- 1Forest Soils and Biogeochemistry, Swiss Federal Institute for Forest, Snow and Landscape Research (WSL), Birmensdorf, Switzerland
- 2Institute of Biogeochemistry and Pollutant Dynamics, Swiss Federal Institute of Technology, ETH Zürich, Zurich, Switzerland
- 3Laboratory for Mechanical Systems Engineering, Swiss Federal Laboratories for Materials Science and Technology (EMPA), Dübendorf, Switzerland
Plastic pollution poses a threat to terrestrial ecosystems, even impacting soils from remote alpine and arctic areas. Biodegradable plastics are a promising solution to prevent long-term accumulation of plastic litter. However, little is known about the decomposition of biodegradable plastics in soils from alpine and polar ecosystems or the microorganisms involved in the process. Plastics in aquatic environments have previously been shown to form a microbial community on the surface of the plastic distinct from that in the surrounding water, constituting the so-called “plastisphere.” Comparable studies in terrestrial environments are scarce. Here, we aimed to characterize the plastisphere microbiome of three types of plastics differing in their biodegradability in soil using DNA metabarcoding. Polylactic acid (PLA), polybutylene adipate terephthalate (PBAT), and polyethylene (PE) were buried in two different soils, from the Swiss Alps and from Northern Greenland, at 15°C for 8 weeks. While physico-chemical characteristics of the polymers only showed minor (PLA, PBAT) or no (PE) changes after incubation, a considerably lower α-diversity was observed on the plastic surfaces and prominent shifts occurred in the bacterial and fungal community structures between the plastisphere and the adjacent bulk soil not affected by the plastic. Effects on the plastisphere microbiome increased with greater biodegradability of the plastics, from PE to PLA. Copiotrophic taxa within the phyla Proteobacteria and Actinobacteria benefitted the most from plastic input. Especially taxa with a known potential to degrade xenobiotics, including Burkholderiales, Caulobacterales, Pseudomonas, Rhodococcus, and Streptomyces, thrived in the plastisphere of the Alpine and Arctic soils. In addition, Saccharimonadales (superphylum Patescibacteria) was identified as a key taxon associated with PLA. The association of Saccharibacteria with plastic has not been reported before, and pursuing this finding further may shed light on the lifestyle of this obscure candidate phylum. Plastic addition affected fungal taxa to a lesser extent since only few fungal genera such as Phlebia and Alternaria were increased on the plastisphere. Our findings suggest that the soil microbiome can be strongly influenced by plastic pollution in terrestrial cryoenvironments. Further research is required to fully understand microbial colonization on plastic surfaces and the biodegradation of plastic in soils.
Introduction
Plastic pollution is a threat to ecosystems all over the globe, with clear implications for animals like birds, marine mammals and fish (Secretariat of the Convention of Biological Diversity, 2016; Thiel et al., 2018). Additionally, plastic pollution potentially causes food safety problems (Wright and Kelly, 2017; Smith et al., 2018), leads to developmental, reproductive and metabolic disorders in invertebrates through the uptake of nano-sized particles (Shen et al., 2019), and even contributes to the spread of pathogens and antibiotic resistance by serving as a raft for microorganisms (Keswani et al., 2016; Laganà et al., 2019). Most of the attention in research is paid to plastic debris in oceans, while knowledge about the occurrence, fate and potential hazards of landfill waste is scarce and its impact is likely underestimated considerably (de Souza Machado et al., 2018). Yet, some studies have examined the quantity of synthetic polymers in terrestrial ecosystems (Piehl et al., 2018; Scheurer and Bigalke, 2018; Zhang and Liu, 2018) and have documented detrimental effects of plastics in soils on earthworms (Huerta Lwanga et al., 2016) and microorganisms (Wang et al., 2016), as well as implications for agricultural productivity (Rillig et al., 2019). It is estimated that the amount of plastic released into terrestrial environments is 4–23 times higher than into marine environments (Horton et al., 2017). Plastics can reach habitats not only by direct littering and transport through rivers and oceans, but also by transport through the atmosphere and deposition in pristine regions such as alpine and Arctic environments (Allen et al., 2019). Plastic pollution has even been reported in remote areas of the Swiss Alps and the Arctic (Ambrosini et al., 2019; Bergmann et al., 2019).
A possible way to deal with the plastic pollution in the environment is to develop biodegradable plastics. However, they make up only around 1% of the total plastic currently being produced (PlasticsEurope, 2018). In addition, very little is known about the fate of biodegradable plastics in terms of microbial colonization and their degradability, in particular in soils from cold environments since new materials are usually tested for decomposition at temperatures above 20°C (Briassoulis and Dejean, 2010). In one study on the microbial degradation of plastic in temperate, marine sediment, there were no signs of biodegradation for both polyethylene (PE) and biodegradable carrier bags after a 3-month incubation at 10°C (Nauendorf et al., 2016). A second study showed a weight loss of 37% for biodegradable plastic bags incubated in agricultural soil in the lab for 3 months at 25°C (Accinelli et al., 2012). However, in the same study samples incubated in the field under natural conditions were far less prone to biodegradation. Given that the rate of biodegradation of plastics, besides of intrinsic material parameters, largely depends on external factors like temperature, moisture, and UV radiation (O’Brine and Thompson, 2010; Wilkes and Aristilde, 2017), biodegradation in soils of cold regions is assumed to be rather slow. The resident microbiome might be another critical parameter determining the biodegradability of plastics in soils. The composition of the soil microbiome strongly depends on various climatic and soil variables (Fierer, 2017). Alpine and Arctic soils are low in carbon and nitrogen content, and knowledge of their microbial communities is limited (Frasson et al., 2015; Frey et al., 2016; Malard and Pearce, 2018; Adamczyk et al., 2019; Pontes et al., 2020; Pushkareva et al., 2020). Plastic deposition may introduce a carbon and energy source into oligotrophic soils, possibly leading to profound changes in the soil microbiome and its functions in alpine and Arctic ecosystems. However, it has not been investigated whether the enzymatic ability to degrade plastics is pervasive in all soils or whether, especially in remote soils that have only recently been affected by pollution, plastic-degrading microorganisms are lacking.
The term “plastisphere” was introduced to describe the diverse microbial community influenced by plastic surfaces, analogous to the rhizosphere surrounding plant roots (Zettler et al., 2013; Amaral-Zettler et al., 2020). Since then, researchers have analyzed the composition of this novel habitat in many studies from aquatic environments and have shown that the plastisphere selects for particular microbial communities that differ from those in the surrounding environment (De Tender et al., 2015; Oberbeckmann et al., 2018; Ogonowski et al., 2018; Kirstein et al., 2019) and from communities on other inert surfaces like glass (Kirstein et al., 2018). However, data about the plastisphere microbiome in terrestrial ecosystems are scarce, and to our knowledge plastic-colonizing microbes in soils from cold environments have not previously been studied with culture-independent approaches to cover the entire microbial community on the plastic surface.
In the present mesocosm experiment, we buried pieces of three different plastic types in two different top soils, from the Swiss Alps and from Northern Greenland, at 15°C for 8 weeks. We used polylactic acid (PLA), polybutylene adipate terephthalate (PBAT), and PE, plastics that vary in their biodegradability. We characterized the plastisphere microbiome of the three types of plastics using soil DNA metabarcoding. We aimed to determine: (1) whether different polymer types are colonized by different microbial communities; (2) whether the plastisphere microbiome is different in the two soils; and (3) whether the plastisphere microbial communities can be linked to the biodegradability of the tested plastics. We hypothesized that the plastic material would determine the associated plastisphere microbiome, and that shifts in the plastisphere microbial communities compared to in bulk soil would be smallest in PE, which has the lowest biodegradability.
Materials and Methods
Plastic Types
Three different types of plastic were used in the experiments. The first specimen, purchased at Coop AG (Basel, Switzerland), was a compostable plastic bag made of Ecovio, a plastic blend primarily made of PLA. The second specimen was an agricultural mulch foil, purchased at Oeremansplastic (Genderen, Netherlands), made of PBAT. While PLA is made of renewable plant sources, PBAT is fabricated from fossil fuels. The third specimen was a common non-biodegradable waste bag made of low-density PE, purchased from TopPac (Schwarzenbach, Switzerland). The exact composition of the plastics was not known, as they are commercially available products. The plastics were chosen based on their different expected biodegradabilities, ranging from high biodegradability (PLA) to medium biodegradability (PBAT) to very low biodegradability (PE). The plastic surfaces were not sterilized to avoid chemical changes of the polymers. However, we surveyed the surfaces of non-incubated plastic pieces for the presence of indigenous microorganisms by plating 4 × 4 cm pieces of plastic on R2A (Carl Roth GmbH + Co., Karlsruhe, Germany) and LB (Merck KGaA, Darmstadt, Germany) agar plates and were only able to obtain 0–2 colonies per piece of plastic.
Soils Used for Incubation
We used two different top soils (0–10 soil depth), one from an alpine and one from an Arctic environment. The alpine soil was collected on the northwestern flank of “Muot da Barba Peider” (Barba Peider) in the eastern Swiss Alps at 2979 m a.s.l. (Frey et al., 2016). The Arctic soil was sampled in Villum at Station Nord in Northern Greenland. The coordinates, soil characteristics and climatic parameters of the two soils used are given in Table 1. We sampled three independent locations (field replications) for each soil type and replicated them in the mesocosm experiment (see below). Soils were transported in cold boxes to the laboratory and stored in closed plastic bags at 4°C in the dark until the start of the incubation experiment (less than 3 months).
Experimental Set-Up
Half of the soil collected from each site was autoclaved (1× at 120°C for 20 min) to produce sterile controls for detecting possible abiotic degradation of the plastics. Sterilization of the investigated soils was confirmed by the lack of cultivable microorganisms. Incubation mesocosms were then prepared using 100 ml beaker glasses containing approximately 80 g of soil, with three replicates for each soil origin (Barba Peider, Villum), treatment (natural soil, autoclaved control) and plastic type (PLA, PBAT, PE) (36 beakers in total). Prior to incubation, the plastic pieces (4 × 4 cm) were quickly submerged in a soil suspension for 1 min to ensure contact of the indigenous microbial community of the respective soil with the plastic surface. The suspension was prepared by shaking 10 g of the corresponding soil in 40 ml of sterile dH2O for 60 min. Plastic pieces for sterile controls were submerged in sterile dH2O instead of the suspension. The beaker glasses were covered by parafilm and incubated at 15°C in the dark for 8 weeks. The incubation temperature represents the maximum temperature in the topsoils at both sites during snow-free season. The moisture content was determined gravimetrically and balanced once a week by adding sterile dH2O.
At the end of the incubation experiment, samples were prepared for further processing. Bulk soil was collected from a distance of >2 cm to the incubated plastic sample. For the plastisphere samples, small soil particles adherent to the plastic piece were manually scratched off and added to the extraction tube. In addition, 4−5 small pieces of plastic (1 × 2 mm) were added to the extraction tube. For bulk soil samples approximately 250 mg fresh weight was used for DNA extraction, while for plastisphere samples only 100 mg fresh weight could be used due to the low mass of soil adherent to the plastic surfaces. Samples were added into extraction tubes and were frozen at −20°C until DNA extraction was performed. Small pieces of plastic (1 × 2 mm) were added to 5 ml Falcon tubes containing sterile tap water for cultivation of microorganisms. The remaining material from each plastic piece was rinsed with sterile dH2O to provide a clean surface and dried at 35°C for 4 days prior to the physico-chemical analysis.
Cultivation of Microorganisms
The 5 ml Falcon tubes containing the plastic pieces were shaken with a vortex for 10 min at half speed. The resulting cell suspensions were serially diluted 1:10, 1:100, and 1:1000 in sterile tap water. All dilutions were plated in duplicate on R2A agar plates (Carl Roth GmbH + Co., Karlsruhe, Germany). The agar plates were incubated at 15°C for 2 months and growing colonies were picked once a week with a sterile tooth pick. Strains were phylogenetically characterized by sanger Sequencing as recently outlined (Lapanje et al., 2012; Brunner et al., 2018). Briefly, the 16S rDNA (bacteria) and ITS (fungi) regions of isolated organisms were amplified by colony PCR. For bacteria universal primers 27F (Heuer et al., 1997) and 907R (Muyzer et al., 1995) were used. For fungi, primers ITS1 and ITS4 (White et al., 1990) were used. PCR products were sent to Macrogen B.V. (Amsterdam, Netherlands) for sequencing. Retrieved sequences were trimmed to the high quality portion and closest related sequences were searched on the NCBI nucleotide database with the blastn algorithm and default parameters.
Light Microscopy of Plastic Surfaces
Washed plastic pieces were analyzed with a VHX-500f digital microscope (Keyence International, Mechelen, Belgium). Images were acquired with a 5× and a 50× magnification.
Physico-Chemical Analysis
Fourier-transform infrared spectroscopy (FTIR) was performed with a Tensor 27 spectrometer (Bruker, Billerica, MA, United States). Spectra were obtained with a diamond Attenuated Total Reflectance (ATR) crystal plate. For each sample, 32 scans were recorded with wavelengths in the range of 4000–650 cm–1 for the analysis of Barba Peider soil and 4000–320 cm–1 for Villum soil. The scans were recorded with a spectral resolution of 4 cm–1, and the resulting spectra averaged. OPUS software (Winterthur, Switzerland) was used to collect and correct the data (baseline correction). As a standard, three measurements per replicate were performed. Carbonyl indices were used to evaluate the degradation of plastics by semi-quantitative data. The indices were calculated with the OPUS software (Winterthur, Switzerland) using the height of the peaks (K algorithm). With this technique it is possible to calculate the ratio between C = O and C-H chemical bonds (AC = O/AC–H). Depending on the plastic type, different wavelengths were chosen for the carbonyl index calculations (CIPLA = A1713/A1456; CIPBAT = A1713/A729). No carbonyl index calculations were possible for PE because no C = O bonds were detected in the incubated samples.
Contact angle analysis was used to determine the wettability of plastic surfaces by measuring the contact angle of a sitting drop. For this purpose a Drop Shape Analyzer DSA30 (Krüss, Hamburg, Germany) was used. A 2 μl drop of distilled water was placed on the plastic surface, and the contact angle was determined using the Krüss Advance software package with the Ellipse fitting method. The contact angle was measured ten times per drop at three different locations per replicate. The values obtained for the ten drops per location were averaged in advance to statistical analysis. This method was applied for all plastic types. Cleaned specimens from the mesocosm experiments were compared to plastic controls without prior incubation in the soils and to the controls incubated in sterilized soil.
DNA Extraction, PCR Amplification, and Illumina MiSeq Sequencing
DNA extraction was performed with the DNeasy PowerSoil kit (Qiagen, Hilden, Germany) according to the manufacturer’s protocol. DNA was quantified using the QubitTM high-sensitivity assay for double stranded DNA (Thermo Fisher Scientific, Waltham, MA, United States). DNA concentrations for sterile controls were below the detection limit. The V3–V4 region of the prokaryotic small-subunit (16S) rRNA gene (bacteria and archaea) and the internal transcribed spacer region 2 (ITS2) of the eukaryotic (fungal groups and some groups of protists and green algae) ribosomal operon were PCR amplified from 5 ng of DNA template, using primers and conditions previously described (Frey et al., 2016). PCRs of individual samples were run in duplicate, pooled, and purified using Agencourt Ampure XP (Beckman Coulter). Prokaryotic and fungal amplicon pools were sent to the Génome Québec Innovation Centre at McGill University (Montreal, QC, Canada) for barcoding using the Fluidigm Access Array technology (Fluidigm) and paired-end sequencing on the Illumina MiSeq v3 platform (Illumina Inc., San Diego, CA, United States).
Sequence Quality Control, OTU Clustering, and Taxonomic Assignments
Quality filtering, OTU clustering, and assignment were conducted similarly to methods described previously (Frey et al., 2016; Luláková et al., 2019) using a customized pipeline based on UPARSE (Edgar, 2013). Briefly, paired-end reads were merged using the fastq_mergepairs algorithm (Edgar and Flyvbjerg, 2015), filtering for sequences with a minimum length of 300 bp (16SV3V4) or 200 bp (ITS2) and a minimum overlap of 50 bp. PCR primers were removed using Cutadapt (Martin, 2011) allowing for a maximum of one mismatch in the forward and reverse primer. Next, reads were quality filtered using the USEARCH fastq_filter function, discarding reads with an expected error of one or greater. De-replicated sequences were clustered into OTUs at 97% sequence identity using the cluster_otu function. The clustering step includes an “on the fly” chimera removal algorithm. The OTU centroid sequences were then filtered for the presence of ribosomal signatures using Metaxa2 (Bengtsson-Palme et al., 2015) or ITSx (Bengtsson-Palme et al., 2013). Subsequently, sequences were mapped back on the OTU centroid sequences and taxonomic classification was conducted using a naïve Bayesian classifier (Wang et al., 2007) implemented in Mothur (Schloss et al., 2009) with a minimum bootstrap support of 0.6. Prokaryotic 16SV3V4 sequences were queried against the SILVA v132 database (Quast et al., 2013), whereas eukaryotic ITS2 sequences were queried against the fungal ITS database UNITE v8.0 (Nilsson et al., 2019). Prokaryotic sequences identified as originating from organelles (chloroplast, mitochondria), as well as eukaryotic sequences identified as originating from soil animals (metazoa) or plants (viridiplantae) were not included in the analyses. Raw sequences were deposited in the NCBI Sequence Read Archive under the accession number PRJNA630025.
