- 1Risk and Vulnerability Science Center, University of Limpopo, Sovenga, South Africa
- 2Communities Living Among Wildlife Sustainably (CLAWS) Botswana, Seronga, Botswana
- 3School of Agriculture and Environmental Sciences, University of Limpopo, Sovenga, South Africa
- 4Institute of Crop Science and Resource Conservation, University of Bonn, Bonn, Germany
- 5Working Group (WG) Biodiversity/Systematic Botany, Institute of Biochemistry and Biology, University of Potsdam, Potsdam, Germany
Grasslands cover ca. 30% of the global land surface and provide critical ecosystem services. Among them, carbon storage is one of the most important. However, grasslands are increasingly threatened by drought and overgrazing which might negatively affect soil carbon stocks. Despite this threat, there is a dearth of information on how drought and grazing jointly impact soil carbon stocks and CO2 fluxes in dryland grasslands. With the aid of a large field experiment, we studied the combined effects of a 5-year extreme drought and moderate grazing on soil carbon stocks, CO2 fluxes and soil chemical properties. Extreme drought was induced by reducing ambient rainfall by 66% using large rainout shelters. We found CO2 fluxes to strongly respond to the 5-year experimental drought. Extreme drought reduced CO2 emission rates by 32% compared to ambient conditions. CO2 fluxes averaged 5.7 mg m–2min–1 under drought compared to 8.3 mg m–2 min–1 under ambient conditions. CO2 fluxes were, however, not influenced by grazing. At the end of the growth period, grazed plots under ambient rainfall had released 16.3 tons of CO2 ha–1 which was 58% higher than observed on grazed plots subjected to severe drought. Soil carbon stocks were higher under drought conditions due to slower decomposition rates. Drought resulted in increased concentrations of primary macronutrients (N, P, and K), micronutrients (Zn and Mn) and pH in the top 30 cm of the soil relative to ambient conditions. The results also showed that grazing reduced the concentration of N and P in the topsoil compared to the ungrazed plots. This study provided insights on the soil carbon storage, CO2 emission rates and nutrient dynamics in a semi-arid dryland grassland as influenced by both drought and grazing. Our study also revealed that long-term extreme drought may be favorable in terms of preserving the existing soil carbon stocks through reduced CO2 release. This finding is critical for understanding future soil carbon dynamics in dryland grasslands in the face of climate change.
Introduction
Grasslands are the largest terrestrial biome, covering up to 30% of the global land surface (Suttie et al., 2005). They are also a large reservoir of organic carbon, storing 10–30% of the global soil organic carbon (Zhou et al., 2019a). Despite their low productivity, dryland grasslands play an important role in global carbon sequestration as decomposition rates are generally low due to lack of water. It is estimated that about 32% of the world’s soil organic carbon is stored in dryland soils (Plaza et al., 2018; Gaitán et al., 2019). In dryland grasslands, grazing and variable rainfall are among the major determinants of grassland productivity (Bat-Oyun et al., 2016; Guuroh et al., 2018). In recent years, the frequency and intensity of drought have increased considerably in global drylands, while overgrazing continues to be a problem (IPCC, 2019). This is also the case in drylands situated in Sub-Saharan Africa, SSA. Combined effects of grazing and drought can have particularly strongly impacts on the ecosystem functions and services delivered by these ecosystems (Ruppert et al., 2015; Pfeiffer et al., 2019).
The decomposition of the large soil organic matter pools could be a major source of CO2 emission into the atmosphere, thus providing a potentially large climate change feedback and a major source of uncertainty in climate projections (Pries et al., 2017). Due to the large size of soil carbon pools in dryland grasslands (Plaza et al., 2018) and due to the uncertainty of emission capacity of these ecosystems, it is critical to carry out precise quantifications of carbon stocks and emissions. This would not only help to obtain reliable global carbon budgets but to also develop mitigation options for low-emission through proper land use management practices (Berger et al., 2019). The quantification of grassland carbon (C) fluxes is also required to identify major control factors and to clarify whether specific grassland ecosystems sequester more atmospheric CO2 through photosynthesis than they emit to the atmosphere through respiration (Lefèvre et al., 2017). Currently, there is limited empirical carbon storage and emission data available from natural ecosystems, particularly in SSA. This is despite reports that CO2 emissions are the largest contributor to greenhouse gas (GHG) emissions and global warming potential in SSA natural terrestrial systems (Kim et al., 2016).
Grazing and drought both have strong effects on soil carbon storage and GHG emission in dryland grasslands, mainly indirectly through their effect on biomass production (Jones et al., 2005; Ferner et al., 2018; Gaitán et al., 2019). Among these two drivers, drought has been reported to have a greater influence on biomass production than grazing (Bat-Oyun et al., 2016). It is well documented that precipitation promotes plant growth which in turn leads to increased CO2 assimilation (Berger et al., 2019). However, that same precipitation that promotes CO2 uptake also stimulates its emission through increased respiration (Xu et al., 2004). Accordingly, the lack of precipitation during a drought may suppress both CO2 assimilation and emission due to constrained plant growth and reduced soil respiration. Grazing on the other hand, can impact carbon storage and emission by altering the species composition of the vegetation, and via a reduction of aboveground biomass from livestock feeding (Chen et al., 2017). In overgrazed dryland grasslands, annual grass species tend to dominate relative to perennial species (Linstädter et al., 2014; Mudongo et al., 2016). Annual grasses are known to invest more on aboveground biomass and less on belowground biomass, leading to low root respiration (Bat-Oyun et al., 2016). Chen et al. (2017) reported a reduction of between 25 and 47% in CO2 emission due to grazing in different rangeland types.
Although grazing mainly affects soil carbon storage emission via its imprint on vegetation structure and composition, grazing may also influence these variables through its impact on soil properties (Sandhage-Hofmann et al., 2015; Linstädter et al., 2016). Though not well understood, overgrazing has the potential to directly alter soil properties and processes. For example, trampling by livestock can cause soil compaction leading to reduced soil water infiltration and, consequently, reduced moisture availability to plants and microbes (Bilotta et al., 2007; Savadogo et al., 2007; Batey, 2009). Such changes in soil properties can also affect soil organic matter build-up, because processes such as decomposition and root growth are hindered (Okach et al., 2019a). Nonetheless, the effect of grazing on carbon content has not been conclusive. For instance, two studies conducted in a typical steppe Mongolia showed opposite responses: While Liu et al. (2012) reported higher soil organic carbon Wang B. et al. (2020) found decreases in soil organic carbon due to grazing. On the other hand, grazing may actually promote soil aeration through the breaking of soil caps. Livestock also adds dung to the soil, thus increasing soil organic carbon content (Sandhage-Hofmann et al., 2015) and promoting plant growth. In addition, grazing and drought both have the potential to alter soil chemical properties, which in turn might also influence carbon storage and emission. However, we still know very little how long-term drought and grazing jointly affect soil chemical properties (see Lucci, 2019). The only experimental study we are aware of Okach et al. (2019a) implemented a short-term drought, and mainly focused on soil physical properties such as bulk density and hydraulic properties, and on the commonly reported C and N.
In summary, there is a need not only for empirically measured C storage and emissions data in dryland grasslands, but also for a better understanding of how grazing and drought jointly affect soil organic carbon (Lucci, 2019). Accordingly, this study aims to disentangle joint effects of long-term drought and grazing on soil carbon stocks, CO2 fluxes, and soil chemical properties, focusing on a semi-arid African savanna grassland. We hypothesized that (1) both long-term drought and moderate grazing reduce C storage leading to slower CO2 emission rates, and that (2) soil chemical properties are interactively influenced by drought and grazing.
Materials and Methods
Study Site
The study was conducted at the University of Limpopo’s Syferkuil experimental farm, Limpopo Province, South Africa (Figure 1). The climate is classified as arid steppe hot climate (Kottek et al., 2006) which receives annual average rainfall ranging from 400 to 600 mm (Benhin, 2006). Average minimum and maximum temperatures are 4–20°C in winter and 17–27°C in summer. In the 2018/19 rainy season, a total of 352 mm of rain fall was received from September 2018 to April 2019. Daily temperature, rainfall and other weather date were recorded from an Agricultural Research Council automatic weather station installed at the experimental farm and was about 2 km from the drought act experiment.
