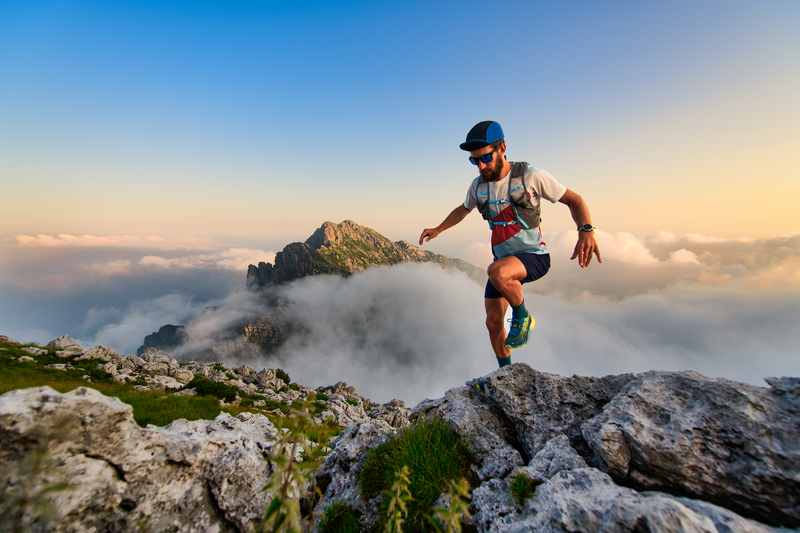
95% of researchers rate our articles as excellent or good
Learn more about the work of our research integrity team to safeguard the quality of each article we publish.
Find out more
ORIGINAL RESEARCH article
Front. Environ. Sci. , 19 August 2020
Sec. Freshwater Science
Volume 8 - 2020 | https://doi.org/10.3389/fenvs.2020.00147
This article is part of the Research Topic Challenges and Innovative Solutions in River Sciences View all 13 articles
Floodplains can perform nutrient buffering functions and therefore influence the riverine nutrient dynamics depending on the extent of the hydrological connectivity. This work focused on quantifying the adsorption/desorption potential of a degraded floodplain of the Danube River (Lower Lobau) based on sediment characterization (grain size distribution, organic content, and P-fractions) and sediment type-specific P-adsorption via batch experiments. We established an adsorption/desorption budget model with a high temporal and spatial resolution. With this model, we identified spatial patterns related to hydrology and calculated the phosphorus-buffering capacity of permanent and temporary floodplain water bodies. Sediment characteristics were defined by the distance to the inflow area and the hydrological connectivity of the floodplain water bodies. The main factor for the concentration of total phosphorus (Ptot) in the sediments was the grain size distribution. Ptot was 10 times higher in silt-dominated sediments compared with gravel-dominated sediments. Inorganic phosphorus (Pinorg) ranged between 36 and 90%, depending on the organic content of the sediments. Both the adsorption and the desorption potential of soluble reactive phosphorus (SRP) were highest in large, frequently connected water bodies and were strongly controlled by hydrology. The total adsorption potential of the floodplain was up to 40 times higher in wet years (e.g., 2002) than in dry years (e.g., 2003), when floodplain water bodies were connected less frequently to the main channel of the Danube. Up to 75% of the adsorbed SRP was desorbed and released into the water column after periods of connection. Consequently, SRP adsorption directly reduced the P-load in the Danube River main channel. The adsorption/desorption mechanisms worked as a buffering system by taking up the SRP imported during floods and releasing it over a longer period after the floods. This stimulated high primary production in the floodplain water bodies and impacted the overall P-retention of the floodplain.
Floodplains are highly productive habitats (Tockner and Stanford, 2002; Mitsch and Gosselink, 2015) and of crucial importance for nutrient dynamics and retention in riverine landscapes (Hoffmann et al., 2009; Noe and Hupp, 2009). Lateral hydrological exchange processes between the main channel and the floodplain water bodies are both sources and drivers for biogeochemical cycling (Amoros and Bornette, 2002; Junk and Wantzen, 2004). These processes do not only control the floodplain water quality, but they also affect downstream riverine and marine ecosystems (Billen et al., 1991). In functionally intact river–floodplain systems, discharge fluctuations determine the hydrological connectivity and the exchange and storage of matter across the floodplain, thereby controlling the net impact of floodplains on the river water quality (Reddy et al., 1999; Van der Lee et al., 2004).
Phosphorus plays a central role in the biogeochemical cycling in floodplains (Wolf et al., 2013) and river systems (Records et al., 2016; Weigelhofer et al., 2018). The availability of soluble reactive phosphorus (SRP) in floodplains is controlled by a variety of internal biotic and abiotic processes, such as, e.g., assimilation by organisms, organic matter mineralization, and adsorption/desorption processes at the sediment/water interface (Noe et al., 2019). However, unlike other standing water bodies, floodplains are also subject to frequent temporally and spatially restricted inputs of river water. These inputs facilitate the exchange of chemically different water sources and the creation of chemical gradients between the water column and the floodplain sediments, thereby stimulating various abiotic and biotic processes, such as, e.g., SRP adsorption and desorption (Froelich, 1988; Borggaard et al., 2005).
Adsorption originates from diverse geochemical and physical processes, which lead to the fast and usually reversible phosphorus fixation to sediment particles (REF). Adsorption reactions can buffer water column SRP concentrations and increase the overall SRP availability in floodplain water bodies (Mainstone and Parr, 2002; James and Barko, 2004). The adsorption or desorption potential can be determined by analyzing the equilibrium phosphate concentration (EPC0) (House and Denison, 2000; Dunne et al., 2005; Yoo et al., 2006). This EPC0 represents the SRP concentration in the water column where no adsorption or desorption takes place. Under conditions with higher SRP concentrations in the overlying water column than the EPC0, the sediments have the potential to take up SRP from the water column. If the SRP concentration is below the EPC0, the sediments can act as a source of phosphate (Taylor and Kunishi, 1971; House and Denison, 2000; Jarvie et al., 2006). Many factors, which determine the adsorption capacity of the sediments, may change during floods, such as, e.g., the particle size, the concentration of amorphous or less crystalline iron and aluminum oxides, the pH, and the oxygen content (Withers and Jarvie, 2008; Records et al., 2016). Thus, hydrological connectivity is a key factor for SRP adsorption processes in floodplain water bodies.
