- 1Department of Zoology, University of Cambridge, Cambridge, United Kingdom
- 2BioRISC, St. Catharine’s College, Cambridge, United Kingdom
Freshwater ecosystems provide essential resources and vital ecosystem services. These ecosystems exist in a delicate state of balance and are under increasing anthropogenic and climatic pressures. One of the major anthropogenic threats to freshwater ecosystems is eutrophication that often leads to algal blooms, some of which may be extremely harmful. Current chemical and physical interventions to prevent algal blooms can be expensive, ephemeral and disruptive to other aspects of the ecosystem. Therefore, there is interest in utilising biological methods of control. This study aimed to assess the viability of allelopathic repression of nuisance algae species by invasive aquatic plants. The allelopathic effect of Hydrocotyle ranunculoides (Floating Pennywort) and Crassula helmsii (Swamp Stonecrop) were tested in both whole plant and crushed plant states for their ability to affect the average population growth of monocultures and co-cultures of the green algae Chlorella vulgaris and the cyanobacterium Synechocystis sp. PCC6803. Methanol extracts from these species have been identified in the literature to have high allelopathic potential. The key findings of this study are that, for Chlorella: whole H. ranunculoides and crushed C. helmsii had a negative effect on the average population growth; whole H. ranunculoides had a greater negative effect than crushed H. ranunculoides; and crushed C. helmsii had a more negative effect than crushed H. ranunculoides. For Synechocystis: crushed C. helmsii had a greater negative effect on the average population growth than crushed H. ranunculoides; and the presence of Chlorella in co-culture experiments had a universally positive effect on its average population growth. The species-specific nature of these allelopathic interactions suggests that the use of allelopathy for algal bloom control may have to be assessed on a case-by-case basis and the use of combination treatments should be assessed. Moreover, the effects exerted by allelochemicals in open water systems is likely to be direct, indirect and context specific. Although this study explores the possibility of embracing the allelopathic potential of invasive aquatic plants, we do not encourage novel introductions of invasive species into open freshwater systems. However, potential allelopathic effects could be leveraged in already invaded systems, or in closed systems within an invaded range.
Introduction
Freshwater ecosystems deliver numerous essential ecosystem services, including the provision of drinking water, food resources, diverse habitat, irrigation, and recreation. However, anthropogenic pressures on freshwater environments through increased nutrient run-off from agriculture, pollution from industrial operations, increased abstraction and the effects of climate change are counteracting the favourable clear water state freshwater systems require to provide many of these services (Moss et al., 2011; Jeppesen et al., 2017).
Many freshwater ecosystems, especially shallow lakes, exist in a delicate state of balance between alternative equilibria, either occupying a favourable clear water state dominated by bottom-rooting aquatic vegetation, or a turbid state characterised by high microalgal biomass (Scheffer et al., 1993). The most prominent anthropogenic disruption to this balance is eutrophication (Michalak et al., 2013). Eutrophication refers to the pollution of a waterbody through the addition of excess nutrient elements, such as nitrogen (N), phosphorus (P) and potassium (K). Potential sources of additional nutrients include agricultural and aquaculture runoff, sewage, atmospheric deposition and groundwater flow. Elevated nutrient conditions are one of the key causes of Harmful Algal Blooms (HABs). These accumulations of harmful algae vastly reduce water quality and disrupt aquatic ecosystems through increased turbidity (Capuzzo et al., 2015), oxygen depletion (Sun et al., 2018), shading out other organisms in the water column (Hu and Hong, 2008) and the release of algal toxins which can poison ecosystems and water supplies (Paerl et al., 2001; Azevedo et al., 2002). These effects can result in the death of large numbers of aquatic organisms; reducing biodiversity (Lund, 1965; Reynolds and Walsby, 1975; Nasri et al., 2008); reducing the aesthetic value of affected waterbodies (Mitra and Flynn, 2006); and toxins and necrotic material threaten human health by entering drinking water supplies (Lam et al., 1995).
Current methods used to control HABs include chemical, physical and biological approaches. Chemical methods include use of metals (Magdaleno et al., 2014), herbicides (Nagai et al., 2016) and photosensitisers (Pohl et al., 2015). Physical methods include ultrasound disruption (Park et al., 2017), vertical destratification through mechanical mixing (Paerl et al., 2001) and membrane filtration (Zhao et al., 2017). Despite the efficacy of chemical and physical disruption of HABs, these methodologies can be expensive, ephemeral, labour intensive and disruptive to other aspects of the ecosystem while chemical interventions can be toxic to non-target species, including humans (Sun et al., 2018). Biological approaches offer the potential of a more efficient, cost-effective, and sustainable control through the use of aquatic animals, plants and algicidal microorganisms (McLaughlan and Aldridge, 2013; Backer et al., 2015; Harke et al., 2016; Sun et al., 2018).
This study seeks to assess the feasibility of masking the effects of eutrophication by leveraging the allelopathic effects of invasive aquatic plants in freshwater ecosystems. Allelopathy is the term that describes the inhibitory or stimulatory effects of one plant or phytoplankton on another plant or phytoplankton via the release of chemical compounds into the environment (Rice, 1983). These chemical compounds are secondary metabolites referred to as allelochemicals. The allelochemicals released by aquatic plants belong to different chemical classes including oxygenated fatty acids, sulphur compounds, polyacetylenes and polyphenols (Nakai et al., 2012). These allelochemicals can have a wide range of effects such as reducing the growth of competitors, repelling herbivores, resisting pathogens and interfering with decomposition (Grutters et al., 2017). Identifying aquatic plants which may have the ability to produce and release allelochemicals which suppress the growth of harmful phytoplankton species such as cyanobacteria, would provide a highly useful tool for the management and manipulation of eutrophic freshwater ecosystems. It has been shown in numerous studies that cyanobacteria are more sensitive than green algae and diatoms to allelopathic substances (Gross and Jüttner, 2003; Hilt and Gross, 2008; Jasser, 1995; Planas et al., 1981; van Donk and van de Bund, 2002).
The majority of the research which has explored the ability of aquatic plants to affect the growth of cyanobacteria has been carried out using water soluble allelochemicals extracted from isolated plant material using methanol or through the use of exudate from target plant species (Supplemental Table S1). A recent experiment by Grutters et al. (2017) illustrated that the phylogeny, growth strategy and stoichiometry of a plant can be used to determine its allelopathic potential. Grutters et al. (2017) used methanol extracts and agar diffusion assays, to measure the allelopathic potential of 34 plants. The authors concluded that eudicot plant species with an emergent growth strategy and a high plant carbon-to-phosphorus (C:P) ratio exhibited the highest allelopathic potential. It is important to highlight that there is a fundamental difference between allelopathic potential and a true allelopathic effect. Bioassays provide a broad view of allelopathic potential, reflecting the effect of all chemicals produced by a plant species. This standardised, effect-based, comparison of allelopathic potential allows plant species with differing chemistries to be compared on an equivalent basis (Meiners, 2014). However, plants identified as having high allelopathic potential using this methodology is not evidence of allelopathy, as there is no confirmation that the chemicals driving the observed effect would be naturally excreted by the plant (Gross et al., 2007).