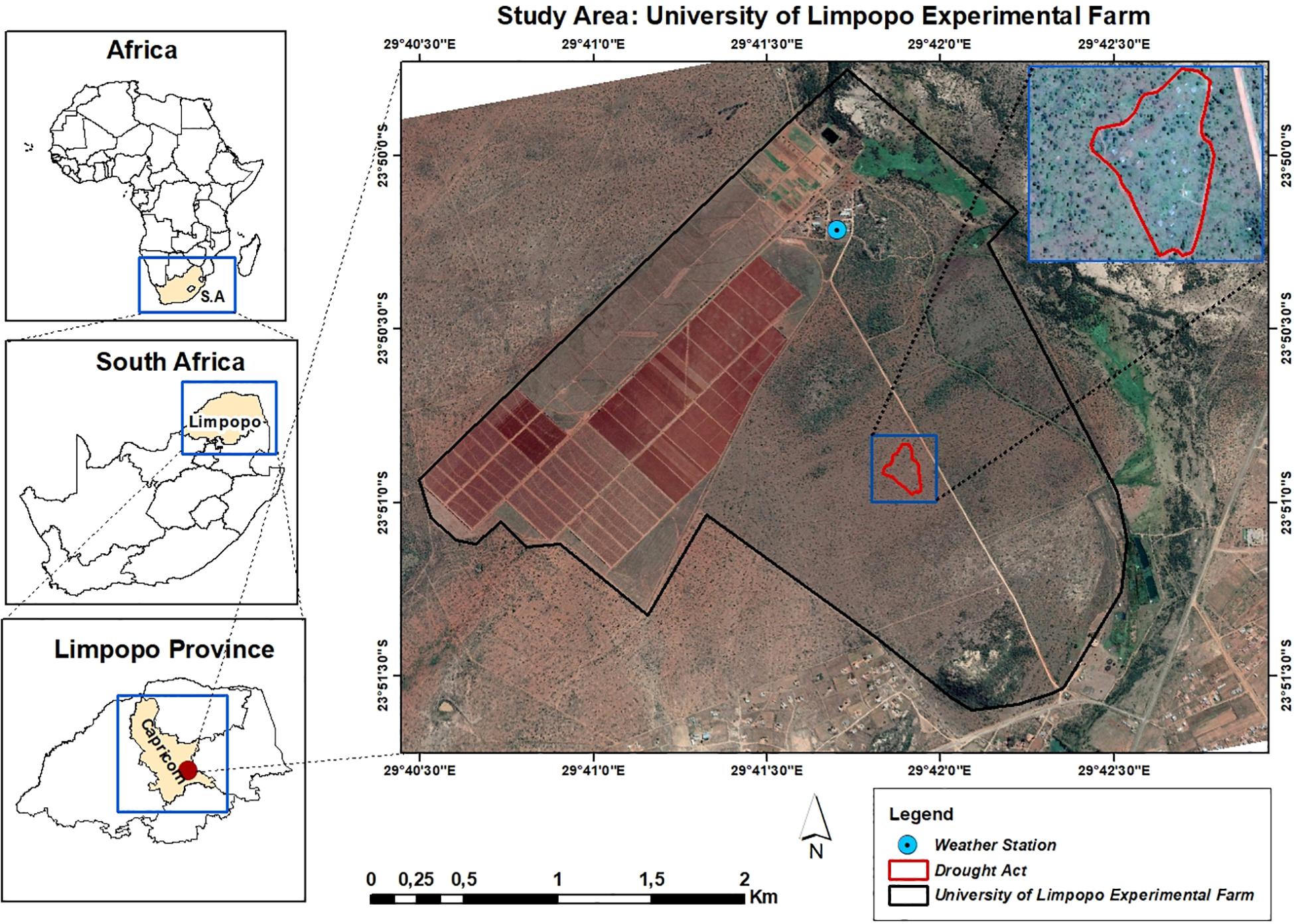
Figure 1. Location of the DroughtAct experiment at the University of Limpopo Experimental Farm in South Africa’s Limpopo Province.
The soils are mainly shallow, reddish-brown loamy sand soils, underlain by soft plinthic B horizon and classified as Plinthisols (WRB, 2014; Dlamini et al., 2019). The vegetation has been described as an open thorn bush savanna belonging to the Pietersburg Plateau False Grassveld type (Acocks, 1994). The herbaceous layer is dominated by perennial C4 grasses such as Themeda triandra Forssk., Digitaria eriantha Steud., Schmidtia pappophoroides Steud. ex J.A.Schmidt, and Eragrostis spp., while the woody component is dominated by Vachellia tortilis (Forssk.) Galasso & Banfi (Low and Rebelo, 1998).
The camp (i.e., paddock) on which the experiment was implemented has a size of about 40 ha. It is part of a rotational camp system that is moderately grazed at a stocking density of 9 ha LSU–1 where a livestock unit (LSU) is equivalent to a mature cow with a bodyweight of 450 kg (Meissner, 1983). Grazing periods did not exceed 30 days, with intermittent recovery of not less than 6 weeks during the growing season (8 weeks during the dry season, respectively). The rotational management was established 6 years prior to the set-up of the experiment, resulting in good rangeland condition at the onset of treatments.
Experimental Design
The experiment “DroughtAct” combines a severe drought treatment with a resting treatment. A full factorial experimental design was applied, with crossed drought treatments (two levels: severe drought D+, or ambient rainfall D−) and grazing treatments (two levels: moderate grazing G+ or resting, G−) replicated over four blocks. The resulting 24 plots (10 × 10 m) were separated by corridors with a width of 5 m. Cattle grazing on G- plots was excluded with the aid of permanent wire fences. On D + plots, rainfall was reduced by 66% through the use of large rainout shelters with a size of 36 m2 (Figure 2A), established at the center of the plots. The rainout shelters allowed for cattle to roam and graze under them. Because the shelters were open on all sides and had a minimum height of 2 m, they had negligible effects on the air temperature beneath them (data not shown). We also oriented the lower sides of the shelters to intercept rain from the dominant wind direction (Carlyle et al., 2014). To prevent interference of lateral soil water movements, we trenched around the perimeter of the rainout shelters to maximum soil depth (≤70 cm) and inserted an impermeable plastic sheet. Sampling was restricted to a central subplot area in each plot (4.8 m × 4.8 m) to reduce marginal effects. Grazing and drought treatments were established in October 2014 and maintained permanently.
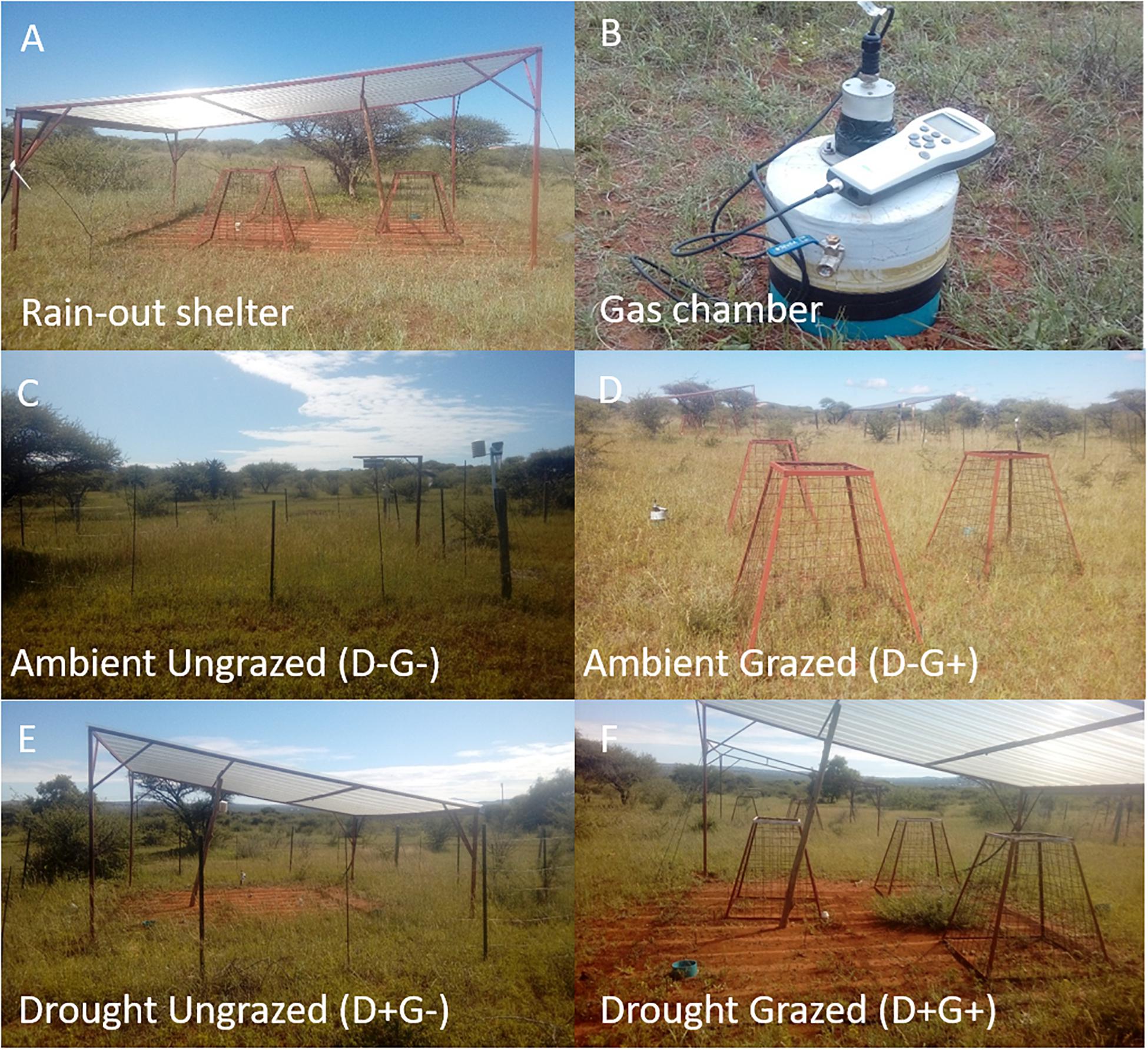
Figure 2. The experimental platform DroughtAct for studying grassland ecosystem responses to combined effects of extreme drought and grazing. (A) Details of a rainout shelter (36 m2) used in DroughtAct to simultaneously study drought and grazing effects; (B) details of a flux chamber used to measure CO2 release. (C–F) Effects of drought and grazing treatments on grassland vegetation. Pictures were taken in the sixth treatment year during the time of peak standing biomass (February 2020). (C) Ambient rainfall with grazing exclusion (D–G–); (D) ambient rainfall under grazed conditions; the moveable cages are used to assess ANPP without grazing offtake (D – G+); (E) drought with grazing exclusion (D + G–); (F): drought with moderate grazing (D + G +).
Installation of CO2 Chambers and Measurement of CO2
In December 2018, two CO2 chambers were installed in the central subplot of each plot (32 chambers in total). The DroughtAct plots had been under the grazing and drought treatments for four growth periods prior to the installation of the chambers, and treatment effects on vegetation were clearly visible (Figures 2C–F). The CO2 chambers were constructed and installed in the field according to the USDA-ARS GRACEnet Project Protocols (Parkin and Venterea, 2010). The gas chambers were, however, modified and interfaced with a CO2 probe GMP343 along with MI70 data logger (Viasala, Vantaa, Finland) to allow for in situ CO2 measurements (Figure 2B). The chambers consisted of two separate PVC rings, i.e., a PVC collar (0.20 m diameter, 0.15 m height) and another PVC ring (0.20 m diameter, 0.10 m height) sealed on one side with a PVC circle to make a chamber lid. The chamber lid had a small vent on it to prevent pressure build-up in the chambers during measurements. The chamber lid was also perforated on top to fix the CO2 probe. The collars were hammered into the soil to a depth of 0.05 m leaving 0.1 m above the soil. These collars were left in the soil for a few days to settle before measurements were taken.