In addition to abiotic processes, phosphorus can also be bound in the sediments in organic form due to assimilation by autotrophic and heterotrophic microbes or accumulation of dead plant material from macrophytes or riparian vegetation (Fisher and Acreman, 2004; Watson et al., 2019). Part of the organic bound P is released back to the overlying water column as SRP through microbial mineralization processes (House, 2003; Ahlgren, 2006; Kleeberg et al., 2008; Palmer-Felgate et al., 2011). Like P adsorption, P assimilation and mineralization are influenced by environmental factors, such as levels of dissolved oxygen and temperature.
However, river engineering measures have reduced the hydrological connectivity of floodplains worldwide (Tockner et al., 2010). Along the Upper Danube, up to 90% of the former floodplain areas have disappeared due to river regulation, large-scale land-use changes, and terrestrialization processes (Tockner et al., 2009; Hein et al., 2016). Initially, the floodplain Lower Lobau was part of the braided Danube River system, where transport processes dominated river–floodplain interactions. In contrast, storage and internal processes were limited to peripheral zones. Nowadays, due to large-scale regulations of the Danube River, the Lower Lobau presents a floodplain forest with water bodies of varying degrees of hydrological connectivity, which are connected via a downstream opening in the flood protection dike (Preiner et al., 2018). The decoupling of the floodplain and the main channel changed floodplain processes fundamentally, as internal processes gained in importance. With hydrological connectivity as the main driver of the SRP availability in the floodplain of the Lower Lobau (Weigelhofer et al., 2015), phosphorus adsorption/desorption mechanisms may play an important role in the P retention and floodplain primary production.
The main objective of the present study was to analyze the impact of the hydrological connectivity on adsorption/desorption patterns and the buffering capacity of the floodplain Lower Lobau in a high spatial and temporal resolution. We measured standing stocks of five different phosphorus fractions to quantify phosphorus allocation in the floodplain water bodies (n = 162) and relate them to hydrological connectivity and other abiotic conditions of the water bodies. We classified the sediments and determined the EPC0 of the different sediment types. We set up a segment based model with a high spatial resolution, which combined the EPC0 with estimated daily water SRP concentrations in the floodplain water bodies for the period 1999–2019. The model was used to identify hydrologically driven patterns of the adsorption/desorption potential and the SRP buffering capacity of the floodplain.
The Lower Lobau is a floodplain of the Danube River, located on the left river bank at the eastern border of Vienna (river kilometer 1908–1918) (Funk et al., 2013). It is composed of an approximately 10 km long main side-arm, which is subdivided by check dams and natural fords into a chain of connected basins, accompanied by isolated pools and temporary water bodies (Figure 1). The floodplain is connected to the main channel of the Danube River via back-flooding through a 10-m wide opening of the flood protection levee at the downstream end of the floodplain. With increasing water levels in the main channel of the river, large parts of the main side-arm and larger areas of the floodplain become connected and inundated. Groundwater tables are low due to drinking water abstraction for the supply of the city of Vienna. Groundwater input to surface water bodies is, therefore, negligible, and the inundation of the floodplain is instead seen as groundwater recharge (Danielopol et al., 1997). At Vienna, the Danube is a ninth order river, characterized by an alpine regime with highly variable patterns. For the study, we defined the hydrological connectivity of the floodplain water bodies as the average number of days per year, where a surface water connection with the main channel of the Danube is established. At the mean discharge of 1,930 m3s–1, which correlates to the mean water level of 142.41 m a.s.l. (MW, Austrian River Authority, gauge at Wildungsmauer), the floodplain is disconnected from the main channel. A surface water connection of the floodplain water bodies with the main river channel is established at water levels above 143.02 m a.s.l. This level was exceeded on 75 days per year on average in the period 1999–2019. Therefore, the hydrological connectivity of the various floodplain water bodies ranged from 75 days per year in the most downstream parts of the main side-arm adjacent to the inflow to 9 days per year in the upstream regions of the main side-arm. Isolated ponds were connected less than 2 days per year on average at water levels above 146 m a.s.l. We used the water body types defined by Weigelhofer et al. (2015) for the Lower Lobau (LFW-large frequently connected water bodies, LRW-large rarely connected water bodies, SW-small, isolated water bodies) in this study and added three more types FL, TF, and TR. While LFW, LRW, and SW are all back-flooded, FL (flowing) water bodies are characterized by a unidirectional flow from upstream to downstream during surface water connection (connection level 142.7 m a.s.l., exceeded on 106 days per year). The types TF (temporary, frequently connected) and TR (temporary, rarely connected) represent temporary water bodies with different connectivity. We defined TF or TR as areas adjacent to FL and the main side channel (LFW, LRW), which are inundated only during floods (TF: connection level 146 m a.s.l., exceeded on 1.6 days per year; TR, connection level 146.5 m a.s.l., exceeded on 0.8 days per year on average in the period from 1999 to 2019). TF and TR are only wetted as long as the water level of the main channel is higher than the connection level.
Figure 1. Study area Lower Lobau (area: 1,480 ha), sediment and water column sampling sites, and sites where adsorption/desorption experiments were done are shown. Arrows indicate inflow/outflow areas of the floodplain.
Monitoring data of the Lower Lobau between 2005 and 2014, provided by the Municipal Authorities of Vienna (MA45), were integrated into the present study.
Limnochemical data of water samples were collected from 2005 to 2014. Sampling was performed four times per year between 2005 and 2008 and in monthly intervals between 2009 and 2014. Additional sampling with a higher frequency was done during three floods in 2009, 2012, and 2013 at nine sites (n = 63–87 per site; Weigelhofer et al., 2015). Sediments were sampled between 2008 and 2010 in the main side-arm, in isolated water bodies, and also in temporary water bodies (total n = 142) to cover the full gradient of hydrological connectivity within the floodplain (Figure 1).
Sediment samples were taken following cross-sections from a boat or, in shallow water, from the ground with a Gilson-Corer (65 mm diameter). The upper 5-cm layers of three samples per spot were mixed and used for the analysis. The depth of the fine sediment layer was measured with a U-profiled bar. In the lab, grain size distribution (silt < 0.2 mm, sand 0.2–2 mm, gravel > 2 mm) was determined by sieving the dried sediments (70°C, until constant weight) followed by combustion (450°C, 4 h) to define the organic content (OC) of each size class. Before drying, we took subsamples to analyze nutrient concentrations of the sediments. We determined four phosphorus fractions (triplicates per sample)—total phosphorus (Ptot), inorganic phosphorus (Pinorg, HCL extraction), loosely sorbed phosphorus (Psol, NH4Cl extraction), and reductive soluble phosphorus (Pred, dithionite extraction)—according to Ruban et al. (2001). Organic phosphorus (Porg) was calculated as the difference between Ptot and Pinorg.
