- 1Centre for Aquatic Environments, University of Brighton, Brighton, United Kingdom
- 2r3 Environmental Technology Ltd., Reading, United Kingdom
- 3School of Geography, Queen Mary University of London, London, United Kingdom
- 4Institute of Agriculture and Environmental Sciences, Estonian University of Life Sciences, Tartu, Estonia
- 5Program Advisor for Inter-State Technology and Regulatory Council Sustainable Remediation and Resilience Team, Oakland, CA, United States
- 6School of Ocean and Earth Science, National Oceanography Centre (Southampton), University of Southampton, Southampton, United Kingdom
The sustainable management of post-industrial coasts is a major emerging issue globally. Along such coasts, there may be a significant legacy of both contaminated land (including historic landfills and non-managed waste disposal) and contaminated sediments in and around urban and industrial areas, which require new strategies for cost-effective and integrated risk management under future sea-level rise and climate change scenarios. Here, we review current approaches to managing contamination in post-industrial coastlines, discuss emerging integrated management strategies (building on low input approaches to sustainable brownfields regeneration) and present an approach and framework for assessing and comparing different scenarios for coastal brownfield regeneration to soft re-use and other end-points. This framework can be applied to explore the opportunities for synergy and realization of wider environmental, economic and societal benefits between coastal protection, dredged material re-use and the management of brownfield land. As such, the approach we propose supports planning and options appraisal to realize maximum benefit and value from integrated coastal management strategies.
Introduction
Sea-level rise and the increasing magnitude and frequency of coastal storm surges, and consequent impacts (including coastal erosion, and saline flooding or inundation of urban areas and coastal assets), are major challenges for the sustainable development of coastal areas and communities over the 21st century. Many coastlines in areas subject to former extensive urban and industrial development (post-industrial coasts) contain a significant legacy of brownfield land1, including areas of land contaminated with a range of toxic organic, inorganic and microbial (and other) contaminants, and land areas which have been subject to historic landfill and unmanaged waste disposal (Brand et al., 2017; O’Connor et al., 2019). Erosion, reworking and remobilization of contaminants, solid particulates, debris (e.g., asbestos), pathogens and plastics from this land and solid waste material could pose a significant risk to human and ecological health. For each individual site, contaminant load may not be significant. However, on regional, national and global scales the problem may be underestimated (O’Shea et al., 2018). For example, in England there are >1200 vulnerable coastal landfills with 1 in 10 of these sites at risk of erosion over the next few decades (Brand et al., 2017) and more widely across Europe, there are ca. 10,000 historic landfills containing industrial, domestic and hazardous waste in coastal and riparian areas prone to flooding and/or erosion (Wille, 2018).
In addition, industrialized coasts and estuaries may host significant volumes of historically contaminated subtidal and intertidal sediments (Vane et al., 2015). These sediments may cause continuing contamination of coastal systems even after primary contaminant discharges have ceased, due to advective sediment mixing and supply of reworked, secondary contamination from erosion of contaminated sediments elsewhere along the coast or estuary (e.g., Machado et al., 2016; Cundy and Croudace, 2017; Premier et al., 2019). This diffuse legacy pollution is considered to be one of the major causes for the United Kingdom’s rivers and transitional waters failing ecological and chemical water quality standards (Defra, 2012). Removal or dredging, and subsequent safe disposal or beneficial re-use, of these contaminated sediments pose major ongoing challenges. These challenges may be particularly severe where dredged sediments are required for beach nourishment, land reclamation and land raising as part of climate change adaptation strategies. In addition, an expected change in coastal erosion and sedimentation patterns, including an increased frequency of extreme events, may mean that more reactive dredging is needed than currently required, whereas in other cases proactive dredging may be more appropriate to deal with the implications of long-term seasonal changes in flow (Hakstege, 2013).
Achieving sustainability had been considered an integral outcome of brownfields or contaminated site management (including at coastal sites) since its inception in the 1970s, as through this process under-utilized or damaged land was returned to the land use cycle, avoiding greenfield use (Bardos et al., 2016). From the late 1990s however this assumption began to be questioned, in recognition of the realization that poorly selected, designed or implemented remediation and site management activities may in fact cause greater impact than the contamination or other land issues that they seek to address. This has led to an emerging international literature and (recently) consensus on sustainable remediation, which has focused on promoting “the use of more sustainable practices during environmental clean-up activities, with the objective of balancing economic viability, conservation of natural resources and biodiversity, and the enhancement of the quality of life in surrounding communities” (Bardos et al., 2016; International Organization for Standardization [ISO], 2017). For coastal brownfields (that are often fragmented, may be sited in post-industrial areas subject to declining property values, and may be at real or perceived risk of flooding or erosion) remediation and regeneration for so-called “hard” re-use (e.g., housing or infrastructure developments) may be problematic. Indeed Leger et al. (2016) noted that economic circumstances and frequent policy shifts have impeded the redevelopment of brownfield land in coastal areas, and there is a need for new imaginative approaches that will help coastal communities realize the undoubted benefits of redevelopment of brownfield sites.
One such set of approaches are those combining risk management with nature-based approaches or “soft” re-use (e.g., redevelopment of brownfield as green space, habitat, or for biomass and other natural product generation). This includes the use of so-called low input or gentle remediation approaches: “risk management strategies or technologies involving plant (phyto-), fungi (myco-), and/or bacteria-based methods that result in a net gain (or at least no gross reduction) in soil function as well as effective risk management” (Cundy et al., 2016). Soft re-use has historically tended to be overlooked in brownfields management (Bardos et al., 2016). However, in response to the sustainable development vision, there is a broad agreement among stakeholders that soft re-use of brownfields can bring significant environmental, societal and economic benefits (Bardos et al., 2011, 2016; Cundy et al., 2016), as evidenced by a number of case studies and practical applications (e.g., Cundy et al., 2013, 2016; Li et al., 2019). The soft re-use approach also broadly aligns with an emergence and increased utilization of soft-engineering coastal management approaches such as managed realignment and other habitat recreation, which offer potential synergies (or possibly conflicts in some situations) with brownfields and contaminated sediments management.