When taking measurements, the chamber lid with the fixed CO2 probe on it was attached to the collar and secured with a tube strip to make it airtight. The CO2 probe GMP343 was set to record measurements every 30 s for 5 min. All measurements were taken between 10:00 h and 14:00 h. CO2 fluxes were measured over a period of 5 months from January to May 2019 at 2-week intervals, resulting in eight measurement events.
Calculation of CO2 Fluxes
The CO2 probe GMP343 gives measurements of CO2 in parts per million (ppm). The measurements were first converted to mg m–3 using the ideal gas law (Eq. 1):
Where P = pressure, V = volume, n = moles of gas, R = gas law constant (8.3145 J mol–1 K–1), and T = temperature in Kelvin. The molar volume of an ideal gas at 1atm pressure (101.325 kPa) and 25°C is 22.4 L mol–1. Thus, at different pressures, the molar volume of the gas can be calculated as follows:
At different temperature and pressure the concentration of CO2 in mg/m3 was calculated as follows:
Where CO2 ppm is the measured concentration of CO2 at any given time, T is the chamber temperature (Temperature in°C + 273.15 K) and P is the ambient pressure.
CO2 concentration in mg m–3 was then plotted against time (min) giving a slope in mg m–3min–1. The slope of the resulting regression lines was then determined for each installed chamber. The slope was then multiplied by the volume of the chamber (0.00628 m3) and divided by the area covered by the chamber (0.0314 m2), giving the resultant flux in mg m–2 min–1, which describes the CO2 flux out of the soil. Cumulative CO2 was calculated by assuming that the rate of CO2 release was constant between two measurement points.
Determination of Bulk Density, Infiltration Rate, and Particle Size
Bulk density was measured using the core ring method. Cores with a diameter of 5 cm and a height of 5 cm were used to collect an undisturbed soil core sample in the topsoil of each treatment plot. The soils were then oven-dried at 105°C for 24 h before being weighed. Infiltration rate was measured using mini-disk infiltrometers (Decagon Devices, United States). The infiltration measurements were taken once per plot. The particle size was determined by the pipette method (Day, 1965).
Soil Chemical Analyses
Soil samples were collected in plots’ central subplot area for two depths i.e., 0–15 and 15–30 cm using augers. To minimize destructive sampling on monitoring plots, we only sampled once per plot. The samples were then air-dried and sieved to pass through a 2 mm sieve. Soil pH was determined in both water and KCl. De-ionized water and 1M KCl solution were used in a ratio of 1:2.5 and measured using a glass electrode. Calcium and Mg were determined by atomic absorption after being extracted using 1M KCl, filtered and diluted with 20 ml of 0.0356M SrCl2. Phosphorus, K, Zn, Cu, and Mn were extracted using Ambic-2 solution and determined using atomic absorption. Total C and N were analyzed by the Automated Dumas dry combustion method using a LECO CNS 2000 (LECO Corporation, Michigan).
Determination of Soil Carbon Stocks
Soil carbon stocks are the amount of carbon in a soil layer of known bulk density. Soil carbon stocks are normally given as weight per unit area and are commonly limited to the soil fraction of less 2 mm in size. Soil C stocks are determined by the following formula (Batjes, 1996):
Here, soil C stock is soil carbon stock (kg C m–2), Ctot is the total carbon content (g C g–1 soil), BD is bulk density (kg m–3); and d is soil depth (m). Carbon stocks were calculated from the top 5 cm since bulk density was only determined in that depth. This was mainly because the cores were of 5 cm height and there was need to minimize destructive sampling.
Aboveground Net Primary Production
Aboveground net primary production (ANPP) was assessed via destructive biomass sampling. Grass biomass was collected at the time of peak standing biomass (i.e., during May and June) of the years 2018 and 2019. During this time, most plant species present on the plots had fully grown and flowered. On grazed plots, standing biomass was harvested within three 1 m2 quadrats which were protected from grazing offtake during the growth period with the aid of moveable cages (Figures 3D,F). We created a uniform starting conditions for plant growth at the onset of each growth period by removing residual biomass and carryover material from the previous growth period. This was done by clipping plants to stubble height (∼5 cm). On ungrazed plots, sampling quadrats (3 per plot) only had a size of 50 × 50 cm to minimize the impact of destructive sampling on monitoring plots (see Linstädter and Baumann, 2013). We discarded all moribund plant material, and only collected green and senescent material. Plant material was oven-dried (60°C, 48 h), and weighed to the nearest gram. ANPP was then estimated from aboveground biomass data via the peak standing crop method (Scurlock et al., 2002; Ruppert and Linstädter, 2014).
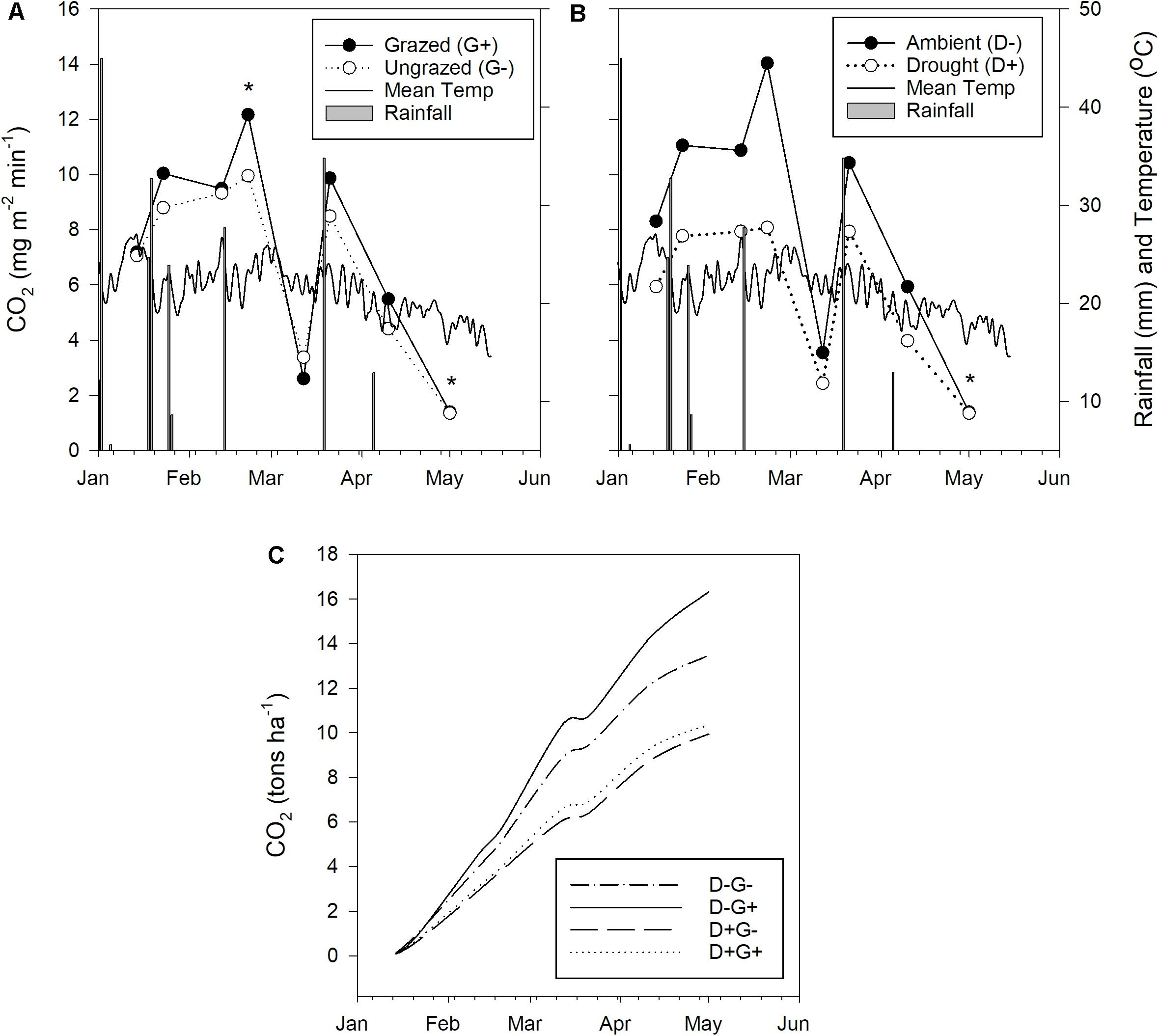
Figure 3. Effect of grazing and drought on CO2 fluxes. (A) Comparison of CO2 fluxes between grazed and ungrazed plots; (B) comparison of CO2 fluxes between plots under drought and ambient rainfall conditions, and (C) cumulative CO2 emissions over the measurement period in the four treatments (D–G– ambient rainfall with grazing exclusion; D– G+ ambient rainfall with moderate grazing; D + G– drought with grazing exclusion; and D + G + drought with moderate grazing).
Data Analysis
Two-way ANOVAs were performed to determine the effect of grazing, drought and their interaction on C stocks, CO2 fluxes (individual points, cumulative and average rates), and chemical soil properties. Where effects of factors or their interaction were significant, Tukey HSD was used for post hoc comparisons. Linear regression analysis was done to determine the impact of ANPP, bulk density, and C stocks on CO2 flux rates. We also performed linear regression between C stocks and CO2 flux rates to search for dependencies. All data were analyzed using SPSS 25 (SPSS, United States).
Results
CO2 Emission as Influenced by Grazing and Drought
CO2 fluxes ranged from 1.35 mg m–2 min–1 at the time of peak standing biomass (May 2019) to 14.03 mg m–2 min–1 recorded in February 2019, i.e., at the time of maximum plant growth (Figure 3). Daily rainfall and mean daily temperature data indicate that CO2 fluxes were higher when rainfall was higher (Figure 3). This is clearly shown by the sharp decrease in CO2 fluxes in mid-March when there was a month-long period of sporadic and ineffective daily rainfall (<5 mm). The CO2 flux rate then sharply rose after 35 mm of rainfall were received. In contrast, air temperature did not influence the variation in CO2 fluxes.
