- 1Shamir Research Institute, University of Haifa, Qatzrin, Israel
- 2Water Science Department, Tel-Hai College, Upper Galilee, Israel
- 3Department of Biotechnology Engineering, ORT Braude College, Karmiel, Israel
- 4Department of Geography and Environmental Studies, University of Haifa, Haifa, Israel
A very effective removal of nitrate in batch and continuous experiments was achieved by a newly biofilm-formative isolated bacterium, identified by 16S rRNA as Acinetobacter EMY. The anoxic denitrifying capabilities of Acinetobacter EMY, attached to plastic biocarriers in batch and continuous moving bed bioreactors, demonstrated up to 1.75 times higher nitrate removal compared with a bacterial suspension. The denitrification rates of nitrate (200 mg/l) in the continuous operation mode were 0.39, 0.65, 1.23, and 1.14 kg-N/m3/d, with hydraulic retention times (HRTs) of 12, 8, 4, and 2 h, respectively, whereas the batch reactor removal performance showed up to 1.49 kg-N/m3/d. To the best of our knowledge, these findings are the highest values obtained for nitrate removal in comparison to previous studies focused on the characterization of denitrifying isolates. In addition, this bacterium is able to consume all of the organic matter provided in solution together with the nitrate, without leaving any residuals of organic matter in the water. This is advantageous since nitrate removal treatments by heterotrophic bacteria usually require addition of organic matter to the system, leading to secondary pollution. The isolated bacterium therefore provides a good solution for biological treatment of nitrogen in water, particularly in treatment systems that integrate immobilized biomass in the treatment process.
Introduction
Accumulation of nitrogen compounds in the environment and in drinking water reservoirs may cause the development of eutrophication and algal blooms, which threaten the quality of the water and the ecosystem. High concentrations of nitrate in drinking water can cause health problems (methanoglobanemia in infants and stomach cancer in adults). Therefore, wastewater treatment and aquaculture facilities are investing great efforts to develop highly efficient denitrification processes, and various technologies have been developed to improve the efficiency of nitrate removal (Bernhard, 2010; Paul, 2014; Zhang et al., 2015; Pan et al., 2017). While chemical and physical removal methods such as ion exchange, reverse osmosis, and electrodialysis have been found to be cost-ineffective due to high costs (installation and maintenance), biological removal of nitrogen compounds from water has been found to be both effective and much more economical (Garbisu et al., 1991; Sharma and Sobti, 2012; Chen et al., 2020).
Biological removal of inorganic nitrogen compounds is usually based on a two-stage process involving nitrification followed by denitrification. The main drawback of biological treatment, however, is that it relies on the microorganisms populating the water treatment plants and the environmental conditions prevailing in the system. Furthermore, nitrifying and denitrifying bacteria generally require a relatively long hydraulic retention time (HRT) due to their slow growth rate. Therefore, stress events, low temperatures, continuous operation, and insufficient HRTs are likely to lead to the withdrawal and dilution of the nitrifying and denitrifying bacteria biomass from the treatment facility bioreactors (Chen et al., 1998; Joo et al., 2005).
Biomass immobilization has been found to be a cost-effective and efficient solution to the above mentioned challenges. Biomass immobilization offers many advantages, including protection from harsh environmental conditions (pH fluctuations, temperature fluctuations, presence of toxic compounds), increased biodiversity in the bioreactor (suspended and attached bacteria), the bacterial community can be selectively controlled and the amount of biomass per unit volume can be significantly increased. However, most importantly, immobilization of the biomass to surfaces in the bioreactor prevents the dilution of the bacterial community also under low HRT (Takei et al., 2011; Kurzbaum et al., 2017).
Yuan et al. (2015) and Hou et al. (2019) suggested that denitrification by an immobilized biofilm on carriers is an effective approach with high potential for biological treatment of water containing with high nitrate concentrations. The biomass may be originate from the natural microflora of the treated water body or added as a specific cultures that are inoculated to the water, as was done in the present study. Bioaugmentation is effective mainly in cases where the natural bacterial community does not remove a sufficient amount of nitrogen. In this context, immobilization of the added culture strain(s) enables control of the location and amount of the bacterial community and its long-term maintenance (Rezaee et al., 2010; Naik and Setty, 2012; Menashe and Kurzbaum, 2016; Bassin et al., 2017; Quan et al., 2018; Hou et al., 2019).
Biological denitrification may be achieved in an autotrophic or a heterotrophic manner. Heterotrophic denitrification is favorable in water treatment facilities as it demonstrates higher efficiencies (Wilawan et al., 2010). However, secondary pollution by bacterial growth and the presence of organic residuals due to excessive organic nutrient supplementation, may damage the quality of the effluent. Thus, the challenge of growing microbial populations that exploit a low C:N ratio on the one hand, and consume most or all of the supplemented organic matter, on the other hand, is important for reaching the best results (Kim et al., 2008; Godini et al., 2010; Rezaee et al., 2010; Wilawan et al., 2010; Kube et al., 2019). Therefore, finding bacteria with denitrifying capabilities and efficient organic matter consumption, which are able to form a biofilm that will enhance their immobilization, opens up new opportunities for effective treatment of water rich in nitrogen compounds.
The current study examined the effectiveness of nitrate removal from a synthetic wastewater by a new bacterium isolated from a phenols treating bioreactor. This isolate showed an impressive ability to form biofilm under both aerobic and anoxic conditions, and to perform a very effective denitrification. The nitrogen-removal process was examined under different C:N ratios in both batch and continuous moving bed bioreactors by immobilizing the bacterium on polyethylene carriers, and nitrate and organic matter consumption were determined.
Materials and Methods
Bacterial Isolation and Identification Using the 16S rRNA Gene Sequencing
A denitrifying bacterium, named EMY, capable of degrading phenol as a sole carbon source under both aerobic and anoxic conditions, was isolated from a laboratory bioreactor treating high concentrations of phenolic compounds. This isolate exhibited a very rapid growth rate and the ability to form a very thick and fast-growing biofilm when grown on phenol and sodium citrate (data not shown, manuscript in preparation), thus it was further investigated for its denitrifying capabilities under anoxic conditions in suspension and when immobilized in a moving bed bioreactor (MMBR) see section “Batch Moving Bed Bioreactor (Different C:N Ratios” and “Continuous Moving Bed Bioreactor Operation.” The isolate was grown on a nutrient agar plate using streak plate procedure and incubated for 24 h at 28°C. Subsequently, a single colony was collected using a sterile loop and subjected to PCR analysis using (Sebastião et al., 2015) primers targeting the 16S rRNA gene [27F (AGAGTTTGATCMTGGCTCAG) and 1513R (ACGGYTACCTTGTTACGACTT)]. The PCR procedure was as follows: DNA was denatured at 95°C for 5 min, followed by 30 cycles comprising 95°C for 30 s, 58°C for 30 s and 72°C for 1 min, followed by 5 min at 72°C. The PCR product was sequenced by Sanger method at Hy-labs (Rehovot, Israel). The partial 16S rRNA gene sequence (798 bp) was analyzed by BLASTN against the NCBI nt database. Aligned sequences of > 96% sequence similarity were obtained from full genome entries, representing related named species. Those species, which included the Acinetobacter EMY novel strain sequence, were used to construct a phylogenetic tree. The sequences were aligned using SINA (version 1.2.11; Pruesse et al., 2012) and an approximate-maximum likelihood tree was calculated with FastTree (Price et al., 2010) using a GTR model and Gamma distribution of likelihood. The sequence of the 16S rRNA gene fragment was submitted to GenBank under Accession number MT410617.
Synthetic Wastewater Medium
Bacteria for the different experiments were grown in synthetic wastewater (Minimal Salts Medium – MSM) at 30°C and shaken at 120 rpm. The composition of this substrate (in g/l) was 6.15 Na2HPO4∗H2O, 1.53 KH2PO4, 0.2 MgSO4∗7H2O, and 0.038 CaCl2 and 10 ml of a metal medium containing (in g/l) 0.5 EDTA – NaFe salt, 0.2 FeSO4∗7H2O, 0.01 ZnSO4∗7H2O, 0.003 MnCl2∗4H2O, 0.03 H3BO3, 0.02 CoCl2∗6H2O, 0.001 CuCl2∗2H2O, 0.002 NiCl2∗6H2O, and 0.003 Na2MoO4∗2H2O. 200 mg/l nitrate (KNO3) as nitrogen source and 600 mg/l sodium citrate tribasic dihydrate as carbon source were supplemented to the MSM.
Analytical Methods
Water Quality Tests
Concentrations of nitrate, ammonium, nitrite, total nitrogen, and chemical oxygen demand (COD) were measured and calibrated against a calibration curve using designated solutions; 1.14538.0065, 1.14682.0495, and 1.14539.0495 (Merck Millipore, Israel) for spectrophotometers. The reading was performed on a UV-visible spectrophotometer (Ultrospec 2100 UV-visible, Biochrom). Estimation of the biomass of the bacterial suspension in the water samples was measured by a spectrophotometer at 600 nm wavelength. The concentration of dissolved oxygen was measured by an optical electrode (ProODO, YSI) and the pH was measured by an electrode (Eutech pH 450, Thermo Fisher Scientific).