Here, we present an approach and framework for assessing and comparing different scenarios for coastal brownfield regeneration to soft re-use and other end-points, based around “sustainability linkages,” which draws on concepts and case studies from sustainable remediation and low input approaches to sustainable brownfields regeneration. Following a review of contemporary responses and challenges to the management of contaminated land and sediments in and around coastal urban and industrial areas, we examine (and present examples of) the potential synergies and wider benefits from integrated low input approaches. We then present and discuss the sustainability linkages approach, based on sustainability assessment criteria produced by the UK Sustainable Remediation Forum (SuRF-UK), as a potential planning and decisional aid to support integrated and sustainable management of post-industrial coasts.
Contemporary Responses and Challenges
Historically estuaries and coasts have frequently been areas for waste disposal due to their proximity to industrial and commercial centers, the perceived low value of coastal wetland habitats, and due to the ability of tidal flushing to rapidly dilute and disperse contaminants so reducing acute impact. This occurred through either the use of adjacent land for landfilling of solid wastes and industrial activities or the discharge of contaminated effluent streams and particulates. There are potentially millions of landfill and waste disposal sites along coastlines globally that are at risk of marine inundation, erosion or catastrophic failure. Most of these pre-date environmental regulation developed in the latter half of the 20th Century with little attempt to isolate solid, liquid or gaseous contaminants from the surrounding environment and these are generally described as “historic” or “legacy” landfills (Brand et al., 2017). In lower and middle income economies this is of even greater concern. Rapid economic development has been accompanied by increasing waste production and globally solid wastes are being generated faster than any other environmental pollutant (Hoornweg et al., 2013). Lack of regulation and poor infrastructure means many solid waste disposal sites are frequently uncontrolled (Gupta et al., 2015; Gu et al., 2017) and “open” waste sites are a major source of marine litter, contaminate water bodies through the release of wide-ranging wastes and pollution (e.g., metals, batteries, tires) and present significant human health risk (Ferronato and Torretta, 2019). This has recently been recognized as a significant issue in the management of plastic debris, where mismanaged land-based wastes are estimated to contribute significantly to marine plastics debris inputs and without significant infrastructure improvements to waste management, the cumulative release of plastics will increase by an order of magnitude by 2025 (Jambeck et al., 2015; Waldschläger et al., 2020). For 2010, the top 10 countries ranked by mass of mismanaged plastic waste were China, Indonesia, the Philippines, Vietnam, Sri Lanka, Thailand, Egypt, Malaysia, Nigeria, and Bangladesh. Apart from Egypt and Malaysia, these countries all showed a percentage of mismanaged waste exceeding 75% (as a proportion of total waste), with a number handling additional waste imported for processing from Western nations. Historic and uncontrolled waste disposal sites present two significant potential contaminant pathways to the marine environment that need to be managed. Firstly, as solid wastes degrade, soluble contaminants (leachates) migrate through the waste and surrounding inter-tidal sediments before entering groundwater and the marine environment, and secondly, solid particulate wastes can be released directly to coastal waters.
As a means of managing soluble leachates there is an assumption than contaminant impact would be mitigated via natural attenuation through either the precipitation of contaminants in anoxic inter-tidal sediments and/or sorption to fine-grained minerogenic and organic material (Michalak and Kitanidis, 2002; Njue et al., 2012). The release of any remaining soluble contaminants in the leachate plume present above natural background would then be diluted by tidal flushing. However, there is evidence that this “do nothing” approach has resulted in localized “hot spots” of estuarine sediment contamination (Cox and Preda, 2005; O’Shea et al., 2018) and long term ammonia release (Gooddy et al., 2014). Future climate change scenarios also increase the likelihood for tidal flooding and enhanced leachate generation. However, it is generally recognized that following decades of burial leachate is likely to be relatively dilute and any release would be rapidly dispersed in coastal waters (Beaven et al., 2020; Brand and Spencer, 2020).
The management of solid waste release has perhaps received more consideration, and for controlled disposal sites solid waste is usually isolated from the marine environment by installing a physical barrier such as a hard engineered coastal defense or through geographic location by placing landfills on cliff tops above mean high water (Nicholls et al., 2020). However, with sea level rise and increased frequency and intensity of storm surges and coastal erosion, there are multiple scenarios for contaminant release through; (1) over-topping of barriers and inundation with saline waters, (2) damage to coastal defenses and waste release, (3) catastrophic failure of coastal defenses, and (4) cliff failure (Beaven et al., 2020). In the United Kingdom, 1 in 10 vulnerable historic coastal landfills are at risk of erosion if coastal defenses are not maintained (Brand et al., 2017) and there is also increasing evidence that extreme flood and erosion events can result in catastrophic failure and release large volumes of solid waste. For example, 13 toxic waste sites were flooded in Texas by Hurricane Harvey in 2017 (U.S. Environmental Protection Agency, 2019) and more recently, in New Zealand, floods washed out a closed landfill on the Fox River releasing solid wastes over an area ca. 1000 ha and impacting 63 km of coastline (Department of Conservation, 2019). Even following decades of burial landfill waste can be highly toxic, with the release of matrix material, textiles, wood, paper, asbestos and plastics all presenting significant ecological risk (Brand and Spencer, 2019; Su et al., 2019).
For coastal brownfield sites more generally, there is a risk that coastal and climatic change may undermine remediation and risk management strategies previously used at contaminated sites, particularly where risk management strategies have involved source stabilization or pathway management (i.e., strategies where contaminants have been stabilized or managed in situ and remain in the subsurface). The US Sustainable Remediation Forum (SuRF) spearheaded 3 years of collaborative research and knowledge exchange that culminated in: Resilient Remediation: Addressing Extreme Weather and Climate Change, Creating Community Value (Maco et al., 2018). They found that “At hazardous sites, climate change and extreme weather events can undermine the effectiveness of the original site remediation design and can also impact contaminant toxicity, exposure, organism sensitivity, fate and transport, and long-term operations, management, and stewardship of remediation sites.” For example, a detailed modeling study at a Superfund site impacted by Hurricane Florence showed that, “in general, higher-infiltration events could mobilize vadose-zone residual contaminants, raising contaminant concentrations in groundwater for a prolonged period.” Further, in the US nearly two million people, the majority in low-income communities, live within 1 mile of one of 327 Superfund sites in areas prone to flooding or vulnerable to sea-level rise caused by climate change (Maco et al., 2018). In October 2019, the U.S. Government Accounting Office identified that ca. 60% of all non-federal National Priorities List (most contaminated) sites are located in areas that may be impacted by potential climate change impacts of flooding, storm surge, wildfires, and sea level rise (U.S. Government Accountability Office [GAO], 2019). The majority of sites are located in coastal areas (Figure 1), with 7% of sites expected to be inundated by a sea-level rise of 3 ft (0.9 m), and 11.9% at risk of flooding from Category 4 or 5 hurricane surges.