Previous studies carried out in the Donau-Auen National Park (Welti et al., 2012) showed a strong relation of grain-size distribution and OC of the sediments with processes like denitrification and respiration. For that reason, we categorized the sediments based on the predominating grain-size class in “gravel-dominated,” “sand-dominated,” “silt-dominated,” and a category with more or less equal shares of the size classes (“mixed”). The categories were then split up into two subcategories based on their OC (below or above the average OC of each group (Table 1).
Table 1. Categorization of sediments into eight types according to their prevailing grain-size and their organic content (OC).
We determined EPC0 for the different sediment types in the laboratory via batch equilibrium experiments (Froelich, 1988; House, 2003; Jarvie et al., 2005). The analysis was done in triplicates, and the average values were used for further calculations. Ten grams of fresh sediments were incubated in 50-ml deionized water enriched with KH2PO4 (0, 0.1, 0.3, 0.5, 1, 5 10, 25, 50, 75, and 100 mg P L–1). Sediment samples were shaken at 20°C in the dark for 24 h. After the incubation, the extract was centrifuged (3,000 rpm, 15 min). SRP concentrations of the supernatant (Peq) were analyzed spectrophotometrically with a continuous flow analyzer (CFA, Systea Analytical Technology) following the molybdenum blue method (APHA, 1998). The amount of adsorbed SRP per gram sediment was calculated as
with Psorb as adsorbed SRP (μg g DW–1), Pini and Peq as initial and equilibrium SRP concentrations in the water (μg L–1), respectively, V as the volume of the solution (L), and wsed as dry weight of the sediments (g).
We plotted Psorb against Peq and fitted a two-component Langmuir isotherm (Barrow, 1978) using a least-squares method (SPSS version 18.0):
where Cmax1 and Cmax2 were the adsorption maxima of the two Langmuir components, and Kd1 and Kd2 were adsorption parameters. The maximum P adsorption capacity was calculated as the sum of Cmax1 and Cmax2 (Manning et al., 2006).
The EPC0 was calculated as the x-intercept of these adsorption curves.
After removing the supernatant of the adsorption experiment, 25 ml of deionized water was added to the sediment pellets (soil:solution ratio of 1:100) to determine the desorption of the freshly adsorbed SRP (Lair et al., 2009). The tubes were shaken for 1 h and centrifuged. The SRP concentrations (Pdesorp) of the supernatants were analyzed, as described earlier. The desorption procedure was repeated three times. The percentages of desorbed SRP were averaged, multiplied by 24 to obtain a desorption potential per day, and related to Psorb to estimate the sediment-specific potential desorption rates as a percentage of the freshly adsorbed SRP per day.
We applied segmentation to the whole floodplain area (number of segments: 326) based on hydro-morphological differences and different macrophyte coverage. Each segment was assigned a sediment category and a water body type to calculate and upscale the adsorption/desorption potential. We set up a model for 20 years (1999 to 2019) to quantify the influence of hydrology on the adsorption/desorption potential of the floodplain.
Hydromorphological data like depth, water area, volume, and flow velocity were computed by a steady-state hydrodynamic surface water model (Baart et al., 2010). We divided the water bodies of the Lower Lobau, which had defined hydrological connectivity, roughly into three depth categories (0–0.5 m, 0.5–1.5 m, and >1.5 m) and used macrophyte coverage to refine it into 326 segments (ArcGIS 10.4, ESRI). Macrophyte coverage was gained from aerial images (Federal Office of Metrology and Surveying [BEV]) by manually encircling macrophyte-covered areas divided in floating leaf plants, emerged and submerged macrophytes. We cross-checked with samples taken in the area (unpublished report, Systema GmbH). If different life forms were located within the same depth category of a water body, we separated them into different segments. If areas with high coverage and low coverage were in the same depth class of a water body, we also separated them into different segments. Macrophyte coverage of a segment was calculated as the sum of areas covered by each life form divided by the total area of the segment.
The segments, therefore, represented subareas of permanent or temporary water bodies, which were different from the adjacent segments in their hydro-geomorphology or the coverage of macrophytes. The segments were defined as areal base units for the calculations of the standing stocks of different phosphorus fractions and the upscaling of the adsorption/desorption potential (Supporting Information, Supplementary Figure 1). The median area of the segments was 6,184 m2 with a range from 248 to 42,759 m2.
Sediment characteristics of each segment were defined by the characteristics of the samples taken within a segment or of the closest sampling sites within the same water body. If two or more sediment sampling sites were located within the same segment, we checked the variability of grain size distribution, OC, and P-fractions and either used average values or refined the segments in the case of high variability. Sediment categories (based on the dominant grain-size and the OC) were assigned to each segment to allow the application of adsorption/desorption patterns (with EPC0 curves) and to upscale it to the water body and floodplain scale. Water bodies were composed of 1–30 segments depending on their size. Although sediment types of segments were different within a water body, the limnological conditions were assumed to be constant for water bodies because they were mostly driven by surface water connection with the main channel. SRP concentrations in the Danube main channel usually are higher than in the water bodies of the Lower Lobau. The nutrient availability in the main side-arm of the Lower Lobau is strongly determined by the hydrological situation (Weigelhofer et al., 2015). We defined SRP concentrations for the six different water body types and two different hydrological conditions: “flood,” which referred to a state of surface water connection with the main channel of the Danube, and “no-flood,” based on monitoring data from 2005 to 2014 (Municipal Authorities of Vienna, MA 45) and described in detail in Weigelhofer et al. (2015). For every waterbody type, we defined connectivity levels by analyzing water levels measured in the river main channel and within different parts of the floodplain (water level data 2008–2011, Municipal Authorities of Vienna, MA45). We calculated average SRP concentrations by water body (and therefore by segment) and hydrological situation (Table 2). This enabled us to link the main channel water level (Austrian River Authority, gauge at Wildungsmauer) directly with SRP concentrations in the floodplain water bodies for the period 1999 to 2019.
Table 2. Connectivity levels (cl), average hydrological connectivity, and SRP concentrations of water bodies (wb) with different hydrological connectivity compared with Danube River concentrations (D) for two hydrological situations—flood and no-flood—as a basis for upscaling.
Finally, each segment had assigned (i) a sediment category, which determined sediment characteristics, the standing stock of sediment phosphorus fractions, and the potential for SRP adsorption or desorption (based on EPC0), and (ii) a water body type, which defined the SRP concentrations in the water column for each hydrological condition: flooded and not connected to the main channel of the river. We assumed homogeneous conditions of water and sediment within the segments.
