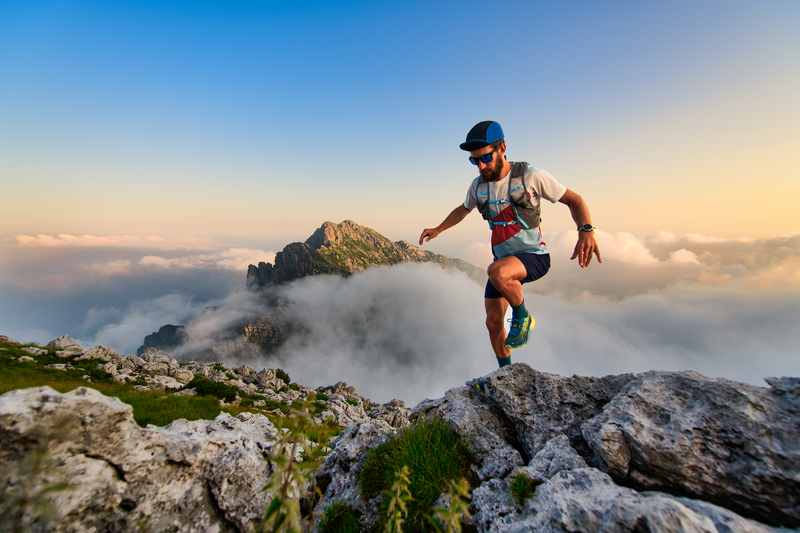
95% of researchers rate our articles as excellent or good
Learn more about the work of our research integrity team to safeguard the quality of each article we publish.
Find out more
ORIGINAL RESEARCH article
Front. Environ. Sci. , 30 June 2020
Sec. Biogeochemical Dynamics
Volume 8 - 2020 | https://doi.org/10.3389/fenvs.2020.00089
This article is part of the Research Topic Biogeochemistry of Anthropogenic Particles View all 20 articles
Substantial efforts have been undertaken to isolate and characterize plastic contaminants in different sample matrices in the last years as the ubiquitous presence of particulate plastic in the environment has become evident. In comparison, plastic particles <1 µm (nanoplastic) in the environment remain mostly unexplored. Adequate techniques for the enrichment, as well as the detection of nanoplastic, are lacking but are urgently needed to assess the full scope of (potential) nanoplastic pollution. Use of Pd-doped nanoplastic particles constitutes a powerful tool to develop new analytical approaches, as they can be traced accurately and with ease in a variety of complex matrices by highly sensitive, time-efficient and robust ICP-MS(/MS) techniques. In this lab-scale study, for the first time, the capability of continuous flow centrifugation to retain nanoplastic particles (∼160 nm) from ultrapure water, as well as from filtered and unfiltered water from the German Elbe River was evaluated. Depending on the pump rate, the retention efficiency for the nanoplastic particles in ultrapure water ranged from 92% ± 8% (1 L h−1) to 53% ± 5% (5 L h−1) [uc (n = 3)] and from 75% ± 5% to 65% ± 6% (uc) (2.5 L h−1) in river water. Recirculating the water through the system two and three times at the highest tested flow rate led to retention efficiencies >90%. In a proof-of-principle setup, it was demonstrated that operating two continuous flow centrifuges sequentially at different rotational speeds bears the potential to enable size- and density-selective sampling of the colloidal fraction. A significant fraction of the spiked nanoplastic particles [76% ± 5% (uc)] could be separated from a model mixture of natural particles with a well-defined mean size of approximately 3 µm. While the certified reference plankton material used here was quantitatively retained in the first centrifuge rotor together with 23.0% ± 2.2% of the effective dose of the spiked nanoplastic, the remaining fraction of the nanoplastic could be recovered in the second rotor (53% ± 5%) and the effluent [24.4% ± 2.4% (uc)]. Based on the good retention efficiencies and the demonstrated separation potential, continuous flow centrifugation has proven to be a very promising technique for nanoplastic sampling and enrichment from natural water samples.
Particulate plastic has gained high importance as an emerging environmental contaminant for researchers, authorities and society as a whole (Stöven et al., 2015; Gago et al., 2016; Rochman et al., 2016; Peng et al., 2017; Burns and Boxall, 2018; Science Advice for Policy by European Academies, 2019). Notwithstanding research on other engineered nanoparticles (e.g., inorganic nanoparticles) (Hüffer et al., 2017), evidence on nanoplastic particles [NPPs, defined as 1 nm to 1000 nm in size for this article (Gigault et al., 2018)] and even on particles smaller than 10 µm in natural systems is very scarce (Ter Halle et al., 2017; Meyns et al., 2019). NPPs are contained in many consumer products and can additionally be released as secondary NPPs from macro- and microplastic (MP, 1 µm – 5 mm) due to UV-induced and mechanical degradation processes (Lambert and Wagner, 2016; Lehner et al., 2019; Mitrano et al., 2019). Furthermore, NPPs are expected to exhibit different properties than particles >1 µm (Mattsson et al., 2015) as easier translocation and uptake into cells are also probable. Nanoplastic beads were shown to enhance toxicity of persistent organic pollutants toward Rotifers (Jeong et al., 2018), hinder algal photosynthesis (Bhattacharya et al., 2010) and exhibit different adverse effects on human lung epithelial cells (Xu et al., 2019).
Due to analytical challenges related with sampling and analysis, there is no definite proof of NPPs being present in the environment so far. Currently, there are also no validated standard operation procedures to analyze particulate plastic (Löder and Gerdts, 2015; Besley, 2017), despite some activities aiming at methodological standardization from institutions such as the International Organization for Standardization (ISO/TC 61/SC 14) and JPI Oceans. Furthermore, no certified reference materials exist which could enable a valid comparison of the performance of different sampling, sample processing and characterization approaches (Frias et al., 2019).
The quantification of NPPs is highly challenging. Well-established techniques for MP particle analysis, such as FTIR and Raman microspectroscopy, have size detection limits of approximately 10 µm and 1 µm, respectively (Käppler et al., 2016; Ivleva et al., 2017; Primpke et al., 2017). Method optimization can slightly decrease these limits (to a few micrometer for FTIR and several hundred nanometers for Raman microscopy), but analyzing submicron plastic particles still requires combination of FTIR spectroscopy with atomic force microscopy (AFM), or SEM-EDX in order to achieve the necessary lateral resolution and chemical information (Shim et al., 2017; Meyns et al., 2019). However, these kinds of analysis are very time-consuming and inefficient in terms of spatial coverage (Koelmans, 2019). Methods which determine mass concentrations of particulate plastic based on separation of characteristic combustion products by gas chromatography hyphenated to mass spectrometry require extensive sample purification and high preconcentration of colloidal plastic to meet the current quantification limits (Dümichen et al., 2017; Fischer and Scholz-Böttcher, 2017).
Some techniques which are technically capable of measuring particles in the nanometer range are inadequate for assessing NPP concentration or sizes in complex matrices. For example, Dynamic light scattering (DLS) and asymmetric flow field-flow fractionation (AF4) coupled with multi-angle light scattering (MALS) provide size-related information, but no information on the polymer type and potential aggregation. Quantitative plastic-specific isolation from the matrix and in addition a priori knowledge of the refractive index would be required (Correia and Loeschner, 2018; Ekvall et al., 2019).
Chemically labeled particles have previously been used to circumvent these analytical limitations to mechanistically understand NPP fate, transport and biological interactions (Cole et al., 2013; Lusher et al., 2017). Fluorescence-labeled particles, for instance, can easily be identified in tissues and complex structures using fluorescence microscopes. However, in biological exposure experiments, it was reported that these dyes can leach out and lead to fluorescence in examined organisms without particle uptake (Schür et al., 2019). A second problem related with fluorescence-labeled particles is photobleaching (Sullivan and Gugliada, 2018).
Using metal-doped particles is a promising new approach. Even with a trace metal content embedded in the polymer of <0.5% w/w, the sensitivity of ICP-MS enables particle detection at lower concentrations in a variety of complex matrices (Keller et al., 2019; Mitrano et al., 2019; Schmiedgruber et al., 2019) after total microwave-assisted acid digestion (MWAD) of the bulk sample. While only a model, metal-doped particles can also be a valuable tool when it comes to the investigation of potential techniques for environmental NPP extraction and enrichment.