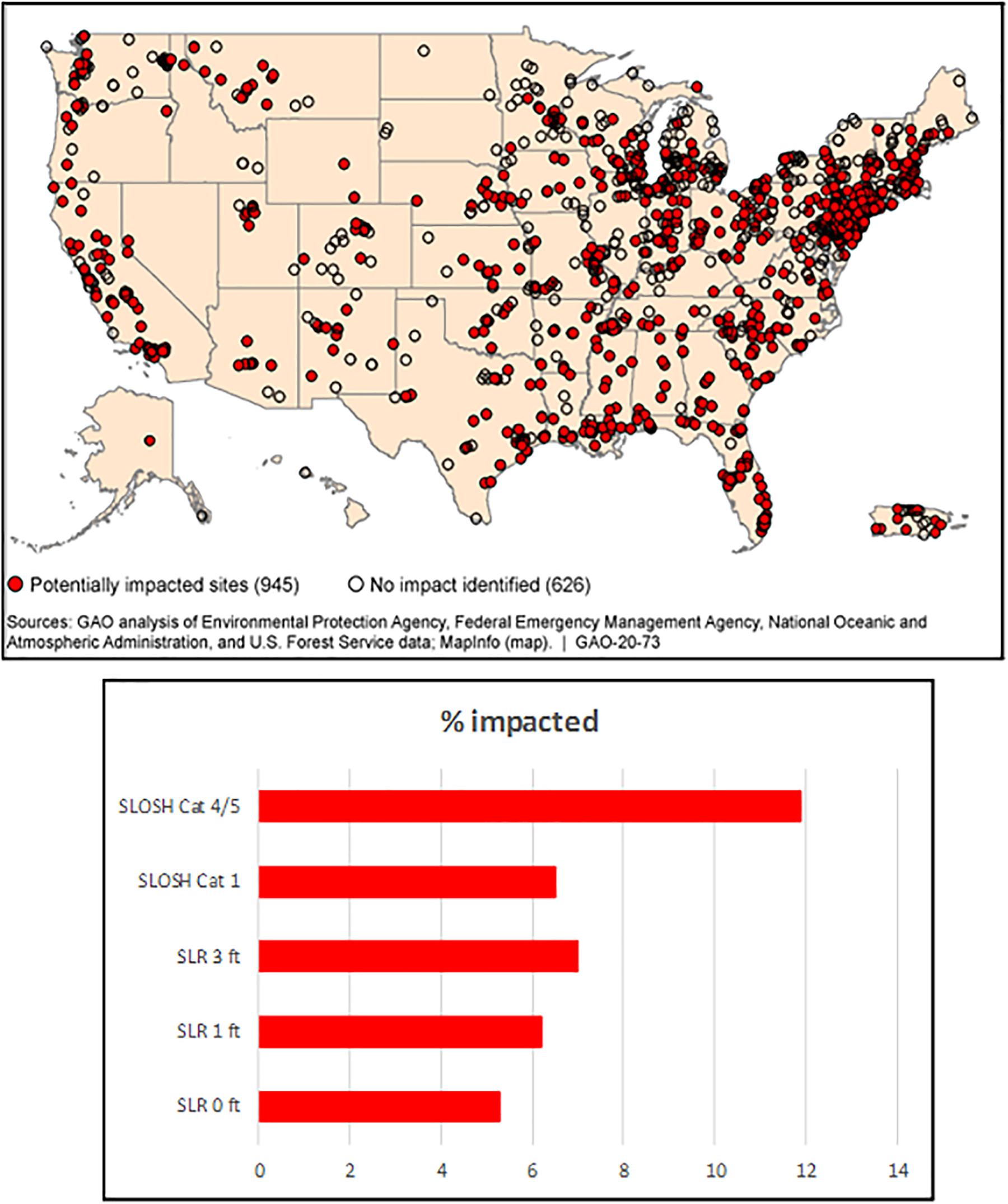
Figure 1. Superfund sites located in areas that may be impacted by flooding, storm surge, wildfires, or sea level rise (top). Bottom graph shows (from top) percentage of sites impacted by Sea, Lake, and Overland Surges by Hurricanes (SLOSH) of category 4 or 5, and category 1, and those expected to be inundated by a sea-level rise of 3, 1, and 0 ft (source: U.S. Government Accountability Office [GAO], 2019).
Therefore, the presence of waste and residual contamination in the coastal environment can place significant constraints on coastal managers. For example, the presence of historic landfills can preclude the selection of more sustainable coastal management approaches such as managed realignment, which adapt to sea level rise and create flood storage by moving coastal defenses inland, or “do nothing” approaches, where coastlines are allowed to retreat in response to natural coastal processes. In addition, estuaries are also frequently areas of significant sediment contamination resulting from decades (if not centuries) of industrial use and effluent release with pollution being a significant, long-term threat to estuarine and coastal ecosystem health (e.g., Kennish, 2002). These legacy pollutants may cause deterioration in water quality and ecological health through reworking, erosion, natural advective mixing and diffusion, which may be enhanced under climate change scenarios (e.g., Machado et al., 2016), and through dredging activities whereby sediments are disturbed via mechanical means (e.g., Spencer et al., 2006). As such, contaminated sediments have the potential to be released to marine waters or deposited on coastal floodplains and wetlands (Bert et al., 2009).
