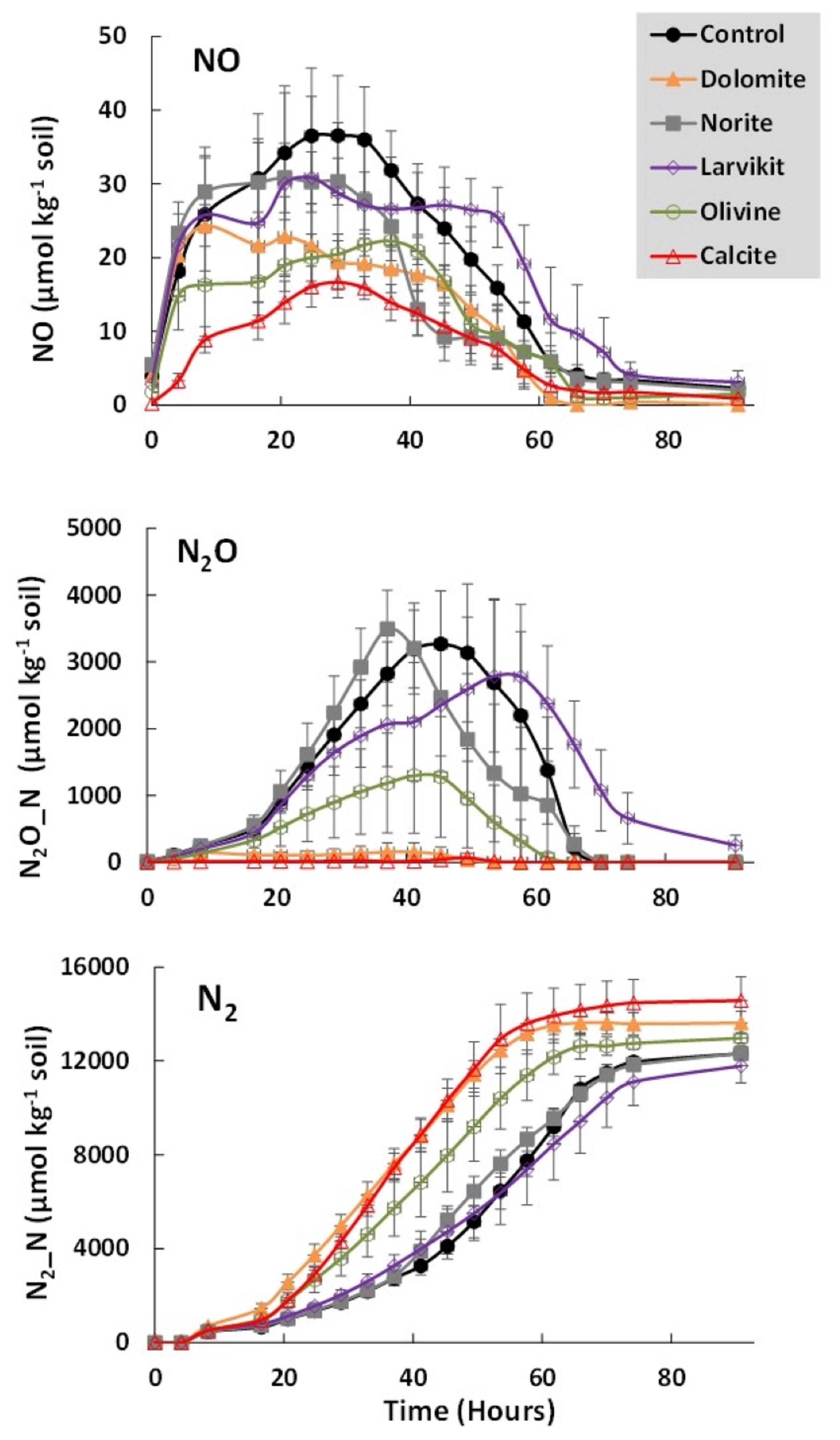
Figure 1. Denitrification in soil slurries. NO and N2O are shown as measured, while N2 is the cumulative production (measured values corrected for sampling loss, see Molstad et al., 2007). Plotted values are means and standard errors of four field-replicates for each treatment, sampled 27 months after mineral applications in the field experiment. Samples taken 15 months after mineral treatment showed very similar response to soil pH whereby the N2O production index (IN2O) declined with soil pH. IN2O for individual soil samples in both experiments are shown in Supplementary Figure 4.
Nitrification and N2O Yield in Oxic Slurry Incubations
Figure 2 shows the kinetics of oxygen consumption, transient accumulation of NO2– and the accumulation of N2O + NO3– in oxic slurries of soils sampled in December 2015 and 2016 (15 and 27 months after incorporation of lime and siliceous minerals). Similar kinetics were observed for soils sampled 21 months after liming and Table 3 summarizes essential variables for all three sampling dates. The oxygen consumption rate was largest in calcite and dolomite treated soils, followed by olivine (p < 0.0005), whereas larvikite and norite treatments were indistinguishable from the control.
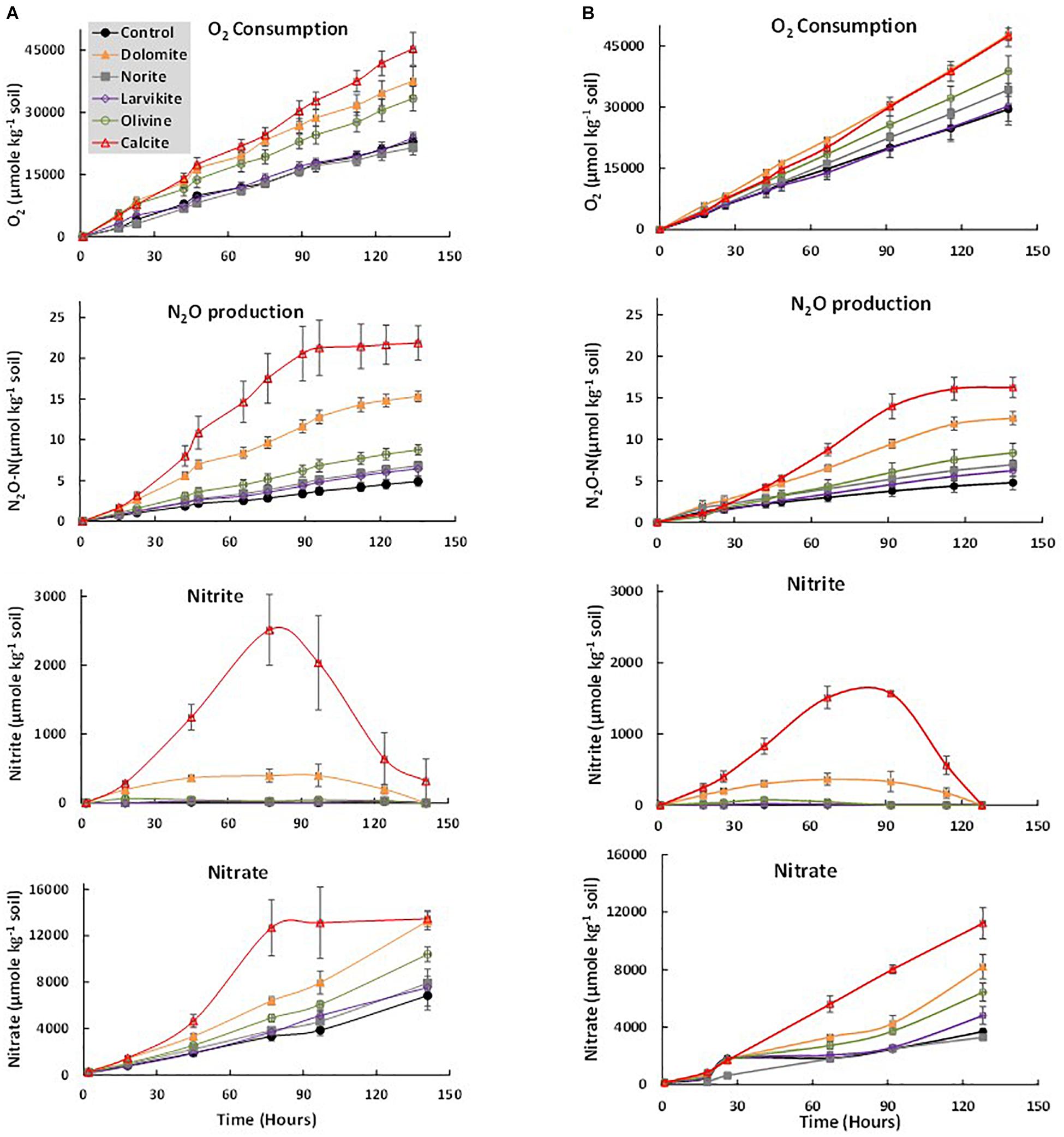
Figure 2. Nitrification in oxic soil slurries. Oxygen consumption (cumulative), and production of N2O nitrite and nitrate oxic soil slurries. Plotted values are the means and standard errors of four field replicates of each mineral treatment. Plotted gas kinetics are from two incubations experiments with soil collected from the field December 2015 (Panel A) and 2016 (Panel B) (15 and 27 months after application of minerals).