Though a high percentage of NPPs occur as heteroaggregates in the environment with either natural organic or inorganic materials (Hüffer et al., 2017; Oriekhova and Stoll, 2018), sampling techniques for the colloidal fraction still need to be developed with NPPs in mind. Suitable sampling and concentration techniques are understudied but crucial aspects of the analytical method development workflow (Koelmans et al., 2015; Schwaferts et al., 2019; Zhou et al., 2019). Schwaferts et al. (2019) presume that the mass of NPPs in the aquatic environment is likely very low (Schwaferts et al., 2019). Thus, for NPP analysis considerable pre-concentration may be necessary in order to have enough material to analyze and characterize as well as to meet the LODs/LOQs of the currently used analytical techniques. Using conventional nanofiltration is technically not feasible due to the low volume flows (Hernandez et al., 2017; Mintenig et al., 2018) (i.e., small sample sizes are not likely to capture the NPPs). Even in the range of 10 µm – 20 µm, filtration is quite problematic when high contents of suspended particulate matter (SPM) are present and large volumes need to be processed to obtain representative samples (Enders et al., 2015; Lenz and Labrenz, 2018; Bannick et al., 2019).
Alternatives to filtration-based approaches are needed which enable nanometer-size-selective and time-efficient sampling. The application of continuous flow centrifugation (CFC) for efficient sampling of the fine particulate fraction (1 µm – 25 µm) in natural water at high flow rates of several hundred liters per hour has already been shown (Douglas et al., 1993; Ran et al., 2000; Conn et al., 2016). Recently, CFC proved to be a suitable technique to quantitatively enrich small MP (model MP ranged from 1 µm to 1 mm) of a variety of polymer types with densities ranging from 0.94 g mL−1 to 1.63 g mL−1 (Hildebrandt et al., 2019). In a second step, it was applied to sample small MP particles from the German Elbe estuary. After the sampling, the content of the centrifuge had to be subjected to a purification protocol including oxidative-chemical treatment and density separation for matrix reduction prior to final spectroscopic analysis.
From a physical perspective, CFC is also capable of retaining particles in the submicron range when the flow rate is further reduced (see section “Data processing and calculations”). It poses a promising option whenever particles with a sedimentation coefficient >50 Svedberg must be separated from volumes >2 L (Dorin and Cummings, 2015). If the density of the particles, the viscosity and density of the medium and the geometry of the CFC rotor are known, the optimal flow rate for maximum particle removal can be estimated (see section “Data processing and calculations”).
The good performance of CFC for MP sampling (Hildebrandt et al., 2019) and its wide application in the biomedical sector to pellet subcellular components (Goodenough, 1974; Kahane et al., 1976; Cacace et al., 1977; Turk et al., 1988) and viruses (Shibley et al., 1980; Wheeler et al., 1986; Anderson et al., 1991) were a motivation to test the enrichment of NPPs by CFC as well. In this study, Pd-doped NPPs were spiked into ultrapure water to assess the efficiency of CFC in retaining NPPs and evaluate the effect of different pump rates (1) and recirculation (2), into natural water to test NPP retention under realistic environmental conditions (3) and into a model matrix suspension with a high concentration of micrometer-sized SPM to evaluate the potential of a serial CFC approach for nanometer-size-selective sampling (separation) (4). Through this procedure, we could assess which conditions are most applicable to concentrate NPPs under laboratory conditions, and propose a workflow for enriching NPPs from environmental water samples.
Pd-doped nanoplastic particles were previously developed and characterized, as described by Mitrano et al. (2019), with additional characterization of the particles used in this study as detailed below. Briefly, the particles had a core/shell structure featuring a polyacrylonitrile core doped with Pd and a polystyrene shell. The hydrodynamic diameter of the particles was 175 nm ± 1.3 nm, with a low polydispersity (PDI: 0.02) as measured by dynamic light scattering. A slightly smaller diameter (approximately 160 nm) was measured by transmission electron microscopy. A 1:10 dilution of the original stock suspension was performed to a final volume of 50 mL, to which 2 drops of Triton X-100 (VWR Life Science, Darmstadt, Germany) were added to ensure particle stability through storage. This dilution served as a working stock suspension for all experiments. To confirm that the Pd was completely incorporated into the polymer matrix and there was not free Pd remaining from particle synthesis, 100 µL of the suspension was filled up to 10 mL with Milli-Q water (MQW) and centrifuged at 10000 rpm for 7 h (Centrifuge 5804 R, Eppendorf AG, Hamburg, Germany). Measurement of the supernatant showed that at maximum 0.767% ± 0.017% [1 SD (n = 3)] of the Pd was not associated with the particles. The solid content in the NPP suspension was determined gravimetrically after freeze-drying (Gamma 1–16 LSC plus Christ, Osterode, Germany). The stock suspension used for all spiking experiments contained 0.848% ± 0.021% (w/w) [1 SD (n = 3)] NPPs. The Pd content of the working stock suspension was 22.8 mg L−1 ± 1.3 mg L−1 [uc (n = 12)]. Consequently, the Pd mass fraction of the NPPs was approximately 0.27% (w/w).
Preparatory laboratory work was performed in a class 10,000 clean room inside a class 100 clean bench. Type I reagent-grade water (18.2 MΩ cm) was obtained from a Milli-Q Integral water purification system (Merck-Millipore, Darmstadt, Germany), equipped with a Q-Pod Element. Suprapur® nitric acid [65% (w/w), Merck-Millipore] and suprapur® hydrochloric acid [30% (w/w), Merck-Millipore] were further purified by double sub-boiling in PFA stills (Savillex, Eden Prairie, United States) operated under clean room conditions. Polyethylene and polypropylene flasks, tubes and pipette tips (VWR International, Radnor, United States), as well as perfluoroalkoxy (PFA) screw cap vials (Savillex, Eden Prairie, United States) were pre-cleaned in a two-stage washing procedure using nitric acid [10% (w/w) and 1% (w/w), respectively]. A single-element standard of Pd (C = 1 g L−1) obtained from Agilent Technologies (Santa Clara, CA, United States) was used for external calibration.
Elbe river water was sampled from a dock in the Elbe river located in Geesthacht, Germany (53° 24.6′ N, 10° 25.6′ E, 12/17/2019) with a cleaned 25 L HDPE bottle (Kautex Textron, Bonn, Germany). As we aimed to specifically only quantify nanoplastics with the Pd-doping, any potential plastic contamination during the sampling procedure was inconsequential. An aliquot of the water was directly filled into 5 L glass bottles with PFA screw caps (Schott AG, Mainz, Germany). A second aliquot was filtered over bottle-top SCFA filters (0.2 µm; Nalgene, Rochester, NY, United States) directly into 5 L glass bottles. The SPM concentration was determined gravimetrically prior to (2.60 mg L−1 ± 0.23 mg L−1) and after [6.5 mg L−1 ± 0.7 mg L−1 (1 SD; n = 3)] the incubation, by filtration of 1 L over glass fiber membrane filters (0.7 µm, Merck, Darmstadt, Germany). A Multi 3430© sensor system (WTW GmbH, Weilheim, Germany) was employed to determine physical water parameters, such as temperature, conductivity, oxygen content and pH (T = 4.3°C, EC = 1084 mS cm−1, CO2 = 12.33 mg L−1, pH = 8.165). 1 mL of the working stock Pd-doped NPP suspension was spiked into replicates of 5 L of the filtered and unfiltered river water and stirred for 31 days (magnetic stirrer at 500 rpm, Tmean = 20°C) prior to being pumped through the CFC system. The number of added NPPs was chosen based on achieving a sufficient mass of Pd for straightforward tracing in different scenarios.