In order for allelopathic plants to be used in the management of eutrophic waters allelochemicals must be naturally excreted. Therefore, we aimed to observe the allelopathic effect of two invasive plant species, Hydrocotyle ranunculoides (Floating pennywort) and Crassula helmsii (Swamp stonecrop) which, according to Grutters et al. (2017), meet the criteria for high allelopathic potential. Instead of using plant extractions, we focused on the effect of the whole plant, compared to the crushed plant, on their ability to affect axenic populations of the green algae Chlorella vulgaris (hereafter Chlorella) and the cyanobacteria Synechocystis sp. PCC6803 (hereafter Synechocystis), in both co-culture and in monoculture. Testing whole plants will provide evidence of whether the allelochemicals identified through methanol extraction are naturally excreted by plants into the environment in high enough concentrations to affect phytoplankton population growth under natural conditions.
Testing for allelopathic effects in phytoplankton co-culture as well as monoculture allows for observations of potential interactions between phytoplankton taxa which may occur in natural phytoplankton communities when exposed to allelopathic compounds. If allelopathic effects are observed to consistently vary between phytoplankton taxa, particularly in co-culture experiments, this could have implications for algal bloom dynamics. For example, it has been suggested that the allelopathic impact of plants on cyanobacteria can have opposite effects depending on the presence or absence of green algae, with green algae negating the suppressive effect of allelopathy on cyanobacterial growth, and actually resulting in increased cyanobacterial growth in co-culture experiments (Chang et al., 2012).
Synechocystis and Chlorella are both small unicellular phytoplankton of similar size (∼2 µm). They were selected for this study as they have been widely used in experiments which seek to investigate algal bloom dynamics (Schubert et al., 1995; Oudra et al., 2000; Martins et al., 2005; Murray et al., 2010; Qu et al., 2014; Dervaux, Mejean and Brunet, 2015; Chen and Bridgeman, 2017). The rise of cyanobacterial blooms has been reported across many freshwater ecosystems (Huisman et al., 2018). However, due partly to the small size of these cyanobacteria, single celled, non-colonial picoplankton are still a relatively poorly studied section of phytoplankton communities, despite their increasing impact on aquatic ecosystems and water quality (Chorus and Bartrum, 1999; Paerl and Otten, 2013).
H. ranunculoides and C. helmsii are introduced non-native species in the United Kingdom. H. ranunculoides originates from the Americas, forming dense mats of vegetation which float at the water surface. C. helmsii is native to Australia and New Zealand, occurring in free floating, submerged, emergent or terrestrial forms. Although Grutters et al. (2017), conclude that invasive species do not have significantly greater allelopathic effects compared to non-invasive plants which share the same key indicators of allelopathic potential, known invasive species were selected for this study due to their ability to become ecosystem engineers. Species which can successfully invade and establish themselves in new ecosystems have characteristics which make them attractive tools for the manipulation of eutrophic freshwaters. Firstly, invasive species have the potential to assume a dominant role as ecosystem engineers in their receptive environment, as established organisms in the introduced range will often be naïve to their competitive growth strategies (Hastings et al., 2006). This ability to act as ecosystem engineers can lead to the displacement of existing organisms from the system (Crooks, 2002). Additionally, invasive species are highly resilient, allowing them to survive and thrive in unfamiliar environments. These qualities are key attributes in the potential for harnessing invasive species to manipulate eutrophic freshwater systems. It is important to emphasise that we would not advocate the introduction of invasive plants to open water systems for water quality management, but rather propose that their potential allelopathic attributes could be embraced in already invaded systems or closed systems within an invaded range.
Our research had three main hypotheses. The first hypothesis was that both whole plants and crushed plants have a negative effect on the population growth of Synechocystis and Chlorella, as these plants were identified as having high allelopathic potential by Grutters et al. (2017). The second hypothesis was that crushed plants would have a greater effect on the population growth of Synechocystis and Chlorella compared to whole plants, as allelochemicals contained within the plant would be released into the environment without having to be naturally exudated. The final hypothesis was that the relationships between the plants and Synechocystis and Chlorella, may vary between phytoplankton monoculture and co-culture conditions, with green algae potentially promoting the population growth of cyanobacteria (Chang et al., 2012). The evaluation of these hypotheses will help inform how the potential allelopathic effect of these aquatic plants could be harnessed to favourably manipulate phytoplankton communities in freshwater systems.
Methods
Cultivating Phytoplankton Species
The cyanobacteria Synechocystis sp. PCC6803 (Strain 6803 Wild Type, Pasteur Culture Collection of Cyanobacteria, Paris, France) and green alga Chlorella vulgaris (Strain 211/11B Culture Collection of Algae and Protozoa, Oban, United Kingdom) were selected for this experiment. In order to produce sufficient quantities of stock culture these species were grown in their prescribed optimal growth media at stock concentrations prior to the experiment.
Chlorella was cultured in sterile 3N-BBM-V growth media (25 g/L NaNO3; 2.5 g/L CaCl2.2H2O; 7.6 g/L MgSO4.7H2O; 7.5 g/L K2HPO4.3.H2O; 17.5 g/L KH2PO4; 2.5 g/L NaCl) and trace element solution (Minerals were added to DI water in the following sequence: 97 mg/L FeCl3.6H2O; 41 mg/L MnCl2.4H2O; 5 mg/L ZnCl2; 2 mg/L CoCl2.6H2O; 4 mg/L Na2MoO4.2H2O). Further details can be obtained at www.ccap.ac.uk. Synechocystis was grown in BG11 media (Sigma-Aldrich Cyanobacteria BG11 Freshwater Solution, Dorset, England).
Plant Collection and Processing
Hydrocotyle ranunculoides was collected from the River Cam at Fen Ditton, Cambridge, Cambridgeshire, United Kingdom (52°13′18.1″N 0°10′01.7″E). Crassula helmsii was collected from Bewl Water Reservoir, East Sussex, United Kingdom (51°04′22.5″N 0°23′38.6″E). One week prior to the experiment, plants were cleaned with dechlorinated water to remove any soil and debris. The plants were kept in dechlorinated water with saturated levels of nutrient solution (1.5 mg P/L as K2HPO4 and 12 mg N/L as NaNO3) (Vanderstukken et al., 2014) and 24 h light.
