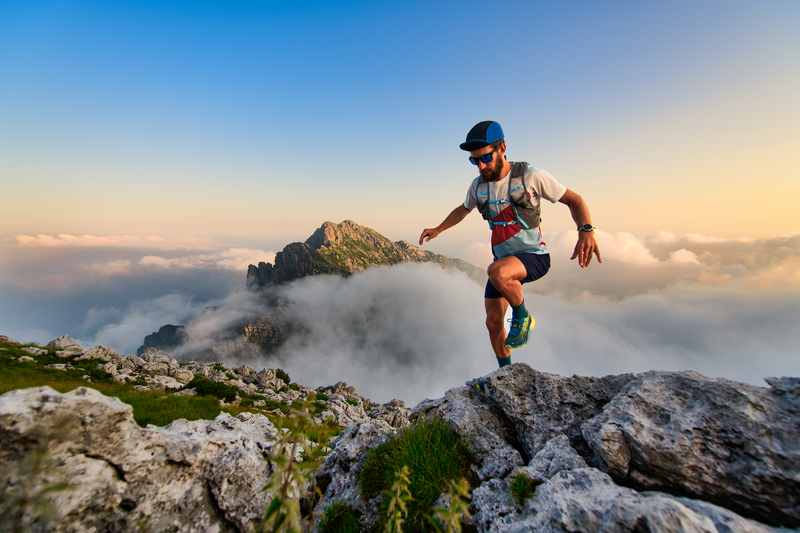
95% of researchers rate our articles as excellent or good
Learn more about the work of our research integrity team to safeguard the quality of each article we publish.
Find out more
ORIGINAL RESEARCH article
Front. Environ. Sci. , 09 October 2020
Sec. Freshwater Science
Volume 8 - 2020 | https://doi.org/10.3389/fenvs.2020.540607
This article is part of the Research Topic Climate Change and Light in Aquatic Ecosystems: Variability & Ecological Consequences View all 10 articles
Cyanobacterial harmful algal blooms are one of the most prominent threats to water quality in freshwater ecosystems and are expected to become more common as the climate continues to change. While traditional strategies to manage algal blooms have focused on controlling nutrients, manipulating light as a way to reduce cyanobacteria is less frequently explored. Here, we propose the addition of glacial rock flour (GRF), a fine particulate that floats on the water’s surface and remains suspended in the water column, to reduce light availability and in turn, phytoplankton biomass dominated by cyanobacteria. To determine if a sustained reduction in light could lower cyanobacteria biomass and microcystin concentrations, we applied GRF to large-scale (11 kL) mesocosm tanks for 9 consecutive days. Mesocosm tanks were amended by adding nitrogen and phosphorus to generate chlorophyte- and cyanophyte- dominated experimental tanks. To assess how the phytoplankton community was impacted in each tank, we measured photosynthetic irradiance parameters, the maximum quantum yield of photosystem II, gross primary productivity (GPP), phytoplankton biovolume, and phytoplankton community composition before and after the addition of GRF. GRF effectively reduced cyanophyte biovolume by 78% in the cyanophyte-dominated tanks, despite no significant change in total phytoplankton community biovolume. Cyanophytes were replaced by cryptophytes, which increased by 106% in the chlorophyte-dominated tanks and by 240% in the cyanophyte-dominated tanks. The change in photosynthetic irradiance parameters and GPP after the addition of GRF was not significantly different between any of the treatment or control groups, suggesting that either the cyanophytes will likely recover if light availability increases, or that the new cryptophyte-dominated community was well suited to a reduced light environment. Cyanobacterial blooms are expected to increase in frequency and magnitude as climate change progresses, but our study suggests that light manipulation may be a useful in-lake management strategy for controlling these blooms and warrants further investigation.
Cyanobacterial harmful algal blooms often occur in lakes and reservoirs with high nutrient concentrations (Heisler et al., 2008). These blooms are increasing in frequency and magnitude across the globe and are a threat to aquatic resources (Brooks et al., 2016). Cyanobacteria are of poor nutritional food quality compared to other phytoplankton taxa for zooplankton grazers, which rely on foods high in polyunsaturated fatty acids, and can cause inefficiencies in trophic transfers (Brett et al., 2009; Grosbois et al., 2017). Some cyanobacteria produce secondary metabolites, the most common of which is the cyanotoxin microcystin, that are toxic to animals and have been identified as potentially carcinogenic to humans (Grosse et al., 2006). Cyanotoxins have caused livestock (Van Halderen et al., 1995), pet (Backer et al., 2013), and wildlife (Miller et al., 2010) mortality and in extreme instances can result in human fatalities (Carmichael et al., 2001). Given the human and animal health hazard posed by cyanobacterial blooms, water body advisories and closures are common during bloom events, which can strain local economies during cyanobacterial outbreaks (Dodds et al., 2009).
Light is a critical resource for all phytoplankton. Phytoplankton use stored sugars and starches or rely on mixotrophy to temporarily survive in the absence of light (Lee, 2008), but prolonged light limitation can result in cell death or cause the cell to enter a resting phase (Bellinger and Sigee, 2010). Light requirements vary among individual taxa, and many cyanobacteria can be superior competitors at both low and high light intensities (Yang and Jin, 2008). Cyanobacteria are tolerant of reduced light conditions because of low maintenance energy requirements and their ability to maintain higher growth rates at lower light levels than many other phytoplankton (Van Liere and Mur, 1980). This tolerance allows them to persist deeper in the water column underneath other phytoplankton groups until conditions become favorable (Chorus and Bartram, 1999). Light also influences the ability of buoyancy regulating cyanobacteria to position themselves at a favorable depth in the water column. Low light levels induce gas vacuole production, giving the cell positive buoyancy (Deacon and Walsby, 1990), while high light intensities increase photosynthetic rates, allowing cells to store dense carbohydrate ballasts (Wallace and Hamilton, 2000). Cyanobacteria are also superior competitors under high light conditions due to their ability to withstand high levels of UV radiation (Paerl et al., 1983; Sinha and Häder, 2008). The ability to withstand variable light intensities may be allowing cyanobacteria to adapt to a changing climate more favorably compared to eukaryotic algae.
Cyanobacteria are anticipated to benefit from climate-induced environmental changes. Warmer water temperatures will favor cyanobacteria, many of which have peak growth rates at temperatures between 25 and 34°C (Robarts and Zohary, 1987; but see Lürling et al., 2013). Warming waters will result in earlier and stronger thermal stratification, which will benefit buoyancy regulating taxa (Paerl and Huisman, 2009). Projected climate change scenarios indicate that some states will experience more severe droughts while others will have higher rates of precipitation (U.S. Environmental Protection Agency, 2016). Extended droughts can increase the salinity of surface waters, which may favor cyanobacteria over other phytoplankton taxa (Paerl and Paul, 2012; Lehman et al., 2013). Higher rates of precipitation will result in increased nutrient runoff from the landscape, potentially leading to an increase in cyanobacterial blooms (Paerl and Paul, 2012). These impending climatic changes emphasize the need for a reliable method to mitigate cyanobacterial blooms.
A commonality among cyanobacterial management strategies is to reduce water column nutrient availability. Each management strategy has tradeoffs and no single strategy has been successful at controlling all types of cyanobacterial blooms (Ibelings et al., 2016). For example, beneficial management practices (BMPs) are often used to reduce external nutrient loading and subsequent cyanobacteria biomass (Sharpley et al., 2000). While BMPs can be effective, it can take several decades before noticeable improvements to water quality are observed (Osgood, 2017). Another strategy to control cyanobacterial blooms is to lower in-lake phosphorus (P) concentrations. Commercially available solid-phase P sorbents can reduce available P, and in turn cyanobacterial biomass, but these reductions are not permanent unless external nutrient loading is also reduced (Mackay et al., 2014). Where it is not always practical to reduce nutrient availability, other approaches could provide a more realistic way to reduce cyanobacterial blooms. One strategy is to negate the advantage that buoyancy regulation provides some taxa with approaches such as artificial mixing or lake flushing (Visser et al., 2016).
When nutrient reduction methods are not feasible, light reduction management strategies may help mitigate cyanobacterial blooms. To the best of our knowledge, the only management strategy designed to control algal growth by altering the light environment is the application of artificial dye products. These dyes come in a variety of colors, but all are designed to absorb incoming photosynthetically active radiation (PAR), thus reducing the amount available for aquatic photosynthesizers. These dyes are touted as environmentally friendly and published research shows they have no effect on fish, crayfish, nor tadpoles (Spencer, 1984; Bristow et al., 1996; Bartson et al., 2018), but can reduce zooplankton diversity (Suski et al., 2018). The main drawback to these dyes is that they are designed to control rooted macrophytes and have limited effectiveness in controlling cyanophyta, bacillariophyta, euglenophyta, or chlorophyta biomass (Ludwig et al., 2008). In this study, we designed an experiment to test the efficacy of an alternative way to reduce light availability through the addition of glacial rock flour (GRF).
Glacial rock flour is defined broadly as the fine particulate derived from glacial erosion and occurs naturally as the erosional silt- and clay-sized particles formed from a glacier passing over bedrock (Rampe et al., 2017). No standards exist to characterize it by size class or composition because GRF is composed of minerals reflecting the local geology in a lake catchment (Chanudet and Filella, 2009). As the glacier melts, either from seasonal receding (Casassa et al., 2009) or climate change (Moore et al., 2009), GRF runs off into the lake, often via a tributary. In glacial lakes, GRF attenuates 63% of the total water column PAR (Rose et al., 2014). Lakes that receive glacial meltwater have reduced primary productivity due to the decreased light availability, though mixotrophic phytoplankton are less sensitive to this change in light than those who rely solely on photosynthesis (Slemmons et al., 2013; Sommaruga and Kandolf, 2014).
We conducted an experiment where we reduced light through the addition of GRF with the objective of decreasing cyanophyta biomass and microcystin concentrations. Phytoplankton growth was stimulated in mesocosm tanks with amendments of P, nitrogen (N), or a combination of both to produce phytoplankton communities dominated by either chlorophytes or cyanophytes. The light environment in experimental tanks was manipulated through the addition of GRF. We hypothesize that cyanophyte biovolume and microcystin concentrations would be impacted by GRF additions, and that this change would be reflected in the physiology and primary productivity of the phytoplankton community. Our results have important implications for the future management of our changing water bodies.
This experiment was conducted at the University of Kansas field station in Lawrence, KS, United States (39.049674°N, 95.190777°W) in closed bottom, fiberglass mesocosm tanks. Each was filled to a volume of 11,000 L and depth of 1.25 m. To insulate from fluctuations in air temperatures, mesocosm tanks were kept in a ∼1,300 m2 shallow pond and were surrounded with ∼1 m of water (Figure 1). The insulation pond was surrounded by low vegetation, mainly grasses and wildflowers that were mowed periodically throughout the summer (Supplementary Figure 1). The closest trees were over 100 m away from the mesocosm tank set. Tanks were positioned within 50 cm of each other and were accessed via anchored walkways. On July 23, 2018, we filled each tank with 10,840 L of water from an on-site storage impoundment, then inoculated each tank with 160 L of surface water from a nearby reservoir (Milford Lake, KS, United States) with a well-documented history of cyanobacterial harmful algal blooms (Harris et al., 2020). For 8 consecutive weeks prior to the beginning of our experiment, 20 of the 23 mesocosm tanks received weekly N and P amendments. Treatments were designed to result in tanks with N- or P- deficient conditions for phytoplankton growth. Three tanks were maintained as ambient control tanks and received no nutrient nor GRF additions (Table 1). Each tank was randomly assigned a nutrient amendment, or in the case of the controls, no nutrient amendment.
Figure 1. Birds-eye view of mesocosm tank set-up. We added glacial rock flour (GRF) to 14 of the 17 mesocosm tanks used in this experiment. Phytoplankton communities were dominated by either chlorophytes or cyanophytes (n = 8) at the beginning of the experiment. Of the 9 chlorophyte dominated tanks, a subset (n = 3) did not receive any nutrient nor GRF amendments and were treated as a control (Con). The remaining chlorophyte dominated tanks (n = 6) received daily GRF additions. Tanks were kept in a ∼1300 m2 shallow pond that was ∼1 m deep to insulate for changes in air temperatures. The pond was surrounded by low vegetation. Mesocosm tanks were ∼3 m across, 1.25 m high, and had a volume of 11,000 L. Crossbeams were placed across each mesocosm. Sediment traps were suspended from each crossbeam.