MWAD was utilized to determine the concentration of the certified elements in BCR-414 (As, Cd, Cr, Cu, Hg, Mn, Ni, Pb, Se, V, and Zn) and ERM®-EC680m (As, Cd, Cr, Hg, Pb, Sb, Sn, and Zn) (both Joint Research Centre (JRC), European Commission) and the Pd concentration for all fractions that could not be introduced directly into the ICP-MS/MS due to the presence of SPM in the micrometer size range. After freeze-drying (Gamma 1–16 LSC plus Christ), residues were digested with 4 mL HNO3 and 1 mL HCl in pre-cleaned 35 mL Quartz vials (Discover-SP-D 35; CEM Corp., Kamp Lintfort, Germany) at 230°C (20 min ramp and 5 min hold time). After digestion, the clear solutions were transferred quantitatively to 50 mL pre-cleaned DigiTubes® (SCP Science) and diluted to 50 mL with MQW. The plastic certified reference material (CRM) ERM®-EC680m (JRC, Geel, Belgium) was utilized to validate the MWAD protocol in terms of complete dissolution of the polymeric matrix and quantitative elemental recoveries. BCR-414 was used to as a model for interfering matrix particles and traced by its Ni content using ICP-MS/MS (see section “Dependence of Retention Efficiency in MQW on Number of Cycles”).
Metal content determination of all samples was performed using an inductively coupled plasma tandem mass spectrometry (ICP-MS/MS) instrument (Agilent 8800, Agilent Technologies, Tokyo, Japan) coupled to an ESI SC-4 DX FAST autosampler (Elemental Scientific, Omaha, United States). The instrument was tuned in a daily routine using a tune solution containing Li, Co, Y, Ce and Tl. Quantification was conducted by an external calibration, covering a concentration range from 0.1 µg L−1 to 100 µg L−1 for all analytes. Solutions were prepared volumetrically on a daily basis from a combination of multi-element standards (Inorganic Ventures, Christiansburg, United States) made in-house. Wash blanks were measured after each sample triplicate to minimize carry over effects. ICP-MS/MS operating parameters, cell gas modes used and a list of measured isotopes and their detection modes for all analytes can be found in Supplementary Tables S2, S3. Selection of the optimal isotope and measurement mode for Pd quantification was based on analysis of all six stable isotopes in all gas modes (including mass-shift) in a multielement solution (CPd = 500 µg L−1) containing 51 other metals (C = 25 – 250 µg L−1) (Supplementary Table S1). Multi-elemental data were processed using Mass Hunter version 4.2 (Agilent Technologies) and a custom written Excel© spreadsheet.
Two continuous flow centrifuges (Contifuge Stratos, Thermo Scientific, Waltham, United States) in conjunction with two titanium rotors with a sedimentation capacity of 300 mL (Continuous Flow Rotor 3049, Thermo Scientific) were available for all experiments.
In the first three variants of the experiment (see section “Experimental Setups Using One Centrifuge”), the setup comprised only of one centrifuge, whereas for the fourth objective two centrifuges were sequentially connected. For all experiments, peristaltic pumps (Masterflex L/S, Cole-Parmer, Vernon Hills, United States) were applied to pump 5.22 L ± 0.22 L (1 SD, n = 18) of NPP-spiked ultrapure or natural water from a 5 L bottle through the rotating centrifuge rotor. The final spiked water contained 1 mL of working stock suspension. The temperature of the centrifuge was kept constant at 20°C. Before the sample was pumped through the rotor, it was filled with MQW at 4000 rpm and accelerated to the final rotational speed (17000 rpm). To avoid air bubbles entering the centrifuge, the experiments were stopped at a residual volume of 0.29 L ± 0.06 L (1 SD, n = 14) remaining in the glass sample bottle.
The first experiments focusing on the retention of nanoplastic from MQW and river water in a linear setup as well as from MQW in a circular setup were based on usage of one centrifuge (Figure 1). A setup based on a single CFC system leads to enrichment of all retained particulate material ranging from the micrometer to the nanometer size in the system’s rotor. Therefore, no separation of only NPPs is achieved with this configuration.
Figure 1. Schematic drawing of the experimental setup for CFC. (A): Linear setup (1: Suspension bottle containing the NPPs on a magnetic stirrer. 2: Peristaltic pump. 3: Flow Centrifuge. 4: Inflow. 5: Outflow. 6: Collected effluent fraction). (B) Circular setup (Heraeus Instruments GmbH, 1998, modified) © Thermo Scientific.
The water was pumped from the suspension bottle by a peristaltic pump through the CFC rotor into an effluent beaker (Figure 1A). The peristaltic pump was placed between the suspension bottle and the centrifuge. After the procedure, the three fractions of the original spiked water were separated into “residual water in suspension bottle,” “rotor content,” and “effluent”. Using this linear setup, the influence of the pump rate (1 L h−1, 2.5 L h−1, and 5 L h−1) was investigated, as its importance for the degree of retention has previously been explored (Berman, 1966; Brown, 1989). These experiments were run with MQW which allowed direct introduction of the resulting fractions into the ICP-MS/MS, as there was no organic matter and it was tested that the plasma could fully decompose/atomize the NPPs without prior digestion. For all experimental runs, the rotational speed was set to the maximum of 17000 rpm (∼24200 g-forces). After centrifugation, ∼ 5 µL of the detergent Triton X-100 were added (for stabilization) to each of the fractions prior to ultrasonication for 15 min to avoid adhesion to the vessels. Replicates of 50 mL of the fractions suspension bottle (n = 3), rotor content (n = 6) and effluent (n = 6) were taken with a glass pipette and transferred to DigiTubes® (SCP Science, Quebec, Canada). Thorough shaking of the tubes prior to the measurement ensured homogeneous mixing.
The NPP-spiked filtered and unfiltered river water was pumped through the centrifuge at a rate of 2.5 L h−1 and a rotational speed of 17000 rpm. The same pumping procedure as used for the MQW samples was used. However, due to the presence of SPM in the fractions, the content of the suspension bottle and the rotor body were subjected to MWAD prior to ICP-MS/MS measurement to avoid blockages of the nebulizer and other unwanted matrix-based effects. Therefore, replicates (nbottle = 3, nrotor = 6) of 20 mL were transferred to pre-cleaned 35 mL Quartz vials (CEM Corp., Kamp Lintfort, Germany) and freeze-dried. The effluent was sampled and analyzed without need for MWAD, as it was highly clarified through the centrifugation process.
The water was pumped from the suspension bottle by a peristaltic pump through the CFC rotor (17000 rpm) and then back into the suspension bottle (Figure 1B). In this instance, the impact of one and two additional pumping cycles (i.e., by recycling water 2-times and 3-times through the centrifuge) on the retention efficiency was evaluated at 5 L h−1. The peristaltic pump was placed at the same position as in the linear approach.
For the circular approach, only samples from the rotor content and the suspension bottle were obtained and sampled analogously (n = 6). This experimental variant was also run with MQW enabling direct introduction of the resulting fractions into the ICP-MS/MS without MWAD.
The use of a sequence of two CFC systems is our concept for size- and density-selective nanoplastic sampling from water. The goal was to evaluate to what extent separation of nanoparticles from microparticles can be achieved by using a sequence of the two CFC systems operated at different rotational speeds.
In the sequential CFC setup, the sample suspension was pumped from the bottle through the first CFC system and subsequently through a second CFC (Figure 2). The clarified liquid that passed both centrifuges was collected in the effluent beaker. Hereby, a peristatic pump with a dual-channel pump head, which was placed between the bottle and the first centrifuge as well as between both centrifuges, was utilized.
Figure 2. Schematic drawing of the experimental setup for sequential CFC. In our experimental design, the first centrifuge was operated at 4000 rpm and the second one at 17000 rpm (modified, © Thermo Scientific).
In order to mimic natural particles which may aggregate with nanoplastic in environmental samples, BCR-414 [Plankton (trace elements)] was added into the solution which passed through the sequential CFC system. BCR-414 is a powder [dmean = 2.894 µm ± 0.003 µm (1 SD)] of milled organisms such as cladocera and Si-, Ca, CaP-rich, organic and clay particles with a Corg-content of 30% ± 5% [1 SD (n = 6)]. Certified mass fractions for the elements As, Cd, Cr, Hg, Mn, Ni, Pb, Se, V and Zn were provided by the supplier. 5 L MQW were spiked with 500 mg of the CRM. The suspension was stirred for 15 min before spiking 1 mL of NPP suspension and conducting sequential CFC at a pump rate of 2.5 L h−1 (Figure 2). The two centrifuges were operated at different rotational speeds (CFC 1 at 4000 rpm and CFC 2 at 17000 rpm). Subsamples of 20 mL (n = 6) of the fractions suspension bottle and rotor content 1 were transferred to 35 mL Quartz vials (CEM Corp.) and freeze-dried (for MWAD). Subsamples of 50 mL (n = 6) of the clarified fractions rotor content 2 and effluent were sampled and analyzed by ICP-MS/MS directly without MWAD.