The plants were prepared on 14 and 15 February 2018. Both H. ranunculoides and C. helmsii were tested for their allelopathic effects in a whole plant and crushed plant state, in both cases 40 g of plant wet weight was used. A dual blade food processor was used to prepare the crushed plants. The plant was loaded into the food processor and run on high setting for 3–5 min until the plant matter was sufficiently processed. In the case of whole plants, a sufficient number of stems and leaves were used in order to reach a wet weight of 40 g. For the control, H. ranunculoides and C. helmsii were replaced by 40 g of fake aquarium plant (Tall Aquarium Plant, Pistachio Pet Ltd, London, United Kingdom). Only whole state aquarium plant was used.
The whole, crushed and fake plants were placed in dialysis tubing with a molecular weight cut-off of 14,000 (Sigma-Aldrich Dialysis Tubing Cellulose Membrane D9402, Dorset, England). The tubing was cut into ninety sections of equal length and each section was tied closed at one end. After the addition of the relevant plant material to the tubing, the section of tubing was filled with nutrient saturated dechlorinated water and the open end tied closed. The use of dialysis tubing is an established methodology in testing for allelopathic interactions between aquatic plants and phytoplankton with the highest degree of realism (Gross et al., 2007). The membrane prevents direct contact between the plant and microalgae while still allowing the movement of nutrients and allelochemicals, and therefore any noted effects should be attributable to allelopathy (Körner and Nicklisch, 2002; Chang et al., 2012; Priyadarshani and Rath, 2012; Vanderstukken et al., 2014).
Experimental Design
The experiment used a nested design with six replicates per treatment. Treatments monitored the population growth of Chlorella alone, Synechocystis alone, or Chlorella + Synechocystis in co-culture. Experiments were run in the presence and absence of intact and processed H. ranunculoides; intact and processed C. helmsii; and a control experiment was run in the presence of intact aquarium-safe plastic plants, such that a total of ninety experiments were undertaken.
Experiments were run in 1 L capacity aquaria (129 × 133 × 133 mm). Each aquarium was filled to a total volume of 250 ml. The aquaria were first filled with the required volume of dechlorinated and nutrient saturated water (1.5 mg P/L as K2HPO4 and 12 mg N/L as NaNO3) (Vanderstukken et al., 2014); followed by the phytoplankton inoculum added from a stock culture using a syringe attached to a 4 mm diameter hose; and the allocated plant, inside the dialysis tubing, was added last.
A LED lighting unit (Mithril Technology Ltd., Surrey, United Kingdom) was fitted 20 cm above the aquaria, emitting light 24 h per day at specific wavelengths (370 nm, 470 nm, 525 nm, 570 nm, 590 nm and 610 nm). The average Photosynthetically Active Radiation (PAR) level was 26 μmol m−2 s−1. The positioning of the aquaria was randomised to eliminate the effect of differential lighting on algal growth in different treatments. Each aquarium was supplied with constant air through a 4 mm internal diameter silicone air line from a standard aquarium pump and permeated through an air stone. The temperature of the room was maintained between 18 °C and 20 C.
A calibrated FluoroProbe (bbe Moldaenke GmbH, Schwentinental, Germany) was used to estimate the number of Chlorella and Synechocystis cells present in each sample. The bbe++ software package (bbe Moldaenke GmbH, 2013) was used to set the measurement parameters, operate the fluoroprobe and record the results of the experiments. The fluoroprobe uses spectral fluorescence to quantify phytoplankton biomass through selective excitation of accessory pigments which differ between the major taxonomic groups of phytoplankton (Catherine et al., 2012). The measurement of subsequent emissions of fluorescence by reaction centre chlorophyll α (Chlα) allows the probe to estimate µgL−1 of Chlα which can be transformed into an estimate of phytoplankton cells/ml. The bbe++ software package applies conversion factors for calculating cell counts derived from group specific Chlα properties, these conversion factors are: 5.30 × 105 for green algae, and 1.0 × 106 for cyanobacteria (Hartmann et al., 2019). The fluoroprobe was mounted in the ‘workstation 25 standard version' in which the fluoroprobe can analyse the contents of a 25 ml cuvette.
The measurements were carried out between the 15 and February 26, 2018. Samples from each aquarium were taken at 12 intervals, once per day at approximately midday. After resuspending settled phytoplankton, a 25 ml sample was collected from each aquarium at each time point using a syringe then added to the fluoroprobe cuvette for measurement. After the measurement was recorded, the 25 ml sample was replaced in the aquarium to maintain an overall aquarium volume of 250 ml for the duration of the experiment. The cuvette was rinsed with deionised (DI) water and dried between each measurement. The aquaria were twice topped up, once per week, with additional saturating concentrations of nutrient stock solution, to maintain a nutrient saturated state.
Statistical Analysis
To discern whether the growth of Chlorella or Synechocystis was affected by the type of plant present in the treatment (H. ranunculoides, C. helmsii or Fake), the state of the plant (crushed or whole) or co-culture conditions the following methodology was used. The average increase or decrease in the number of cells per hour for each of the 120 recorded timeseries (a single timeseries for each phytoplankton monoculture experiment, and two timeseries for each mixed phytoplankton experiment) was calculated by taking the value of the slope of a fitted linear model, which represents the average change in phytoplankton cell numbers per hour. Subsequently, a linear model for each treatment condition was fitted to the relevant slope values calculated from the timeseries, to examine how the average population growth can be predicted by the treatment variables (Supplementary Figure S1). The linear models underwent a two-way Analysis of Variance (ANOVA) test and a post-hoc Tukey test, to discern the significance of the various treatment conditions on the average population growth of each species. Q-Q plots were assessed to confirm the data met the premisses for ANOVA. All analysis was performed in R Studio (v.1.0.136) (R Core Team, 2018).
Results
There was both a significant interaction between the effect of plant type on the population growth of Chlorella (F = 4.0183, df = 2,54, p = 0.0236), and the effect of the state of the plant (F = 12.4120, df = 1,54, p = 0.0008). As illustrated in Figure 1, and demonstrated through a post-hoc Tukey test, in both co-culture and single phytoplankton conditions the average population growth for Chlorella under whole H. ranunculoides treatments was significantly lower when compared to the fake plant control (p = 0.0186). Additionally, whole H. ranunculoides had a significantly more negative effect on the average population growth of Chlorella than crushed H. ranunculoides (p = 0.0255). The crushed form of C. helmsii had a greater negative effect on the population growth of Chlorella than crushed H. ranunculoides, while whole C. helmsii had no effect on the population growth of Chlorella. Finally, crushed C. helmsii had a significantly negative effect on the population growth of Chlorella when compared to the control (p = 0.0183).