A preliminary experiment was conducted on 6 of the 20 amended tanks to assess the quantity of GRF required to achieve the greatest reduction in light. We compared light conditions in tanks that received zero (n = 3), 5 (n = 2), 10 (n = 2), and 20 (n = 2) kg of GRF and determined that adding more than 5 kg of GRF did not result in any additional reduction in light based on no significant difference in PAR among tanks that received the 3 doses of GRF (Kruskal-Wallis p = 0.2551, df = 3, χ2 = 18.01). We chose 5 kg of GRF as the optimum quantity to add in our subsequent experiment (Supplementary Figure 2). The 6 tanks that received GRF during this preliminary experiment were then removed from the experiment described below.
The GRF used was commercially available from Vital Earth’s®. Its elemental composition was determined in triplicate by Activation Laboratories Ltd. (ActLabs, Ancaster, ON) via lithium metaborate/tetraborate fusion followed by aqueous phase analyses using inductively coupled plasma (ICP)–optical emission spectrometry (OES) and ICP–mass spectrometry (MS) for major and trace elements, respectively (Supplementary Table 1). We determined GRF sediment particle fractionation using a hydrometer (Gee and Bauder, 1979). Sediment particles were measured in the following size fractions: 0.5 – 2 mm (sand), 0.002 – 0.05 mm (silt), and < 0.002 mm (clay). GRF was 18.3% sand, 70.7% silt, and 11.2% clay. We did not explicitly test for any biota associated with the GRF.
Algal communities were created by weekly nutrient amendments by adding different forms of nutrients so that algal communities would vary (Table 1). We kept tanks that received the same nutrient forms together, even if a single tank in the group was not dominated by the same phytoplankton. We included 1 tank in the chlorophyte-dominated group where chlorophytes only comprised 9.2% of the total biovolume (Tank #3, Supplementary Table 2). Cryptophytes were the dominant taxa in this tank on day 0 (71.2%), but we decided to include it with the other tanks that received the same nutrient amendments because we do not believe excluding it would have changed our results. If this tank were excluded, cryptophyte biovolume would have increased in the 5 remaining chlorophyte-dominated tanks by 111.0% between day 0 and day 9, compared to an increase of 105.7% if the tank were included. In 1 of the cyanophyte-dominated tanks as well, cyanophytes were not the dominant taxa on day 0, comprising only 14.3% (Tank #11, Supplementary Table 2). We included this tank for the same reasons. When it was included, cryptophytes increased by 240.0% between day 0 and day 9. When this tank was excluded, they increased by 408.0%. Ultimately, we considered phytoplankton dominance to be the taxa with the highest biovolume, averaged across all tanks within the group. On day 0, 6 of the 17 tanks were included in the chlorophyte-dominated group (biovolume mean and range = 1.51, 0.06 – 3.11 mm3 L–1; % of total phytoplankton biovolume mean and range = 51.4, 9.2 – 89.4%) and 8 were included in the cyanophyte-dominated group (biovolume mean and range = 28.17, 0.98 – 71.87 mm3 L–1; % of total biovolume mean and range = 71.3, 14.3 – 92.0%). Oocystis was the most prevalent phytoplankton in chlorophyte-dominated tanks, comprising a mean 35.4% of total phytoplankton biovolume (range = 0 – 86.9%), while Aphanizomenon, which comprised a mean 22.8% total phytoplankton biovolume (range = 0 – 49.4%), was the most prevalent phytoplankton in cyanophyte-dominated tanks (Table 2 and Supplementary Table 2). Control tanks (n = 3) were also dominated by chlorophytes (biovolume mean and range = 3.17, 0.28 – 8.08 mm3 L–1; % of total biovolume mean and range = 52.3, 25.1 – 82.0%) when the experiment began, particularly from the genus Tetraedron, which comprised a mean 26.2% of total phytoplankton biovolume (range = 0 – 78.5%, Table 2 and Supplementary Table 2). We added GRF to each of the chlorophyte- and cyanophyte-dominated treatment tanks, exclusive of the 3 control tanks. For 9 consecutive mornings between 9:00–10:30 AM (CST), we distributed 5 kg of GRF evenly across the surface of each tank with a sifter. GRF was allowed to float on the tank surface, although some mixed into the water column over the next 24 h. PAR measurements were taken in the air above the water surface, just below the water’s surface, 0.5, and 1 m below the surface in each tank using a cosine Li-Cor underwater quantum sensor (LI-192). To account for changes in PAR from the time we measured the first tank to the time that we measured the last, we corrected each underwater PAR measurement by dividing it by the air PAR measurement we made above the water’s surface. We report this value as the PAR ratio (0.5 m water/air reading). To ensure that a reduction in light was maintained throughout the entire day, light was measured one hour after GRF addition on all experimental days, 4 h after GRF addition on days 0, 5, 6, and 7, and 6 h after GRF addition on day 4. PAR measurements from just below the surface, 0.5, and 1 m below the surface, were used to determine the light attenuation coefficient (Kd) in all tanks from the natural logarithm of irradiance versus depth (Kirk, 1994). Temperature profiles were collected from just below the surface, 0.5, and 1 m below the surface using a Yellow Springs Instrument EXO3, which is accurate to ± 0.01°C.
We collected water samples from each tank on days 0 and 9 with a PVC integrated sampler with a check valve from the surface to a depth of 1.0 m to avoid collecting or resuspending any material that had settled to the tank bottom. We then filtered water samples onto pre-combusted, 1.2 μm, GF/C filters for total suspended solids (TSS), which were frozen until analysis. Integrated whole water for total P (TP) and total N (TN) analyses were stored in glass digestion test tubes. Filtrate from 0.7 μm glass fiber filters (GFF) was also stored in glass digestion test tubes within 30 h of collection to measure total dissolved P (TDP) and total dissolved N (TDN). Filtrate from 0.45 μm nitrocellulose membrane filters was frozen for nitrate (NO3–) and ammonium (NH4+) analysis. Dissolved organic carbon (DOC) samples were stored frozen as filtrate from pre-combusted, 0.7 μm GFF filters. A subset of the integrated water sample was immediately frozen at −20°C in amber, HDPE bottles for microcystin analysis. Samples for phytoplankton identification and enumeration were collected by taking a subset of the integrated water sample and preserving it with 1% Lugols solution in amber vials. Within 30 h of collection, water filtered onto 0.7 μm GF/F filters were frozen until they were analyzed for chlorophyll-a (chl-a) and the phytoplankton pigment absorption coefficient (αϕ). Whole water for P–E parameters, gross primary production (GPP), and the maximum quantum yield of photosystem II (ϕPSII) were stored in the dark prior to running on the Water-Pulse Amplitude Modulated fluorometer (PAM, Heinz Walz GmbH) within 30 h of collection.
We collected samples for zooplankton identification and enumeration throughout the entire water column of each tank (1.25 m deep) using a 243 μm Wisconsin net (diameter = 200 mm) raised at approximately one-third of a meter per second. Zooplankton samples were immediately preserved with 4% formalin for enumeration.
We used sediment traps, positioned 0.5 m below the water’s surface, to determine sedimentation rates in each tank. Sediment traps were constructed from PVC with a 7.6 cm diameter and 6:1 height to aspect ratio (Bloesch and Burns, 1980). On each sampling date, the entire 2 L sediment trap was emptied into a HDPE collection bottle and homogenized by inverting 3 times. A portion of homogenized water was retained on pre-combusted, 1.2 μm, GF/C filters and later analyzed for TSS.
We measured TSS from both whole water integrated samples and sediment traps using standard methods (Section 2540 D and E; APHA 2017). Pre-weighed filters with retained material were dried at 105°C for 30 min and then weighed. Filters were then incinerated at 550°C for 20 min to burn off organic material before being weighed again. This loss-on-ignition analysis allowed us to differentiate TSS by subtracting the mass left after incineration, which is particulate inorganic matter (PIM), from the total filter mass before incineration, which is TSS. The difference is particulate organic matter (POM). TSS had a detection limit of 0.1 mg L–1. We determined sedimentation rates from the TSS measured in our sediment traps using (Kalff, 2002):
Total sample volume was always 2000 cm3, sediment trap area was 45.6 cm2, and the period that traps were deployed was 9 days.
Mean daily mixed layer irradiance () is a measure of the amount of light phytoplankton in the mixed layer are exposed to over 24 h. It was calculated using the formula:
Incident irradiance, , was calculated as the 24 h mean of PAR measurements taken at an onsite meteorological station (Natural Resources Conservation Service, site Ku-Nesa1) on day 0 (9/24/18) and day 9 (10/2/18). Mixing depth within each tank, Zmix, was calculated from temperature profiles using the rLakeAnalyzer package (Winslow et al., 2017). Profiles were taken one hour after GRF addition on all experimental days, 4 h after GRF addition on days 0, 5, 6, and 7, and 6 h after GRF addition on day 4. Occasionally, tanks would temporarily stratify in the afternoon between the water’s surface and 0.5 m. Tanks were isothermal every morning, indicating that even if a tank did stratify at the end of the previous day, it mixed during the night. We used the maximum depth of each tank, 1.25 m, as the value for Zmix when calculating .
Total P and TDP were measured spectrophotometrically using the ascorbic acid colorimetric method (Section 4500-P E; APHA, 2017) with a detection limit of 0.03 μmol L–1. TN and TDN were measured with the second derivative spectroscopy procedure (Crumpton et al., 1992) with a detection limit of 2.50 μmol L–1. Total and total dissolved P and N samples were measured in triplicate. NO3–, which was measured in duplicate on a Lachat QuikChem Flow Injection Analyzer (Lachat Method 10-107-04-1-B/C), had a detection limit of 0.36 μmol L–1. This method reports NO3– as NO3– plus nitrite (NO2–) based on the assumption that environmental NO2– concentrations are minimal. We measured NH4+ in duplicate on a Lachat QuikChem Flow Injection Analyzer (Lachat Method 10-107-06-1-K) based on the Berthelot reaction with a limit of detection of 0.71 μmol L–1. We report NH4+ as NH3 plus NH4+. We measured DOC in duplicate using a Shimadzu total organic C analyzer with the high-temperature combustion method (Section 5310B; APHA, 2017), with a limit of detection of 16.7 μmol L–1. Intracellular microcystin was extracted via 3 freeze-thaw cycles from whole water samples, which were then filtered through 0.45 μm GFFs. We measured total microcystin, both the intracellular microcystin previously released from freeze-thaw cycles and the extracellular microcystin present in the water, using indirect competitive ELISA (Enzyme Linked Immunosorbent Assay) kits from Abraxis LLC, which have a limit of detection of 0.15 μg L–1. Chl-a concentrations were quantified fluorometrically with a Turner Design Fluorometer (TD-700) after ethanol extraction and phaeophytin acid-correction (Knowlton, 1984; Sartory and Grobbelaar, 1986). The chl-a detection limit was 0.09 μg L–1.
Phytoplankton were identified to genus (Supplementary Table 2) by BSA Environmental Services Inc. (Guiry and Guiry, 2020), and enumerated using the Ütermohl method (Lund et al., 1958). Phytoplankton were allowed to settle for at least 20 h in a dark enclosure protected from vibrations and temperature changes prior to enumeration (Burkholder and Wetzel, 1989). Cell biovolume estimates are based on measurements from 10 cells in each taxon and were calculated using the formula of Hillebrand et al. (1999) for solid geometric shapes that most closely match cell shape. All enumerations were conducted using a LEICA DMiL inverted microscope at 800 × and 1260 × magnification, depending on the size of the dominant taxa, particulates, and variation in the range of taxon sizes. Heterocyte abundance was calculated for all phytoplankton samples. It is beneficial to group phytoplankton into functional groups when trying to understand ecological function (Salmaso et al., 2015). For example, both cryptophytes and dinoflagellates have 2 flagella, are known to participate in diel vertical migrations to take advantage of both the nutrient-rich hypolimnion and light-replete surface waters, and can supplement metabolic requirements with mixotrophy (Raven and Richardson, 1984; Lee, 2008). We classified phytoplankton by the following 6 taxonomic groups: (1) potentially toxigenic cyanophyta (Chapman and Foss, 2019), (2) non-toxin producing cyanophyta, (3) chlorophyta, (4) euglenophyta, (5) cryptophyta and dinoflagellates, and (6) chrysophyta, including chrysophytes, bacillariophyta, ochrophytes, and haptophytes (Supplementary Table 3).