Statistical Analyses
Results from all statistical tests performed in this study were considered significant at P < 0.05 unless indicated otherwise. Unless indicated otherwise, all figures were created with the ggplot2 package (Wickham, 2016) implemented in R Development Core Team (2008). Differences in contact angle and carbonyl index data were assessed using factorial ANOVA and Tukey’s HSD post hoc test, implemented in R. OTU richness (Sobs) and Shannon index (H’) were retrieved with the estimate_richness function implemented in the phyloseq package in R (McMurdie and Holmes, 2013). Differences in soil Sobs and H’ data were assessed using factorial ANOVAs and paired t-tests implemented in StatView, 2nd edition (SAS Institute Inc., Cary, NC, United States).
Principal coordinate analysis (PCoA) of microbial β-diversity was performed using the ordinate function based on Bray-Curtis dissimilarities and plotted using the plot_ordination function, both implemented in the phyloseq package in R (McMurdie and Holmes, 2013). Differences in β-diversity were tested by permutational multivariate analysis of variance (Anderson, 2001) and pairwise PERMANOVA functions in PRIMER version 7 (Clarke and Gorley, 2006) based on 105 permutations (Hartmann et al., 2017). For the pairwise tests, the Monte Carlo approximated level of significance [P(MC)] was determined.
Relative abundances of the most abundant taxa were assessed in R by agglomerating OTUs on phylum and order levels using the tax_glom function implemented in the phyloseq package (McMurdie and Holmes, 2013), normalizing the abundances using the function normalise_data from the microbiomeSeq package (Ssekagiri, 2020) and using the function aggregate_top_taxa implemented in the microbiome package in R (Lahti and Shetty, 2019). Differences in relative abundance of the most abundant taxa were assessed using factorial ANOVAs and Tukey’s HSD post hoc tests implemented in R. Relative abundances of taxa were plotted using the function plot_taxa implemented in the microbiomeSeq package (Ssekagiri, 2020).
Differential abundance analysis was performed using the differential_abundance function implemented in the microbiomeSeq package in R (Ssekagiri, 2020). First, the analysis was carried out using OTUs as a taxonomic classifier. In a second analysis, OTUs were aggregated to the next highest classifiable taxonomic rank for bacteria (mostly genus). For fungi, only sequences classifiable to the genus level were considered in the second analysis. In order to assess which OTUs/taxa were significantly affected by plastic type, we calculated log2-fold changes relative to the bulk samples using DESeq2 (Love et al., 2014). OTUs and taxa were considered significantly different (Wald test) between plastisphere and bulk soil samples if the false discovery rate (adjusted P) was < 0.05.
Results
Physico-Chemical Measurements of Polymer Degradation
After 8 weeks of incubation in the Alpine (Barba Peider) or Arctic (Villum) soil, no clear signs of degradation were observed in any of the three plastic types (PLA, PBAT, and PE). However, PLA incubated in natural soil was covered with white stains, some of which appeared cloudy and others mycelium-like (Supplementary Figure 1).
Carbonyl indices of PLA and PBAT were significantly affected by both incubation and soil type without interactions (Table 2a). The contact angle of PBAT was significantly affected by incubation, soil type and their interactions, whereas PE was not influenced by incubation or soil type. In contrast, PLA was affected by soil type and interactions between incubation and soil type (Table 2a).
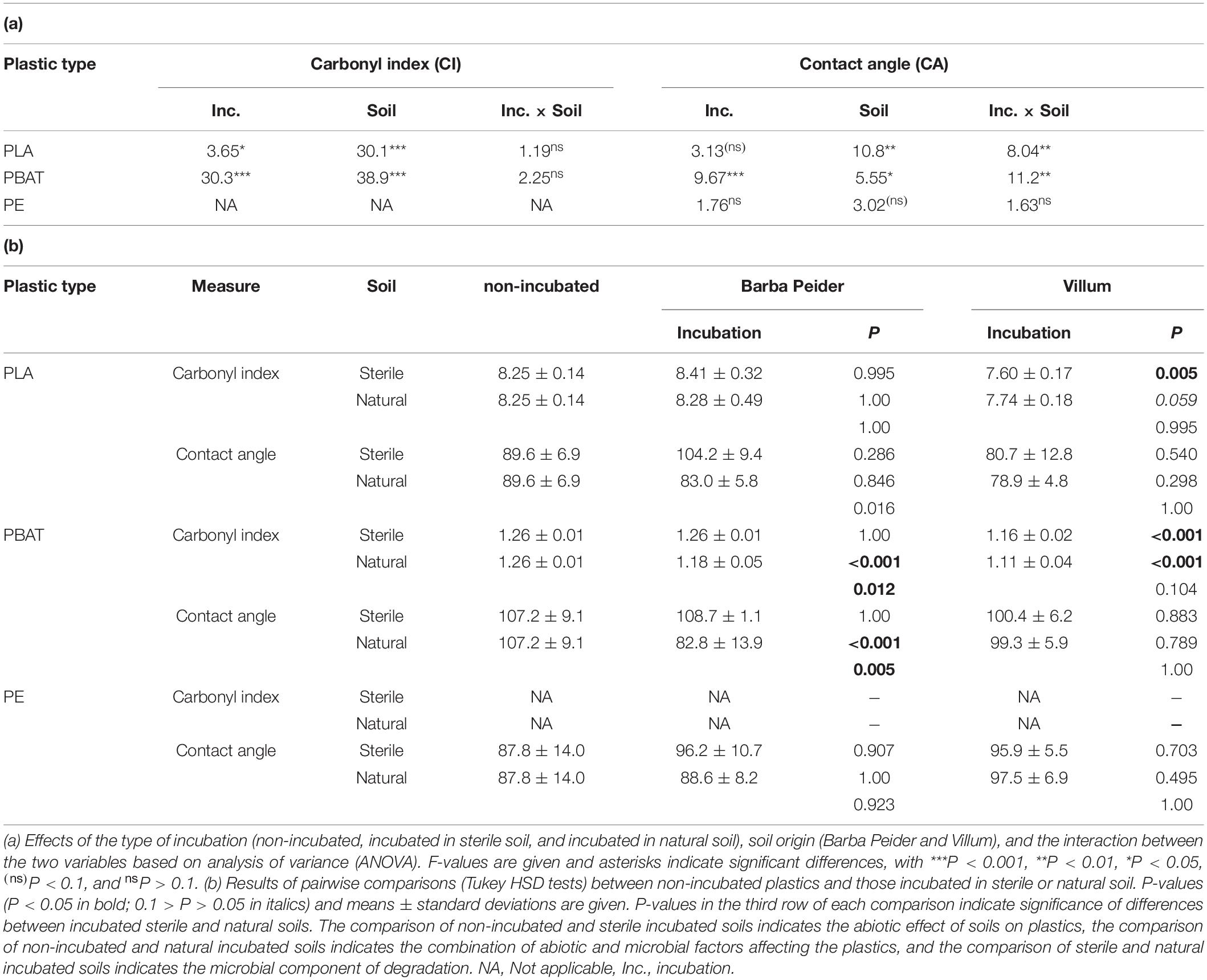
Table 2. Physico-chemical characterization of plastics before and after an 8-week incubation in autoclaved (sterile) and natural (non-sterile) soil from Barba Peider or Villum.
In Barba Peider soil, the contact angle of PLA incubated in natural-soil was significantly different than that incubated in sterile soil (Table 2b). However, neither of these incubated PLAs differed from the non-incubated PLA regarding contact angle. For PLA in Villum soil, only the difference between the carbonyl index of sterile-soil incubated and non-incubated plastics was statistically significant. However, the carbonyl index of PLA incubated in natural soil was very similar to that of the sterile-soil incubated samples but lower than the non-incubated PLA (P = 0.059).
In Barba Peider soil, the carbonyl index and the contact angle of PBAT from the 8-week incubation in natural soil were significantly different from the non-incubated sample, as well as from PBAT incubated in sterile soil (Table 2b). In contrast, the carbonyl index of natural-soil and sterile-soil incubated samples in Villum soil was significantly different from non-incubated PBAT, but no difference was detected between natural-soil and sterile-soil incubated PBAT. No difference was detected for the contact angle of non-incubated, sterile-soil incubated and natural-soil incubated PBAT.
Polymer-Dependent Microbiome
Sequencing of Barba Peider samples yielded a total of 1,169,088 (64,949 ± 4,606 per sample; mean ± standard error) prokaryotic 16SV3V4 and 335,637 (18,647 ± 394) eukaryotic ITS2 high-quality sequences that were clustered into 2,360 and 334 OTUs, respectively. Samples from Villum yielded a total of 412,004 (22,889 ± 851 per sample) prokaryotic 16SV3V4 and 1,007,107 (55,950 ± 3,404) eukaryotic ITS2 high-quality sequences that were clustered into 2,918 and 280 OTUs, respectively.
Bacterial and Fungal α-Diversity
We estimated the prokaryotic and fungal α-diversity of plastisphere and bulk soil samples in Barba Peider and Villum soils by analyzing the observed OTU richness (Sobs) and Shannon indices (H’). ANOVA of plastisphere and bulk soil samples showed significant differences for all α-diversity measures except for prokaryotic Sobs in Barba Peider soil (Table 3a). By pairwise comparisons of plastisphere and bulk soil of each plastic and soil type, we observed a significant reduction in prokaryotic H’ in both soils in the PLA plastisphere when compared with the bulk soil (Table 3b). Similar trends were observed for Sobs (Figure 1). In addition, prokaryotic Sobs was significantly lower in the PE plastisphere when compared with the bulk soil in the Villum samples. Fungal α-diversity was significantly lower in the PLA plastisphere than in the bulk soil in samples from Barba Peider, and the same trend was true for Villum samples (Figure 2). Fungal α-diversity was lower in the plastisphere of PBAT compared with the bulk soil in the Villum samples, but no such effects of PBAT were found for Barba Peider samples. Furthermore, fungal Sobs of the plastisphere of PE was lower compared with the bulk soil in Villum samples.
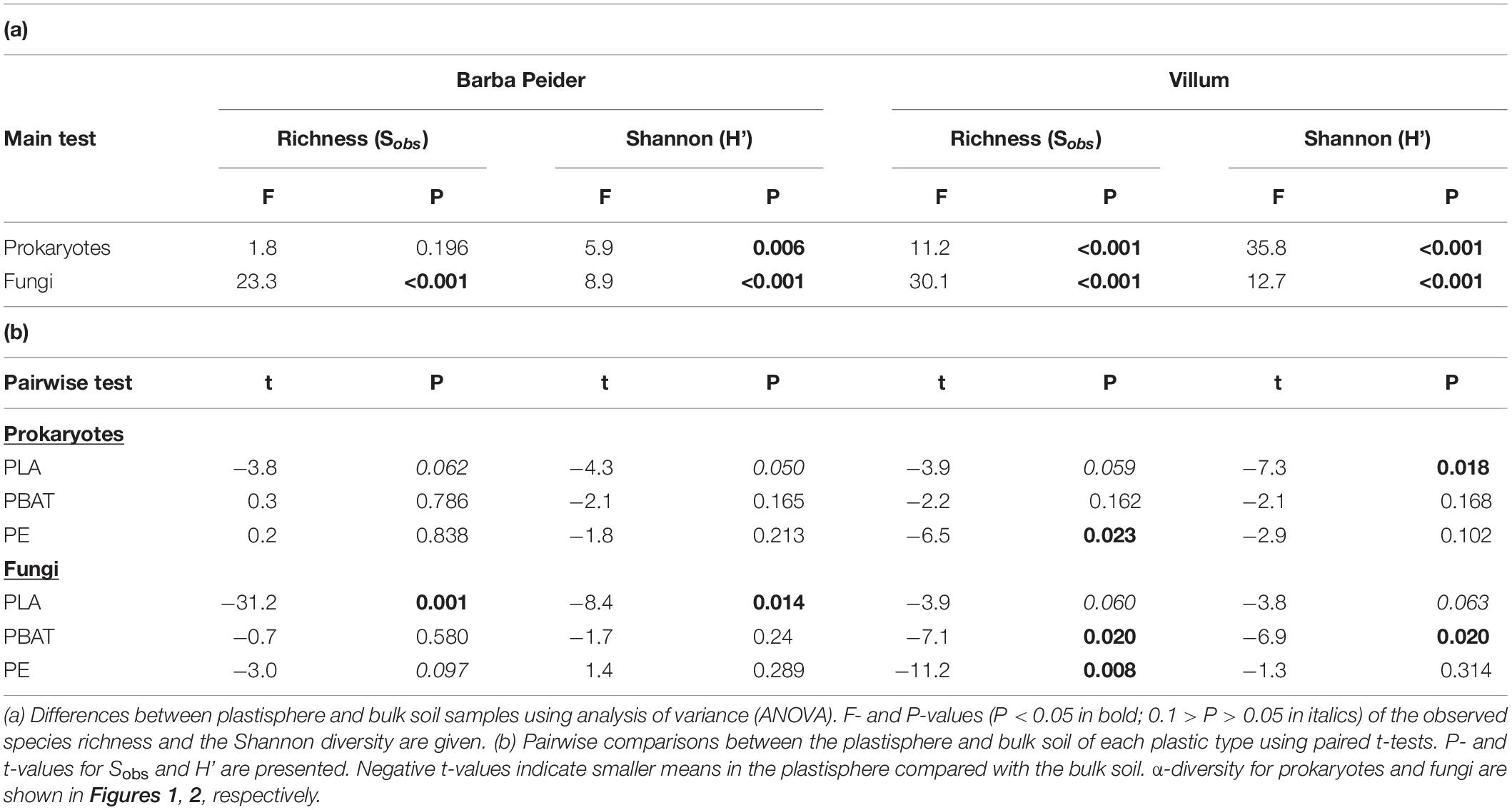
Table 3. α-diversity of prokaryotes and fungi in the plastisphere and bulk soil of Barba Peider and Villum soils.
