- 1Department of Agriculture and Natural Resources, Delaware State University, Dover, DE, United States
- 2Department of Chemistry, Delaware State University, Dover, DE, United States
- 3College of Chemistry and Materials Science, Sichuan Normal University, Chengdu, China
Recent research suggests that biochar amendment is a promising approach to mitigate soil contamination via immobilizing heavy metals and organic pollutants. Through intensive literature review, this paper was aimed to better understand the processes, mechanisms, and effectiveness of biochar in immobilizing chemical contaminants in soil. The quality characteristics of biochar as a soil amendment varied greatly with the feedstock materials and the pyrolysis conditions. Biochar products from different sources demonstrated remarkably diversified capacities and efficiencies for stabilizing soil contaminants. Soil-incorporated biochar was able to stabilize Cd, Cu, Ni, Pb, and Zn and reduce their bioavailability through enhanced sorption (based on electrostatic attraction, ion exchange, and surface complexation) and chemical precipitation (incurred from soil pH elevation and ash addition of carbonates and phosphates). The stabilization efficacy was largely determined by cation exchange capacity, pH, and ash content of the biochar. Biochar amendment increased the mobility of anionic toxic elements [e.g., , , and ] in soil. Soil-incorporated biochar was also able to adsorb non-polar organic compounds (through pore filling, partition, and hydrophobic effect) and polar organic compounds (via H-bonding, electrostatic attraction, specific interaction, and surface precipitation). The adsorption efficiency was controlled by the biochar surface properties specific surface area, microporosity, and hydrophobicity. Biochar may facilitate the mineralization of organic pollutants by enhancing soil microbial activities. The effectiveness of biochar-facilitated soil remediation was case specific, changing with the biochar source, amendment rate, placement, soil type, and pollutant species. More field studies are needed to evaluate the long-term effectiveness of biochar-facilitated soil remediation under practical circumstances.
Introduction
Biochar is charcoal prepared by pyrolytic processing (i.e., O2-absent heating at 300–700°C) of residual biomass materials and used as a soil amendment in agricultural and environmental applications (Lehmann and Joseph, 2012). The black carbonaceous solid is porous, environmentally recalcitrant, and abundant in surface functional groups (Guo et al., 2016). The relatively high porosity and surface functionality engender biochar with great specific surface area (SSA) and cation exchange capacity (CEC), enabling the material to retain water, nutrients, and pollutants in soil (Ahmad et al., 2014). In addition, biochar contains significant portions of labile organic carbon (OC) and possibly plant nutrients (e.g., N, P, K, Ca, Mg, and S) (Song and Guo, 2012). Intensive laboratory studies and field trials have demonstrated that appropriate biochar amendment is effective to ameliorate soil physical, chemical, and biological properties, increase crop productivity, and reduce the bioavailability of heavy metals and organic contaminants in soil (Chan et al., 2007; Park et al., 2011; Novak et al., 2016; Ghorbani et al., 2019). As a promising soil amendment, biochar has been explored in uses of soil health improvement, pollution alleviation, land reclamation, and climate change mitigation.
Soil contamination by heavy metal(loid)s (e.g., Cd, Cr, Hg, Pb, Cu, Zn, As, Co, Ni, and Se) and persistent organic pollutants [POPs, such as polycyclic aromatic hydrocarbons (PAHs), polychlorinated biphenyls (PCBs), polychlorinated dibenzo-ρ-dioxin (PCDD), and polychlorinated dibenzofurans] is a worldwide problem that threatens environmental sustainability, food safety, and human health (Fabietti et al., 2009; Su et al., 2014; Sun et al., 2018). To control the hazardous effects and ideally restore the ecosystem services of contaminated soils, an array of in situ and ex situ remediation techniques have been developed, including surface capping, encapsulation, landfilling, soil flushing, soil washing, soil venting (air sparging), vacuum ventilation (vapor extraction), thermal extraction, electrokinetic extraction, chemical degradation, stabilization, solidification, vitrification, bioremediation, and phytoremediation (Zhu et al., 2010; Liu L. et al., 2018). These techniques employ physical, chemical, thermal, electrical, and biological methods and processes to contain, immobilize, and ultimately eliminate soil contaminants. In field practice, all the available techniques demonstrate particular advantages and disadvantages of remediation efficiency, cost effectiveness, and applicability (Liu L. et al., 2018).
Recently, biochar amendment has been investigated to alleviate soil contamination and facilitate soil remediation (Chai et al., 2012; Houben et al., 2013; Koltowski et al., 2016; Ippolito et al., 2017). Existing literature reviews suggest that biochar amendment immobilizes heavy metals and POPs in contaminated soils and reduces their bioavailability primarily through precipitation, electrostatic interaction, surface adsorption, structural sequestration, and facilitated decomposition; the decontamination efficacy varies with the biochar source, amendment rate, soil type, and pollutant species (Ahmad et al., 2014; O'Connor et al., 2018; Zama et al., 2018; Kumpiene et al., 2019; Yuan et al., 2019). Most of the reported research trials were in short-term laboratory experiments. The long-term (e.g., >5 years) effects of biochar amendment on contaminant mobility and bioactivity in field soils remain unclear. By comprehensively reviewing the literature, this paper was aimed to further understand the mechanisms and the processes through which biochar amendment mitigates soil contamination, with the ultimate goal to provide instructions of appropriate biochar utilization for effective soil remediation.
Quality Variations of Biochar as a Soil Amendment
Biochar products from different sources vary widely in characteristics and function capacity as a soil amendment. Biochar is produced from biomass materials using the thermochemical technique pyrolysis, through which organic residues are heated in O2-free or highly limited, ambient-pressure environments for a certain time to be carbonized into charcoal, with the generation of pyrolysis bio-oil and syngas as byproducts (Guo et al., 2016). Common biochar feedstocks extend to forest debris, crop residues, food processing waste, and manures including sewage sludge and biosolids (Table 1). These biomass materials are significantly different in organic and ash compositions, attributing to the notable quality variations of the resulting biochar products. The carbonization (pyrolysis) conditions further influence the quality characteristics of biochar. Three parameters are usually used to manipulate the carbonization conditions: pyrolysis (peak) temperature, solid residence time, and heating rate, each stretching over a wide range of values (Guo et al., 2020). The pyrolysis temperature for biochar production is mostly in the range of 300–700°C (Table 1). A higher temperature accelerates the carbonization process, allowing the pyrolytic transformation of biomass to reach a deeper level and be completed in a shorter time (Song and Guo, 2012; Chen et al., 2017). Complete pyrolysis is critically important to transform all feedstock OC into carbonized, pyrogenic OC (i.e., altered, amorphous C structure). The solid residence time for achieving complete pyrolysis is determined by the pyrolysis temperature and the heating rate, ranging from seconds to days. The heating rate may be as low as <1°C s−1 and as high as >200°C s−1, varying with the pyrolysis temperature, feed characteristics (particle size, moisture content, and density), and feed mass flow (Guo et al., 2020). Biochar products from incomplete pyrolysis also contain noticeable portions of uncarbonized C (i.e., with crystalline character of the precursor materials) (Chun et al., 2004; Keiluweit et al., 2010).
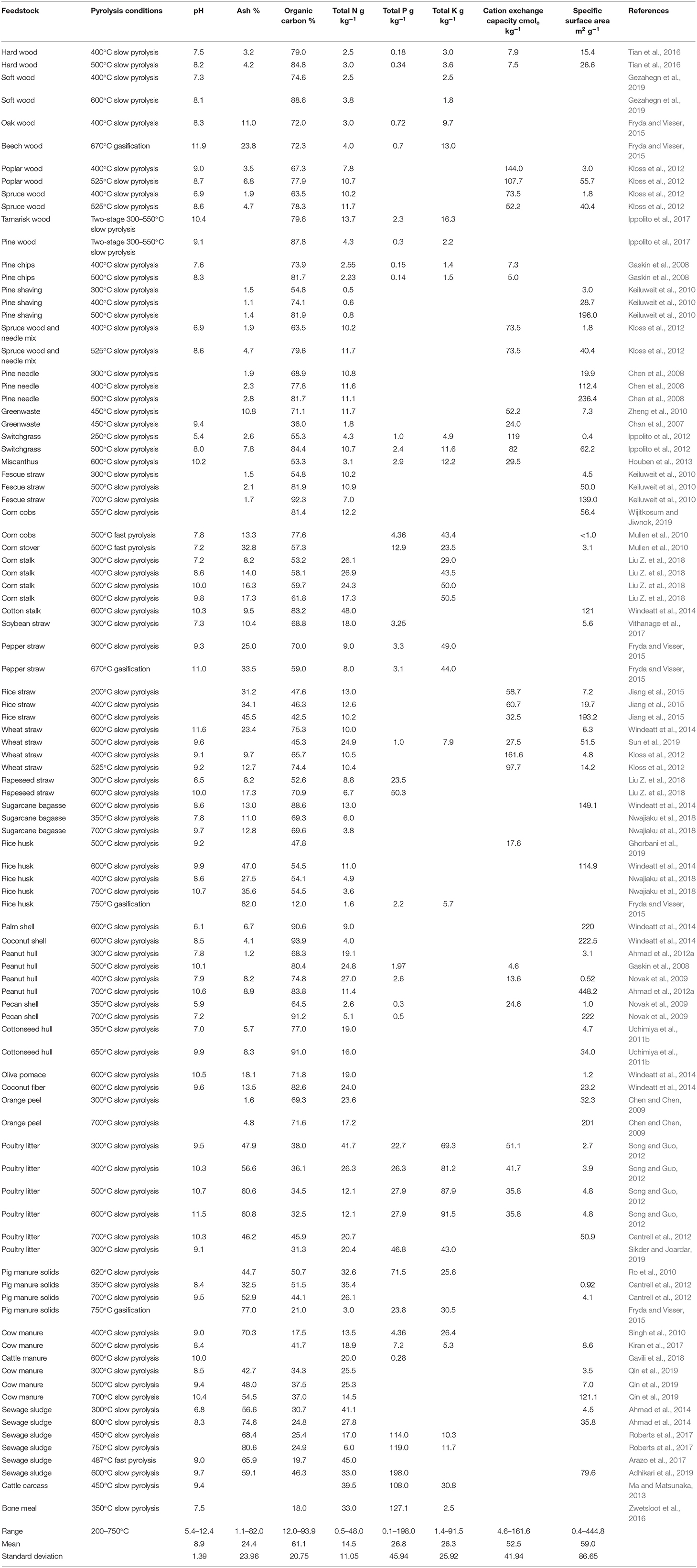
Table 1. Quality characteristics of biochar as influenced by feedstock and carbonization conditions.
Biochar is porous, showing a rough morphological surface with honeycomb-like anatomical or other irregular structures under the microscope (Figure 1). The inherent micropores (diameter in the range of 0.8–235 μm and mostly <22 μm, with average at 10 μm; Hardie et al., 2014) engender the material a relatively high intrapore volume (e.g., 0.9–1 cm3 g−1; Batista et al., 2018) and low envelope density (e.g., 0.2–0.6 g cm−3; Joseph et al., 2019). Biochar is composed primarily of amorphous, aromatic carbon, and possesses abundant O-containing surface functional groups (e.g., –C=O, –COOH, and –OH). A disorderly stacked graphene sheet structure has been proposed for biochar (Figure 1). The aromaticity of biochar generally increases while the surface functionality decreases as the pyrolysis temperature is elevated (Song and Guo, 2012; Zhao et al., 2016). This is largely a result of the progressive losses of aliphatic C–H, olefinic C=C, carbonyl, carboxyl, and hydroxyl groups at a higher pyrolysis temperature (Fu et al., 2012; Tan et al., 2015).