We assessed phytoplankton physiology, including ϕPSII, P–E parameters (alpha normalized to chl-a [αB], the light saturation threshold normalized to chl-a [EkB], and the maximum relative electron transport rate [rETRMAX]), and gross primary productivity normalized to chl-a (GPPB) following the procedures outlined in Petty et al. (2020). Within 30 h of collection, we measured ϕPSII in triplicate with a Water-PAM fluorometer on whole, integrated water that had been dark adapted for 30 min. Water samples were corrected for background fluorescence with 0.2 μm PTFE sample water filtrate. We also used the Water-PAM to perform rapid light curves. For each light curve, the light limited slope (α) and light saturation threshold (Ek) were defined as ϕPSII fit against irradiance to a light intensity (E) using the normalized version of Webb et al. (1974):
where rETRMAX was the product of α and Ek. We divided by Ek to assess light deficiency within each tank. When this ratio is above 1, light availability is greater than phytoplankton light saturation and light does not limit photosynthesis. Below 1, phytoplankton do not receive enough light to reach saturation and may experience light deficiency (Hecky and Guildford, 1984). The areal pigment absorption coefficient (αϕ), a factor in our calculation of GPPB, was quantified using the quantitative filter technique by measuring absorbance at 350 – 750 with a scanning spectrophotometer (Agilent Cary60 UV/VIS) before and after depigmentation with a sodium hypochlorite solution (4.00 – 4.99% available chlorine). aϕ was then calculated using:
where AP is absorption before and ANAP is absorption after depigmentation, β is the path-length amplification factor to adjust for differences in absorption between water and filter, and Vf/Af is the ratio of volume of water filtered to the filter area (Silsbe et al., 2012). We determined GPP using the R package phytotools (Silsbe and Malkin, 2015). This package is based on the primary production model of Fee (1990) and incorporates chl-a, P–E parameters, aϕ, Kd, and .
Zooplankton were enumerated by the Central Plains Center for Bioassessment to the following taxonomic groups: cladocerans, adult copepods, and copepod nauplii (Thorp and Covich, 2001). Before being photographed, samples were switched from the 4% fixation preservative from the field to 80% ethanol. Images of each zooplankton were taken using a Motic Plus 2.0 digital camera at 10 × magnification (87.7 pixels mm–1) using Image-J software (National Institutes of Health, Bethesda, MD, United States). All abundance calculations of each zooplankton taxa assumed 100% net filtering efficiency.
A Shapiro–Wilk test was used to test for normality and a Levene’s test to assess homoscedasticity. The alpha value for all statistical tests was set at 0.05. All statistical analyses were performed in Program R (R Core Team, 2019) and all figures were created using the ggplot2 package (Wickham, 2009).
We tested for a significant difference in Kd and the PAR ratio (0.5 m water/air reading) between tanks that received GRF and the control tanks that did not receive GRF. We took the mean of control (n = 3) and GRF (n = 14) tanks. Neither parameter was normally distributed, even after transformation, so we used a Kruskal–Wallis test to look for significant differences at one, 4, and 6 h after GRF addition and a Dunn’s post hoc test to identify where significant differences existed.
Unless stated otherwise, statistical analyses were performed on the change in each parameter between days 0 and 9. We calculated this change by subtracting the value for each parameter on day 9 from the value for that parameter on day 0 (i.e., day 9 - day 0). Positive values indicate an increase in the parameter on day 9, while negative values indicate a decrease. We used a One-Way Analysis of Variance (ANOVA) to test whether the change in each parameter was significantly different between the control (n = 3), chlorophyte-dominated (n = 6), and cyanophyte-dominated (n = 8) tanks. In instances where a parameter was not normally distributed, even after transformation, we used a Kruskal–Wallis test. When a significant difference did exist, we used a Tukey post hoc to identify significant differences for parameters that followed a parametric distribution, and a Dunn’s test on parameters that did not follow a normal distribution. For tanks where the microcystin concentrations were below the limit of detection, we used the limit of detection, 0.15 μg L–1, for statistical analyses. We compared chl-a concentrations and phytoplankton biovolume to assess photoacclimation. Neither chl-a nor biovolume were normally distributed, even after transformation, so we used the non-parametric Spearman’s Rank correlation to assess the relationship between these 2 variables.
We assessed water clarity by measuring Kd and the PAR ratio (0.5 m water/air reading) in each tank. Kd was significantly higher (Kruskal–Wallis p < 0.0001, df = 1, χ2 = 118.84) and the PAR ratio (Kruskal–Wallis p < 0.0001, df = 1, χ2 = 112.18) was significantly lower in tanks that received GRF. The PAR ratio was significantly lower one hour after GRF addition than 4 (Kruskal–Wallis p < 0.0001, df = 2, χ2 = 26.46) or 6 hours (Kruskal–Wallis p = 0.0002, df = 2, χ2 = 26.46), but not significantly different between 4 and 6 h (Kruskal–Wallis p = 0.1709, df = 2, χ2 = 26.46; Figure 2). In tanks that received GRF, Kd was significantly higher one hour after GRF addition than 4 (Kruskal–Wallis p < 0.0001, df = 2, χ2 = 37.10) or 6 (Kruskal–Wallis p < 0.0001, df = 2, χ2 = 37.10) hours, but not significantly different between 4 and 6 h (Kruskal–Wallis p = 0.2347, df = 2, χ2 = 37.10; Figure 2). Kd was ∼3 × higher in tanks that received GRF compared to the control tanks (Figure 2). Tanks that received GRF had PAR ratios 86.4, 60.4, and 67.7% lower, and mean Kds 80.5, 73.6, and 68.8% higher, compared to control tanks for one, 4, and 6 h after GRF application, respectively.
Figure 2. Comparison of the light environment between tanks that received glacial rock flour (GRF) and tanks that did not receive GRF. PAR ratio (0.5 m water/air reading; (A) and vertical light attenuation coefficient (Kd; B) were measured in all tanks after GRF was added to all (n = 14) but the control tanks (n = 3). Both the PAR ratio and Kd were measured one hour after GRF addition every day of the experiment, 4 hours after addition on days 0, 5, 6, and 7, and 6 h after addition on day 4. The light ratio was determined by dividing photosynthetically active radiation (PAR) measurements made at 0.5 m depth by PAR measurements from above the water’s surface in the air. Among GRF tanks, the light ratio was significantly lower one hour after GRF addition than 4 (Kruskal–Wallis p < 0.0001, df = 2, χ2 = 26.46) or 6 h (Kruskal–Wallis p = 0.0002, df = 2, χ2 = 26.46), but not significantly different between 4 and 6 hours (Kruskal-Wallis p = 0.1709, df = 2, χ2 = 26.46; Figure 2). Kd was significantly higher one hour after GRF addition (Kruskal–Wallis p < 0.0001, df = 2, χ2 = 37.10), but not 4 nor 6 hours after addition (Kruskal–Wallis p = 0.2347, df = 2, χ2 = 37.10). For each time, the light ratio was significantly lower (Kruskal–Wallis p < 0.0001, df = 1, χ2 = 75.99) in tanks that received GRF compared to control tanks that did not. For each time, Kd was significantly higher (Kruskal–Wallis p < 0.0001, df = 1, χ2 = 87.88) in tanks that received GRF compared to control tanks that did not.
Water temperatures remained below 25°C throughout the experiment (Table 3). The change in temperature over the course of the experiment was not significantly different between control, chlorophyte-, and cyanophyte- dominated tanks (Table 4 and Supplementary Figure 3).
Table 3. Water quality and phytoplankton physiology parameters. Control (Con), chlorophyte- (Chloro), and cyanophyte- (Cyano) dominated mesocosm tanks prior to glacial rock flour addition (day 0) and at the end of the experiment (day 9).
Mean TSS sedimentation rates were 0.1 g m–2 day–1 (range = below detection limit [BDL] – 0.1 g m–2 day–1) in control, 1.9 g m–2 day–1 (range = 0.8 – 2.9 g m–2 day–1) in chlorophyte-dominated, and 2.3 g m–2 day–1 (range = 1.0 – 3.4 g m–2 day–1) in cyanophyte-dominated tanks. Mean PIM sedimentation rates were BDL (range = BDL – 0.1 g m–2 day–1) in control, 1.9 g m–2 day–1 (range = 0.8 – 2.8 g m–2 day–1) in chlorophyte-dominated, and 2.2 g m–2 day–1 (range = BDL – 0.1 g m–2 day–1) in cyanophyte-dominated tanks. Mean POM sedimentation rates were always BDL in control and 0.1 g m–2 day–1 (range = BDL – 0.1 g m–2 day–1) in both chlorophyte- and cyanophyte-dominated tanks (Table 3).
Between days 0 and 9, increased from 106.67 to 115.45 μmol photons m–2 s–1 in control, and from 57.14 to 64.36 μmol photons m–2 s–1 in cyanophyte-dominated tanks. decreased from 114.16 to 110.66 μmol photons m–2 s–1 in chlorophyte-dominated tanks (Table 3 and Supplementary Figure 3). The change in throughout the experiment was not significantly different between the control, chlorophyte-, and cyanophyte- dominated tanks (Table 4). The values we observed throughout the experiment were above the light deficiency threshold of 41.7 μmol photons m–2 s–1 (Hecky and Guildford, 1984) in all tanks.
We report all water chemistry parameters as the mean of control, chlorophyte-, and cyanophyte- dominated mesocosm tanks (Table 3). To determine the influence of GRF on water chemistry, we examined the change in each parameter between day 9 and day 0 and tested to see if this change was different between the control, chlorophyte-, and cyanophyte- dominated tanks, but in most cases it was not (Tables 3, 4). The change in water column TSS, PIM, and POM was not significantly different between any of the experimental groups. TSS increased from 0.8 to 3.1 mg L–1, from 1.5 to 7.3 mg L–1, and from 4.5 to 20.2 mg L–1 in the control, chlorophyte-, and cyanophyte- dominated tanks, respectively. PIM decreased from 0.4 to 0.3 mg L–1 in the control, but increased from 1.0 to 5.5 mg L–1 and from 2.2 to 10.5 mg L–1 in the chlorophyte- and cyanophyte- dominated tanks, respectively. POM increased in the control and cyanophyte-dominated tanks from 0.9 to 2.8 mg L–1 and from 6.6 to 9.7 mg L–1, respectively, but decreased in the chlorophyte-dominated tanks from 2.9 to 1.8 mg L–1 (Table 3 and Supplementary Figure 3).
TP concentrations decreased from 0.74 to 0.67 μmol L–1 in the control and from 5.89 to 3.85 μmol L–1 in the cyanophyte-dominated tanks. All cyanophyte-dominated tanks received P amendments for 8 weeks prior to the beginning of the experiment (Table 1). The change in TP concentrations was not significantly different between the control and chlorophyte-dominated, nor the control and cyanophyte-dominated tanks (Table 4). TP concentrations were unchanged over the course of the experiment in the chlorophyte-dominated tanks (mean = 0.57 μmol L–1; Table 3 and Supplementary Figure 4). The change in TP concentrations between days 9 and 0 in the cyanophyte-dominated tanks was significantly greater than the change between days 9 and 0 in the chlorophyte-dominated tanks.
The change in TN concentrations between days 9 and 0 was not significantly different between the control, chlorophyte-, and cyanophyte- dominated tanks (Table 4). TN concentrations were stable in the control and chlorophyte-dominated, but decreased from 163.06 to 153.54 μmol L–1 in the cyanophyte-dominated tanks (Table 3 and Supplementary Figure 4).
The TN:TP molar ratio can serve as an indicator of phytoplankton nutrient deficiency. We report the natural log (ln) of the TN:TP ratio as this transformation reduces bias inherent with calculating the mean of a ratio (Isles, 2020). If the ln(TN:TP) molar ratio exceeds 3.91, then the phytoplankton community is P-deficient, and if it is lower than 3.00 then the phytoplankton community is N-deficient (Guildford and Hecky, 2000). Between 3.00 and 3.91, it could be N, P, or some other factor restricting growth (Guildford and Hecky, 2000). The mean ln(TN:TP) molar ratios for control and chlorophyte-dominated tanks on day 0 were 4.61 (range = 4.07 – 4.96) and 4.74 (range = 4.30 – 5.47), respectively, indicating P-deficient conditions (Tables 1, 3; Guildford and Hecky, 2000). Cyanophyte-dominated tanks had a mean ln(TN:TP) molar ratio of 3.39 (range = 2.74 – 4.23) on day 0 (Tables 1, 3 and Supplementary Figure 4). This value was between P-deficient and N-deficient conditions, suggesting that these tanks could have been either N or P deficient. Alternatively, cyanophyte-dominated tanks could have been growth-limited by other factors such as light (Guildford and Hecky, 2000). Heterocytes were only present in the cyanophyte-dominated tanks. They were identified in 6 tanks on day 0 and only 4 tanks on day 9 in low densities (mean = 5,850, range = 27 – 13,800 cells L–1). The change in the ln(TN:TP) ratio between days 9 and 0 was not significantly different between the control, chlorophyte- and cyanophyte- dominated tanks (Table 4).