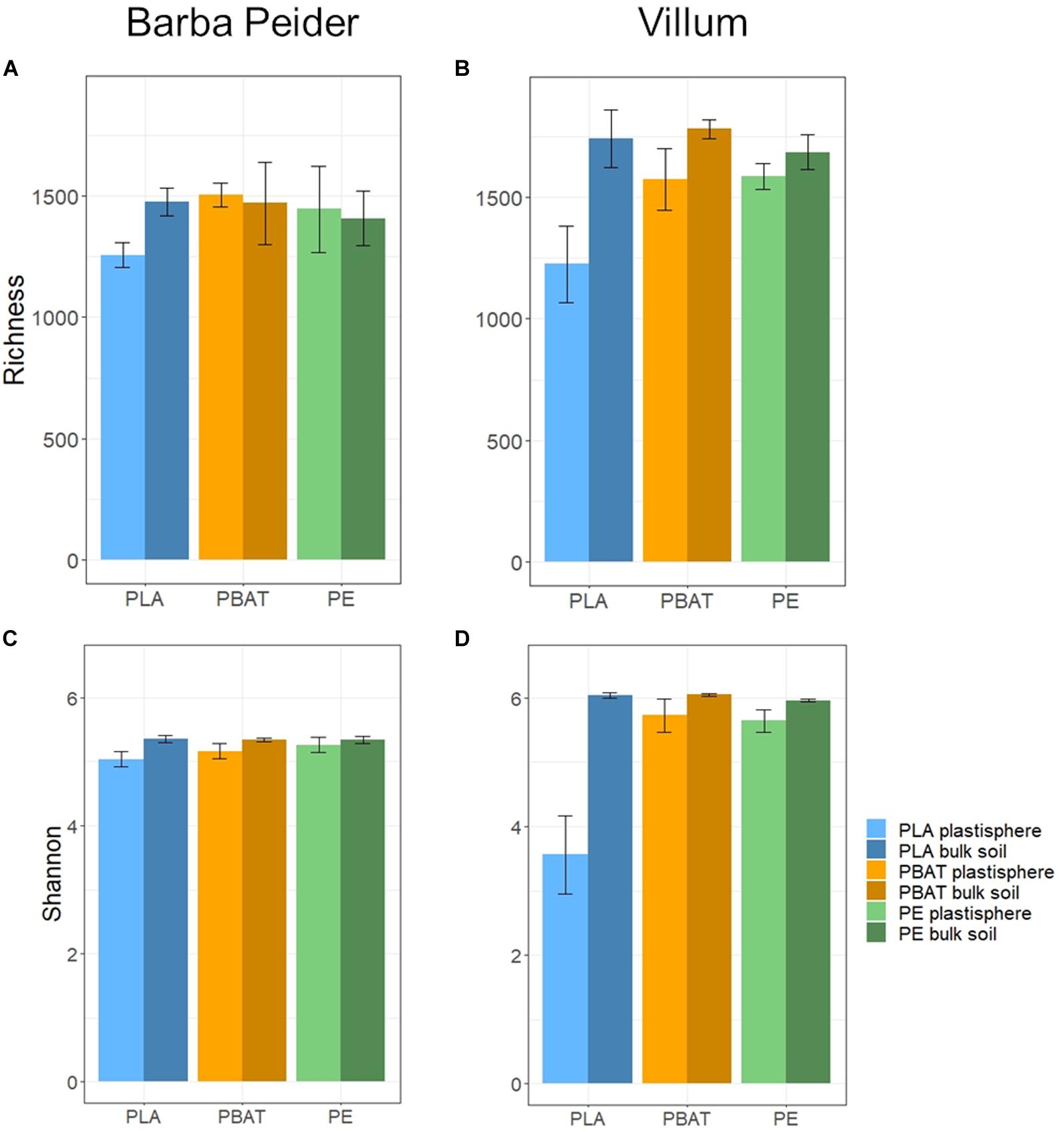
Figure 1. Prokaryotic α-diversity (mean ± sd; n = 3) of plastisphere and bulk soil samples showing observed richness (A,B) and Shannon Index (C,D) in Barba Peider (Swiss Alps) (A,C) and Villum (Northern Greenland) (B,D) soil.
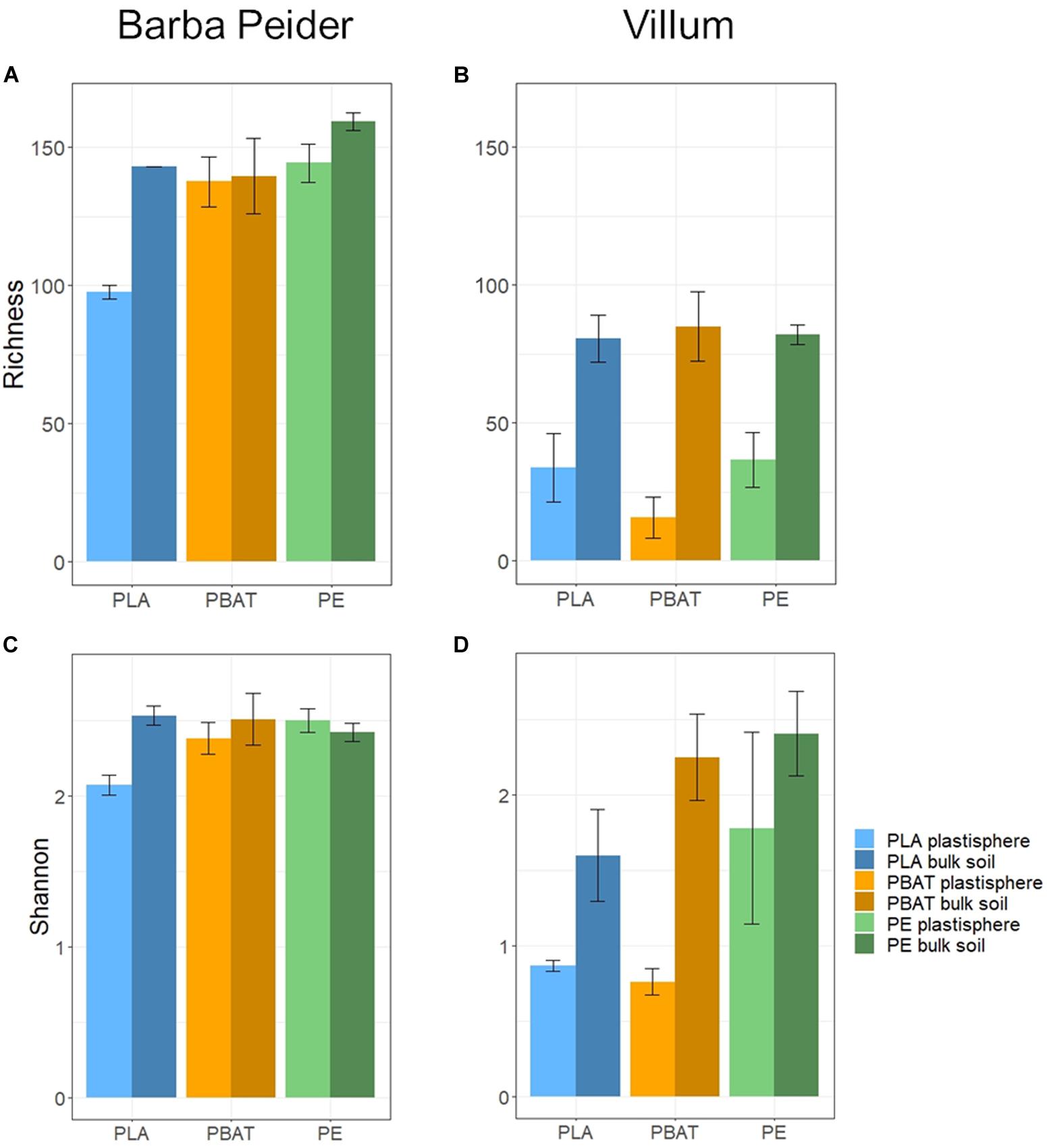
Figure 2. Fungal α-diversity (mean ± sd; n = 3) of plastisphere and bulk soil samples showing observed richness (A,B) and Shannon Index (C,D) in Barba Peider (Swiss Alps) (A,C) and Villum (Northern Greenland) (B,D) soil.
Bacterial and Fungal β-Diversity
Principal coordinate analysis and permutational analysis of variance (PERMANOVA) revealed that microbial community structures in the PLA plastisphere microbiome were significantly distinct from the microbial communities in the bulk soil from both sampling sites (Figure 3 and Table 4). In addition, PBAT plastisphere microbial community structures were significantly distinct from the microbiome in the bulk soil in Villum samples, but this was not true for samples from Barba Peider. In contrast, PE plastisphere community structures did not differ significantly from the bulk soil in samples from both sites. Bulk soil in samples with the different plastic types did not differ significantly regarding community structures (data not shown).
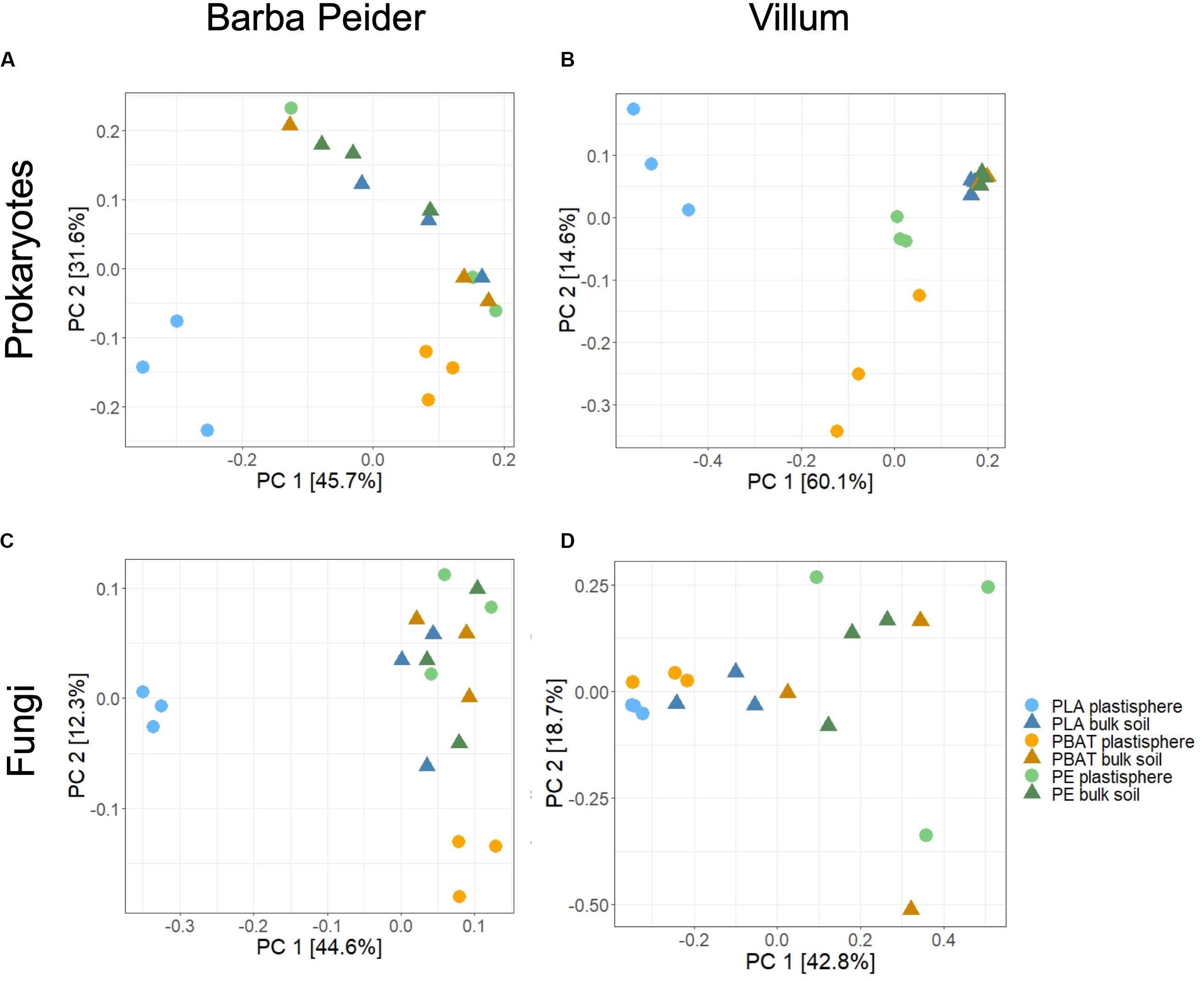
Figure 3. Differences in β-diversity between plastisphere and bulk soil samples of the three plastic types, visualized by principal coordinate analysis (PCoA) based on Bray-Curtis dissimilarities. The variance explained by each PC axis is given in parentheses, and quantitative statistical results based on PERMANOVA are provided in Table 4. Results are displayed for prokaryotes (A,B) and fungi (C,D) in Barba Peider (Swiss Alps) (A,C) and Villum (Northern Greenland) (B,D) soils.
“Plastisphere Taxa”
Relative Abundances of Microbial Taxa
The search for taxa at the phylum and order level that are affected by the plastics revealed that most community structural changes occurred in the PLA plastisphere at both taxonomic levels and in both soils (Figure 4, Table 5, and Supplementary Figure 2). Bacterial phyla that increased in relative abundance in the plastisphere of PLA were Actinobacteria, Proteobacteria and Patescibacteria in Barba Peider and only Patescibacteria in Villum. Mainly Acidobacteria, Chloroflexi, Gemmatimonadetes, Planctomycetes, and Nitrospirae decreased in relative abundance in the PLA plastisphere in both soils. β-Proteobacteriales, Rhizobiales, and Saccharimonadales (superphylum Patescibacteria) increased in abundance in the plastisphere of Barba Peider, whereas in Villum only the latter was enriched. Archaea were not affected by any of the plastic types. Fungal phyla and orders were largely unaffected by PLA (Table 5 and Supplementary Figures 3, 4). The only changes in the plastisphere of PLA in Barba Peider soil were a decrease in the phylum Mortierellomycota and an increase in the order Thelebolales. The only phyla enriched in the plastisphere of PBAT were Proteobacteria in Villum soil and Mortierellomycota in Barba Peider soil (Table 5 and Supplementary Figures 2, 3). In Villum, only the orders β-Proteobacteriales and Rhizobiales were significantly increased in relative abundance in the plastisphere of PBAT, whereas only Helotiales were favored in Barba Peider (Figure 4, Table 5, and Supplementary Figure 3). In the PE plastisphere of both soils, the only significant increase in relative abundance was observed for Actinobacteria and the associated order of Propionibacteriales (Figure 4, Table 5, and Supplementary Figure 2).
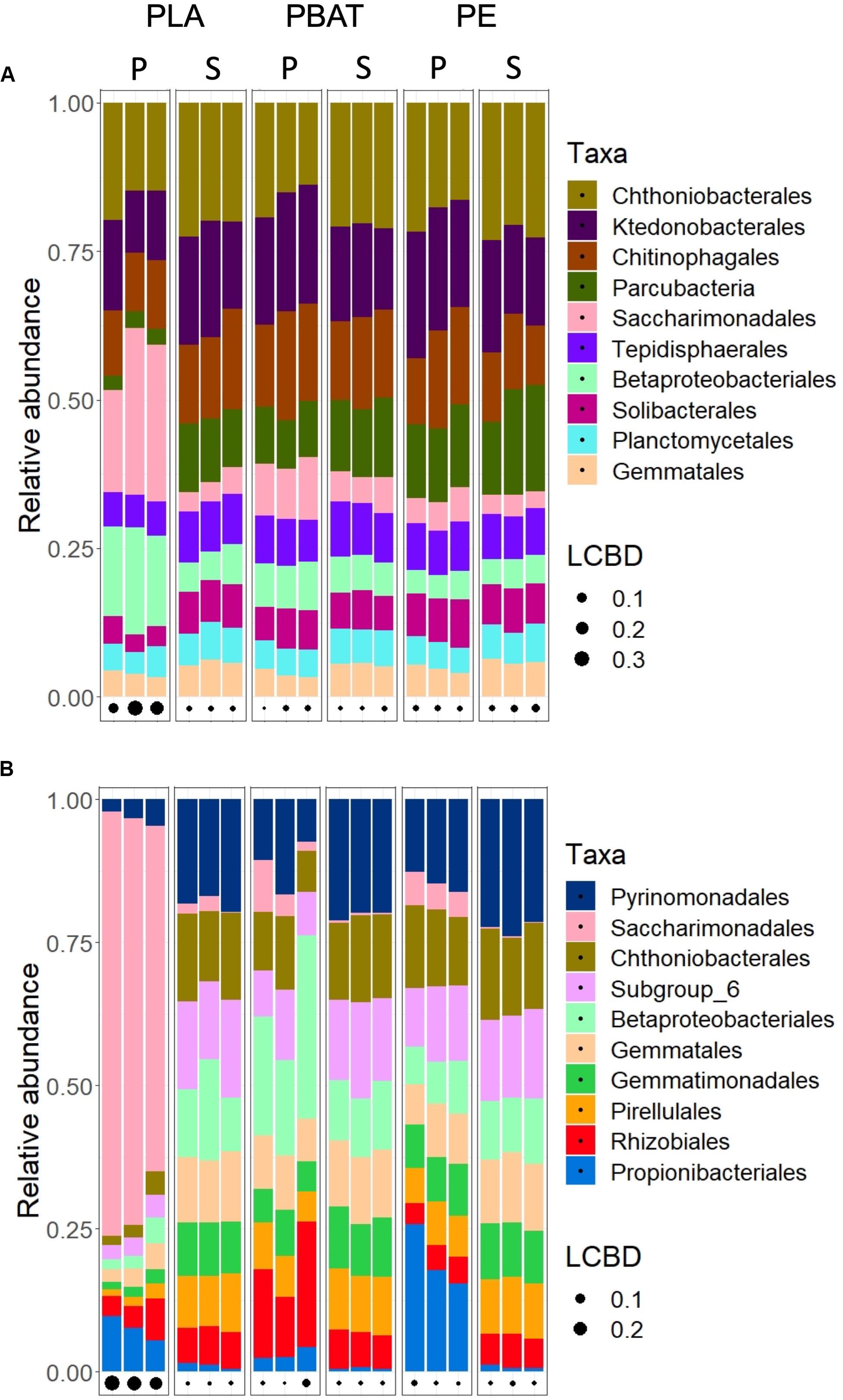
Figure 4. Relative abundance of the ten most abundant bacterial orders in the plastisphere (P) and bulk soil (S) of Barba Peider (Swiss Alps) (A) and Villum (Northern Greenland) (B) soils. The three replicates for each habitat are shown as separate columns. LCBD, local contribution to β-diversity.