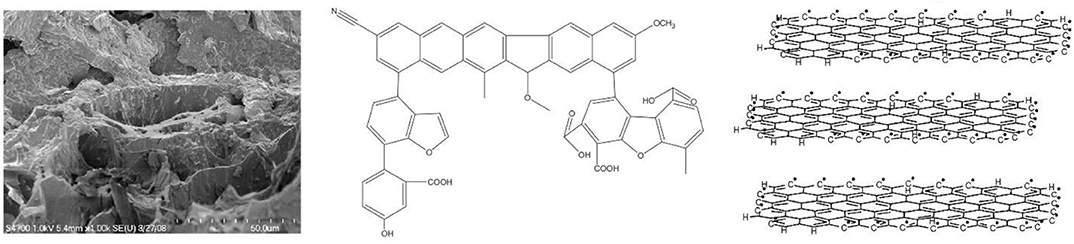
Figure 1. A scanning electron micrograph of corn stover-derived biochar (left), an aromatic-C molecular biochar model (middle), and a disorderly stacked graphene sheet structure proposed for biochar (right) showing the high porosity, aromaticity, and surface functional group abundance of the material (Lehmann and Joseph, 2012; Chen et al., 2017; Zhao et al., 2017).
Biochar is the major product of slow pyrolysis and yet the byproduct of fast pyrolysis (aiming at pyrolysis bio-oil) and gasification (pyrolysis combined with partial oxidation—aiming at syngas). Within each of the three thermochemical techniques, the carbonization conditions (i.e., temperature, solid residence time, and heating rate) can be adjusted to optimize major product generation. Even with the same feedstock, biochars from gasification and fast pyrolysis are evidently lower in OC content and higher in ash content than the products from slow pyrolysis. Overall, biochars from different sources demonstrated a pH value (in 1:5 solid/water extract) ranging from 5.4 to 12.4 and mineral ash content from 1.1 to 82.0% (Table 1). The pH is a comprehensive expression of the water-soluble base cations (e.g., Na+, K+, Ca2+, and Mg2+) and organic acids (e.g., formic acid, acetic acid, hydroxybutyric acid, and benzoic acid) in biochar. The base cations originate from the mineral ash components in the feedstock, whereas the organic acids are generated during biomass pyrolytic transformation. Biochar products from complete pyrolysis contain minimal organic acids and demonstrate a pH value typically >7.5 to reflect the presence of basic ash minerals. In general, the pH of biochar is related to its mineral ash content, with higher-ash-content products showing a higher pH value (Table 1). Biochars from higher-temperature pyrolysis are normally greater in ash content (Figure 2) and pH, and the products derived from manure and rice plant residues are higher in ash content and pH than those derived from wood and other plant residues. The OC content of biochar ranged from 12.0 to 93.9%, generally decreasing as the ash content increased. For low-ash feedstocks (e.g., wood and many other plant residues), the derived biochars showed an increasing trend in OC content as the pyrolysis temperature was elevated, whereas biochars derived from ash-rich feedstocks (e.g., manures, rice plant residues, and bones) illustrated an opposite trend (Table 1). Biochars also contain more or less the plant nutrients N, P, K, and others. The N, P, and K contents of the reported biochars are 0.5–48.0, 0.1–198.0, and 1.4–91.5 g kg−1, respectively, being largely correlated with the products' ash contents. Most of the feedstock P and K would be recovered in biochar, yet the vast majority of feedstock N would be lost to pyrolysis vapors (bio-oil and syngas), especially at high pyrolysis temperatures (e.g., >500°C) (Song and Guo, 2012). The nutrients in biochar are slowly releasable (Wang et al., 2015), supplementing the nutrient supply of amended soils to support plant growth. Sewage sludge- and bone-derived biochars may contain P at >100 g kg−1 and therefore serve as an ideal source of P to crops. Moreover, biochars generated from specialty biomass like municipal organic solids and contaminated-soil-grown plant materials may contain toxic elements at above-threshold concentrations for use as a soil amendment (Buss et al., 2016).
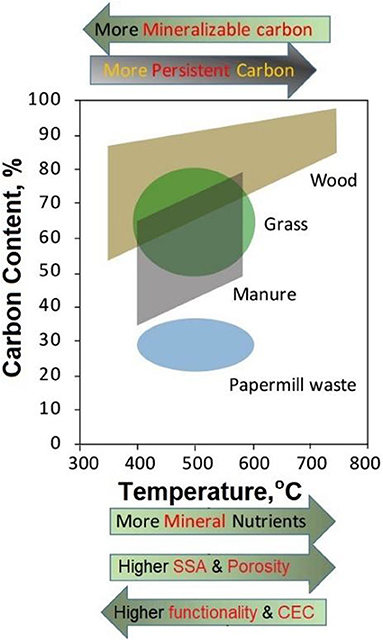
Figure 2. General change trends of biochar's total carbon content, carbon stability, mineral ash content, porosity, specific surface area, abundance of surface functional groups, and cation exchange capacity in response to the feedstock and pyrolysis temperature. The graph was modified from Joseph et al. (2019).
Biochars demonstrate a CEC value of 5–162 cmolc kg−1 and a Brunauer–Emmett–Teller (BET)–SSA value of 0.4–448 m2 g−1 (Table 1). The CEC indicates the capacity of biochar to adsorb cationic nutrients and metal contaminants, while the SSA implicate biochar's ability to retain water, gases, and organic molecules. Research has revealed that biochar is generally sorptive to polar gases (e.g., H2S, NH3, NOx, and volatile organic compounds), water-soluble metal ions (e.g., Cu2+, Pb2+, Zn2+, and Cd2+), and various organic pollutants (e.g., agrochemicals, antibiotics, and PAHs) (Ahmad et al., 2014; Tan et al., 2015). The CEC of biochar is originated primarily from the surface functional groups, while SSA is related to biochar's porosity. A higher pyrolysis temperature in the range of 300–700°C favors micropore development while promoting O-containing functional group elimination, resulting in biochar products with increased SSA yet reduced CEC (Figure 2). Biochars with high ash contents, like those derived from manures, typically demonstrate low SSA values, likely due to ash blockage of the inherent micropores. After applying to soil, the SSA and CEC of biochar may gradually increase over time as a result of natural weathering (Steiner, 2016).
As a soil amendment, biochar has a vital advantage over compost and other raw bio-materials in its high environmental recalcitrance. The mean residence time of biochar in the natural soil environment was estimated at 90–1,600 years (Singh et al., 2012). The environmental stability of biochar originates from its condensed aromatic carbon. Biochars with higher aromaticity and greater C condensation are generally more recalcitrant (Lehmann et al., 2015). Biochar OC can be nominally divided into three fractions: labile, intermediate stable, and stable (Singh et al., 2012). Research suggests that >65% of the OC in biochars with H/OC molar ratio <0.7 would remain in natural soils 100 years after field application (Joseph et al., 2019). Biochars prepared at higher pyrolysis temperatures generally contain a greater proportion of stable OC (Figure 2).
The International Biochar Initiative recommends the following parameters for evaluating the quality of biochar as a soil amendment: pH, lime equivalence, EC, mineral ash content, OC content, SSA, H/OC molar ratio, particle size distribution, germination inhibition assay, total and available plant nutrients, and the presence of inorganic and organic pollutants. The OC content needs to exceed 10% and the H/OC molar ratio surrender 0.7 for a pyrogenic material to be qualified as biochar (IBI, 2015). The European Biochar Certificate (EBC) program suggested similar quality variables for biochar: pH, EC, volatile matter content, total ash content, total carbon (TC) content, SSA, H/OC molar ratio, O/C molar ratio, water content, bulk density, macronutrient (N, P, K, Ca, and Mg) contents, and contents of heavy metals and organic contaminants. A certified biochar product is required to contain ≥50% TC at ≤0.4 O/C and ≤0.7 H/OC molar ratios. The contents of Zn, Pb, Cu, Cr, Ni, Cd, and Hg are capped at 400, 150, 100, 1.5, 90, 50, 1.5, and 1 mg kg−1, respectively, and PAHs, PCBs, and PCDD/Fs at 12, 0.2, and 0.00002 mg kg−1, respectively (EBC, 2012). Considering the mechanisms and the processes through which biochar amendments facilitate soil remediation (as discussed below), biochars selected for soil remediation should be weighted more in pH, lime equivalence, SSA, CEC, and nutrient (especially P) content when the TC content and H/OC molar ratio criteria are met.
Biochar for Remediation of Heavy-Metal-Contaminated Soils
Biochar has been explored for mitigating soil heavy metal contamination. Reported research using laboratory vessel, greenhouse pot, and field plot experiments suggests that biochar is capable of effectively sorbing heavy metal cations from water and immobilizing heavy metal elements in soil and therefore serves as a promising amendment for reducing the ecotoxicity of heavy-metal-contaminated soils (Guo et al., 2010; Ahmad et al., 2014; Tan et al., 2015; O'Connor et al., 2018).
Mechanisms
Unlike soil washing, leaching, and extraction that ultimately remove heavy metals from contaminated soils (Liu L. et al., 2018), biochar amendment does not eradicate but stabilizes heavy metals in soil, transforming the toxic elements into less soluble and less bioaccessible forms. The overall process is rather similar to that of chemical stabilization in which treatment agents such as phosphate- and carbonate-containing chemicals are applied to contaminated soils to react with heavy metal contaminants and convert them into precipitates (Houben et al., 2013; Liu L. et al., 2018). The bioavailability and the ecotoxicity of heavy metals in the treated soils are consequently reduced to below the risky level, restoring the desirable soil function to grow safe crops. In biochar-facilitated soil remediation, selected biochar products are applied at appropriate rates to heavy-metal-polluted sites and thoroughly mixed with the contaminated soil. The applied biochar interacts with heavy metals in the soil, adsorbing heavy metal ions on the pore surfaces and potentially transforming them into hydroxide, carbonate, and phosphate precipitates. As the water-soluble, bioactive fraction of heavy metals in soil decreases, potential uptake and bioaccumulation of heavy metals by soil organisms (including plant roots) are minimized (Ahmad et al., 2014). Since heavy metals are not removed and the immobilization effect may diminish over time, biochar-amended soils need to be regularly monitored for heavy metal toxicity.
Biochar stabilizes cationic heavy metals primarily through sorption and chemical precipitation (Figure 3). Biochar possesses various surface functional groups such as hydroxyls, carbonyls, and carboxyls (Tan et al., 2015). Depending on the pH, dissociation or protonation of these functional groups entails biochar electrical charges. The zeta potential of canola-straw- and peanut-straw-derived biochars became more negative as the solution pH increased between 3.0 and 8.0, indicating a greater negative charge density on the biochar surface at a higher pH (Xu et al., 2011). Biochars derived from corn stover, red oak, cottonseed hull, pecan shell, and pure cellulose through 200–900°C slow pyrolysis showed pHZNC (pH at which biochar has zero net charge) in the range of 3.1–8.5, increasing as the pyrolysis temperature was raised (Uchimiya et al., 2011a; Banik et al., 2018). This corroborates that biochars produced at higher temperatures had a lower CEC value owing to further losses of surface functional groups (Figure 2), as a higher pHZNC indicates fewer negative charges on the surface. At pH < pHZNC, biochar may be positively charged and therefore able to adsorb anionic pollutants such as , , and (Fidel et al., 2018). Both hydrolyzable non-bridging (degraded and negatively charged at high pH) and non-hydrolyzable bridging (positively charged even at high pH) oxonium groups (oxygen heterocycles) were detected in biochars produced at >500°C pyrolysis temperature (Ippolito et al., 2017). The negatively charged surface functional groups enable biochar to adsorb heavy metal cations through electrostatic attraction. Furthermore, the aromatic biochar surface abundant in π electrons could exert electrostatic attraction to electron-deficient metal cations through π-π* donor–acceptor interactions (Vithanage et al., 2017). Cations adsorbed through electrostatic attraction remain in the diffusion layer of biochar particles (i.e., outer-sphere adsorption) and are subject to leaching losses (Tan et al., 2015). Heavy metal ions in the soil solution can be adsorbed to biochar via ion exchange by replacing those cations (e.g., Ca2+, Mg2+, Na+, K+, and H+) originally associated with the biochar surface functional groups (Fidel et al., 2018). This specific interaction (i.e., inner-sphere adsorption) is stronger than electrostatic attraction, yet the adsorbed metal cations are exchangeable, as influenced by the solution pH and ionic strength (Ding et al., 2014; Fidel et al., 2018). A lower pH and a higher solution ionic strength generally reduce the sorption of metal cations onto biochar through ion exchange, owing to the increased competition from other cations. Shim et al. (2015) reported that increasing the solution pH in the range of 3.0–6.0 significantly promoted the removal of Cu2+ by Miscanthus straw-derived biochar from water. Metal cations may also form complexes with the surface functional groups and be strongly sorbed. Using positive matrix factorization analysis, Uchimiya et al. (2011a) validated surface complexation as the major mechanism for biochar sorbing Cd2+, Cu2+, Ni2+, and Pb2+ via the ligand-like surface functional groups (e.g., carboxylic, hydroxyl, and phenolic groups). With the formation of biochar–metal complexes, Na+, K+, Ca2+, Mg2+, and other cations were released into soil, causing soil pH changes (Uchimiya et al., 2011a). Formation of organo-metal complexes on biochar surface and in pores was further evidenced by spectroscopic analyses (Kumar et al., 2018). Uchimiya et al. (2010a) reported that the addition of dissolved organic matter (DOM) substantially reduced the sorption of Cu2+, Cd2+, and Ni2+ by biochars. Natural DOM contains surface ligands capable of complexing metal ions (Weng et al., 2002). The DOM released from biochar may reduce its sorption for heavy metal ions (Oustriere et al., 2016).