We calculated the adsorption potential according to a modified version of Lair et al. (2009) for the period 1999 to 2019.
where SRP was the SRP concentration in the overlying water column, EPC0 was the determined phosphate equilibrium concentration, and Cmax1, Cmax2, Kd1, and Kd were adsorption parameters. fSed was a factor to convert the potential from mg kg–1 to g m–2. We assumed that the upper 5 cm of the sediments was involved in adsorption/desorption processes and used bulk densities for silt-, sand-, and gravel-dominated sediments, according to Smith and Wheatcraft (1993) (Table 3).
Adsorption took place when the SRP concentration in the water was higher than EPC0, which usually was during periods of connection. After surface water connection periods (flood conditions), the adsorbed SRP was the basis for the estimation of desorption processes. Based on the sediment-specific potential desorption rates, we calculated stepwise desorption from day-to-day. It also accounted for changes in the desorption potential as a response to decreasing adsorbed SRP amounts.
We performed multivariate adaptive regression splines (MARS, R-package earth, Milborrow et al., 2019) using the techniques from Friedman (1991) to analyze how general parameters of floodplain hydrology, sediment characteristics, and macrophyte cover affected the distribution of phosphorus fractions in the sediment. We used the hydrology of the water bodies (permanent or temporary, hydrological connectivity), the relative share of different sediment grain size classes (silt: <0.2 mm, sand: 0.2–2 mm, and gravel: >2 mm), the OC of the sediments, and the macrophyte coverage as basic data. MARS is a form of non-parametric regression analysis, which can be seen as a generalization of stepwise linear regression that integrates non-linearities and interactions between variables automatically (Hastie et al., 2017). MARS creates subsets of terms describing all included variables (forward pass). The following backward pass then reduces the number of terms by using generalized cross-validation that allows to compare the performance of different model subsets and choose the best performing model with the lowest residual sum-of-squares (RSS). The predictive power of the selected models was calculated analogously to R2 as (1-RSS)/TSS, with TSS as total sum-of-squares. The used R-package conducts basic checks for collinear terms (Milborrow et al., 2019) and performs well even when collinearity is present (Dormann et al., 2013).
The hydrological connectivity to the main channel drove sediment characteristics in the Lower Lobau. The amount of imported suspended solids increased with increasing connectivity of the water bodies. The fine sediment layer was significantly higher in water bodies near the inflow than in more distant areas of the floodplain, with a maximum depth of up to 270 cm. With increasing distance to the inflow, the OC of the sediments increased, whereas grain sizes generally decreased. Sand-dominated and mixed sediments characterized the parts of the main side-arm close to the inflow area, the adjacent flooding areas by silt-dominated sediments with low OC (Figure 2). In the middle and upstream parts of the side-arm (LFW, LRW), silt-dominated or mixed sediments with low or high organic matter content were found. Silt-dominated sediments characterized isolated waterbodies. In water bodies with flowing conditions (FL), sediments were dominated by gravel and sand with low OC.
Phosphorus concentrations in the sediments strongly depended on the predominating sediment type of a water body. The highest levels of Ptot were found in silt-dominated sediments (529 ± 202 mg kg–1) and the lowest in gravel-dominated sediments (46 ± 31 mg kg–1) (Table 3). The organic phosphorus fraction was between 7% in sediments with low organic matter content and 52% in sediments with high organic matter content. The smaller the average sediment grain size was, the higher were the concentrations of reductive soluble phosphorus (Pred). The amounts of Pred ranged between 3.0 ± 3.1 mg kg–1 in gravel-dominated sediments and 74 ± 69 mg kg–1 in silt-dominated sediments. The maximum relative share of Pred was 13% in silt-dominated sediments with high OC. Psol was considerably lower in sediments with low OC than in sediments with high OC with a maximum relative share of 1.1%.
In summary, Ptot in water bodies with prevailing flowing conditions was mainly composed of Pinorg, showing low concentrations of Pred and Psol (Figure 3). Ptot was higher in the other water body types and also showed a higher variability there. The lower the connectivity was, the lower was the fraction of Pinorg and the higher was the fraction of Pred. Psol was low in all water bodies.
Figure 3. Distribution of P-fractions in different water body types - FL, LW (including LFW and LRW), SW, and temporary water bodies (TF and TR).
The predictive power of the MARS models for the different phosphorus fractions was between 0.68 and 0.8 (Table 4). Explaining variables were the relative shares of the various sediment grain-sizes, the hydrological connectivity, macrophyte coverage, and the permanent or temporary character of a water body. The highest effect of connectivity was found for the distribution of Psol.
Table 4. Results of multilinear regression for Ptot, Pinorg, Pred, and Psol for the water body types MH, LW (LFW and LRW), and SW with the significant determining factors.
EPC0 was highest for the silt-dominated sediments (8.5 μg L−1) and lowest for gravel-dominated sediments (3.2 μg L–1) and mixed sediments with low OC (2.1 μg L–1) (Table 5).
Depending on the location of the water bodies and the predominant sediment type, the adsorption/desorption potential was calculated for flood and no-flood situations. Surface water connection with the main river channel was usually established for short periods. During this period, nutrients were imported into the floodplain, whereby the SRP concentrations decreased with distance to the inflow. At sites where the level of SRP in the water was higher than EPC0 of the particular area, sediments had the potential to act as a sink for SRP (Figure 4). The adsorption potential increased with increasing differences between the SRP concentrations and the EPC0. Therefore, the phosphorus adsorption potential was higher in the downstream parts of the floodplain than in the upstream parts during connection with the main river channel. Isolated water bodies were characterized by SRP concentrations exceeding the EPC0 during disconnection.
Figure 4. Maximum SRP adsorption potential in different floodplain water bodies under flooding conditions (in g m–2 d–1).
bd, bulk density; Cmax and Kd are adsorption parameters of the fitted two-compound Langmuir curve. Desorption as a percentage of adsorbed P per day.
After periods of connection, SRP concentrations in the water column rapidly decreased (Supporting Information, Supplementary Figure 2). At water SRP concentrations below the EPC0, phosphate was potentially desorbed from the sediments to the water column, and the sediments changed from a sink to a source for SRP.
We analyzed water level characteristics of the Danube main channel for the period from 1999 to 2019, summarizing the days of flooding conditions and the number of floods for the studied water body types, and simulated adsorption/desorption patterns (Figure 5). FL was connected during 106 ± 50 days per year on average in the period from 1999 to 2019 (Table 2). In FL, flood conditions were associated with flow velocities higher than 0.5 m s–1 and low hydrological retention. Therefore, water SRP concentrations in FL were similar to the concentrations in the Danube during connection. Besides, the higher flow velocities resulted in the occurrence of gravel-dominated or mixed sediments, with low adsorption and desorption potential (Table 5). LFW was connected to the main channel at 76 ± 42 days per year. The connection increased the water levels of LFW, resulting in flow velocities lower than 0.05 m s–1 in most parts of the water bodies and inputs of SRP from the river. The sediments were mainly silt-dominated and showed a high potential for SRP adsorption (Table 5).