Approximately 200 million m3 of sediment is dredged annually from Europe’s harbors, ports and coastal waters, and up to 50% of this is contaminated (SedNet, 2004; Todaro et al., 2016). The European Waste Directive encourages us to view these dredged materials as a resource (Apitz, 2010) and if possible either reduce their production (through e.g., management of dredge licensing) and to re-use where possible. Even contaminated sediments can be treated to immobilize or transform the contaminants, and de-water and stabilize the sediments (Todaro et al., 2016). Increasingly, these sediments can then be used for coastal protection, environmental enhancement and sustainable restoration of tidal wetlands and beaches (e.g., Martin et al., 2019). However, whilst there is enormous potential to utilize dredged material as a resource, many projects are small, piecemeal and lack long term monitoring or assessment. Most projects deal only with uncontaminated materials (Costa-Pierce and Weinstein, 2002; Martin et al., 2019) and practices and technology uptake can vary, driven by nuances in national dredging policy and the communication difficulties associated with coupling sediment supply to suitable, local receptor sites (Ausden et al., 2018).
Low Input Approaches – Their Benefits, and Toward an Integrated Management Approach
A variety of integrated, low input remediation/restoration approaches are possible for coastal brownfields which may offer some advantages over contemporary approaches. In broad terms remediation2 describes the mitigation of risks from brownfields. For a risk to be present, a source (of hazardous substance or property), a receptor (that could be affected adversely by the contamination) and a pathway (linking the source to the receptor) must be present, as shown in Figure 2 (Tack and Bardos, 2020).
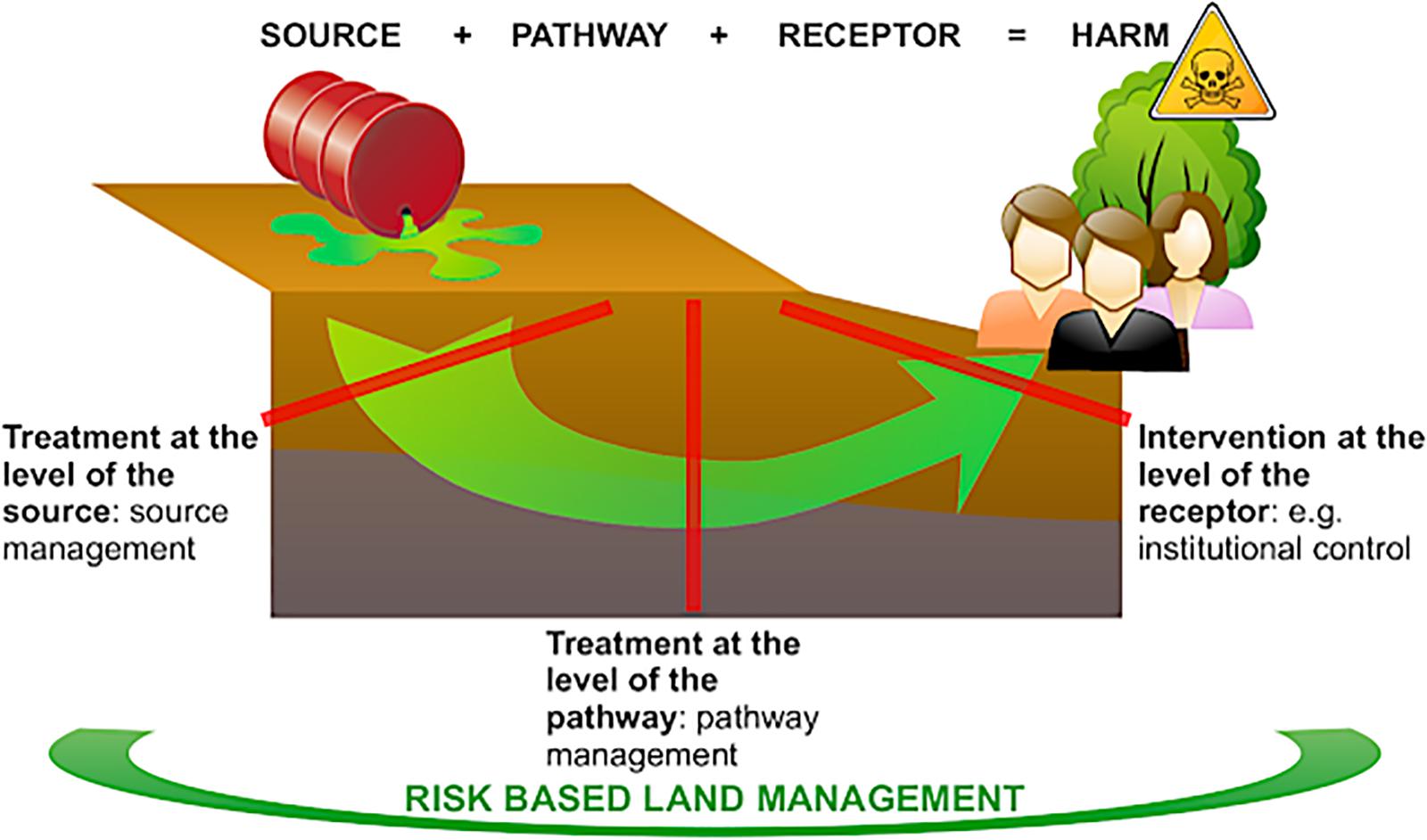
Figure 2. Risk management along a contaminant (S-P-R) linkage (Tack and Bardos, 2020).
A receptor might be a human, an ecologically sensitive site, surface or underground water resources, or a building. Moreover, risks to the ecosystem “goods or services” provided by the wider environment3 may become an increasingly important receptor to consider in many scenarios. A risk management intervention can take place at any point in the S-P-R linkage provided that it breaks the linkage, which might be by removing the source, intercepting the pathway, or modifying the receptor behavior or location. A range of risk management/remediation options are available at different points across any particular linkage4. This risk-based approach to contaminated sites is termed Risk Based Land Management – RBLM (Vegter et al., 2002).
Remediation is not intrinsically sustainable. Poorly planned projects can have serious negative impacts, and so (ideally) risk management should also therefore meet sustainable development principles. Together this constitutes sustainable risk based contaminated land management, SRBLM (NICOLE, and COMMON FORUM, 2013).