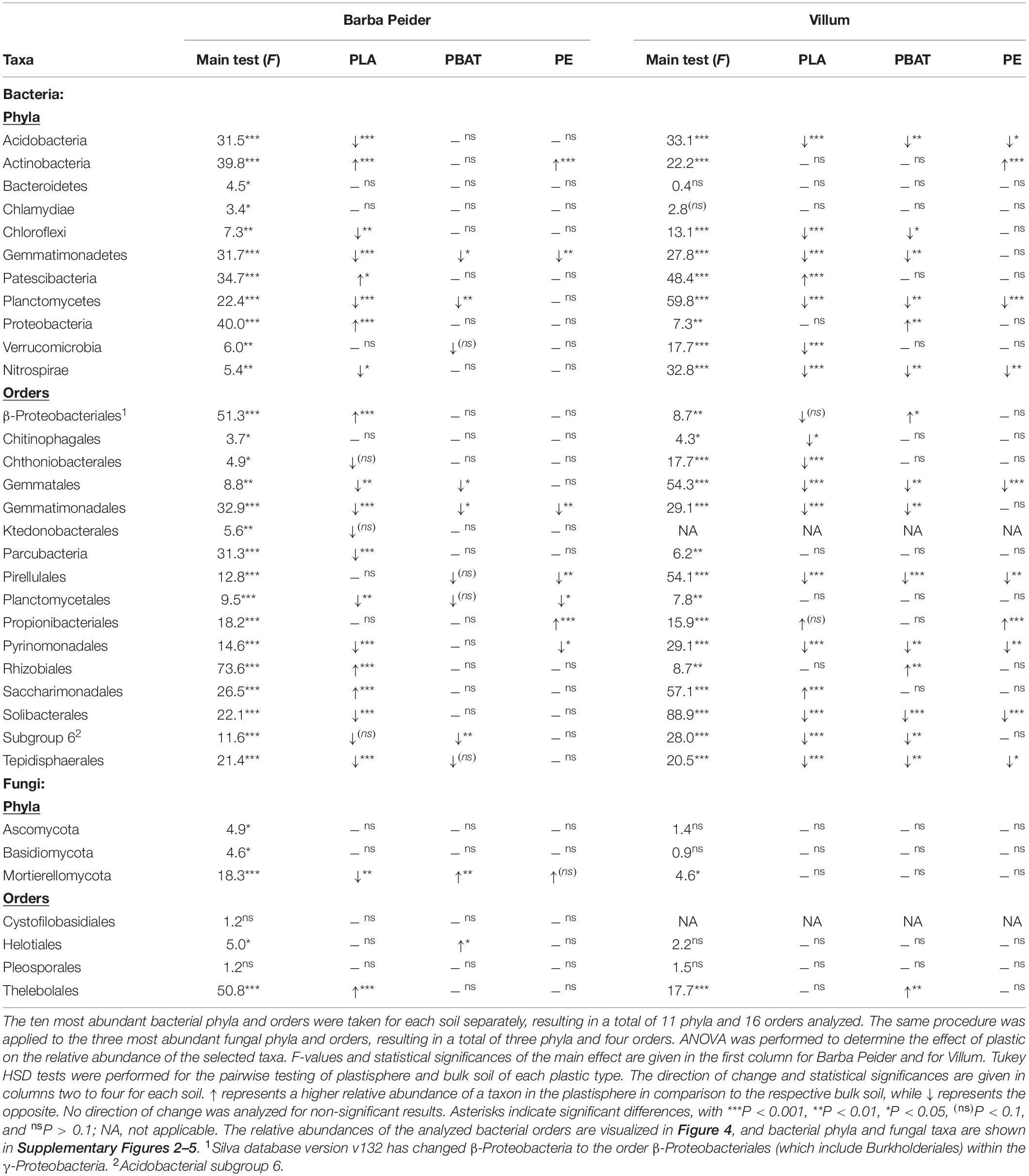
Table 5. Changes in the relative abundance of prokaryotic and fungal phyla and orders in the plastisphere.
Specific “Plastisphere Taxa”
Differential abundance analysis was performed by comparing total abundances of OTUs in plastisphere and bulk soil samples. The number of differentially abundant OTUs for both prokaryotes and fungi in both soils was highest for PLA, followed by PBAT and lastly PE (Supplementary Table 1). Whereas more bacterial OTUs showed positive log2-fold changes in the plastisphere compared with bulk soils, a larger portion of the fungal OTUs in Villum soil showed negative log2-fold changes in the plastisphere compared with bulk soils.
A repetition of the differential abundance analysis with OTUs aggregated to the lowest classifiable taxonomic level (mostly genus) revealed the bacterial taxa most strongly affected by the different plastics, as shown in Figures 5–7. Most taxa with significant log2-fold changes belonged to the phyla Proteobacteria and Actinobacteria, and only few exceptions were identified (Bacteroidetes, Verrucomicrobia, and Patescibacteria). No archaeal taxa were significantly influenced by the plastics.
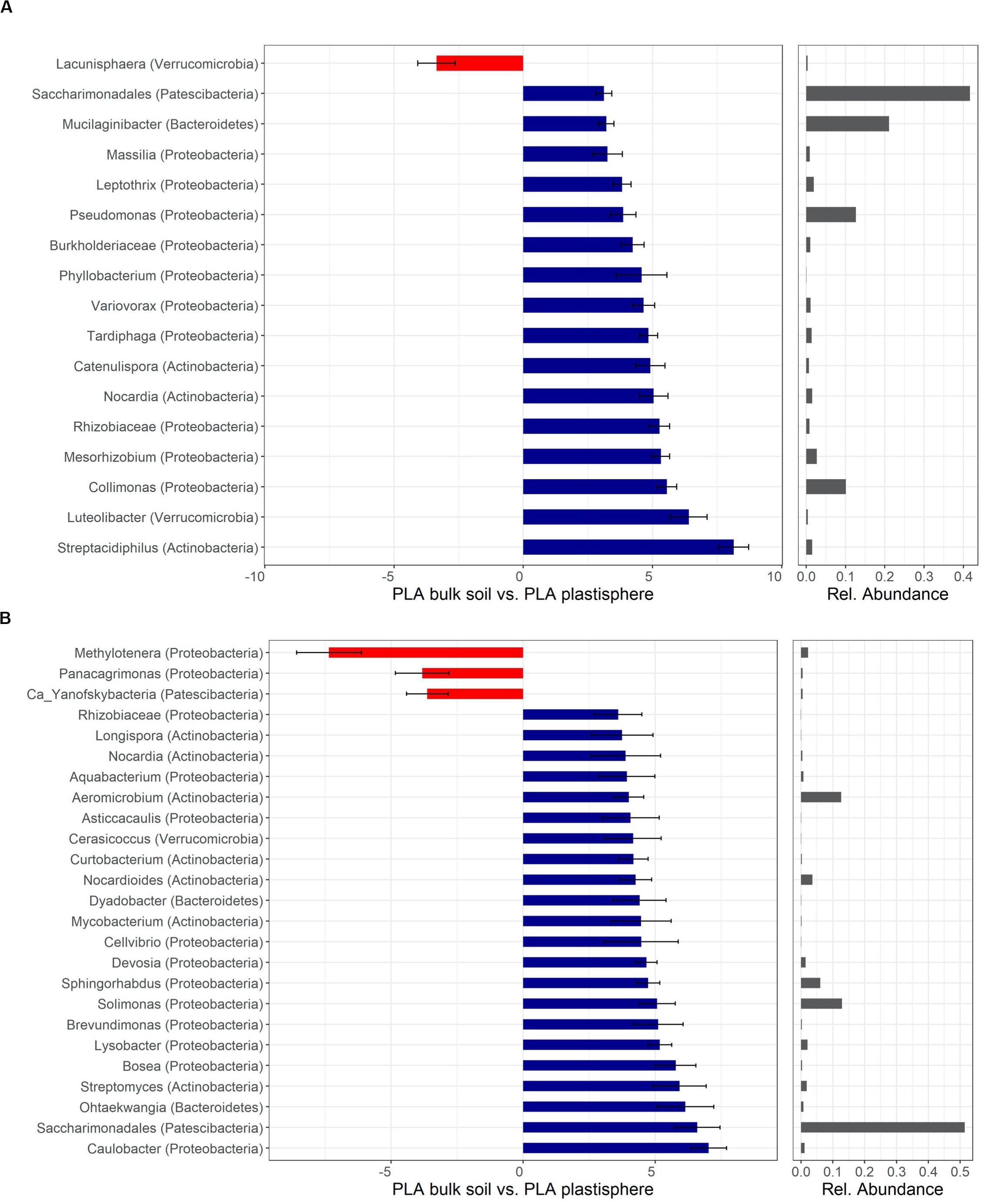
Figure 5. Differentially abundant taxa of PLA in Barba Peider (Swiss Alps) (A) and Villum (Northern Greenland) (B) soil. Taxa are classified to the lowest possible taxonomic level. Values on the X-axis in the left panels show the differential abundances in the plastisphere and bulk soil as log2-fold changes. Only significant taxa (P < 0.05) with log2-fold changes >3 for panel (A) and >3.5 for panel (B) are shown. Note that the scales in the x-axis in panels (A,B) are different. Positive values indicate a higher occurrence in the plastisphere, whereas negative values indicate a higher occurrence in the bulk soil. Names in brackets specify the phylum the taxa belong to. Values in the right panels indicate the abundances of specific taxa relative to the taxa shown in the figure. Ca, candidatus.
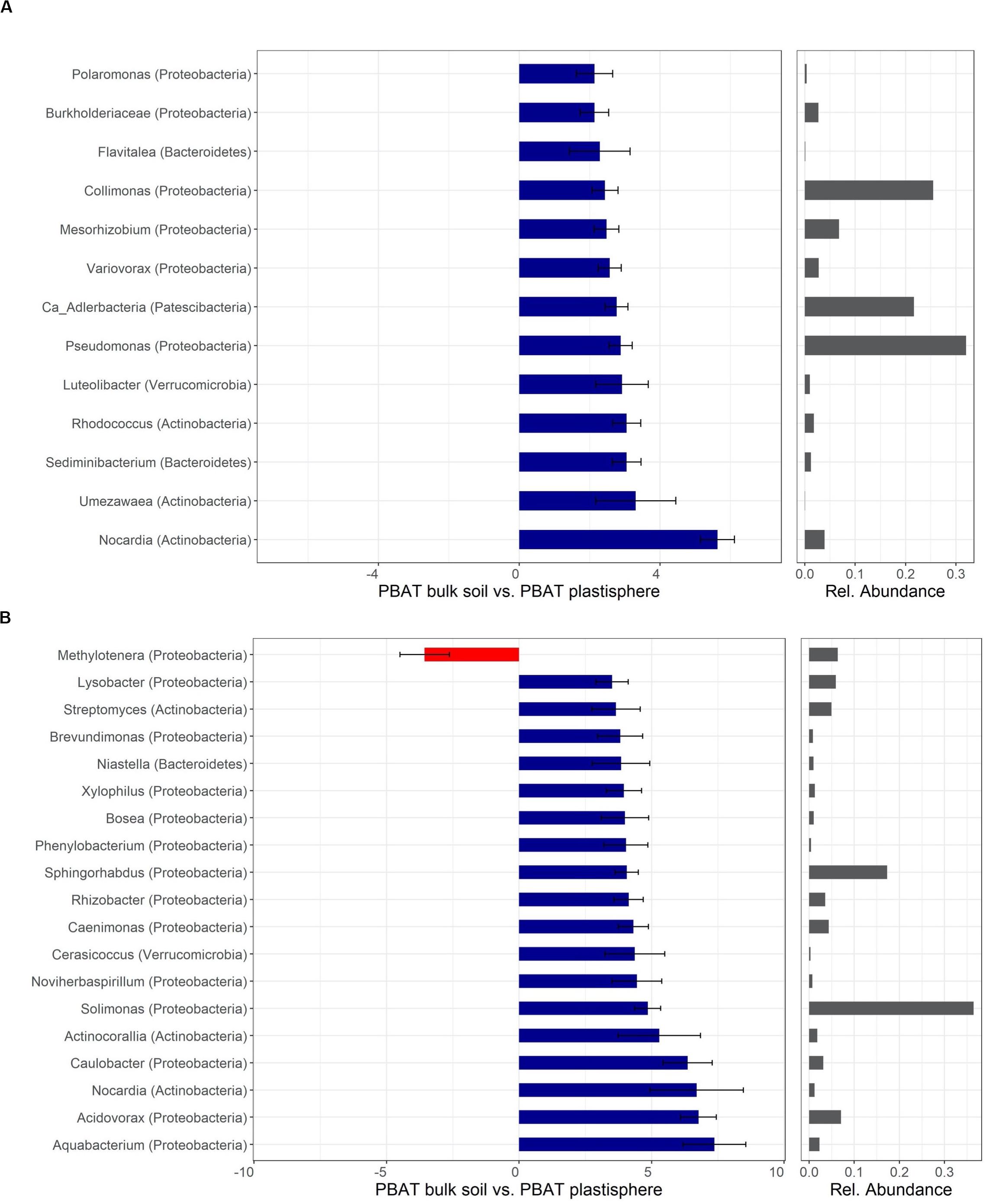
Figure 6. Differentially abundant taxa of PBAT in Barba Peider (Swiss Alps) (A) and Villum (Northern Greenland) (B) soil. Taxa are classified to the lowest possible taxonomic level. Values on the X-axis in the left panels show the differential abundances in the plastisphere and bulk soil as log2-fold changes. Only significant taxa (P < 0.05) with log2-fold changes >2 (A) and >3.5 (B) are shown. Note that the scales in the x-axis in panels (A,B) are different. Positive values indicate a higher occurrence in the plastisphere, whereas negative values indicate a higher occurrence in the bulk soil. Names in brackets specify the phylum the taxa belong to. Values in the right panels indicate the abundances of specific taxa relative to the taxa shown in the figure. Ca, candidatus.
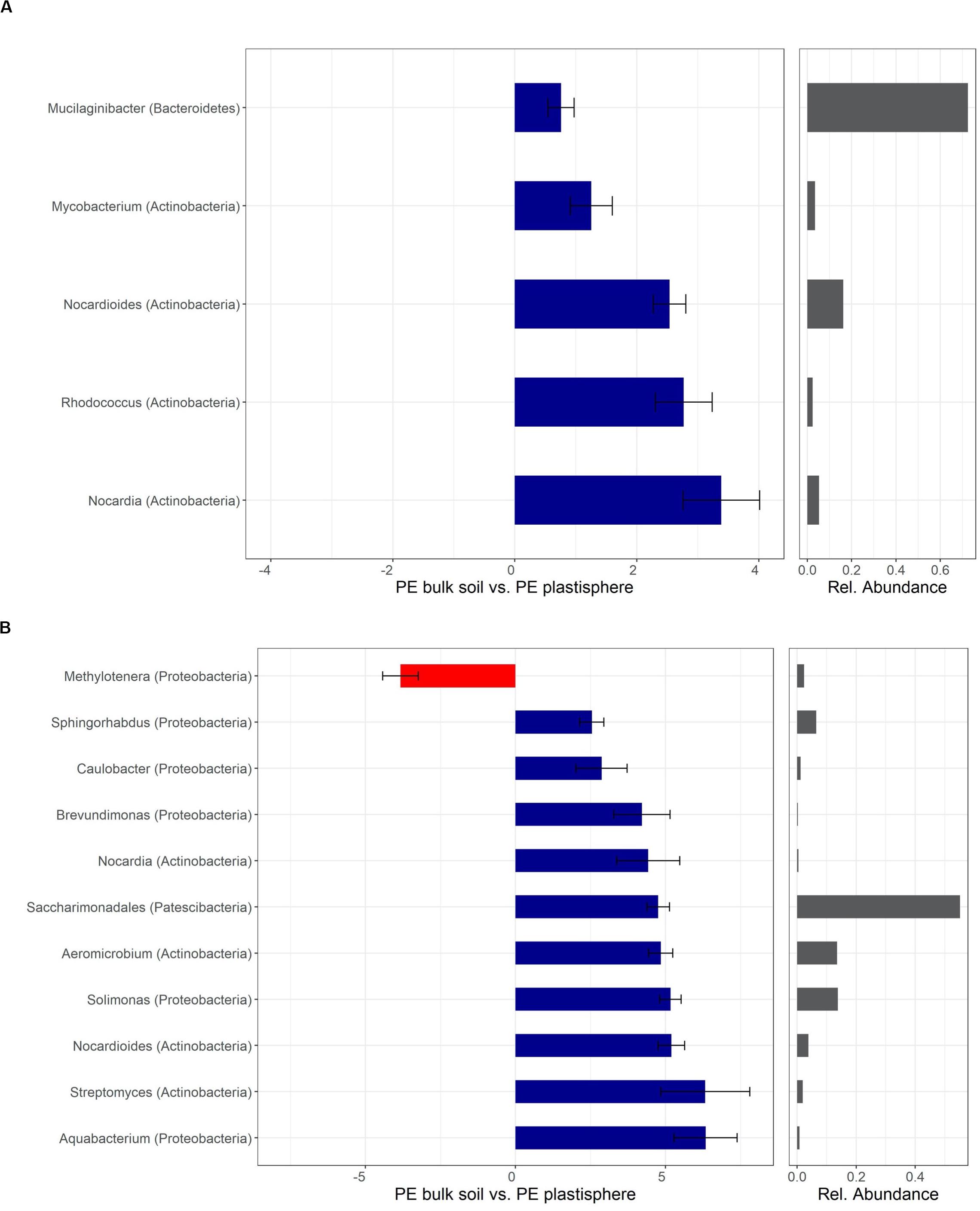
Figure 7. Differentially abundant taxa of PE in Barba Peider (Swiss Alps) (A) and Villum (Northern Greenland) (B) soil. Taxa are classified to the lowest possible taxonomic level. Values on the X-axis in the left panels show the differential abundances in the plastisphere and bulk soil as log2-fold changes. Only significant taxa (P < 0.05) with log2-fold changes >2.5 are shown. Note that the scales in the x-axis in panels (A,B) are different. Positive values indicate a higher occurrence in the plastisphere, whereas negative values indicate a higher occurrence in the bulk soil. Names in brackets specify the phylum the taxa belong to. Values in the right panels indicate the abundances of specific taxa relative to the taxa shown in the figure.