The change in TDN, TDP, NO3–, and NH4+ concentrations were not significantly different between any of the experimental groups (Table 4). TDN and TDP concentrations were stable in all tanks throughout the experiment, though a notable deviation was the decrease in TDP from 1.52 to 1.27 μmol L–1 in the cyanophyte-dominated tanks (Table 3). NO3– concentrations were never above the limit of detection in control, and decreased from 3.94 to 2.78 μmol L–1 and from 1.75 to 0.45 μmol L–1 in chlorophyte- and cyanophyte- dominated tanks, respectively (Table 3). NH4+ concentrations increased from 1.51 to 1.83 μmol L–1 and from 8.53 to 12.17 μmol L–1 in the control and cyanophyte-dominated tanks, respectively, but decreased from 3.49 to 3.03 μmol L–1 in the chlorophyte-dominated tanks (Table 3 and Supplementary Figure 4).
Over the course of the experiment, DOC concentrations increased from 1009.25 to 1063.20 μmol L–1 in the control, from 1015.00 to 1030.73 μmol L–1 in the chlorophyte-dominated, and from 1032.98 to 1081.02 μmol L–1 in the cyanophyte-dominated tanks (Table 3 and Supplementary Figure 4). The difference between day 9 and day 0 DOC concentrations in the cyanophyta-dominated tanks were significantly larger than in the chlorophyte-dominated tanks (Table 4), but there was no significant difference between the control and neither the chlorophyte- nor cyanophyte-dominated tanks (Table 4).
Microcystin concentrations ranged from BDL to 4.04 μg L–1 (Table 3). Over this 9-day experiment, they increased from 0.17 to 0.19 μg L–1 in the control, from 0.16 to 0.19 μg L–1 in the chlorophyte-dominated, and from 0.70 to 0.87 μg L–1 in the cyanophyte-dominated tanks (Table 3). The change in microcystin concentrations between days 9 and 0 was not significantly different among any of the experimental groups (Table 4 and Supplementary Figure 3).
Photoacclimation is photosynthesizer’s physiological response to changes in light and displays as an increase or decrease in chl-a concentrations relative to phytoplankton biomass (Falkowski and LaRoche, 1991). To check if photoacclimation was occurring in the experimental tanks to which we added GRF, we examined the relationship between chl-a concentrations and phytoplankton biovolume. There was a significant positive correlation between these parameters prior to GRF addition (day 0; Spearman’s Rank, p = 0.0023, rho = 0.76, n = 14) and on day 9 (Spearman’s Rank, p = 0.0038, rho = 0.74, n = 14), indicating that the phytoplankton did not exhibit photoacclimation. Chl-a concentrations decreased from 3.54 to 2.09 μg L–1 and from 2.97 to 1.53 μg L–1 in the control and chlorophyte-dominated, respectively, but increased from 48.7 to 63.52 μg L–1 in the cyanophyte-dominated tanks (Table 3 and Supplementary Figure 5). The change in chl-a concentrations and phytoplankton biovolume between days 9 and 0 was not significantly different between the control, chlorophyte- and cyanophyte- dominated tanks (Table 4). Total phytoplankton biovolume decreased from 4.43 to 2.64 mm3 L–1 and from 35.31 to 28.13 mm3 L–1 in the control and cyanophyte-dominated tanks, respectively. Total phytoplankton biovolume remained unchanged in the chlorophyte-dominated tanks (mean = 2.55 mm3 L–1; Table 3). Within all tanks, dinoflagellates comprised only 23.0, 13.3, and 0.1%, respectively, of the cryptophyte and dinoflagellate functional group. Throughout the rest of this study, we refer to the group containing cryptophytes and dinoflagellates as the cryptophyte functional group.
Within the chlorophyte- and cyanophyte-dominated tanks, declines in cyanophytes (49.4 and 77.9%, respectively) were compensated by an increase in cryptophytes (105.7 and 240%, respectively; Figure 3). Much of this cryptophyte increase can be attributed to the genus Cryptomonas, which comprised 32.2 and 37.1% of total phytoplankton biovolume on day 9 in chlorophyte- and cyanophyte-dominated tanks, respectively (Table 2 and Supplementary Table 2). The change in potentially toxigenic cyanophyta biovolume was significantly different in the cyanophyte-dominated tanks compared to the control and chlorophyte-dominated tanks (Table 4). Potentially toxigenic cyanophytes increased by 16.7% in control, and declined by 100 and 76.2% in chlorophyte and cyanophyte-dominated tanks, respectively. All cyanobacteria (potentially toxigenic taxa and non-toxin producing taxa combined) declined by 22.8, 49.4, and 77.9% in the control, chlorophyte- and cyanophyte-dominated tanks, respectively, between days 9 and 0. While the change in biovolume from days 9 to 0 of all other taxonomic groups was not significantly different between experimental tanks, cryptophytes increased by 18.8, 105.7, and 240.0% in the control, chlorophyte- and cyanophyte-dominated tanks, respectively.
Figure 3. Phytoplankton biovolume categorized by functional group. Biovolume for day 0 (A) and day 9 (B) for control tanks, tanks dominated by chlorophytes at the beginning of the experiment, and tanks dominated by cyanophytes at the beginning of the experiment. The chrysophyta* group includes chrysophyta, bacillariophyta, haptophyta, and ochrophyta.
Phytoplankton samples with ϕPSII values below 0.65 can indicate that the communities are physiologically stressed due to light, nutrients, or some combination thereof (Kromkamp et al., 2008). At no point on days 0 nor 9 did ϕPSII exceed the empirical optimum threshold of ∼0.65 in any tank (Table 3 and Supplementary Figure 6). ϕPSII decreased from 0.57 to 0.53 and from 0.61 to 0.56 in the control and chlorophyte-dominated tanks, respectively, but increased from 0.34 to 0.48 in the cyanophyte-dominated tanks (Table 3 and Supplementary Figure 6). We observed no significant difference in the change in ϕPSII between the control, chlorophyte-, and cyanophyte- dominated tanks.
Additional indicators of phytoplankton physiology also remained largely unchanged after the addition of GRF (Table 4). The light utilization efficiency parameter (αB) increased from 0.51 to 0.58 and from 0.48 to 0.65 in the control and chlorophyte-dominated tanks, respectively, but decreased from 0.07 to 0.05 in the cyanophyte-dominated tanks. The light saturation parameter (EkB) increased from 153.23 to 377.22 μmol photons (μg Chl-a–1) m s–1 in the control, but decreased in both the chlorophyte- and cyanophyte- dominated tanks from 428.24 to 388.69 and from 80.90 to 32.34 μmol photons (μg Chl-a–1) m s–1, respectively (Table 3). rETRMAX decreased from 315.69 to 291.40 in the control, but increased from 175.63 to 222.69 and from 136.12 to 197.21 photons reemitted photons absorbed–1 in the chlorophyte- and cyanophyte- dominated tanks, respectively (Table 3). The light deficiency parameter (/Ek) decreased in control, chlorophyte-, and cyanophyte-dominated tanks from 0.85 to 0.45, 0.55 to 0.43, and from 0.20 to 0.18, respectively (Table 3 and Supplementary Figure 6). We observed no significant difference in the change in αB, EkB, rETRMAX, nor /Ek, between the control, chlorophyte- and cyanophyte- dominated tanks.
Gross primary productivity normalized to chl-a (GPPB) increased from 25.9 to 33.3, from 15.4 to 18.2, and from 15.2 to 22.8 mmol O2 (μg Chl-a–1) m day–1 in the control, chlorophyte- and cyanophyte- dominated tanks, respectively (Table 3 and Supplementary Figure 6). We did not observe a significant difference in the change in GPPB between any of the tanks (Table 4).
To evaluate whether zooplankton dynamics were influenced by GRF addition and/or if grazing was impacting phytoplankton populations differently between tanks and over the course of the experiment, we compared total zooplankton, cladoceran, and copepod abundance between days 0 and 9 (Supplementary Table 4 and Figure 4). Copepods were separated into adults and nauplii. Between days 9 and 0, the change in total zooplankton, cladoceran, adult copepod, and copepod nauplii abundance was not significantly different in any of the tanks (Table 4). While not significant (Table 3 and Supplementary Figure 7), total zooplankton abundance increased from 70.85 to 111.87 in control, but decreased from 79.56 to 31.78 and from 211.84 to 126.04 in chlorophyte- and cyanophyte-dominated tanks, respectively. Adult copepod abundance increased from 14.82 to 16.00 individuals L–1 in the control, but decreased from 17.36 to 12.73 and from 89.05 to 59.59 individuals L–1 in chlorophyte- and cyanophyte- dominated tanks, respectively. Copepod nauplii abundance decreased from 26.73 to 16.59 in control, from 31.46 to 12.75 in chlorophyte-dominated, and from 63.54 to 39.01 individuals L–1 in cyanophyte-dominated tanks. Cladocerans increased from 29.30 to 79.27 in control tanks, but decreased from 30.75 to 6.30 and from 59.25 to 27.43 individuals L–1 in chlorophyte- and cyanophyte- dominated tanks, respectively (Table 3 and Supplementary Figure 7).
Figure 4. Zooplankton abundance in mesocosm tanks. Abundance was measured on day 0 (A) and day 9 (B) for control tanks, tanks dominated by chlorophytes at the beginning of the experiment, and tanks dominated by cyanophytes at the beginning of the experiment.
Light availability throughout the water column was significantly reduced in all mesocosm tanks that received GRF relative to the control tanks. This reduction in light was maintained throughout the 9-day experiment and resulted in a 77.9% decline in cyanophyte biovolume in tanks dominated by cyanophytes. While total phytoplankton biovolume did not change after the addition of GRF, cryptophytes increased by 240.0% in cyanophyta-dominated tanks. In the chlorophyte-dominated tanks, cyanophytes decreased by 49.4% while cryptophytes increased by 105.7%. Changes in cyanophytes and cryptophytes were less in the control tanks where cyanophytes decreased by 22.8% and cryptophytes increased by 18.8% between days 0 and 9.
The addition of GRF to mesocosm tanks significantly reduced PAR by half and maintained this relatively low light level throughout the 9-day experiment. PIM concentrations increased by ∼80% from day 0 to day 9 in the chlorophyte- and cyanophyte-dominated tanks that received GRF. POM decreased by 37.9% in chlorophyte-dominated tanks and increased by only 32.0% in cyanophyte-dominated tanks between day 0 and day 9, suggesting that the reduction in light is due to increases in suspended inorganic particles derived from the GRF. In tanks that received GRF, the mean Kd value was 3.77 m–1. This was higher than what occurs in many glacially fed, natural lakes. Glacially fed lakes in New Zealand, Chile, and Canada have mean Kds of 0.96 m–1 and a maximum of 2.28 m–1 (Rose et al., 2014). Lakes in the US Rocky Mountains do not exceed Kd of 0.30 m–1 (Slemmons and Saros, 2012) and Kd in glacially fed Mascardi Lake in Argentina is often between 0.40 and 0.75 m–1 (Modenutti et al., 2000; Hylander et al., 2011). Our Kd values are likely higher than those that occur in water bodies, due to the smaller scale of our experiment, the lack of flow in our mesocosm tanks, and the higher rate of GRF addition. In natural systems, GRF inputs are relatively constant due to consistent tributary inflows creating horizontal gradients in turbidity as particles fall out of suspension with increasing distance from tributary inflows (Laspoumaderes et al., 2013).
Our experimental approach was to manipulate light while maintaining nutrient concentrations. We wanted to ensure that the GRF additions were not adding or precipitating nutrients in the experimental tanks. TN, TDN, NO3–, NH4+, and TDP concentrations remained largely unchanged throughout this 9-day experiment. The change in TP from days 9 to 0 was significantly different in the chlorophyte-dominated tanks compared to the cyanophyte-dominated tanks, but not compared to the control tanks. If the addition of GRF precipitated P from the water column, we would have expected to see a decline in TP in all of the GRF tanks, including both the chlorophyte- and cyanophyte- dominated tanks. This was not the case as TP only declined by 0.01 μmol L–1 in the chlorophyte-dominated tanks. We believe the decrease in cyanophyta and increase in cryptophyta can be attributed to reduced light and not an increase in P deficiency because we also saw no significant change in the ln(TN:TP) molar ratio in any of the tanks.