The calculation of retention efficiencies (RE) (Eq. 1) is based on the effective dose of particles (using the Pd mass fraction) that entered the centrifuge and that could be recovered in the rotor content and effluent. The recoveries in all fractions and cumulative recoveries can be found in Supplementary Table S5.
Equation 1: Formula for the calculation of the retention efficiency (RE) based on the effective NPP dose.
Eq. 2 was used to estimate the optimal flow rate for maximum particle retention. Knowledge and/or estimates of the rotor geometry, the medium’s density and viscosity as well as of the NPPs’ diameter and density were required for the calculations.
Equation 2: Formula for the calculation of the optimal flow rate F [m3 s−1] for CFC with ρp: density of the particles [kg m–3], ρm: density of the medium [kg m–3], η: viscosity of the medium [kg m−1 s−1], D: diameter of the particles [m], h: height of the rotor core [m], ω: angular velocity [s−1], rmax: maximum radius of the core [m], rt: radius at top of core [m], rb: radius at bottom of core [m] (Svedberg and Pedersen, 1940; Berman, 1966; Dorin and Cummings, 2015).
Combined uncertainties (uc) (calculated according to the “Guide to the expression of uncertainty in measurement” [GUM (JCGM 100:2008)] comprise instrument replicates (measurement precision), sample replicates (repeatability), experiment replicates using different centrifuge systems (reproducibility) (uc: square root of sums of squared errors), and their propagation in the calculation of the retention efficiencies. If certified values in conjunction with expanded uncertainties are provided, these uncertainties are also considered (Ellison and Williams, 2012).
Four CFC experiments were conducted which focused on the influence of the pump rate on the retention efficiency (1) under simplified conditions and (2) under realistic environmental conditions, (3) the influence of recirculation and (4) the potential of CFC to enable nanometer-size-selective sampling. Except for experiment 4, for which a sequence of two CFC systems was used, all experiments were based on application of one CFC system.
The MWAD protocol proved suitable for complete polymer digestion. The obtained digests were completely clear and the recoveries for the certified metals (As, Cd, Cr, Hg, Pb, Sn, and Zn) ranged from 97.4% ± 1.5% to 103% ± 4% (Supplementary Table S4). Only Sb posed a slight outlier with a recovery of 115.9% ± 1.5% [1 SD (n = 3)].
Measuring 105Pd in helium mode was determined to be optimal in terms of trueness and precision of Pd concentration. Recovery for the ICP-MS standard solution was 100.0% ± 1.2% [n = 4 (1 SD)]. The determined Pd concentration in the working stock NPP suspension (1:10 dilution of the suspension as synthesized) was 22.8 mg L−1 ± 1.3 mg L–1 [uc (n = 12)]. Direct measurements of diluted NPP suspension based on instrument calibration using the Pd standard solution was possible.
The optimized protocol including surfactant and ultrasonication led to good agreement between the calibration and the corresponding dilution series of the NPP suspension (Supplementary Figure S1). Near perfect linearity in the working range was achieved, with R2 ≥ 99.98%. The instrumental LOD was 0.0017 µg L–1 (3 × SD of the Blank) which equated to approximately 3.3 × 108 particles L−1.
Except for the flow rate, all other relevant parameters influencing the retention behavior of particles during CFC depend on either the medium and particles of interest, the angular velocity or the geometry of the used CFC rotor. Thus, the flow rate is the decisive parameter to vary size- and density-selectivity of the applied setup (Eq. 2). The lower the flow is set, the more time the medium needs to pass the centrifugal field and the higher the achievable retention of small particles in the rotor is. Correct adjustment and optimization of the pump rate will ultimately lead to maximum particle removal.
The pump rate was assessed using the known geometry of the rotor, the angular velocity, the density and viscosity of the liquid, and estimating the density of the plastics (details in Supplementary Material). Using assumption of a NPP density between 1.14 g mL−1 and 1.18 g mL−1 and a particle diameter of 160 nm led to ideal estimated pump rates between 2.6 L h–1 and 3.4 L h–1 (Figure 3).
Figure 3. Parabolic relationship (based on Eq. 2) between the flow rates required for quantitative retention and the particle diameter for operation of the CFC Rotor at 17000 rpm with water as medium (for the density range of polyacrylonitrile).
In order to evaluate the fitness of the calculated flow rates according to Equation 2, three different pump rates were tested. The retention efficiency for the NPPs in MQW in the linear setup was 92% ± 8% for 1 L h–1, 67% ± 6% for 2.5 L h–1 and 53% ± 5% for 5 L h–1 [uc (n = 3)] (Table 1). The results show that, despite the calculations, a pump rate of 2.5 L h−1 was not sufficient for quantitative retention, whereas 1 L h−1 led to efficient NPP removal. Nevertheless, as the flow rate of the CFC systems used in these experiments can be adjusted between a few mL h−1 and 66 L h−1, the calculations gave a good estimate which could be further refined experimentally. The gap between the estimations and the experimental findings refers either to a counterflow induced by diffusional drift, which is described by Brownian dynamics (which is applicable to particles <1 µm in diameter) (Meireles et al., 2010; Minoura et al., 2010; de la Torre et al., 2018), or slight deviations of particle density and/or size. The retention efficiency values describe the partitioning of the effective NPP dose between the rotor content and the effluent fraction. Thus, for a pump rate of 5 L h−1, approximately half of the NPPs were retained in the rotor and half passed through. It is important not to confuse the retention efficiency with the overall recovery. For the linear setup, the spiked NPPs could be quantitatively recovered in the three fractions: (1) suspension bottle, (2) rotor content and (3) effluent {85% ± 5% – 98% ± 4% [uc (n = 3)]; Table 1}. This is encouraging, since such high recoveries are not always achieved when working with (sub)micron particles (Imhof et al., 2012; Nguyen et al., 2019).
Table 1. Retention efficiencies and recoveries for the Pd-doped NPPs in the different fractions in the different setup using one CFC system (17000 rpm).
In the circular setup (Figure 1B), the retention efficiency rose to 91% ± 10% for two cycles and 95% ± 8% for three cycles [uc (n = 2)] (Table 1). This is an >40% gain from the linear setup. On the other hand, the overall recoveries were significantly lower in this setup, 60% ± 5% and 68% ± 4% for two and three cycles, respectively. We hypothesize that in this configuration a considerable fraction of the NPPs adhered to the walls of the suspension bottle and/or the tubing, which was only evident when the NPPs spent extended times in the system. Nevertheless, by increasing the number of pumping cycles the retention efficiency of NPPs in the CFC system can be significantly enhanced.
Heteroaggregation of NPPs with inorganic and organic natural matter was observed in various environmental circumstances (Koelmans et al., 2015; Gigault et al., 2018; Oriekhova and Stoll, 2018; Li et al., 2019). Therefore, the influence of suspending the NPPs in water from the German Elbe River (∼1 month incubation time) on the retention efficiency of the CFC system run at 2.5 L h−1 was investigated. Two reasons led to selecting this flow rate instead of 1 L h−1, which had a higher retention in the linear system with one rotor:
1. It poses a tradeoff between the possibility to process larger volumes (necessary for sufficient preconcentration under environmental conditions) and a high retention.
2. It enabled identification of an increase in the retention efficiency due to the formation of larger particles by heteroaggregation (in contrast to a flow rate that already leads to quantitative retention in MQW).
An equal percentage of NPPs were retained for filtrated river water (65% ± 6%) and MQW at 2.5 L h−1 [67% ± 6% (n = 3)] in the linear setup. This indicates that no influence of the natural colloids (i.e., particles <0.2 µm) and humic substances led to formation of larger heteroaggregates. Moreover, the mean retention efficiency was higher for the unfiltered water (75% ± 5%) than for NPPs spiked into the filtered river water.