Test to Quantify the Protein on the Polyethylene Biocarriers
Protein quantification, as a measure of the amount of biomass in the biofilm on the polyethylene (plastic) biocarriers, was performed according to the Bradford protocol (Bradford, 1976). Briefly, this method includes separating the biomass from one, previously washed, biocarrier (with sterile saline and Tween 20) to 4 ml 1 N NaOH solution in a test 50 ml tube, followed by heating in a water bath (80°C for 30 min) and sedimentation by centrifugation (10,000 rpm for 15 min). Hundred microliter l of each suspension was added to 5 ml of 0.01% (w/v) G-250 Coomassie brilliant blue (Bio-Rad, Israel) reagent. Optical density (OD) measurements were performed by spectrophotometer (Ultrospec 2100 UV-visible, Biochrom) at 595 nm wavelength against standards prepared with bovine serum albumin. A control with clean biocarrier was treated the same in order to verify that the quantification of the protein is accurate and that the OD reflect only the proteins originated from the bacterial cells in the biofilm. All measurements were done in triplicates.
Visualization of Acinetobacter EMY Biofilm on a Biocarrier Using a Binocular Fluorescent Microscope
The bacterial biofilm on the biocarrier was visualized using SYTO 9 (Thermo Fisher Scientific) to stain live bacteria during the experimental runs. The biofilm was stained by soaking the biocarrier in SYTO 9 solution in room temperature for 15 min. Imaging was performed with a fluorescent binocular microscope (Olympus SZX16, Japan). Sterile biocarriers (without bacteria), as controls, were stained as described above and examined using the same procedure.
Flask Experiment (Variable C:N Ratios)
In preliminary experiments, the new isolate was found to have a very efficient nitrate removal rate under anoxic conditions. In order to characterize the most efficient C:N ratio for anoxic denitrification by the new isolate, an experiment was planned to examine denitrification activity at C:N ratios of 1:1, 2:1, and 3:1, where the bacterium grew in suspension. Sodium citrate was added as organic matter after being filtered at 0.2 μm through a syringe filter at a suitable concentration for the experiment (according to the C:N ratio) and the initial nitrogen concentration was set at 200 mg/l as nitrate. The flasks were hermetically sealed and shaked at 100 rpm at 32°C for homogeneity of the experimental solution. Water samples were taken every 24 h to test the water quality and the amount of bacteria. The sterile control treatment was performed identically, without the presence of the bacterium. Each treatment and control pair was performed in triplicate.
The parameters measured included concentrations of nitrate, ammonium, nitrite, COD and reading of the optical density (OD600). The total nitrogen concentration was measured at the beginning and end of the experiment, dissolved oxygen and pH were measured at the end of the experiment. All of the liquids and the experimental equipment were sterilized in an autoclave. All of the inoculation, isolation, acclimation and water sampling procedures were performed in a biological laminar flow unit.
Batch Moving Bed Bioreactor (Different C:N Ratios)
In this experiment the biomass denitrification capabilities was examined while immobilized as a biofilm to a plastic (polyethylene) surface. Immobilization of Acinetobacter EMY on polyethylene biocarriers was performed in a 500 ml batch bioreactor into which we introduced 100 sterile biocarriers. The biofilm growth of Acinetobacter EMY on biocarriers took 10 days, where each day the MSM medium containing 200 mg/l nitrate, was replaced with fresh medium. Both length and diameter of an individual biocarrier is 25 mm and the surface area is 420 m2/m3 (Hel-X, Stoehr, Germany).
Three consecutive treatments were performed in this bioreactor; in each treatment the source of carbon was introduced at a different C:N ratio—1:1, 2:1 or 3:1—where the initial nitrate concentration was 200 mg/l and the sodium citrate concentration varied according to the planned C:N ratio for each treatment. Between each treatment the biomass was washed gently using sterile saline (0.85%) (two volume beds) in order to exclude excess organic matter between the treatments. Before each treatment, nitrogen gas was bubbled gently for 20 min until the level of dissolved oxygen in the medium was < 0.1 mg/l in order to provide anoxic condition.
Nitrate, ammonium, nitrite and COD concentrations were measured throughout the experiment. At the end of each treatment three biocarriers were taken for quantification of the protein in the biofilm as a measure of bacterial biomass. The temperature was maintained at 32°C using a circulating water bath (Cario CD, Julabo); the substrate was slowly whirled with a magnetic stirrer throughout the experiment to create homogeneous conditions but without introducing oxygen into the substrate. An identical bioreactor was set up in parallel, as a sterile control, with identical conditions, without the presence of the bacterium, in order to examine changes in different parameter values that were not derived from biological activity.
Continuous Moving Bed Bioreactor Operation
A continuous degradation study was conducted in the same bioreactor described above in the batch operation mode but this time under continuous flow. The influent MSM medium was fed into the bioreactor by a peristaltic pump (Masterflex, Cole-Parmer) at different flow rates (0.0624, 0.1248, 0.2496, and 0.0198 l/h) to obtain different HRTs of 12, 8, 4, and 2 h. Before each treatment, nitrogen gas was bubbled gently for 20 min until the level of dissolved oxygen in the medium was < 0.1 mg/l in order to provide anoxic condition. Nitrate, COD, ammonium and nitrite concentrations in the influent and effluent were monitored throughout the experiments, while dissolved oxygen and pH were measured in the effluent once for each flow rate. Control experiments were conducted in exactly the same way, but with sterile biocarriers, to ascertain that nitrate removal is a biological process. These experiments were conducted with a C:N ratio of 3:1. The temperature was maintained at 32°C using a circulating water bath (Cario CD, Julabo); the substrate was slowly whirled with a magnetic stirrer throughout the experiment. At the end of each treatment three biocarriers were removed for quantification of the protein in the biofilm. Figure 1 presents the experimental setup.
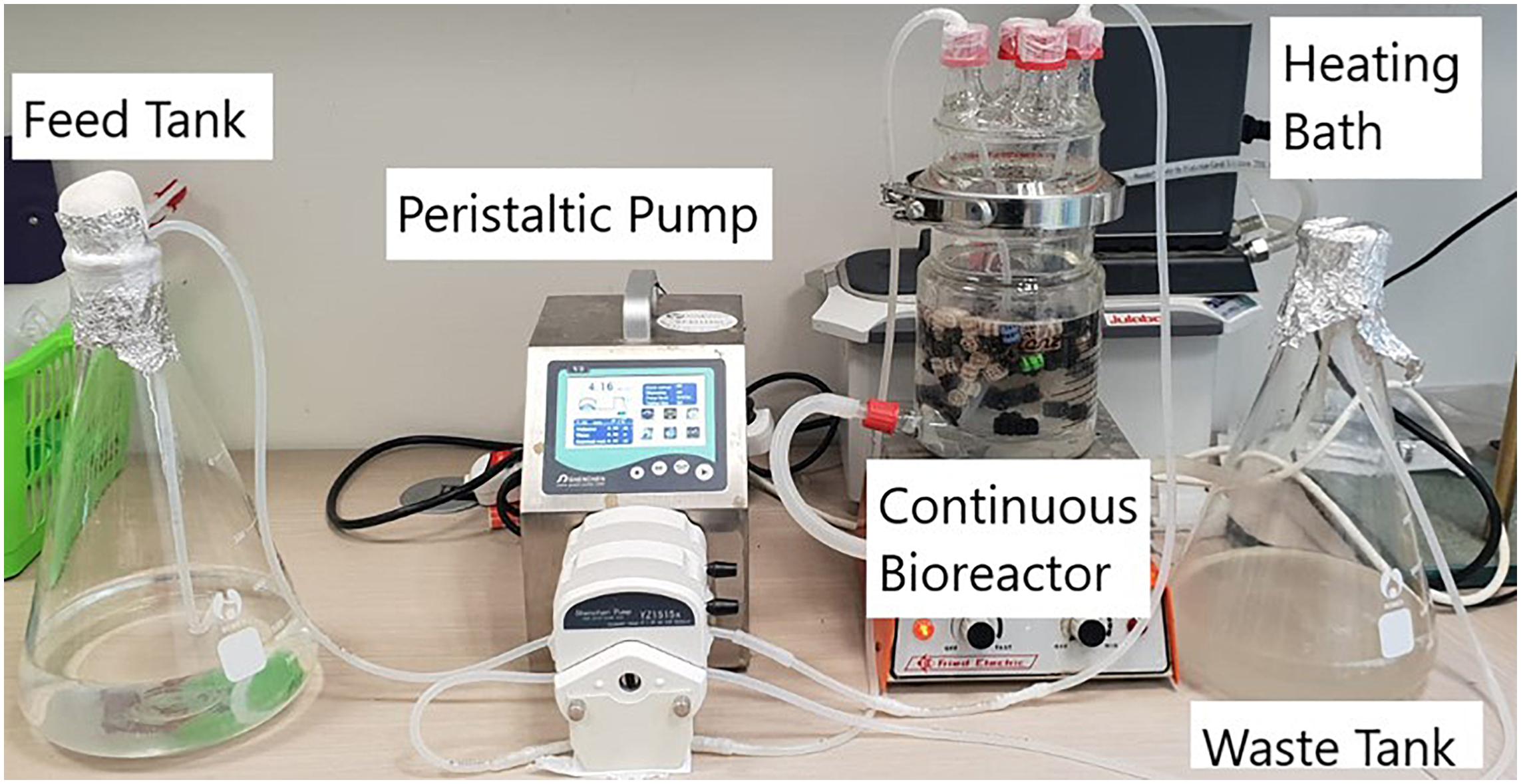
Figure 1. Photograph of the continuous moving bed bioreactor setup including immobilized biomass of Acinetobacter EMY.