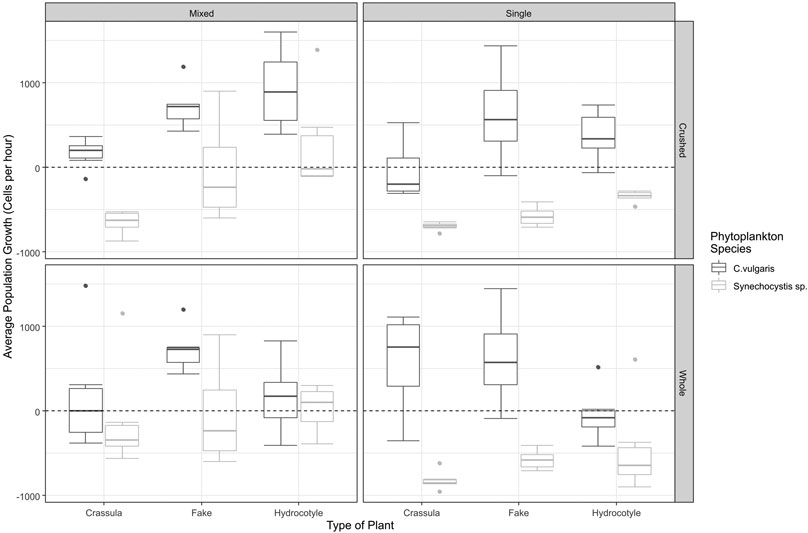
FIGURE 1. The average population growth (cells per hour) of phytoplankton under varying conditions. The boxplots indicate the average population growth of both Chlorella (Dark Grey) and Synechocystis (Light Grey) under each set of conditions. The dotted line across the centre of each graph represents an average population growth of 0. The mean value is represented by the dark central line, the upper and lower bounds of the box represent the 75% and 25% percentile respectively. The whiskers represent the highest and lowest value observed within 1.5 times the inner quartile range. The circles represent datapoints that lie beyond 1.5 times the inner quartile range. Mixed and Single refer to co-culture and monoculture phytoplankton conditions, respectively. Crushed and Whole refer to the state of the plant.
There was both a significant interaction between the effect of plant type on the average population growth of Synechocystis (F = 7.6837, df = 2,54, p = 0.0012), and the effect of the treatment type i.e. whether Synechocystis was grown in a co-culture or in a monoculture (F = 12.4120, df = 1,54, p < 0.0008). However, unlike Chlorella, H. ranunculoides had no significant effect on the population growth of Synechocystis. As illustrated in Figure 1, and demonstrated through a post-hoc Tukey test, the average population growth of Synechocystis was lower in treatments containing crushed C. helmsii when compared to treatments containing crushed H. ranunculoides (p = 0.0029), however the population growth did not differ significantly from the fake plant control. A large influence on the population growth of Synechocystis was the presence of Chlorella, the average population growth of Synechocystis was significantly elevated across all treatments when grown in a co-culture (p < 0.0001).
Discussion
The results present a mixed picture; whole H. ranunculoides supressed only the growth rate of C. vulgaris, while crushed H. ranunculoides had no effect on either phytoplankton species. Conversely, whole C. helmsii had no effect on either phytoplankton species, whereas crushed C. helmsii supressed the growth rate of both phytoplankton species. Additionally, the presence of Chlorella in co-culture experiments had a universally positive effect on Synechocystis population growth (Figure 2).
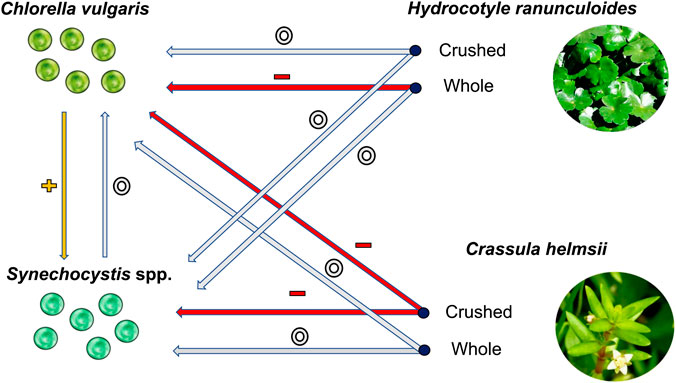
FIGURE 2. A simplified diagram of the interactions between the different plant species, plant states and phytoplankton species. The arrows indicate interactions between the plants and phytoplankton. All interactions relate to effects on the average population growth for the indicated phytoplankton species. The red arrows accompanied by the (−) symbol indicate negative interactions; the grey arrows accompanied by the (o) symbol indicate neutral interactions; and the yellow arrows accompanied by the (+) symbol indicate positive interactions. The bold text refers to the species names, whereas the bullet points indicate the state of the plant species.
The Effect of Plant Species on Phytoplankton Population Growth
The first hypothesis proposed by our study was that plants, regardless of their state, will have a negative effect on the average population growth of each phytoplankton species. This hypothesis can be rejected based on the results of our study. Both H. ranunculoides and C. helmsii were identified by Grutters et al. (2017) as meeting the criteria for high allelopathic potential, based upon their inhibition of the growth of the cyanobacterium Dolichospermum flos-aquae following methanol extraction. In our study, however, the allelopathic effect of both plant species varied in their effects. With H. ranunculoides only affecting the average growth of Chlorella negatively when in its whole state and having no effect on Synechocystis average growth. While C. helmsii had a negative effect on the average growth of both Cholrella and Synechocystis when in a crushed state. This highlights how species-specific effects might be important in determining the effect of allelopathic agents on different algal communities (Jasser, 1995; Körner and Nicklisch, 2002; Gross et al., 2003; Mulderij et al., 2005). In addition, whereas most studies show that the allelopathic effects of aquatic plants are inhibitory, there have been studies which revealed that certain macrophytes are ineffective or even stimulatory to certain phytoplankton (Hilt and Gross, 2008). For example, Van Aller et al. (1985) observed that Eleocharis microcarpa could inhibit the growth of Anabaena flos-aquae. Whereas Oscillatoria tenuis, had a stimulatory effect on Euglena gracilis (Van Aller et al., 1985). While Ceratophyllum demersum has been shown to stimulate the growth of the green algae Chlorella and Scenedesmus (Kogan, 1972), it inhibits the growth of the cyanobacteria Anabaena (Van Vierssen and Prins, 1985).
The Effect of Plant State on Phytoplankton Population Growth
The second hypothesis posed by our study was that crushed plants would have a greater effect on the average population growth of phytoplankton than whole, intact, plants. This hypothesis can also be rejected based on the results of our study. The design of our study allowed us to test whether the allelopathic potential of the plants persisted when the plant was in a whole or crushed state. The maintenance of saturating levels of nutrients was implemented to offset any positive effects on phytoplankton growth that may be brought about by nutrient release from crushed plants. In the experiment, the dialysis tubing acted to prevent the movement of bacteria and cell debris from coming into contact with the phytoplankton, while allowing potential allelochemicals to diffuse through the dialysis membrane. Therefore, if the plant contained water soluble allelopathic substances, the allelochemical will have been able to cross the membrane. It was hypothesised that the crushed plant experiments should contain a higher available concentration of the allelopathic substances due to the mechanical disruption of the plant cell walls and membranes.