Longer incubation times and higher concentrations of SPM and dissolved organic substances, whose interactions with different nanoparticles have previously been shown (Grillo et al., 2015; Milne et al., 2017; Wu et al., 2019), could be an explanation for the increase in the retention efficiencies. Future experiments can give further insights into the aggregation kinetics of NPPs with SPM in real-world scenarios. In this way, CFC may be an additional way to assess NPP attachment to larger particles, as particles which are attached to SPM would be efficiently removed in the centrifuge and those which remain unattached would pass it to a higher extent.
The dependence between the flow rates and retention efficiency was demonstrated for NPPs with the same density. However, in an environmental situation, density differences can be used for separation of particles of different materials and sizes. It was calculated, that at 4000 rpm, particles with a diameter of 3 µm and a density >1.01 g mL−1 can be quantitatively retained at flow rates of approximately 4 L h−1 (Supplementary Figure S2). Thus, we hypothesize that in a sequence of two CFC systems, plankton particles (d ≈ 3 µm) should be retained in the first centrifuge at the operative pump rate of 2.5 L h−1. Moreover, the calculations indicate quantitative retention of ∼ 200 nm particles with a density >1.1 g mL−1 at a flow rate of ∼ 3 L h−1 at 17000 rpm in centrifuge 2. Therefore, the separation of the NPPs from the natural particles appeared feasible.
Separation efficiency of the NPPs from natural particles in the micrometer-range was evaluated by using a sequence of two CFC systems with differential rotation speeds between rotors and a pump rate of 2.5 L h−1. A plankton CRM (BCR-414), that is certified for a variety of metals, was used as a model for undesired interfering matrix constituents (high load of organic carbon and minerals). The micron-sized plankton particles were retained in the first rotor as it can be seen in Figure 4 (left panel). Results of this experiment are shown in Table 2.
Figure 4. Left: Rotor 1 (4000 rpm) containing most of the plankton particles. Right: Rotor 2 (17000 rpm) containing primarily NPPs.
Table 2. Retention efficiencies and recoveries for the Pd-doped NPPs and the plankton CRM BCR-414 for a sequential setup of two centrifuges operated at 4000 rpm and 17000 rpm.
According to calculations, the entire free NPPs should have passed the first rotor, as very low flow rates of 0.15 L h−1 to 0.19 L h−1 are theoretically required for NPP retention at 4000 rpm (Supplementary Figure S2). However, only 77% ± 5% of the effective NPP dose passed rotor 1 (23.0% ± 2.2% retention efficiency). The NPPs retained in rotor 1 were likely bound to the SPM in the short amount of time between spiking and passing through the sequential CFF system. The entire contact time between SPM and NPPs was 120 min throughout the whole CFC procedure. 53% ± 5% of NPPs was retained in the second rotor.
In order to estimate the amount of plankton CRM retained by centrifugation, an element which was associated by the CRM was chosen to be measured as a proxy according to the following criteria: (1) no detectable background concentration in the Pd-doped NPPs, (2) negligible background value (contamination) stemming from the CFC setup and MWAD and (3) quantitative cumulative recovery. Based on these requirements, Ni was chosen to track the plankton CRM through the separation process. Accordingly, 89% ± 9% (n = 12) of BCR-414 were retained in rotor 1, whereas 3% ± 4% and 2% ± 4% (probably dissolved Ni) were recovered in rotor 2 and the effluent (Recoverytotal = 113% ± 12%). The ratio of the Ni mass fractions detected in Rotor 1 and Rotor 2 was >30.
The lab-scale study emphasizes the potential of a sequence of CFC systems for separation of the micron fraction from the colloidal fraction (Figure 5). The results show CFC’s potential for the preconcentration of colloidal plastic particles, which is of high importance for the future detection of NPPs in environmental aquatic systems. For mechanistic experiments, the Pd-labeled particles served as a valuable model to accurately show the high NPP retention rates under optimal conditions. However, in practice, polymer-specific techniques such as Py-GC-MS or TED-GC-MS (Dümichen et al., 2017; Fischer and Scholz-Böttcher, 2017) would be additionally needed to quantify the enriched colloidal plastic particle sample.
Figure 5. Schematic explanation of the extended utility of the sequential CFC setup as a tool for size- and density selective MP and nanoplastic sampling (modified, © Thermo Scientific).
This study focused on the development of a feasible separation and preconcentration method for NPPs, further optimization of NPP quantification was beyond the scope of this study. Nevertheless, some additional sample preparation steps can be recommended. As the CFC enrichment technique would lead to pre-concentration of both NPPs and natural organic colloids contained in environmental waters, purification (i.e., organic matrix digestion) protocols will be required to better isolate NPPs from this heterogeneous mixture (Hurley et al., 2018). The experiments have shown a tendency that heteroaggregation can occur quickly. If only one rotor is used, natural micro- and nanoparticles, MP, free colloidal plastic particles and NPPs included in micron-sized heteroaggregates will be sampled as a mixture.
The sequential CFC setup opens up the possibility to fractionate different sizes of plastic particles and natural colloids. Figure 5 visually expands on this concept. To sample particles in the desired size range from natural waters, the rotational speeds of both centrifuges have to be coordinated. For example, at a flow rate of 2.5 L h−1, adjusting CFC 1 to 2000 rpm would quantitatively remove particles of ≥3 µm with a density >1.02 g mL– and particles ≥1 µm with a density >1.24 g mL−1. One striking advantage would be the additional removal of all particles ≥500 nm with a density ≥2 g mL−1 (online density separation), which includes all minerals but no polymers of interest (Schön, 2011). As SPM contains a high weight fraction of small sediment particles (Imhof et al., 2012; Vidmar et al., 2017), which cannot be removed by oxidative treatments, samples obtained by filtration approaches usually have to be subjected to density separation before their analysis.
Results indicate a linear CFC setup may be preferable over the circular CFC approach for environmental samples. The natural water can be pumped through the CFC directly on-site without any storage in a container where particle sedimentation and adhesion to walls may occur. A second important argument for omitting an intermediary container is minimizing contamination, which is a tremendous issue for research on particulate plastic (Löder and Gerdts, 2015; Wesch et al., 2017; Hermsen et al., 2018). Furthermore, using a rotor of titanium in conjunction with perfluorinated sealing and tubing keeps the potential contamination to a minimum.
The centrifuge can be run in the continuous operation mode for multiple days with ease. In contrast to filtration approaches, no permanent observation is necessary. Operation of the centrifuge for 5 days at a pump rate of 5 L h−1 would enable time-integrated sampling of 600 L of source water. Thus, the larger volumes which can be processed compared to ultracentrifugation (10 mL – 100 mL) and the avoidance of membrane blockages compared to ultrafiltration are the main advantages of CFC compared to other centrifugation techniques (De Bruijn et al., 2005; Schwaferts et al., 2019; Enfrin et al., 2020).
The application in the field has already been tested for MP sampling (Hildebrandt et al., 2019). Due to the system’s weight (>100 kg), operation on a stationary basis is beneficial. In order to enrich NPPs from water, which presumably contains very low amounts of NPPs, it may be necessary to implement a high-performance CFC with ∼100,000 g (compared to ∼24,000 g) to enable higher sampling flow rates (e.g., CF-32-Ti, Beckman Coulter, Brea, United States).
As the proof-of-principle has been made, CFC can be tested in even more demanding scenarios. Though application to natural waters is still hypothetical due to challenges arising from other stages of the analytical workflow (e.g., chemical identification of the polymers), the authors would suggest the sequential use of two CFC systems. MP and micron-sized matrix particles would be retained in the first centrifuge, as well as mineral particles down to a few hundred nanometers. In the second rotor, colloidal particles with a density <2 g mL−1 could further be enriched. Waters which contain a high concentration of particulate matter, such as water from wastewater treatment plants or street run-off, should be subjected to CFC sampling first. Subsequently, evaluation of the degree of preconcentration necessary to meet the LOD of e.g., TED-GC-MS, to which the freeze-dried sample could be subjected directly, has to be conducted. This requires further method development with respect to both nanoplastic-specific sample preparation and thermoanalytical detection.