A clear trend toward higher CO2 emissions from grazed plots was discernible, the differences were, however, not significant (Table 1 and Figure 4). An exception was one sampling event in February, where grazed plots released significantly more CO2 than rested plots (12.17 ± 4.86 mg m–2 min–1 compared to 9.95 ± 3.55 mg m–2 min–1; Figure 3A, marked with a star). Contrastingly, drought significantly reduced CO2 flux almost for all measurement events (Figure 4) until the beginning of the dry season in May, when the measured CO2 fluxes were almost equal on the last sampling event (Figure 3B, marked by a star). Average CO2 emission from drought plots were 32% lower than those under ambient rainfall.

Table 1. Full factorial ANOVA, F statistics, with P-values showing how C stocks, CO2 emission rates and bulk density were influenced by grazing (G), drought (D), and the interaction of grazing and drought (G × D).
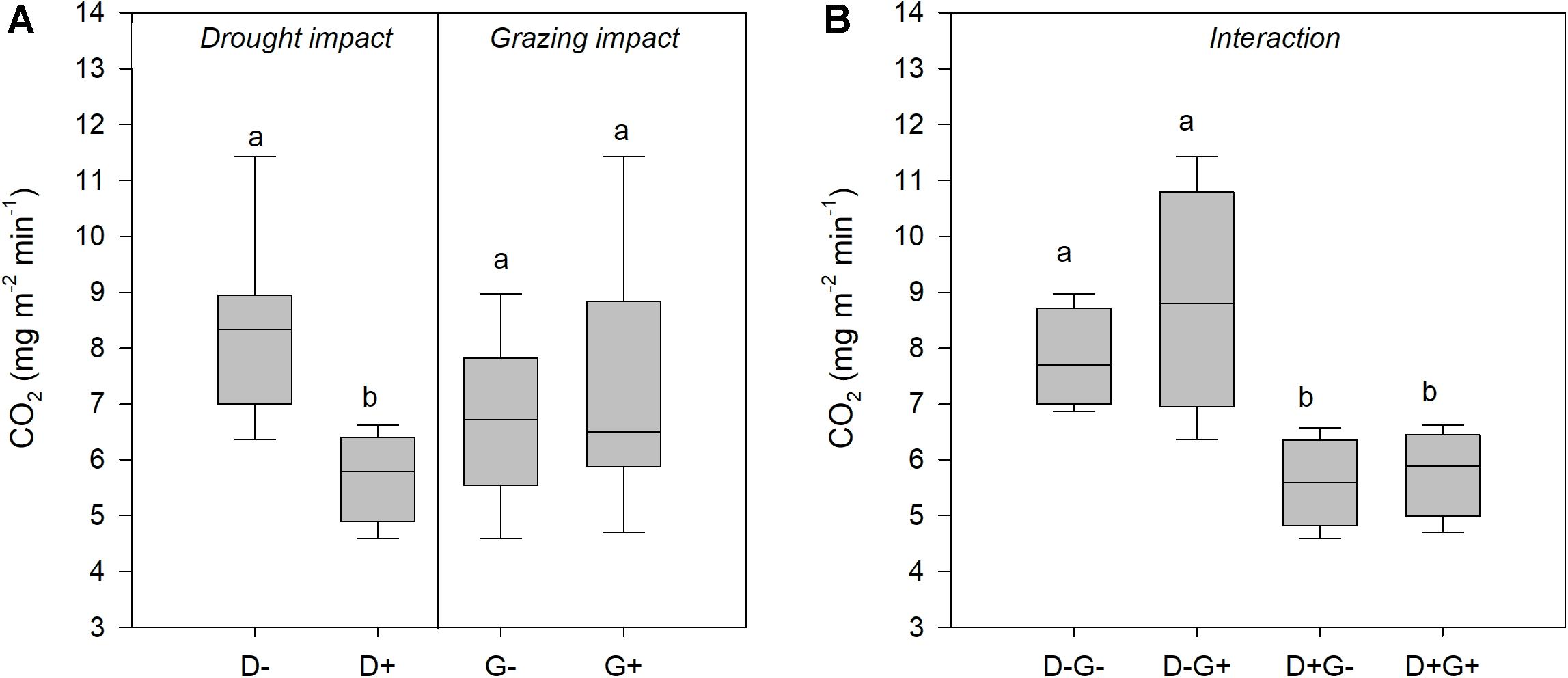
Figure 4. Comparison of the effect of drought and grazing on CO2 fluxes throughout the measurement period. (A) Impact of drought and impact of grazing; and (B) the combined effect of drought and grazing on CO2 fluxes. Different lowercase letters indicate significant differences among treatments (P < 0.05).
The interaction effect between grazing and drought was not significant for CO2 fluxes (Table 1). However, Figure 4B clearly showed that the impact of drought overrode the impact of grazing as treatments that included drought (D + G− and D + G+) had lower CO2 flux rates compared to those that excluded drought (D−G− and D−G+). Looking at each treatment individually, cumulative CO2 emission were higher under ambient rainfall with moderate grazing followed by ambient conditions with grazing exclusion while the least emissions were observed for the treatments that were under drought (Figure 3C). By the end of the growth period, grazed plots under ambient rainfall (D−G+) had released 16.3 tons of CO2 ha–1 which was 58% higher than observed on grazed plots subjected to severe drought (D+ G+). The results also showed that the average CO2 flux rate under ambient conditions was 8.3 mg m–2 min–1 equating to 43.6 tons CO2 ha–1 year–1 (assuming the rate remained constant throughout the year), while under drought, the CO2 flux rate was 5.7 mg m–2 min–1, resulting in 30 tons CO2 ha–1 year–1.
Effect of Drought and Grazing on Infiltration Rate, Bulk Density, and Carbon Stocks
Drought did not affect bulk density of the top 5 cm of the soil but grazing did (Table 1 and Figure 5A). Bulk density was higher under grazed plots (1.53 ± 0.07 g cm–3) compared to ungrazed plots (1.39 ± 0.08 g cm–3) (Figure 5A). Infiltration rates also had a similar result where infiltration was also not influenced by drought but only with grazing, whereby the infiltration rate was higher under grazed plots compared to rested plots (results not shown). Soil carbon stocks, on the other hand, were significantly affected by drought (P < 0.05) but not by grazing (P > 0.05) (Figure 5C). Soil carbon stocks were higher under drought (1.118 ± 0.130 kg C m–2) compared to ambient conditions (0.999 ± 0.115 kg C m–2). Although no variations were observed, soil carbon stocks tended to be relatively higher under grazed plots compared to ungrazed ones.
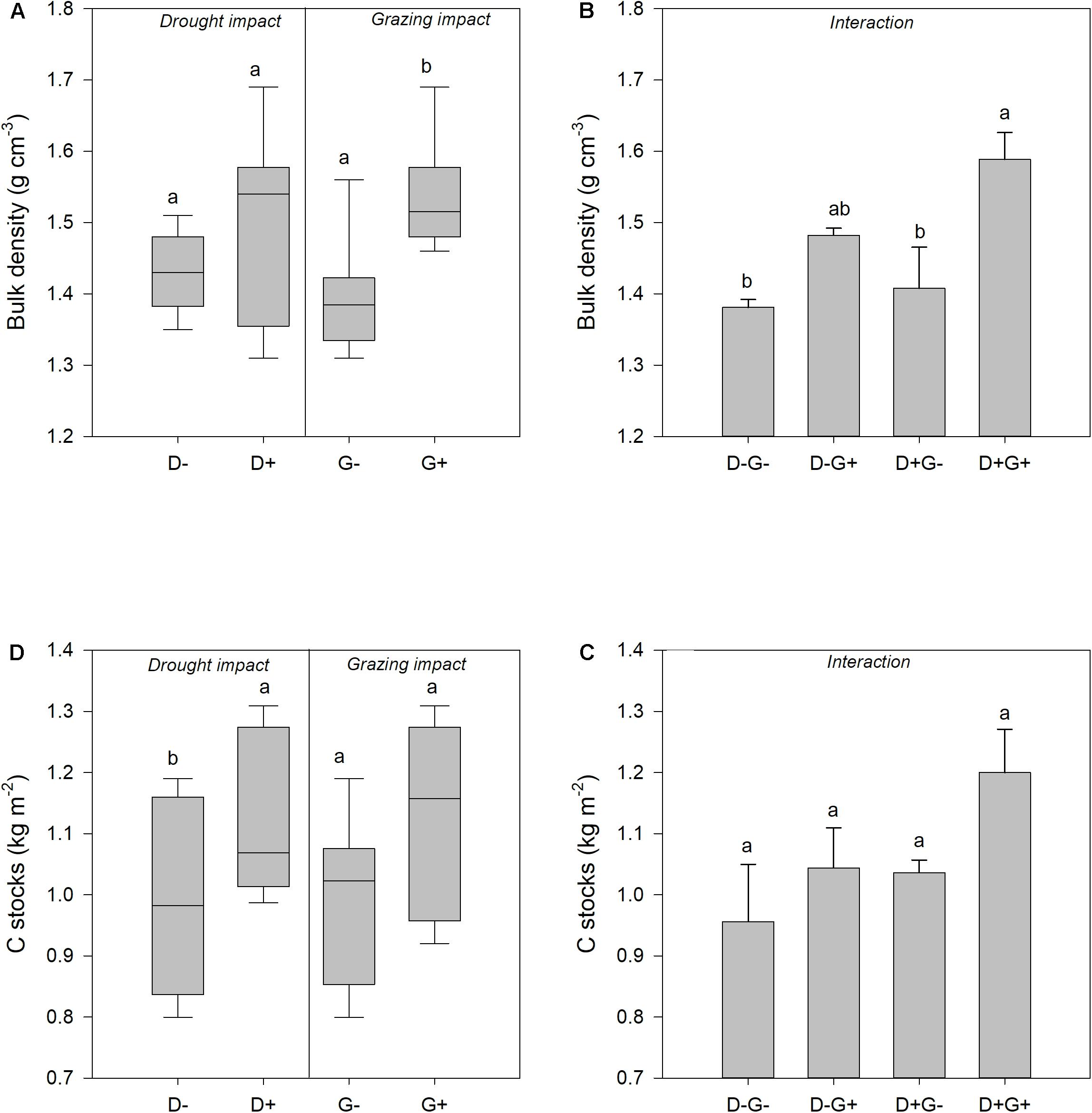
Figure 5. Effect of drought and grazing on bulk density and C stocks. (A) The impact of the main effects (drought and grazing) on bulk density; (B) combined effect of drought and grazing on bulk density; (C) the impact of the main effects (drought and grazing) on C stocks; and (D) combined effect of drought and grazing on C stocks. Different lowercase letters indicate significant differences among treatments (P < 0.05).