Figure 5. Riverine water level and corresponding adsorption/desorption potential of all floodplain water bodies from 1999 to 2019. Water level of the main channel of the Danube; dashed line—mean water level (MW2010, KWD 2010). Number of floods per year is indicated (gray boxes). Deviation from average water level calculated as the difference between the average water level per year and the mean water level of the Danube (MW 2010, KWD 2010). Adsorption/desorption potential of the whole floodplain per year; labels indicate net adsorption (gray) or release (orange) of SRP are shown in boxes.
The adsorption/desorption dynamics in LRW were similar to the ones in LFW, but the average hydrological connectivity was only 10 ± 9 days per year. Therefore, adsorption phases were short and less intense because of SRP concentration and, consequently, the difference between SRP and EPC0, were much lower than in LFW (Table 2). In SW, P dynamics were generally different from the other water bodies. Water SRP concentrations were higher than in the other parts of the floodplain and the main channel of the Danube during disconnection (Table 2), which theoretically would facilitate a high potential for SRP adsorption. However, as the SRP concentrations remained high in these water bodies during disconnection, we assume that other SRP release mechanisms, like reductive phosphate release, superimposed the adsorption/desorption processes. Connection during floods resulted in a decrease of SRP concentrations due to dilution.
The hydrological connection of a water body with the Danube main channel and the import of nutrients resulted in a general increase in the SRP adsorption potential of the sediments, outbalancing the desorption potential. It resulted in an annual positive adsorption/desorption balance. Only in the year 2003, which was characterized by low water levels, the P-desorption potential was higher than the adsorption potential, and the annual balance was negative. The highest annual net adsorption of the entire floodplain, with an average water surface area of 137.5 ha, was calculated for the exceptionally wet year 2002 (3,274 kg SRP per year). A net release of SRP of the whole floodplain area was only found for the dry year 2003 (−344 kg SRP per year) (Figure 5). The average adsorption/desorption potential for the period from 1999 to 2019 was 751 kg SRP per year, showing overall net adsorption of SRP.
The present study underlines the high importance of phosphorus adsorption and desorption processes in river floodplains. The fluctuations of the main channel water level determined the adsorption and desorption processes of phosphate to the sediment on both the short- and the long-term. It influenced the availability of SRP in water bodies with different connectivity levels and, thereby, the SRP balance of the Lower Lobau floodplain.
Prior studies have shown that the hydrology is a reliable driver for phosphorus availability in the floodplain Lower Lobau (Reckendorfer et al., 2013; Weigelhofer et al., 2015). Danube water imports (average SRP concentration during floods: 20.6 ± 5.2 μg L–1) into the main side-arm of the floodplain result in a decreasing gradient of water column SRP concentrations in the floodplain water bodies, determined by their distance to the inflow area and their connection level. Due to the fast turnover rates, peak concentrations of water column SRP decrease within 2–3 days after the flood peak in the main side-arm (Weigelhofer et al., 2015; Supporting information, Supplementary Figure 3). Similar observations have been made by House et al. (1995), who reported a fast initial reaction of P-adsorption under oxic conditions, with about 60% uptake in the first half-hour.
Various mechanisms of phosphate removal were studied in floodplains, such as, e.g., assimilation by autotrophic and heterotrophic organisms, interactions with the sediment, and output to the main channel (Noe et al., 2019). Despite the high importance of these processes for the phosphorus cycling, in general, we think that biotic uptake played a minor role in the removal of the imported P in the Lower Lobau due to decreased phytoplankton densities during flooding (Weigelhofer et al., 2015). Instead, we assume that adsorption is a major removal pathway in this floodplain system, whereby a large part of the water column SRP imported during floods is already adsorbed in the upper sediment layers of LFW and a minor part in LRW. Within a few days after floods, SRP declined to concentrations below EPC0 of the sediments, which potentially enabled the desorption of SRP. The implemented EPC0 method included the adsorption or desorption of easily soluble phosphate under idealized conditions and was therefore calculated as adsorption or desorption potential. SRP adsorption or desorption in the field depends on both large- and micro-scale characteristics and conditions of the sediments. For example, diffusive boundary layers that indicate the change from oxic to anoxic conditions in the sediment could inhibit or slow down SRP diffusion from the pore water to the water column (Golterman, 2004).
After floods, SRP concentrations were consistently low and below EPC0 in all water bodies except SW. Other studies showed increasing algal biomass and total phosphorus concentrations in the Lower Lobau water bodies after floods, especially in more isolated sections (Reckendorfer et al., 2013; Weigelhofer et al., 2015). We attribute this release to the desorption of the previously adsorbed SRP, leading to the observed phytoplankton peaks after floods.
To be able to set up an SRP adsorption/desorption balance for the floodplain, we assumed constant sediment characteristics and conditions throughout the study period. It enabled us to use a segment based model with a high spatial resolution to upscale adsorption/desorption patterns of SRP at floodplain scale. As shown, adsorption/desorption potentials were strongly determined by the hydrological connectivity of the water bodies. It was also indicated that water bodies with higher hydrological connectivity showed much higher potential for SRP adsorption if the sediments were not gravel-dominated, such as in FL. About 65% of the calculated adsorption potential occurred in LFW (Table 6) due to the high SRP concentrations during connection periods and the mainly silt-dominated sediments. In other water bodies, only minor P-adsorption and -desorption potential was indicated. A large portion (about 75%) of the adsorbed SRP in LFW was released gradually in the periods after floods (Table 6). The calculated desorption potential can, therefore, be described as the buffering capacity of the floodplain. About 30% of the annual adsorption potential was attributed to temporary water bodies (T), inundated only during floods. We calculated the potential based on the sediment characteristics and the corresponding EPC0. Other studies (Schönbrunner et al., 2012; Dieter et al., 2015) reported that repeated drying and wetting resulted in an elevated phosphorus release from the sediments. These processes were not included in our calculations and may outmatch the considered adsorption processes. As temporary water areas were inundated only for short periods (about 1 week during floods), the desorption potential was low, and the adsorbed SRP was removed from the water column for more extended periods.
Table 6. Average adsorption/desorption potential of SRP per year in the period 1999–2019 (in kg ha–1 per year) per water body type (wb).