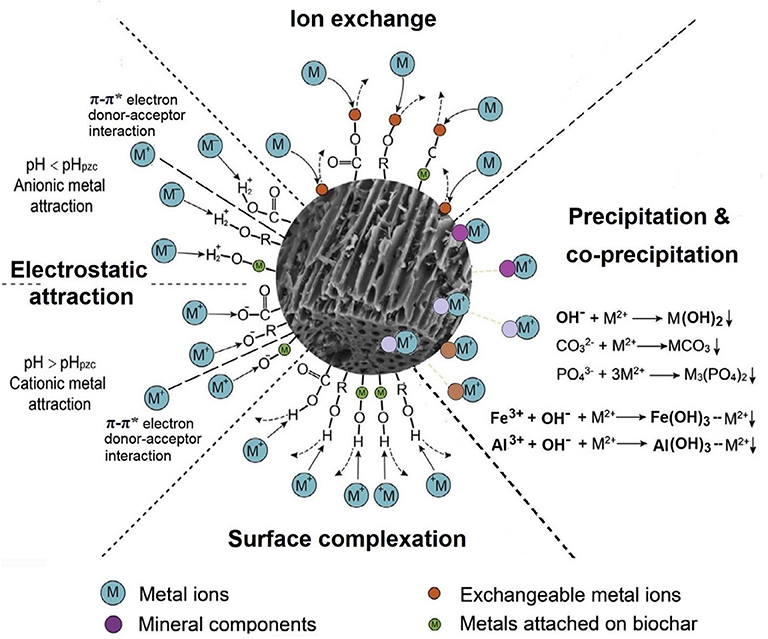
Figure 3. Major mechanisms through which biochar stabilizes heavy metals in contaminated soils. The graph was modified from Tan et al. (2015).
The abundance of surface functional groups as indicated by CEC is the most important trait that determines the capacity of biochar for stabilizing heavy metals through sorption-based interactions. Jiang et al. (2015) noticed that, among the biochars generated from rice straw through 200, 400, 500, and 600°C slow pyrolysis, the 400°C product had the highest CEC (60.65 cmolc kg−1) and demonstrated the greatest sorption capacity for aqueous Cu2+ in batch sorption trials. Activation improves the SSA, aromaticity, and hydrophobicity of biochar but decreases its CEC, surface functional groups, and polarity (Uchimiya et al., 2010a; Shim et al., 2015; Yuan et al., 2019). Steam or CO2 activation of willow-wood-derived biochar, for instance, increased the biochar's SSA from 11.4 to >510 m2 g−1 but enhanced little its capability to reduce the ecotoxicity of a heavy-metal-contaminated soil (Zn, Pb, Cr, Cu, Ni, and Cd) at 5 wt% amendment rate (Kołtowski et al., 2017).
Precipitation is equally important as sorption in biochar stabilizing soil heavy metals. In most cases, biochar amendment elevates soil pH by introducing additional alkalinity (Table 2). Hydrolysis of heavy metals is promoted at higher pH, in which cationic metals react with hydroxyls in water to form metal hydroxide precipitates, reducing the concentration of water-soluble metal ions. Research has identified soil pH elevation as an important mechanism for biochar amendment to mitigate soil heavy metal contamination (Table 2). Ippolito et al. (2017) noticed that addition of wood-based biochars (pH >9.1) at 5–15 wt% elevated the pH levels of mine land soils (pH <5.4) by 0.5–3.5 units and evidently reduced the 0.01 M CaCl2-extractable portions of the inherent Cu, Pb, Zn, and Cd. Other researchers observed similar soil pH elevation and soluble metal decreases when acidic soils were amended with pH 8.8–10.2 biochars (Park et al., 2011; Ahmad et al., 2012b; Houben et al., 2013; Zheng et al., 2015; Cui et al., 2016). In rice paddy soils, the elevated pH favored the formation of Fe/Al hydroxide precipitates; soluble metal ions could be encapsulated by co-precipitation in iron plaques deposited on rice roots (Zheng et al., 2012). Precipitation also occurs when cationic metal ions react with biochar-introduced carbonate, sulfate, and phosphate (Vithanage et al., 2017). Nearly all biochars contain carbonates (e.g., K2CO3, Na2CO3, CaCO3, and MgCO3) that contribute to alkalinity and high pH (Cao et al., 2011; Jiang et al., 2012). Biochars derived from manures and sewage sludge usually contain substantial phosphates (Song and Guo, 2012). Mixing poultry-litter-, dairy-manure-, and sewage-sludge-derived biochars at 2–5 wt% with Pb-contaminated soils resulted in substantial decreases of soil 0.01 M CaCl2-extractable, physiologically based extraction test (PBET)-extractable, and toxicity characteristic leaching procedure (TCLP)-extractable Pb by forming pyromorphite [Pb5(PO4)3Cl], hydroxypyromorphite [Pb5(PO4)3(OH)], and Pb phosphate (Cao et al., 2011; Netherway et al., 2019). Sequential extraction analysis suggested that, in mining soils amended with wood-derived biochar that contained little P, precipitation of Cd2+ was chiefly through carbonate formation, Pb2+ through oxyhydroxide formation, and Cu2+ and Zn2+ through both carbonate and oxyhydroxide formation (Ippolito et al., 2017).
Clearly, biochar amendment facilitates the stabilization of heavy metals in contaminated soils through surface interactions (electrostatic attraction, ion exchange, and surface complexation) and (co-)precipitation (Figure 3). The carboxyl, hydroxyl, and phenolic groups on the biochar surface are particularly effective in binding cationic heavy metal contaminants (Uchimiya et al., 2011a).
Efficacy Variations
The efficacy of biochar amendment on immobilizing soil heavy metals is influenced by an array of factors, including the biochar source, amendment rate, metal species, soil type, and placement in soil (Ahmad et al., 2014; O'Connor et al., 2018). Biochar products manufactured from various biomass feedstocks through different pyrolysis conditions demonstrate great variations in pH, mineral ash content and composition, surface functionality, and CEC (Table 1) and consequently possess varied capability and effectiveness for stabilizing soil heavy metals (Table 2). For example, Ding et al. (2014) reported that the maximum sorption capacity of sugarcane-bagasse-derived biochar for Pb2+ in water (as soluble Pb2+ in soil solution) decreased as the pyrolysis temperature of biochar production increased in the range of 250–600°C. When mixing with contaminated rice paddy soil in field plots at 20 Mg ha−1, rice-straw-derived biochar (pH 10.5; CEC 32.1 cmolc kg−1) reduced soil NH4NO3-extractable and rice-root-accumulated Cd, Pb, and Zn but increased As (arsenic) to significantly higher extents than soybean-straw-derived biochar (pH 9.2; CEC 27.5 cmolc kg−1) prepared under the same carbonization conditions (Zheng et al., 2015); the concentration of As in rice shoot was more than tripled relative to the unamended control (Zheng et al., 2012). At 1, 5, and 10 wt% amendment rates, poultry-litter-based biochar reduced the Pb concentration of Indian mustard roots grown in pH 5.4 Australian soil from 3,243 to 1,276, 513, and 367 mg kg−1, respectively, while greenwaste-based biochar reduced the level to 2,769, 2,299, and 1,196 mg kg−1, respectively (Park et al., 2011), implicating the importance of biochar source and amendment dosage in developing biochar-facilitated soil remediation programs. A higher biochar amendment rate in the practical range (e.g., <5 wt%) typically yields greater results of heavy metal stabilization. Soil characteristics, in particular the clay and OC contents, and co-existing cations also affect the effectiveness of biochar amendment in stabilizing heavy metals. Uchimiya et al. (2011a) reported that addition of plant residues-derived 350°C pyrolysis biochars at 10 wt% significantly enhanced the sorption of loamy sand (pH 5.6; OC 0.6%) for Cu2+ and Pb2+ but not Cd2+ and Ni2+ from a mixed metal solution (each 300 μM), whereas the sorption for Cu2+ of clay soil (clay 50%, pH 9.2, OC 0.89%) from a mixed Cu2+/Cd2+/Ni2+ solution (each 1.5 mM) was noticeably reduced with 10 wt% amendment of poultry-litter-derived 350°C pyrolysis biochar. The researchers believed that biochar products from low-temperature-pyrolysis could not compete with soil clay particles for sorbing Cu2+. In the same tests, replacement with 700°C-pyrolysis poultry litter biochar resulted in evident increases of Cu2+ sorption by the clay soil (Uchimiya et al., 2010a). Biochar amendment affects plant growth. Lu et al. (2014) grew 60-day greenhouse amaranth (Amaranthus tricolor L.) in soil pots (pH 6.0; Cd 6.1 mg kg−1) amended separately with poultry-litter- and wood-based biochars both at 3 wt%. Relative to the control without biochar amendment, the poultry litter biochar amendment tripled the amaranth biomass yield, while the wood biochar amendment decreased such by 50%. Meanwhile, the total amount of amaranth-absorbed Cd reduced from 35.0 to 17.3 and 7.7 mg, respectively. In soils without chemical fertilization, manure-derived biochar furnishes additional nutrients and promotes plant growth, whereas plant-residue-derived biochar may immobilize available N and restrict plant growth (Guo, 2020). Furthermore, the effect of biochar amendment on stabilizing heavy metals may be initially strong but diminishes over time. In a 5-year field plot study, Cui et al. (2016) noticed that the leachability (by TCLP extraction), availability (by 0.01 M CaCl2 extraction), and bioaccessibility (by simplified bioaccessibility extraction test) of Cu and Cd in contaminated paddy soil (pH 4.2) were significantly reduced by 3 wt% wood-based biochar amendment, 1 wt% apatite incorporation, and 0.2 wt% lime addition, yet the effect decreased gradually as time progressed.
In general, soil amendment with biochar at >2.0 wt% helps stabilize cationic heavy metals (e.g., Cd2+, Cu2+, Ni2+, Pb2+, and Zn2+) and reduce the bioaccessible portion and the bioaccumulation of these toxic elements in soil. The efficacy is greater for biochar products possessing higher pH, CEC, and ash content levels, is more evident in acidic, coarse-textured, low OM soils, and becomes diminishing over time (Table 2). Manure-derived biochars are typically more efficient than wood-derived biochars in stabilizing soil heavy metals. Biochar amendment does not stabilize but helps mobilize anionic toxic elements [e.g., Cr in Cr2 and , As in and , and Sb in ] in soil. In biochar application, thorough and uniform incorporation of the amendment into contaminated soil is necessary to allow the biochar to get into direct contact with heavy metal contaminants. In all the reported research studies, biochar in <2 mm particles (mostly <1 mm) was fully mixed with the treated soils to achieve the remediation effect (Table 2). The soil moisture content was controlled at >60% of the soil water holding capacity to secure efficient biochar–heavy metal interactions that only occur in the soil solution phase. In combination with chemical fertilization, biochar amendment normally stimulates plant growth by improving the overall soil health (Guo, 2020). Practically, biochar amendment may be employed to temporarily rectify soils with slight to moderate heavy metal contamination. It may also be used jointly with phytoremediation to facilitate the “cleanup” of Cr (VI)-, As-, and Sb-contaminated soils. Long-term (e.g., >5 years) field research trials are warranted to validate the method feasibility. To date, merely one such study (Cui et al., 2016) was reported.