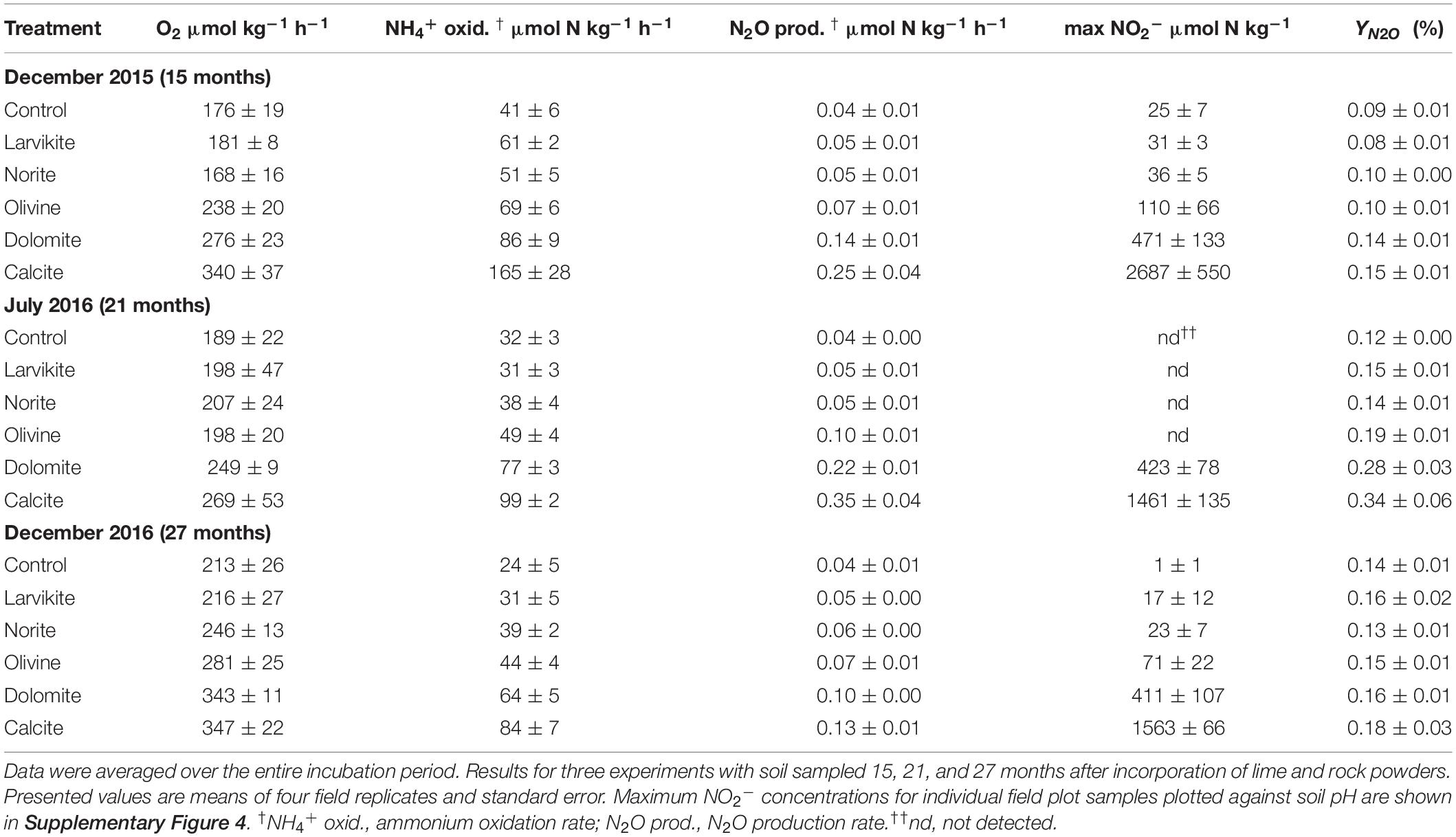
Table 3. Rates of O2 consumption, ammonium oxidation, N2O production, NO2– accumulation and N2O yield (YN2O) in oxic slurry incubations.
The NH4+ oxidation rates (measured as NO2– + NO3– accumulation) increased linearly with pH (Figure 3). NH4+ was evidently not depleted during the incubation, except for the calcite-amended soil sampled 15 months after mineral application (Figure 2: the NO3– plateaus reached after 60–90 h match the initial amounts of NH4+, which was 1.4 mmol NH4+ kg–1). Transient accumulation of NO2– was substantial in the high pH soils (calcite > dolomite), whereas it was marginal in the other treatments and was found to increase exponentially with pH (Supplementary Figure 4). The calculated N2O yield (YN2O) ranged from 0.09 to 0.34% and was significantly higher in the calcite and dolomite treatments than in the other treatments. Assuming that the oxidation of 1 mole of NH3 to NO3– consumes 2 moles of O2, the measured nitrification accounted for 20–90% of the measured oxygen consumption rate (consistently highest for calcite).
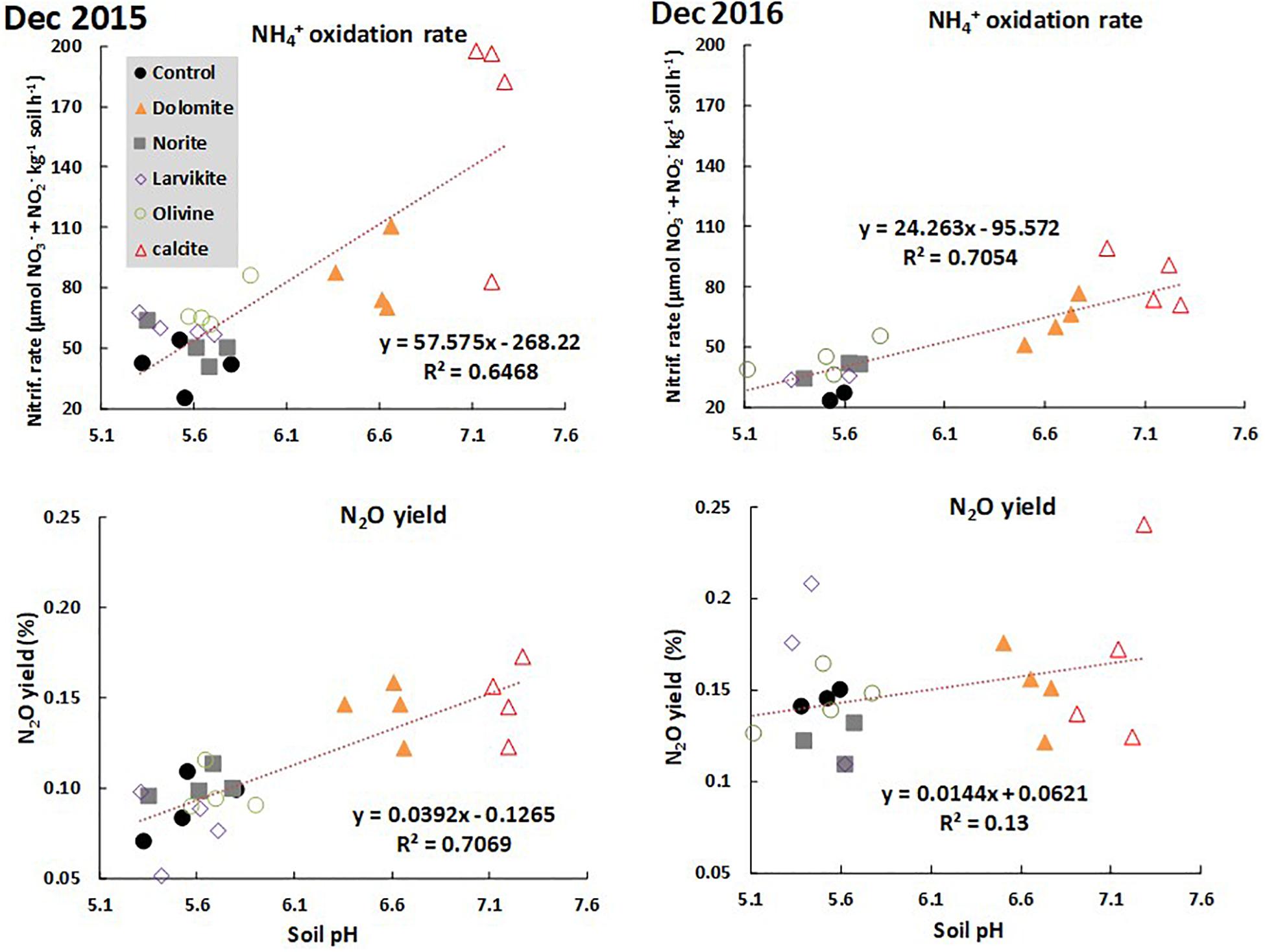
Figure 3. Relationship between soil slurry pH, NH4+ oxidation rate and N2O yield (% of oxidized N emitted as N2O-N) in oxic nitrification assays. The plotted values are single flask values for four field replicates of each treatment. Plotted values are from two incubation experiments with soils collected from field 15 and 27 months after application of minerals.