Only bulk density was affected by the combined effect of grazing and drought (Figure 5). Bulk density was higher under drought and grazing with a bulk density of 1.59 g cm–3. Soil carbon stocks did not respond to the interaction effect of grazing and drought but was also relatively higher under drought and grazing (D + G +).
Relationships Between C Stocks, ANPP, Bulk Density, and CO2 Flux
Regression analyses (Figure 6) were performed to enhance the understanding of the factors that affect carbon storage and CO2 flux in the soil. The relationship between bulk density and soil C stocks was positive but not significant. Soils with high bulk density tended to have a higher carbon content. The relationship between soil C stocks and CO2 flux revealed a negative association. ANPP from the previous growth period (2017/18) did not show any relationship with soil C stocks, while ANPP from the same growth period (2018/19) explained about 20% of the variation observed in soil C stocks (Figure 6C). While soil C stocks slightly decreased with an increase in biomass production, CO2 fluxes increased with an increase in production. ANPP of the previous growth period and of the current growth period explained 32% and 48% of the variation observed in CO2 fluxes, respectively.
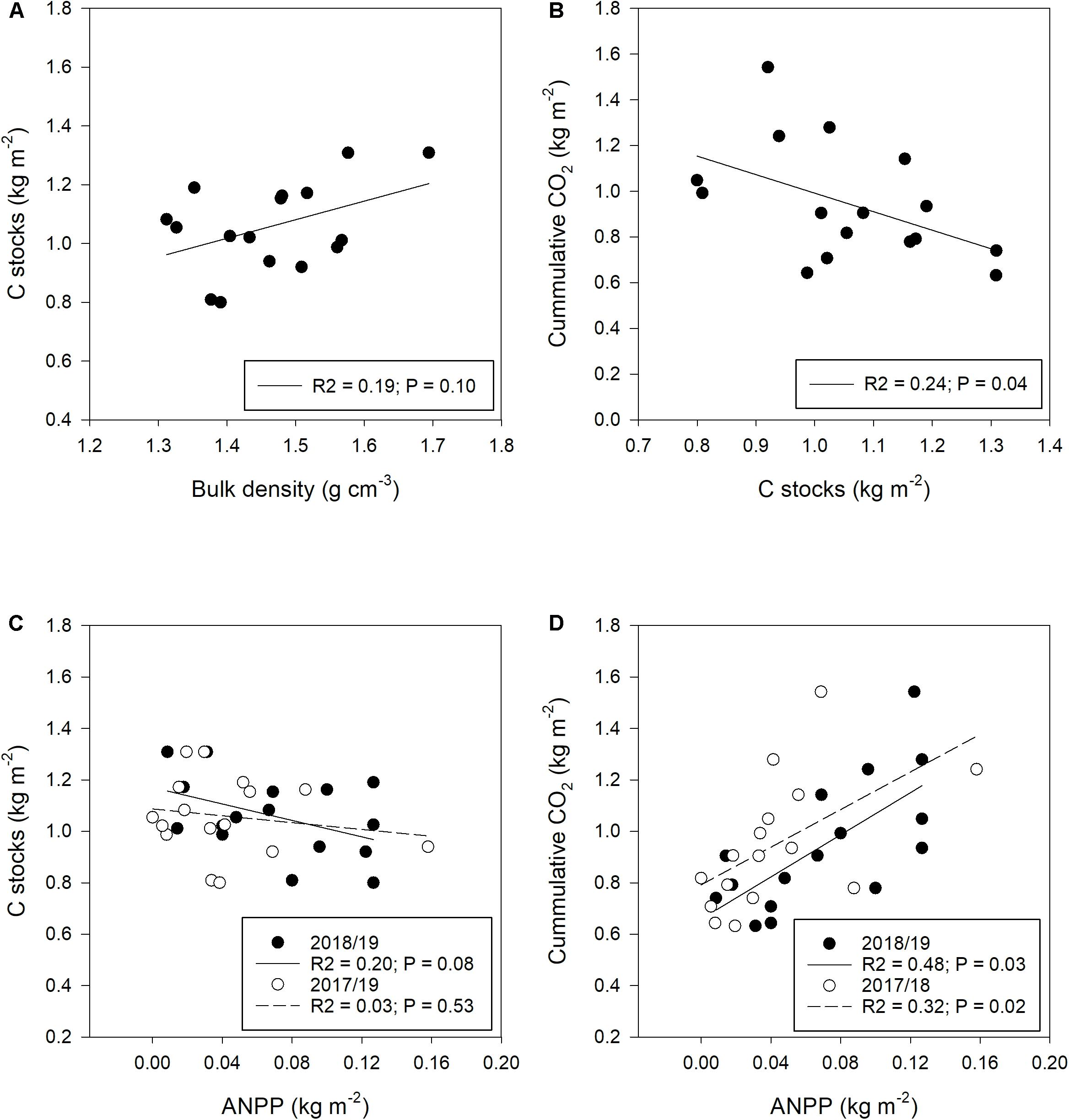
Figure 6. Dependence of carbon stocks and CO2 fluxes on environmental variables (A,C,D) and on each other (B). (A) Carbon stocks in dependence of bulk density; (B) CO2 fluxes in dependence of C stocks; (C) carbon stocks in dependence of aboveground net primary production (ANPP), and (D) CO2 fluxes in dependence of ANPP. For ANPP, open circles indicate data from the 2017/18 growth period, while shaded circles represent data from the 2018/19 period, i.e., the same growth period for which CO2 flux and carbon stocks were assessed. Dashed lines on panels C and D represent regression lines for 2017/18 while solid lines represent regression for 2018/19.
Soil Chemical Characteristics as Affected by Drought Treatments
Chemical properties in the topsoil (0–15 cm) did not significantly differ from properties in 15–30 cm depth (results not shown). However, visual observations revealed that P, K, C, Cu, and Zn tended to be relatively higher in the top 15 cm compared to the 15–30 cm, while Ca and Mg were relatively higher in the 15–30 cm layer compared to the 0–15 cm layer. Due to the lack of significant differences between the two depths, data were combined and jointly analyzed for the effect of drought and grazing (Table 2) and their combined effect (Table 3).

Table 3. Soil chemical parameters (0–30 cm) as influenced by the interaction effects of drought and grazing.
The results showed that N and P were lower in the grazed plots compared to the ungrazed, while Mg and Mn were higher in grazed plots. The concentration of potassium, Ca, Zn, Cu, and the soil pH were not influenced by grazing. On the other hand, drought significantly increased N, P, K, Zn, and Mn concentrations in the topsoil. Soil pH was also 0.16 units higher in the plots that were under drought compared to those that were under ambient rainfall conditions.
The combined effect of drought and grazing had a significant influence on P, K, Zn, N, and on C:N ratios (Table 3). Phosphorus, Zn and N were all higher for plots under drought and ungrazed conditions (D + G−) compared to the other three treatments. Similarly, the C:N ratios were lowest under D + G−. Potassium, however, was highest under drought and grazed (D + G +) with a concentration of 237 mg kg–1 and lowest in ambient and grazed (D−G+) with a concentration of 140 mg kg–1. Even though the combined effect of drought and grazing did not influence carbon, D + G− plots tended to have higher total carbon in soil compared to the plots. Soil pH was slightly acidic, ranging from 4.5 to 4.8 and did not respond to the impact of drought and grazing.
Discussion
Effect of Drought and Grazing on Soil Carbon Storage and CO2 Fluxes
This study used a large field experiment in a dryland grassland to provide novel insights into soil carbon storage and release under long term exposure to extreme drought and moderate grazing. While grazing had no clear effect, drought reduced CO2 fluxes and increased soil C stocks. Intuitively, ambient conditions would be expected to have higher carbon storage due to higher biomass accumulation (see Figure 2) and higher photosynthetic rates (Li et al., 2017), which should result in more carbon input into the soil than is lost through respiration. There are indeed studies that have shown a higher soil organic carbon accumulation due to higher plant biomass production (Zhao et al., 2018; Prommer et al., 2020). The results of the current study are consistent with other studies comparing decomposition rates under drought and ambient conditions. Sanaullah et al. (2012) reported more than 50% decrease in grass litter decomposition due to drought when compared to ambient conditions. The lower CO2 flux rate observed under drought conditions could possibly mean that less soil carbon was lost thus maintaining higher carbon storage (Figure 5C). Decomposition rates are known to be slower under dry conditions (Sanaullah et al., 2012; Lefèvre et al., 2017) mainly because microbial activity decreases under water stress conditions (Manzoni et al., 2012; Schimel, 2018).
Therefore, the observed higher soil C stocks under extreme drought could probably be attributed to the low CO2 emission rates under drought. This means that less C was lost when compared to ambient conditions. In addition, the higher soil C stocks observed under drought could also be partially explained by the higher concentration of multivalent cations such as Ca, Zn, and Mn. These multivalent cations have been reported to contribute to the stabilization of soil organic carbon in the same study area (Dlamini et al., 2019). Multivalent cations, such as Ca, Al, Fe, and Mn offer chemical protection of soil organic carbon through the formation of organo-mineral complexes (Jastrow et al., 2007).
Studies have shown that most of the CO2 released from the soil is due to respiration by roots (autotrophic) and/or soil microorganisms and fauna (heterotrophic) (Hanson et al., 2000; Bond-Lamberty et al., 2004), both of which are sensitive to soil moisture (Li et al., 2018; Wang Y. et al., 2020). Low soil moisture content reduces microbial biomass (Liu et al., 2009). It also hinders root growth due to inhibition of shoot growth and the subsequent decrease in translocation of assimilates to the roots and the rhizosphere (Li et al., 2018). Furthermore, drought may massively reduce root exudates which promote rhizospheric respiration. The current results point to a reduction in both autotrophic and heterotrophic respiration due to drought. However, from the field observations, plots under drought conditions had very sparse vegetation if not completely bare (Figures 2E,F), suggesting a higher contribution of heterotrophic respiration to the measured CO2 flux than autotrophic respiration. In support, Balogh et al. (2016) found that rhizospheric respiration, a component of autotrophic respiration was highly sensitive to drought. Moreover, Hanson et al. (2000), asserts that the root contribution to total soil respiration is higher during the growing season and lower during the dormant periods of the year while Wang Y. et al. (2020) allude to the stronger relationship between plant growth and root respiration.