By reviewing the literature, Ahmad et al. (2014) concluded that biochars with more O-containing functional groups were desirable to immobilize inorganic and polar organic pollutants in soil and water. Accordingly, manure-derived biochar products from low pyrolysis temperature (e.g., <500°C) may be more efficient in mitigating soil heavy metal contamination. Since biochar amendment does not remove heavy metals from soil, the method may only be applicable to slightly to moderately contaminated soils. In practice, appropriate biochar amendment rate and thorough soil incorporation have to be considered, with the remediation effect being regularly monitored.
Biochar for Remediation of Organic-Contaminated Soils
Research has also demonstrated that biochar is capable of retaining and even promoting the decomposition of various organic contaminants in soils (Table 3). Biochar amendment may be a practical approach to mitigate soils polluted by pesticides, herbicides, antibiotics, PAHs, PCBs, petroleum hydrocarbons, and other POPs (Ahmad et al., 2014; Tan et al., 2015; Zama et al., 2018).
Mechanisms
After incorporation into contaminated soil with thorough mixing, biochar promptly interacts with organic contaminants and soil microorganisms. Organic contaminants are stabilized on the biochar surface and in pores and may be further decomposed by microbes as stimulated by biochar amendment. The porous, functional-group-abundant, and aromatic-C-condensed biochar surface is able to adsorb various organic compounds through different mechanisms. As organic pollutants are adsorbed by biochar, their concentrations in soil water are decreased, and bioaccessibility to soil organisms, including plant roots, is reduced. Meanwhile, biochar amendment enhances overall soil health by improving soil physical, chemical, and biological properties (Guo, 2020). In addition to furnishing mineral nutrients such as N, P, K, Ca, Mg, and S, biochar amendment introduces substantial amounts of biodegradable OC as soil microbial substrate. Following the initial perturbation period, biochar-amended soils generally demonstrate improved microbial community structure and promote microbial activity (indicated by soil respiration rate, soil enzyme activity, and soil microbial biomass) (Liao et al., 2016; Irfan et al., 2019). Consequently, the microbial mineralization of many organic pollutants in soil is accelerated.
Biochar is able to stabilize organic contaminants via a number of physical and chemical sorption mechanisms (Figure 4). Biochar interacts with organic molecules through van der Waals forces, the universal electrostatic attraction between non-polar molecules (London dispersion forces) and between polar molecules (dipole-dipole forces) (Petrucci et al., 2007). The van der Waals forces, though fairly weak, increase with the molecules' surface area (e.g., higher molecular weight) and can be dominant as the particle size of biochar decreases (Yang and Evans, 2007). Biochar consists of C, O, H, N, and other mineral elements (Table 1). It may interact with polar organic compounds by forming H bonds through the O-, N-, and H-containing functional groups (Figure 4). H-bonding is another form of electrostatic attraction stronger than van der Waals forces (Tan et al., 2015). The abundant functional groups in biochar become dissociated at pH > pHZNC and protonated at pH < pHZNC, engendering biochar with negative charges and positive charges, respectively (Fidel et al., 2018). The surface charges enable biochar to electrically attract polar organic molecules and ionized organic pollutants with counter charges (e.g., many pesticides, hormones, and antibiotics). This type of electrostatic attraction, as conventionally referred to, is generally stronger than H-bonding and van der Waals interactions. Intensified electrostatic attraction may lead to inner-sphere adsorption (specific surface interaction), in which ionized organic compounds react chemically with the biochar surface functional groups and are retained (Xu et al., 2011). In addition, the aromatic C in biochar possesses excess π-electrons, while many organic molecules, especially those containing O, N, S, P, Cl, and Br, are electron-deficit; biochar may interact with these organic contaminants through π-π* electron donor–acceptor interactions (Xie et al., 2014; Vithanage et al., 2017).
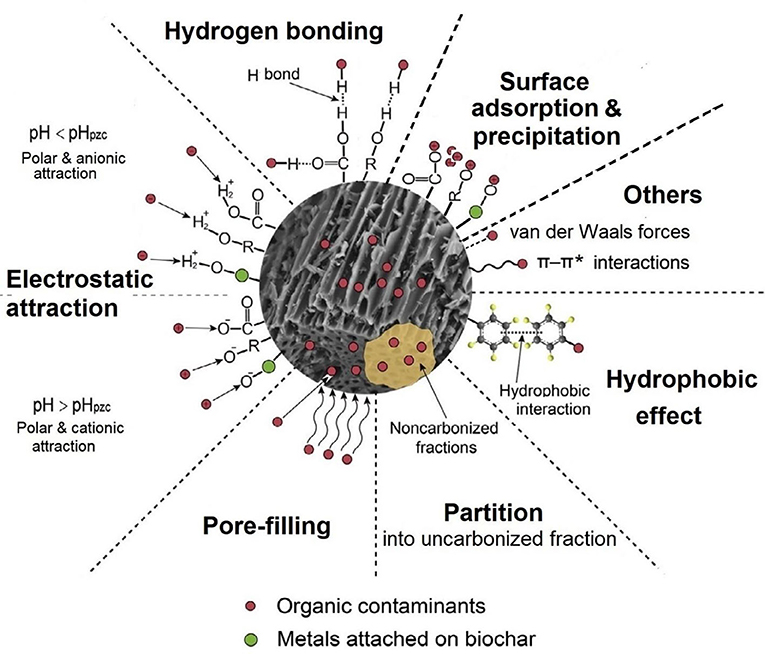
Figure 4. Major mechanisms through which biochar stabilizes organic contaminants in soil. The graph was modified from Tan et al. (2015).
Biochar adsorbs non-ionic organic compounds through surface adsorption and/or partition. Smaller pores have higher surface energy, and therefore sorption of organic pollutants occurs first in biochar micropores (Uchimiya et al., 2010b). At low surface coverage [i.e., equilibrium solute concentration (Ce) to solute water solubility (Sw) ratio ≤0.2], non-linear, competitive adsorption of organic solutes into micropores in biochar's carbonized porous surface is dominant; at higher surface coverage (i.e., Ce/Sw >0.2), the adsorption shifts increasingly to linear, non-competitive partition into biochar's uncarbonized C moiety (Uchimiya et al., 2010b; Chiou et al., 2015; Zhao et al., 2019). Pore filling (“in-pore” surface adsorption) was suggested as an important mechanism for biochar adsorbing organic compounds (Figure 4). Research indicates that the extent of surface adsorption is proportionally related to biochar's SSA and surface porosity as well as aromaticity (Zhu et al., 2014), while the capacity of partition adsorption is dependent on biochar's OC content and mineral ash content (Chiou et al., 2015; Zhao et al., 2019). The hydrophobic effect (avoidance of non-polar molecules and hydrophobic molecular moieties from contacting water) also facilitates the sorption of hydrophobic organic pollutants by biochar (Tan et al., 2015).
In addition, biochar contains more or less multivalent metal elements such as Fe, Al, Ca, and Mg on the surface (Bian et al., 2014). Polar and ionized organic compounds may form complexes with the metal ions and be deposited on the biochar surface or precipitated in soil. The sorption kinetics of many organic molecules on biochar follow the pseudo-second-order pattern, suggesting the importance of “chemisorption” (surface precipitation, Figure 4) in biochar–organic interactions (Tan et al., 2015). Xu et al. (2011) investigated the removal of methyl violet from water by two crop-residue-derived biochars as influenced by the solution pH and ionic strength and concluded that sorption of methyl violet by biochar was mainly through electrostatic attraction, specific interaction, and surface precipitation.
The surface properties of biochar, in particular SSA, pore size, pore volume, polarity, aromaticity, and hydrophobicity, have a predominant effect on biochar–organic compound interactions (Tan et al., 2015). In general, biochars produced at higher pyrolysis temperatures are greater in SSA, aromaticity, and hydrophobicity and lower in surface polarity due to the loss of O- and H-containing functional groups. Jiang et al. (2015) reported that, as the pyrolysis temperature was elevated from 200 to 400, 500, and 600°C, the biochar products from rice straw increased in SSA from 7.2 to 19.7, 44.0, and 193.2 m2 g−1, respectively, and their sorption capacities for cyromazine in water also increased steadily, with the 600°C biochar showing the highest organic sorption capacity. The mineral ash in biochar, especially the manure-derived products, may block the surface micropores and alter the SSA, hydrophobicity, polarity, and other surface properties. Zhang et al. (2013) observed that de-ashing by HCl–HF treatment increased the SSA value of pig-manure-based 350°C (ash 45.3%) and 700°C (ash 66.8%) pyrolysis biochars from 23.8 and 32.6 to 67.1 and 218.1 m2 g−1, respectively, but decreased the CEC level from 96.8 and 112.5 cmolc kg−1 to 5.6 and 8.7 cmolc kg−1, respectively. As a result, the sorption capacities for the pesticides carbaryl and atrazine of pig-manure-based biochars were greatly enhanced, indicating the importance of biochar surface properties (Zhang et al., 2013). Clearly, different biochar products have varied sorption capacities and primary sorption mechanisms for stabilizing soil organic contaminants. Non-polar, hydrophobic organic contaminants such as PAHs and petroleum hydrocarbons are adsorbed by biochar mainly through pore filling, partition, and hydrophobic effect, while polar and ionized organic pollutants, including most pesticides and antibiotics, are adsorbed via H-bonding, electrostatic attraction, specific interaction, and surface precipitation (Figure 4).
Efficacy Variations
The efficiency of biochar amendment to stabilize organic pollutants and facilitate their elimination from soil is case specific, varying with biochar source, particle size, amendment dosage, pollutant chemical nature, and soil type (Table 3). The biochars produced from canola straw, peanut straw char, soybean straw char, and rice hull through the same 350°C pyrolysis processing, for example, demonstrated evidently different sorption capacities for removing methyl violet from water (Xu et al., 2011). Using 30-day incubation experiments, Koltowski et al. (2016) noticed that, at 5 wt% amendment rate, willow-, coconut shell-, and wheat straw-derived biochars reduced the freely dissolved (extractable by POM strips) and bioaccessible (extractable by silicon rods) fractions of PAHs in soils collected from three different industrial sites by 28–87% relative to the unamended controls. Steam activation greatly increased the biochars' SSA and pore volume and subsequently the sorption capacity for PAHs (Koltowski et al., 2016). Biochar itself may contain PAHs (up to 65 mg kg−1; Keiluweit et al., 2012; Quilliam et al., 2013), but mostly at insignificant, low-environmental-risk levels (Li et al., 2016; Weidemann et al., 2018). Amending sandy loam (pH 5.7; OC 0.54%) and clay (pH 5.8; OC 2.4%) soils with sugarcane-residue-derived biochar (pH 8.6; SSA 58.9 m2 g−1; CEC 113.7 cmolc kg−1) at 5 wt% improved the sorption of 17α-ethinylestradiol (EE2, an estrogen hormone) on both soils and reduced their microbial mineralization (Wei et al., 2019). Furthermore, the sandy loam soil demonstrated a remarkably higher sorption efficiency for EE2 than the clay soil; the biochar amendment decreased the adsorption coefficient Koc of the former but increased that of the latter (Wei et al., 2019). When a strongly acidic (pH 3.2) mine spoil soil with mixed heavy metal and phenanthrene contamination was treated with 1 wt% biochar (N 2.1%; SSA 3.5 m2 g−1) generated from stinging nettle (Urtica dioica L.), the phenanthrene degradation in soil microcosms was improved by 44% over a 56-day lab incubation period (Sneath et al., 2013). It was possible that the added biochar mainly functioned to stabilize the heavy metals and did not significantly adsorb phenanthrene. Nevertheless, the biochar amendment did ameliorate soil health and promoted microbial activity, as indicated by the stimulated sunflower (Helianthus annuus) grown in the amended soil pots (Sneath et al., 2013). Qin et al. (2016) reported that the enhanced microbial activity by biochar amendment was responsible for the 20% facilitation in petroleum hydrocarbon biodegradation in a petroleum spill site soil (clay loam; pH 6.5) amended with rice-straw-derived biochar (pH 8.9) at 2 wt% over a 180-day lab incubation period. In addition to absorbing non-polar, hydrophobic organic compounds, biochar also showed high affinity for polar, hydrophilic organic pollutants. Jones et al. (2011) examined the effect of biochar amendment on leaching and biodegradation of the herbicide simazine in different soils. Two particle size fractions of commercial-wood-derived biochar were tested: coarse (2–10 mm) and fine (<2 mm). With addition at 0.5 and 5 wt% to soil, both biochar fractions suppressed the biodegradation of simazine and reduced its leaching in loamy sand (pH 4.8; OC 1.0%) and sandy clay loam (pH 6.2; OC 3.5%) soils, with more significant effects at a higher amendment rate, in finer particle size, and in greater-OC soils (Jones et al., 2011). The particle size of biochar influences the amendment efficacy through affecting the uniformity of biochar distribution in the treated soil. Denyes et al. (2013) noticed that soil incorporation of biochar by mechanical mixing was much more effective than hand or shovel mixing to achieve even distribution of biochar in soil and consequently enhance the retention of PCBs in 2.8 wt% biochar-amended brownfield soil and reduce PCB bioaccumulation in pumpkin (Cucurbita pepo) roots by >60%. Adsorption of pesticides, such as atrazine and simazine, by biochar may reduce the pesticidal efficacy of field-applied agrochemicals. To most surface-sprayed pesticides, the impact should be marginal.