Bacteria
Most taxa with positive log2-fold changes in the plastisphere of PLA compared with bulk soils belonged to the phyla Actinobacteria and Proteobacteria, and a few belonged to Bacteroidetes, Patescibacteria, and Verrucomicrobia. Nocardia and Saccharimonadales exhibited positive log2-fold changes in the plastisphere of both soils. Several genera increased substantially in the plastisphere of PLA in Barba Peider soil, where Actinobacteria (e.g., Streptacidiphilus, Catenulispora), Proteobacteria (e.g., Collimonas, Rhizobiaceae, Variovorax, Pseudomonas) and Verrucomicrobia (Luteolibacter) dominated (Figure 5A). Furthermore, strong positive log2-fold changes in the PLA plastisphere of Villum soil compared with bulk soils were observed for genera of Actinobacteria (e.g., Nocardioides, Streptomyces), Proteobacteria (e.g., Caulobacter, Brevundimonas, Sphingorhabdus), and Bacteroidetes (e.g., Dyadobacter, Ohtaekwangia) (Figure 5B).
Taxa with positive log2-fold changes in the plastisphere of PBAT, as with PLA, belong to Actinobacteria and Proteobacteria, with a few belonging to Bacteroidetes and Verrucomicrobia. Positive log2-fold changes on PBAT in both soils were observed for Nocardia. Furthermore, genera within Actinobacteria (e.g., Rhodococcus, Umezawaea), Proteobacteria (e.g., Collimonas, Pseudomonas, Variovorax, Mesorhizobium), Bacteroidetes (Sediminibacterium) and others had positive log2-fold changes in the PBAT plastisphere of Barba Peider soil (Figure 6A). Genera of Actinobacteria (e.g., Actinocorallia, Streptomyces) and Proteobacteria (e.g., Aquabacterium, Acidovorax, Caulobacter, Brevundimonas) showed positive log2-fold changes on the PBAT surface of Villum soils (Figure 6B).
Mostly genera of the phyla Actinobacteria and Proteobacteria showed positive log2-fold changes in the plastisphere of PE. The genera Nocardia and Nocardioides (Actinobacteria) had positive log2-fold changes on PE in both soils. In addition, Rhodococcus (Actinobacteria) showed a positive log2-fold change in the plastisphere of PE in Barba Peider soil (Figure 7A), and Streptomyces, Aeromicrobium (Actinobacteria), Aquabacterium, Brevundimonas, Sphingorhabdus and Solimonas (Proteobacteria) had positive log2-fold changes on PE in Villum soil (Figure 7B).
Fungi
Even though the proportion of differentially abundant fungal OTUs in Villum soil was very high (Supplementary Table 1), most of the found OTUs were non-classifiable at low taxonomic ranks. Of the fungal OTUs in Villum soil, 43% were, e.g., only classifiable to the phylum level. Only fungi classifiable to the genus level were included in the subsequent analysis (36% of OTUs in Villum soil) (Supplementary Figures 5–7). Fungi with differential abundances in the plastisphere compared with the bulk soils mostly belonged to Ascomycota, with the exception of the plastisphere of PE in Villum soil, where more fungal taxa showing an increase belonged to Basidiomycota.
Generally, observed effects were much stronger in Villum than in Barba Peider soil for PLA. In Barba Peider soil, the genera Oidiodendron and Kabatiella had the greatest positive log2-fold changes in the plastisphere of PLA (Supplementary Figure 5A). In Villum soil, we found positive log2-fold changes for Mycosphaerella, Alternaria and Mycoarthris in the plastisphere of PLA (Supplementary Figure 5B).
The only classifiable genus showing a positive log2-fold change in the plastisphere of PBAT in Barba Peider soil was Trichocladium (Supplementary Figure 6A). Whereas the genus Pseudogymnoascus had the greatest positive log2-fold change in the plastisphere of PBAT in Villum soil, it exhibited a negative log2-fold change on the PLA surface in Barba Peider soil (Supplementary Figure 6). Many fungal genera were found to have negative log2-fold changes especially in the plastisphere of PBAT in Villum soil (Supplementary Figure 6B).
The fungal genus Mycena was found to be strongly increased in abundance in the plastisphere of PE in Villum soil (Supplementary Figure 7). However, the enrichment of Mycena was only observed in one of the three replicates, and the genus was additionally not detected in the bulk soil samples. Other genera with significant positive log2-fold changes in the PE plastisphere in Villum soil belonged to Basidiomycota (i.e., Phlebia and Tricholoma) or Ascomycota (i.e., Aspergillus and Colletotrichum) (Supplementary Figure 7). No significant differences between the plastisphere of PE and the bulk soil in Barba Peider samples were found.
Cultivable “Plastisphere” Strains
Eighty bacterial and 14 fungal strains were isolated from the plastic surfaces (Supplementary Table 2). Bacterial isolates belonged to 28 different genera within the phyla Actinobacteria, Bacteroidetes and Proteobacteria. Fungal isolates belonged to four different genera within the phyla Ascomycota and Mortierellomycota. A large part of the cultivated microorganisms (i.e., Streptomyces, Rhodococcus, Pseudomonas, Variovorax and Pseudogymnoascus) were found to be increased on the plastisphere by differential abundance analysis.
Discussion
In the present study, we linked FTIR and contact angle measurements of plastics incubated in soils with shifts in the resident microbiome. To our knowledge, this is the first experimental study comparing the effects of different plastic types on the plastisphere microbiome in alpine and Arctic soils. Increasing plastic pollution in these regions due to increased human activities highlights the need for pollution control and clean-up suitable for cold regions. The biotechnological potential of terrestrial cryoenvironments is strongly linked to patterns of taxonomical and functional diversity. Microorganisms in cold environments are known to harbor special adaptations, and particular types of genes are only in these environments present (Shi et al., 2015; da Silva et al., 2017). Our findings suggest that plastic debris provides a habitat for complex microbial assemblages that differ from those in bulk soil. The observed effects of the plastic on the microbiome were strongly dependent on the biodegradability of the plastics and were most pronounced for PLA, followed by PBAT and lastly PE.
Physico-Chemical Measurements of Polymer Degradation
Overall, analyses of the biodegradable (PLA and PBAT) and non-biodegradable (PE) plastics showed no or only weak signs of degradation after 8 weeks (initial stage of degradation) of incubation in Alpine (Barba Peider) and Arctic (Villum) soils at 15°C. The contact angle and FTIR analyses confirmed that PE is almost non-biodegradable. FTIR data allow to conclude that no oxidation of the PE occurred. Oxidation of the C-C backbone of PE is considered an important requirement for the biological degradation and assimilation of non-hydrolyzable plastics (O’Brine and Thompson, 2010; Kumar Sen and Raut, 2015).
In contrast, results for PBAT in Barba Peider soil suggested that chemical and physical changes on the polymer surface occurred due to microbial activity in this soil, as the plastic pieces incubated in natural soil differed from those incubated in sterile soil and from non-incubated pieces. On the other hand, the findings for Villum soil suggest that chemical changes occurred in the PBAT pieces as a result of unknown abiotic factors.
Notably, for the contact angle of PLA in Barba Peider soil, we observed a higher value for the sterile-soil incubated plastic relative to the non-incubated pieces and a lower angle for the natural-soil incubated plastic. Even though these results seem contradictory at first glance, the observation might be explained by opposing effects caused by abiotic degradation and microbial colonization of PLA (i.e., higher hydrophilicity of the surface due to biofilm formation). This circumstance might lead to diverging results in the physico-chemical analysis of the plastic pieces depending on the context (i.e., incubation duration). Our results demonstrate that degradation of PLA is partly abiotically driven. Abiotic degradation of PLA involves a temperature and moisture dependent chemical hydrolysis reaction (Karamanlioglu et al., 2017). It was previously shown that abiotic processes in PLA degradation (i.e., breaking down to a low molecular weight) precede microbial degradation and result in a material that is more accessible to microorganisms (Castro-Aguirre et al., 2017). The measured carbonyl indices of PLA incubated in sterile Villum soil provide further evidence that both abiotic and microbial degradation are affecting the breakdown of PLA at low temperatures.
The low level of plastic degradation observed in our study might be due to the short incubation period (8 weeks) and relatively low incubation temperature (15°C), an overall low microbial activity, or a lack of specific plastic-degrading microorganisms. Earlier soil burial experiments showed little biodegradation of PLA when incubated at 25°C for 120 days (Kamiya et al., 2007) or at 0–30°C for 1 year (Shogren et al., 2003). Another study showed little degradation of PLA after a 1-year incubation in compost and soil at 25°C, but significant degradation in compost at 45°C after only 3 weeks of incubation (Karamanlioglu and Robson, 2013). Low temperatures and a lack of sunlight, as in our experimental set-up, potentially prevent abiotic degradation that could stimulate further microbial degradation (Singh and Sharma, 2008; Castro-Aguirre et al., 2017). Nonetheless, we detected small differences between non-incubated, sterile-soil incubated and natural-soil incubated biodegradable plastics by FTIR and contact angle measurements. It would be interesting to test different incubation temperatures and durations to investigate whether greater degrees of degradation could be achieved.
The optimal temperature for biodegradation in cold terrestrial environments remains unknown, as fast biodegradation is mostly reached at considerably higher temperatures like in industrial compost, whereas microorganisms in Arctic and alpine soils are adapted to low temperatures. Furthermore, other techniques such as respirometric measurements could be applied to better differentiate between abiotic and microbial degradation of plastics (Castro-Aguirre et al., 2017). Respirometric measurements and the analysis of more than one time-point could be possible approaches to elucidate the interplay between abiotic and biotic factors taking place in the plastisphere.
Plastics Decrease Diversity and Alter Microbial Community Structures
The two soils showed many similarities regarding the effects of plastics on microbial α-diversity. Overall, the microbial diversity was lower in the plastisphere than in bulk soil, in particular with PLA. While the PLA plastisphere clearly was most prone to changes in bacterial α-diversity in both soils, the results for fungi were less consistent. Even though effects of PLA were similar in the two soils, the fungal community in Villum soil was by far more affected by PBAT and PE. Moreover, PCoA and PERMANOVA revealed clear shifts in the microbial community structures that took place in the plastisphere of the various plastics.
Parallel to the changes in α-diversity, we observed pronounced shifts in the microbial community structures with PLA and moderate changes with PBAT, whereas PE had no effect in either soil. Based on our hypothesis concerning the degree of biodegradability of the different plastics, we expected to find the largest changes in microbial community structures in the plastisphere of PLA and little change for PE; this was confirmed with our DNA metabarcoding. In general, it seems that the microbial community in Villum soil is more affected by plastic addition than that in Barba Peider soil. The different C and N content, and resulting higher initial microbial biomass in Villum soil could be a possible reason for these findings. In support of this explanation, Kamiya et al. (2007) found that several bioplastics were more prone to biodegradation in a soil with more organic matter than in one with little organic matter. To improve our understanding on the biodegradation of plastics in cold environments, soils with different characteristics, e.g., varying in C, N and organic matter content, should be tested. Moreover, analyzing the plastisphere at different time-points might demonstrate if the colonization and degradation of the plastics occurs along with a succession of taxa, or if initial microbial colonizers remain unchanged with time and at later degradation stages.
Reduced observed richness on the surface of plastics incubated in soil for 90 days was previously reported by Huang et al. (2019). A decrease in α-diversity on plastic substrates compared with in the surroundings after only 2 weeks was previously reported in a study from a marine environment (Ogonowski et al., 2018). Most other experiments in marine environments documented higher α-diversity on plastics than in the sea water (Zettler et al., 2013; Debroas et al., 2017; Dussud et al., 2018). De Tender et al. (2015) showed that only a very small set of OTUs in their marine study belonged to a core plastisphere microbiome present in all samples with an abundance of at least 0.01%, providing evidence that plastisphere microbiomes are very diverse even among marine environments. However, studies in aquatic and soil environments are difficult to compare (Bryant et al., 2016; Oberbeckmann et al., 2016; Kirstein et al., 2018). While plastic floating in water is in contact with a large number of different organisms over time and can serve as a raft for them (Oberbeckmann et al., 2016; Brunner et al., 2018), plastic substrates in soil are relatively immobile and stay permanently in contact with the inhabiting soil microbes, which are strongly adsorbed at soil surfaces (i.e., clay) (Jiang et al., 2007). There are several conceivable reasons for the reduction in the observed richness. By gaining access to a new energy and carbon source, they might outcompete other microbes not able to metabolize this carbon source in this specific niche (Dini-Andreote et al., 2015). This growth advantage can lead to the decline of other taxa, a phenomenon named “competitive exclusion” (Hibbing et al., 2010). Secondly, plastic addition can change the microenvironment of the soils by releasing potentially harmful compounds (Atuanya et al., 2016), changing the pH, or creating a barrier for water, oxygen, and nutrients (Bandopadhyay et al., 2018).
Plastic-Dependent Microbiome
Relative Abundances of Microbial Taxa
Overall, the microbial communities of the plastisphere and bulk soil were characterized by taxonomic groups commonly observed in Arctic and alpine soils (Frey et al., 2016; Rime et al., 2016; Donhauser and Frey, 2018; Malard and Pearce, 2018). Only few phyla of this endemic microbiome showed an altered relative abundance in the plastisphere. Plastic addition might have little influence on the relative abundances of fungi if higher taxonomic ranks, for example phyla, are regarded. However, this interpretation might be misleading regarding the investigated soils, as the phylum Ascomycota is very dominant in these soils. Therefore, a plastic-induced selection for Ascomycota, as reported elsewhere (Muroi et al., 2016), cannot be detected by solely comparing relative abundances at the phylum level. The relative abundances of high-ranking bacterial taxa shared many similarities among the two soils. Only few phyla and orders seemed to be competitive in the plastisphere of PLA and PBAT. Especially Actinobacteria, Proteobacteria, and Patescibacteria seemed to profit from the plastisphere environment. While Proteobacteria increased in relative abundance on PLA in Barba Peider soil, it showed growth advantages on PBAT in Villum soil. Actinobacteria benefitted from PE in both soils, as well as from PLA in Barba Peider soil. Patescibacteria strongly increased in relative abundance in the PLA plastisphere in Villum soil and also profited from this plastic type in Barba Peider soil. Only little research has dealt with the colonization and biodegradation of plastics in soils so far. In one study, the effect of PBAT on the microbial community was analyzed by genetic profiling (DGGE), and Ascomycota were found to profit from the plastic, while the bacterial community did not change considerably (Muroi et al., 2016). Whereas this earlier study analyzed the long-term effects of plastic addition on a whole agricultural field, we investigated the region in proximity to single plastic pieces. A spatial limitation of the effects caused by plastic in soil might be one reason we observed more pronounced effects on the bacterial community than reported previously. Other studies investigated the microbial community changes on PE microplastics and PLA/PHB (poly(3-hydroxybutyrate)) blend foils in laboratory incubation experiments (Jeszeová et al., 2018; Huang et al., 2019). Consistent with our findings, Huang et al. (2019) detected a significant increase in Actinobacteria on the plastic surface, and Jeszeová et al. (2018) found significant changes in the microbial community structure of PLA/PHB foil, i.e., increased numbers of Streptomyces and Rhodococcus.