Statistical Analysis
Two-way analysis of variance (ANOVA) was performed with GraphPad Prism version 6.05 (GraphPad Software Inc.). The data in the graphs are means of three replicates ++ standard deviation. Mean differences were considered to be significant at p ≤ 0.05.
Results and Discussion
Bacterial Taxonomic Identification by 16S Marker Gene (Phylogenetic Tree)
The 16S rRNA sequence of the bacterium showed 99% similarity to an Acinetobacter pittii strain present in the NCBI database. This sequence was used to construct a phylogenetic tree (Figure 2), which showed a clear affiliation to the Acinetobacter genus. The new strain isolated was named Acinetobacter EMY (Accession number MT410617).
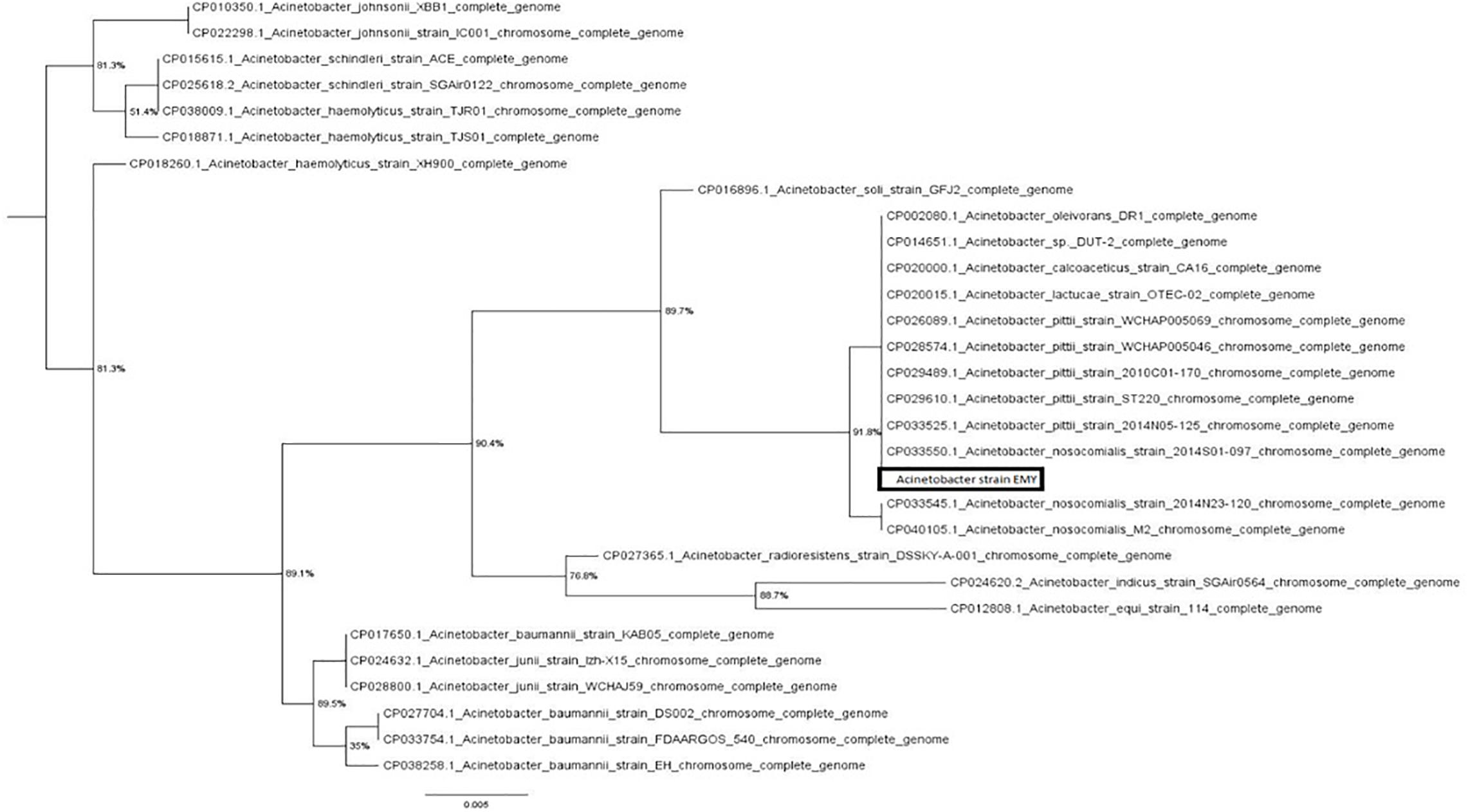
Figure 2. Approximate maximum likelihood tree based on a partial 16S rRNA gene sequence of Acinetobacter strain EMY. The scale bar represents the rate of substitution per site. Numbers at nodes indicate local support values estimated by the Shimodaira-Hasegawa test based on 1,000 re-samplings.
Bacteria of the genus Acinetobacter are known to be Gram-negative and occur usually in pairs. Most of them are aerobic, but some have been identified as facultative aerobes. They are most commonly found in soil and water, and play an important role in the decomposition of soil pollutants (e.g., aromatic compounds). Some have the ability to grow in saline environments and high temperatures. Most of the species in this genus do not reduce nitrate to molecular nitrogen (Doughari et al., 2009; Bitrian et al., 2013). However, several studies pointed on Acinetobacter spp. as a good candidate for nitrogen removal from water. For example, ammonium removal by Acinetobacter sp. Y16 capable of heterotrophic nitrification–aerobic denitrification at low temperature and at low C/N was shown to have a napA gene that proved its aerobic denitrification ability (Huang et al., 2013). Another study by Ren et al. (2014) isolated Acinetobacter junii form activated sludge and studied its hydroxylamine, nitrite and nitrate utilization. They found that this bacterium has a high aggregation and hydrophobicity nature a fact that may explain the biofilm growth nature as shown in this study.
Flask Experiment
Initially, in order to determine the nitrate removal ability of Acinetobacter EMY by denitrification at variable C:N ratios, a batch experiment was conducted in flasks with variable organic matter (COD) concentrations. A decrease in the concentrations of nitrate and COD took place in parallel to an increase in the amount of biomass (OD600) and significant differences were observed among the C:N ratios (Figure 3). A C:N ratio of 1:1 enabled a limited decrease in the nitrate concentration and cessation of biomass growth was observed after 24 h. The COD concentration decreased to only ca. 50 mg/l during this time. In contrast, a C:N ratio of 2:1 enabled a 50% decrease in nitrate concentration within 48 h, and it seems that the COD was depleted and biomass growth ceased. Complete nitrate removal was recorded with a C:N ratio of 3:1, where 200 mg/l were removed within 72 h. There was no decrease in nitrate concentration or COD in any of the three control treatments, emphasizing the necessity of the biological process for nitrate removal from the water. Measurements of dissolved oxygen, pH and concentrations of ammonium and nitrite supported the presence of the denitrification process (Table 1). The pH of the treatments with C:N ratios of 1:1, 2:1, and 3:1 was 8.68, 8.77, and 8.43, respectively, while dissolved oxygen was 5.33, 4.68, and 0.46 mg/l, respectively (the dissolved oxygen at the beginning of the experiment was 7.8 ± 0.1).