Grazing did not significantly influence both soil carbon storage (Figure 5C) and CO2 flux (Figure 4B). These findings are contrary to the findings of Li et al. (2017). However, soil carbon storage was relatively higher in grazed plots compared to ungrazed plots which is in agreement with the findings of Sun et al. (2019). Increased belowground biomass under moderate grazing is reported to be due to the translocation of photosynthates to the roots (Shi et al., 2013; Sun et al., 2019). It is also believed that moderate grazing promotes root-associated microbes and fauna that leads to an increase in below-ground biomass. Li et al. (2017) on the other hand found that grazing reduced root biomass compared to ungrazed plots.
In this study we did not observe a strong confounding effects of drought and grazing on CO2 flux and carbon stocks after a 5-year long extreme drought. As observed in Figure 4B, the impact of the long extreme drought seemed to override any impact that grazing might have had. This is also evident on Figures 2E,F where there is rarely any vegetation on plots exposed to the extreme drought. It can be postulated that the interaction might have been significant and additive in the earlier stages of the experiment but got progressively weaker to non-existence with time due to the deleterious effects of extended extreme drought. In support of our postulation, Okach et al. (2019b) reported a minimal impact of grazing on plots with less rainfall compare to those under higher rainfall. Furthermore, Zhou et al. (2019b) also reported higher impact of drought on CO2 flux compared to that of grazing. However, grazing and drought can have confounding impact on carbon storage and soil CO2 flux (Okach et al., 2019b; Zhou et al., 2019a,b).
With reference to the challenges of global climate change and the need to reduce greenhouse gas emissions, this study has provided inventory data on the CO2 emission levels in African dryland grasslands. The results show that by early May, ambient rainfall with moderate grazing had resulted in cumulative CO2 emissions of about 16.3 tons ha–1. These values are comparable to those reported in other parts of SSA (Kim et al., 2016; Berger et al., 2019). Kim et al. (2016) reported emission rates of 3.3–57 tons CO2 ha–1 year–1 in natural terrestrial systems of the SSA of which grasslands emitted an average of 15.5 ± 3.8 tons CO2 ha–1 year–1. On the other hand, we found that ungrazed dryland grasslands to store about 0.996 kg C m–2 (i.e., 9.7 tons C ha–1), while grazed ones stored about 1.122 kg C m–2 (i.e., 11.2 tons C ha–1). These quantities are in line in with those obtained by Dlamini et al. (2019) in the same area which ranged from 0.40 to 1.87 kg C m–2.
Relationships Between C Stocks, ANPP, Bulk Density, and CO2 Flux
Our study showed that soils with higher bulk density had higher soil C stocks (R2 = 0.19, P = 0.10). This is because soils with higher bulk density are compacted and have a curtailed air filled porosity (van Verseveld and Gebert, 2020). The curtailment of air filled porosity reduces oxygen availability to microbes leading to reduced carbon oxidation and thus preserves carbon in the soil. Neilson and Pepper (1990) found reduced CO2 evolution on soils with higher bulk density. Consequently, a negative relationship between soil C stocks and CO2 emission (R2 = 0.25; P = 0.04) was observed. ANPP on the other hand was positively related to CO2 emission rate with a third to almost half of the variation observed in CO2 emission rate being explained by it. Higher ANPP suggests an increase in root biomass and thus also an increased root respiration (Luo and Zhou, 2006). According to the study by Luo and Zhou (2006), root respiration contributes about half of the total soil respiration. Finally, we found ANPP to be negatively related to carbon stocks. This is at the first glance surprising, but is in line with recent studies stating that ANPP may either increase or decrease soil organic carbon in grasslands, depending on the net result of plant-C inputs and C decomposition (Peterson and Lajtha, 2013; Chen et al., 2018).
Effect of Drought and Grazing on Soil Properties
This study provides an indication of combined effects of drought and grazing on soil chemical properties other than the commonly reported C and N (e.g., Okach et al., 2019a). The results revealed that grazing decreased P and N in the topsoil but did not influence other chemical properties such as pH, K, and the C:N ratio. The decrease in nitrogen due to grazing is a common phenomenon in heavily grazed grasslands (Han et al., 2008; He et al., 2019). Under low grazing intensities, the concentration of N in soils is usually unaffected due to the balance between N removal and N returned. It is believed that livestock returns the majority of the ingested N to the soil in the form of urine and feces (Han et al., 2008). In addition, the trampling by livestock increases the incorporation and the microbial mineralization of the litter thus returning C and N to the soil (Li et al., 2017). On the other hand, the effect of grazing on P is not much reported. The only study we found that reported on P showed the P response to grazing is dependent on mean annual precipitation. It was shown that grazing reduces soil P when precipitation is lower than 400 mm but increases when it higher (He et al., 2019).
While grazing reduced soil P, drought, on the other hand, resulted in increased soil P. Drought also increased other soil chemical properties such as pH, K, Zn, Mn, N as well as lowering the C:N ratio (Table 2). The higher concentrations observed under drought could be attributed to less leaching of the nutrients as the plots under drought received less than 40% of the ambient rainfall. The generally low pH observed in this study is a testament to the prevalence of basic ions leaching from the topsoil. Leaching is a problem particularly in light-textured soils (Matichenkov et al., 2020). Also, the higher concentrations of some of the determined soil chemical properties could just be a result of reduced nutrient mining by plants as drought impeded growth and nutrient uptake. Nutrient mining which is the removal of nutrients from the soil by plants (Vanlauwe et al., 2015; Kodzwa et al., 2020) and it can be an issue in poorly managed dryland grasslands due to inefficient nutrient cycling (Bilotta et al., 2007; Han et al., 2008).
In addition, drought and grazing acted together to influence the concentration of macronutrients (NPK) as well as Zn and C:N ratio in the top soil. Exposure to drought with grazing (D + G−) resulted in increased concentration of P and N and subsequently lower C:N ratios. These findings of increased N and relatively lower C:N ratios under D+ G− are contrary to the findings of Okach et al. (2019a). It is not clear why N, P, and Zn were higher under D + G− but this could be the attributed to reduced leaching and no removal by feeding animals.
Grazing increased the bulk density of the surface soil. The average bulk density of grazed plots was 1.54 g cm–3 while that of ungrazed plots was 1.39 g cm–3. These results are in agreement with findings from previous studies (Chen et al., 2017; Li et al., 2017; Okach et al., 2019a; Sun et al., 2019; Zhou et al., 2019a). Soils may become compacted because of trampling by livestock, especially when grazing takes place in damp areas. According to Bilotta et al. (2007), hooves of an adult cow weighing between 300 and 600 kg can exert static pressures of approximately 200 kPa on the soil, which is a high enough force to compact soil. Thus, if the stocking rate is high and the soil is wet, trampling could be highly detrimental for soil physical properties. The effect of drought on bulk density has rarely been reported and in this study it was observed that drought tended to increase the bulk density of the soil compared to under ambient conditions. Slightly higher bulk densities under drought could be due to the lack of vegetation which exposes the soil to compaction from external forces such as raindrop impact (Neave and Rayburg, 2007) and livestock trampling (Bilotta et al., 2007). Also, plant roots enhance soil aggregation, improving soil structure and hence reducing bulk density (Rillig et al., 2015).
Conclusion
This study provides an indication of carbon storage and emission rates in a dryland grassland. Both grazing and severe drought had a positive effect on soil carbon storage but had dissimilar impacts on CO2 fluxes. Drought drastically reduced the amount of CO2 released from the soil, while moderate grazing did not influence it. At the end of the growth period, grazing under ambient rainfall had released 16.3 tons of CO2 ha–1 which was 58% higher than observed on grazed plots subjected to severe drought. Moderate grazing reduced P in the soil compared to the ungrazed plots. On the other hand, drought increased P concentration in the soil when compared to under ambient conditions. Our results imply that long-term extreme drought in dryland grasslands may be favorable in terms of preserving the existing soil carbon stocks through reduced CO2 release to the atmosphere. This finding is critical in understanding future soil carbon dynamics in dryland grasslands in the face of climate change.
Data Availability Statement
The datasets presented in this article are not readily available because some data were used as predictor variables and that data still needs to be published. Requests to access the datasets should be directed to AL, YW5qYS5saW5zdGFlZHRlckB1bmkta29lbG4uZGU=.
Author Contributions
AL and KKA conceived and designed the experiments. LM and TM redesigned some parts of the experiments. LM, KB, EM, and MM carried out the field and lab-work. LM analyzed the data. LM wrote the manuscript. All authors commented on the analysis, interpretation, presentation of the data, and critically revised the manuscript.
Funding
This research was funded by the German Federal Government (BMBF) through the SPACES initiative (“Limpopo Living Landscapes” project – grant 01LL1304D; “SALLnet” project – grant 01LL1802C). EM acknowledges financial support from the South African National Research Fund through ACCESS DST and from the German Academic Exchange Program, DAAD. MM acknowledges funding by the South African National Research Foundation (NRF) and by the DAAD. AL acknowledges funding by the German Research Foundation (DFG) through the collaborative research center “Future Rural Africa,” funding code TRR 228/1. We also acknowledge support by the Risk and Vulnerability Science Center, University of Limpopo.
Conflict of Interest
The authors declare that the research was conducted in the absence of any commercial or financial relationships that could be construed as a potential conflict of interest.
Acknowledgments
We thank Jan C. Ruppert and the managers of Syferkuil Experimental Farm (Louis Eloff, Fhatuwani Ndwambi) and the University of Limpopo security service for logistical support during the construction and maintenance of the DroughtAct experiment. We also acknowledge support with data collection and laboratory analyses from various student assistants.
References
Acocks, J. P. H. (1994). Veld Types of South Africa. Cape Town, South Africa: Botanical Research Institute.