Members of the Proteobacteria are physiologically and ecologically extremely diverse but are key players in C and N cycling. In particular, members of the Burkholderiales within Proteobacteria thrived in the plastisphere of both soils. Actinobacteria are ubiquitous and frequently saprophytic organisms able to degrade recalcitrant C sources. They play a vital role in the C cycle in the soil (Ventura et al., 2007; Bull, 2011; Rosenberg et al., 2014; Mohammadipanah and Wink, 2016). The ability to decompose recalcitrant compounds is an important trait in oligotrophic soil environments when more readily available substrates are rather limited. Furthermore, many members of the Actinobacteria are capable of spore formation and filamentous growth, which facilitates survival under low soil moisture (Wolf et al., 2013). With these capabilities, Actinobacteria are known to compete well under oligotrophic conditions. In contrast, Acidobacteria (i.e., subgroup Gp6) decreased in the plastisphere of all polymers. While the broad metabolic potential of Actinobacteria and Proteobacteria is widely known, it was surprising to find Patescibacteria and the associated order Saccharimonadales accumulating in the plastisphere.
Bacterial Key Taxa Associated With Plastics
Since different subgroups within a phylum can show ambiguous responses to polymer addition, we identified specific “plastisphere taxa” at lower taxonomic levels. Several actinobacterial taxa, such as Rhodococcus, Nocardia and Streptomyces, increased in the plastisphere in our study. Cultivated strains of these genera isolated from the incubated plastics yield further evidence for their presence on the plastic surfaces and complement our DNA metabarcoding data. Actinobacteria are, in general, promising candidates for biodegradation of all kinds of plastics, including PLA, PBAT, and strikingly even PE. Butbunchu and Pathom-Aree (2019) summarized the known abilities of Actinobacteria to degrade PLA. Interestingly, PLA biodegradation has been demonstrated for only a very limited number of actinobacterial families, and biodegradation by cold-adapted Actinobacteria has not yet been reported. PBAT degradation has so far only been shown for two thermophilic Thermomonospora fusca strains (Kleeberg et al., 1998). Other researchers reported that various isolated strains, including Rhodococcus spp., Streptomyces spp. and Nocardia, were associated with the biodegradation of PE (Kumar Sen and Raut, 2015; Pathak and Navneet, 2017). However, we have to consider that in most studies examining PE degradation, pretreated polymers or commercial products bearing additives have been used.
Most of the plastic-associated taxa within the Proteobacteria in our tested soils belong to a small number of orders, such as Burkholderiales, Caulobacterales, Rhizobiales and a few γ-Proteobacteria. Members of these orders were also found among the isolated strains obtained from the incubated plastics. Especially Pseudomonas is well known for its broad metabolic capabilities, and various strains of the genus are associated with the degradation of PLA, PBAT, PE, and many other plastics (Wallace et al., 2017; Wilkes and Aristilde, 2017; Bubpachat et al., 2018). Burkholderiales possess a broad range of enzymes able to degrade polyaromatic hydrocarbons (PAHs), which include important soil pollutants (Pérez-Pantoja et al., 2012). Aquabacterium was first isolated from biofilms of a drinking water system (Kalmbach et al., 1999), and a species of the genus was shown to degrade oil (Pham et al., 2015). Strains of the genus Variovorax hold highly diverse catabolic capabilities, including the degradation of 3,3’-Thiodipropionic acid (TDP), an additive widely used to stabilize polymers, as well as dimethyl terephthalate and vinyl chloride, two other chemicals used in plastic production (Satola et al., 2013; Wilson et al., 2016). Collimonas comprises chitinolytic species shown to degrade xenobiotics (Leveau et al., 2010); however, the genus has not been linked to plastic degradation yet. Species of the genera Acidovorax and Leptothrix are able to degrade the bioplastic poly(tetramethylene succinate)-co-adipate (PBSA) (Uchida et al., 2000; Nakajima-Kambe et al., 2009b). Additionally, the Leptothrix strain has been shown to degrade PLA (Nakajima-Kambe et al., 2009a). In our study, Leptothrix was increased in the PLA plastisphere of Barba Peider soil.
In a recent study, Rhizobiales were recognized as important taxon present on plastics floating in the North Atlantic garbage patch (Debroas et al., 2017). Mesorhizobium spp. have been shown to be associated with anaerobic PLA degradation (Yagi et al., 2014). Sphingorhabdus, a genus of the order Sphingomonadales, has been found to be able to degrade oil (Jeong et al., 2016). Caulobacter crescentus is a model laboratory organism that has been extensively studied concerning the process of the attachment of bacterial cells to surfaces and the formation of biofilms (Entcheva-Dimitrov and Spormann, 2004). In addition, Caulobacter spp. have been shown to degrade PAHs including pyrene and phenantrene (Chang et al., 2014; Al-Thukair and Malik, 2016). A Brevundimonas species has been reported to degrade the biodegradable plastic poly(ε-caprolactone) (Nawaz et al., 2015).
We found members of the Saccharimonadales to be the major “plastisphere taxa” in both soils. To our knowledge, this is the first report of Saccharimonadales within the Patescibacteria superphylum thriving in the plastisphere. Very little is known about the ecology of Saccharimonadales, which belongs to the candidate phylum Saccharibacteria (formerly TM7), and knowledge almost exclusively stems from genomic data. Saccharibacteria have been shown to possess very small genomes and cell sizes, and they have therefore been proposed to live in symbiosis with other microorganisms depending on co-metabolism (Lemos et al., 2019). Further, Lemos et al. (2019) stated that the majority of unique genes found in nearly complete genomes of two Saccharibacteria are of unknown function, underlining the knowledge gaps existing regarding this bacterial order. Several genes involved in the catabolism of complex C sources were identified in TM7 strain RAAC3 and other Saccharibacteria (Kantor et al., 2013; Starr et al., 2018). In addition, some Saccharibacteria were shown to take up oleic acid and to possess lipase and other exoenzymatic activities (Kindaichi et al., 2016). Interestingly, many Saccharibacteria seem to possess a D−lactate dehydrogenase−like protein acting in the conversion of pyruvate into lactate in a fermentation pathway (Lemos et al., 2019). Our data implicate that lactate, the monomer of PLA, might be used as a C source instead of being only a final waste product in the metabolism of some Saccharibacteria. Furthermore, metagenomic data indicate that representatives of the phylum are able to degrade a variety of polymers (Kantor et al., 2013; Starr et al., 2018). However, the involvement of Saccharibacteria in the degradation of PLA is speculative and needs to be addressed in future studies.
In our study, some taxa within the Bacteroidetes (i.e., Sediminibacterium, Dyadobacter, Ohtaekwangia) increased in the plastisphere. Sediminibacterium was previously shown to degrade vinylchloride (Wilson et al., 2016). This chemical is the monomer of an important plastic, namely polyvinylchloride (PVC). Dyadobacter and Ohtaekwangia, which both increased significantly in the plastisphere of PLA in Villum soil, were previously identified as potential biodegraders of xenobiotic pollutants (Willumsen et al., 2005; Tejeda-Agredano et al., 2013; Bao et al., 2020).
As far as we know, the only link between Verrucomicrobia and plastics in earlier investigations was made in a study where Verrucomicrobia subdivision 1 was shown to be associated with polyethylene terephthalate (PET) in a marine environment, whereas subdivision 4 was rather found in the surrounding water (Oberbeckmann et al., 2016). Our results partly fit with this observation, as we found Luteolibacter (subdivision 1) to be increased in the proximity of plastic and Lacunisphaera (subdivision 4) increased in the bulk soil. However, Cerasicoccus (subdivision 4) was augmented in the plastisphere as well, which contrasts with the previous findings.
Fungal Key Taxa Associated With Plastics
Oidiodendron spp., found to be enriched in the plastisphere of PLA in Barba Peider soil, are closely related to the dominant Pseudogymnoascus and are found on a great variety of substrates, including wood, peat and human hair (Rice and Currah, 2005). The genus has been shown to possess the ability to degrade a broad spectrum of substrates, e.g., cellulose and lignin (Rice and Currah, 2005).
The genus Alternaria, found to be increased in the plastisphere of PLA in Villum soil, comprises saprobic and endophytic species and is known to cause serious disease in plants (Woudenberg et al., 2013). The genera Alternaria and Pseudogymnoascus (synonym Geomyces) were previously linked to polyurethane degradation (Loredo-Treviño et al., 2012). Mycosphaerella, another genus that increased in the plastisphere of PLA in Villum soil, likewise comprises plant pathogenic species. Species of this genus have been shown to produce a wide variety of polymer-degrading enzymes involved in the break-down of plant cell walls (Douaiher et al., 2007).
Phlebia, a basidiomycetous genus found to be increased in the plastisphere of PE in Villum soil, is known to possess enzymes for lignin decomposition (Kantelinen et al., 1989) and has been linked to the biodegradation of PAHs (Mori et al., 2003). In our experiment, Mycena showed the largest growth advantage of all fungi in the plastisphere of all plastics in both soils. However, the strong increase in abundance was only observed in one replicate. Due to the lack of consistency, these results have to be interpreted with caution. Like Phlebia, Mycena has been linked to PAH degradation (Winquist et al., 2014).
Conclusion
As far as we know, this is the first report on the plastisphere microbiome of different plastic types (biodegradable and non-biodegradable) in terrestrial cryoenvironments using DNA metabarcoding. We found clear differences between microbial consortia on plastics and the surrounding environment, as previously reported in marine environments. Our findings suggest that plastic debris form a habitat for complex microbial assemblages with lifestyles and possibly metabolic pathways distinct from those of the microbial communities in bulk soil.
Even though degradation in our mesocosm experiment was slight, we detected pronounced shifts in bacterial and fungal α-diversity and community structures in the plastisphere compared with values in adjacent soils not affected by the plastics. Our results supported our hypothesis that the effects were larger on biodegradable (PLA, PBAT) than non-biodegradable plastics (PE).
Interestingly, members of only a few phyla (Actinobacteria, Proteobacteria, and Patescibacteria) were found to benefit from the plastics in both soils. Similarly, the key microbial taxa associated with plastics were similar in the two soils (Barba Peider and Villum). Plastic addition affected fungal taxa to a lesser extent, and we have not found any common taxa that reacted similarly in both soils. However, it is noteworthy that some genera (i.e., Mycena and Plebia) were increased on PE in the Villum. We found several candidate taxa in the plastisphere with known potential to biodegrade xenobiotics, e.g., Burkholderiales, Pseudomonas, Caulobacter, Rhodococcus, Nocardia and Streptomyces indicating the potential of cold-adapted microorganisms to degrade bioplastics. Plastic degraders from terrestrial cryoenvironments are thus conceivable but further investigations with single isolates or consortia of microorganisms degrading plastics needs to be performed.
In addition to identifying many organisms that are already prominently published in the context of plastics and especially their biodegradation, we found members of the Saccharimonadales to be the major “plastisphere taxa.” To our knowledge, this is the first report of Saccharimonadales within the Patescibacteria superphylum thriving in the plastisphere. Further research is needed to evaluate their occurrence in the plastisphere of other soils from cold or temperate environments, to find traits responsible for their thriving on the PLA surface, and to analyze if they potentially even degrade PLA.
Increased human activities in the Arctic and the Alps demand environment-friendly processes for pollution control and clean-up, suitable for cold regions. Microorganisms from terrestrial cryoenvironments harbor great biotechnological potential, as they are known to possess special adaptations to cold temperatures. Major knowledge gaps still exist about the microbial life in the plastisphere, especially in terrestrial cryoenvironments. The expansive geographical dimensions of these environments point to their global importance, and we plead for further clarification of existing ambiguities. Our findings highlight that correct waste management is crucial not only for non-biodegradable but also for biodegradable plastics in order to protect environments all over the world against this serious threat.
Data Availability Statement
The datasets presented in this study can be found in online repositories. The names of the repository/repositories and accession number(s) can be found below: https://www.ncbi.nlm.nih.gov/, PRJNA630025.
Author Contributions
JR, BF, and IB designed the study. JR and DB performed the experiment. JR, LP-C, and DB participated in data analysis. JR wrote the manuscript with the help of BF and IB. All authors contributed to the article and approved the submitted version.
Funding
This study was partly funded by the Swiss National Science Foundation (SNSF) under the grant IZLSZ2_170941, the Swiss Polar Institute (SPI), and the BNP Paribas Swiss Foundation.
Conflict of Interest
The authors declare that the research was conducted in the absence of any commercial or financial relationships that could be construed as a potential conflict of interest.
Acknowledgments
We thank C. Perez-Mon for providing soils from Villum Research Station and Muot da Barba Peider; B. Stierli for laboratory assistance; R. Köchli for completing soil analyses; and S. Gunz and A. Dharmarajah for providing advice in data analysis. We acknowledge the Genetic Diversity Centre (GDC) of the ETH Zürich and the contribution of scientists at the McGill University and Génome Québec Innovation Center in Montreal, Canada for performing Illumina MiSeq sequencing. We also thank M. Dawes for her valuable contribution to the editing of this article.
Supplementary Material
The Supplementary Material for this article can be found online at: https://www.frontiersin.org/articles/10.3389/fenvs.2020.562263/full#supplementary-material
SUPPLEMENTARY DATA SHEET 1 | Supplementary Tables S1, S2.
SUPPLEMENTARY DATA SHEET 2 | Supplementary Figures S1–S7.
SUPPLEMENTARY DATA SHEET 3 | List of significant (q > 0.05) differently abundant (log2-fold change) bacterial and fungal OTUs in the plastisphere compared to bulk soils.
SUPPLEMENTARY DATA SHEET 4 | List of significant (q > 0.05) differently abundant (log2-fold change) bacterial and fungal genera in the plastisphere compared to bulk soils.
SUPPLEMENTARY DATA SHEET 5 | 16S rDNA sequences of isolated bacterial and fungal strains from the plastisphere.
Abbreviations
CA, contact angle; CI, carbonyl index; PAHs, polyaromatic hydrocarbons; PBAT, polybutylene adipate terephthalate; PE, polyethylene; PLA, polylactic acid.