Anoxic Incubations of Remolded Soil
For loosely remolded soil sampled 27 months after liming and incubated under fully anoxic conditions, denitrification gas kinetics exhibited similar N gas accumulation patterns as observed in the anoxic soil slurries. Calcite and dolomite treated soils accumulated less N2O than any other treatment (Figure 4). Denitrification rate, maximum N2O and N2O index (IN2O) in remolded soil experiments are shown in Table 4. Denitrification rates were calculated for the period 0–50 h of incubation, and the N2O index for the time point when 500 μmol N kg–1 soil was recovered as NO + N2O + N2-N.
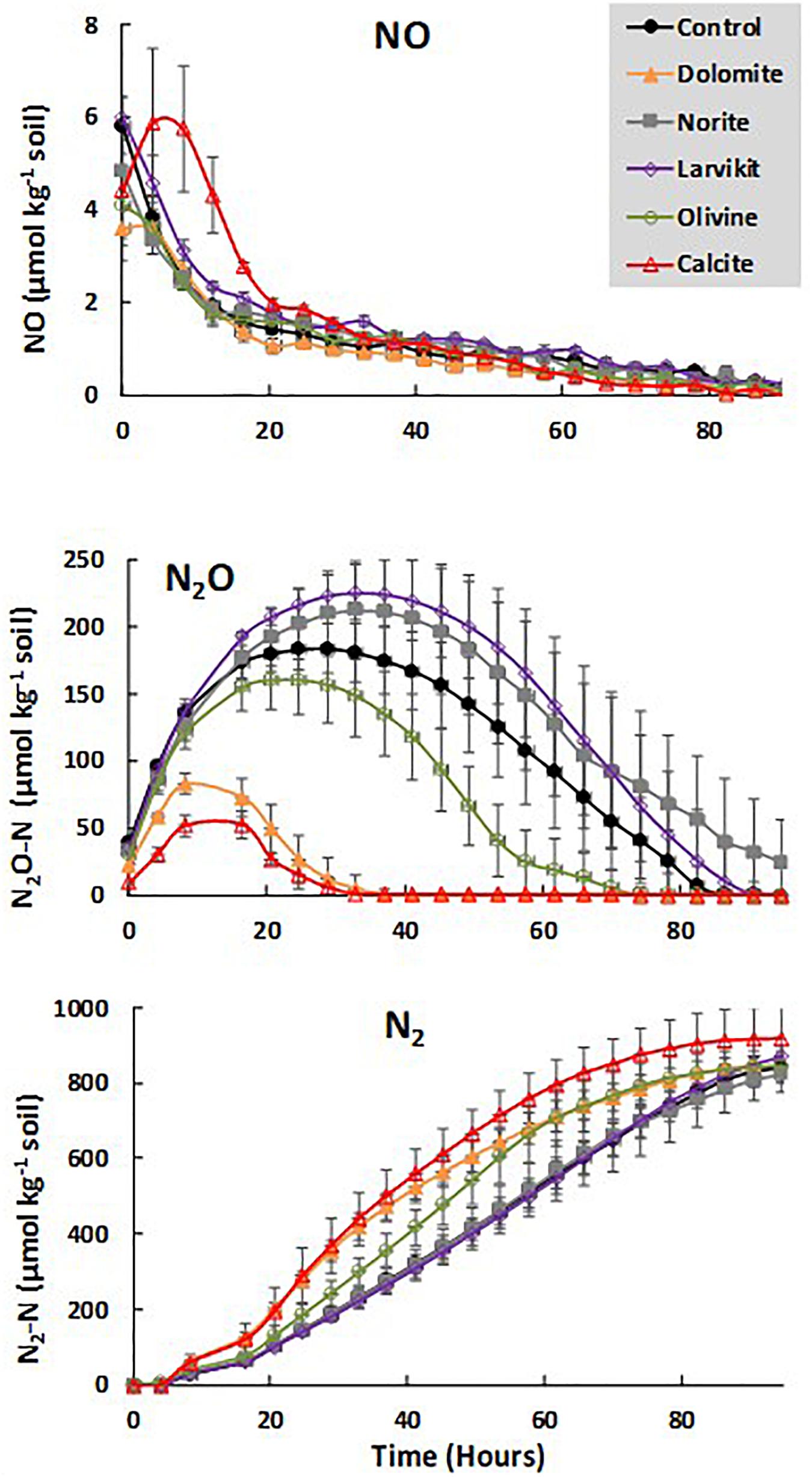
Figure 4. Gas kinetics of denitrification in remolded soil under anoxic conditions. Plotted values are the means and standard error of four field replicates. Soil samples were collected in December 2016 (27 months after application of minerals).
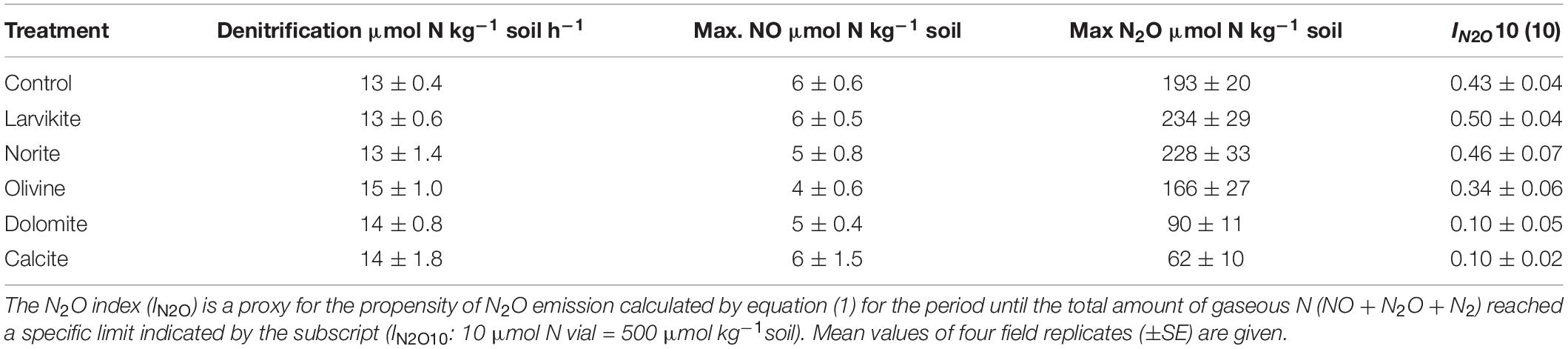
Table 4. Denitrification rate and production of NO and N2O during anoxic incubation of remolded soils amended with nitrate.
Oxic Incubations of Remolded Soils
The O2 consumption rates, NH4+ oxidation rates, maximum NO2– concentrations, and the N2O yields for these incubations are all summarized in Table 5, and the N2O kinetics is shown in Figure 5A. The O2 consumption rate increased gradually with increasing soil moisture content: the average O2 consumption rates (all treatments) were 75, 106, and 153 μmol O2 kg–1 soil h–1 at 60, 70, and 85% WFPS, respectively. The higher O2 consumption rate at 70% than at 60% WFPS likely reflects a higher concentration of available organic carbon: soils used for the 60% WFPS experiments were sampled in December 2015, while the 70 and 85% WFPS experiments used soil samples taken in December 2016, when the ley was fully established. This is confirmed by oxygen consumption rates measured in oxic soil slurries (Table 3): the average rates for the December samples were 230 and 274 μmol O2 kg–1 soil h–1 in 2015 and 2016, respectively, i.e., a 20% increase from 2015 to 2016.