Microcystin concentrations were low throughout the experiment but increased in all tanks between days 0 and 9. The decline in cyanophyta in the chlorophyte- and cyanophyte- dominated tanks could explain the increase in microcystin concentrations. As cyanobacterial cells lyse, they can release intracellular microcystin into the water column (Greenfield et al., 2014). Microcystin concentrations never exceeded 4.04 μg L–1 on day 9 and increases in microcystin between days 0 and 9 were never more than 0.17 μg L–1, despite the declines we observed in cyanophyta. Non-toxic strains of the cyanobacterium Microcystis outcompete toxic strains at low light levels (Kardinaal et al., 2007), which could be why we did not see a greater increase in microcystin concentrations following the addition of GRF.
We anticipated that increased turbidity resulting from GRF additions would negatively impact zooplankton abundance and diversity, especially for filter-feeding cladocerans (Sommaruga, 2015). High concentrations of suspended particles can make it difficult for filter-feeding cladocerans to obtain food and they are often absent from lakes with high GRF inputs (Barouillet et al., 2019). Copepods are able to survive higher turbidities than other zooplankton (Sommaruga, 2015). We observed a decline in cladoceran, adult copepod, and copepod nauplii abundance in the chlorophyte- and cyanophyte- dominated tanks. The decline in cladocerans could be the result of an increase in fine particulates, which interferes with their ability to filter organic particles out of the water (Sommaruga, 2015; Barouillet et al., 2019). In our experiment, cladocerans declined in tanks that received GRF, but increased in the control tanks. A longer study period is necessary to properly evaluate whether the decline in zooplankton we observed is a direct result of GRF application, or simply natural variation in the population. Our 9-day experiment was not long enough to see a dramatic change in the zooplankton community life cycle as cladocerans can live for 15 – 50 days depending on species (Sarma et al., 2002), and lay new egg clutches every 3 – 7 days (Dodson and Frey, 1991). It is not uncommon for many copepods to live up to a year (Allan, 1976), and it can take a month before nauplii reach sexual maturity (Gilbert and Williamson, 1983). The additional time it would take for at least one generation of zooplankton to be born likely explains why we did not see a significant change in zooplankton abundance by day 9 of our experiment. Our findings suggest that additional work is needed over a longer time period to better understand how the addition of GRF impacts zooplankton communities.
The dominant cyanophyte in cyanophyte-dominated tanks at the beginning of the experiment was Aphanizomenon. While Aphanizomenon has been shown to persist at light levels as low as 50 μmol photons m–2 s–1 in culture, its highest growth rates occur above 150 μmol photons m–2 s–1 (Hadas et al., 2002; Üveges et al., 2012) and it is often outcompeted in reduced light environments (Huisman et al., 1999). Cryptomonas, the most common cryptophyte on day 9, can dominate algal communities when light levels are as low as 15 μmol photons m–2 s–1 (Lizotte and Priscu, 1992). The reduced light environment created from GRF addition could have enabled Cryptomonas to replace Aphanizomenon as the most prevalent genera.
The switch from a cyanophyte-dominated community to one where cryptophytes are the dominant taxon is efficient and beneficial for trophic interactions. Cyanophytes create inefficient trophic transfers due to their low composition of polyunsaturated fatty acids (Brett et al., 2009). Zooplankton meet most of their polyunsaturated fatty acid requirements from eukaryotic phytoplankton and are not able to survive long periods when these fatty acids are unavailable (Grosbois et al., 2017). Cyanophytes can be too large for many gape-limited grazers to consume, either because they form mucilaginous colonies or long filaments (Haney, 1987). The production of cyanotoxins can also be detrimental to grazers, though the magnitude of these effects are debated (Rohrlack et al., 2001; Paes et al., 2016). Conversely, cryptophytes are highly nutritious and are an important component of aquatic food webs (Stemberger and Gilbert, 1985; Sarnelle, 1993). Thus, the switch from cyanophyte- to cryptophyte-dominated phytoplankton communities likely provided a net benefit to the treated mesocosms by creating a highly edible phytoplankton community for primary consumers.
Both cryptophytes and cyanophytes can tolerate low light, but in our study, cryptophytes replaced cyanophytes when GRF was added. Cryptophytes, especially of the genus Cryptomonas, are better suited to sustained periods of reduced light availability and are often the dominant phytoplankton in permanently ice-covered lakes (Gervais, 1998). Cryptomonas was the most common cryptophyte we observed on day 9 in tanks that were originally dominated by cyanophytes on day 0 (Supplementary Table 2). Cryptophytes can position themselves at favorable light intensities using their 2 flagella, and many are mixotrophs that can supplement their metabolic (Porter, 1988) and nutrient (Urabe et al., 2000) requirements with heterotrophy. This is consistent with existing projections that protists, like cryptophytes, sometimes use phagotrophy to survive and outcompete phototrophs at low irradiance levels (Jones, 2000; Schwaderer et al., 2011). The ability to acquire energy through both heterotrophy and autotrophy provides mixotrophs with a competitive advantage (Tittle et al., 2003). Some models even suggest a positive relationship between mixotrophy and primary productivity (Hammer and Pitchford, 2005; Stoecker et al., 2017) because mixotrophs can use phagotrophy to relieve nutrient stress, enabling them to be productive in nutrient-deficient conditions (Jost et al., 2004). None of our tanks were nutrient sufficient (Guildford and Hecky, 2000). Phagotrophy might explain why GPPB increased in all of them, albeit at low rates. Our maximum increases of 7.6 mmol O2 [μg Chl-a–1] m day–1 are consistent with low increases in GPP for phagocytic phytoplankton (Hammer and Pitchford, 2005).
The increase in GPPB over the course of the experiment also suggests that the new cryptophyte-dominated community used light more efficiently than the previous chlorophyte- or cyanophyte- dominated communities. Phycobiliproteins, secondary pigments present in both cyanophytes and cryptophytes, can lead to an inverse relationship between αB and PAR that is contrary to the positive relationship observed in other phytoplankton groups (MacIntyre et al., 2002; Overkamp et al., 2014). This could explain why αB in the chlorophyte-dominated tanks, which switched from a chlorophyte- to a cryptophyte- dominated community increased, and why αB in the cyanophyte-dominated tanks did not change. Cyanophyta can have Ek values between 150.82 and 783.30 μmol photons m–2 s–1 (Zhang et al., 2011), while cryptophytes under ice cover have been between 15 and 45 μmol photons m–2 s–1 (Lizotte and Priscu, 1992). Our cyanophyte-dominated tanks experienced a 2.5-fold decrease in EKB from day 0 to 9, suggesting that the shift to dominance by cryptophytes impacted P–E parameters.
Eukaryotic phytoplankton are physiologically stressed at ϕPSII values below 0.65, while cyanophyta thresholds are lower, typically between 0.4 and 0.6 (Campbell et al., 1998; Kromkamp et al., 2008). In our cyanophyte-dominated tanks, ϕPSII increased from 0.34 to 0.48 over the course of the experiment, corresponding with a decline in prokaryotic cyanophyta and an increase in eukaryotic cryptophytes. The control and chlorophyte tanks remained dominated by eukaryotes throughout the experiment and exhibited little change in ϕPSII. Phytoplankton communities in all tanks were physiologically stressed throughout the experiment.
Our findings provide important insights for the nutrient load hypothesis (Brauer et al., 2012), which predicts phytoplankton dominance based on nutrient concentrations and light availability. It postulates that in low-nutrient environments, the ln(TN:TP) ratio will determine phytoplankton composition while in high-nutrient systems, absolute nutrient concentrations explain which species dominate (Brauer et al., 2012). Increased nutrient enrichment leads to an increase in algal biomass, which in turn reduces light availability through self-shading. Thus, when absolute nutrient concentrations are high, light becomes the single limiting resource. This hypothesis assumes that cyanophyta are superior competitors for light and will dominate the community unless light limitation is reached, at which point total phytoplankton biomass is expected to decline (Brauer et al., 2012). Our results suggest the assumption that cyanophytes will be sole “winners” in this scenario should be revised to include phytoplankton functional traits (Figure 5). These functional traits could enable certain taxa to thrive in the reduced light environment created by bloom formation and self-shading.
Figure 5. Adaptation of the nutrient load hypothesis from Brauer et al. (2012). This hypothesis predicts which phytoplankton dominate based on competition for nitrogen (N), phosphorus (P), and light. The nutrient load hypothesis is based on the assumption that cyanobacteria are superior competitors for light and are able to dominate when N and P concentrations are high (Brauer et al., 2012). We add an additional box to highlight the nuance that functional traits can add to competition for these resources, specifically light. While cyanobacteria often dominate when light is the limiting resource, our findings suggest that cryptophytes can outcompete cyanobacteria under specific light levels due to functional traits.
The nutrient load hypothesis does consider some functional traits for cyanophyta such as their ability to fix atmospheric N2 or regulate their buoyancy with gas vacuoles. In our experiment, heterocystous N2 fixation rates were insubstantial given the low (mean = 5,850 cells L–1) number enumerated. The ln(TN:TP) ratios were lowest in the cyanophyte-dominated tanks, but were always between the N- and P- deficiency thresholds where it can be N, P, or something else that limits growth (Guildford and Hecky, 2000). This, along with the increase in NH4+ concentrations observed from days 0 to 9, suggest that the cyanophyte-dominated tanks were not N-deficient. Functional traits for other phytoplankton groups, such as mixotrophy or flagella, both of which might enable other phytoplankton to dominate in low light environments, are not considered (Brauer et al., 2012). Contrary to predictions from the nutrient load hypothesis (Brauer et al., 2012), cyanophyta were not the dominant taxa at the end of our experiment. Cryptophytes were, suggesting that at high nutrient concentrations, functional traits add nuance to competition for light not previously considered.
While cyanobacteria often dominate when light is the limiting resource, our findings suggest that cryptophytes can outcompete cyanobacteria under low light levels. In Lake Peipsi, Estonia/Russia, cyanobacteria are replaced by cryptophytes shortly after the formation of ice and its associated reduction in light availability (Blank et al., 2009). Further experimentation is required to quantify the thresholds where this shift from nutrient stoichiometry to functional traits occurs, but the importance of functional traits in determining phytoplankton community composition should not be overlooked.
One of the challenges faced by lake managers and drinking water treatment plant operators is mitigating or controlling harmful algal blooms. In 2014, a toxic cyanobacterial bloom in Lake Erie cost the Toledo drinking water plant ∼$4 million and had a total economic impact of ∼$65 million (Bingham et al., 2015). Reductions in external nutrient loading can successfully lower cyanobacterial biomass, but it usually takes years after government regulations have been enacted before declines in biomass are observed (Osgood, 2017). Sometimes, managers need to reduce phytoplankton biomass over a much shorter time scale if, for example, the resource is being used for drinking water. In other instances, it might not always be feasible to reduce external nutrient loading, such as in water bodies with watersheds dominated by agriculture or urban areas. In these situations, alternative geoengineering strategies can be used. Solid-phase P sorbents may be the most common geoengineering approach. These materials are clays enriched with aluminum (Gibbs et al., 2010), iron (Zamparas et al., 2012), or lanthanum (Haghseresht et al., 2009) and work by binding to any soluble reactive P they contact as they sink through the water column.
One benefit of GRF over other geoengineering techniques is its effectiveness on a short timescale. We saw a 49.4 and 77.6% decline in cyanobacteria in chlorophyte- and cyanophyte-dominated tanks, respectively, after 9 days. This decline occurred more quickly than the several months to a year phytoplankton biomass declines after the addition of solid-phase P sorbents (Epe et al., 2017; Wagner et al., 2017). Re-application of solid-phase P sorbents can be required in as short as a couple of years to as long as a decade, depending on lake morphology, nutrient inputs, inorganic particle inputs, and sedimentation rates (Lürling and van Oosterhout, 2012; Mackay et al., 2014; Wagner et al., 2017). Another benefit of GRF is that it results in a decrease in cyanobacteria, but not total phytoplankton biovolume, while solid-phase P sorbents are typically associated with a decline in total phytoplankton biomass (Epe et al., 2017). Maintaining phytoplankton biomass is important in systems where fish yield is a concern (Downing and Plante, 1993).