The results of our study were mixed. The average population growth of Synechocystis was only affected by C. helmsii in its crushed form, and unaffected by H. ranunculoides, whereas the average population growth of Chlorella was only affected by the whole form of H. ranunculoides and the crushed form of C. helmsii.
There are several potential explanations for the differing effects between the states of the plants on phytoplankton population growth. Firstly, it has been shown that certain environmental factors may influence the production and release of allelopathic compounds. For example, phosphorus and nitrogen limitation has been found to prompt the production and release of allelochemicals in certain macrophytes, such as Myriophyllum spicatum (Gross et al., 2003). The maintenance of a state of nutrient saturation employed in this experiment, while eliminating nutrient competition as a potential confounding factor, is likely to have prevented the plants from experiencing nutrient limitation and therefore may have resulted in the plants limiting the production of allelopathic compounds. Light has also been shown to affect allelochemical production (Cronin and Lodge, 2003), with Sun exposed shoots of M. spicatum and Myriophyllum verticilliatum, containing higher levels of phenolic compounds than shade adapted plants (Choi et al., 2002). The light levels used in this experiment may have encouraged the whole plants to produce and release allelochemicals, whereas the crushed plants would have not been able to produce further allelochemicals.
Bacteria have also been shown to affect the efficacy of allelochemicals. Müller et al., 2007 demonstrated that certain epiphytic bacteria isolated from M. spicatum displayed polyphenol-degrading activity which prevented its ability to inhibit cyanobacterial growth. While, in contrast, it has also been shown that photolytically and microbially degraded tannic acid from M. verticilliatum had an increased allelopathic effect, indicating that in some cases the degraded by-products of allelochemicals could be more harmful than the allelochemical itself (Bauer et al., 2012). In addition to these local effects, the season has also been shown to influence the allelopathic activity of certain macrophytes (Hilt et al., 2006). Therefore, environmental factors such as the light regime and seasonality of the plant material used in this experiment may have implications for the observed allelopathic effects. The potential impact of environmental factors may further indicate that experiments focused on isolated plant extracts and exudates to identify potentially allelopathic compounds, may overlook many important interactions which play a role in defining the allelopathic relationship between aquatic plants and phytoplankton in natural communities.
The Effect of Co-culture on Cyanobacteria
The final objective of this study was to evaluate whether the allelopathic effect altered when the phytoplankton were grown in monoculture or co-culture. In this experiment co-culture had no significant effect on the average population growth of Chlorella, however it did have a significantly positive effect on the average population growth of Synechocystis. In all experiments, regardless of the plant type or state, Synechocystis had an elevated average population growth when grown in co-culture with Chlorella. This result may imply a commensal relationship, where Synechocystis receives a benefit from the presence of Chlorella without affecting Chlorella’s growth, however, further work would be required to confirm this. There is a significant amount of information in the literature detailing symbiosis between algae and bacteria, as many micro-algae are reliant on exogenous supplies of vitamins to grow, such as cobalamin, thiamine and biotin (Kazamia et al., 2012; Xie et al., 2013; Grant et al., 2014). However, there is a lack of literature detailing symbioses between green algae and cyanobacteria. The results of this study align with the findings of Chang et al. (2012), which suggests this area may warrant further investigation, if cyanobacteria can derive a benefit from the presence of green algae there may be fundamental implications for the understanding of phytoplankton community and ecosystem dynamics.
Implications for Allelopathy in the Ecological Engineering of Phytoplankton Communities in Freshwater Systems
This study demonstrates that there may be opportunities to harness aquatic plants to manipulate algal communities towards a desired end point. Although much of the literature indicates that the allelopathic effects of aquatic plants on phytoplankton are of an equally inhibitory nature, this study is one of many emerging studies which indicate that some plants can simultaneously elicit no significant response from one species of phytoplankton, while inhibiting the growth of another (Mulderij et al., 2007; Mohamed and Al Shehri, 2010; Wang et al., 2013). Therefore, the harnessing of the allelopathic capacity of certain aquatic plants could not be used generically. To be truly effective, the plants used should be carefully selected based on their ability to supress the specific species of harmful algae present in the target waterbody. For example, this study would suggest that the presence of H. ranunculoides, may act to supress Chlorella, while Synechocystis is unaffected. Furthermore, the results of this study suggest that allelochemicals may be used to affect desirable outcomes through indirect pathways. For example, using allelopathy to supress Chlorella could have the benefit of reducing growth in harmful cyanobacteria such as Synechocystis which appears to benefit from the presence of Chlorella. This study may have implications for the management of invasive species in areas where cyanobacterial blooms are also of significant risk. For example, it is possible that breaking down C. helmsii either through mechanical disruption or grazing pressure may help to supress the growth of cyanobacteria.
Of course, as an invasive species, the risks and benefits of introducing or encouraging the proliferation of invasive aquatic plants, in either new or established systems, need to be carefully assessed on a site-by-site basis for any proposed application of allelopathy to be used responsibly and successfully. The risk profile varies vastly when comparing the use of invasive plants in a closed system within an invaded range, to an open system with links to surrounding waterways, in an uninvaded range. We would never recommend introducing invasive species into open water systems in an uninvaded range, however, there may be opportunities to leverage allelopathic effects within already invaded systems or closed systems within an invaded range. Any decision to encourage invasive plants should only be taken following discussion and appraisal with the relevant authorities at the regional, national or international level, depending on the perceived risks (McLaughlan and Aldridge, 2013).
Although inferring the ecological relevance of allelopathic effects observed in mesocosm experiments can be difficult, the methods employed in this study, through the use of dialysis tubing, allows for testing with the highest degree of realism (Gross et al., 2007). While mesocosms allow for control of nutrient competition and light availability, which aids the identification of allelopathic effects, in some natural systems these factors may overshadow the impact of a plant’s allelopathic properties (Hilt and Gross, 2008). To build on the findings of this study, further research should explore how allelopathic interactions may change across a range of plant biomass to phytoplankton ratios, in order to identify at which plant densities allelopathic impacts may be greatest or become insignificant. It is clear, however, that allelopathy offers considerable potential as part of a wider biomanipulation approach. Research into biological controls for manipulating and managing toxic or nuisance algae is of increasing importance, as biological solutions require minimal management and are free of the cost and supply chain issues associated with chemical or mechanical solutions.
Data Availability Statement
The raw data supporting the conclusions of this article will be made available by the authors, without undue reservation.