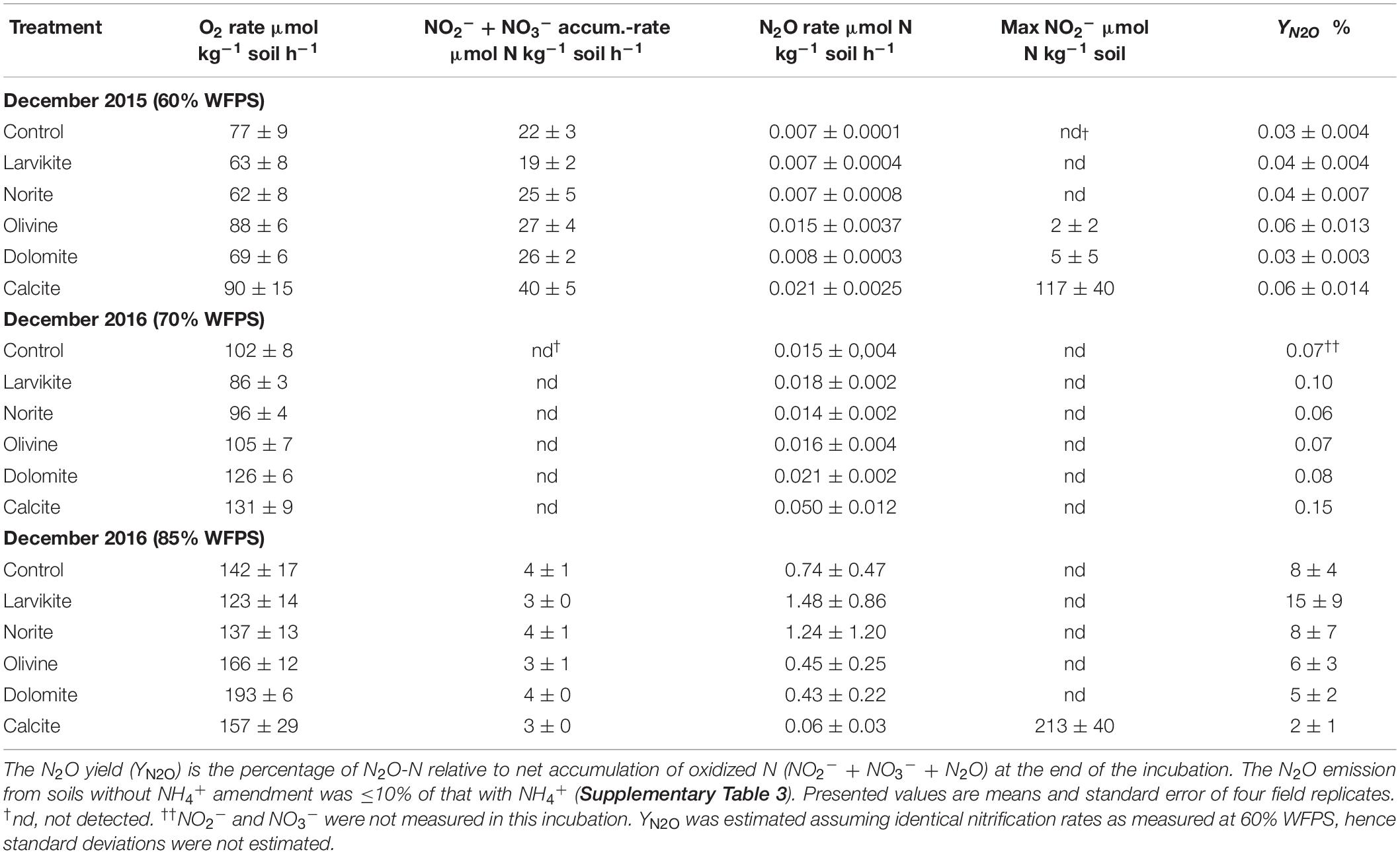
Table 5. Rates of O2 consumption, net NO2– + NO3– accumulation, maximum NO2– accumulation and N2O production rate during oxic incubation of remolded soils amended with ammonium at 60, 70, and 85% WFPS.
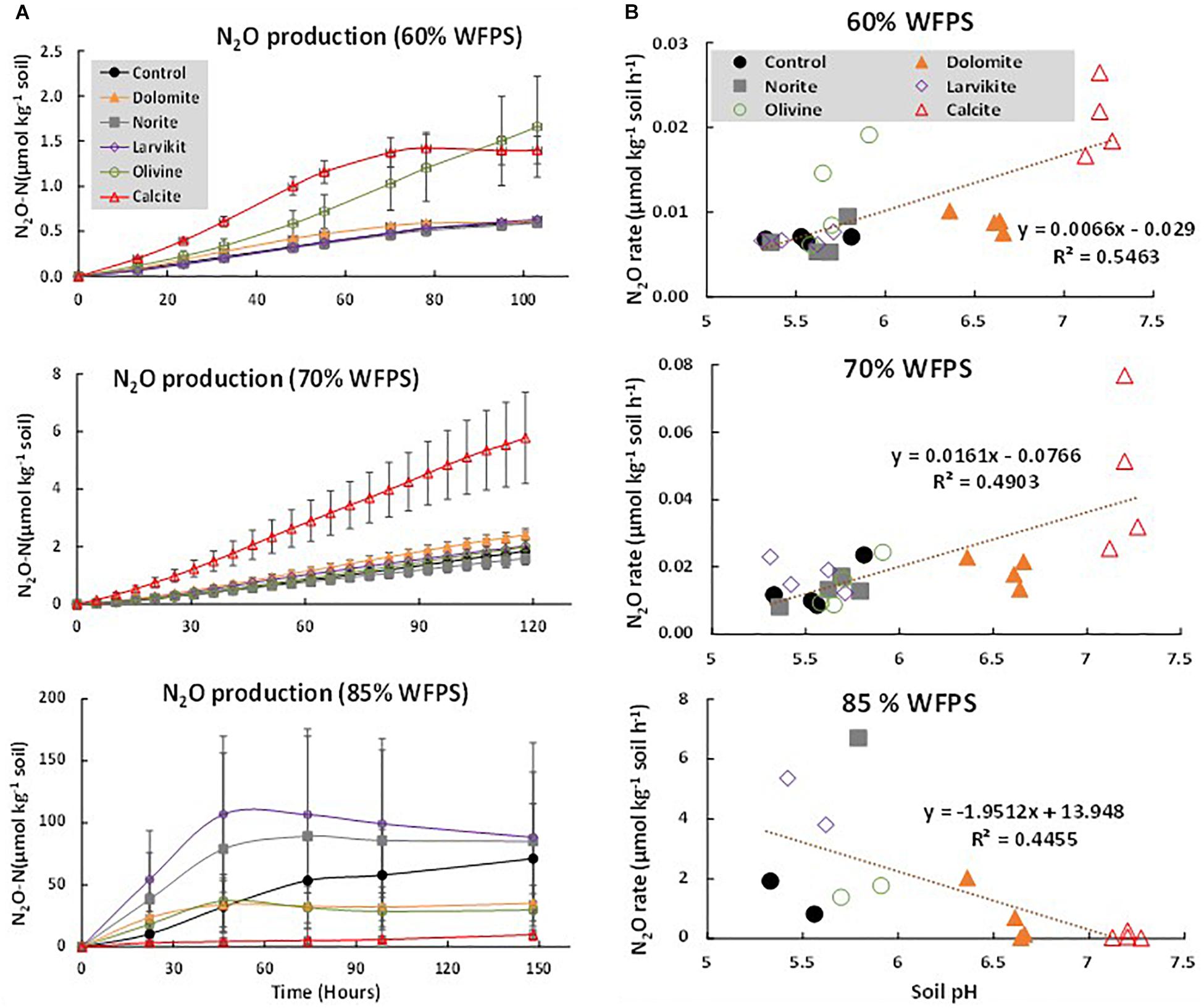
Figure 5. N2O accumulation during oxic incubations of remolded soils amended with NH4+. Panel (A) shows the N2O kinetics throughout the incubations at the three moisture levels (means and standard error, n = 4). Panel (B) shows the N2O production rates during the first 50 h of incubation plotted against the pH of the soils (individual flasks). Soils were collected from the field 15 months after liming (December 2015) for the 60% WFPS experiment and 27 months after liming (December 2016) for the 70 and 85% WFPS experiment. The NO2– + NO3– accumulation throughout the incubations at 60 and 85% WFPS are shown in Supplementary Figure 6.