A consideration to using GRF is that it adds inorganic particles into the water, a concern in reservoirs with limited storage capacity (deNoyelles and Kastens, 2016). Of the 14 tanks that received GRF, the highest TSS sedimentation rate was 3.4 g m–2 day–1. This rate is well within the range of natural sedimentation and is lower than some rates found in the North American Midwest. Sedimentation rates in Iowa lakes can range from 11.6 to as high as 203.0 g m–2 day–1 during the summer (Canfield et al., 1982). We added GRF based on tank surface area at a rate of 0.68 kg m–2 day–1. For the average small impoundment of 0.027 km2 (Downing et al., 2006), this would come to 18,360 kg day–1. At this application rate, it would cost $16,579 USD per day, based on the $0.90 USD per kg price at which we purchased GRF. Such a high application rate and cost likely makes GRF an untenable management strategy in large systems.
Another consideration to the use of GRF is that it must be applied more frequently than most other geoengineering techniques, at least initially. Frequent GRF additions may not be required if the phytoplankton community shifts to an alternative stable state. The alternative stable state hypothesis posits that ecosystems maintain resilience and do not experience state change until a dramatic disturbance shifts the ecosystem to a different state with its own resilience (Scheffer et al., 2001; Carpenter and Brock, 2006). We were able to shift a cyanophyte- dominated phytoplankton community to dominance by cryptophytes in 9 days but we did not continue the experiment long enough to see if an alternate stable state was achieved, nor the time point at which further GRF additions would be unnecessary. In Shidou Reservoir, China, cyanophyta made up ∼100% of the phytoplankton biovolume before an abrupt reduction in the human population within the watershed shifted the phytoplankton community to dominance by chlorophytes (Yang et al., 2017). This shift was sustained for ∼3 years, during which time cyanophytes made up less than 20% of phytoplankton biomass, before a climatic disturbance event shifted the community back to dominance by cyanophytes (Yang et al., 2017). Additional experimentation should investigate long-term trends in the phytoplankton community to determine if GRF application is able to shift the phytoplankton community to an alternative stable state.
The effects of GRF to higher trophic levels will be important when determining whether it should be used in cyanoHAB management. The reduction in light availability from GRF additions could reduce the foraging efficiency of visual predators (Miner and Stein, 1996; Vogel and Beauchamp, 1999). GRF also increases the amount of suspended inorganic particles, which can have a negative impact on fish by damaging gills (Lake and Hinch, 1999) or reducing the filtering efficiency of filter-feeders (Sommaruga, 2015). These effects to biota might not be substantial if short periods of GRF application result in a shift to an alternate stable state. In addition to aquatic biota, the extraction of GRF might impact the terrestrial environment. Commercial GRF is mined from terminal moraines located in the northwestern United States and southwestern Canada, and is sold as a soil amendment for micronutrients. Additional studies should evaluate the long-term effects of GRF addition to higher trophic levels and on the terrestrial environment before its adoption as a widespread management strategy.
We demonstrate that GRF application reduces light availability, resulting in a decline in cyanophytes and replacement by cryptophytes. Our findings provide valuable insights to the nutrient load hypothesis (Brauer et al., 2012), suggesting that cryptophytes might outcompete cyanophytes for light when nutrients are abundant. Further iterations of this hypothesis should consider incorporating functional traits into predictionsof phytoplankton community composition. Before GRF can be advocated as a harmful algal bloom management strategy, further investigation is needed to determine whether an alternative stable state was reached after cryptophytes became the dominant taxa. Regardless, we believe that manipulating light is an important and overlooked strategy for algal bloom management. As the effects of climate change become more pronounced, algal blooms will continue to increase in frequency and magnitude (Paerl and Paul, 2012), and it will become even more important for lake managers to have a variety of techniques to address this challenge.
All datasets generated for this study are available upon request.
RN conceived the idea of using GRF to control cyanobacteria. JG, RN, and TH planned the experiment, which was conducted by JG and TH. Statistical and laboratory analyses were performed by JG, except for phytoplankton and zooplankton counts which were contracted to external labs. JG wrote the manuscript with input from RN and TH. All authors contributed to the article and approved the submitted version.
This research was funded through internal funds from the School of Natural Resources and the Richard Wallace MU Faculty Incentive Grant, University of Missouri, and through the Kansas Water Resource Institute.
The authors declare that the research was conducted in the absence of any commercial or financial relationships that could be construed as a potential conflict of interest.
Thank you to Steven Baker, Amy Burgin, Scott Campbell, Jerry deNoyelles, Emily Kinzinger, Josh Hagerty, Josh Horne, Regina Klepikow, Dan Obrecht, Carol Pollard, Amy Shields, Tony Thorpe, Laura Webb, and Clare Vanderwerken for all of your help in both the field and lab. This project would not have been possible without your hard work, positive attitudes, and generosity with your time. We are grateful. Thank you also to LeeAnn Bennett for graciously enumerating zooplankton, Thomas Anderson for assistance with statistical analyses, and to members of the School of Natural Resources Writing Workshop, whose suggestions greatly improved the quality and clarity of this manuscript.
The Supplementary Material for this article can be found online at: https://www.frontiersin.org/articles/10.3389/fenvs.2020.540607/full#supplementary-material
Allan, J. D. (1976). Life history patterns in zooplankton. Am. Nat. 110, 165–180. doi: 10.1086/283056
APHA (2017). Standard Methods for the Examination of Water and Wastewater, 23rd Edn, eds R. B. Baird, A. D. Eaton, and E. W. Rice (Washington, DC: American Public Health Association).
Backer, L. C., Landsberg, J. H., Miller, M., Keel, K., and Taylor, T. K. (2013). Canine cyanotoxin poisonings in the United States (1920s–2012): review of suspected and confirmed cases from three data sources. Toxins 5, 1597–1628. doi: 10.3390/toxins5091597
Barouillet, C., Cumming, B. F., Laird, K. R., Perrin, C. J., and Selbie, D. T. (2019). Influence of glacial flour on the primary and secondary production of sockeye salmon nursery lakes: a comparative modern and paleolimnological study. Can. J. Fish. Aquat. Sci. 76, 2303–2314. doi: 10.1139/cjfas-2018-0372
Bartson, S., Ogilvie, J., Petroff, A. J., Smith, G. R., and Rettig, J. E. (2018). Effect of pond dye on the response of Southern Leopard Frog tadpoles (Lithobates sphenocephalus) to Western Mosquitofish (Gambusia affinis) cues. Basic Appl. Herpetol. 32, 71–76.
Bellinger, E. G., and Sigee, D. C. (2010). Freshwater Algae: Identification and Use as Bioindicators. Chichester: John Wiley & Sons, Ltd.
Bingham, M., Sinha, S. K., and Lupi, F. (2015). Economic benefits of reducing harmful algal blooms in Lake Erie. Gainesville, FL: Environmental Consulting and Technology Inc.
Blank, K., Haberman, J., Haldna, M., and Laugaste, R. (2009). Effect of winter conditions on spring nutrient concentrations and plankton in a large shallow Lake Peipsi (Estonia/Russia). Aquat. Ecol. 43, 745–753. doi: 10.1007/s10452-009-9283-2
Bloesch, J., and Burns, N. M. (1980). A critical review on sedimentation trap technique. Schweiz. Z. Hydrol. 42, 15–55. doi: 10.1007/bf02502505
Brauer, V. S., Stomp, M., and Huisman, J. (2012). The nutrient-load hypothesis: patterns of resource limitation and community structure driven by competition for nutrients and light. Am. Nat. 179, 721–740. doi: 10.1086/665650
Brett, M. T., Kainz, M. J., Taipale, S. J., and Seshan, H. (2009). Phytoplankton, not allochthonous carbon, sustains herbivorous zooplankton production. Proc. Natl. Acad. Sci. U.S.A. 106, 21197–21201. doi: 10.1073/pnas.0904129106
Bristow, B. T., Summerfelt, R. C., and Clayton, R. D. (1996). Comparative performance of intensively cultured larval walleye in clear, turbid, and colored water. Prog. Fish Cult. 58, 1–10. doi: 10.1577/1548-8640(1996)058<0001:cpoicl>2.3.co;2
Brooks, B. W., Lazorchak, J. M., Howard, M. D. A., Johnson, M. V., Morton, S. L., Perkins, D. A. K., et al. (2016). Are harmful algal blooms becoming the greatest inland water quality threat to public health and aquatic ecosystems? Environ. Toxicol. Chem. 35, 6–13. doi: 10.1002/etc.3220
Burkholder, J. M., and Wetzel, R. G. (1989). Epiphytic microalgae on natural substrata in a hardwater lake: seasonal dynamics of community structure, biomass and ATP content. Arch. Hydrobiol. 83, 1–56.
Campbell, D., Hurry, V., Clarke, A. K., Gustafsson, P., and ?quist, G. (1998). Chlorophyll fluorescence analysis of cyanobacterial photosynthesis and acclimation. Microbiol. Mol. Biol. Rev. 62, 667–683. doi: 10.1128/mmbr.62.3.667-683.1998
Canfield, D. E., Jones, J. R., and Bachmann, R. W. (1982). Sedimentary losses of phosphorus in some natural and artificial Iowa lakes. Hydrobiologia 87, 65–76. doi: 10.1007/bf00016663
Carmichael, W. W., Azevedo, S. M., An, J. S., Molica, R. J., Jochimsen, E. M., Lau, S., et al. (2001). Human fatalities from cyanobacteria: chemical and biological evidence for cyanotoxins. Environ. Health Perspect. 109, 663–668. doi: 10.1289/ehp.01109663
Carpenter, S. R., and Brock, W. A. (2006). Rising variance: a leading indicator of ecological transition. Ecol. Lett. 9, 311–318. doi: 10.1111/j.1461-0248.2005.00877.x
Casassa, G., Lüpez, P., Pouyaud, B., and Escobar, F. (2009). Detection of changes in glacial run-off in alpine basins: examples from North America, the Alps, central Asia and the Andes. Hydrol. Process 23, 31–41. doi: 10.1002/hyp.7194
Chanudet, V., and Filella, M. (2009). Size and composition of inorganic colloids in a peri-alpine, glacial flour-rich lake. Geochim. Cosmochim. Acta 72, 1466–1479. doi: 10.1016/j.gca.2008.01.002
Chapman, A., and Foss, A. (2019). Potentially Toxigenic (PTOX) Cyanobacteria List. Palatka, FL: GreenWater Laboratories.
Chorus, I., and Bartram, J. (1999). Toxic Cyanobacteria in Water: A Guide to their Public Health Consequences, Monitoring and Management. London: E & FN Spon.
Crumpton, W. G., Isehart, T. M., and Mitchell, P. D. (1992). Nitrate and organic N analyses with second derivative spectroscopy. Limnol. Oceanogr. 37, 907–913. doi: 10.4319/lo.1992.37.4.0907
Deacon, C., and Walsby, A. E. (1990). Gas vesicle formation in the dark, and in the light of different irradiances, by the cyanobacterium Microcystis sp. Br. Phycol. J. 25, 133–139. doi: 10.1080/00071619000650121
deNoyelles, F., and Kastens, J. H. (2016). Reservoir sedimentation challenges Kansas. Trans. Kans. Acad. Sci. 119, 69–81. doi: 10.1660/062.119.0110
Dodds, W. K., Bouska, W. W., Eitzmann, J. L., Pilger, T. J., Pitts, K. L., Riley, A. J., et al. (2009). Eutrophication of U.S. freshwaters: analysis of potential economic damages. Envir. Sci. Technol. 43, 12–19. doi: 10.1021/es801217q
Dodson, S. I., and Frey, D. G. (1991). “Cladocera and other Banchiopoda,” in Ecology and Classification of North American Freshwater Invertebrates, eds J. H. Thorp and A. P. Covich (Londong: Academic Press), 723–763.
Downing, J. A., and Plante, C. (1993). Production of fish populations in lakes. Can. J. Fish. Aquat. Sci. 50, 110–120. doi: 10.1139/f93-013
Downing, J. A., Prairie, Y. T., Cole, J. J., Duarte, C. M., Tranvik, L. J., Striegl, R. G., et al. (2006). The global abundance and size distribution of lakes, ponds, and impoundments. Limnol. Oceanogr. 51, 2388–2397. doi: 10.4319/lo.2006.51.5.2388
Epe, T. S., Finsterle, K., and Yasseri, S. (2017). Nine years of phosphorus management with lanthanum modified bentonite (Phoslock in a eutrophic, shallow swimming lake in Germany). Lake Reserv. Manage. 33, 119–129. doi: 10.1080/10402381.2016.1263693
Falkowski, P. G., and LaRoche, J. (1991). Acclimation to spectral irradiance in algae. J. Phycol. 27, 8–14. doi: 10.1111/j.0022-3646.1991.00008.x
Fee, E. J. (1990). Computer programs for calculating in-situ phytoplankton photosynthesis. Can. Tech. Rep. Fish. Aquat. Sci. 1740, 1–27.