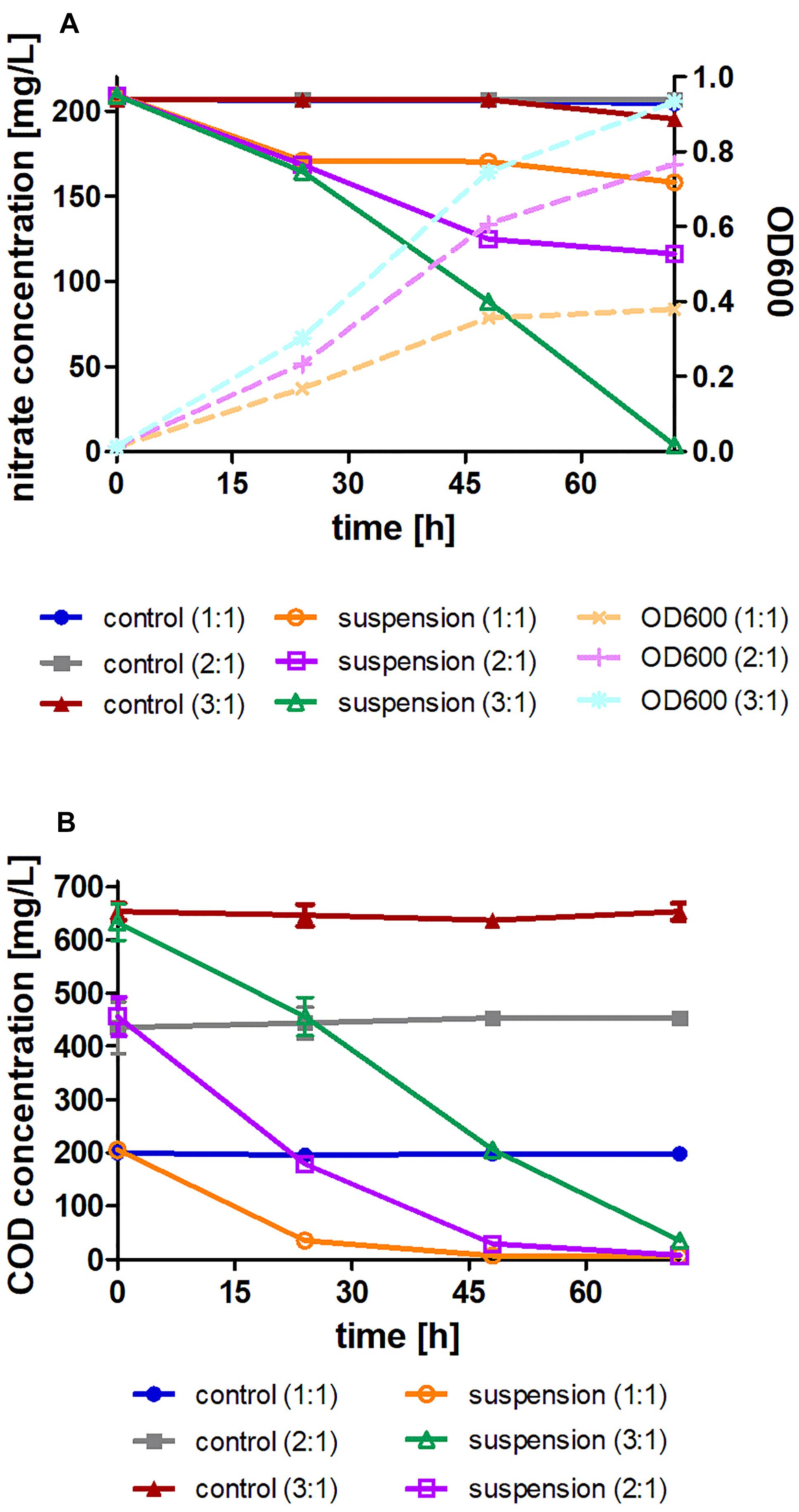
Figure 3. Flask experiment. (A) Nitrate concentration over time, where the initial concentration was 200 mg/l; treatment vs. sterile control with three different C:N ratios (1:1, 2:1, 3:1); estimation of bacterial biomass growth in suspension is presented as OD600. (B) COD concentration over time with the initial concentration varying according to the C:N ratio; treatment vs. sterile control at three different C:N ratios (1:1, 2:1, 3:1). The data are means of three replicates + standard deviation.
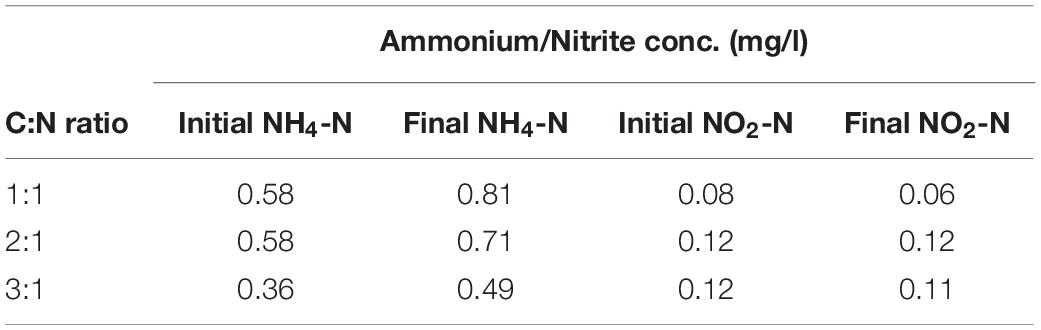
Table 1. Ammonium and nitrite concentrations in mg/l at the beginning and end of the flask experiment, at C:N ratios of 1:1, 2:1, and 3:1.
It can be concluded that nitrate removal by anoxic denitrification was achieved only in the 3:1 C:N ratio treatment as the dissolved oxygen showed 0.46 mg/l at the end of the experiment, probably due to the fact that less organic matter brought to lower bacterial activity. Additionally, the obtained results of nitrogen removal depend directly on the concentration of the organic matter. The study by Hamlin et al. (2008) supports this result and further claims that the type of organic matter and the amount added to the system is highly significant when conducting experiments to improve the efficacy of the denitrification process. The study by Wilawan et al. (2010) also reported similar results to ours. Their study reported that a C:N ratio of 1.5:1 leads to removal of 46.5% of the nitrate, a ratio of 2.5:1 leads to removal of 52% of the nitrate, and a ratio of 3.5:1 leads to removal of 63.1% of the nitrate. In contrast, the study by Sobieszuk and Szewczyk (2006) shows that the type and amount of organic matter are indeed significant, but the amount added should be limited because excess addition may be ineffective. Their study proved that a C:N ratio greater than 6:1 is not effective and hinders the bacterial denitrification activity. Conversely, Chen et al. (2020) studied Pseudomonas denitrificans G1, a facultative anaerobic denitrifying strain. They concluded that at a C:N ratio of 3:1 the denitrification was poor and the strain needed a ratio of at least 5:1 with best performance at a ratio of 8:1. These studies show that the new Acinetobacter EMY strain may be a good candidate to be used in denitrification processes in water treatment facilities as low C:N ratios are more cost-effective to operate and cause less bacterial growth (sludge accumulation) and residuals of organic matter in effluents.
Based on the measurement of total nitrogen concentrations at the beginning and the end of each treatment it is proved that removal of nitrogen by anoxic denitrification indeed took place. It is assumed that the conversion of the nitrate to molecular nitrogen and its expulsion from the system caused the reduction of the total nitrogen parameter (Figure 4). Likewise, it can be seen that a C:N ratio of 3:1 enabled removal of ca. 50% of total nitrogen, while the remainder of the nitrogen was accumulated to the biomass as no nitrate was remained in the medium. Although the nitrate removal was high, 50% was consumed by the culture to build biomass and was not used as an electron acceptor in the denitrification process. This ratio is higher than in the experiments conducted by Chen et al. (2020) on aquaculture effluents purification bioaugmented with a Pseudomonas denitrificans G1 culture. However, previous studies by Zhang et al. (2018) and Zhao et al. (2018), which focused on pure denitrifying cultures (Paracoccus versutus KS293 Pseudomonas stutzeri strain XL-2) and measured the intracellular nitrogen of the culture, showed a markedly lower assimilation ratio of nitrogen into the cells (only 12–15% of the nitrate was assimilated by the cells). Another pathway for nitrogen loss in such conditions is its conversion to Nitrous Oxides which were not measured in the present study (but is suggested in further studies).
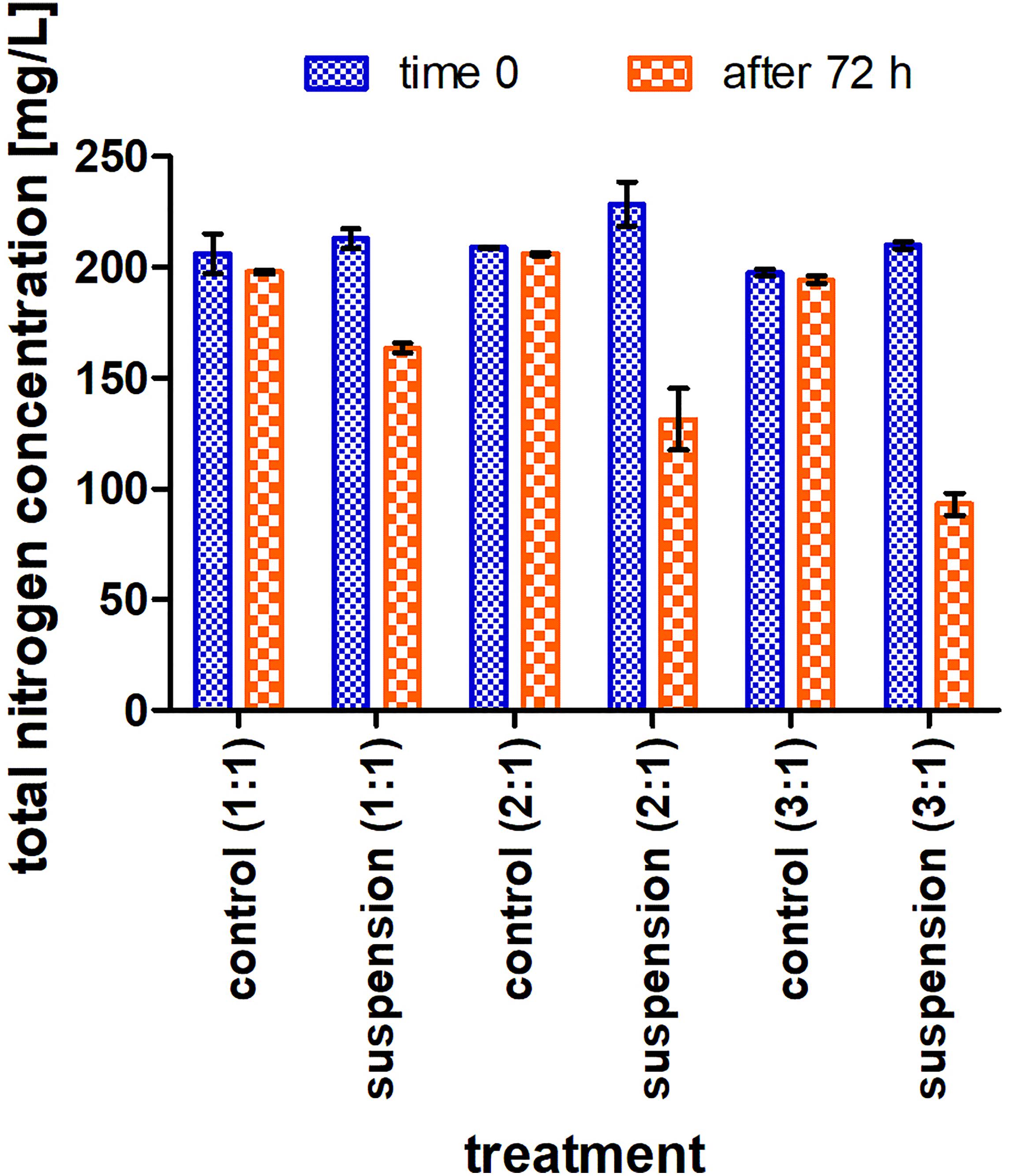
Figure 4. Total nitrogen concentration at time 0 and at the end of the flask experiment, where the nitrogen source is nitrate at an initial concentration of 200 mg/l; treatment vs. sterile control at three different C:N ratios (1:1, 2:1, and 3:1). The data are means of three replicates + standard deviation.