The percentage of O2 consumption theoretically accounted for by NH3 oxidation (assuming 2 mol O2 consumed per mol NH4+ oxidized) varied between 20 and 40% at 60% WFPS, which is somewhat lower than in the soil slurries. For the 85% WFPS treatment, much lower percentages were calculated, reflecting that NO3– consumption by denitrification resulted in grossly underestimated nitrification rates.
On average, the estimated N2O yield (YN2O) at 70% WFPS was twice as high as at 60% WFPS (0.088 versus 0.043%, Table 5). The YN2O values for 70% WFPS are uncertain, however, since they are based on the assumption that nitrification rates were the same as at 60% WFPS. The 85% WFPS treatment differed markedly from the two other moisture levels in that O2 consumption rates were larger, rates of NO2– + NO3– accumulation were lower, and N2O production rates were two orders of magnitude higher. The apparent N2O yields calculated for the initial 75 h (Table 5) were high, ranging from 2 to 15%, and approximately twice as high if calculated for the first 45 h of incubation, except for calcite, for which the N2O production was low compared to the others, and more constant throughout. The pH response of the N2O production rate in the 85% WFPS treatment differed fundamentally from those of the lower WFPS treatments (Figure 5B): while the N2O production rates increased with pH at 60 and 70% WFPS, they declined sharply at 85% WFPS.
AOA, AOB, and Nitrite Oxidizer Abundances
The relative abundance of AOA-, AOB-, and NOB-SSU in the overall bacterial community, as indicated by SSU rRNA amplicon sequencing, are shown in Figure 6. AOA identified in the samples consisted of 42 ASVs in the phylum Thaumarchaeota including the genera Candidatus Nitrosotalea, Nitrososphaera and Nitrocosmicus, as well as undescribed taxa within the phylum (Supplementary File 1). The identified AOB consisted of 77 ASVs from the family Nitrosomonadaceae within the phylum Proteobacteria and consisted of the genera Nitrosomonas and Nitrosospira, as well as several uncharacterized genera (Supplementary File 1). We also identified SSU rRNA genes affiliated to the phylum Nitrospirae and the genus Nitrospira, which are nitrite oxidizers (Supplementary File 1 and Supplementary Figure 8). Regression analyses showed that the relative abundances of AOB and NOB increased significantly with soil pH (p < 0.001 for both), while AOA declined with pH (p = 0.02), and the AOB/AOA abundance ratio increased with pH (p < 0.001). SSU rRNA identified as Nitrospira may include comammox, i.e., organisms that oxidize ammonium to nitrate (Daims et al., 2015), but the ability of a given Nitrospira to carry out comammox as opposed to only nitrite oxidation may only be discerned by analysis of their functional genes (Daims et al., 2015). Hence, our SSU rRNA analysis cannot discern whether the Nitrospira ASVs detected are simple NOB or comammox.
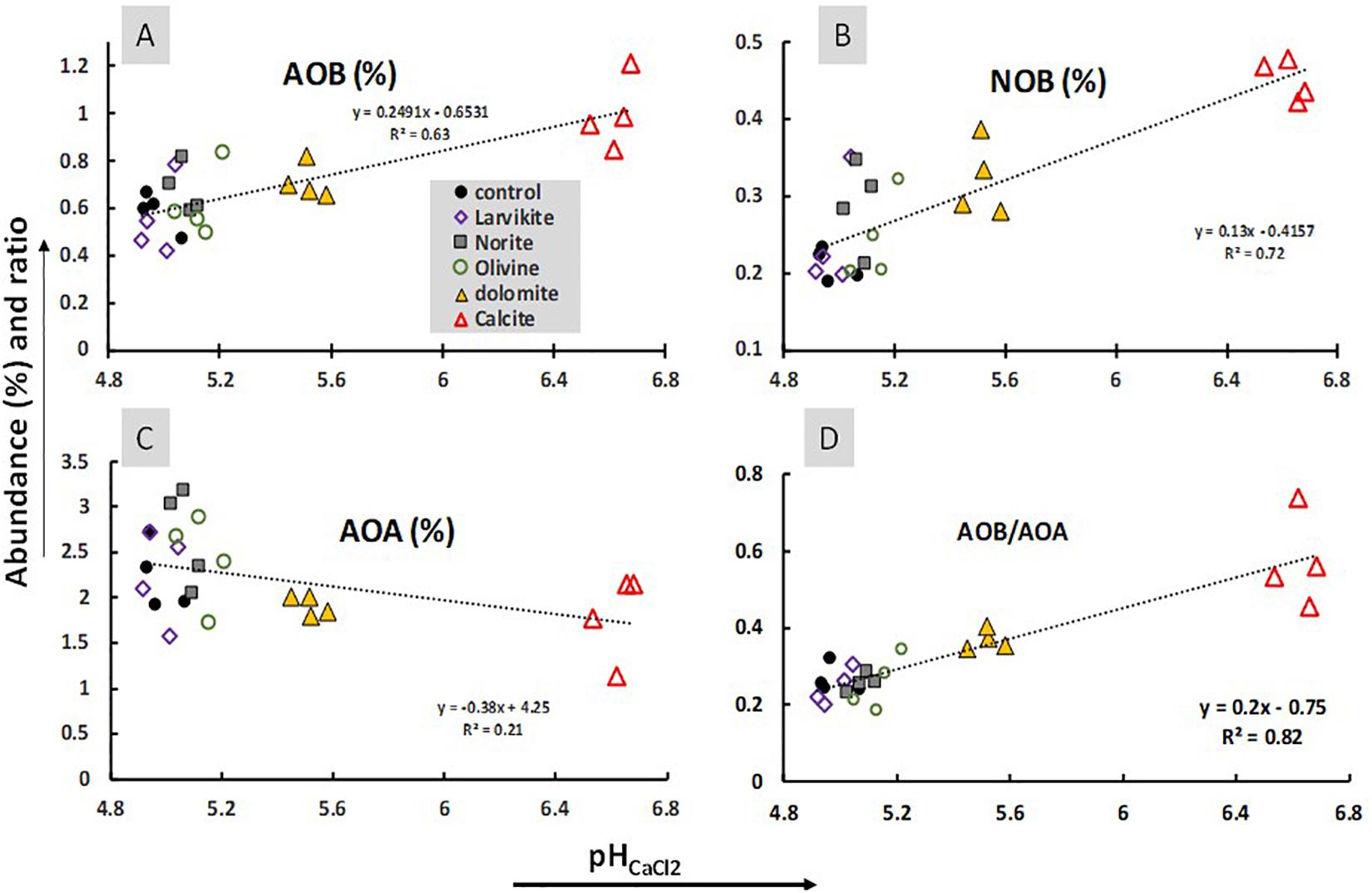
Figure 6. The relative abundance (%) of nitrifying organisms as affected by soil pH. The figures show the relative abundance SSU rRNA copies (%) for AOB (A), NOB (B) and AOA (C), and the ratio between AOB and AOA abundance (D); all plotted against pH measured in 0.01 M CaCl2. Each point represents one individual field plot. The equation for the linear regression is included (all were significant, p < 0.05). The abundance of dominating groups within AOA and AOB are shown in Supplementary Table 4. The N2O yield of nitrification in soil slurries (YN2O. mol N2O-N per mol oxidized NH4+) correlated strongly with the AOB/AOA ratio (Supplementary Figure 7).
Discussion
It has become increasingly clear that the reason why emissions of N2O from soils increase with acidity (Wang et al., 2018) is that the synthesis of functional N2O reductase is impeded by low pH (Liu et al., 2014). Therefore, liming of acidic soil should reduce N2O emissions and would justify the use of high doses of lime/biochar to mitigate these emissions, hence reducing the climate forcing that occurs as a result of fertilizer use in crop production. In theory, this beneficial effect of liming on climate forcing could be outweighed by emission of carbonate-CO2, although the assumption that all carbonate-C is released as CO2 has been contested (Hamilton et al., 2007; Page et al., 2009). Nevertheless, the emission of carbonate-CO2 was our motive for testing finely ground siliceous minerals as alternatives to carbonates. The weathering of siliceous minerals will increase the pH of acidic soils, but our results so far are discouraging: while the carbonate materials caused a substantial increase in soil pH, larvikite had no significant effect, and norite and olivine increased the pH by only 0.11 and 0.16 pH units, respectively (Table 1 and Supplementary Figure 1). Thus, although demonstrating the potential for two of the siliceous minerals, the weathering rates were obviously too low to achieve substantial increase in soil pH within 3 years after application. Improvement could have been achieved by grinding the minerals to finer particle size, since the weathering rate is proportional to the surface area, but the cost would be prohibitively high (van Noort et al., 2018).