In practice, wood- and plant-residue-derived biochars from higher pyrolysis temperatures and steam-activated biochars usually possess high SSA, porosity, and aromaticity levels and therefore may be selected with preference over manure-derived biochars for sorption-based stabilization of organic contaminants. Over the long term, biochar amendment may facilitate the mineralization and the eventual elimination of organic contaminants in soil through improving soil microbial activities. The mechanisms through which biochar amendment improve soil health and soil microbial activities can be found elsewhere (Paz-Ferreiro et al., 2016; Guo, 2020).
Conclusive Remarks
A notable number of studies have been conducted to investigate the feasibility of using biochar to remediate soils contaminated by heavy metals and various organic pollutants. The literature data indicate that biochar amendment is able to stabilize heavy metals and organic compounds in soil and mitigate soil contamination; the efficacy, however, is case specific, changing with biochar source, amendment dosage, soil type, pollutant species, and even biochar placement in soil. Differently sourced biochars vary significantly in physical and chemical quality properties and demonstrate diverse capacities and efficiencies for stabilizing soil heavy metals and organic contaminants. Biochar amendment does not remove heavy metals from soil; soil-incorporated biochar instead transforms the water-soluble and bioaccessible fractions of heavy metals into immobilized forms, reducing the bioavailability and the ecotoxicity of the toxic elements. This is achieved primarily through elevating the soil pH, introducing carbonates and phosphates, and enhancing surface sorption. Biochar stabilizes cationic heavy metals via electrostatic attraction, ion-exchange-based surface adsorption, surface complexation, and (co-)precipitation. For anionic forms of toxic elements, such as , Cr2, , , and , biochar amendment generally improves their mobility in soil, suggesting that biochar may be used to facilitate phytoextraction-based remediation of Cr (VI)-, As-, and Sb-contaminated soils. The abundance of surface functional groups and the CEC of biochar determine its ability to adsorb heavy metals, while the pH/lime equivalence and the phosphate content control its capacity to precipitate heavy metal contaminants. This remark serves as a guideline for designing and selecting biochar products to mitigate soil heavy metal contamination. In general, manure-derived biochars at lower pyrolysis temperature were more efficient than plant-residue-derived biochars in stabilizing soil heavy metals. Soil-incorporated biochar also stabilizes non-polar and polar organic pollutants through adsorption and reduces their ecotoxicity and bioaccumulation. Biochar amendment may promote the mineralization of organic contaminants in soil by enhancing soil health and microbial activity. Adsorption of biochar for non-polar organic contaminants is chiefly via pore filling, partition, and hydrophobic effect and for polar organic pollutants via hydrogen bonding, electrostatic attraction, specific surface interaction, and surface precipitation (chemisorption). The surface properties of biochar including SSA, microporosity, polarity, and aromaticity influence its capability for adsorbing organic pollutants. Plant-residue-derived biochars at higher pyrolysis temperature mostly outperformed manure-derived biochars in adsorbing organic contaminants. Overall, biochar amendment serves as a promising approach to stabilize soil heavy metals and organic contaminants and mitigate the hazardous soil pollution effects. Though the stabilization efficiency may not be comparable to other agents such as lime, phosphate salts, and activated carbon, biochar is more economically available and can furnish other environmental benefits such as carbon sequestration and soil health improvement when appropriately applied. Biochar amendment may be implemented in slightly polluted cropland to secure safe food production, in mine land restoration efforts to promote vegetation, and in soil bioremediation and phytoextraction projects to facilitate the process. The pollutant stabilization effect of biochar in field soils, however, may diminish over time. Field studies at larger scales are clearly needed to examine the long-term effect of biochar amendment on mitigating soil contamination under practical circumstances.
The application of biochar for improving soil health, facilitating soil remediation, and enhancing carbon sequestration remains currently at the fledgling stage. An emerging biochar industry with profitable commercial production and wide field application is foreseen. The research community has been focusing on validating the agricultural and the environmental benefits of soil–biochar amendment programs and developing biochar quality standards. Though Japan enacted the Soil Productivity Improvement Act in 1987 to sanction the practice of conditioning agricultural soils with charcoal and pot ash (Ogawa and Okimori, 2010), the US and the European countries have not established any governmental policies to regulate biochar use as a soil amendment. Production and application of biochar as a soil amendment may be subject to legislative regulations such as fertilizer policy, soil remediation and protection policy, urban planning policy, and biodiversity policy (van Laer et al., 2015). A sustainable biochar industry warrants systems thinking of feedstock supply security, bio-oil and producer gas standards, and national policies of renewable energy, CO2 emission tax, and carbon sequestration credits (van Laer et al., 2015).
Author Contributions
MG reviewed the literature, synthesized the information, and prepared the manuscript. WS helped with literature data collection and manuscript development and reviewed the manuscript. JT initiated the topic, conducted preliminary experiments and literature review, and examined the draft. All authors contributed to the article and approved the submitted version.
Funding
Financial support for compiling information was partially from the USDA-NIFA Evans-Allen project no. 1017883 and the USDA-NRCS Grant No. NR183A750010C001.
Conflict of Interest
The authors declare that the research was conducted in the absence of any commercial or financial relationships that could be construed as a potential conflict of interest.
References
Adhikari, S., Gascó, G., Méndez, A., Surapaneni, A., Jegatheesan, V., Shah, K., et al. (2019). Influence of pyrolysis parameters on phosphorus fractions of biosolids derived biochar. Sci. Total Environ. 695:133846. doi: 10.1016/j.scitotenv.2019.133846
Ahmad, M., Lee, S. S., Dou, X., Mohan, D., Sung, J. K., Yang, J. E., et al. (2012a). Effects of pyrolysis temperature on soybean stover- and peanut shell-derived biochar properties and TCE adsorption in water. Bioresour. Technol. 118, 536–544. doi: 10.1016/j.biortech.2012.05.042
Ahmad, M., Rajapaksha, A. U., Lim, J. E., Zhang, M., Bolan, N., Mohan, D., et al. (2014). Biochar as a sorbent for contaminant management in soil and water: a review. Chemosphere 99, 19–33. doi: 10.1016/j.chemosphere.2013.10.071
Ahmad, M., Soo Lee, S., Yang, J. E., Ro, H. M., Han Lee, Y., and Sik Ok, Y. (2012b). Effects of soil dilution and amendments (mussel shell, cow bone, and biochar) on Pb availability and phytotoxicity in military shooting range soil. Ecotoxicol. Environ. Saf. 79, 225–231. doi: 10.1016/j.ecoenv.2012.01.003
Arazo, R. O., Genuino, D. A. D., de Luna, M. D. G., and Capareda, S. C. (2017). Bio-oil production from dry sewage sludge by fast pyrolysis in an electrically-heated fluidized bed reactor. Sust. Environ. Res. 27, 7–14. doi: 10.1016/j.serj.2016.11.010
Banik, C., Lawrinenko, M., Bakshi, S., and Laird, D. A. (2018). Impact of pyrolysis temperature and feedstock on surface charge and functional group chemistry of biochars. J. Environ. Qual. 47, 452–461. doi: 10.2134/jeq2017.11.0432
Batista, E. M. C. C., Shultz, J., Matos, T. T. S., Fornari, M. R., Ferreira, T. M., Szpoganicz, B., et al. (2018). Effect of surface and porosity of biochar on water holding capacity aiming indirectly at preservation of the amazon biome. Sci. Rep. 8:10677. doi: 10.1038/s41598-018-28794-z
Beesley, L., Inneh, O. S., Norton, G. J., Moreno-Jimenez, E., Pardo, T., Clemente, R., et al. (2014). Assessing the influence of compost and biochar amendments on the mobility and toxicity of metals and arsenic in a naturally contaminated mine soil. Environ. Pollut. 186, 195–204. doi: 10.1016/j.envpol.2013.11.026
Beesley, L., and Marmiroli, M. (2011). The immobilisation and retention of soluble arsenic, cadmium and zinc by biochar. Environ. Pollut. 159, 474–480. doi: 10.1016/j.envpol.2010.10.016
Beesley, L., Moreno-Jiménez, E., and Gomez-Eyles, J. L. (2010). Effects of biochar and greenwaste compost amendments on mobility, bioavailability and toxicity of inorganic and organic contaminants in a multi-element polluted soil. Environ. Pollut. 158, 2282–2287. doi: 10.1016/j.envpol.2010.02.003
Bian, R., Joseph, S., Cui, L., Pan, G., Li, L., Liu, X., et al. (2014). A three-year experiment confirms continuous immobilization of cadmium and lead in contaminated paddy field with biochar amendment. J. Hazard. Mater. 272, 121–128. doi: 10.1016/j.jhazmat.2014.03.017
Buss, W., Graham, M. C., Shepherd, J. G., and Mašek, O. (2016). Suitability of marginal biomass-derived biochars for soil amendment. Sci. Total Environ. 547, 314–322. doi: 10.1016/j.scitotenv.2015.11.148
Cantrell, K. B., Hunt, P. G., Uchimiya, M., Novak, J. M., and Ro, K. S. (2012). Impact of pyrolysis temperature and manure source on physicochemical characteristics of biochar. Bioresour. Technol. 107, 419–428. doi: 10.1016/j.biortech.2011.11.084
Cao, X., Ma, L., Liang, Y., Gao, B., and Harris, W. (2011). Simultaneous immobilization of lead and atrazine in contaminated soils using dairy-manure biochar. Environ. Sci. Technol. 45, 4884–4889. doi: 10.1021/es103752u
Chai, Y., Currie, R. J., Davis, J. W., Wilken, M., Martin, G. D., Fishman, V. N., et al. (2012). Effectiveness of activated carbon and biochar in reducing the availability of polychlorinated dibenzo-p-dioxins/dibenzofurans in soils. Environ. Sci. Technol. 46, 1035–1043. doi: 10.1021/es2029697
Chan, K. Y., van Zwieten, E. L., Meszaros, I., Downie, A., and Joseph, S. (2007). Agronomic values of greenwaste biochar as a soil amendment. Aust. J. Soil Res. 45, 629–634. doi: 10.1071/SR07109
Chen, B., and Chen, Z. (2009). Sorption of naphthalene and 1-naphthol by biochars of orange peels with different pyrolytic temperatures. Chemosphere 76, 127–133. doi: 10.1016/j.chemosphere.2009.02.004
Chen, B., Zhou, D., and Zhu, L. (2008). Transitional adsorption and partition on nonpolar and polar aromatic contaminants by biochars of pine needles with different pyrolytic temperatures. Environ. Sci. Technol. 42, 5137–5143. doi: 10.1021/es8002684
Chen, D., Guo, H., Li, R., Li, L., Pan, G., Chang, A., et al. (2016). Low uptake affinity cultivars with biochar to tackle Cd-tainted rice - a field study over four rice seasons in Hunan, China. Sci. Total Environ. 541, 1489–1498. doi: 10.1016/j.scitotenv.2015.10.052
Chen, Y., Zhang, X., Chen, W., Yang, H., and Chen, H. (2017). The structure evolution of biochar from biomass pyrolysis and its correlation with gas pollutant adsorption performance. Bioresour. Technol. 246, 101–109. doi: 10.1016/j.biortech.2017.08.138
Chiou, C. T., Cheng, J., Hung, W. N., Chen, B., and Lin, T. F. (2015). Resolution of adsorption and partition components of organic compounds on black carbons. Environ. Sci. Technol. 49, 9116–9123. doi: 10.1021/acs.est.5b01292
Chun, Y., Sheng, G., Chiou, C. T., and Xing, B. (2004). Compositions and sorptive properties of crop residue-derived chars. Environ. Sci. Technol. 38, 4649–4655. doi: 10.1021/es035034w
Cui, H., Fan, Y., Fang, G., Zhang, H., Su, B., and Zhou, J. (2016). Leachability, availability and bioaccessibility of Cu and Cd in a contaminated soil treated with apatite, lime and charcoal: a five-year field experiment. Ecotoxicol. Environ. Saf. 134, 148–155. doi: 10.1016/j.ecoenv.2016.07.005
Cui, L., Li, L., Zhang, A., Pan, G., Bao, D., and Chang, A. (2011). Biochar amendment greatly reduces rice Cd uptake in a contaminated paddy soil: a two-year field experiment. Bioresources 6, 2605–2618. doi: 10.15376/biores.6.3.2605-2618
Denyes, M. J., Rutter, A., and Zeeb, B. A. (2013). In situ application of activated carbon and biochar to PCB-contaminated soil and the effects of mixing regime. Environ. Pollut. 182, 201–208. doi: 10.1016/j.envpol.2013.07.016
Ding, W., Dong, X., Ime, I. M., Gao, B., and Ma, L. Q. (2014). Pyrolytic temperatures impact lead sorption mechanisms by bagasse biochars. Chemosphere 105, 68–74. doi: 10.1016/j.chemosphere.2013.12.042
EBC (2012). European Biochar Certificate—Guidelines for a Sustainable Production of Biochar. European Biochar Foundation (EBC). Available online at: http://www.europeanbiochar.org/en/download (accessed July 30, 2020).