Author Contributions
SR and DA established the hypotheses and experimental design. SR performed the measurements, analysed the data and wrote the manuscript. DA supervised the research and reviewed the manuscript.
Funding
SR was supported by the Biotechnology and Biological Sciences Research Council and DA was supported by a Dawson Lectureship at St. Catharine’s College, Cambridge.
Conflict of Interest
The authors declare that the research was conducted in the absence of any commercial or financial relationships that could be construed as a potential conflict of interest.
Acknowledgments
This study was supported by a studentship to SR from the Biotechnology and Biological Sciences Research Council. DA was supported by a Dawson Lectureship from St. Catharine’s College, Cambridge. We would like to thank Andrew Tanentzap for supplying the fluoroprobe and Alison Smith for providing laboratory space and equipment.
Supplementary Material
The Supplementary Material for this article can be found online at: https://www.frontiersin.org/articles/10.3389/fenvs.2020.551803/full#supplementary-material.
SUPPLEMENTARY FIGURE S1. The change in the number of phytoplankton cells over time under varying conditions. All figures are linear regression models indicating the average change in the number of cells between the different treatments. The shaded grey area represents the 95% confidence interval. The dotted line across the bottom of each graph represents 0 cells per ml. The solid red line represents Chlorella cell numbers, and the dashed blue line represents Synechocystis cell numbers. The red and blue circles represent the average Chlorella and Synechocystis cell numbers at each time point respectively, the error bars indicate the standard error. The underlined red numbers indicate the intercept, coefficient, R2 value and P value of the regression models relating to Chlorella. The blue, non-underlined numbers, represent the same values for the regression models relating to Synechocystis. Figures (a), (b) and (c), represent the results for each plant type, H. ranunculoides, C. helmsii and Fake plant respectively. The vertical facets (Chlorella, Mixed and Synechocystis) refer to monoculture treatments for each species, and the co-culture treatments. The horizontal facet (Crushed, Whole and Fake) refers to the state of the plant.
References
Azevedo, S. M., Carmichael, W. W., Jochimsen, E. M., Rinehart, K. L., Lau, S., Shaw, G. R., et al. (2002). Human intoxication by microcystins during renal dialysis treatment in Caruaru-Brazil. Toxicology 181–182, 441–446. doi:10.1016/s0300-483x(02)00491-2
Backer, L., Deana, B., Rebecca, L., and Birgit, B. (2015). Cyanobacteria and algae blooms: review of health and environmental data from the harmful algal bloom-related illness surveillance system (HABISS) 2007–2011’. Toxins 7, 1048–1064. doi:10.3390/toxins7041048
Bauer, N., Zwirnmann, E., Grossart, H. P., and Hilt, S. (2012). Transformation and allelopathy OF natural dissolved organic carbon and tannic acid are affected BY solar radiation and BACTERIA(1). J. Phycol. 48, 355–364. doi:10.1111/j.1529-8817.2012.01134.x
bbe Moldaenke GmbH (2013). bbe++ software version 2.4.2. Schwentinental, Germany: bbe Moldaenke GmbH.
Capuzzo, E., Stephens, D., Silva, T., Barry, J., and Forster, R. M. (2015). Decrease in water clarity of the southern and central north sea during the 20th centuryglobal change biology. Glob. Chang. Biol. 21, 2206–2214. doi:10.1111/gcb.12854
Catherine, A., Escoffier, N., Belhocine, A., Nasri, A. B., Hamlaoui, S., Yéprémian, C., et al. (2012). On the use of the FluoroProbe®, a phytoplankton quantification method based on fluorescence excitation spectra for large-scale surveys of lakes and reservoirs. Water Res. 46 (6), 1771–1784. doi:10.1016/j.watres.2011.12.056
Chang, X., Eigemann, F., and Hilt, S. (2012). Do macrophytes support harmful cyanobacteria? Interactions with a green alga reverse the inhibiting effects of macrophyte allelochemicals on Microcystis aeruginosa. Harmful Algae 19, 76–84. doi:10.1016/j.hal.2012.06.002
Chen, E. S., and Bridgeman, T. B. (2017). The reduction of Chlorella vulgaris concentrations through UV-C radiation treatments: a nature-based solution (NBS). Environ. Res. 156, 183–189. doi:10.1016/j.envres.2017.03.007
Choi, C., Bareiss, C., Walenciak, O., and Gross, E. M. (2002). Impact of polyphenols on growth of the aquatic herbivore acentria ephemerella. J. Chem. Ecol. 28, 2245–2256. doi:10.1023/A:1021049332410
Chorus, J., and Bartrum, I. (1999). Toxic Cyanobacteria in water: a guide to their public health consequences, monitoring and management. New York, NY E & FN Spon.
Cronin, G., and Lodge, D. M. (2003). Effects of light and nutrient availability on the growth, allocation, carbon/nitrogen balance, phenolic chemistry, and resistance to herbivory of two freshwater macrophytes. Oecologia 137, 32–41. doi:10.1007/s00442-003-1315-31
Crooks, J. A. (2002). Characterizing ecosystem-level consequences of biological invasions: the role of ecosystem engineers. Hoboken, NJ: John Wiley & Sons.