There is high public, political and scientific interest in environmental plastic pollution, but the smaller the polymer pieces are, the greater the analytical challenges become. This leaves severe data gaps in understanding the occurrence, concentration and identity of nanoplastics and finally their impact on the environment.
In exposure studies, the colloidal plastic fraction was shown to potentially be the most hazardous (Koelmans et al., 2015; Burns and Boxall, 2018). However, a meaningful ecological risk assessment also requires knowledge of relevant exposure levels. As of yet, crossflow ultrafiltration was considered the only technique suitable for nanometer-size-selective sampling of representative volumes. Our results prove that continuous flow centrifugation (CFC) is a valuable alternative for nanoplastic sampling and enrichment, as it does not use a polymer membrane, features a high retention of NPPs and can be used for time-integrated sampling of several hundred liters of source water (Hildebrandt et al., 2019). The proof-of-principle-experiments presented here, in conjunction with expanded theoretical calculations underpin the techniques’ potential for size- and density-selective preconcentration of colloidal plastic particles. >90% retention of model NPPs (d ≈ 160 nm) at a relatively low flow rate in MQW (1 L h−1) and good retention efficiencies (>75%) in river water at higher flow rates (2.5 L h−1) can be achieved. High-performance CFC systems (>100,000 g) (Dorin and Cummings, 2015) may enable higher water throughput with considerably greater flow rates. The scientific community already knows a lot about macro and microplastic pollution (Lorenz et al., 2019), but to successfully answer the question “Where is all the plastic?” (Thompson et al., 2004) it is crucial to also understand the distribution and fate of NPPs.
All datasets generated for this study are included in the article/Supplementary Material.
LH has conducted the major part or the experimental work and the data evaluation and the writing. DM has synthesized and provided the Pd-doped-nanoplastic as an analytical key element of the experiments and substantially contributed to the planning of the experimental setup and the experiments. TZ has substantially contributed to the planning of the experiments and with the instrumental analysis as well as data evaluation. DP has considerably contributed to the experimental design and conception of the manuscript. DM, TZ, and DP have also extensively helped with revising and proof-reading the manuscript. All authors contributed to the article and approved the submitted version.
DM was supported by the Swiss National Science Foundation, Ambizione Grant number PZP002_168105.
The authors declare that the research was conducted in the absence of any commercial or financial relationships that could be construed as a potential conflict of interest.
The Supplementary Material for this article can be found online at: https://www.frontiersin.org/articles/10.3389/fenvs.2020.00089/full#supplementary-material
Anderson, K. P., Low, M.-A. L., Lie, Y. S., Keller, G.-A., and Dinowitz, M. (1991). Endogenous origin of defective retroviruslike particles from a recombinant Chinese hamster ovary cell line. Virology 181, 305–311. doi: 10.1016/0042-6822(91)90496-x
Bannick, C. G., Szewzyk, R., Ricking, M., Schniegler, S., Obermaier, N., Barthel, A. K., et al. (2019). Development and testing of a fractionated filtration for sampling of microplastics in water. Water Res. 149, 650–658. doi: 10.1016/j.watres.2018.10.045
Berman, A. S. (1966). The Development of Zonal Centrifuges and Ancillary Systems for Tissue Fractionation and Analysis. Washington, DC: U. S. Government Printing Office.
Besley, A. (2017). A standardized method for sampling and extraction methods for quantifying microplastics in beach sand. Mar. Pollut. Bull. 114, 77–83. doi: 10.1016/j.marpolbul.2016.08.055
Bhattacharya, P., Lin, S., Turner, J., and Ke, C. (2010). Physical adsorption of charged plastic nanoparticles affects algal photosynthesis. J. Phys. Chem. C 114, 16556–16561. doi: 10.1021/jp1054759
Brown, R. I. (1989). The physics of continuous flow centrifugal cell separation. Artif. Organs 13, 4–20. doi: 10.1111/j.1525-1594.1989.tb02827.x
Burns, E. E., and Boxall, A. B. A. (2018). Microplastics in the aquatic environment: evidence for or against adverse impacts and major knowledge gaps. Environ. Toxicol. Chem. 37, 2776–2796. doi: 10.1002/etc.4268
Cacace, M. G., Nucci, R., and Reckert, H. (1977). Large scale preparation of calf liver nuclei by continuous flow centrifugation. Experientia 33, 855–857. doi: 10.1007/bf01951241
Cole, M., Lindeque, P., Fileman, E., Halsband, C., Goodhead, R., Moger, J., et al. (2013). Microplastic ingestion by zooplankton. Science 47, 6646–6655. doi: 10.1021/es400663f
Conn, K. E., Dinicola, R. S., Black, R. W., Cox, S. E., Sheibley, R. W., Foreman, J. R., et al. (2016). “Continuous-flow centrifugation to collect suspended sediment for chemical analysis,” in Techniques and Methods, Plus Appendixes, (Reston, VA: U.S. Geological Survey), 1–D6. doi: 10.3133/tm1D6
Correia, M., and Loeschner, K. (2018). Detection of nanoplastics in food by asymmetric flow field-flow fractionation coupled to multi-angle light scattering: possibilities, challenges and analytical limitations. Analyt. Bioanalyt. Chem. 410, 5603–5615. doi: 10.1007/s00216-018-0919-8
De Bruijn, J. P. F., Salazar, F. N., and Bórquez, R. (2005). Membrane blocking in ultrafiltration: a new approach to fouling. Food Bioprod. Proc. 83, 211–219. doi: 10.1205/fbp.04012
de la Torre, J. G., Cifre, J. G. H., and Peña, A. I. D. (2018). Prediction and analysis of analytical ultracentrifugation experiments for heterogeneous macromolecules and nanoparticles based on Brownian dynamics simulation. Eur. Biophys. J. 47, 845–854. doi: 10.1007/s00249-018-1322-2
Dorin, M., and Cummings, J. (2015). Principles of Continuous Flow Centrifugation. Technical Application Note. Indianapolis, IN: Beckman Coulter Lifer Sciences.
Douglas, G. B., Beckett, R., and Hart, B. T. (1993). Fractionation and concentration of suspended particulate matter in natural waters. Hydrol. Process. 7, 177–191. doi: 10.1002/hyp.3360070208
Dümichen, E., Eisentraut, P., Bannick, C. G., Barthel, A.-K., Senz, R., and Braun, U. (2017). Fast identification of microplastics in complex environmental samples by a thermal degradation method. Chemosphere 174, 572–584. doi: 10.1016/j.chemosphere.2017.02.010
Ekvall, M. T., Lundqvist, M., Kelpsiene, E., Šileikis, E., Gunnarsson, S. B., and Cedervall, T. (2019). Nanoplastics formed during the mechanical breakdown of daily-use polystyrene products. Nanoscale Adv. 1, 1055–1061. doi: 10.1039/c8na00210j
Ellison, S. L. R., and Williams, A. (2012). Quantifying Uncertainty in Analytical Measurement, 3rd Edn, Washington, DC: CITAC.