Restoration describes improving and extending the functionality of land so it can serve a wider range of purposes, which can encompass remediation, but also measures to improve soil and water quality, ecological management, and establishment of new higher value (including more sustainable) land uses. These land uses may be for buildings or other infrastructure where the soil is sealed (“hard” re-uses), or land use dependent on the soil function such as for parkland or where the soil is otherwise unsealed such as for photovoltaic arrays (“soft re-uses”). In the context of coastal brownfields soft re-uses may be particularly important as they can offer a range of ancillary services, for example flood management capacity, carbon sequestration and storage, and/or public amenity in areas where buildings and infrastructure are either no longer feasible or considered at risk. The processes of remediation, restoration and ongoing management may all be included in an overarching strategy that yields multiple benefits from the brownfield management process (Bardos et al., 2016), creating a greater overall value from the land re-use, a wider partnership of interested parties able to support the brownfield regeneration and greater resilience to future threats, such as climate change mitigated impacts (e.g., Maco et al., 2018).
Low input remediation measures, so called “gentle” remediation approaches or options, may be particularly advantageous given their passive nature and relatively low cost base. “Gentle” remediation options, or GRO, are defined as “risk management strategies or technologies that result in a net gain (or at least no gross reduction) in soil function as well as achieving effective risk management” (Cundy et al., 2013). They include a range of plant (phyto-), fungi (myco-), and/or bacteria-based approaches (Cundy et al., 2016; Table 1), with or without chemical additives or soil amendments, which reduce contaminant transfer to local receptors by extraction, transformation, or degradation of contaminants, or by in situ stabilization. “Gentle” remediation options are closely aligned with the concept of “nature-based solutions” for the longer-term restoration of land. Indeed, approaches such as bioremediation and phytoremediation are increasingly being used as a low input approach to coastal brownfield land remediation (Hassan et al., 2019; O’Connor et al., 2019). Phytoremediation is particularly effective where hyperaccumulator plants are used, e.g., those that have the capability of assimilating high levels of metals such as Au, Ag, Cd, Se, Ta, Cu, Co, Cr, Ni, Pb, U, As, Mn, and Zn (Mahar et al., 2016) including coastal plant species such as Phragmites australis, Deschampsia cespitosa, Festuca rubra, Juncus maritimus, Spartina alterniflora, Distichlis spicata, and Ruppia maritima (Peer et al., 2005). In addition, where metal-excluding plants are used (with or without soil amendments), phytostabilisation shows promise as a low-cost method for contaminated dredged sediment management (e.g., Bert et al., 2008; Bert et al., 2009). Bioremediation utilizes microbial activity to decrease available contaminants within degraded systems. This method is particularly effective for groundwater treatment although this is dependent on appropriate geochemical conditions, available nutrients and the abundance of microorganisms (Sam and Zabbey, 2018), and does not provide the same landscape and visual amenity, nor ecosystem service benefits available from other nature-based solutions (Song et al., 2018). Phytoremediation can offer a range of benefits to coastal brownfield site remediation including lower energy input, higher material efficiency and resilience from global environmental change as well as providing a range of ecosystem service benefits such as flood protection, estuarine filtering of environmental pollutants, habitat and nursery for marine animals, and carbon sequestration and storage amongst others (Blanco-Canqui, 2016; Burges et al., 2017, 2018). In spite of these benefits, nature based solutions are often only used where there is a cost benefit to implementation rather than taking into account the wider socio-economic and environmental benefits (Song et al., 2018).
Potential low input approaches to the redevelopment of coastal brownfield sites include conversion of brownfield land to coastal wetland, parkland or forest providing resilience to pressures from global environmental change (Sarkis et al., 2016; Zhu et al., 2017; Song et al., 2018). These options all provide strong aesthetic value improvement to sites (Hartig et al., 2012), as well as habitat creation (or replacement) for plants, mammals, birds and invertebrates (Harrison and Davies, 2002; Sellers et al., 2006; Woods, 2012; Latham et al., 2016), increased infiltration or flood storage capacity and resultant decrease in flood risk (EPA, 2014), and low life cycle environmental footprints. They also provide a meeting/socializing space, which improves well-being, particularly important in crowded urban areas such as are found in Asia, N. America and Europe (Faivre et al., 2017). Forest planting schemes at coastal brownfield sites can help reduce noise, improve air quality and reduce heat island effects (Solecki et al., 2005; Tan et al., 2016). Importantly, given the adoption of ambitious zero net carbon targets by a number of urban areas5, there is significant potential for planted sites to provide a very visible contribution to integrated carbon reduction strategies, via carbon storage and sequestration, although the degree of carbon offsetting generated will depend on planting type and density, stand area, etc. (e.g., Zhao et al., 2010; Tang et al., 2016; Wilkes et al., 2018). Conversion of brownfield sites to forest, coastal wetland or parkland can also have wider societal benefits improving the longer-term liveability and environmental quality for local communities (Mitchell et al., 2015). This can also strongly interface with “bluefields” strategies, around the linking or integration of abandoned or underutilized sites along rivers or other waterfronts, as part of urban waterfront regeneration initiatives (e.g., Pinch and Munt, 2002; Tolnai, 2018).
In addition, the EU has set a target of at least 20% of energy from renewable sources by 2020, with a consequent requirement for an increase in biomass fuels. The State of California has committed to procuring 50% of energy from renewables by 2050, while the State of New York’s Climate Action Plan includes a goal of 100% Renewable energy by 2050. To support these targets, coastal brownfield sites can be repurposed for biomass production utilizing plant species such as: Salix spp.; Phalaris arundinacea; Panicum virgatum; Miscanthus giganteus; rapid growth species with a tolerance for high levels of soil contamination and a tolerance/preference for wet soils (Lord et al., 2008; Lord, 2015). The autecological requirements of these species make them ideal for coastal brownfield site plantation. These species can also provide phytoremediation through removal of some soil contaminants (e.g., Zn, Cd, and Cu) (Lord et al., 2008). Infrastructure linked to coastal brownfield sites (e.g., roads, grid connections), as well as proximity to consumers and appropriate zoning make these sites ideal for potential repurposing as brightfield sites (sites for renewable energy generation) (Converse, 2007), addressing three major global challenges: climate change; urban revitalization; and contaminated land remediation (Adelaja et al., 2010).