N2O emission from denitrification typically occurs during hypoxic/anoxic spells and is caused by transiently high soil moisture, high oxygen consumption rates, or both. The N2O emissions during such “hot events,” or in “hot spots” if localized in microsites with high oxygen consumption rates (Schlüter et al., 2019) depends on the enzyme kinetics: early/efficient synthesis of active N2O reductase secures a low N2O/(N2 + N2O) product ratio, hence minimizing emission of N2O, whereas delayed synthesis will lead to a high N2O product ratio and emission. Since the synthesis of functional N2O reductase is severely impeded/delayed by low pH (Liu et al., 2014), the emission of N2O will decrease with increasing soil pH. Anoxic soil incubations are commonly used to mimic anoxic spells, and such experiments have invariably shown that the transient accumulation of N2O is controlled by pH (Čuhel and Šimek, 2011; Raut et al., 2012; Qu et al., 2014). The present result is no exception: increasing the pH by liming clearly lowered the N2O/(N2O + N2) product ratio during anaerobic incubations of both soil slurries (Figure 1 and Table 2) and remolded soil (Figure 4). Thus, our findings corroborate previous investigations of denitrification as affected by pH. Our main aim, however, was to investigate the effect of liming on both denitrification and nitrification, and their contribution to N2O emission.
Based on Hink et al. (2018) we hypothesized that increasing the pH by using lime or siliceous minerals would increase the potential nitrification rates in the soil, and selectively stimulate bacterial (AOB) over archaeal ammonia oxidizers (AOA). We further hypothesized that the N2O yield of nitrification (YN2O) would increase with soil pH (as observed previously by Mørkved et al., 2007), because AOB have an inherently higher YN2O than AOA (Hink et.al. 2017a). The SSU rRNA gene quantification corroborated the hypothesis regarding selective stimulation of AOB (Figure 6). The effect of soil pH on AOA and AOB abundances has also been studied by Hu et al. (2013), who observed that the qPCR-determined ratio of AOA/AOB decreased with increasing soil pH, and hence the inverse of that ratio, or the AOB/AOA ratio increases, which supports our findings (Figure 6). The AOA taxa included several candidatus genera as well as a number of uncharacterized taxa, suggesting that there remains considerable diversity to be explored among AOA, whereas for the AOB, all the ASVs were from the Nitrosomonadaceae and mostly affiliated with named genera, although most were apparently uncultivated phylotypes such as “mle1-7,” “MND1,” etc. (Supplementary File 1). In addition, the slurry experiments confirmed the effect on nitrification rates and N2O yields (Figure 3). The soil slurry experiments were designed to secure oxic conditions, thus eliminating denitrification as a source of N2O. This is important, because it means that the N2O produced in these experiments largely reflects the inherent N2O production by the ammonia oxidation pathways. A similar increase in abundance of the nitrite-oxidizing Nitrospira (Supplementary Figure 8) with increasing pH was observed by Rousk et al. (2010), although in that study the r2 between pH and Nitrospira relative abundance was 0.16 with P = 0.20.
We further hypothesized that the enhancement of nitrification by liming would increase the risk that the oxygen consumption by nitrification creates hypoxic/anoxic microsites in soil, thus inducing heterotrophic denitrification. This would be aggravated by high soil moisture content (Schurgers et al., 2006). The results with remolded soils shed some light on this (Table 5): at low soil moisture content (60 and 70% WFPS), YN2O was lower than in the soil slurries, but increased with soil pH (as in the soil slurries) thus suggesting that nitrification was the main dominating process. At high soil moisture content (85% WFPS), the apparent YN2O was 2–3 orders of magnitude higher than in the low moisture (60 and 70% WFPS) experiments, plausibly because oxygen consumption by nitrification along with high WFPS resulted in hypoxic/anoxic microsites and hence denitrification. This N2O-production by denitrification could be expected to be promoted by high soil pH because of the higher nitrification rate (providing NO2– and NO3– for denitrification), but the opposite was the case; the N2O production rates clearly declined with soil pH. Our interpretation of this finding is that, while denitrification was induced at all pH levels, the N2O/(N2 + N2O) product ratio declined with increasing soil pH due to more efficient synthesis of functional N2O reductase at high pH (Liu et al., 2014). This necessarily implies that heterotrophic denitrification, rather than nitrifier denitrification must have been dominating, because ammonia oxidizing bacteria lack the gene for N2O-reductase. A recent study of the electron flow to denitrification in ammonia oxidizing bacteria support this interpretation, showing that although the electron flow to nitrite- and nitric oxide reductase in these organisms increased in response to hypoxia, it never amounted to more than 1.2% of the total electron flow (Hink et al., 2017b). This finding lends little support to the common notion that nitrifier denitrification is a strong source of N2O in soils under partially hypoxic conditions (Zhu et al., 2013; Mushinski et al., 2019). As argued elsewhere (Bakken and Frostegård, 2017), the evidence for significant N2O production by nitrifier denitrification is in fact rather weak. The notion emerged as a result of circumstantial evidence (co-occurrence, and selective inhibition of ammonia oxidation), and later by employing 18O/15N tracing to differentiate between N2O from nitrifier denitrification and heterotrophic denitrification (Wrage-Mönning et al., 2018). The differentiation is based on the assumption that nitrite produced by ammonia oxidizers is exclusively reduced by the ammonia oxidizers themselves, because heterotrophs prefer nitrate. This is highly improbable, however: a fraction of the denitrifying heterotrophs carrying nitrite reductase lack nitrate reductase (Lycus et al., 2017), and those with nitrate reductase will reduce external nitrite along with nitrate because nitrite is a free intermediate that is reduced outside the cytoplasmic membrane. This, together with the fact that heterotrophic denitrifiers grossly outnumber ammonia oxidizing organisms, and that nitrifiers allocate miniscule fractions of the electron flow to denitrification (Hink et al., 2017a), suggests the opposite: under hypoxic conditions the nitrite produced by ammonia oxidizers is more likely to be reduced by heterotrophs than by ammonia oxidizers themselves.
Our results are important because they explain why increasing the pH of acidic soils (by lime or biochar) has a contingent effect on their N2O emission. Highly variable effects may be expected for systems/conditions where N2O emission is driven by high ammonium oxidation rates such as urine patches (Clough et al., 2004; Carter, 2007; Khan et al., 2011), while consistent reduction of the N2O emission can be expected for systems/conditions where heterotrophic denitrification is dominant. Laboratory experiments may be designed to isolate nitrification- and denitrification driven N2O emissions, but in agronomically realistic field experiments, the contributions of nitrification and denitrification merge and fluctuate (Russenes et al., 2016), being further modulated by a plethora of other factors (Butterbach-Bahl et al., 2013; Saggar et al., 2013; Rochette et al., 2018). As a result, the net effect of pH management on N2O emissions in single field experiments is to some extent anecdotic, and the evaluation of pH management as a mitigation option should be based on ensembles of well-designed field experiments. Unfortunately, most of the existing emission data are from field experiments designed to test other factors than pH. Nevertheless, a recent meta-study by Wang et al. (2018), which used emission data from 117 studies worldwide, demonstrated an overall reduction of N2O emission by increasing soil pH, creating a basis for recommending liming for mitigating N2O emissions from acidified soils.
Data Availability Statement
The datasets generated for this study can be found in online repositories. The names of the repository/repositories and accession number(s) can be found in the article/Supplementary Material.
Author Contributions
SN: experimental design and writing, did the laboratory work, and analyses of kinetics. LB: experimental design and writing. ÅF: molecular analyses and writing. JG: bioinformatic analyses and writing. PD: experimental design and writing. All authors contributed to the article and approved the submitted version.
Funding
This research was financed by the Norwegian Research Council; project number 234382 (MIGMIN). PD received funding from the FACCE-ERA-GAS project MAGGE-pH under the Grant Agreement No. 696356.
Conflict of Interest
The authors declare that the research was conducted in the absence of any commercial or financial relationships that could be construed as a potential conflict of interest.
Acknowledgments
We thank Trygve Fredriksen for support during the field sampling, and Natalie Y. N. Lim for conducting the DNA extractions.