Gee, G. W., and Bauder, J. W. (1979). Particle size analysis by hydrometer: a simplified method for routine textural analysis and a sensitivity test of measurement parameters. Soil Sci. Soc. Am. J. 43, 1004–1007. doi: 10.2136/sssaj1979.03615995004300050038x
Gervais, F. (1998). Ecology of cryptophytes coexisting near a freshwater chemocline. Freshw. Biol. 39, 61–78. doi: 10.1046/j.1365-2427.1998.00260.x
Gibbs, M. M., Hickey, C. W., and ?zkundakci, D. (2010). Sustainability assessment and comparison of efficacy of four P-inactivation agents for managing internal phosphorus loads in lakes: sediment incubations. Hydrobiologia 658, 253–275. doi: 10.1007/s10750-010-0477-3
Gilbert, J. J., and Williamson, C. E. (1983). Sexual dimorphism in zooplankton (Copepoda, Cladocera, and Rotifera). Annu. Rev. Ecol. Syst. 14, 1–33. doi: 10.1146/annurev.es.14.110183.000245
Greenfield, D. I., Duquette, A., Goodson, A., Keppler, C. J., Williams, S. H., Brock, L. M., et al. (2014). The effects of three chemical algaecides on cell numbers and toxin content of the cyanobacteria Microcystis aeruginosa and Anabaenopsis sp. Environ. Manage. 54, 1110–1120. doi: 10.1007/s00267-014-0339-2
Grosbois, G., Mariash, H., Schneider, T., and Rautio, M. (2017). Under-ice availability of phytoplankton lipids is key to freshwater zooplankton winter survival. Sci. Rep. 7:11543. doi: 10.1038/s41598-017-10956-0
Grosse, Y., Baan, R., Straif, K., Secretan, B., Ghissassi, F. E., and Cogliano, V. (2006). Carcinogenicity of nitrate, nitrite, and cyanobacterial peptide toxins. Lancet Oncol. 7, 628–629. doi: 10.1016/s1470-2045(06)70789-6
Guildford, S. J., and Hecky, R. E. (2000). Total nitrogen, total phosphorus, and nutrient limitation in lakes and oceans: is there a common relationship? Limnol. Oceanogr. 45, 1213–1223. doi: 10.4319/lo.2000.45.6.1213
Hadas, O., Pinkas, R., Malinsky-Rushansky, N., Shalev-Alon, G., Delphine, E., Berner, T., et al. (2002). Physiological variables determined under laboratory conditions may explain the bloom of Aphanizomenon ovalisporum in Lake Kinneret. Eur. J. Phycol. 37, 259–267. doi: 10.1017/s0967026202003645
Haghseresht, F., Wang, S., and Do, D. (2009). A novel lanthanum-modified bentonite, Phoslock§, for phosphate removal from wastewaters. Appl. Clay Sci. 46, 369–375. doi: 10.1016/j.clay.2009.09.009
Hammer, A. C., and Pitchford, J. W. (2005). The role of mixotrophy in plankton bloom dynamics, and the consequences for productivity. ICES J. Mar. Sci. 5, 833–840. doi: 10.1016/j.icesjms.2005.03.001
Haney, J. F. (1987). Field studies on zooplankton-cyanobacteria interactions. N. Z. J. Mar. Freshw. 21, 467–475. doi: 10.1080/00288330.1987.9516242
Harris, T., Yun, J., Baker, D. S., Kastens, J., Sturm, B., Leavitt, P., et al. (2020). Phytoplankton and Water Quality in Milford Reservoir: Results of Paleolimnological Sediment Core and Historical Data Analyses. Kansas Biological Survey Report No. 197. Lawrence: University of Kansas.
Hecky, R. E., and Guildford, S. J. (1984). Primary productivity of Southern Indian Lake before, during, and after impoundment and Churchill River diversion. Can. J. Fish. Aquat. Sci. 41, 591–604. doi: 10.1139/f84-072
Heisler, J., Glibert, P. M., Burkholder, J. M., Anderson, D. M., Cochlan, W., Dennison, W. C., et al. (2008). Eutrophication and harmful algal blooms: a scientific consensus. Harmful Algae 8, 3–13. doi: 10.1016/j.hal.2008.08.006
Hillebrand, H., Dürselen, C. D., Kirschtel, D., Pollingher, U., and Zohary, T. (1999). Biovolume calculation for pelagic and benthic microalgae. J. Phycol. 35, 403–424. doi: 10.1046/j.1529-8817.1999.3520403.x
Huisman, J., Jonker, R. R., Zonneveld, C., and Weissing, F. J. (1999). Competition for light between phytoplankton species: experimental tests of mechanistic theory. Ecology 80, 211–222. doi: 10.1890/0012-9658(1999)080[0211:cflbps]2.0.co;2
Hylander, S., Jephson, T., Lebret, K., Einem, J., Fagerberg, T., Balseiro, E., et al. (2011). Climate-induced input of turbid glacial meltwater affects vertical distribution and community composition of phyto- and zooplankton. J. Plankton Res. 33, 1239–1248. doi: 10.1093/plankt/fbr025
Ibelings, B. W., Bormans, M., Fastner, J., and Visser, P. M. (2016). CYANOCOST special issue on cyanobacterial blooms: synopsis – a critical review of the management options for their prevention, control and mitigation. Aquat. Ecol. 50, 595–605. doi: 10.1007/s10452-016-9596-x
Isles, P. D. F. (2020). The misuse of ratios in ecological stoichiometry. Ecology. doi: 10.1002/ecy.3153 [Epub ahead of print].
Jones, R. I. (2000). Mixotrophy in planktonic protists: an overview. Freshw. Biol. 45, 219–226. doi: 10.1046/j.1365-2427.2000.00672.x
Jost, C., Lawrence, C. A., Campolongo, F., van de Bund, W., Hill, S., and DeAngelis, D. L. (2004). The effects of mixotrophy on the stability and dynamics of a simple planktonic food web model. Thor. Popul. Biol. 66, 37–51. doi: 10.1016/j.tpb.2004.02.001
Kardinaal, W. E. A., Tonk, L., Janse, I., Hol, S., Slot, P., Huisman, J., et al. (2007). Competition for light between toxic and nontoxic strains of the harmful cyanobacterium Microcystis. Appl. Environ. Microbiol. 73, 2939–2946. doi: 10.1128/aem.02892-06
Kirk, J. T. (1994). Light and Photosynthesis in Aquatic Ecosystems. Oxford: Oxford University Press.
Knowlton, M. F. (1984). Flow-through microcuvette for fluorometric determination of chlorophyll. Water Resour. Bull. 20, 795–799. doi: 10.1111/j.1752-1688.1984.tb04763.x
Kromkamp, J. C., Dijkman, N. A., Peene, J., Simms, S. G. H., and Gons, H. J. (2008). Estimating phytoplankton primary production in Lake Ijsselmeer (The Netherlands) using variable fluorescence (PAM-FRRF) and C-uptake technique. Eur. J. Phycol. 43, 327–344. doi: 10.1080/09670260802080895
Lake, R. G., and Hinch, S. G. (1999). Acute effects of suspended sediment angularity on juvenile coho salmon (Oncorhynchus kisutch). Can. J. Fish. Aquat. Sci. 56, 862–867. doi: 10.1139/f99-024
Laspoumaderes, C., Modenutti, B., Souza, M. S., Navarro, M. B., Cuassolo, F., and Balseiro, E. (2013). Glacier melting and stoichiometric implications for lake community structure: zooplankton species distributions across a natural light gradient. Glob. Change Biol. 19, 316–326. doi: 10.1111/gcb.12040
Lehman, P. W., Marr, K., Boyer, G. L., Acuna, S., and Teh, S. J. (2013). Long-term and causal factors associated with Microcystis abundance and toxicity in San Francisco Estuary and implication for climate change impacts. Hydrobiologia 718, 141–158. doi: 10.1007/s10750-013-1612-8
Lizotte, M. P., and Priscu, J. C. (1992). Photosynthesis–Irradiance relationships in phytoplankton from the physically stable water column of a perennially ice-covered lake (Lake Bonney, Antarctica). J. Phycol. 28, 179–185. doi: 10.1111/j.0022-3646.1992.00179.x
Ludwig, G. R., Perschbacher, P., and Edziyie, R. (2008). The effect of the dye Aquashade§on water quality, phytoplankton, zooplankton, and sunshine bass, Morone chrysops x M-saxatilis, fingerling production in fertilized culture ponds. J. World Aquacult. Soc. 41, 40–48. doi: 10.1111/j.1749-7345.2009.00331.x
Lund, J. W. G., Kipling, C., and LeCren, E. D. (1958). The inverted microscope method of estimating algal numbers and the statistical basis of estimates by counting. Hydrobiologia 11, 143–170. doi: 10.1007/bf00007865
Lürling, M., Eshetu, F., Faassen, E. J., Kosten, S., and Husza, V. L. M. (2013). Comparison of cyanobacterial and green algal growth rates at different temperatures. Freshw. Biol. 58, 552–559. doi: 10.1111/j.1365-2427.2012.02866.x
Lürling, M., and van Oosterhout, F. (2012). Case study on the efficacy of a lanthanum-enriched clay (Phoslock§) in controlling eutrophication in Lake Het Groene Eiland (The Netherlands). Hydrobiologia 710, 253–263. doi: 10.1007/s10750-012-1141-x
MacIntyre, H. L., Kana, T. M., Anning, T., and Geider, R. J. (2002). Photoacclimation of photosynthesis irradiance response curves and photosynthetic pigments in microalgae and cyanobacteria. J. Phycol. 38, 17–38. doi: 10.1046/j.1529-8817.2002.00094.x
Mackay, E. B., Maberly, S. C., Pan, G., Reitzel, K., Bruere, A., Corker, N., et al. (2014). Geoengineering in lakes: welcome attraction or fatal distraction? Inland Waters 4, 349–356. doi: 10.5268/iw-4.4.769
Miller, M. A., Kudela, R. M., Mekebri, A., Crane, D., Oates, S. C., Tinker, M. T., et al. (2010). Evidence for a novel marine harmful algal bloom: cyanotoxin (microcystin) transfer from land to sea otters. PLoS One 5:e12576. doi: 10.1371/journal.pone.0012576
Miner, J. G., and Stein, R. A. (1996). Detection of predators and habitat choice by small bluegills: effect of turbidity and alternative prey. Trans. Am. Fish. Soc. 125, 97–103. doi: 10.1577/1548-8659(1996)125<0097:dopahc>2.3.co;2
Modenutti, B., Pérez, G., Balseiro, E., and Queimalinos, C. (2000). The relationship between light attenuation, chlorophyll a and total suspended solids in a Southern Andes glacial lake. Verh. Int. Ver. Limnol. 27, 1–4. doi: 10.1080/07438141.2011.554962
Moore, R. D., Fleming, S. W., Menounos, B., Wheate, R., Fountain, A., Stahl, K., et al. (2009). Glacier change in western North America: influences on hydrology, geomorphic hazards and water quality. Hydrol. Process. 23, 42–61. doi: 10.1002/hyp.7162
Osgood, R. A. (2017). Inadequacy of best management practices for restoring eutrophic lakes in the United States: guidance for policy and practice. Inland Waters 7, 401–407. doi: 10.1080/20442041.2017.1368881
Overkamp, K. E., Gasper, R., Kock, K., Herrmann, C., Hofmann, E., and Frankenberg-Dinkel, N. (2014). Insights into the biosynthesis and assembly of cryptophycean phycobiliproteins. J. Biol. Chem. 289, 26691–26707. doi: 10.1074/jbc.m114.591131
Paerl, H. W., and Huisman, J. (2009). Climate change: a catalyst for global expansion of harmful cyanobacterial blooms. Environ. Microbiol. Rep. 1, 27–37. doi: 10.1111/j.1758-2229.2008.00004.x
Paerl, H. W., and Paul, V. J. (2012). Climate change: links to global expansion of harmful cyanobacteria. Water Res. 46, 1349–1363. doi: 10.1016/j.watres.2011.08.002
Paerl, H. W., Tucker, J., and Bland, P. T. (1983). Carotenoid enhancement and its role in maintaining blue-green algal (Microcystis aeruginosa) surface blooms. Limnol. Oceanogr. 28, 847–857. doi: 10.4319/lo.1983.28.5.0847
Paes, T. A. S. V., Costa, I. A. S., Silva, A. P. C., and Eskinazi-Sant’Anna, E. M. (2016). Can microcystins affect zooplankton structure community in tropical eutrophic reservoirs? Braz. J. Biol. 76, 450–460. doi: 10.1590/1519-6984.21014
Petty, E. L., Obrecht, D. V., and North, R. L. (2020). Filling in the flyover zone: high phosphorus in midwestern (USA) reservoirs results in high phytoplankton biomass but not high primary productivity. Front. Environ. Sci. 8:111. doi: 10.3389/fenvs.2020.00111
Porter, K. G. (1988). Phagotrophic phytoflagellates in microbial food webs. Hydrobiologia 159, 89–97. doi: 10.1007/bf00007370
R Core Team (2019). R: A Language and Environment for Statistical Computing. Vienna: R Foundation for Statistical Computing.