Potential Synergies, and Maximizing Benefits
As we discuss above, there are a range of synergies potentially offered by the application of low input remediation or restoration approaches for soft end-use, which utilize coastal brownfields and/or contaminated dredged materials within an integrated overall coastal management approach. A number of current and historical examples have gone part-way toward realizing this synergetic management approach. These include:
• Beneficial use of dredging materials and construction wastes for habitat recreation and other benefits, SE England and the Thames estuary, United Kingdom. Increasingly, dredged materials from maintenance and capital dredging, but also sediments from engineered tunneling have been used for coastal and brownfield restoration in the Thames Estuary and south east England. For example, 6 million tonnes of excavation material from London’s Crossrail project has been re-used and recycled to create 670 ha of coastal wetland habitat at Wallasea Island, Blackwater Estuary, Essex, United Kingdom, and to raise the land by 1.5 m as an adaptation to sea level rise (Dixon et al., 2008; Cross, 2017).
• Restoration of a former landfill site for ecological and community benefit, Mersey estuary, United Kingdom. Port Sunlight River Park is a 28-hectare community park space near Birkenhead in the Wirral, Merseyside, U.K. located on a capped and covered former coastal landfill site. After closure to new waste in 2006, the site was leased to the Land Trust (a United Kingdom charity) and following a £3.4 million investment was repurposed as a riverside park in 2013 (opening to the public in 2014). The River Park is managed through a partnership with a local non-profit organization, Autism Together (a charity providing services and support to people with autism and their families), who manage the park on a day-to-day basis and lead local community engagement in park activities, as well as providing work opportunities for local community members affected by autism. The completed park provides visitors with waterfront access (with views over the Liverpool city skyline), a variety of walks (and other leisure and recreation opportunities) and access to nature. A section of wetland in the north of the site, linking to the adjacent River Mersey mud flats, provides important habitat for water birds. Based on a qualitative sustainability assessment, Li et al. (2019) show that the establishment of the Port Sunlight River Park has clear sustainability advantages (using a range of environmental, economic and social indicators) over a baseline of having left the site under its previous management regime.
• “Designing with water” to improve storm and climate change resiliency, Boston, MA, United States. Boston, Massachusetts, has been aggressively researching options to protect the city post-Hurricane Sandy as part of its “Designing with Water” efforts (Aiken et al., 2014; Sutton-Grier et al., 2015). In 2015, Boston ran an international competition for design solutions imagining a more resilient, more sustainable, and more beautiful Boston prepared for both sea level rise and climate change up to the end of the 21st century (Sutton-Grier et al., 2015). This utilized different examples of hybrid approaches to make urban areas more resilient to climate change and storms, including Dutch “Living with Water” strategies, where planners design to accommodate flood waters in urban settings and build floating communities for flood control and socioeconomic prosperity. Successful projects were expected to help build resilience to disturbances to existing built infrastructure and to social and community networks, and do “double duty” in terms of providing flood protection in times of need whilst also providing other uses and benefits (such as recreational opportunities) when protection is not needed (Sutton-Grier et al., 2015). Outputs were used to inform revisions to building plans and zoning codes, and influence the city’s “Imagine Boston 2030” strategy, Boston’s first citywide plan in 50 years.
• Renewable energy on coastal brownfields for local energy generation and community benefit, San Francisco Bay, United States. A recently completed renewable energy project in the San Francisco Bay Area, the Marin Clean Energy (MCE) Solar One partnership, highlights the benefits of synergetic management approaches to coastal brownfields. Sixty acres of a remediated brownfield site were leased (by the site owners Chevron) to the Partnership for $1 per year. This land was then repurposed for solar power generation. At 10.5 megawatts capacity, the site is expected to eliminate 3,234Mt of carbon dioxide emissions per year, the equivalent to taking more than 680 cars off the road annually. As well as leveraging significant inward investment (almost two million dollars were spent on project materials purchased or rented locally), the MCE Solar One Partnership provided additional community benefits by partnering with RichmondBUILD, who are a public–private partnership focusing on training community members from low income households for skilled construction, hazardous waste removal, and renewable energy jobs. The project also includes an innovative procurement approach called “community choice energy,” in which citizens and businesses are offered an alternative to the standard energy utility for purchasing their electricity. As a result, homes and businesses benefit from a low-carbon electricity option that costs 2–5% less than traditional Bay Area utility rates6.
• Park Spoor Nord, Antwerp, Belgium. Antwerp, a major and diverse port city on the Scheldt estuary in Belgium, in common with many port cities contains a significant legacy of underused industrial and harbor space, as well as high density residential areas and limited public green space. Regeneration in the Spoor Nord area of the city has focused on attracting investment in residential and commercial land, and generating public support for on-going regeneration. Based on local residents’ feedback, green and open areas, space and light were identified as the main priorities of the regeneration process. To facilitate this, the City of Antwerp supported the restoration of a 24 ha former railway complex as an urban landscape park, integrating residential areas, a Sports center, and green open space (parkland), to bring green public space into the densely populated Spoor Nord area, and act as a catalyst for new development and inward investment. This example is one of several considered within the EU Seventh Framework Program project TIMBRE7, which focused on the regeneration of large and complex contaminated “megasites” in Europe. To support this, the project developed a web-based tool (the Timbre Brownfield Prioritization Tool) which integrates sustainability assessment and multiple-criteria decision analysis (MCDA) to facilitate assessment and prioritization of a portfolio of sites on the basis of the probability of successful and sustainable regeneration (Bartke et al., 2016).
One of the key issues in applying such approaches at a specific site or regional level, and realizing as full a range of benefits as possible, is identifying the synergies or conflicts between interventions, in terms of maximizing the benefits (services) from coastal brownfield restoration and dredged sediment re-use, and how this might encompass wider opportunities for better coastal management. In addition, in order to gain support for soft re-use, it is also important to not just illustrate sustainability in the redevelopment process, but also understand how it can create value for stakeholders. Here, we present an approach and framework for assessing and comparing different scenarios for coastal brownfield regeneration to soft re-use and other end-points, to support planning and options appraisal to realize maximum benefit and value. We use as the basis for this a “sustainability linkages” approach, based on sustainability assessment criteria produced by the UK Sustainable Remediation Forum (SuRF-UK) (CL:AIRE, 2011; Li et al., 2019).