Dervaux, J., Mejean, A., and Brunet, P. (2015). Irreversible collective migration of cyanobacteria in eutrophic conditions. PloS One 10 (3), e0120906. doi:10.1371/journal.pone.0120906
Grant, M. A., Kazamia, E., Cicuta, P., and Smith, A. G. (2014). Direct exchange of vitamin B12 is demonstrated by modelling the growth dynamics of algal-bacterial cocultures. ISME J. 8 (7), 1418–1427. doi:10.1038/ismej.2014.9
Gross, E. M., Erhard, D., and Iványi, E. (2003). Allelopathic activity of Ceratophyllum demersum L. and Najas marina ssp. intermedia (Wolfgang) Casper’. Hydrobiologia 506 (1–3), 583–589. doi:10.1023/B:HYDR.0000008539.32622.91
Gross, E. M., Sabine, H., Paola, L., and Gabi, M. (2007). Searching for allelopathic effects of submerged macrophytes on phytoplankton - state of the art and open questions. Hydrobiologia 584 (1), 77–88. doi:10.1007/s10750-007-0591-z
Gross, E. M., and Jüttner, F. (2003). Allelopathy of aquatic autotrophs. Crit. Rev. Plant Sci. 22, 313–339. doi:10.1080/713610859org/10.1080/713610859
Grutters, B. M. C., Benedetta, S., Elisabeth, M. G., Dedmer, B., and Van de Waal Elisabeth, B. (2017). Growth strategy, phylogeny and stoichiometry determine the allelopathic potential of native and non-native plants. Oikos 126, 1770–1779. doi:10.1111/oik.03956
Harke, M. J., Steffen, M. M., Gober, C. J., Otten, T. G., Wilhelm, S. W., Wood, S. A., et al. (2016). A review of the global ecology, genomics, and biogeography of the toxic cyanobacterium, Microcystis spp. Harmful Algae 54, 4–20. doi:10.1016/J.HAL.2015.12.007
Hartmann, A., Horn, H., Röske, I., and Röske, K. (2019). Comparison of fluorometric and microscopical quantification of phytoplankton in a drinking water reservoir by a one-season monitoring programAquatic Sciences. Aquatic Sciences 81, 1–16. doi:10.1007/s00027-018-0608-x
Hastings, A., Byers, J., Crooks, J., Cuddington, K., Jones, C., Lambrinos, J., et al. (2006). ‘Ecosystem engineering in space and time’. Ecology Letters 10, 153–164. doi:10.1111/j.1461-0248.2006.00997.x
Hilt, S., Ghobrial, M. G. N., and Gross, E. M. (2006). In situ allelopathic potential of Myriophyllum verticillatum (Haloragaceae) against selected phytoplankton species. J. Phycol. 42 (6), 1189–1198. doi:10.1111/j.1529-8817.2006.00286.x
Hilt, S., and Gross, E. M. (2008). Can allelopathically active submerged macrophytes stabilise clear-water states in shallow lakes?’. Urban & Fischer 9 (4), 422–432. doi:10.1016/J.BAAE.2007.04.003
Hu, H., and Hong, Y. (2008). Algal-bloom control by allelopathy of aquatic macrophytes - a review. Front. Environ. Sci. Eng. China 2 (4), 421–438. doi:10.1007/s11783-008-0070-4
Huisman, J., Geoffrey, A., Hans, W., Bas, W., Jolanda, M. H., and Verspagen, J. (2018). ‘Cyanobacterial blooms’. Berlin, Germany: Nature Publishing Group, 471–483.
Jasser, I. (1995). ‘The influence of macrophytes on a phytoplankton community in experimental conditions’, Hydrobiologia 306, 21–32. doi:10.1007/BF00007855
Jeppesen, E., Søndergaard, M., and Liu, Z. (2017). Lake restoration and management in a climate change perspective. Water 9, 122. doi:10.3390/w9020122
Kazamia, E., Czesnick, H., Nguyen, T. T., Croft, M. T., Sherwood, E., Sasso, S., et al. (2012). Mutualistic interactions between vitamin B12 -dependent algae and heterotrophic bacteria exhibit regulation. Environ. Microbiol. 14 (6), 1466–1476. doi:10.1111/j.1462-2920.2012.02733.x
Kogan, S. C. G. (1972). Relations between Ceratophyllum demersum (L.) and some blue-green algae. Hydrobiol. J. 5, 14–19.
Körner, S., and Nicklisch, A. (2002). Allelopathic growth inhibition of selected phytoplankton species by submerged macrophytes. J. Phycol. 38, 862–871. doi:10.1046/j.1529-8817.2002.t01-1-02001.x
Lam, A. K.-Y., Prepas, E., Spink, D., and Hrudey, S. (1995). ‘Chemical control of hepatotoxic phytoplankton blooms: implications for human health’. Water Research 29 (8), 1845–1854. doi:10.1016/0043-1354(94)00348-B
Lund, B. (1965). The ecology of the freshwater phytoplankton. Algae 40, 231–290. doi:10.1111/j.1469-185X.1965.tb00803.x
Magdaleno, A., Vélez, C. G., Wenzel, M. T., and Tell, G. (2014). Bulletin of environmental contamination and toxicology. Bull. Environ. Contam. Toxicol. 92, 202–207. doi:10.1007/s00128-013-1171-8
Martins, R., Pereira, P., Welker, M., Fastner, J., and Vasconcelos, V. M. (2005). Toxicity of culturable cyanobacteria strains isolated from the Portuguese coast. Toxicon 46 (4), 454–464. doi:10.1016/J.TOXICON.2005.06.010
McLaughlan, C., and Aldridge, D. C. (2013). Cultivation of zebra mussels (Dreissena polymorpha) within their invaded range to improve water quality in reservoirs. Water Res. 47, 4357–4369. doi:10.1016/j.watres.2013.04.043
Meiners, S. J. (2014). Functional correlates of allelopathic potential in a successional plant community. Plant Ecol. 215 (6), 661–672. doi:10.1007/s11258-014-0331-1
Michalak, A. M., Anderson, E. J., Beletsky, D., Boland, S., Bosch, N. S., Bridgeman, T. B., et al. (2013). Record-setting algal bloom in Lake Erie caused by agricultural and meteorological trends consistent with expected future conditions. Proc. Natl. Acad. Sci. U.S.A. 110 (16), 6448–6452. doi:10.1073/pnas.1216006110
Mitra, A., and Flynn, K. J. (2006). Promotion of harmful algal blooms by zooplankton predatory activity, Biol. Lett., 2, 194–197. doi:10.1098/rsbl.2006.0447
Mohamed, Z. A., and Al Shehri, A. M. (2010). Differential responses of epiphytic and planktonic toxic cyanobacteria to allelopathic substances of the submerged macrophyte stratiotes aloides. Int. Rev. Hydrobiol. 95, 224–234. doi:10.1002/iroh.200911219
Moss, B., Ellen, D., and Elisabeth, M. (2011). Allied attack: climate change and eutrophication. Inland Waters 1, 101–105. doi:10.5268/IW-1.2.359
Mulderij, G., Ellen, D., and Elisabeth, M. (2007). ‘Allelopathic activity of Stratiotes aloides on phytoplankton—towards identification of allelopathic substances’, Hydrobiologia 584, 89–100. doi:10.1007/s10750-007-0602-0
Mulderij, G., Mooij, W. M., and Donk, E. V. (2005). ‘Allelopathic growth inhibition and colony formation of the green alga Scenedesmus obliquus by the aquatic macrophyte Stratiotes aloides’, Aquat. Ecol. 39, 11–21. doi:10.1007/s10452-004-1021-1