Supplementary Material
The Supplementary Material for this article can be found online at: https://www.frontiersin.org/articles/10.3389/fenvs.2020.598513/full#supplementary-material
Footnotes
References
Apprill, A., Mcnally, S., Parsons, R., and Weber, L. (2015). Minor revision to V4 region SSU rRNA 806R gene primer greatly increases detection of SAR11 bacterioplankton. Aquat. Microb. Ecol. 75, 129–137. doi: 10.3354/ame01753
Bakken, L. R., and Frostegård, Å (2017). Sources and sinks for N2O, can microbiologist help to mitigate N2O emissions? Environ. Microbiol. 19, 4801–4805. doi: 10.1111/1462-2920.13978
Blomquist, J., Simonsson, M., Etana, A., and Berglund, K. (2018). Structure liming enhances aggregate stability and gives varying crop responses on clayey soils. Acta Agric. Scand. B Soil Plant Sci. 68, 311–322. doi: 10.1080/09064710.2017.1400096
Butterbach-Bahl, K., Baggs, E. M., Dannemann, M., Kiese, R., and Zechmeister-Boltenstern, S. (2013). Nitrous oxide emissions from soils: how well do we understand the processes and controls? Philos. Trans. R. Soc. B 368:20130122. doi: 10.1098/rstb.2013.0122
Callahan, B. J., McMurdie, P. J., Rosen, M. J., Han, A. W., Johnson, A. J., and Holmes, S. P. (2016). DADA2: High-resolution sample inference from Illumina amplicon data. Nat. Methods 13, 581–583. doi: 10.1038/nmeth.3869
Carter, M. S. (2007). Contribution of nitrification and denitrification to N2O emissions from urine patches. Soil Biol. Biochem. 39, 2091–2102. doi: 10.1016/j.soilbio.2007.03.013
Cayuela, M. L., Zwieten, L., Singh, B. P., Jeffery, S., Roig, A., and Sanchez-Mondero, M. A. (2014). Biochar’s role in mitigating soil nitrous oxide emissions: a review and metaanalysis. Agric. Ecosyst. Environ. 191, 5–16. doi: 10.1016/j.agee.2013.10.009
Chadwick, O. A., and Chorover, J. (2001). The chemistry of pedogenic thresholds. Geoderma 100, 321–353. doi: 10.1016/s0016-7061(01)00027-1
Clough, T. C., Kelliher, F. M., Sherlock, R. R., and Ford, C. D. (2004). Lime and soil moisture effects on nitrous oxide emissions from a urine patch. Soil Soc. Am. J. 68, 1600–1609. doi: 10.2136/sssaj2004.1600
Čuhel, J., and Šimek, M. (2011). Proximal and distal control by pH of denitrification rate in a pasture soil. Agric. Ecosyst. Environ. 141, 230–233. doi: 10.1016/j.agee.2011.02.016
Daims, H., Lebedeva, E. V., Pjevac, P., Han, P., Herbold, C., Albertsen, M., et al. (2015). Complete nitrification by Nitrospira bacteria. Nature 528, 504–509. doi: 10.1038/nature16461
Doane, T. A., and Horwath, W. R. (2003). Spectrophotometric determination of nitrate with a single reagent. Anal. Lett. 36, 2713–2722. doi: 10.1081/al-120024647
Goulding, K. W. T. (2016). Soil acidification and the importance of liming agricultural soils with particular reference to the United Kingdom. Soil Use Manag. 32, 390–399. doi: 10.1111/sum.12270
Guo, J., Liu, X., Zhang, Y., Shen, J., Han, W., Zhang, W., et al. (2010). Significant acidification in major Chinese croplands. Science 327, 1008–1010. doi: 10.1126/science.1182570
Hamilton, S. K., Kurzman, A. L., Arango, C., Jin, L., and Robertson, G. P. (2007). Evidence for carbon sequestration by agricultural liming. Glob. Biogeochem. Cycles 21:GB2021. doi: 10.1029/2006GB002738
Hansen, V. T., and Grimenes, A. A. (2015). Meteorologisk Data for Ås 2014. Oslo: Norwegian Meteorological Institute.
Hink, L., Gubry-Rangin, C., Nicol, G. W., and Prosser, J. I. (2018). The consequences of niche and physiological differentiation of archaeal and bacterial ammonia oxidizers for nitrous oxide emissions. ISME J. 12, 1048–1093.
Hink, L., Lycus, P., Gubry-Rangin, C., Frostegård, Å, Nicol, G. W., Prosser, J. I., et al. (2017b). Kinetics of NH3-oxidation, NO-turnover, N2O production and electron flow during oxygen depletion in model bacterial and archaeal ammonia oxidisers. Environ. Microbiol. 19, 4882–4896. doi: 10.1111/1462-2920.13914
Hink, L., Nicol, G. W., and Prosser, J. I. (2017a). Archaea produce lower yields of N2O than bacteria during aerobic ammonia oxidation in soil. Environ. Microbiol. 19, 4829–4837. doi: 10.1111/1462-2920.13282
Hu, H. W., Zhang, L. M., Dai, Y., Di, H. J., and He, J. Z. (2013). pH-dependent distribution of soil ammonia oxidizers across a large geographical scale as revealed by high-throughput pyrosequencing. J. Soils Sediments 13, 1439–1449. doi: 10.1007/s11368-013-0726-y
Huang, T., Gao, B., Hu, X. K., Lu, X., Well, R., Christie, P., et al. (2014). Ammonia-oxidation as an engine to generate nitrous oxide in an intensively managed calcareous Fluvo-aquic soil. Sci. Rep. 4:3950. doi: 10.1038/srep03950
Keeney, D. R., and Nelson, D. W. (1982). “Nitrogen inorganic forms,” in Methods of Soil Analysis, Part 2- Chemical and Microbiological Properties, eds A. L. Page, R. H. Miller, and D. R. Keeney (Madison, WI: American Society of Agronomy Inc), 643–698. doi: 10.2134/agronmonogr9.2.2ed.c33
Khan, S., Clough, T. J., Goh, K. M., and Sherlock, R. R. (2011). Influence of soil pH on NOx and N2O emissions from bovine urine applied to soil columns. New Zeal. J. Agric. Res. 54, 285–301. doi: 10.1080/00288233.2011.607831
Klotz, M. G., and Stein, L. Y. (2011). “Genomics of ammonia−oxidizing bacteria and insights into their evolution,” in Nitrification, eds B. B. Ward, D. J. Arp, and M. G. Klotz (Washington, DC: ASM Press), doi: 10.1128/9781555817145.ch4
Kochian, L. V., Hoekenga, A., and Pineros, M. A. (2004). How do crop plants tolerate acid soils? Mechanisms of aluminum tolerance and phosphorous efficiency. Annu. Rev. Plant Biol. 55, 459–493. doi: 10.1146/annurev.arplant.55.031903.141655
Kool, D. M., Dolfing, J., Wrage, N., and Van Groeningen, J. W. (2011b). Nitrifier denitrification as a distinct and significant source of nitrous oxide from soil. Soil Biol. Biochem. 43, 174–178. doi: 10.1016/j.soilbio.2010.09.030
Kool, D. M., van Groenigen, J. W., and Wrage, N. (2011a). Source determination of nitrous oxide based on nitrogen and oxygen isotope tracing: dealing with oxygen exchange. Methods Enzymol. 496, 139–160. doi: 10.1016/b978-0-12-386489-5.00006-3
Lim, N. Y. N., Roco, A. C., and Frostegård, Å (2016). Transparent DNA/RNA co-extraction workflow protocol suitable for inhibitor-rich environmental samples that focuses on complete DNA removal for transcriptomic analyses. Front. Microbiol. 7:1588. doi: 10.3389/fmicb.2016.01588
Liu, B., Frostegård, Å, and Bakken, L. R. (2014). Impaired reduction of N2O to N2 in acid soil is due to a post-transcriptional interference with the expression of nosZ. mBio 5:e01383-14. doi: 10.1128/mBio.01383-14
Liu, B., Mørkved, P. T., Frostegård, Å, and Bakken, L. R. (2010). Denitrification gene pools, transcription and kinetics of NO, N2O and N2 production as affected by soil pH. FEMS Microbiol. Ecol. 72, 407–417. doi: 10.1111/j.1574-6941.2010.00856.x
Lycus, P., Bøthun, K. L., Bergaust, L., Shapleigh, J. P., Bakken, L. R., Frostegård, Å., et al. (2017). Phenotypic and genotypic richness of denitrifiers revealed by a novel isolation strategy. ISME J. 11, 2219–2232. doi: 10.1038/ismej.2017.82
McMurdie, P. J., and Holmes, S. (2013). Phyloseq: an R package for reproducible interactive analysis and graphics of microbiome census data. PLoS One 8:e61217. doi: 10.1371/journal.pone.0061217
Molstad, L., Dörsch, P., and Bakken, L. R. (2007). Robotized incubation system for monitoring gases (O2, NO, N2O and N2) in denitrifying cultures. J. Microbiol. Methods 71, 202–211. doi: 10.1016/j.mimet.2007.08.011
Molstad, L., Dörsch, P., and Bakken, L. R. (2016). Report: New Improved Robot for Gas Kinetics in Batch Cultures. Berlin: Research Gate, doi: 10.13140/RG.2.2.30688.07680
Mørkved, P. T., Dörsch, P., and Bakken, L. R. (2007). The N2O product ratio of nitrification and its dependence on long-term changes in soil pH. Soil Biol. Biochem. 39, 2048–2057. doi: 10.1016/j.soilbio.2007.03.006
Mushinski, R. M., Phillips, R. P., Payne, Z. C., Avney, R. B., Jo, I., Fei, S., et al. (2019). Microbial mechanisms and ecosystem flux estimation for aerobic NOy emissions from deciduous forest soils. Proc. Natl. Acad. Sci. U.S.A. 116, 2138–2145. doi: 10.1073/pnas.1814632116
Nadeem, S., Bakken, L. R., Köster, J. R., Mørkved, P. T., Simon, N., and Dörsch, P. (2015). Soil pH management without lime, a strategy to reduce greenhouse gas emissions from cultivated soils. Geophys. Res. Abstracts 17, EGU2015–EGU9790.