Rampe, E. B., Horgan, B., Scudder, N., Smith, R. J., and Rutledge, A. M. (2017). Mineralogy of Rock Flour in Glaciated Volcanic Terrains: An Analog for a Cold and Icy Early Mars. NASA Technical Report JSC-CN-38727. Washington, DC: NASA.
Raven, J. A., and Richardson, K. (1984). Dinophyte flagella: a cost-benefit analysis. New Phytol. 98, 259–276. doi: 10.1111/j.1469-8137.1984.tb02736.x
Robarts, R. D., and Zohary, T. (1987). Temperature effects on photosynthetic capacity, respiration, and growth rates of bloom-forming cyanobacteria. N. Z. J. Mar. Freshw. Res. 21, 391–399. doi: 10.1080/00288330.1987.9516235
Rohrlack, T., Dittmann, E., Börner, T., and Christoffersen, K. (2001). Effects of cell-bound microcystins on survival and feeding of Daphnia spp. Appl. Environ. Microbiol. 67, 3523–3529. doi: 10.1128/aem.67.8.3523-3529.2001
Rose, K. C., Hamilton, D. P., Williamson, C. E., McBride, C. G., Fischer, J. M., Olson, M. H., et al. (2014). Light attenuation characteristics of glacially-fed lakes. J. Geophys. Res. Biogeosci. 119, 1446–1457. doi: 10.1002/2014jg002674
Salmaso, N., Naselli-Flores, L., and Padisák, J. (2015). Functional classifications and their applications in phytoplankton ecology. Freshw. Biol. 60, 603–619. doi: 10.1111/fwb.12520
Sarma, S. S. S., Nandini, S., and Gulati, R. D. (2002). Cost of reproduction in selected species of zooplankton (rotifers and cladocerans). Hydrobiologia 481, 89–99.
Sarnelle, O. (1993). Herbivore effects on phytoplankton succession in a eutrophic lake. Ecol. Monogr. 63, 129–149. doi: 10.2307/2937177
Sartory, D. P., and Grobbelaar, J. U. (1986). Extraction of chlorophyll-a from freshwater phytoplankton for spectrophotometric analysis. Hydrobiologia 114, 117–187.
Scheffer, M., Carpenter, S., Foley, J. M., Folke, C., and Walker, B. (2001). Catastrophic shifts in ecosystems. Nature 413, 591–596. doi: 10.1038/35098000
Schwaderer, A. S., Yoshiyama, K., Pinto, P., Swenson, N. G., Klausmeier, C. A., and Litchman, E. (2011). Eco-evolutionary differences in light utilization traits and distributions of freshwater phytoplankton. Limnol. Oceanogr. 56, 589–598. doi: 10.4319/lo.2011.56.2.0589
Sharpley, A., Foy, B., and Withers, P. (2000). Practical and innovative measures for the control of agricultural phosphorus losses to water: an overview. J. Environ. Qual. 29, 1–9. doi: 10.2134/jeq2000.00472425002900010001x
Silsbe, G. M., Hecky, R. E., and Smith, R. E. H. (2012). Improved estimation of carbon fixation rates from active flourometry using spectral fluorescence in light-limited environments. Limnol. Oceanogr. Methods 10, 736–751. doi: 10.4319/lom.2012.10.736
Silsbe, G. M., and Malkin, S. Y. (2015). phytotools: Phytoplankton Production Tools. R package version 1.0. Available online at: https://CRAN.R-project.org/package=phytotools (accessed March 1, 2020).
Sinha, R. P., and Häder, D. P. (2008). UV-protectants in cyanobacteria. Plant Sci. 174, 278–289. doi: 10.1016/j.plantsci.2007.12.004
Slemmons, K. E. H., and Saros, J. E. (2012). Implications of nitrogen-rich glacial meltwater for phytoplankton diversity and productivity in alpine lakes. Limnol. Oceanogr. 57, 1651–1663. doi: 10.4319/lo.2012.57.6.1651
Slemmons, K. E. H., Saros, J. E., and Simon, K. (2013). The influence of glacial meltwater on alpine aquatic ecosystems: a review. Environ. Sci. Proc. Imp. 15, 1794–1806. doi: 10.1039/c3em00243h
Sommaruga, R. (2015). When glaciers and ice sheets melt: consequences for planktonic organisms. J. Plankton Res. 37, 509–518. doi: 10.1093/plankt/fbv027
Sommaruga, R., and Kandolf, G. (2014). Negative consequences of glacial turbidity for the survival of freshwater planktonic heterotrophic flagellates. Sci. Rep. 4:4113. doi: 10.1038/srep04113
Spencer, D. F. (1984). Oxygen consumption by the crayfish Orconectes propinquus (Girard) exposed to Aquashade. Bull. Environ. Contam. Toxicol. 33, 373–378. doi: 10.1007/bf01625557
Stemberger, R. S., and Gilbert, J. J. (1985). Body size, food concentration, and population growth in planktonic rotifers. Ecology 66, 1151–1159. doi: 10.2307/1939167
Stoecker, D. K., Hansen, P. J., Caron, D. A., and Mitra, A. (2017). Mixotrophy in the marine plankton. Annu. Rev. Mar. Sci. 9, 311–335. doi: 10.1146/annurev-marine-010816-060617
Suski, J. G., Swan, C. M., Salice, C. J., and Wahl, C. F. (2018). Effects of pond management on biodiversity patterns and community structure of zooplankton in urban environments. Sci. Total. Environ. 619, 1441–1450. doi: 10.1016/j.scitotenv.2017.11.153
Thorp, J. H., and Covich, A. P. (2001). Ecology and Classification of North American Freshwater Invertebrates. San Diego, CA: Academic Press.
Tittle, J., Bissinger, V., Zipple, B., Gaedke, U., Bell, E., Lorke, A., et al. (2003). Mixotrophs combine resource use to outcompete specialists: implications for aquatic food webs. Proc. Natl. Acad. Sci. U.S.A. 100, 12776–12781. doi: 10.1073/pnas.2130696100
U.S. Environmental Protection Agency (2016). Climate Change Indicators in the United States, 2016, Fourth Edn. Washington, DC: U.S. Environmental Protection Agency.
Urabe, J., Gurung, T. B., Yoshida, T., Sekino, T., and Nakanishi, M. (2000). Diel changes in phagotrophy by Cryptomonas in Lake Biwa. Limnol. Oceanogr. 45, 1558–1563. doi: 10.4319/lo.2000.45.7.1558
Üveges, V., Tapolczai, K., Krienitz, L., and Padisák, J. (2012). Photosynthetic characteristics and physiological plasticity of an Aphanizomenon flos-aquae (Cyanobacteria, Nostocaceae) winter bloom in a dep oligo-mesotrophic lake (Lake Stechlin, Germany). Hydrobiologia 698, 263–272. doi: 10.1007/978-94-007-5790-5_20
Van Halderen, A., Harding, W. R., Wessels, J. C., Schneider, D. J., Heine, E. W. P., Van Der Merwe, J., et al. (1995). Cyanobacterial (blue-green algae) poisoning of livestock in the Western Cape Province of South Africa. J. S. Afr. Vet. Assoc. 66, 260–264.
Van Liere, L., and Mur, L. R. (1980). “Occurrence of Oscillatoria Agardhii and some related species, a survey,” in Hypertrophic Ecosystems: Developments in Hydrobiology, Vol. 2, eds J. Barica and L. R. Mur (Dordrecht: Springer), 66–77.
Visser, P. M., Ibelings, B. W., Bormans, M., and Huisman, J. (2016). Artificial mixing to control cyanobacterial blooms: a review. Aquat. Ecol. 50, 423–441. doi: 10.1007/s10452-015-9537-0
Vogel, J. L., and Beauchamp, D. A. (1999). Effects of light, prey size, and turbidity on reaction distances of lake trout (Salvelinus namaycush) to salmonid prey. Can. J. Fish. Aquat. Sci. 56, 1293–1297. doi: 10.1139/f99-071
Wagner, K. J., Meringolo, D., Mitchell, D. F., Moran, E., and Smith, S. (2017). Aluminum treatments to control internal phosphorus loading in lakes on Cape Cod, Massachusetts. Lake Reserv. Manage. 33, 171–186. doi: 10.1080/10402381.2017.1308449
Wallace, B. B., and Hamilton, D. P. (2000). Simulation of water-bloom formation in the cyanobacterium Microcystis aeruginosa. J. Plankton Res. 22, 1127–1138. doi: 10.1093/plankt/22.6.1127
Webb, W. L., Newton, M., and Starr, D. (1974). Carbon dioxide exchange of Alnus rubra: a mathematical model. Oecologia 17, 281–291. doi: 10.1007/bf00345747
Winslow, L., Read, J., Woolway, R., Brentrup, J., Leach, T., Zward, J., et al. (2017). rLakeAnalyzer: Lake Physics Tools. Available online at: https://cran.r-project.org/package=rLakeAnalyzer (accessed March 1, 2020).
Yang, J. R., Lv, H., Isabwe, A., Liu, L., Yu, X., Chen, H., et al. (2017). Disturbance-induced phytoplankton regime shifts and recovery of cyanobacteria dominance in two subtropical reservoirs. Water Res. 120, 52–63. doi: 10.1016/j.watres.2017.04.062
Yang, S., and Jin, X. (2008). Critical light intensities for Microcystis aeruginosa, Scenedesmus quadricauda and Cytoclotella sp. and competitive growth patterns under different light:N:P ratios. J. Freshw. Ecol. 23, 387–396. doi: 10.1080/02705060.2008.9664215
Zamparas, M., Gianni, A., Stathi, P., Deligiannakis, Y., and Zacharias, I. (2012). Removal of phosphate from natural waters using innovative modified bentonites. Appl. Clay Sci. 62, 101–106. doi: 10.1016/j.clay.2012.04.020
Keywords: algal blooms, climate change, functional traits, geoengineering, mesocosm tanks
Citation: Gaskill JA, Harris TD and North RL (2020) Phytoplankton Community Response to Changes in Light: Can Glacial Rock Flour Be Used to Control Cyanobacterial Blooms? Front. Environ. Sci. 8:540607. doi: 10.3389/fenvs.2020.540607
Received: 05 March 2020; Accepted: 08 September 2020;
Published: 09 October 2020.
Edited by:
Bas Ibelings, Université de Genève, SwitzerlandReviewed by:
Guillaume Grosbois, Swedish University of Agricultural Sciences, SwedenCopyright © 2020 Gaskill, Harris and North. This is an open-access article distributed under the terms of the Creative Commons Attribution License (CC BY). The use, distribution or reproduction in other forums is permitted, provided the original author(s) and the copyright owner(s) are credited and that the original publication in this journal is cited, in accordance with accepted academic practice. No use, distribution or reproduction is permitted which does not comply with these terms.
*Correspondence: Jacob A. Gaskill, amFnY2d6QG1haWwubWlzc291cmkuZWR1
Disclaimer: All claims expressed in this article are solely those of the authors and do not necessarily represent those of their affiliated organizations, or those of the publisher, the editors and the reviewers. Any product that may be evaluated in this article or claim that may be made by its manufacturer is not guaranteed or endorsed by the publisher.
Research integrity at Frontiers
Learn more about the work of our research integrity team to safeguard the quality of each article we publish.