References
Accinelli, C., Saccà, M. L., Mencarelli, M., and Vicari, A. (2012). Deterioration of bioplastic carrier bags in the environment and assessment of a new recycling alternative. Chemosphere 89, 136–143. doi: 10.1016/j.chemosphere.2012.05.028
Adamczyk, M., Hagedorn, F., Wipf, S., Donhauser, J., Vittoz, P., Rixen, C., et al. (2019). The soil microbiome of Gloria Mountain summits in the Swiss Alps. Front. Microbiol. 10:1080. doi: 10.3389/fmicb.2019.01080
Allen, S., Allen, D., Phoenix, V. R., Le Roux, G., Durántez Jiménez, P., Simonneau, A., et al. (2019). Atmospheric transport and deposition of microplastics in a remote mountain catchment. Nat. Geosci. 12, 339–344. doi: 10.1038/s41561-019-0335-5
Al-Thukair, A. A., and Malik, K. (2016). Pyrene metabolism by the novel bacterial strains Burkholderia fungorum (T3A13001) and Caulobacter sp (T2A12002) isolated from an oil-polluted site in the Arabian Gulf. Int. Biodeter. Biodegr. 110, 32–37. doi: 10.1016/j.ibiod.2016.02.005
Amaral-Zettler, L. A., Zettler, E. R., and Mincer, T. J. (2020). Ecology of the plastisphere. Nat. Rev. Microbiol. 18, 139–151. doi: 10.1038/s41579-019-0308-0
Ambrosini, R., Azzoni, R. S., Pittino, F., Diolaiuti, G., Franzetti, A., and Parolini, M. (2019). First evidence of microplastic contamination in the supraglacial debris of an alpine glacier. Environ. Pollut. 253, 297–301. doi: 10.1016/j.envpol.2019.07.005
Anderson, M. J. (2001). Non-parametric MANOVA. Aust. Ecol. 26, 32–46. doi: 10.1111/j.1442-9993.2001.01070.pp.x
Atuanya, E., Udochukwu, U., and Dave-Omoregie, A. (2016). Bioavailability and toxicity of plastic contaminants to soil and soil bacteria. Br. Microbiol. Res. J. 13, 1–8. doi: 10.9734/bmrj/2016/25128
Bandopadhyay, S., Martin-closas, L., and Pelacho, A. M. (2018). Biodegradable plastic mulch films: impacts on soil microbial communities and ecosystem functions. Front. Microbiol. 9:819. doi: 10.3389/fmicb.2018.00819
Bao, H., Wang, J., Zhang, H., Li, J., Li, H., and Wu, F. (2020). Effects of biochar and organic substrates on biodegradation of polycyclic aromatic hydrocarbons and microbial community structure in PAHs-contaminated soils. J. Hazard. Mater. 385:121595. doi: 10.1016/j.jhazmat.2019.121595
Bengtsson-Palme, J., Hartmann, M., Eriksson, K. M., Pal, C., Thorell, K., Larsson, D. G. J., et al. (2015). metaxa2: improved identification and taxonomic classification of small and large subunit rRNA in metagenomic data. Mol. Ecol. Resour. 15, 1403–1414. doi: 10.1111/1755-0998.12399
Bengtsson-Palme, J., Ryberg, M., Hartmann, M., Branco, S., Wang, Z., Godhe, A., et al. (2013). Improved software detection and extraction of ITS1 and ITS2 from ribosomal ITS sequences of fungi and other eukaryotes for analysis of environmental sequencing data. Methods Ecol. Evol. 4, 914–919. doi: 10.1111/2041-210X.12073
Bergmann, M., Mützel, S., Primpke, S., Tekman, M. B., Trachsel, J., and Gerdts, G. (2019). White and wonderful? Microplastics prevail in snow from the Alps to the Arctic. Sci. Adv. 5, 1–11. doi: 10.1126/sciadv.aax1157
Briassoulis, D., and Dejean, C. (2010). Critical review of norms and standards for biodegradable agricultural plastics part I. Biodegradation in soil. J. Polym. Environ. 18, 384–400. doi: 10.1007/s10924-010-0168-1
Brunner, I., Fischer, M., Rüthi, J., Stierli, B., and Frey, B. (2018). Ability of fungi isolated from plastic debris floating in the shoreline of a lake to degrade plastics. PLoS One 13:e0202047. doi: 10.1371/journal.pone.0202047
Bryant, J. A., Clemente, T. M., Viviani, D. A., Fong, A. A., Thomas, K. A., Kemp, P., et al. (2016). Diversity and activity of communities inhabiting plastic debris in the north Pacific gyre. mSystems 1, 1–19. doi: 10.1128/msystems.00024-16
Bubpachat, T., Sombatsompop, N., and Prapagdee, B. (2018). Isolation and role of polylactic acid-degrading bacteria on degrading enzymes productions and PLA biodegradability at mesophilic conditions. Polym. Degrad. Stabil. 152, 75–85. doi: 10.1016/j.polymdegradstab.2018.03.023
Bull, A. T. (2011). “Actinobacteria of the extremobiosphere,” in Extremophiles Handbook, ed. K. Horikoshi (Tokyo: Springer), 1203–1240. doi: 10.1007/978-4-431-53898-1_58
Butbunchu, N., and Pathom-Aree, W. (2019). Actinobacteria as promising candidate for polylactic acid type bioplastic degradation. Front. Microbiol. 10:2834. doi: 10.3389/fmicb.2019.02834
Castro-Aguirre, E., Auras, R., Selke, S., Rubino, M., and Marsh, T. (2017). Insights on the aerobic biodegradation of polymers by analysis of evolved carbon dioxide in simulated composting conditions. Polym. Degrad. Stabil. 137, 251–271. doi: 10.1016/j.polymdegradstab.2017.01.017
Chang, Y. T., Hung, C. H., and Chou, H. L. (2014). Effects of polyethoxylate lauryl ether (Brij 35) addition on phenanthrene biodegradation in a soil/water system. J. Environ. Sci. Heal. A 49, 1672–1684. doi: 10.1080/10934529.2014.951228
Clarke, K., and Gorley, R. (2006). PRIMER v6Q15: User Manual/Tutorial. PRIMER-E (Plymouth: Plymouth Routines in Multivariate Ecological Research).
da Silva, A. C., Rachid, C. T. C. C., de Jesus, H. E., Rosado, A. S., and Peixoto, R. S. (2017). Predicting the biotechnological potential of bacteria isolated from Antarctic soils, including the rhizosphere of vascular plants. Polar Biol. 40, 1393–1407. doi: 10.1007/s00300-016-2065-0
de Souza Machado, A. A., Kloas, W., Zarfl, C., Hempel, S., and Rillig, M. C. (2018). Microplastics as an emerging threat to terrestrial ecosystems. Glob. Chang. Biol. 24, 1405–1416. doi: 10.1111/gcb.14020
De Tender, C. A., Devriese, L. I., Haegeman, A., Maes, S., Ruttink, T., and Dawyndt, P. (2015). Bacterial community profiling of plastic litter in the Belgian part of the North Sea. Environ. Sci. Technol. 49, 9629–9638. doi: 10.1021/acs.est.5b01093
Debroas, D., Mone, A., and Ter Halle, A. (2017). Plastics in the North Atlantic garbage patch: a boat-microbe for hitchhikers and plastic degraders. Sci. Total Environ. 599–600, 1222–1232. doi: 10.1016/j.scitotenv.2017.05.059
Dini-Andreote, F., Stegen, J. C., Van Elsas, J. D., and Salles, J. F. (2015). Disentangling mechanisms that mediate the balance between stochastic and deterministic processes in microbial succession. PNAS 112, E1326–E1332. doi: 10.1073/pnas.1414261112
Donhauser, J., and Frey, B. (2018). Alpine soil microbial ecology in a changing world. FEMS Microbiol. Ecol. 94, 1–31. doi: 10.1093/femsec/fiy099
Douaiher, M. N., Nowak, E., Dumortier, V., Durand, R., Reignault, P., and Halama, P. (2007). Mycosphaerella graminicola produces a range of cell wall-degrading enzyme activities in vitro that vary with the carbon source. Eur. J. Plant Pathol. 117, 71–79. doi: 10.1007/s10658-006-9073-9
Dussud, C., Meistertzheim, A. L., Conan, P., Pujo-Pay, M., George, M., Fabre, P., et al. (2018). Evidence of niche partitioning among bacteria living on plastics, organic particles and surrounding seawaters. Environ. Pollut. 236, 807–816. doi: 10.1016/j.envpol.2017.12.027
Edgar, R. C. (2013). UPARSE: highly accurate OTU sequences from microbial amplicon reads. Nat. Methods 10, 996–998. doi: 10.1038/nmeth.2604
Edgar, R. C., and Flyvbjerg, H. (2015). Error filtering, pair assembly and error correction for next-generation sequencing reads. Bioinformatics 31, 3476–3482. doi: 10.1093/bioinformatics/btv401
Entcheva-Dimitrov, P., and Spormann, A. M. (2004). Dynamics and control of biofilms of the oligotrophic bacterium Caulobacter crescentus. J. Bacteriol. 186, 8254–8266. doi: 10.1128/JB.186.24.8254-8266.2004
Fierer, N. (2017). Embracing the unknown: disentangling the complexities of the soil microbiome. Nat. Rev. Microbiol. 15, 579–590. doi: 10.1038/nrmicro.2017.87
Frasson, D., Udovièiæ, M., Frey, B., Lapanje, A., Zhang, D. C., Margesin, R., et al. (2015). Glaciimonas alpina sp. nov. isolated from alpine glaciers and reclassification of glaciimonas immobilis Cr9-12 as the type strain of glaciimonas alpina sp. nov. Int. J. Syst. Evol. Microbiol. 65, 1779–1785. doi: 10.1099/ijs.0.000174
Frey, B., Rime, T., Phillips, M., Stierli, B., Hajdas, I., Widmer, F., et al. (2016). Microbial diversity in European alpine permafrost and active layers. FEMS Microbiol. Ecol. 92:fiw018. doi: 10.1093/femsec/fiw018
Hartmann, M., Brunner, I., Hagedorn, F., Bardgett, R. D., Stierli, B., Herzog, C., et al. (2017). A decade of irrigation transforms the soil microbiome of a semi-arid pine forest. Mol. Ecol. 26, 1190–1206. doi: 10.1111/mec.13995
Heuer, H., Krsek, M., Baker, P., Smalla, K., and Wellington, E. M. (1997). Analysis of actinomycete communities by specific amplification of genes encoding 16S rRNA and gel-electrophoretic separation in denaturing gradients. Appl. Environ. Microbiol. 63, 3233–3241. doi: 10.1128/aem.63.8.3233-3241.1997
Hibbing, M. E., Fuqua, C., Parsek, M. R., and Peterson, S. B. (2010). Bacterial competition: surviving and thriving in the microbial jungle. Nat. Rev. Microbiol. 8, 15–25. doi: 10.1038/nrmicro2259
Horton, A. A., Walton, A., Spurgeon, D. J., Lahive, E., and Svendsen, C. (2017). Microplastics in freshwater and terrestrial environments: evaluating the current understanding to identify the knowledge gaps and future research priorities. Sci. Total Environ. 586, 127–141. doi: 10.1016/j.scitotenv.2017.01.190
Huang, Y., Zhao, Y., Wang, J., Zhang, M., Jia, W., and Qin, X. (2019). LDPE microplastic films alter microbial community composition and enzymatic activities in soil. Environ. Pollut. 254:112983. doi: 10.1016/j.envpol.2019.112983
Huerta Lwanga, E., Gertsen, H., Gooren, H., Peters, P., Salánki, T., Van Der Ploeg, M., et al. (2016). Microplastics in the terrestrial ecosystem: implications for Lumbricus terrestris (Oligochaeta, Lumbricidae). Environ. Sci. Technol. 50, 2685–2691. doi: 10.1021/acs.est.5b05478
Jeong, H. I., Jin, H. M., and Jeon, C. O. (2016). Complete genome sequence of Sphingorhabdus sp. M41, a versatile hydrocarbon degrader, isolated from crude oil-contaminated costal sediment. J. Biotechnol. 227, 41–42. doi: 10.1016/j.jbiotec.2016.04.016
Jeszeová, L., Puškárová, A., Bučková, M., Kraková, L., Grivalský, T., Danko, M., et al. (2018). Microbial communities responsible for the degradation of poly(lactic acid)/poly(3-hydroxybutyrate) blend mulches in soil burial respirometric tests. World J. Microbiol. Biotechnol. 34:101. doi: 10.1007/s11274-018-2483-y
Jiang, D., Huang, Q., Cai, P., Rong, X., and Chen, W. (2007). Adsorption of Pseudomonas putida on clay minerals and iron oxide. Colloids Surf. B 54, 217–221. doi: 10.1016/j.colsurfb.2006.10.030
Kalmbach, S., Manz, W., Wecke, J., and Szewzyk, U. (1999). Aquabacterium gen. nov., with description of Aquabacterium citratiphilum sp. nov., Aquabacterium parvum sp. nov. and Aquabacterium commune sp. nov., three in situ dominant bacterial species from the Berlin drinking water system. Int. J. Syst. Bacteriol. 49, 769–777. doi: 10.1099/00207713-49-2-769
Kamiya, M., Asakawa, S., and Kimura, M. (2007). Molecular analysis of fungal communities of biodegradable plastics in two Japanese soils. Soil Sci. Plant Nutr. 53, 568–574. doi: 10.1111/j.1747-0765.2007.00169.x
Kantelinen, A., Hatakka, A., and Viikari, L. (1989). Production of lignin peroxidase and laccase by Phlebia radiata. Appl. Microbiol. Biotechnol. 31, 234–239.
Kantor, R. S., Wrighton, K. C., Handley, K. M., Sharon, I., Hug, L. A., Castelle, C. J., et al. (2013). Small genomes and sparse metabolisms of sediment-associated bacteria from four candidate phyla. mBio 4:e00708-13. doi: 10.1128/mBio.00708-13
Karamanlioglu, M., Preziosi, R., and Geoffrey D. R. (2017). Abiotic and biotic environmental degradation of the bioplastic polymer poly(lactic acid): a review. Polym. Degrad. Stabil. 137, 122–130. doi: 10.1016/j.polymdegradstab.2017.01.009
Karamanlioglu, M., and Robson, G. D. (2013). The influence of biotic and abiotic factors on the rate of degradation of poly(lactic) acid (PLA) coupons buried in compost and soil. Polym. Degrad. Stabil. 98, 2063–2071. doi: 10.1016/j.polymdegradstab.2013.07.004
Keswani, A., Oliver, D. M., Gutierrez, T., and Quilliam, R. S. (2016). Microbial hitchhikers on marine plastic debris: human exposure risks at bathing waters and beach environments. Mar. Environ. Res. 118, 10–19. doi: 10.1016/j.marenvres.2016.04.006
Kindaichi, T., Yamaoka, S., Uehara, R., Ozaki, N., Ohashi, A., Albertsen, M., et al. (2016). Phylogenetic diversity and ecophysiology of Candidate phylum Saccharibacteria in activated sludge. FEMS Microbiol. Ecol. 92, 1–11. doi: 10.1093/femsec/fiw078
Kirstein, I. V., Wichels, A., Gullans, E., Krohne, G., and Gerdts, G. (2019). The plastisphere – uncovering tightly attached plastic “specific” microorganisms. PLoS One 14:e215859. doi: 10.1371/journal.pone.0215859
Kirstein, I. V., Wichels, A., Krohne, G., and Gerdts, G. (2018). Mature biofilm communities on synthetic polymers in seawater – specific or general? Mar. Environ. Res. 142, 147–154. doi: 10.1016/j.marenvres.2018.09.028
Kleeberg, I., Hetz, C., Kroppenstedt, R. M., Müller, R. J., and Deckwer, W. D. (1998). Biodegradation of aliphatic-aromatic copolyesters by Thermomonospora fusca and other thermophilic compost isolates. Appl. Environ. Microbiol. 64, 1731–1735. doi: 10.1128/aem.64.5.1731-1735.1998
Kumar Sen, S., and Raut, S. (2015). Microbial degradation of low density polyethylene (LDPE): a review. J. Environ. Chem. Eng. 3, 462–473. doi: 10.1016/j.jece.2015.01.003
Laganà, P., Caruso, G., Corsi, I., Bergami, E., Venuti, V., Majolino, D., et al. (2019). Do plastics serve as a possible vector for the spread of antibiotic resistance? First insights from bacteria associated to a polystyrene piece from King George Island (Antarctica). Int. J. Hyg. Environ. Health 222, 89–100. doi: 10.1016/j.ijheh.2018.08.009
Lahti, L., and Shetty, S. (2019). Microbiome R Package (1.8.0). Available online at: http://microbiome.github.io (accessed March 4, 2020).