Müller, N., Hempel, M., Philipp, B., and Gross, E. M. (2007). Degradation of gallic acid and hydrolysable polyphenols is constitutively activated in the freshwater plant-associated bacterium Matsuebacter sp. FB25. Aquat. Microb. Ecol. 47 (1), 83–90. doi:10.3354/ame047083
Murray, D., Jefferson, B., Jarvis, P., and Parsons, S. A. (2010). Inhibition of three algae species using chemicals released from barley straw. Environ. Technol. 31 (4), 455–466. doi:10.1080/09593331003663294
Nagai, T., Taya, K., and Yoda, I. (2016). Comparative toxicity of 20 herbicides to 5 periphytic algae and the relationship with mode of action. Environ. Toxicol. Chem. 35, 368–375. doi:10.1002/etc.3150
Nakai, S., Zou, G., Okuda, T., Nishijima, W., Hosomi, M., and Okada, M. (2012). Polyphenols and fatty acids responsible for anti-cyanobacterial allelopathic effects of submerged macrophyte Myriophyllum spicatum. Water Sci. Technol. 66 (5), 993–999. doi:10.2166/wst.2012.272
Nasri, H., El Herry, S., and Bouaïcha, N. (2008). First reported case of turtle deaths during a toxic Microcystis spp. bloom in Lake Oubeira, Algeria, Ecotoxicol. Environ. Saf. 71, 535–544. doi:10.1016/J.ECOENV.2007.12.009
Oudra, B., El Andaloussi, M., Franca, S., Barros, P., Martins, R., Oufdou, R., et al. (2000). Harmful cyanobacterial toxic blooms in waste stabilization ponds. Water Sci. Technol. 42 (10–11), 179–186. doi:10.2166/wst.2000.0637
Paerl, H. W., Fulton, R. S., Moisander, P. H., and Dyble, J. (2001). Harmful freshwater algal blooms, with an emphasis on cyanobacteria. Sci. World J. 1, 76–113. doi:10.1100/tsw.2001.16
Paerl, H. W., and Otten, T. G. (2013). Harmful cyanobacterial blooms: causes, consequences, and controls. Microb. Ecol. 65 (4), 995–1010. doi:10.1007/s00248-012-0159-y
Park, J., Church, J., Son, Y., Tae Kim, K., and Hyoung, W. (2017). Recent advances in ultrasonic treatment: challenges and field applications for controlling harmful algal blooms (HABs). Ultrason. Sonochem., 38, 326–334. doi:10.1016/j.ultsonch.2017.03.003
Planas, D., Sarhan, F., Dube, L., and Godmaire, H. (1981). Ecological significance of phenolic compounds of Myriophyllum spicatum. SIL Proceedings 21, 1492–1496. doi:10.1080/03680770.1980.11897219
Pohl, J., Saltsman, I., Mahammed, A., Gross, Z., and Röder, B. (2015). Inhibition of green algae growth by corrole-based photosensitizers. J. Appl. Microbiol. 118, 305–312. doi:10.1111/jam.12690
Priyadarshani, I., and Rath, B. (2012). Commercial and industrial applications of micro algae – a review’. J. Algal Biomass 3 (4), 89–100.
Qu, L., Wang, R., Zhao, P., Chen, R., Zhou, W., Tan, L., et al. (2014). Interaction between Chlorella vulgaris and bacteria: interference and resource competitionActa oceanologica sinica. Acta Oceanolog Sin 33, 135–140. doi:10.1007/s13131-014-0432-7
R Core Team, (2018). R: a language and environment for statistical computing. in Software version 1.1.442.(Vienna, Austria: R Foundation for Statistical Computing)
Reynolds, C. S., and Walsby, A. E. (1975). ‘Water-Blooms’. Biolog. Rev. 50 (4), 437–481. doi:10.1111/j.1469-185X.1975.tb01060.x
Scheffer, M., Hosper, S. H., Meijer, M. L., Moss, B., and Jeppesen, E. (1993). Alternative equilibria in shallow lakes. Trends Ecol. Evol. 8 (8), 275–279. doi:10.1016/0169-5347(93)90254-M
Schubert, H., Hans, C. P., Matthijs, L., Murb, R., Schiewera, U., et al. (1995). Blooming of cyanobacteria in turbulent water with steep light gradients: the effect of intermittent light and dark periods on the oxygen evolution capacity of Synechocystis sp. PCC 6803. FEMS Microbiol. Ecol. 18 (3), 237–245. doi:10.1016/0168-6496(95)00063-8
Sun, R., Sun, P., Zhang, J., Esquivel-Elizondo, S., and Wu, Y. (2018). Microorganisms-based methods for harmful algal blooms control: a review. Bioresour Technol. 18, 12–20. doi:10.1016/j.biortech.2017.07.175
Van Aller, R. T., Pessoney, G. F., Rogers, V. A., Watkins, E. J., and Leggett, H. G. (1985). Oxygenated fatty acids: a class of allelochemicals from aquatic plants. American Chem. Soc. 387–400. doi:10.1021/bk-1985-0268.ch026
van Donk, E., and van de Bund, W. J. (2002). Impact of submerged macrophytes including charophytes on phyto- and zooplankton communities: allelopathy versus other mechanisms. Aquat. Bot. 72, 261–274. doi:10.1016/S0304-3770(01)00205-4
Van Vierssen, W., and Prins, T. C. (1985). On the relationship between the growth of algae and aquatic macrophytes in brackish water. Aquat. Bot. 21, 165–179. doi:10.1016/0304-3770(85)90087-7
Vanderstukken, M., Steven, A. J., Declerck, D., and Koenraad, M. (2014). Long-term allelopathic control of phytoplankton by the submerged macrophyte Elodea nuttallii. Freshw. Biol. 59 (5), 930–941. doi:10.1111/fwb.12316
Wang, J., Zhu, J. Y., Gao, Y., and Liu, B. (2013). Toxicity of allelochemicals released by submerged macrophytes on phytoplankton. Allelopathy J. 31 (1), 199–210.
Xie, B., Bishop, S., Stessman, D., Wright, D., Spalding, M. H., and Halverson, L. J. (2013). Chlamydomonas reinhardtii thermal tolerance enhancement mediated by a mutualistic interaction with vitamin B12-producing bacteria. ISME J. 7 (8), 1544–1555. doi:10.1038/ismej.2013.43
Keywords: invasive aquatic plants, allelopathy, harmful algal bloom, cyanobacteria, green algae, eutrophication
Citation: Reynolds SA and Aldridge DC (2021) Embracing the Allelopathic Potential of Invasive Aquatic Plants to Manipulate Freshwater Ecosystems. Front. Environ. Sci. 8:551803. doi: 10.3389/fenvs.2020.551803
Received: 09 October 2020; Accepted: 29 December 2020;
Published: 15 February 2021.
Edited by:
Vinicius Fortes Farjalla, Federal University of Rio de Janeiro, BrazilReviewed by:
Paulo Sergio Salomon, Federal University of Rio de Janeiro, BrazilXiufeng Zhang, Jinan University, China
Copyright © 2021 Reynolds and Aldridge. This is an open-access article distributed under the terms of the Creative Commons Attribution License (CC BY). The use, distribution or reproduction in other forums is permitted, provided the original author(s) and the copyright owner(s) are credited and that the original publication in this journal is cited, in accordance with accepted academic practice. No use, distribution or reproduction is permitted which does not comply with these terms.
*Correspondence: Sam A. Reynolds, c2FyODdAY2FtLmFjLnVr