Nicol, G. W., Leininger, S., Schleper, C., and Prosser, J. I. (2008). The influence of soil pH on the diversity, abundance and transcriptional activity of ammonia oxidizing archaea and bacteria. Environ. Microbiol. 10, 2966–2978. doi: 10.1111/j.1462-2920.2008.01701.x
Oo, A. Z., Sudo, S., Akiyama, H., Win, K. T., Shibata, A., Yamamoto, A., et al. (2018). Effect of dolomite and biochar addition on N2O and CO2 emissions from acidic tea field soil. PLoS One 13:e0192235. doi: 10.1371/journal.pone.0192235
Page, K. L., Allen, D. E., Dalal, R. C., and Slattery, W. (2009). Processes and magnitude of CO2, CH4, and N2O fluxes from liming of Australian acidic soils: a review. Soil Res. 47, 747–762. doi: 10.1071/sr09057
Parada, A. E., Needham, D. M., and Fuhrman, J. A. (2016). Every base matters: Assessing small subunit rRNA primers for marine microbiomes with mock communities, time series and global field samples. Environ. Microbiol. 18, 1403–1414. doi: 10.1111/1462-2920.13023
Qu, Z., Wang, J., Almøy, T., and Bakken, L. R. (2014). Excessive use of nitrogen in Chinese agriculture results in high N2O/(N2O+ N2) product ratio of denitrification, primarily due to acidification of the soils. Glob. Change Biol. 20, 1685–1698. doi: 10.1111/gcb.12461
Raut, N., Dörsch, P., Sitaula, B. K., and Bakken, L. R. (2012). Soil acidification by intensified crop production in South Asia results in higher N2O/(N2 + N2O) product ratios of denitrification. Soil Biol. Biochem. 55, 104–112. doi: 10.1016/j.soilbio.2012.06.011
Rochette, P., Liang, C., Pelster, D., Bergeron, O., Lemke, R., Kroebel, R., et al. (2018). Soil nitrous oxide emissions from agricultural soils in Canada: exploring relationships with soil, crop and climate variables. Agric. Ecosyst. Environ. 254, 69–81. doi: 10.1016/j.agee.2017.10.021
Rousk, J., Bååth, E., Brookes, P. C., Lauber, C. L., Lozupone, C., Caporaso, J. G., et al. (2010). Soil bacterial and fungal communities across a pH gradient in an arable soil. ISME J. 4, 1340–1351. doi: 10.1038/ismej.2010.58
Russenes, A. L., Korsaeth, A., Bakken, L. R., and Dörsch, P. (2016). Spatial variation in soil pH controls off-season N2O emission in an agricultural soil. Soil Biol. Biochem. 99, 36–46. doi: 10.1016/j.soilbio.2016.04.019
Saggar, S., Jha, N., Deslippe, J., Bolan, N. S., Luo, J., Giltrap, D. L., et al. (2013). Denitrification and N2O:N2 production in temperate grasslands: processes, measurements, modelling and mitigating negative impacts. Sci. Total Environ. 465, 173–195. doi: 10.1016/j.scitotenv.2012.11.050
Schlüter, S., Zawallich, J., Vogel, H. J., and Dörsch, P. (2019). Physical constraints for respiration in microbial hotspots in soil and their importance for denitrification. Biogeosciences 16, 3665–3678. doi: 10.5194/bg-2019-2
Schurgers, G., Dörsch, P., Bakken, L. R., Leffelaar, P., and Haugen, L. E. (2006). Modelling soil anaerobiosis from water retention characteristics and soil respiration. Soil Biol. Biochem. 39, 2637–2644. doi: 10.1016/j.soilbio.2006.04.016
Shaaban, M., Peng, Q., Hu, R., Wu, Y., Lin, S., and Zhao, J. (2015). Dolomite application to acidic soils: a promising option for mitigating N2O emissions. Environ. Sci. Pollut. Res. 22, 19961–19970. doi: 10.1007/s11356-015-5238-4
Shaaban, M., Peng, Q., Lin, S., Wu, Y., Zhao, J., and Hu, R. (2014). Nitrous oxide emission from two acidic soils as affected by dolomite application. Soil Res. 52, 841–848. doi: 10.1071/sr14129
Shaaban, M., Wu, Y., Khalid, M. S., Peng, Q., Xu, X., Wu, L., et al. (2018). Reduction in soil N2O emissions by pH manipulation and enhanced nosZ gene transcription under different water regimes. Environ. Pollut. 235, 625–631. doi: 10.1016/j.envpol.2017.12.066
Ulén, B., and Etana, A. (2014). Phosphorus leaching from clay soils can be counteracted by structure liming. Acta Agric. Scand. B Soil Plant Sci. 64, 425–433. doi: 10.1080/09064710.2014.920043
van Noort, R., Mørkved, P. T., and Dundas, S. H. (2018). Acid neutralization by mining waste dissolution under conditions relevant for agricultural applications. Geosciences 8:380. doi: 10.3390/geosciences8100380
von Uexküll, H. R., and Mutert, E. (1995). Global extent, development and economic impact of acid soils. Plant Soil 171, 1–15. doi: 10.1007/bf00009558
Wang, Y., Guo, J., Vogt, R. D., Mulder, J., Wang, J., and Zhang, X. (2018). Soil pH as the chief modifier for regional nitrous oxide emissions: new evidence and implications for global estimates and mitigation. Glob. Change Biol. 24, e617–e626.
White, P. F., and Robson, A. D. (1989). Rhizosphere acidification and Fe3+ reduction in lupins and peas: Iron deficiency in lupins is not due to a poor ability to reduce Fe3+. Plant and Soil 119, 163–175. doi: 10.1007/BF02370281
Wijler, J., and Delwiche, C. C. (1954). Investigations on the denitrifying process in soil. Plant Soil 5, 155–169. doi: 10.1007/bf01343848
Wrage-Mönning, N., Horn, M., Well, R., Müller, C., Velthof, G., and Oenema, O. (2018). The role of nitrifier denitrification in the production of nitrous oxide revisited. Soil Biol. Biochem. 123, A3–A16.
Yilmaz, P., Parfrey, L. W., Yarza, P., Gerken, J., Pruesse, E., Quast, C., et al. (2014). The SILVA and “all-species living tree project (LTP)” taxonomic frameworks. Nucleic Acids Res. 42, D643–D648. doi: 10.1093/nar/gkt1209
Keywords: soil pH, nitrification, N2O-reductase, N2O, moisture
Citation: Nadeem S, Bakken LR, Frostegård Å, Gaby JC and Dörsch P (2020) Contingent Effects of Liming on N2O-Emissions Driven by Autotrophic Nitrification. Front. Environ. Sci. 8:598513. doi: 10.3389/fenvs.2020.598513
Received: 24 August 2020; Accepted: 11 November 2020;
Published: 04 December 2020.
Edited by:
Muhammad Shaaban, Bahauddin Zakariya University, PakistanReviewed by:
Alessandro Florio, Institut National de Recherche pour l’Agriculture, l’alimentation et l’Environnement (INRAE), FranceMeihua Deng, ZheJiang Academy of Agricultural Sciences, China
Copyright © 2020 Nadeem, Bakken, Frostegård, Gaby and Dörsch. This is an open-access article distributed under the terms of the Creative Commons Attribution License (CC BY). The use, distribution or reproduction in other forums is permitted, provided the original author(s) and the copyright owner(s) are credited and that the original publication in this journal is cited, in accordance with accepted academic practice. No use, distribution or reproduction is permitted which does not comply with these terms.
*Correspondence: Lars R. Bakken, bGFycy5iYWtrZW5Abm1idS5ubw==