The overall buffering capacity of the floodplain water bodies was mainly determined by large, frequently connected water bodies (LFW). The main reason was the higher and more frequent SRP inputs and the large area compared with other water body types. Furthermore, in these water bodies, mostly silt-dominated and mixed sediments with low OC were found, which had the highest capacity for SRP adsorption and, therefore, also for desorption. The buffering mechanism of SRP had a crucial impact on the phosphorus availability in the floodplain water bodies after floods. It was of high importance for the algal primary production, resulting in algal peaks after floods (up to 45 μg Chl-a L–1 in LFW, compared with about 10 μg L–1 before floods, Weigelhofer et al., 2015).
Further hydrological decoupling of the Lower Lobau floodplain from the main river channel, as predicted by Hohensinner et al. (2008), will result in a reduction of water bodies and a decline of connection periods. This will, in turn, lead to a decrease in the adsorption potential, the buffering capacity, and the retention of phosphorus in the floodplain. The re-establishment of floodplain-typical hydrodynamics (connection at low water level; 141.1 m a.s.l.) could completely change the P dynamics within the floodplain and the floodplain–main channel interactions. It includes a changing availability of SRP due to the higher nutrient import from the main channel and altered sediment characteristics and, therefore, a changed adsorption potential. We estimated that the lateral channels of the main side-arm with non-flowing conditions would show the most dynamic adsorption/desorption patterns. Because of the increased water SRP concentrations due to continuous inputs from the Danube main channel, the desorption potential would also decrease. Although the buffering function of the sediments would be primarily limited to backwater areas, the overall phosphorus retention of the floodplain could be higher than under current conditions because of the increased area of water bodies and the more dynamic water level fluctuations. Besides, increased SRP concentrations in the main side-arm would result in higher phytoplankton primary production (Amoros and Bornette, 2002; Preiner et al., 2008) and can increase the overall P-retention of the floodplain.
Under the current conditions, river–floodplain interactions in the Danube floodplain Lower Lobau are reduced to short periods of connection during increased riverine water levels. Hydrology has a temporal but also a very distinct spatial component. While flooding controls the exchange with the main river channel, the location and morphology of the floodplain water bodies determine the degree and duration of this connection. Based on the SRP dynamics in different water bodies, we could show that the hydrology strongly affects the net adsorption/desorption potential of the floodplain with a vast impact on the overall role of the floodplain for the P dynamics. The SRP adsorption in the floodplain has the potential to reduce the SRP load of the Danube main channel by SRP retention. The floodplain sediments can act as a buffering system by taking up high amounts of SRP during floods and releasing parts of the adsorbed P time-delayed over a more extended period within the floodplain water bodies. This had a crucial impact on phosphorus availability in the floodplain water bodies and is of high importance for the primary production in the floodplain water bodies after flood events.
The datasets generated for this study are available on request to the corresponding author.
SP: conceptualization, funding acquisition, project administration, investigation, data curation, formal analysis, and writing – original draft. EB-K: conceptualization, investigation, data curation, formal analysis, and writing – review. BP: data curation, formal analysis, and writing – review. GW: conceptualization and writing – review. TH: conceptualization, funding acquisition, project administration, supervision, and writing – review. All authors contributed to the article and approved the submitted version.
This research was funded by the European Regional Development Fund, no. 323A/2010/043, the Vienna City Authorities (MA45), and the Austrian Science Fund (FWF), project FLASHMOB, no. I3216-N29; it was supported by the National Park Donau-Auen GmbH.
The authors declare that the research was conducted in the absence of any commercial or financial relationships that could be construed as a potential conflict of interest.
We thank the two reviewers whose comments helped to improve earlier versions of the manuscript. We thank Gruppe Wasser®, DonauConsult Ingenieurbüro GmbH, Systema GmbH, and the Institute of Hydraulic Engineering and Water Resources Management, Vienna University of Technology for hydro-morphological and macrophyte related data.
The Supplementary Material for this article can be found online at: https://www.frontiersin.org/articles/10.3389/fenvs.2020.00147/full#supplementary-material
Ahlgren, J. (2006). Organic Phosphorus Compounds in Aquatic Sediments: Analysis, Abundance and Effects. Available at: http://urn.kb.se/resolve?urn=urn:nbn:se:uu:diva-6701 (accessed February 17, 2020)
Amoros, C., and Bornette, G. (2002). Connectivity and biocomplexity in waterbodies of riverine floodplains. Freshw. Biol. 47, 761–776. doi: 10.1046/j.1365-2427.2002.00905.x
APHA (1998). Standard Methods for the Examination of Water and Waste Water, 20th Edn. Washington, DC: American Public Health Association.
Baart, I., Gschöpf, C., Blaschke, A. P., Preiner, S., and Hein, T. (2010). Prediction of potential macrophyte development in response to restoration measures in an urban riverine wetland. Aquat. Bot. 93, 153–162. doi: 10.1016/j.aquabot.2010.06.002
Barrow, N. J. (1978). The description of phosphate adsorption curves. J. Soil Sci. 29, 447–462. doi: 10.1111/j.1365-2389.1978.tb00794.x
Billen, G., Lancelot, C., and Meybeck, M. (1991). “N, P, and Si retention along the aquatic continuum from land to ocean,” in Ocean Margin Processes in Global Change, eds R. F. C. Mantoura, R. F. Mantoura, J.-M. Martin, R. Wollast, and T. D. Jickells (New York, NY: John Wiley & Sons), 19–44.