The “Sustainability Linkages” Approach
Context
Land Contamination practitioners are familiar with the source-pathway-receptor, or contaminant linkage, paradigm for providing a structure for assessing risks, evaluating them and planning a risk management response. An analogous thought process can be applied to consider the various individual considerations of a sustainability assessment, to produce a series of sustainability linkages. A sustainability linkage describes the connection between a driver (a pressure or a change), something that might be affected (i.e., a receptor) and the mechanism by which a pressure or change affects a receptor, see Figure 3. Analogous to the source-pathway-receptor model, a sustainability effect only takes place when there is also a receptor that might be affected and a mechanism (“pathway”) by which this affect can happen. Li et al. (2019) show how individual sustainability linkages can be collated following site stakeholder consultation to provide a conceptual site model for sustainability for a brownfields project, using the example of a coastal legacy landfill site (the Port Sunlight River Park example discussed in section “Potential Synergies, and Maximizing Benefits”) regenerated for soft re-use as community parkland and coastal wetland. We discuss this example and the wider applicability of the sustainability linkages approach further here, within the context of the integrated management of post-industrial coasts.
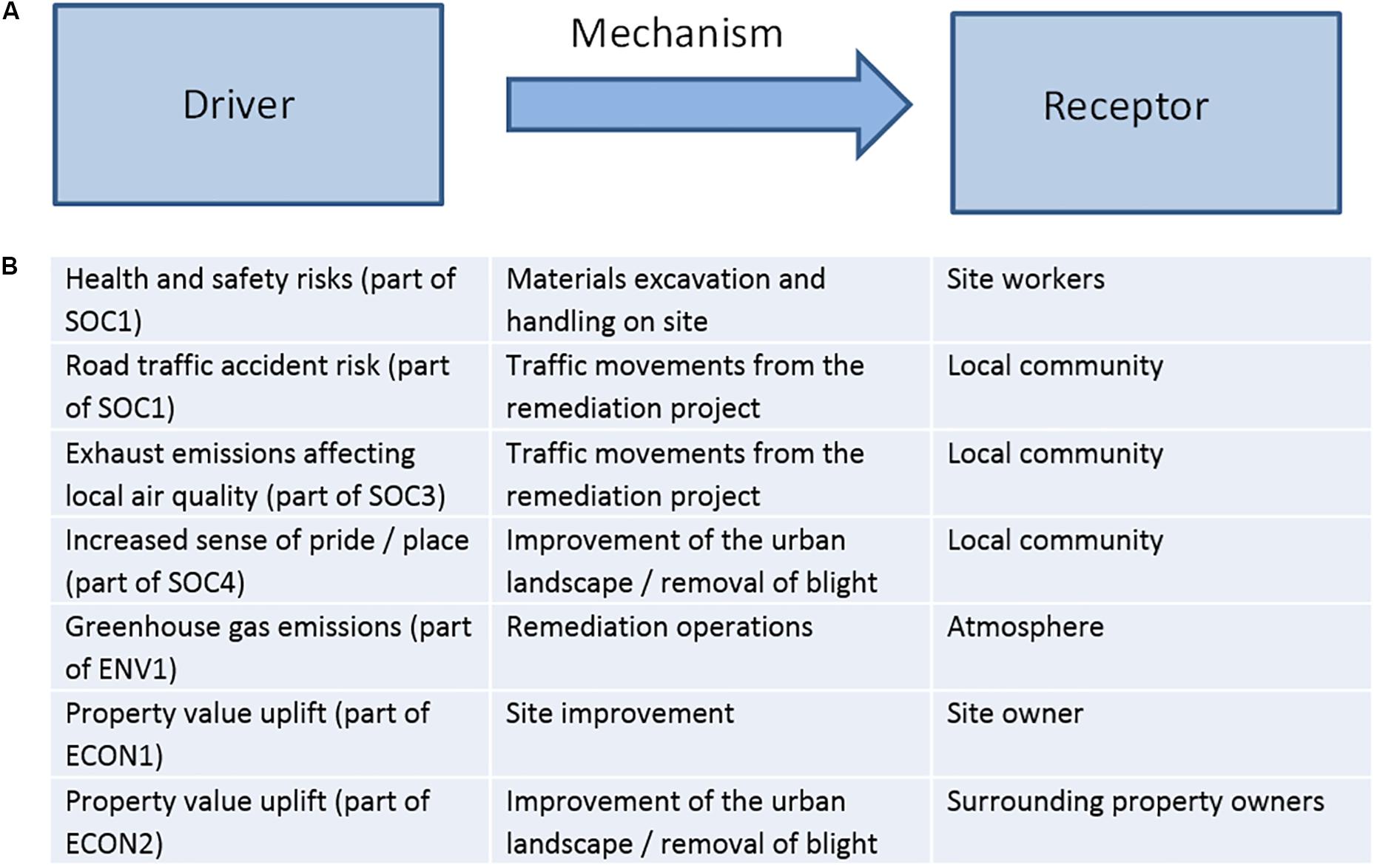
Figure 3. A sustainability linkage (A), and some possible examples (by no means exhaustive) (B). Abbreviations in left column refer to SURF-UK Sustainability Assessment Criteria (CL:AIRE, 2011).
The Port Sunlight Riverside Park sustainability assessment is based on United Kingdom guidance produced by the Sustainable Remediation Forum UK, an independent cross-sectoral project managed by the United Kingdom contaminated land information forum CL:AIRE8. This guidance offers a range of possible indicators/criteria that can be used in sustainability assessments for brownfields organized across 15 headline categories, summarized in Table 2, and has been recently updated (CL:AIRE, 2020). Sustainability assessments are highly site and project specific, so the conceptual model developed for the Port Sunlight Riverside Park is unique to its context.

Table 2. SuRF-UK headline categories for sustainability indicators (CL:AIRE, 2011, 2020).