El-Naggar, A., Shaheen, S. M., Ok, Y. S., and Rinklebe, J. (2018). Biochar affects the dissolved and colloidal concentrations of Cd, Cu, Ni, and Zn and their phytoavailability and potential mobility in a mining soil under dynamic redox-conditions. Sci. Total Environ. 624, 1059–1071. doi: 10.1016/j.scitotenv.2017.12.190
Fabietti, G., Biasioli, M., Barberis, R., and Ajmone-Marsan, F. (2009). Soil contamination by organic and inorganic pollutants at the regional scale: the case of Piedmont, Italy. J. Soils Sediments 10, 290–300. doi: 10.1007/s11368-009-0114-9
Fidel, R. B., Laird, D. A., and Spokas, K. A. (2018). Sorption of ammonium and nitrate to biochars is electrostatic and pH-dependent. Sci. Rep. 8:17627. doi: 10.1038/s41598-018-35534-w
Fryda, L., and Visser, R. (2015). Biochar for soil improvement: evaluation of biochar from gasification and slow pyrolysis. Agriculture 5, 1076–1115. doi: 10.3390/agriculture5041076
Fu, P., Hu, S., Xiang, J., Sun, L., Su, S., and An, S. (2012). Study on the gas evolution and char structural change during pyrolysis of cotton stalk. J. Anal. Appl. Pyrolysis 97, 130–136. doi: 10.1016/j.jaap.2012.05.012
Gamiz, B., Lopez-Cabeza, R., Facenda, G., Velarde, P., Hermosin, M. C., Cox, L., et al. (2016). Effect of synthetic clay and biochar addition on dissipation and enantioselectivity of tebuconazole and metalaxyl in an agricultural soil: laboratory and field experiments. Agric. Ecosyst. Environ. 230, 32–41. doi: 10.1016/j.agee.2016.05.017
Gaskin, J. W., Steiner, C., Harris, K., Das, K. C., and Bibens, B. (2008). Effect of low-temperature pyrolysis conditions on biochar for agricultural use. Trans. ASABE 51, 2061–2069. doi: 10.13031/2013.25409
Gavili, E., Moosavi, A. A., and Choghamarani, F. M. (2018). Cattle manure biochar potential for ameliorating soil physical characteristics and spinach response under drought. Arch. Agron. Soil Sci. 64, 1714–1727. doi: 10.1080/03650340.2018.1453925
Gezahegn, S., Sain, M., and Thomas, S. C. (2019). Variation in feedstock wood chemistry strongly influences biochar liming potential. Soil Syst. 3:26. doi: 10.3390/soilsystems3020026
Ghorbani, M., Asadi, H., and Abrishamkesh, S. (2019). Effects of rice husk biochar on selected soil properties and nitrate leaching in loamy sand and clay soil. Int. Soil Water Conserv. Res. 7, 258–265. doi: 10.1016/j.iswcr.2019.05.005
Guo, M. (2020). The 3R principles for applying biochar to improve soil health. Soil Syst. 4:9. doi: 10.3390/soilsystems4010009
Guo, M., He, Z., and Uchimiya, S. M. (2016). “Introduction to biochar as an agricultural and environmental amendment,” in Agricultural and Environmental Applications of Biochar: Advances and Barriers. Vol. 63, eds M. Guo, Z. He, S. M. Uchimiya (Madison, WI: Soil Science Society of America), 1–14.
Guo, M., Qiu, G., and Song, W. (2010). Poultry litter-based activated carbon for removing heavy metal ions in water. Waste Manage. 30, 308–315. doi: 10.1016/j.wasman.2009.08.010
Guo, M., Xiao, P., and Li, H. (2020). “Valorization of agricultural byproducts through conversion to biochar and bio-oil,” in Byproducts from Agriculture and Fisheries: Adding Value for Food, Feed, Pharma and Fuels. eds B. K. Simpson, E. M. Kwofie, A. N. Aryee (Somerset, NJ: John Wiley & Sons, Inc.), 501–522. doi: 10.1002/9781119383956.ch21
Hardie, M., Clothier, B., Bound, S., Oliver, G., and Close, D. (2014). Does biochar influence soil physical properties and soil water availability? Plant Soil 376, 347–361. doi: 10.1007/s11104-013-1980-x
Hartley, W., Dickinson, N. M., Riby, P., and Lepp, N. W. (2009). Arsenic mobility in brownfield soils amended with green waste compost or biochar and planted with Miscanthus. Environ. Pollut. 157, 2654–2662. doi: 10.1016/j.envpol.2009.05.011
Houben, D., Evrard, L., and Sonnet, P. (2013). Beneficial effects of biochar application to contaminated soils on the bioavailability of Cd, Pb and Zn and the biomass production of rapeseed (Brassica napus L.). Biomass Bioenerg. 57, 196–204. doi: 10.1016/j.biombioe.2013.07.019
IBI (2015). Standardized Product Definition and Product Testing Guidelines for Biochar that is Used in Soil–Version 2.1. Westerville, OH: International Biochar Initiative. Available online: http://www.biochar-international.org/characterizationstandard (assessed December 7, 2019).
Ippolito, J. A., Berry, C. M., Strawn, D. G., Novak, J. M., Levine, J., and Harley, A. (2017). Biochars reduce mine land soil bioavailable metals. J. Environ. Qual. 46, 411–419. doi: 10.2134/jeq2016.10.0388
Ippolito, J. A., Novak, J. M., Busscher, W. J., Ahmedna, M., Rehrah, D., and Watts, D. W. (2012). Switchgrass biochar affects two aridisols. J. Environ. Qual. 41, 1123–1130. doi: 10.2134/jeq2011.0100
Irfan, M., Hussain, Q., Khan, K. S., Akmal, M., Ijaz, S. S., Hayat, R., et al. (2019). Response of soil microbial biomass and enzymatic activity to biochar amendment in the organic carbon deficient arid soil: a 2-year field study. Arab. J. Geosci. 12:95. doi: 10.1007/s12517-019-4239-x
Jeong, C. Y., Wang, J. J., Dodla, S. K., Eberhardt, T. L., and Groom, L. (2012). Effect of biochar amendment on tylosin adsorption–desorption and transport in two different soils. J. Environ. Qual. 41, 1185–1192. doi: 10.2134/jeq2011.0166
Jiang, J., Peng, Y., Yuan, M., Hong, Z., Wang, D., Xu, R., et al. (2015). Rice straw-derived biochar properties and functions as Cu(II) and cyromazine sorbents as influenced by pyrolysis temperature. Pedosphere 25, 781–789. doi: 10.1016/S1002-0160(15)30059-X
Jiang, T. Y., Jiang, J., Xu, R. K., and Li, Z. (2012). Adsorption of Pb(II) on variable charge soils amended with rice-straw derived biochar. Chemosphere 89, 249–256. doi: 10.1016/j.chemosphere.2012.04.028
Jiménez, E. M., Aceña-Heras, S., Frišták, V., Heinze, S., and Marschner, B. (2018). The effect of biochar amendments on phenanthrene sorption, desorption and mineralisation in different soils. PeerJ 6:e5074. doi: 10.7717/peerj.5074
Jones, D. L., Jones, G. E., and Murphy, D. V. (2011). Biochar mediated alternations in herbicide breakdown and leaching in soil. Soil Biol. Biochem. 43, 804–813. doi: 10.1016/j.soilbio.2010.12.015
Joseph, S., Taylor, P., Rezende, F., Draper, K., and Cowie, A. (2019). The Properties of Fresh and Aged Biochar. Armidale, NSW: Biochar for Sustainable Soils, Starfish Initiatives. Available online at: https://biochar.international/guides/properties-fresh-aged-biochar/ (accessed November 29, 2019).