Enders, K., Lenz, R., Stedmon, C. A., and Nielsen, T. G. (2015). Abundance, size and polymer composition of marine microplastics ≥ 10μm in the Atlantic Ocean and their modelled vertical distribution. Mar. Pollut. Bull. 100, 70–81. doi: 10.1016/j.marpolbul.2015.09.027
Enfrin, M., Lee, J., Le-Clech, P., and Ludovic, D. (2020). Kinetic and mechanistic aspects of ultrafiltration membrane fouling by nano- and microplastics. J. Memb. Sci. 601:117890. doi: 10.1016/j.memsci.2020.117890
Fischer, M., and Scholz-Böttcher, B. M. (2017). Simultaneous trace identification and quantification of common types of microplastics in environmental samples by pyrolysis-gas chromatography–mass spectrometry. Environ. Sci. Technol. 51, 5052–5060. doi: 10.1021/acs.est.6b06362
Frias, J., Filgueiras, A., Gago, J., Pedrotti, M. L., Suaria, G., Tirelli, V., et al. (2019). Standardised protocol for monitoring microplastics in seawater. Techincal Report. doi: 10.13140/RG.2.2.14181.45282
Gago, J., Galgani, F., Maes, T., and Thompson, R. C. (2016). Microplastics in seawater: recommendations from the marine strategy framework directive implementation process. Front. Mar. Sci. 3:219. doi: 10.3389/fmars.2016.00219
Gigault, J., Ter Halle, A., Baudrimont, M., Pascal, P.-Y., Gauffre, F., Phi, L., et al. (2018). Current opinion: what is a nanoplastic? Environ. Pollut. 235, 1030–1034. doi: 10.1016/j.envpol.2018.01.024
Goodenough, D. A. (1974). Bulk isolation of mouse hepatocyte gap junctions : characterization of the principal protein, connexin. J. Cell Biol. 61, 557–563. doi: 10.1083/jcb.61.2.557
Grillo, R., Rosa, A. H., and Fraceto, L. F. (2015). Engineered nanoparticles and organic matter: a review of the state-of-the-art. Chemosphere 119, 608–619. doi: 10.1016/j.chemosphere.2014.07.049
Heraeus Instruments GmbH (1998). Continuous Flow Rotors 3049 (Titanium) and 3054 (Aluminium). Hanau: Heraeus.
Hermsen, E., Mintenig, S. M., Besseling, E., and Koelmans, A. A. (2018). Quality criteria for the analysis of microplastic in biota samples: a critical review. Environ. Sci. Technol. 52, 10230–10240. doi: 10.1021/acs.est.8b01611
Hernandez, L. M., Yousefi, N., and Tufenkji, N. (2017). Are there nanoplastics in your personal care products? Environ. Sci. Technol. Lett. 4, 280–285. doi: 10.1021/acs.estlett.7b00187
Hildebrandt, L., Voigt, N., Zimmermann, T., Reese, A., and Proefrock, D. (2019). Evaluation of continuous flow centrifugation as an alternative technique to sample microplastic from water bodies. Mar. Environ. Res. 151:104768. doi: 10.1016/j.marenvres.2019.104768
Hüffer, T., Praetorius, A., Wagner, S., Von Der, Kammer, F., and Hofmann, T. (2017). Microplastic exposure assessment in aquatic environments: learning from similarities and differences to engineered nanoparticles. Environ. Sci. Technol. 51, 2499–2507. doi: 10.1021/acs.est.6b04054
Hurley, R. R., Lusher, A. L., Olsen, M., and Nizzetto, L. (2018). Validation of a method for extracting microplastics from complex, organic-rich, environmental matrices. Environ. Sci. Technol. 52, 7409–7417. doi: 10.1021/acs.est.8b01517
Imhof, H. K., Schmid, J., Niessner, R., Ivleva, N. P., and Laforsch, C. (2012). A novel, highly efficient method for the separation and quantification of plastic particles in sediments of aquatic environments. Limnol. Oceanogr. Methods 10, 524–537. doi: 10.4319/lom.2012.10.524
Ivleva, N. P., Wiesheu, A. C., and Niessner, R. (2017). Microplastic in aquatic ecosystems. Angew. Chem. Intern. Edn. 56, 1720–1739. doi: 10.1002/anie.201606957
Jeong, C.-B., Kang, H.-M., Lee, Y. H., Kim, M.-S., Lee, J.-S., Seo, J. S., et al. (2018). Nanoplastic ingestion enhances toxicity of persistent organic pollutants (POPs) in the monogonont rotifer brachionus koreanus via multixenobiotic resistance (MXR) disruption. Environ. Sci. Technol. 52, 11411–11418. doi: 10.1021/acs.est.8b03211
Kahane, I., Furthmayr, H., and Marchesi, V. T. (1976). Isolation of membrane glycoproteins by affinity chromatography in the presence of detergents. Biochim. Biophys. Acta Biomemb. 426, 464–476. doi: 10.1016/0005-2736(76)90391-6
Käppler, A., Fischer, D., Oberbeckmann, S., Schernewski, G., Labrenz, M., Eichhorn, K.-J., et al. (2016). Analysis of environmental microplastics by vibrational microspectroscopy: FTIR, Raman or both? Analyt. Bioanalyt. Chem. 408, 8377–8391. doi: 10.1007/s00216-016-9956-3
Keller, A., Jimenez-Martinez, J., and Mitrano, D. (2019). Transport of nano- and microplastic through unsaturated porous media from sewage sludge application. Environ. Sci. Technol. 54, 911–920. doi: 10.1021/acs.est.9b06483
Koelmans, A. A. (2019). Proxies for nanoplastic. Nat. Nanotechnol. 14, 307–308. doi: 10.1038/s41565-019-0416-z
Koelmans, A. A., Besseling, E., and Shim, W. J. (2015). “Nanoplastics in the aquatic environment. critical review,” in Marine Anthropogenic Litter, eds M. Bergmann, L. Gutow, and M. Klages (Cham: Springer International Publishing), 325–340. doi: 10.1007/978-3-319-16510-3_12
Lambert, S., and Wagner, M. (2016). Characterisation of nanoplastics during the degradation of polystyrene. Chemosphere 145, 265–268. doi: 10.1016/j.chemosphere.2015.11.078
Lehner, R., Weder, C., Petri-Fink, A., and Rothen-Rutishauser, B. (2019). Emergence of nanoplastic in the environment and possible impact on human health. Environ. Sci. Technol. 53, 1748–1765. doi: 10.1021/acs.est.8b05512
Lenz, R., and Labrenz, M. (2018). Small microplastic sampling in water: development of an encapsulated filtration device. Water 10:1055. doi: 10.3390/w10081055
Li, Y., Wang, X., Fu, W., Xia, X., Liu, C., Min, J., et al. (2019). Interactions between nano/micro plastics and suspended sediment in water: Implications on aggregation and settling. Water Res. 161, 486–495. doi: 10.1016/j.watres.2019.06.018
Löder, M. G. J., and Gerdts, G. (2015). “Methodology used for the detection and identification of microplastics—a critical appraisal,” in Marine Anthropogenic Litter, eds M. Bergmann, L. Gutow, and M. Klages (Cham: Springer International Publishing), 201–227. doi: 10.1007/978-3-319-16510-3_8
Lorenz, C., Roscher, L., Meyer, M. S., Hildebrandt, L., Prume, J., Löder, M. G. J., et al. (2019). Spatial distribution of microplastics in sediments and surface waters of the southern North Sea. Environ. Pollut. 252, 1719–1729. doi: 10.1016/j.envpol.2019.06.093
Lusher, A. L., Welden, N. A., Sobral, P., and Cole, M. (2017). Sampling, isolating and identifying microplastics ingested by fish and invertebrates. Analyt. Methods 9, 1346–1360. doi: 10.1039/c6ay02415g
Mattsson, K., Hansson, L.-A., and Cedervall, T. (2015). Nano-plastics in the aquatic environment. Environ. Sci. 17, 1712–1721. doi: 10.1039/c5em00227c
Meireles, M., Bourgeois, F., Tourbin, M., Guiraud, P., and Frances, C. (2010). Review: Removal of oversize & recovery of particles from suspensions in the nano size range. [Research Report] CNRS. hal-01186033.
Meyns, M., Primpke, S., and Gerdts, G. (2019). Library based identification and characterisation of polymers with nano-FTIR and IR-sSNOM imaging. arXiv [Preprint], Available online at: https://ui.adsabs.harvard.edu/abs/2019arXiv190610243M (accessed June 01, 2019).