Balogh, J., Papp, M., Pintér, K., Fóti, S., Posta, K., Eugster, W., et al. (2016). Autotrophic component of soil respiration is repressed by drought more than the heterotrophic one in dry grasslands. Biogeosciences 13, 5171–5182. doi: 10.5194/bg-13-5171-2016
Bat-Oyun, T., Shinoda, M., Cheng, Y., and Purevdorj, Y. (2016). Effects of grazing and precipitation variability on vegetation dynamics in a Mongolian dry steppe. J. Plant Ecol. 9, 508–519. doi: 10.1093/jpe/rtv083
Batey, T. (2009). Soil compaction and soil management–a review. Soil Manag. 25, 335–345. doi: 10.1111/j.1475-2743.2009.00236.x
Batjes, N. H. (1996). Total carbon and nitrogen in the soils of the world. Eur. J. Soil Sci. 47, 151–163. doi: 10.1111/j.1365-2389.1996.tb01386.x
Benhin, J. K. (2006). “Climate change and South African agriculture: Impacts and adaptation options,” in Paper Presented at the The Centre for Environmental Economics and Policy in Africa, (Pretoria: University of Pretoria).
Berger, S., Bliefernicht, J., Linstädter, A., Canak, K., Guug, S., Heinzeller, D., et al. (2019). The impact of rain events on CO2 emissions from contrasting land use systems in semi-arid West African savannas. Sci. Tot. Environ. 647, 1478–1489. doi: 10.1016/j.scitotenv.2018.07.397
Bilotta, G., Brazier, R., and Haygarth, P. (2007). The impacts of grazing animals on the quality of soils, vegetation, and surface waters in intensively managed grasslands. Adv. Agron. 94, 237–280. doi: 10.1016/s0065-2113(06)94006-1
Bond-Lamberty, B., Wang, C., and Gower, S. T. (2004). A global relationship between the heterotrophic and autotrophic components of soil respiration? Glob. Change Biol. 10, 1756–1766. doi: 10.1111/j.1365-2486.2004.00816.x
Carlyle, C. N., Fraser, L. H., and Turkington, R. (2014). Response of grassland biomass production to simulated climate change and clipping along an elevation gradient. Oecologia 174, 1065–1073. doi: 10.1007/s00442-013-2833-2
Chen, S., Wang, W., Xu, W., Wang, Y., Wan, H., Chen, D., et al. (2018). Plant diversity enhances productivity and soil carbon storage. Proc. Natl. Acad. Sci. 115, 4027–4032. doi: 10.1073/pnas.1700298114
Chen, W., Zheng, X., Wolf, B., Yao, Z., Liu, C., Butterbach-Bahl, K., et al. (2017). Long-term grazing effects on soil-atmosphere exchanges of CO2, CH4 and N2O at different grasslands in Inner Mongolia: A soil core study. Ecol. Indicat. 105, 316–328 doi: 10.1016/j.ecolind.2017.09.035
Collier, S. M., Ruark, M. D., Oates, L. G., Jokela, W. E., and Dell, C. J. (2014). Measurement of greenhouse gas flux from agricultural soils using static chambers. J. Vis. Exp. 90: 52110.
Day, P. R. (1965). “Particle fractionation and particle−size analysis,” in Methods of Soil Analysis: Part 1 Physical and Mineralogical Properties, Including Statistics of Measurement and Sampling, 9th Edn., ed C. A. Black, (Madison, WI: American Society of Agronomy and Soil Science Society of America), 545–567. doi: 10.2134/agronmonogr9.1.c43
Dlamini, P., Mbanjwa, V., Gxasheka, M., Tyasi, L., and Sekhohola-Dlamini, L. (2019). Chemical stabilisation of carbon stocks by polyvalent cations in plinthic soil of a shrub-encroached savanna grassland, South Africa. CATENA 181:104088. doi: 10.1016/j.catena.2019.104088
Ferner, J., Schmidtlein, S., Guuroh, R. T., Lopatin, J., and Linstädter, A. (2018). Disentangling effects of climate and land-use change on West African drylands’ forage supply. Glob. Environ. Change 53, 24–38. doi: 10.1016/j.gloenvcha.2018.08.007
Gaitán, J., Maestre, F. T., Buono, G., Bran, D., Dougill, A. J., Garcia-Martinez, G., et al. (2019). Biotic and abiotic factors controlling soil organic carbon content have similar effects in regional and global drylands. Ecosystems 4, 1141–1151. doi: 10.1007/s10021-019-00348-y
Guuroh, R. T., Ruppert, J. C., Ferner, J., Čanak, K., Schmidtlein, S., and Linstädter, A. (2018). Drivers of forage provision and erosion control in West African savannas - A macroecological perspective. Agricult. Ecosys. Environ. 251, 257–267. doi: 10.1016/j.agee.2017.09.017
Han, G., Hao, X., Zhao, M., Wang, M., Ellert, B. H., Willms, W., et al. (2008). Effect of grazing intensity on carbon and nitrogen in soil and vegetation in a meadow steppe in Inner Mongolia. Agricult. Ecosys. Environ. 125, 21–32. doi: 10.1016/j.agee.2007.11.009
Hanson, P. J., Edwards, N. T., Garten, C. T., and Andrews, J. A. (2000). Separating root and soil microbial contributions to soil respiration: A review of methods and observations. Biogeochemistry 48, 115–146. doi: 10.1023/A:1006244819642
He, M., Zhou, G., Yuan, T., van Groenigen, K. J., Shao, J., and Zhou, X. (2019). Grazing intensity significantly changes the C: N: P stoichiometry in grassland ecosystems. Glob. Ecol. Biogeogr. 29, 355–369 doi: 10.1111/geb.13028
IPCC (2019). Climate Change and Land. An IPCC Special Report on climate change, desertification, land degradation, sustainable land management, food security, and greenhouse gas fluxes in terrestrial ecosystems. Geneva: Intergovernmental Panel on Climate Change.
Jastrow, J. D., Amonette, J. E., and Bailey, V. L. (2007). Mechanisms controlling soil carbon turnover and their potential application for enhancing carbon sequestration. Climatic Change 80, 5–23. doi: 10.1007/s10584-006-9178-3
Jones, S., Rees, R., Skiba, U., and Ball, B. (2005). Greenhouse gas emissions from a managed grassland. Glob. Planet. Change 47, 201–211. doi: 10.1016/j.gloplacha.2004.10.011
Kim, D.-G., Thomas, A. D., Pelster, D., Rosenstock, T. S., and Sanz-Cobena, A. (2016). Greenhouse gas emissions from natural ecosystems and agricultural lands in sub-Saharan Africa: synthesis of available data and suggestions for further research. Biogeosciences 13, 4789–4809. doi: 10.5194/bg-13-4789-2016
Kodzwa, J. J., Gotosa, J., and Nyamangara, J. (2020). Mulching is the most important of the three conservation agriculture principles in increasing crop yield in the short term, under sub humid tropical conditions in Zimbabwe. Soil Tillage Res. 197:104515. doi: 10.1016/j.still.2019.104515
Kottek, M., Grieser, J., Beck, C., Rudolf, B., and Rubel, F. (2006). World map of the Köppen-Geiger climate classification updated. Meteorol. Zeitschrift 15, 259–263. doi: 10.1127/0941-2948/2006/0130
Lefèvre, C., Rekik, F., Alcantara, V., and Wiese, L. (2017). Soil organic carbon: the hidden potential. Rome: Food and Agriculture Organization of the United Nations (FAO).
Li, C., Peng, Y., Nie, X., Yang, Y., Yang, L., Li, F., et al. (2018). Differential responses of heterotrophic and autotrophic respiration to nitrogen addition and precipitation changes in a Tibetan alpine steppe. Sci. Rep. 8:16546. doi: 10.1038/s41598-018-34969-5
Li, W., Cao, W., Wang, J., Li, X., Xu, C., and Shi, S. (2017). Effects of grazing regime on vegetation structure, productivity, soil quality, carbon and nitrogen storage of alpine meadow on the Qinghai-Tibetan Plateau. Ecol. Eng. 98, 123–133. doi: 10.1016/j.ecoleng.2016.10.026
Linstädter, A., and Baumann, G. (2013). Abiotic and biotic recovery pathways of arid rangelands: Lessons from the High Atlas Mountains. Mor. CATENA 103, 3–15. doi: 10.1016/j.catena.2012.02.002
Linstädter, A., Kuhn, A., Naumann, C., Rasch, S., Sandhage-Hofmann, A., Amelung, W., et al. (2016). Assessing the resilience of a real-world social-ecological system: Lessons from a multi-disciplinary evaluation of a South African pastoral system. Ecol. Soc. 21:35. doi: 10.5751/ES-08737-210335
Linstädter, A., Schellberg, J., Brüser, K., Oomen, R. J., Du Preez, C. C., Ruppert, J. C., et al. (2014). Are there consistent grazing indicators in drylands? Testing plant functional types of various complexity in South Africa’s grassland and savanna biomes. PLoS One 9:e104672. doi: 10.1371/journal.pone.0104672
Liu, N., Zhang, Y., Chang, S., Kan, H., and Lin, L. (2012). Impact of Grazing on Soil Carbon and Microbial Biomass in Typical Steppe and Desert Steppe of Inner Mongolia. PLoS One 7:e36434. doi: 10.1371/journal.pone.0036434
Liu, W., Zhang, Z., and Wan, S. (2009). Predominant role of water in regulating soil and microbial respiration and their responses to climate change in a semiarid grassland. Glob. Change Biol. 15, 184–195. doi: 10.1111/j.1365-2486.2008.01728.x
Low, A. B., and Rebelo, A. G. (1998). Vegetation of South Africa, Lesotho and Swaziland: A Companion to the Vegetation Map of South Africa, Lesotho and Swaziland. Pretoria, South Africa: Department of Environmental Affairs and Tourism.