Karami, N., Clemente, R., Moreno-Jiménez, E., Lepp, N. W., and Beesley, L. (2011). Efficiency of green waste compost and biochar soil amendments for reducing lead and copper mobility and uptake to ryegrass. J. Hazard. Mater. 191, 41–48. doi: 10.1016/j.jhazmat.2011.04.025
Keiluweit, M., Kleber, M., Sparrow, M. A., Simoneit, B. R., and Prahl, F. G. (2012). Solvent-extractable polycyclic aromatic hydrocarbons in biochar: influence of pyrolysis temperature and feedstock. Environ. Sci. Technol. 46, 9333–9341. doi: 10.1021/es302125k
Keiluweit, M., Nico, P. S., Johnson, M. G., and Kleber, M. (2010). Dynamic molecular structure of plant biomass-derived black carbon (biochar). Environ. Sci. Technol. 44, 1247–1253. doi: 10.1021/es9031419
Khan, S., Chao, C., Waqas, M., Arp, H. P., and Zhu, Y. G. (2013b). Sewage sludge biochar influence upon rice (Oryza sativa L.) yield, metal bioaccumulation and greenhouse gas emissions from acidic paddy soil. Environ. Sci. Technol. 47, 8624–8632. doi: 10.1021/es400554x
Khan, S., Wang, N., Reid, B. J., Freddo, A., and Cai, C. (2013a). Reduced bioaccumulation of PAHs by Lactuca satuva L. grown in contaminated soil amended with sewage sludge and sewage sludge derived biochar. Environ. Pollut. 175, 64–68. doi: 10.1016/j.envpol.2012.12.014
Kiran, Y. K., Barkat, A., Cui, X., Feng, Y., Pan, F., Tang, L., et al. (2017). Cow manure and cow manure-derived biochar application as a soil amendment for reducing cadmium availability and accumulation by Brassicachinensis L. in acidic red soil. J. Integr. Agric. 16, 725–734. doi: 10.1016/S2095-3119(16)61488-0
Kloss, S., Zehetner, F., Dellantonio, A., Hamid, R., Ottner, F., Liedtke, V., et al. (2012). Characterization of slow pyrolysis biochars: effects of feedstocks and pyrolysis temperature on biochar properties. J. Environ. Qual. 41, 990–1000. doi: 10.2134/jeq2011.0070
Kołtowski, M., Charmas, B., Skubiszewska-Zieba, J., and Oleszczuk, P. (2017). Effect of biochar activation by different methods on toxicity of soil contaminated by industrial activity. Ecotoxicol. Environ. Saf. 136, 119–125. doi: 10.1016/j.ecoenv.2016.10.033
Koltowski, M., Hilber, I., Bucheli, T. D., and Oleszczuk, P. (2016). Effect of steam activated biochar application to industrially contaminated soils on bioavailability of polycyclic aromatic hydrocarbons and ecotoxicity of soils. Sci. Total Environ. 566–567, 1023–1031. doi: 10.1016/j.scitotenv.2016.05.114
Kumar, A., Joseph, S., Tsechansky, L., Privat, K., Schreiter, I. J., Schüth, C., et al. (2018). Biochar aging in contaminated soil promotes Zn immobilization due to changes in biochar surface structural and chemical properties. Sci. Total Environ. 626, 953–961. doi: 10.1016/j.scitotenv.2018.01.157
Kumpiene, J., Antelo, J., Brännvall, E., Carabanate, I., Ek, K., Komárek, M., et al. (2019). In situ chemical stabilization of trace element-contaminated soil – field demonstrations and barriers to transition from laboratory to the field – a review. Appl. Geochem. 100, 335–351. doi: 10.1016/j.apgeochem.2018.12.003
Lehmann, J., Czimczik, C., Laird, D., and Sohi, S. (2015). “Stability of biochar in soil,” in Biochar for Environmental Management: Science, Technology and Implementation. 2nd Edn, J. Lehmann, and S. Joseph (London: Routledge), 183–198.
Lehmann, J., and Joseph, S. (2012). “Biochar for environmental management: an introduction,” in Biochar for Environmental Management: Science and Technology. eds J. Lehmann, S. Joseph (London: Earthscan), 183–205. doi: 10.4324/9781849770552
Li, Y., Liao, Y., He, Y., Xia, K., Qiao, S., and Zhang, Q. (2016). Polycyclic aromatic hydrocarbons concentration in straw biochar with different particle size. Procedia Environ. Sci. 31, 91–97. doi: 10.1016/j.proenv.2016.02.012
Liao, N., Li, Q., Zhang, W., Zhou, G., Ma, L., Min, W., et al. (2016). Effects of biochar on soil microbial community composition and activity in drip-irrigated desert soil. Eur. J. Soil Biol. 72, 27–34. doi: 10.1016/j.ejsobi.2015.12.008
Liu, L., Li, W., Song, W., and Guo, M. (2018). Remediation techniques for heavy metal-contaminated soils: principles and applicability. Sci. Total Environ. 633, 206–219. doi: 10.1016/j.scitotenv.2018.03.161
Liu, Z., Niu, W., Chu, H., Zhou, T., and Niu, Z. (2018). Effect of the carbonization temperature on the properties of biochar produced from the pyrolysis of crop residues. BioRes. 13, 3429–3446. doi: 10.15376/biores.13.2.3429-3446
Lu, H., Li, Z., Fu, S., Méndez, A., Gascó, G., and Paz-Ferreiro, J. (2014). Can biochar and phytoextractors be jointly used for cadmium remediation? PLoS ONE 9:e95218. doi: 10.1371/journal.pone.0095218
Ma, Y. L., and Matsunaka, T. (2013). Biochar derived from dairy cattle carcasses as an alternative source of phosphorus and amendment for soil acidity. Soil Sci. Plant Nutri. 59, 628–641. doi: 10.1080/00380768.2013.806205
Mullen, C. A., Boateng, A. A., Goldberg, N. M., Lima, I. M., Laird, D. A., Hicks, K. B., et al. (2010). Bio-oil and bio-char production from corn cobs and stover by fast pyrolysis. Biomass Bioenerg. 34, 67–74. doi: 10.1016/j.biombioe.2009.09.012
Netherway, P., Reichman, S. M., Laidlaw, M., Scheckel, K., Pingitore, N., Gascó, G., et al. (2019). Phosphorus-rich biochars can transform lead in an urban contaminated soil. J. Environ. Qual. 48, 1091–1099. doi: 10.2134/jeq2018.09.0324
Niu, L. Q., Jia, P., Li, S. P., Kuang, J. L., He, X. X., Zhou, W. H., et al. (2015). Slash-and-char: an ancient agricultural technique holds new promise for management of soils contaminated by Cd, Pb and Zn. Environ. Pollut. 205, 333–339. doi: 10.1016/j.envpol.2015.06.017
Novak, J. M., Ippolito, J. A., Lentz, R. D., Spokas, K. A., Bolster, C. H., Trippe, K. M., et al. (2016). Soil health, crop productivity, microbial transport, and mine spoil response to biochars. Bioenerg. Res. 9, 454–464. doi: 10.1007/s12155-016-9720-8
Novak, J. M., Lima, I., Xing, B., Gaskin, J. W., Steiner, C., Das, K. C., et al. (2009). Characterization of designer biochar produced at different temperatures and their effects on loamy sand. Ann. Environ. Sci. 3, 195–206.
Nwajiaku, M., Olanrewaju, J. S., Sato, K., Tokunari, T., Kitano, S., Masunaga, T., et al. (2018). Change in nutrient composition of biochar from rice husk and sugarcane bagasse at varying pyrolytic temperatures. Int. J. Recycl. Org. Waste Agric. 7, 269–276. doi: 10.1007/s40093-018-0213-y
O'Connor, D., Peng, T., Zhang, J., Tsang, D. C. W., Alessi, D. S., Shen, Z., et al. (2018). Biochar application for the remediation of heavy metal polluted land: a review of in situ field trials. Sci. Total Environ. 619–620, 815–826. doi: 10.1016/j.scitotenv.2017.11.132
Ogawa, M., and Okimori, Y. (2010). Pioneering works in biochar research, Japan. Aust. J. Soil Res. 48, 489–500. doi: 10.1071/SR10006
Oustriere, N., Marchand, L., Galland, W., Gabbon, L., Lottier, N., Motelica, M., et al. (2016). Influence of biochars, compost and iron grit, alone and in combination, on copper solubility and phytotoxicity in a Cu-contaminated soil from a wood preservation site. Sci. Total. Environ. 566–567, 816–825. doi: 10.1016/j.scitotenv.2016.05.091
Park, J. H., Choppala, G. K., Bolan, N. S., Chung, J. W., and Cuasavathi, T. (2011). Biochar reduces the bioavailability and phytotoxicity of heavy metals. Plant Soil 348, 439–451. doi: 10.1007/s11104-011-0948-y
Paz-Ferreiro, J., Méndez, A., and Gascó, G. (2016). “Application of biochar for soil biological improvement,” in Agricultural and Environmental Applications of Biochar: Advances and Barriers. Vol. 63, eds M. Guo, Z. He, S. M. (Madison, WI: Uchimiya Soil Science Society of America), 145–174.
Petrucci, R. H., Herring, F. G., Madura, J. D., and Dissonnette, C. (2007). General Chemistry: Principles and Modern Applications. Upper Saddle River, NJ: Prentice Hall.
Qi, F., Dong, Z., Lamb, D., Naidu, R., Bolan, N. S., Ok, Y. S., et al. (2017). Effects of acidic and neutral biochars on properties and cadmium retention of soils. Chemosphere 180, 564–573. doi: 10.1016/j.chemosphere.2017.04.014
Qin, G., Gong, D., and Fan, M. (2016). Bioremediation of petroleum-contaminated soil by biostimulation amended with biochar. Int. Biodeter. Biodegr. 85, 150–155. doi: 10.1016/j.ibiod.2013.07.004
Qin, J., Qian, S., Chen, Q., Chen, L., Yan, L., and Shen, G. (2019). Cow manure-derived biochar: its catalytic properties and influential factors. J. Hazard. Mater. 371, 381–388. doi: 10.1016/j.jhazmat.2019.03.024
Quilliam, R. S., Rangecroft, S., Emmett, B. A., Deluca, T. H., and Jones, D. L. (2013). Is biochar a source or sink for polycyclic aromatic hydrocarbon (PAH) compounds in agricultural soils? GCB Bioenergy 5, 96–103. doi: 10.1111/gcbb.12007
Ro, K. S., Cantrell, K. B., and Hunt, P. G. (2010). High-temperature pyrolysis of blended animal manures for producing renewable energy and value-added biochar. Ind. Eng. Chem Res. 49, 10125–10131. doi: 10.1021/ie101155m
Roberts, D. A., Cole, A. J., Whelan, A., de Nys, R., and Paul, N. A. (2017). Slow pyrolysis enhances the recovery and reuse of phosphorus and reduces metal leaching from biosolids. Waste Manag. 64, 133–139. doi: 10.1016/j.wasman.2017.03.012
Schweiker, C., Wagner, A., Peters, A., Bischoff, W. A., and Kaupenjohann, M. (2014). Biochar reduces zinc and cadmium but not copper and lead leaching on a former sewage field. J. Environ. Qual. 43, 1886–1893. doi: 10.2134/jeq2014.02.0084
Shen, Z., Som, A. M., Wang, F., Jin, F., McMillan, O., and Al-Tabbaa, A. (2016). Long-term impact of biochar on the immobilization of nickel (II) and zinc (II) and the revegetation of a contaminated site. Sci. Total Environ. 542, 771–776. doi: 10.1016/j.scitotenv.2015.10.057
Shim, T., Yoo, J., Ryu, C., Park, Y. K., and Jung, J. (2015). Effect of steam activation of biochar produced from a giant Miscanthus on copper sorption and toxicity. Bioresour. Technol. 197, 85–90. doi: 10.1016/j.biortech.2015.08.055
Sikder, S., and Joardar, J. C. (2019). Biochar production from poultry litter as management approach and effects on plant growth. Int. J. Recycl. Org. Waste Agric. 8, 47–58. doi: 10.1007/s40093-018-0227-5
Singh, B., Singh, B. P., and Cowie, A. L. (2010). Characterisation and evaluation of biochars for their application as a soil amendment. Soil Res. 48, 516–525. doi: 10.1071/SR10058
Singh, B. P., Cowie, A. L., and Smernik, R. J. (2012). Biochar carbon stability in a clayey soil as a function of feedstock and pyrolysis temperature. Environ. Sci. Technol. 46, 11770–11778. doi: 10.1021/es302545b
Sneath, H. E., Hutchings, T. R., and de Leij, F. A. (2013). Assessment of biochar and iron filing amendments for the remediation of a metal, arsenic and phenanthrene co-contaminated soil. Environ. Pollut. 178, 361–366. doi: 10.1016/j.envpol.2013.03.009
Song, W., and Guo, M. (2012). Quality variations of poultry litter biochars generated at different pyrolysis temperatures. J. Anal. Appl. Pyrolysis 94, 138–145. doi: 10.1016/j.jaap.2011.11.018
Steiner, C. (2016). “Considerations in biochar characterization,” in Agricultural and Environmental Applications of Biochar: Advances and Barriers. Vol. 63, eds M. Guo, Z. He, S. M. Uchimiya (Madison, WI: Soil Science Society of America), 87–100.