In summary, in the flask experiment it was shown that Acinetobacter EMY removed 200 mg/l nitrate within 72 h. When C:N ratio was 3:1, 50% of the nitrate removal may be attributed to denitrification while the rest is probably adsorbed and assimilated by the biomass for growth.
Batch Moving Bed Bioreactor
In this experiment, the biomass was immobilized onto polyethylene biocarriers and placed in a bioreactor with different C:N ratios (1:1, 2:1, and 3:1), in order to improve the efficacy of the nitrogen removal rate by the isolated bacterium.
The results presented in Figure 5A show the reduction of nitrate concentration over time. Nitrogen removal at all three C:N ratios was performed within 4–6 h with no differences between treatments. The control treatments did not show any change. In comparison to removal in suspension (Figure 3), there is a significant improvement in the rate of nitrate removal by immobilized biomass (0.84 vs. 1.34 kg-N/m3/d, respectively), at a C:N ratio of 3:1.
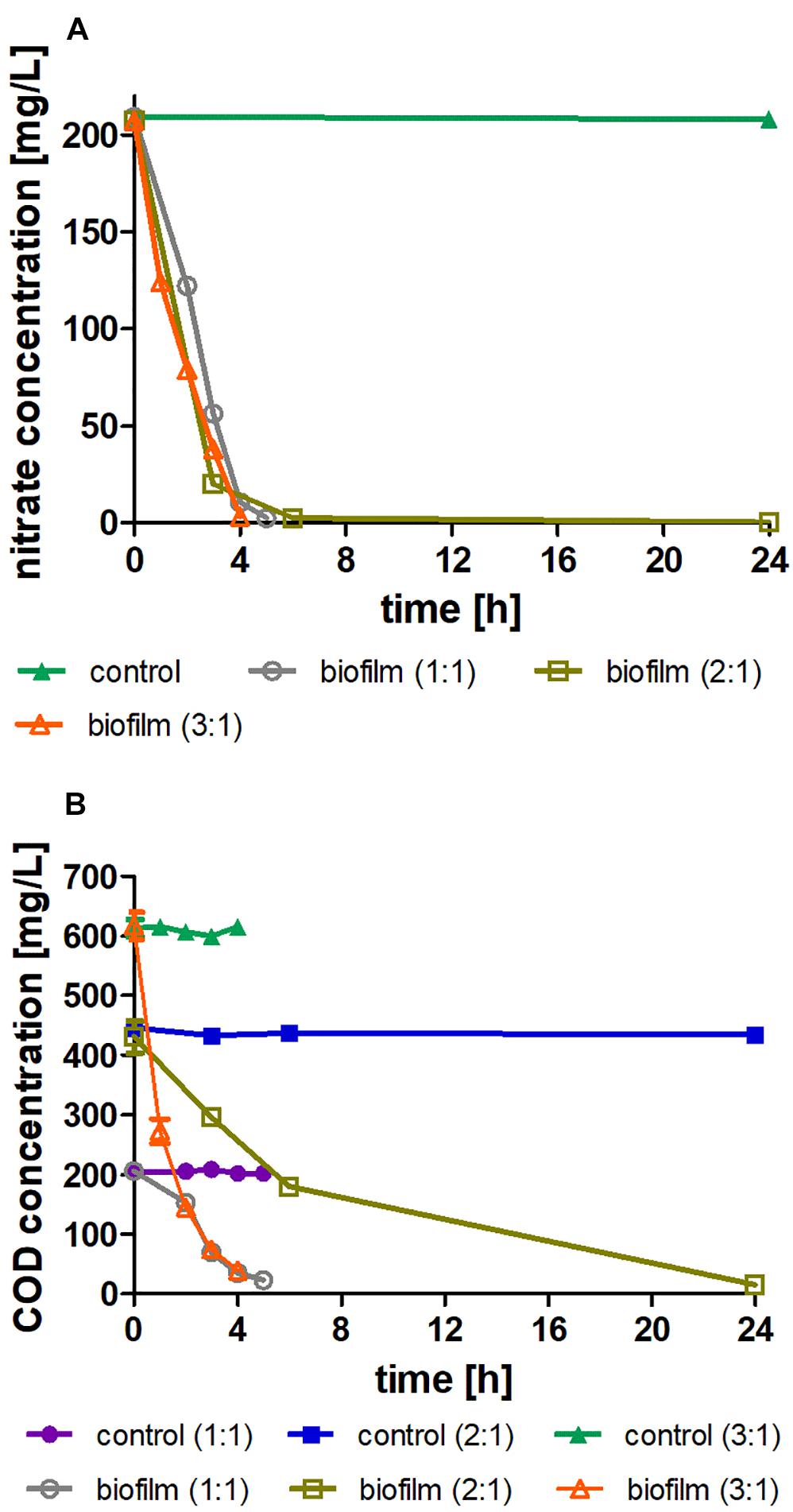
Figure 5. (A) Nitrate concentration with time, where the initial concentration is 200 mg/l; treatment vs. sterile controls in biomass immobilized in aqueous solution (batch moving bed bioreactor) at three different C:N ratios (1:1, 2:1, and 3:1) and in non-immobilized biomass in suspension (flasks) at a C:N ratio of 3:1. (B) COD concentration over time, where the initial concentration varies according to the C:N ratio; treatment vs. sterile controls at three different C:N ratios (1:1, 2:1, and 3:1). The data are means of three replicates + standard deviation.
The decrease in COD concentration (Figure 5B) appears to match the decrease in nitrate concentration. COD consumption is accelerated as more COD is added to the medium, and in the immobilized biomass system the nitrate removal is sufficiently fast even at low amounts of COD (C:N ratio of 1:1). The concentration of ammonium and nitrate in the solution at the beginning and the end of the experiment showed no accumulation of these substances (Table 2).
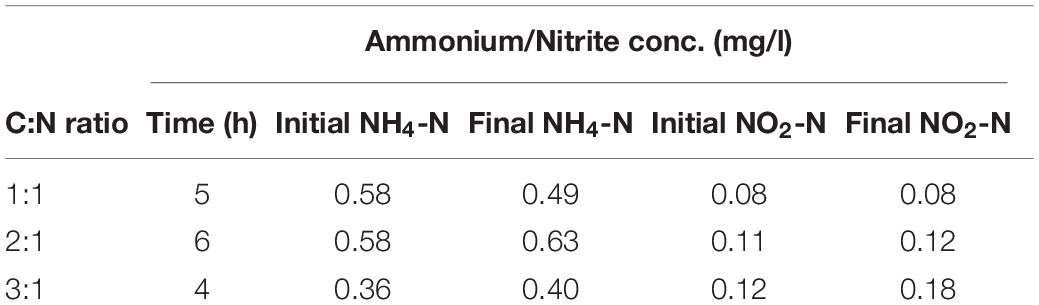
Table 2. Concentration of ammonium and nitrite in mg/l at the beginning and end of the batch moving bed bioreactor experiment; C:N ratios of 1:1, 2:1, and 3:1.
The rapid removal that was achieved in this experiment was facilitated by the amount of biofilm that developed on the carriers. Figure 6 presents a photograph of a plastic carrier that illustrates the amount of living biomass on the carrier. From the estimate of the amount of bacterial protein, the amount of protein removed was found to be 4.25 mg per individual carrier, in other words, 425 mg protein in the entire bioreactor.