Lucci, G. M. (2019). Pastures and drought: a review of processes and implications for nitrogen and phosphorus cycling in grassland systems. Soil Res. 57, 101–112. doi: 10.1071/sr18079
Luo, Y., and Zhou, X. (2006). “CHAPTER 3 - Processes of CO2 Production in Soil,” in Soil Respiration and the Environment, eds Y. Luo and X. Zhou (Burlington: Academic Press), 35–59. doi: 10.1016/b978-012088782-8/50003-6
Manzoni, S., Schimel, J. P., and Porporato, A. (2012). Responses of soil microbial communities to water stress: results from a meta−analysis. Ecology 93, 930–938. doi: 10.1890/11-0026.1
Matichenkov, V., Bocharnikova, E., and Campbell, J. (2020). Reduction in nutrient leaching from sandy soils by Si-rich materials: Laboratory, greenhouse and filed studies. Soil Tillage Res. 196:104450. doi: 10.1016/j.still.2019.104450
Meissner, H. (1983). Classification of Livestock for Realistic Prediction of Substitution Values in Terms of a Biologically Defined Large Stock Unit. Republic of South Africa: Dept. of Agriculture.
Mudongo, E. I., Fusi, T., Fynn, R. W., and Bonyongo, M. C. (2016). The role of cattle grazing management on perennial grass and woody vegetation cover in semiarid rangelands: insights from two case studies in the Botswana Kalahari. Rangelands 38, 285–291. doi: 10.1016/j.rala.2016.07.001
Neave, M., and Rayburg, S. (2007). A field investigation into the effects of progressive rainfall-induced soil seal and crust development on runoff and erosion rates: The impact of surface cover. Geomorphology 87, 378–390. doi: 10.1016/j.geomorph.2006.10.007
Neilson, J. W., and Pepper, I. L. (1990). Soil respiration as an index of soil aeration. Soil Sci. Soc. Am. J. 54, 428–432. doi: 10.2136/sssaj1990.03615995005400020022x
Okach, D. O., Ondier, J. O., Kumar, A., Rambold, G., Tenhunen, J., Huwe, B., et al. (2019a). Interactive influence of livestock grazing and manipulated rainfall on soil properties in a humid tropical savanna. J. Soils Sediments 19, 1088–1098. doi: 10.1007/s11368-018-2117-x
Okach, D. O., Ondier, J. O., Kumar, A., Rambold, G., Tenhunen, J., Huwe, B., et al. (2019b). Livestock grazing and rainfall manipulation alter the patterning of CO 2 fluxes and biomass development of the herbaceous community in a humid savanna. Plant Ecol. 220, 1085–1100. doi: 10.1007/s11258-019-00977-2
Parkin, T., and Venterea, R. T. (2010). “Chapter 3. Chamber-Based Trace Gas Flux Measurements,”in Sampling Protocols., ed. F. RF (USA: USDA).
Peterson, F. S., and Lajtha, K. J. (2013). Linking aboveground net primary productivity to soil carbon and dissolved organic carbon in complex terrain. J. Geophys. Res.: Biogeosci. 118, 1225–1236. doi: 10.1002/jgrg.20097
Pfeiffer, M., Langan, L., Linstädter, A., Martens, C., Gaillard, C., Ruppert, J. C., et al. (2019). Grazing and aridity reduce perennial grass abundance in semi-arid rangelands - insights from a trait-based dynamic vegetation model. Ecol. Model. 395, 11–22. doi: 10.1016/j.ecolmodel.2018.12.013
Plaza, C., Gascó, G., Méndez, A. M., Zaccone, C., and Maestre, F. T. (2018). ”Soil Organic Matter in Dryland Ecosystems,” in The Future of Soil Carbon. Amsterdam: Elsevier, 39–70.
Pries, C. E. H., Castanha, C., Porras, R., and Torn, M. (2017). The whole-soil carbon flux in response to warming. Science 355, 1420–1423. doi: 10.1126/science.aal1319
Prommer, J., Walker, T. W., Wanek, W., Braun, J., Zezula, D., Hu, Y., et al. (2020). Increased microbial growth, biomass, and turnover drive soil organic carbon accumulation at higher plant diversity. Glob. Change Biol. 26, 669–681. doi: 10.1111/gcb.14777
Rillig, M. C., Aguilar−Trigueros, C. A., Bergmann, J., Verbruggen, E., Veresoglou, S. D., and Lehmann, A. (2015). Plant root and mycorrhizal fungal traits for understanding soil aggregation. N. Phytol. 205, 1385–1388. doi: 10.1111/nph.13045
Ruppert, J. C., Harmoney, K., Henkin, Z., Snyman, H. A., Sternberg, M., Willms, W., et al. (2015). Quantifying drylands’ drought resistance and recovery: The importance of drought intensity, dominant life history and grazing regime. Glob. Change Biol. 21, 258–1270. doi: 10.1111/gcb.12777
Ruppert, J. C., and Linstädter, A. (2014). Convergence between ANPP estimation methods in grasslands—A practical solution to the comparability dilemma. Ecol. Indicat. 36, 524–531. doi: 10.1016/j.ecolind.2013.09.008
Sanaullah, M., Rumpel, C., Charrier, X., and Chabbi, A. (2012). How does drought stress influence the decomposition of plant litter with contrasting quality in a grassland ecosystem? Plant Soil 352, 277–288. doi: 10.1007/s11104-011-0995-4
Sandhage-Hofmann, A., Kotzé, E., van Delden, L., Dominiak, M., Fouché, H. J., van der Westhuizen, H. C., et al. (2015). Rangeland management effects on soil properties in the savanna biome, South Africa: A case study along grazing gradients in communal and commercial farms. J. Arid Environ. 120, 14–25. doi: 10.1016/j.jaridenv.2015.04.004
Savadogo, P., Sawadogo, L., and Tiveau, D. (2007). Effects of grazing intensity and prescribed fire on soil physical and hydrological properties and pasture yield in the savanna woodlands of Burkina Faso. Agricult. Ecosys. Environ. 118, 80–92. doi: 10.1016/j.agee.2006.05.002
Schimel, J. P. (2018). Life in dry soils: effects of drought on soil microbial communities and processes. Annu. Rev. Ecol. Evol. Syst. 49, 409–432. doi: 10.1146/annurev-ecolsys-110617-062614
Scurlock, J. M., Johnson, K., and Olson, R. J. (2002). Estimating net primary productivity from grassland biomass dynamics measurements. Glob. Change Biol. 8, 736–753. doi: 10.1046/j.1365-2486.2002.00512.x
Shi, X.-M., Li, X. G., Li, C. T., Zhao, Y., Shang, Z. H., and Ma, Q. (2013). Grazing exclusion decreases soil organic C storage at an alpine grassland of the Qinghai–Tibetan Plateau. Ecol. Eng. 57, 183–187. doi: 10.1016/j.ecoleng.2013.04.032
Sun, J., Zhan, T., Liu, M., Zhang, Z., Wang, Y., Liu, S., et al. (2019). Verification of the biomass transfer hypothesis under moderate grazing across the Tibetan plateau: a meta-analysis. Netherland: Springer.
Suttie, J. M., Reynolds, S. G., and Batello, C. (2005). Grasslands of the World. Rome: Food & Agriculture Organisation.
van Verseveld, C. J. W., and Gebert, J. (2020). Effect of compaction and soil moisture on the effective permeability of sands for use in methane oxidation systems. Waste Manag. 107, 44–53. doi: 10.1016/j.wasman.2020.03.038
Vanlauwe, B., Six, J., Sanginga, N., and Adesina, A. (2015). Soil fertility decline at the base of rural poverty in sub-Saharan Africa. Nat. Plants 1:15101.
Wang, B., Wu, L., Chen, D., Wu, Y., Hu, S., Li, L., et al. (2020). Grazing simplifies soil micro−food webs and decouples their relationships with ecosystem functions in grasslands. Glob. Change Biol. 26, 960–970. doi: 10.1111/gcb.14841
Wang, Y., Wang, D., Shi, B., and Sun, W. (2020). Differential effects of grazing, water, and nitrogen addition on soil respiration and its components in a meadow steppe. Plant Soil 447, 581–598. doi: 10.1007/s11104-019-04410-5
WRB (2014). World Reference Base for Soil Resources 2014. International soil classification system for naming soils and creating legends for soil maps. Rome: FAO’s digital publishing.
Xu, L., Baldocchi, D. D., and Tang, J. (2004). How soil moisture, rain pulses, and growth alter the response of ecosystem respiration to temperature. Glob. Biogeochem. Cycles 18:2004.
Zhao, Y., Wang, M., Hu, S., Zhang, X., Ouyang, Z., Zhang, G., et al. (2018). Economics-and policy-driven organic carbon input enhancement dominates soil organic carbon accumulation in Chinese croplands. Proc. Natl. Acad. Sci. 115, 4045–4050. doi: 10.1073/pnas.1700292114
Zhou, G., Luo, Q., Chen, Y., He, M., Zhou, L., Frank, D., et al. (2019a). Effects of livestock grazing on grassland carbon storage and release override impacts associated with global climate change. Glob. Change Biol. 25, 1119–1132. doi: 10.1111/gcb.14533
Keywords: C stocks, CO2 flux, drought, dryland grasslands, grazing
Citation: Munjonji L, Ayisi KK, Mudongo EI, Mafeo TP, Behn K, Mokoka MV and Linstädter A (2020) Disentangling Drought and Grazing Effects on Soil Carbon Stocks and CO2 Fluxes in a Semi-Arid African Savanna. Front. Environ. Sci. 8:590665. doi: 10.3389/fenvs.2020.590665
Received: 02 August 2020; Accepted: 08 October 2020;
Published: 28 October 2020.
Edited by:
Lu-Jun Li, Northeast Institute of Geography and Agroecology (CAS), ChinaReviewed by:
Dima Chen, China Three Gorges University, ChinaCameron Carlyle, University of Alberta, Canada
Copyright © 2020 Munjonji, Ayisi, Mudongo, Mafeo, Behn, Mokoka and Linstädter. This is an open-access article distributed under the terms of the Creative Commons Attribution License (CC BY). The use, distribution or reproduction in other forums is permitted, provided the original author(s) and the copyright owner(s) are credited and that the original publication in this journal is cited, in accordance with accepted academic practice. No use, distribution or reproduction is permitted which does not comply with these terms.
*Correspondence: Lawrence Munjonji, TGF3cmVuY2UuTXVuam9uamlAdWwuYWMuemE=