Su, C., Jiang, L., and Zhang, W. (2014). A review on heavy metal contamination in the soil worldwide: situation, impact and remediation techniques. Environ. Skep. Crit. 3, 24–38.
Sun, H., Zhang, H., Xiao, H., Shi, W., Müller, K., van Zwieten, L., et al. (2019). Wheat straw biochar application increases ammonia volatilization from an urban compacted soil giving a short-term reduction in fertilizer nitrogen use efficiency. J. Soils Sediments 19, 1624–1631. doi: 10.1007/s11368-018-2169-y
Sun, J., Pan, L., Tsang, D. C. W., Zhan, Y., Zhu, L., and Li, X. (2018). Organic contamination and remediation in the agricultural soils of China: a critical review. Sci. Total Environ. 615, 724–740. doi: 10.1016/j.scitotenv.2017.09.271
Tan, X., Liu, Y., Zeng, G., Wang, X., Hu, X., Gu, Y., et al. (2015). Application of biochar for the removal of pollutants from aqueous solutions. Chemosphere 125, 70–85. doi: 10.1016/j.chemosphere.2014.12.058
Tian, J., Miller, V., Chiu, P. C., Maresca, J. A., Guo, M., and Imhoff, P. T. (2016). Nutrient release and ammonium sorption of poultry litter and wood biochars in stormwater treatment. Sci. Total Environ. 553, 596–606. doi: 10.1016/j.scitotenv.2016.02.129
Uchimiya, M., Chang, S., and Klasson, K. T. (2011a). Screening biochars for heavy metal retention in soil: role of oxygen functional groups. J. Hazard. Mater. 190, 432–441. doi: 10.1016/j.jhazmat.2011.03.063
Uchimiya, M., Lima, I. M., Klasson, K. T., and Wartelle, L. H. (2010a). Contaminant immobilization and nutrient release by biochar soil amendment: roles of natural organic matter. Chemosphere 80, 935–940. doi: 10.1016/j.chemosphere.2010.05.020
Uchimiya, M., Wartelle, L. H., Klasson, K. T., Fortier, C. A., and Lima, I. M. (2011b). Influence of pyrolysis temperature on biochar property and function as a heavy metal sorbent in soil. J. Agric. Food Chem. 59, 2501–2510. doi: 10.1021/jf104206c
Uchimiya, M., Wartelle, L. H., Lima, I. M., and Klasson, K. T. (2010b). Sorption of deisopropylatrazine on broiler litter biochars. J. Agric. Food Chem. 58, 12350–12356. doi: 10.1021/jf102152q
van Laer, T., de Smedt, P., Ronsse, F., Ruysschaert, G., Boeckx, P., Verstraete, W., et al. (2015). Legal constraints and opportunities for biochar: a case analysis of EU law. GCB Bioenergy 7, 14–24. doi: 10.1111/gcbb.12114
Vithanage, M., Herath, I., Almaroai, Y. A., Rajapaksha, A. U., Huang, L., Sung, J. K., et al. (2017). Effects of carbon nanotube and biochar on bioavailability of Pb, Cu and Sb in multi-metal contaminated soil. Environ. Geochem. Health 39, 1409–1420. doi: 10.1007/s10653-017-9941-6
Wagner, A., and Kaupenjohann, M. (2015). Biochar addition enhanced growth of Dactylis glomerata L. and immobilized Zn and Cd but mobilized Cu and Pb on a former sewage field soil. Eur. J. Soil Sci. 66, 505–515. doi: 10.1111/ejss.12246
Wagner, A., Kaupenjohann, M., Hu, Y., Kruse, J., and Leinweber, P. (2015). Biochar-induced formation of Zn-P-phases in former sewage field soils studied by P K-edge XANES spectroscopy. J. Plant Nutr. Soil Sci. 178, 582–585. doi: 10.1002/jpln.201400601
Wang, Y., Lin, Y., Chiu, P. C., Imhoff, P. T., and Guo, M. (2015). Phosphorus release behaviors of poultry litter biochar as a soil amendment. Sci. Total Environ. 512–513, 454–463. doi: 10.1016/j.scitotenv.2015.01.093
Wei, Z., Wang, J. J., Hernandez, A. B., Warren, A., Park, J. H., Meng, Y., et al. (2019). Effect of biochar amendment on sorption-desorption and dissipation of 17α-ethinylestradiol in sandy loam and clay soils. Sci. Total Environ. 686, 959–967. doi: 10.1016/j.scitotenv.2019.06.050
Weidemann, E., Buss, W., Edo, M., Mašek, O., and Jansson, S. (2018). Influence of pyrolysis temperature and production unit on formation of selected PAHs, oxy-PAHs, N-PACs, PCDDs, and PCDFs in biochar—a screening study. Environ. Sci. Pollut. Res. 25, 3933–3940. doi: 10.1007/s11356-017-0612-z
Weng, L., Temminghoff, E. J., Lofts, S., Tipping, E., and van Riemsdijk, W. H. (2002). Complexation with dissolved organic matter and solubility control of heavy metals in a sandy soil. Environ. Sci. Technol. 36, 4804–4810. doi: 10.1021/es0200084
Wijitkosum, S., and Jiwnok, P. (2019). Elemental composition of biochar obtained from agricultural waste for soil amendment and carbon sequestration. Appl. Sci. 9:3980. doi: 10.3390/app9193980
Windeatt, J. H., Ross, A. B., Williams, P. T., Forster, P. M., Nahil, M. A., and Singh, S. (2014). Characteristics of biochars from crop residues: potential for carbon sequestration and soil amendment. J. Environ. Manage. 146, 189–197. doi: 10.1016/j.jenvman.2014.08.003
Xie, M., Chen, W., Xu, Z., Zheng, S., and Zhu, D. (2014). Adsorption of sulfonamides to demineralized pine wood biochars prepared under different thermochemical conditions. Environ. Pollut. 186, 187–194. doi: 10.1016/j.envpol.2013.11.022
Xu, R. K., Xiao, S. C., Yuan, J. H., and Zhao, A. Z. (2011). Adsorption of methyl violet from aqueous solutions by the biochars derived from crop residues. Bioresour. Technol. 102, 10293–10298. doi: 10.1016/j.biortech.2011.08.089
Xu, T., Lou, L., Luo, L., Cao, R., Duan, D., and Chen, Y. (2012). Effect of bamboo biochar on pentachlorophenol leachability and bioavailability in agricultural soil. Sci. Total Environ. 414, 727–731. doi: 10.1016/j.scitotenv.2011.11.005
Yang, S., and Evans, J. R. G. (2007). Metering and dispensing of powder; the quest for new solid freeforming techniques. Power Technol. 178, 56–72. doi: 10.1016/j.powtec.2007.04.004
Yu, X. Y., Ying, G. G., and Kookana, R. S. (2009). Reduced plant uptake of pesticides with biochar additions to soil. Chemosphere 76, 665–671. doi: 10.1016/j.chemosphere.2009.04.001
Yuan, P., Wang, J., Pan, Y., Shen, B., and Wu, C. (2019). Review of biochar for the management of contaminated soil: preparation, application and prospect. Sci. Total Environ. 659, 473–490. doi: 10.1016/j.scitotenv.2018.12.400
Zama, E. F., Reid, B. J., Arp, H. P. H., Sun, G., Yuan, H., and Zhu, Y. (2018). Advances in research on the use of biochar in soil for remediation: a review. J. Soils Sediments 18, 2433–2450. doi: 10.1007/s11368-018-2000-9
Zhang, H., Lin, K., Wang, H., and Gan, J. (2010). Effect of Pinus radiate derived biochars on soil sorption and desorption of phenanthrene. Environ. Pollut. 158, 2821–2825. doi: 10.1016/j.envpol.2010.06.025
Zhang, P., Sun, H., Yu, L., and Sun, T. (2013). Adsorption and catalytic hydrolysis of carbaryl and atrazine on pig manure-derived biochars: impact of structural properties of biochars. J. Hazard. Mater. 244, 217–224. doi: 10.1016/j.jhazmat.2012.11.046
Zhang, R., Li, Z., Liu, X., Wang, B., Zhou, G., Huang, X. X., et al. (2017). Immobilization and bioavailability of heavy metals in greenhouse soils amended with rice straw-derived biochar. Ecol. Eng. 98, 183–188. doi: 10.1016/j.ecoleng.2016.10.057
Zhang, Y., Chen, T., Liao, Y., Reid, B. J., Chi, H., Hou, Y., et al. (2016). Modest amendment of sewage sludge biochar to reduce the accumulation of cadmium into rice (Oryza sativa L.): a field study. Environ. Pollut. 216, 819–825. doi: 10.1016/j.envpol.2016.06.053
Zhao, N., Lv, Y., and Yang, X. (2017). A new 3D conceptual structure modeling of biochars by molecular mechanic and molecular dynamic simulation. J. Soils Sediments 17, 641–655. doi: 10.1007/s11368-015-1308-y
Zhao, Y., Feng, D., Zhang, Y., Huang, Y., and Sun, S. (2016). Effect of pyrolysis temperature on char structure and chemical speciation of alkali and alkaline earth metallic species in biochar. Fuel Process. Technol. 141, 54–60. doi: 10.1016/j.fuproc.2015.06.029
Zhao, Z., Wu, Q., Nie, T., and Zhou, W. (2019). Quantitative evaluation of relationships between adsorption and partition of atrazine in biochar-amended soils with biochar characteristics. RSC Adv. 9, 4162–4171. doi: 10.1039/C8RA08544G
Zheng, R., Chen, Z., Cai, C., Tie, B., Liu, X., Reid, B. J., et al. (2015). Mitigating heavy metal accumulation into rice (Oryza sativa L.) using biochar amendment - a field experiment in Hunan, China. Environ. Sci. Pollut. Res. 22, 11097–11108. doi: 10.1007/s11356-015-4268-2
Zheng, R. L., Cai, C., Liang, J. H., Huang, Q., Chen, Z., Huang, Y. Z., et al. (2012). The effects of biochars from rice residue on the formation of iron plaque and the accumulation of Cd, Zn, Pb, As in rice (Oryza sativa L.) seedlings. Chemosphere 89, 856–862. doi: 10.1016/j.chemosphere.2012.05.008
Zheng, W., Guo, M., Chow, T., Bennett, D. N., and Rajagopalan, N. (2010). Sorption properties of greenwaste biochar for two triazine pesticides. J. Hazard. Mater. 181, 121–126. doi: 10.1016/j.jhazmat.2010.04.103
Zhu, L., Lu, L., and Zhang, D. (2010). Mitigation and remediation technologies for organic contaminated soils. Front. Environ. Sci. Eng. China 4, 373–386. doi: 10.1007/s11783-010-0253-7
Zhu, X., Liu, Y., Zhou, C., Luo, G., Zhang, S., Chen, J., et al. (2014). A novel porous carbon derived from hydrothermal carbon for efficient adsorption of tetracycline. Carbon 77, 627–636. doi: 10.1016/j.carbon.2014.05.067
Keywords: biochar, heavy metals, organic contaminants, adsorption, immobilization
Citation: Guo M, Song W and Tian J (2020) Biochar-Facilitated Soil Remediation: Mechanisms and Efficacy Variations. Front. Environ. Sci. 8:521512. doi: 10.3389/fenvs.2020.521512
Received: 19 December 2019; Accepted: 09 September 2020;
Published: 21 October 2020.
Edited by:
Carmen Monterroso, University of Santiago of Compostela, SpainReviewed by:
Achouak Arfaoui, Ecole Supérieure d'Ingénieurs de Medjez El Bab, TunisiaJuan Antelo, University of Santiago de Compostela, Spain
Copyright © 2020 Guo, Song and Tian. This is an open-access article distributed under the terms of the Creative Commons Attribution License (CC BY). The use, distribution or reproduction in other forums is permitted, provided the original author(s) and the copyright owner(s) are credited and that the original publication in this journal is cited, in accordance with accepted academic practice. No use, distribution or reproduction is permitted which does not comply with these terms.
*Correspondence: Mingxin Guo, bWd1byYjeDAwMDQwO2Rlc3UuZWR1