Milne, C. J., Lapworth, D. J., Gooddy, D. C., Elgy, C. N., and Valsami-Jones, É (2017). Role of humic acid in the stability of ag nanoparticles in suboxic conditions. Environ. Sci. Technol. 51, 6063–6070. doi: 10.1021/acs.est.6b06054
Minoura, I., Katayama, E., Sekimoto, K., and Muto, E. (2010). One-dimensional brownian motion of charged nanoparticles along microtubules: a model system for weak binding interactions. Biophys. J. 98, 1589–1597. doi: 10.1016/j.bpj.2009.12.4323
Mintenig, S. M., Bäuerlein, P. S., Koelmans, A. A., Dekker, S. C., and Van Wezel, A. P. (2018). Closing the gap between small and smaller: towards a framework to analyse nano- and microplastics in aqueous environmental samples. Environ. Sci. Nano 5, 1640–1649. doi: 10.1039/c8en00186c
Mitrano, D. M., Beltzung, A., Frehland, S., Schmiedgruber, M., Cingolani, A., and Schmidt, F. (2019). Synthesis of metal-doped nanoplastics and their utility to investigate fate and behaviour in complex environmental systems. Nat. Nanotechnol. 14, 362–368. doi: 10.1038/s41565-018-0360-3
Nguyen, B., Claveau-Mallet, D., Hernandez, L. M., Xu, E. G., Farner, J. M., and Tufenkji, N. (2019). Separation and analysis of microplastics and nanoplastics in complex environmental samples. Acc. Chem. Res. 52, 858–866. doi: 10.1021/acs.accounts.8b00602
Oriekhova, O., and Stoll, S. (2018). Heteroaggregation of nanoplastic particles in presence of inorganic colloids and natural organic matter. Environ. Sci. Nano 5, 792–799. doi: 10.1039/c7en01119a
Peng, J., Wang, J., and Cai, L. (2017). Current understanding of microplastics in the environment: occurrence, fate, risks, and what we should do. Integr. Environ. Assess. Manag. 13, 476–482. doi: 10.1002/ieam.1912
Primpke, S., Lorenz, C., Rascher-Friesenhausen, R., and Gerdts, G. (2017). An automated approach for microplastics analysis using focal plane array (FPA) FTIR microscopy and image analysis. Analyt. Methods 9, 1499–1511. doi: 10.1039/c6ay02476a
Ran, Y., Fu, J. M., Sheng, G. Y., Beckett, R., and Hart, B. T. (2000). Fractionation and composition of colloidal and suspended particulate materials in rivers. Chemosphere 41, 33–43. doi: 10.1016/s0045-6535(99)00387-2
Rochman, C. M., Cook, A.-M., and Koelmans, A. A. (2016). Plastic debris and policy: using current scientific understanding to invoke positive change. Environ. Toxicol. Chem. 35, 1617–1626. doi: 10.1002/etc.3408
Schmiedgruber, M., Hufenus, R., and Mitrano, D. M. (2019). Mechanistic understanding of microplastic fiber fate and sampling strategies: synthesis and utility of metal doped polyester fibers. Water Res. 155, 423–430. doi: 10.1016/j.watres.2019.02.044
Schön, J. H. (2011). “Chapter 4 - density,” in Handbook of Petroleum Exploration and Production, ed. J. H. Schön (Amsterdam: Elsevier), 97–105.
Schür, C., Rist, S., Baun, A., Mayer, P., Hartmann, N., and Wagner, M. (2019). When fluorescence is not a particle: the tissue translocation of microplastics in Daphnia magna seems an artIfact. Environ. Toxicol. Chem. 38, 1495–1503. doi: 10.1002/etc.4436
Schwaferts, C., Niessner, R., Elsner, M., and Ivleva, N. P. (2019). Methods for the analysis of submicrometer- and nanoplastic particles in the environment. TrAC Trends Analyt. Chem. 112, 52–65. doi: 10.1016/j.trac.2018.12.014
Science Advice for Policy by European Academies (2019). A Scientific Perspective on Microplastics in Nature and Society. Berlin: SAPEA. doi: 10.26356/microplastics
Shibley, G. P., Manousos, M., Munch, K., Zelljadt, I., Fisher, L., Mayyasi, S., et al. (1980). New method for large-scale growth; and concentration of the Epstein-Barr viruses. Appl. Environ. Microbiol. 40, 1044–1048.
Shim, W. J., Hong, S. H., and Eo, S. E. (2017). Identification methods in microplastic analysis: a review. Analyt. Methods 9, 1384–1391. doi: 10.1039/c6ay02558g
Stöven, K., Jacobs, F., and Schnug, E. (2015). Microplastic: a selfmade environmental problem in the plastic age. J. Fur Kulturpflanzen 67, 241–250. doi: 10.5073/JFK.2015.07.01
Sullivan, K. D., and Gugliada, V. (2018). Fluorescence photobleaching of microplastics: a cautionary tale. Mar. Pollut. Bull. 133, 622–625. doi: 10.1016/j.marpolbul.2018.06.019
Ter Halle, A., Jeanneau, L., Martignac, M., Jardé, E., Pedrono, B., Brach, L., et al. (2017). Nanoplastic in the North Atlantic subtropical gyre. Environ. Sci. Technol. 51, 13689–13697. doi: 10.1021/acs.est.7b03667
Thompson, R. C., Olsen, Y., Mitchell, R. P., Davis, A., Rowland, S. J., John, A. W. G., et al. (2004). Lost at sea: where is all the plastic? Science 304, 838–838. doi: 10.1126/science.1094559
Turk, E., Teplow, D. B., Hood, L. E., and Prusiner, S. B. (1988). Purification and properties of the cellular and scrapie hamster prion proteins. Eur. J. Biochem. 176, 21–30. doi: 10.1111/j.1432-1033.1988.tb14246.x
Vidmar, J., Zuliani, T., Novak, P., Drinčić, A., Ščančar, J., and Milačič, R. (2017). Elements in water, suspended particulate matter and sediments of the Sava River. J. Soils Sediments 17, 1917–1927. doi: 10.1007/s11368-016-1512-4
Wesch, C., Elert, A., Wörner, M., Braun, U., Klein, R., and Paulus, M. (2017). Assuring quality in microplastic monitoring: about the value of clean-air devices as essentials for verified data. Sci. Rep. 7:5424. doi: 10.1038/s41598-017-05838-4
Wheeler, C. M., Robertson, B. H., Van Nest, G., Dina, D., Bradley, D. W., and Fields, H. A. (1986). Structure of the hepatitis a virion: peptide mapping of the capsid region. J. Virol. 58, 307–313. doi: 10.1128/jvi.58.2.307-313.1986
Wu, J., Jiang, R., Lin, W., and Ouyang, G. (2019). Effect of salinity and humic acid on the aggregation and toxicity of polystyrene nanoplastics with different functional groups and charges. Environ. Pollut. 245, 836–843. doi: 10.1016/j.envpol.2018.11.055
Xu, M., Halimu, G., Zhang, Q., Song, Y., and Fu, X. (2019). Internalization and toxicity: a preliminary study of effects of nanoplastic particles on human lung epithelial cell. Sci. Total Environ. 694:133794. doi: 10.1016/j.scitotenv.2019.133794
Keywords: metal-doped nanoplastic, nanoplastic sampling, nanoplastic separation, nanoplastic enrichment, submicrometer plastic, plastic contaminants, particulate plastic
Citation: Hildebrandt L, Mitrano DM, Zimmermann T and Pröfrock D (2020) A Nanoplastic Sampling and Enrichment Approach by Continuous Flow Centrifugation. Front. Environ. Sci. 8:89. doi: 10.3389/fenvs.2020.00089
Received: 28 February 2020; Accepted: 02 June 2020;
Published: 30 June 2020.
Edited by:
John Crusius, United States Geological Survey, United StatesReviewed by:
Jes Vollertsen, Aalborg University, DenmarkCopyright © 2020 Hildebrandt, Mitrano, Zimmermann and Pröfrock. This is an open-access article distributed under the terms of the Creative Commons Attribution License (CC BY). The use, distribution or reproduction in other forums is permitted, provided the original author(s) and the copyright owner(s) are credited and that the original publication in this journal is cited, in accordance with accepted academic practice. No use, distribution or reproduction is permitted which does not comply with these terms.
*Correspondence: Daniel Pröfrock, ZGFuaWVsLnByb2Vmcm9ja0BoemcuZGU=
Disclaimer: All claims expressed in this article are solely those of the authors and do not necessarily represent those of their affiliated organizations, or those of the publisher, the editors and the reviewers. Any product that may be evaluated in this article or claim that may be made by its manufacturer is not guaranteed or endorsed by the publisher.
Research integrity at Frontiers
Learn more about the work of our research integrity team to safeguard the quality of each article we publish.