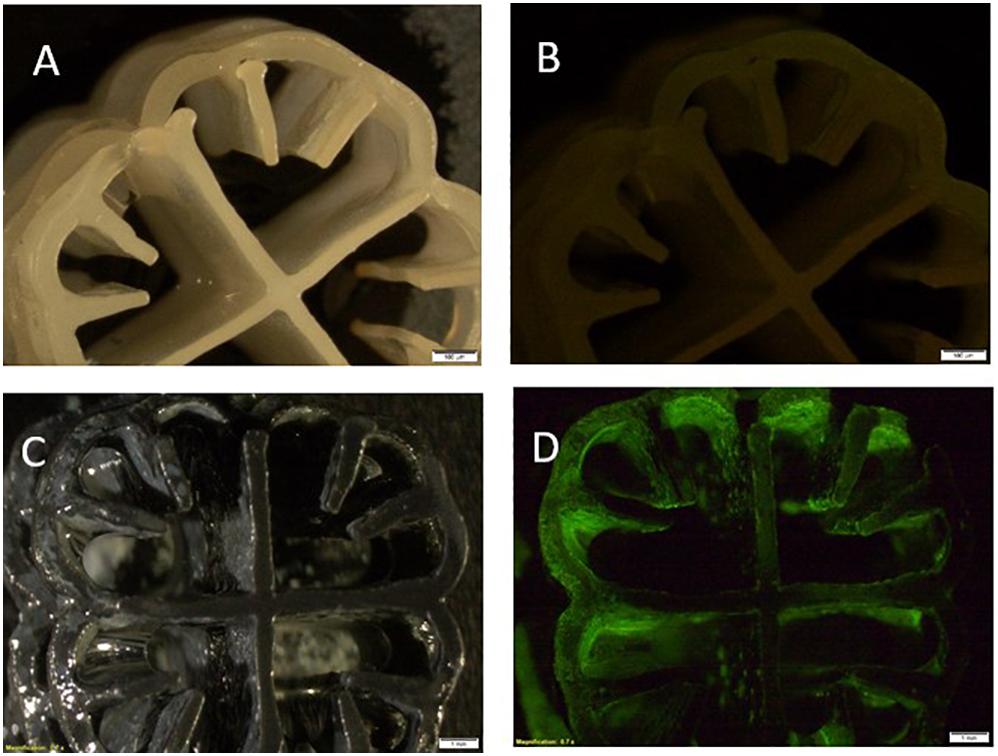
Figure 6. Photograph of polyethylene biocarrier containing biomass of Acinetobacter EMY as a biofilm. Photographs through a microscope: (A) Sterile biocarrier without staining; (B) Sterile biocarrier with fluorescent staining; (C) Biofilm on biocarrier without staining; (D) Biofilm on biocarrier with fluorescent staining.
Similar studies presented slower nitrate removal rate than those reported here (Table 3). Firstly, the study by Li et al. (2019), which focused on treatment of reverse osmosis concentrate containing nitrate by biomass fixed to polyethylene biocarriers, reported a denitrification rate of 0.08 kg-N/m3/d. Similarly, the study by Zhao et al. (2011) focused on nitrate removal in anoxic conditions by immobilizing biomass on biofilm biocarriers. This unique experiment used an electrode to enable nitrate removal at C:N ratios below 1:1 and reported a removal rate of 0.15 kg-N/m3/d. Another study by Yuan et al. (2015) reported a nitrate removal rate of 0.23 kg-N/m3/d with immobilized biomass containing a number of bacterial species on volcanic rocks (tuff). However, the nitrate removal rate reported in the present study for a C:N ratio of 1:1 was much higher with 1.22 kg-N/mg3.d nitrate removal. Indeed, in a comprehensive literature review we found no studies that reported better results than those reported in the present experiment.
Continuous Moving Bed Bioreactor
Biomass immobilization of Acinetobacter EMY was shown to be effective and might be a solution for challenges related to the water treatment process. Thus, the present experiment examined nitrate removal in a continuous system that would allow nitrate removal from large volumes of water.
Figure 7A reports results of nitrate concentration from a continuous bioreactor system, in which synthetic wastewater continuously flowed at different rates. Total removal of nitrate occurs at HRTs of 12 and 8 h. At HRTs of 4 and 2 h, an increase in the nitrate concentration in the effluent was observed, although after acclimation a decrease could be observed. Figure 7B presents the COD concentration at the entrance and exit of the system; the results are in line with those presented in Figure 7A for nitrate removal. Organic matter appears to be important for the nitrate removal process, and may in fact be a limiting factor. We note that COD decreased to a negligible level, indicating total consumption of all of the organic matter provided in the solution. The values of ammonium and nitrite were tested in parallel and found to be under the limit of detection.
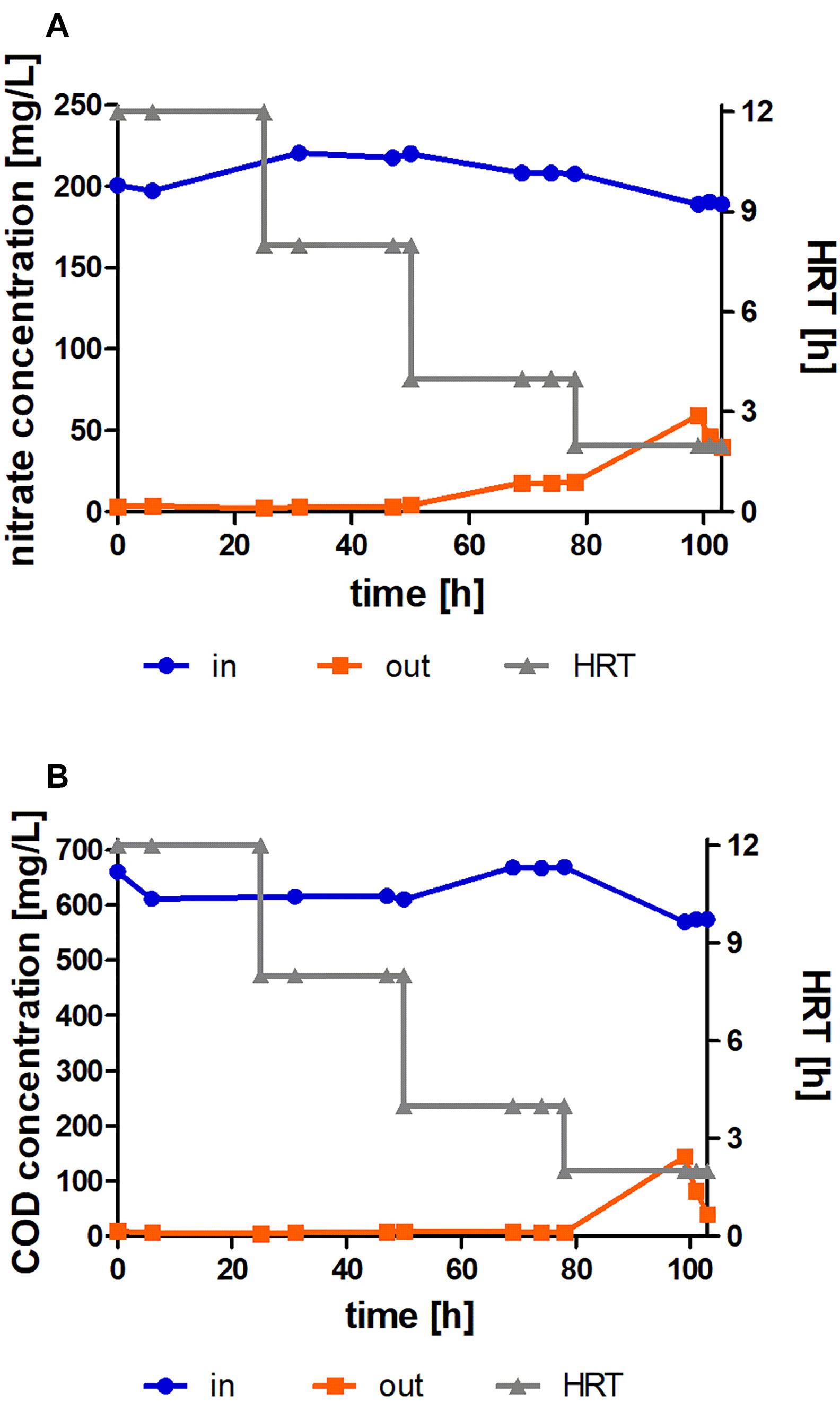
Figure 7. (A) Nitrate concentration at the entrance and exit of the continuous moving bed bioreactor with time, where the initial concentration is 200 mg/l, at variable hydraulic retention times (HRTs) (2, 4, 8, and 12 h) in continuous experiment with biomass immobilized in aqueous solution at a C:N ratio of 3:1. (B) COD concentration at the entrance of the continuous bioreactor with time, where the initial concentration is ca. 700 mg/l, at variable HRTs (2, 4, 8, and 12 h) vs. COD concentration at the exit of the continuous bioreactor, when the initial concentration is ca. 700 mg/l at variable HRTs (2, 4, 8, and 12 h) in biomass immobilized in aqueous solution at a C:N ratio of 3:1. The data are means of three replicates + standard deviation.