Borggaard, O. K., Raben-Lange, B., Gimsing, A. L., and Strobel, B. W. (2005). Influence of humic substances on phosphate adsorption by aluminum and iron oxides. Geoderma 127, 270–279. doi: 10.1016/j.geoderma.2004.12.011
Danielopol, D. L., Rouch, R., Pospisil, P., Torreiter, P., and Mösslacher, F. (1997). “Ecotonal animal assemblages; their interest for groundwater studies,” in Groundwater/Surface Water Ecotones: Biological and Hydrological Interactions and Management Options, eds J. Gibert, J. Mathieu, and F. Fournier (Cambridge, MA: Press Syndicate of the University of Cambridge), 11–20. doi: 10.1017/cbo9780511753381.003
Dieter, D., Herzog, C., and Hupfer, M. (2015). Effects of drying on phosphorus uptake in re-flooded lake sediments. Environ. Sci. Pollut. Res. 22, 17065–17081. doi: 10.1007/s11356-015-4904-x
Dormann, C. F., Elith, J., Bacher, S., Buchmann, C., Carl, G., Carré, G., et al. (2013). Collinearity: a review of methods to deal with it and a simulation study evaluating their performance. Ecography 36, 27–46. doi: 10.1111/j.1600-0587.2012.07348.x
Dunne, E. J., Culleton, N., O’Donovan, G., Harrington, R., and Daly, K. (2005). Phosphorus retention and sorption by constructed wetland soils in Southeast Ireland. Water Res. 39, 4355–4362. doi: 10.1016/j.watres.2005.09.007
Fisher, J., and Acreman, M. (2004). Wetland nutrient removal: a review of the evidence. Hydrol. Earth Syst. Sci. 8, 673–685. doi: 10.5194/hess-8-673-2004
Froelich, P. N. (1988). Kinetic control of dissolved phosphate in natural rivers and estuaries: a primer on the phosphate buffer mechanism. Limnol. Oceanogr. 33, 649–668. doi: 10.4319/lo.1988.33.4_part_2.0649
Funk, A., Gschöpf, C., Blaschke, A. P., Weigelhofer, G., and Reckendorfer, W. (2013). Ecological niche models for the evaluation of management options in an urban floodplain—conservation vs. restoration purposes. Environ. Sci. Policy 34, 79–91. doi: 10.1016/j.envsci.2012.08.011
Golterman, H. L. (2004). The Chemistry of Phosphate and Nitrogen Compounds in Sediments. Dordrecht: Kluwer Academic Publishers.
Hastie, T., Tibshirani, R., and Friedman, J. H. (2017). The Elements of Statistical Learning - Data Mining, Inference, and Prediction. 2nd ed. 2009, corr. 9th printing 2017. Cham: Springer.
Hein, T., Schwarz, U., Habersack, H., Nichersu, I., Preiner, S., Willby, N., et al. (2016). Current status and restoration options for floodplains along the Danube River. Sci. Total Environ. 543, 778–790. doi: 10.1016/j.scitotenv.2015.09.073
Hoffmann, C. C., Kjaergaard, C., Uusi-Kämppä, J., Hansen, H. C. B., and Kronvang, B. (2009). Phosphorus retention in riparian buffers: review of their efficiency. J. Environ. Qual. 38, 1942–1955. doi: 10.2134/jeq2008.0087
Hohensinner, S., Herrnegger, M., Blaschke, A. P., Habereder, C., Haidvogl, G., Hein, T., et al. (2008). Type-specific reference conditions of fluvial landscapes: a search in the past by 3D-reconstruction. CATENA 75, 200–215. doi: 10.1016/j.catena.2008.06.004
House, W. A. (2003). Geochemical cycling of phosphorus in rivers. Appl. Geochem. 18, 739–748. doi: 10.1016/S0883-2927(02)00158-0
House, W. A., and Denison, F. H. (2000). Factors influencing the measurement of equilibrium phosphate concentrations in river sediments. Water Res. 34, 1187–1200. doi: 10.1016/S0043-1354(99)00249-3
House, W. A., Denison, F. H., and Armitage, P. D. (1995). Comparison of the uptake of inorganic phosphorus to a suspended and stream bed-sediment. Water Res. 29, 767–779. doi: 10.1016/0043-1354(94)00237-2
James, W. F., and Barko, J. W. (2004). Diffusive fluxes and equilibrium processes in relation to phosphorus dynamics in the Upper Mississippi River. River Res. Appl. 20, 473–484. doi: 10.1002/rra.761
Jarvie, H. P., Jürgens, M. D., Williams, R. J., Neal, C., Davies, J. J. L., Barrett, C., et al. (2005). Role of river bed sediments as sources and sinks of phosphorus across two major eutrophic UK river basins: the Hampshire Avon and Herefordshire Wye. J. Hydrol. 304, 51–74. doi: 10.1016/j.jhydrol.2004.10.002
Jarvie, H. P., Neal, C., and Withers, P. J. A. (2006). Sewage-effluent phosphorus: a greater risk to river eutrophication than agricultural phosphorus? Sci. Total Environ. 360, 246–253. doi: 10.1016/j.scitotenv.2005.08.038
Junk, W. J., and Wantzen, K. (2004). “The flood pulse concept: new aspects, approaches and applications—an update,” in Proceedings of the Second International Symposium on the Management of Large Rivers for Fisheries (LARS2), London.
Kleeberg, A., Hupfer, M., and Gust, G. (2008). Quantification of phosphorus entrainment in a lowland river by in situ and laboratory resuspension experiments. Aquat. Sci. 70, 87–99. doi: 10.1007/s00027-007-0935-9
Lair, G. J., Zehetner, F., Fiebig, M., Gerzabek, M. H., van Gestel, C. A. M., Hein, T., et al. (2009). How do long-term development and periodical changes of river–floodplain systems affect the fate of contaminants? Results from European rivers. Environ. Pollut. 157, 3336–3346. doi: 10.1016/j.envpol.2009.06.004
Mainstone, C. P., and Parr, W. (2002). Phosphorus in rivers — ecology and management. Sci. Total Environ. 28, 25–47. doi: 10.1016/S0048-9697(01)00937-8
Manning, P., Putwain, P. D., and Webb, N. R. (2006). The role of soil phosphorus sorption characteristics in the functioning and stability of lowland heath ecosystems. Biogeochemistry 81, 205–217. doi: 10.1007/s10533-006-9037-3
>Milborrow, S., Hastie, T., Tibshirani, R., Fortran, A. M., and Lumley’s, T. (2019). earth: Multivariate Adaptive Regression Splines. R package version 5.1.2. Available online at: https://CRAN.R-project.org/package=earth
Noe, G. B., Boomer, K., Gillespie, J. L., Hupp, C. R., Martin-Alciati, M., Floro, K., et al. (2019). The effects of restored hydrologic connectivity on floodplain trapping vs. release of phosphorus, nitrogen, and sediment along the Pocomoke River, Maryland USA. Ecol. Eng. 138, 334–352. doi: 10.1016/j.ecoleng.2019.08.002
Noe, G. B., and Hupp, C. R. (2009). Retention of riverine sediment and nutrient loads by coastal plain floodplains. Ecosystems 12, 728–746. doi: 10.1007/s10021-009-9253-5
Palmer-Felgate, E. J., Mortimer, R. J. G., Krom, M. D., Jarvie, H. P., Williams, R. J., Spraggs, R. E., et al. (2011). Internal loading of phosphorus in a sedimentation pond of a treatment wetland: effect of a phytoplankton crash. Sci. Total Environ. 409, 2222–2232. doi: 10.1016/j.scitotenv.2011.02.034
Preiner, S., Drozdowski, I., Schagerl, M., Schiemer, F., and Hein, T. (2008). The significance of side-arm connectivity for carbon dynamics of the River Danube, Austria. Freshw. Biol. 53, 238–252. doi: 10.1111/j.1365-2427.2007.01888.x
Preiner, S., Weigelhofer, G., Funk, A., Hohensinner, S., Reckendorfer, W., Schiemer, F., et al. (2018). “Danube Floodplain Lobau” in Riverine Ecosystem Management: Science for Governing Towards A Sustainable Future. New York, NY: Springer.