Lapanje, A., Wimmersberger, C., Furrer, G., Brunner, I., and Frey, B. (2012). Pattern of elemental release during the granite dissolution can be changed by aerobic heterotrophic bacterial strains isolated from Damma glacier (Central Alps) deglaciated granite. Microbial. Ecol. 63, 865–882. doi: 10.1007/s00248-011-9976-7
Lemos, L. N., Medeiros, J. D., Dini-Andreote, F., Fernandes, G. R., Varani, A. M., Oliveira, G., et al. (2019). Genomic signatures and co-occurrence patterns of the ultra-small Saccharimonadia (phylum CPR/Patescibacteria) suggest a symbiotic lifestyle. Mol. Ecol. 28, 4259–4271. doi: 10.1111/mec.15208
Leveau, J. H. J., Uroz, S., and de Boer, W. (2010). The bacterial genus Collimonas: mycophagy, weathering and other adaptive solutions to life in oligotrophic soil environments. Environ. Microbiol. 12, 281–292. doi: 10.1111/j.1462-2920.2009.02010.x
Loredo-Treviño, A., Gutiérrez-Sánchez, G., Rodríguez-Herrera, R., and Aguilar, C. N. (2012). Microbial enzymes involved in polyurethane biodegradation: a review. J. Polym. Environ. 20, 258–265. doi: 10.1007/s10924-011-0390-5
Love, M. I., Huber, W., and Anders, S. (2014). Moderated estimation of fold change and dispersion for RNA-seq data with DESeq2. Genome Biol. 15, 1–21. doi: 10.1186/s13059-014-0550-8
Luláková, P., Perez-Mon, C., Šantrůčková, H., Ruethi, J., and Frey, B. (2019). High-alpine permafrost and active-layer soil microbiomes differ in their response to elevated temperatures. Front. Microbiol. 10:668. doi: 10.3389/fmicb.2019.00668
Malard, L. A., and Pearce, D. A. (2018). Microbial diversity and biogeography in Arctic soils. Environ. Microbiol. Rep. 10, 611–625. doi: 10.1111/1758-2229.12680
Martin, M. (2011). Cutadapt removes adapter sequences from high-throughput sequencing reads. EMBnet J. 17, 10. doi: 10.14806/ej.17.1.200
McMurdie, P. J., and Holmes, S. (2013). Phyloseq: an R package for reproducible interactive analysis and graphics of microbiome census data. PLoS One 8:e0061217. doi: 10.1371/journal.pone.0061217
Mohammadipanah, F., and Wink, J. (2016). Actinobacteria from arid and desert habitats: diversity and biological activity. Front. Microbiol. 6:1541. doi: 10.3389/fmicb.2015.01541
Mori, T., Kitano, S., and Kondo, R. (2003). Biodegradation of chloronaphthalenes and polycyclic aromatic hydrocarbons by the white-rot fungus Phlebia lindtneri. Appl. Microbiol. Biotechnol. 61, 380–383. doi: 10.1007/s00253-003-1253-3
Muroi, F., Tachibana, Y., Kobayashi, Y., Sakurai, T., and Kasuya, K. I. (2016). Influences of poly(butylene adipate-co-terephthalate) on soil microbiota and plant growth. Polym. Degrad. Stabil. 129, 338–346. doi: 10.1016/j.polymdegradstab.2016.05.018
Muyzer, G., Teske, A., Wirsen, C. O., and Jannasch, H. W. (1995). Phylogenetic relationships of Thiomicrospiraspecies and their identification in deep-sea hydrothermal vent samples by denaturing gradient gel electrophoresis of 16S rDNA fragments. Arch. Microbiol. 164, 165–172. doi: 10.1007/BF02529967
Nakajima-Kambe, T., Ichihashi, F., Matsuzoe, R., Kato, S., and Shintani, N. (2009a). Degradation of aliphatic-aromatic copolyesters by bacteria that can degrade aliphatic polyesters. Polym. Degrad. Stabil. 94, 1901–1905. doi: 10.1016/j.polymdegradstab.2009.08.006
Nakajima-Kambe, T., Toyoshima, K., Saito, C., Takaguchi, H., Akutsu-Shigeno, Y., Sato, M., et al. (2009b). Rapid monomerization of poly(butylene succinate)-co-(butylene adipate) by Leptothrix sp. J. Biosci. Bioeng. 108, 513–516. doi: 10.1016/j.jbiosc.2009.05.018
Nauendorf, A., Krause, S., Bigalke, N. K., Gorb, E. V., Gorb, S. N., Haeckel, M., et al. (2016). Microbial colonization and degradation of polyethylene and biodegradable plastic bags in temperate fine-grained organic-rich marine sediments. Mar. Pollut. Bull. 103, 168–178. doi: 10.1016/j.marpolbul.2015.12.024
Nawaz, A., Hasan, F., and Shah, A. A. (2015). Degradation of poly(ε-caprolactone) (PCL) by a newly isolated Brevundimonas sp. strain MRL-AN1 from soil. FEMS Microbiol. Lett. 362, 1–7. doi: 10.1093/femsle/fnu004
Nilsson, R. H., Larsson, K. H., Taylor, A. F. S., Bengtsson-Palme, J., Jeppesen, T. S., Schigel, D., et al. (2019). The UNITE database for molecular identification of fungi: handling dark taxa and parallel taxonomic classifications. Nucleic Acids Res. 47, D259–D264. doi: 10.1093/nar/gky1022
Oberbeckmann, S., Kreikemeyer, B., and Labrenz, M. (2018). Environmental factors support the formation of specific bacterial assemblages on microplastics. Front. Microbiol. 8:2709. doi: 10.3389/fmicb.2017.02709
Oberbeckmann, S., Osborn, A. M., and Duhaime, M. B. (2016). Microbes on a bottle: substrate, season and geography influence community composition of microbes colonizing marine plastic debris. PLoS One 11:e159289. doi: 10.1371/journal.pone.0159289
O’Brine, T., and Thompson, R. C. (2010). Degradation of plastic carrier bags in the marine environment. Mar. Pollut. Bull. 60, 2279–2283. doi: 10.1016/j.marpolbul.2010.08.005
Ogonowski, M., Motiei, A., Ininbergs, K., Hell, E., Gerdes, Z., Udekwu, K. I., et al. (2018). Evidence for selective bacterial community structuring on microplastics. Environ. Microbiol. 20, 2796–2808. doi: 10.1111/1462-2920.14120
Pathak, V. M., and Navneet (2017). Review on the current status of polymer degradation: a microbial approach. Bioresour. Bioprocess 4:15. doi: 10.1186/s40643-017-0145-9
Pérez-Pantoja, D., Donoso, R., Agulló, L., Córdova, M., Seeger, M., Pieper, D. H., et al. (2012). Genomic analysis of the potential for aromatic compounds biodegradation in Burkholderiales. Environ. Microbiol. 14, 1091–1117. doi: 10.1111/j.1462-2920.2011.02613.x
Pham, V. H. T., Jeong, S. W., and Kim, J. (2015). Aquabacterium olei sp. nov., an oil-degrading bacterium isolated from oil-contaminated soil. Int. J. Syst. Evol. Microbiol. 65, 3597–3602. doi: 10.1099/ijsem.0.000458
Piehl, S., Leibner, A., Löder, M. G. J., Dris, R., Bogner, C., and Laforsch, C. (2018). Identification and quantification of macro- and microplastics on an agricultural farmland. Sci. Rep. 8:17950. doi: 10.1038/s41598-018-36172-y
Pontes, A., Ruethi, J., Frey, B., Aires, A., Thomas, A., Overy, D., et al. (2020). Cryolevonia gen. nov. and Cryolevonia schafbergensis sp. nov., a cryophilic yeast from ancient permafrost and melted sea ice. Int. J. Syst. Evol. Microbiol. 70, 2334–2338. doi: 10.1099/ijsem.0.004040
Pushkareva, E., Eckhardt, K. U., Hotter, V., Frossard, A., Leinweber, P., Frey, B., et al. (2020). Chemical composition of soil organic matter and potential enzyme activity in the topsoil along a moisture gradient in the High Arctic (Svalbard). Geoderma 368, 114304. doi: 10.1016/j.geoderma.2020.114304
Quast, C., Pruesse, E., Yilmaz, P., Gerken, J., Schweer, T., Yarza, P., et al. (2013). The SILVA ribosomal RNA gene database project: improved data processing and web-based tools. Nucleic Acids Res. 41, 590–596. doi: 10.1093/nar/gks1219
R Development Core Team (2008). R: A Language and Environment for Statistical Computing. (3.6.2). Vienna: R Foundation for Statistical Computing.
Rice, A. V., and Currah, R. S. (2005). Oidiodendron: a survey of the named species and related anamorphs of Myxotrichum. Stud. Mycol. 53, 83–120. doi: 10.3114/sim.53.1.83
Rillig, M. C., Lehmann, A., de Souza Machado, A. A., and Yang, G. (2019). Microplastic effects on plants. New Phytol. 223, 1066–1070. doi: 10.1111/nph.15794
Rime, T., Hartmann, M., and Frey, B. (2016). Potential sources of microbial colonizers in an initial soil ecosystem after retreat of an alpine glacier. ISME J. 10, 1625–1641. doi: 10.1038/ismej.2015.238
Rosenberg, E., DeLong, E. F., Lory, S., Stackebrandt, E., and Thompson, F. (2014). The Prokaryotes: Actinobacteria. Berlin: Springer.
Satola, B., Wübbeler, J. H., and Steinbüchel, A. (2013). Metabolic characteristics of the species Variovorax paradoxus. Appl. Microbiol. Biot. 97, 541–560. doi: 10.1007/s00253-012-4585-z
Scheurer, M., and Bigalke, M. (2018). Microplastics in Swiss Floodplain soils. Environ. Sci. Technol. 52, 3591–3598. doi: 10.1021/acs.est.7b06003
Schloss, P. D., Westcott, S. L., Ryabin, T., Hall, J. R., Hartmann, M., Hollister, E. B., et al. (2009). Introducing mothur: open-source, platform-independent, community-supported software for describing and comparing microbial communities. Appl. Environ. Microbiol. 75, 7537–7541. doi: 10.1128/AEM.01541-09
Secretariat of the Convention of Biological Diversity (2016). Marine Debris: Understanding, Preventing and Mitigating the Significant Adverse Impacts on Marine and Coastal Biodiversity. CBD Technical Series (Vol. 83). Montreal, QC: Convention of Biological Diversity, doi: 10.1080/14888386.2007.9712830
Shen, M., Zhang, Y., Zhu, Y., Song, B., Zeng, G., Hu, D., et al. (2019). Recent advances in toxicological research of nanoplastics in the environment: a review. Environ. Pollut. 252, 511–521. doi: 10.1016/j.envpol.2019.05.102
Shi, Y., Grogan, P., Sun, H., Xiong, J., Yang, Y., Zhou, J., et al. (2015). Multi-scale variability analysis reveals the importance of spatial distance in shaping Arctic soil microbial functional communities. Soil Biol. Biochem. 86, 126–134. doi: 10.1016/j.soilbio.2015.03.028
Shogren, R. L., Doane, W. M., Garlotta, D., Lawton, J. W., and Willett, J. L. (2003). Biodegradation of starch/polylactic acid/poly(hydroxyester-ether) composite bars in soil. Polym. Degrad. Stabil. 79, 405–411. doi: 10.1016/S0141-3910(02)00356-7
Singh, B., and Sharma, N. (2008). Mechanistic implications of plastic degradation. Polym. Degrad. Stabil. 93, 561–584. doi: 10.1016/j.polymdegradstab.2007.11.008
Smith, M., Love, D. C., Rochman, C. M., and Neff, R. A. (2018). Microplastics in seafood and the implications for human health. Curr. Environ. Health Rep. 5, 375–386. doi: 10.1007/s40572-018-0206-z
Ssekagiri, A. (2020). microbiomeSeq: Microbial Community Analysis in an Environmental Context (0.1). Available online at: http://www.github.com/umerijaz/microbiomeSeq (accessed March 4, 2020).
Starr, E. P., Shi, S., Blazewicz, S. J., Probst, A. J., Herman, D. J., Firestone, M. K., et al. (2018). Stable isotope informed genome-resolved metagenomics reveals that Saccharibacteria carbon. Microbiome 6:122.
Tejeda-Agredano, M. C., Gallego, S., Vila, J., Grifoll, M., Ortega-Calvo, J. J., and Cantos, M. (2013). Influence of the sunflower rhizosphere on the biodegradation of PAHs in soil. Soil Biol. Biochem. 57, 830–840. doi: 10.1016/j.soilbio.2012.08.008
Thiel, M., Luna-Jorquera, G., álvarez-Varas, R., Gallardo, C., Hinojosa, I. A., Luna, N., et al. (2018). Impacts of marine plastic pollution from continental coasts to subtropical gyres-fish, seabirds, and other vertebrates in the SE Pacific. Front. Mar. Sci. 5:238. doi: 10.3389/fmars.2018.00238
Uchida, H., Nakajima-Kambe, T., Shigeno-Akutsu, Y., Nomura, N., Tokiwa, Y., and Nakahara, T. (2000). Properties of a bacterium which degrades solid poly(tetramethylene succinate)-co-adipate, a biodegradable plastic. FEMS Microbiol. Lett. 189, 25–29. doi: 10.1016/S0378-1097(00)00246-9
Ventura, M., Canchaya, C., Tauch, A., Chandra, G., Fitzgerald, G. F., Chater, K. F., et al. (2007). Genomics of actinobacteria: tracing the evolutionary history of an ancient phylum. Microbiol. Mol. Biol. Rev. 71, 495–548. doi: 10.1128/mmbr.00005-07
Wallace, P. W., Haernvall, K., Ribitsch, D., Zitzenbacher, S., Schittmayer, M., Steinkellner, G., et al. (2017). PpEst is a novel PBAT degrading polyesterase identified by proteomic screening of Pseudomonas pseudoalcaligenes. Appl. Microbiol. Biotechnol. 101, 2291–2303. doi: 10.1007/s00253-016-7992-8
Wang, J., Lv, S., Zhang, M., Chen, G., Zhu, T., Zhang, S., et al. (2016). Effects of plastic film residues on occurrence of phthalates and microbial activity in soils. Chemosphere 151, 171–177. doi: 10.1016/j.chemosphere.2016.02.076
Wang, Q., Garrity, G. M., Tiedje, J. M., and Cole, J. R. (2007). Naïve Bayesian classifier for rapid assignment of rRNA sequences into the new bacterial taxonomy. Appl. Environ. Microbiol. 73, 5261–5267. doi: 10.1128/AEM.00062-07
White, T., Bruns, T., Lee, S., and Taylor, J. (1990). “Amplification and direct sequencing of fungal ribosomal RNA genes for phylogenetics,” in PCR Protocols: A Guide to Meth- Ods and Applications, eds M. Innis, D. Gelfand, J. Sninsko, and T. White (San Diego, CA: Academic Press), 315–322.
Wickham, H. (2016). ggplot2: Elegant Graphics for Data Analysis (3.2.1). New York, NY: Springer-Verlag.
Wilkes, R. A., and Aristilde, L. (2017). Degradation and metabolism of synthetic plastics and associated products by Pseudomonas sp.: capabilities and challenges. J. Appl. Microbiol. 123, 582–593. doi: 10.1111/jam.13472
Willumsen, P. A., Johansen, J. E., Karlson, U., and Hansen, B. M. (2005). Isolation and taxonomic affiliation of N-heterocyclic aromatic hydrocarbon-transforming bacteria. Appl. Microbiol. Biotechnol. 67, 420–428. doi: 10.1007/s00253-004-1799-8
Wilson, F. P., Liu, X., Mattes, T. E., and Cupples, A. M. (2016). Nocardioides, Sediminibacterium, Aquabacterium, Variovorax, and Pseudomonas linked to carbon uptake during aerobic vinyl chloride biodegradation. Environ. Sci. Pollut. Res. Int 23, 19062–19070. doi: 10.1007/s11356-016-7099-x
Winquist, E., Björklöf, K., Schultz, E., Räsänen, M., Salonen, K., Anasonye, F., et al. (2014). Bioremediation of PAH-contaminated soil with fungi - From laboratory to field scale. Int. Biodeter. Biodegr. 86, 238–247. doi: 10.1016/j.ibiod.2013.09.012
Wolf, A. B., Vos, M., De Boer, W., and Kowalchuk, G. A. (2013). Impact of matric potential and pore size distribution on growth dynamics of filamentous and non-filamentous soil bacteria. PLoS One 8:e83661. doi: 10.1371/journal.pone.0083661
Woudenberg, J. H. C., Groenewald, J. Z., Binder, M., and Crous, P. W. (2013). Alternaria redefined. Stud. Mycol. 75, 171–212. doi: 10.3114/sim0015
Wright, S. L., and Kelly, F. J. (2017). Plastic and human health: a micro issue? Environ. Sci. Technol. 51, 6634–6647. doi: 10.1021/acs.est.7b00423
Yagi, H., Ninomiya, F., Funabashi, M., and Kunioka, M. (2014). Mesophilic anaerobic biodegradation test and analysis of eubacteria and archaea involved in anaerobic biodegradation of four specified biodegradable polyesters. Polym. Degrad. Stabil. 110, 278–283. doi: 10.1016/j.polymdegradstab.2014.08.031
Zettler, E. R., Mincer, T. J., and Amaral-Zettler, L. A. (2013). Life in the “plastisphere”: microbial communities on plastic marine debris. Environ. Sci. Technol. 47, 7137–7146. doi: 10.1021/es401288x
Keywords: alpine soil, arctic soil, biodegradation, bioplastics, microbiome, plastisphere, Saccharimonadales
Citation: Rüthi J, Bölsterli D, Pardi-Comensoli L, Brunner I and Frey B (2020) The “Plastisphere” of Biodegradable Plastics Is Characterized by Specific Microbial Taxa of Alpine and Arctic Soils. Front. Environ. Sci. 8:562263. doi: 10.3389/fenvs.2020.562263
Received: 14 May 2020; Accepted: 28 August 2020;
Published: 24 September 2020.
Edited by:
Montserrat Filella, Université de Genève, SwitzerlandReviewed by:
Cristina Silva Pereira, New University of Lisbon, PortugalCiro Sannino, University of Perugia, Italy
Copyright © 2020 Rüthi, Bölsterli, Pardi-Comensoli, Brunner and Frey. This is an open-access article distributed under the terms of the Creative Commons Attribution License (CC BY). The use, distribution or reproduction in other forums is permitted, provided the original author(s) and the copyright owner(s) are credited and that the original publication in this journal is cited, in accordance with accepted academic practice. No use, distribution or reproduction is permitted which does not comply with these terms.
*Correspondence: Beat Frey, beat.frey@wsl.ch