Figure 3 shows that the biomass of Acinetobacter EMY has doubled during the 24 h of the experiment. However, in Figure 7 an HRT of 4 h, as measured in the continuous experiment, would thus not allow the bacterial population to efficiently remove the nitrate due to biomass wash-out from the bioreactor. This fact strengthens the claim that immobilizing the bacteria allows maintenance of a sufficient bacterial population in the bioreactor for performing nitrate removal during short HRTs (e.g., a few hours).
The literature on nitrate removal in continuous bioreactors with immobilized biomass shows variable results (Table 4). The study by Tatara et al. (2017) achieved a nitrate removal rate of 0.043 kg-N/m3/d with an HRT of 24 h, while the study by Wang et al. (2009) showed a nitrate removal rate of 0.32 kg-N/mg3.d. These results are lower than those reported in the present study. Rezaee et al. (2010) achieved a nitrate removal rate of 0.54 kg-N/m3/d with an HRT of 9 h, in comparison to 1.23 kg-N/mg3.d reported in the present study with an HRT of only 4 h. In contrast to these three previous studies, Godini et al. (2010) presented a nitrate removal rate of 1.61 kg-N/m3/d, with an initial nitrate concentration of 200 mg/l and an HRT of 3 h. These results are slightly better than those reported in our study, perhaps because their study included several bacterial populations.
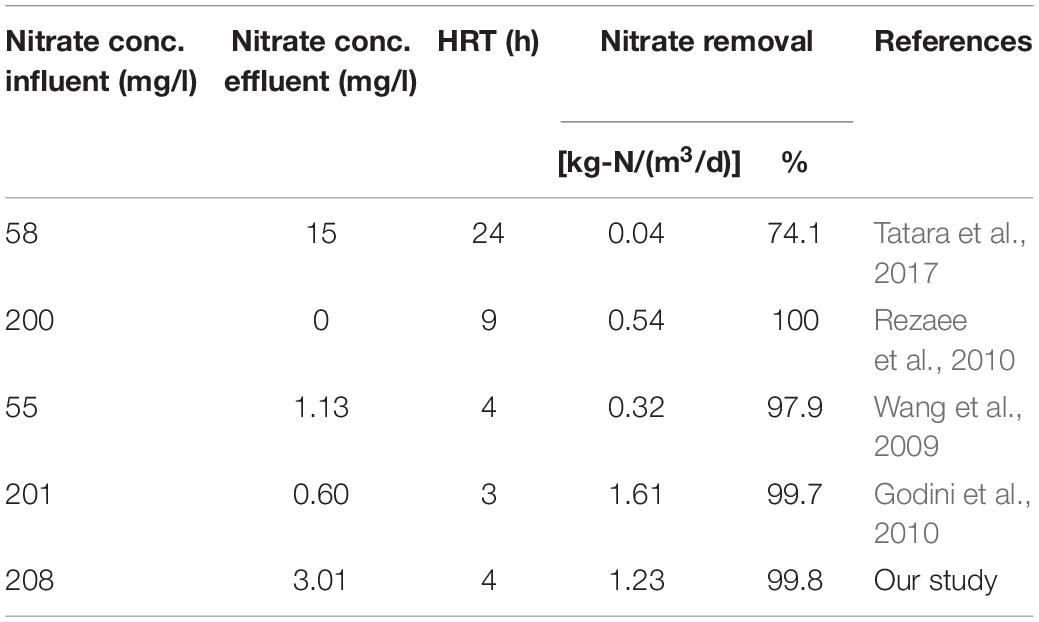
Table 4. Comparison between the literature and our study of the rate and percent of nitrate removal in continuous moving bed bioreactor experiments with immobilized biomass.
In recent years, several studies have investigated the biological removal of nitrate from different emerging sources. These include studies that focused on nitrate removal from reverse osmosis (RO) concentrate or from the regeneration step of ion exchange columns (which may contain more than 1,000 mg/l nitrate). The disposal methods of RO concentrate from groundwater facilities treating saline or/and nitrate-rich groundwater or from polishing of municipal effluent are restricted by laws and high capital costs. Thus, the development of a biological treatment approach is promising since it contributes to prevention of environmental pollution (Panagopoulos et al., 2019). The new isolate reported in this study formed a biofilm and showed a faster denitrification rate than reported in other studies as well as negligible organic matter residues in the treated water. Thus, future research should focus on the development of a bioaugmentation process using this isolate and others with high denitrification efficiency to remove nitrate from RO concentrate.
In summary, this study describes a new isolated bacterium, Acinetobacter EMY, that has shown an impressive ability to perform denitrification in suspension and as a biofilm under anoxic conditions. The optimal C:N ratio for the nitrate removal process was found to be 3:1, with sodium citrate as the organic matter. Measurements of COD indicated that the isolate (as suspension and biofilm) exploits the entire amount of organic matter added to the medium. The continuous bioreactor system proved the ability of Acinetobacter EMY to completely remove ca. 200 mg/l nitrate and ca. 700 mg/l COD with an HRT of only 4 h, removing 1.23 kg-N/m3/d. Higher nitrate removal performance was achieved in the batch bioreactor with 1.49 kg-N/m3/d and a C:N ratio of 2:1. To the best of our knowledge, these findings are the highest values obtained for nitrate removal in comparison to previous studies focused on the characterization of denitrifying isolates.
Conclusion
The new isolated bacterium, belonging to Acinetobacter genus, has a higher nitrate removal rate compared to bacteria reported in previous studies. In addition, this bacterium is able to consume all of the organic matter provided in solution together with the nitrate, without leaving any residuals of organic matter in the water. This is advantageous since nitrate removal treatments by heterotrophic bacteria usually require addition of organic matter to the system, leading to secondary pollution. This combination together with its ability to efficiently form a biofilm suggest it can be used as a potential solution for nitrate polluted water. Future studies should examine how such an efficient denitrifying bacterium functions and survives during the bioaugmentation process in a water treatment system treating high nitrate concentrations (such as the treatment of RO concentrate and ground water rich in nitrate).
Data Availability Statement
The raw data supporting the conclusions of this article will be made available by the authors, without undue reservation, to any qualified researcher.
Author Contributions
EK: conceptualization, resources, visualization, project administration, and funding acquisition. EK, MK, and LI-K: methodology. YS and LI-K: formal analysis. YS and MK: investigation. YS and MK: data curation. YS and SA: writing—original draft preparation. SA and EK: writing—review and editing, supervision. All authors contributed to the article and approved the submitted version.
Funding
This research was funded by the Israeli Ministry of Science, Space and Technology (MOST) (grant no. 313498).
Conflict of Interest
The authors declare that the research was conducted in the absence of any commercial or financial relationships that could be construed as a potential conflict of interest.
Supplementary Material
The Supplementary Material for this article can be found online at: https://www.frontiersin.org/articles/10.3389/fenvs.2020.556226/full#supplementary-material
References
Bassin, J. P., Rachid, C. T., Vilela, C., Cao, S. M., Peixoto, R. S., and Dezotti, M. (2017). Revealing the bacterial profile of an anoxic-aerobic moving-bed biofilm reactor system treating a chemical industry wastewater. Int. Biodeter. Biodegr. 120, 152–160. doi: 10.1016/j.ibiod.2017.01.036
Bernhard, A. (2010). The nitrogen cycle: processes, players, and human impact. Nat. Educ. Knowl. 3(10):25.
Bitrian, M., González, R. H., Paris, G., Hellingwerf, K. J., and Nudel, C. B. (2013). Blue-light-dependent inhibition of twitching motility in Acinetobacter baylyi ADP1: additive involvement of three BLUF-domain-containing proteins. Microbiology 159, 1828–1841. doi: 10.1099/mic.0.069153-0
Bradford, M. M. (1976). A rapid and sensitive method for the quantitation of microgram quantities of protein utilizing the principle of protein-dye binding. Anal. Biochem. 7, 248–254. doi: 10.1016/0003-2697(76)90527-3
Chen, K. C., Lee, S. C., Chin, S. C., and Houng, J. Y. (1998). Simultaneous carbon-nitrogen removal in wastewater using phosphorylated PVA-immobilized microorganisms. Enzyme Microb. Technol. 23, 311–320. doi: 10.1016/s0141-0229(98)00054-4
Chen, Z., Jiang, Y., Chang, Z., Wang, J., Song, X., Huang, Z., et al. (2020). Denitrification characteristics and pathways of a facultative anaerobic denitrifying strain, Pseudomonas denitrificans G1. J. Biosci. Bioeng. 129, 715–722. doi: 10.1016/j.jbiosc.2019.12.011
Doughari, H. J., Ndakidemi, P. A., Human, I. S., and Benade, S. (2009). The ecology, biology and pathogenesis of Acinetobacter spp.: an overview. Microbes Environ. 26, 101–112. doi: 10.1264/jsme2.me10179
Garbisu, C., Gil, J. M., Bazin, M. J., Hall, D. O., and Serra, J. L. (1991). Removal of nitrate from water by foam-immobilized Phormidium laminosum in batch and continuous-flow bioreactors. J. Appl. Phycol. 3, 221–234. doi: 10.1007/bf00003580
Godini, H., Rezaee, A., Jafari, A., and Mirhousaini, S. H. (2010). Denitrification of wastewater containing high nitrate using a bioreactor system packed by microbial cellulose. World Acad. Sci. Eng. Technol. 62, 283–287.