Reckendorfer, W., Funk, A., Gschöpf, C., Hein, T., and Schiemer, F. (2013). Aquatic ecosystem functions of an isolated floodplain and their implications for flood retention and management. J. Appl. Ecol. 50, 119–128. doi: 10.1111/1365-2664.12029
Records, R. M., Wohl, E., and Arabi, M. (2016). Phosphorus in the river corridor. Earth Sci. Rev. 158, 65–88. doi: 10.1016/j.earscirev.2016.04.010
Reddy, K. R., Kadlec, R. H., Flaig, E., and Gale, P. M. (1999). Phosphorus retention in streams and wetlands: a review. Crit. Rev. Environ. Sci. Technol. 29, 83–146. doi: 10.1080/10643389991259182
Ruban, V., López-Sánchez, J. F., Pardo, P., Rauret, G., Muntau, H., and Quevauviller, P. H. (2001). Harmonized protocol and certified reference material for the determination of extractable contents of phosphorus in freshwater sediments - A synthesis of recent works. Fresenius J. Anal. Chem. 370, 224–228. doi: 10.1007/s002160100753
Schönbrunner, I. M., Preiner, S., and Hein, T. (2012). Impact of drying and re-flooding of sediment on phosphorus dynamics of river-floodplain systems. Sci. Total Environ. 432, 329–337. doi: 10.1016/j.scitotenv.2012.06.025
Smith, L., and Wheatcraft, S. W. (1993). “Groundwater flow,” in Handbook of Hydrology, ed. D. Maidment (New York, NY: McGraw-Hill).
Taylor, A. W., and Kunishi, H. M. (1971). Phosphate equilibria on stream sediment and soil in a watershed draining an agricultural region. J. Agric. Food Chem. 19, 827–831. doi: 10.1021/jf60177a061
Tockner, K., Pusch, M., Borchardt, D., and Lorang, M. S. (2010). Multiple stressors in coupled river–floodplain ecosystems. Freshw. Biol. 55, 135–151. doi: 10.1111/j.1365-2427.2009.02371.x
Tockner, K., and Stanford, J. A. (2002). Riverine flood plains: present state and future trends. Environ. Conserv. 29, 308–330. doi: 10.1017/S037689290200022X
Tockner, K., Uehlinger, U., and Robinson, C. T. (2009). Rivers of Europe. Cambridge, MA: Academic Press.
Van der Lee, G. E. M., Venterink, H. O., and Asselman, N. E. M. (2004). Nutrient retention in floodplains of the Rhine distributaries in The Netherlands. River Res. Appl. 20, 315–325. doi: 10.1002/rra.780
Watson, F. T., Smernik, R. J., Doolette, A. L., and Mosley, L. M. (2019). Phosphorus speciation and dynamics in river sediments, floodplain soils and leaf litter from the Lower Murray River region. Mar. Freshw. Res. 70, 1522–1532. doi: 10.1071/MF18360
Weigelhofer, G., Hein, T., and Bondar-Kunze, E. (2018). “Phosphorus and nitrogen dynamics in riverine systems: human impacts and management options,” in Riverine Ecosystem Management: Science for Governing Towards a Sustainable Future Aquatic Ecology Series, eds S. Schmutz and J. Sendzimir (Cham: Springer), 187–202. doi: 10.1007/978-3-319-73250-3_10
Weigelhofer, G., Preiner, S., Funk, A., Bondar-Kunze, E., and Hein, T. (2015). The hydrochemical response of small and shallow floodplain water bodies to temporary surface water connections with the main river. Freshw. Biol. 60, 781–793. doi: 10.1111/fwb.12532
Welti, N., Bondar-Kunze, E., Tritthart, M., Pinay, G., and Hein, T. (2012). Nitrogen dynamics in complex Danube River floodplain systems: effects of restoration. River Syst. 20, 71–85. doi: 10.1127/1868-5749/2011/0047
Withers, P. J. A., and Jarvie, H. P. (2008). Delivery and cycling of phosphorus in rivers: a review. Sci. Total Environ. 400, 379–395. doi: 10.1016/j.scitotenv.2008.08.002
Wolf, K. L., Noe, G. B., and Ahn, C. (2013). Hydrologic connectivity to streams increases nitrogen and phosphorus inputs and cycling in soils of created and natural floodplain wetlands. J. Environ. Qual. 42, 1245–1255. doi: 10.2134/jeq2012.0466
Keywords: phosphorus adsorption, equilibrium phosphorus concentration, floodplain, Danube, phosphorus adsorption and desorption, hydrological connectivity
Citation: Preiner S, Bondar-Kunze E, Pitzl B, Weigelhofer G and Hein T (2020) Effect of Hydrological Connectivity on the Phosphorus Buffering Capacity of an Urban Floodplain. Front. Environ. Sci. 8:147. doi: 10.3389/fenvs.2020.00147
Received: 02 March 2020; Accepted: 29 July 2020;
Published: 19 August 2020.
Edited by:
Rebecca Elizabeth Tharme, Riverfutures Ltd., United KingdomReviewed by:
Gisela Mayora, National Institute of Limnology (INALI), ArgentinaCopyright © 2020 Preiner, Bondar-Kunze, Pitzl, Weigelhofer and Hein. This is an open-access article distributed under the terms of the Creative Commons Attribution License (CC BY). The use, distribution or reproduction in other forums is permitted, provided the original author(s) and the copyright owner(s) are credited and that the original publication in this journal is cited, in accordance with accepted academic practice. No use, distribution or reproduction is permitted which does not comply with these terms.
*Correspondence: Stefan Preiner, c3RlZmFuLnByZWluZXJAYm9rdS5hYy5hdA==
Disclaimer: All claims expressed in this article are solely those of the authors and do not necessarily represent those of their affiliated organizations, or those of the publisher, the editors and the reviewers. Any product that may be evaluated in this article or claim that may be made by its manufacturer is not guaranteed or endorsed by the publisher.
Research integrity at Frontiers
Learn more about the work of our research integrity team to safeguard the quality of each article we publish.