Hamlin, H. J., Michaels, J. T., Beaulaton, C. M., Graham, W. F., Dutt, W., Steinbach, P., et al. (2008). Comparing denitrification rates and carbon sources in commercial scale upflow denitrification biological filters in aquaculture. Aquacult. Eng. 38, 79–92. doi: 10.1016/j.aquaeng.2007.11.003
Hou, T., Chen, N., Tong, S., Li, B., He, Q., and Feng, C. (2019). Enhancement of rice bran as carbon and microbial sources on the nitrate removal from groundwater. Biochem. Eng. J. 148, 185–194. doi: 10.1016/j.bej.2018.07.010
Huang, X., Li, W., Zhang, D., and Qin, W. (2013). Ammonium removal by a novel oligotrophic Acinetobacter sp. Y16 capable of heterotrophic nitrification–aerobic denitrification at low temperature. Bioresour. Technol. 146, 44–50. doi: 10.1016/j.biortech.2013.07.046
Joo, H. -S., Hirai, M., and Shoda, M. (2005). Characteristics of ammonium removal by heterotrophic nitrification-aerobic denitrification by Alcaligenes faecalis No. 4. J. Biosci. Bioeng. 100, 184–191. doi: 10.1263/jbb.100.184
Kim, M., Jeong, S. Y., Yoon, S. J., Cho, S. J., Kim, Y. H., Kim, M. J., et al. (2008). Aerobic denitrification of Pseudomonas putida AD-21 at different C/N ratios. J. Biosci. Bioeng. 106, 498–502. doi: 10.1263/jbb.106.498
Kube, M., Mohseni, A., Fan, L., and Roddick, F. (2019). Impact of alginate selection for wastewater treatment by immobilised Chlorella vulgaris. Chem. Eng. J. 358, 1601–1609. doi: 10.1016/j.cej.2018.10.065
Kurzbaum, E., Raizner, Y., Cohen, O., Suckeveriene, R. Y., Kulikov, A., Hakimi, B., et al. (2017). Encapsulated Pseudomonas putida for phenol biodegradation: use of a structural membrane for construction of a well-organized confined particle. Water Res. 121, 37–45. doi: 10.1016/j.watres.2017.04.079
Li, L., Yan, G., Wang, H., Chu, Z., Li, Z., Ling, Y., et al. (2019). Denitrification and microbial community in MBBR using a. donax as carbon source and biofilm carriers for reverse osmosis concentrate treatment. J. Environ. Sci. 84, 133–143. doi: 10.1016/j.jes.2019.04.030
Menashe, O., and Kurzbaum, E. (2016). A novel bioaugmentation treatment approach using a confined microbial environment: a case study in a membrane ioreactor wastewater treatment plant. Environ. Technol. 37, 1582–1590. doi: 10.1080/09593330.2015.1121293
Naik, S. S., and Setty, Y. P. (2012). Biological denitrification of wastewater with immobilized cells of Pseudomonas stutzeri attached to polypropylene and polyoxymethylene. Int. J. Biol. Ecol. Environ. Sci. 1, 42–45.
Pan, M., Huang, X., Wu, G., Hu, Y., Yang, Y., and Zhan, X. (2017). Performance of denitrifying phosphate removal via nitrite from slaughterhouse wastewater treatment at low temperature. Water 9(11):818. doi: 10.3390/w9110818
Panagopoulos, A., Haralambous, K. J., and Loizidou, M. (2019). Desalination brine disposal methods and treatment technologies-a review. Sci. Total Environ. 693:133545. doi: 10.1016/j.scitotenv.2019.07.351
Price, M. N., Dehal, P. S., and Arkin, A. P. (2010). FastTree 2–approximately maximum-likelihood trees for large alignments. PLoS One 5(3):e9490. doi: 10.1371/journal.pone.0009490
Pruesse, E., Peplies, J., and Glöckner, F. O. (2012). SINA: accurate high-throughput multiple sequence alignment of ribosomal RNA genes. Bioinformatics 28, 1823–1829. doi: 10.1093/bioinformatics/bts252
Quan, X., Huang, K., Li, M., Lan, M., and Li, B. (2018). Nitrogen removal performance of municipal reverse osmosis concentrate with low C/N ratio by membrane-aerated biofilm reactor. Front. Environ. Sci. Eng. 12(6):5. doi: 10.1007/s11783-018-1047-6
Ren, Y. X., Yang, L., and Liang, X. (2014). The characteristics of a novel heterotrophic nitrifying and aerobic denitrifying bacterium, Acinetobacter junii YB. Bioresour. Technol. 171, 1–9. doi: 10.1016/j.biortech.2014.08.058
Rezaee, A., Godini, H., Dehestani, S., and Kaviani, S. (2010). Isolation and characterization of a novel denitrifying bacterium with high nitrate removal: Pseudomonas stutzeri. J. Environ. Health Sci. 7, 313–318.
Sebastião, F. A., Furlan, L. R., Hashimoto, D. T., and Pilarski, F. (2015). Identification of bacterial fish pathogens in Brazil by direct colony PCR and 16S rRNA gene sequencing. Adv Microbiol. 5:409–424. doi: 10.4236/aim.2015.56042
Sharma, S. K., and Sobti, R. C. (2012). Nitrate removal from ground water: a review. J. Chem. 9, 1667–1675. doi: 10.1155/2012/154616
Sobieszuk, P., and Szewczyk, K. W. (2006). Estimation of (C/N) ratio for microbial denitrification. Environ. Technol. 27, 103–108. doi: 10.1080/09593332708618624
Takei, T., Ikeda, K., Ijima, H., and Kawakami, K. (2011). Fabrication of poly (vinyl alcohol) hydrogel beads crosslinked using sodium sulfate for microorganism immobilization. Process Biochem. 46, 566–571. doi: 10.1016/j.procbio.2010.10.011
Tatara, M., Ishikawa, S., and Ueno, Y. (2017). Continuous nitrogen removal by a single-stage reactor packed with ring-laced string medium. J Biosci. Bioeng. 124, 660–667. doi: 10.1016/j.jbiosc.2017.06.013
Wang, Q., Feng, C., Zhao, Y., and Hao, C. (2009). Denitrification of nitrate contaminated groundwater with a fiber-based biofilm reactor. Bioresource Technol. 100, 2223–2227. doi: 10.1016/j.biortech.2008.07.057
Wilawan, K., Tatsuo, S., and Futaba, K. (2010). Influence of carbon source on biological nitrogen removal by immobilised bacteria. J. Water Res. Prot. 2, 527–531. doi: 10.4236/jwarp.2010.26059
Yuan, Q., Wang, H., Hang, Q., Deng, Y., Liu, K., Li, C., et al. (2015). Comparison of the MBBR denitrification carriers for advanced nitrogen removal of wastewater treatment plant effluent. Environ. Sci. Pollut. Res. 22, 13970–13979. doi: 10.1007/s11356-015-4546-z
Zhang, H., Zhao, Z., Chen, S., Kang, P., Wang, Y., Feng, J., et al. (2018). Paracoccus versutus KS293 adaptation to aerobic and anaerobic denitrification: insights from nitrogen removal, functional gene abundance, and proteomic profiling analysis. Bioresource Technol. 260, 321–328. doi: 10.1016/j.biortech.2018.03.123
Zhang, S., Sha, C., Jiang, W., Li, W., Zhang, D., Li, J., et al. (2015). Ammonium removal at low temperature by a newly isolated heterotrophic nitrifying and aerobic denitrifying bacterium Pseudomonas fluorescens wsw-1001. Environ. Technol. 36, 2488–2494. doi: 10.1080/09593330.2015.1035759
Zhao, Y., Feng, C., Wang, Q., Yang, Y., Zhang, Z., and Sugiura, N. (2011). Nitrate removal from groundwater by cooperating heterotrophic with autotrophic denitrification in a biofilm–electrode reactor. J. Hazard. Mater. 192, 1033–1039. doi: 10.1016/j.jhazmat.2011.06.008
Keywords: denitrification, biofilm, MBBR, biocarrier, Acinetobacter
Citation: Shelly Y, Kuc ME, Iasur-Kruh L, Azerrad S and Kurzbaum E (2020) A New Acinetobacter Isolate Is an Extremely Efficient Biofilm-Formative Denitrifying Bacterium. Front. Environ. Sci. 8:556226. doi: 10.3389/fenvs.2020.556226
Received: 27 April 2020; Accepted: 09 September 2020;
Published: 23 October 2020.
Edited by:
Simona Di Gregorio, University of Pisa, ItalyReviewed by:
Zhaoji Zhang, Institute of Urban Environment (CAS), ChinaAng Li, Harbin Institute of Technology, China
Simone Becarelli, University of Pisa, Italy
Copyright © 2020 Shelly, Kuc, Iasur-Kruh, Azerrad and Kurzbaum. This is an open-access article distributed under the terms of the Creative Commons Attribution License (CC BY). The use, distribution or reproduction in other forums is permitted, provided the original author(s) and the copyright owner(s) are credited and that the original publication in this journal is cited, in accordance with accepted academic practice. No use, distribution or reproduction is permitted which does not comply with these terms.
*Correspondence: Eyal Kurzbaum, ZWt1cnpiYXVtQHVuaXYuaGFpZmEuYWMuaWw=