Methodology
The qualitative sustainability assessment was carried out in 2016. The aim of the sustainability assessment was to understand the economic, environmental and social benefits/disbenefits of transforming the former landfill (a brownfield site) into a public open space, managed long term. The sustainability assessment therefore compared two intervention options:
1. Establishment of the park (i.e., the transformation from a restored landfill site to park and long term management, including construction of roads, paths, landscaping, drainage and car parking; but excluding existing landfill management measures); and
2. A hypothetical “no intervention” baseline, where the site continued as a managed former landfill.
Full methodological details are given in Li et al. (2019) but in summary:
The sustainability assessment followed guidance issued by SuRF-UK. Identification and analysis of individual sustainability linkages was carried out across all of the SuRF-UK headline categories (CL:AIRE, 2011), in consultation with the Land Trust (site owner) and Autism Together (the charity that manages the site on a day to day basis on behalf of the Land Trust). Fifty individual specific sustainability linkages were identified and individually ranked. These were combined into a conceptual model for the site, which can be used to rationalize the pressures/mechanisms and receptors, show where effects are desirable or not desirable, check for possible duplicated effects and show interconnections between effects. In the case of the Port Sunlight River Park comparison: 30 pressures, 31 mechanisms and 6 receptors encapsulated the 50 linkages identified (no duplicates were found).
Results
The network diagram (conceptual model) produced for Port Sunlight River Park is shown in Figure 4. The linkages assist in making individual cause and effect chains explicit, so that different management options can be more readily compared, and different linkages can be more explicitly valued. This has the benefit of identifying and so reducing unintentional duplications of sustainability criteria, and can also highlight synergies and conflicts for different risk management or regeneration scenarios. For example, short-term greenhouse gas emissions from the operation of remediation or engineering plant may be offset in the longer-term by carbon sequestration and storage in park soils and vegetation, or “blue carbon” storage in the wetlands at the north of the site. Specific synergies can be identified between flood water storage capacity and improved habitat, inward investment creation and property value uplift, and community inclusivity, access to parkland and public health benefits, amongst others.
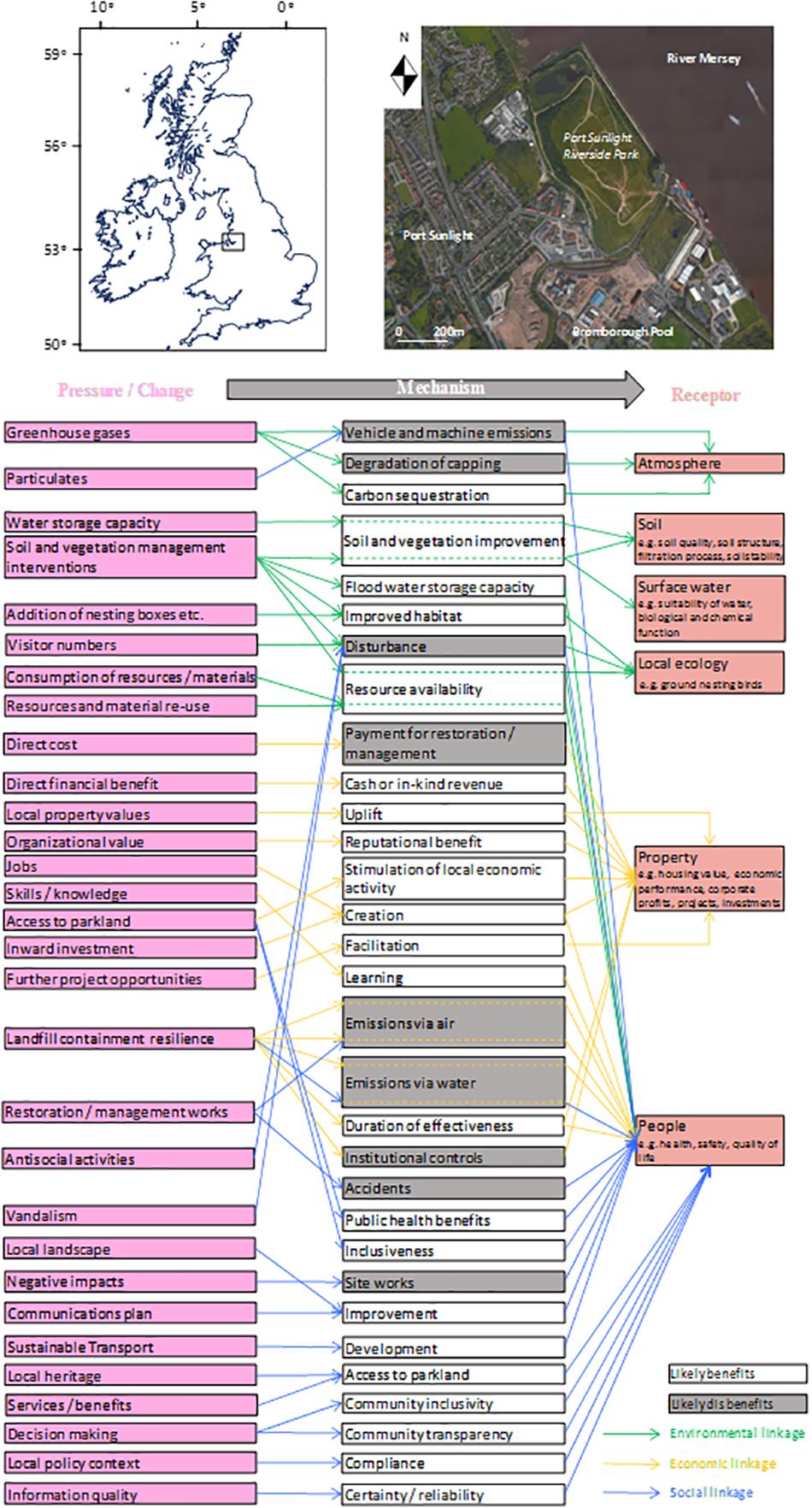
Figure 4. Conceptual site model for sustainability (network diagram) for the Port Sunlight River Park (after Li et al., 2019). Mechanisms are colored depending on whether they are considered deleterious (as gray) or beneficial (white). Linkages are shown as arrows, color-coded to environmental, economic and social elements of sustainability, using green, yellow and blue, respectively.
Our suggestion is that the sustainability linkages approach can be used as a planning and decisional aid to assist a more robust valuation of the wider benefits from coastal brownfield regeneration to soft re-use and other end-points, by: (1) ensuring that any cost benefit assessment is consistent with a conceptual model of sustainability, rather than being based on a different set of premises or indicators; and (2) providing a more targeted valuation approach. For the latter point (in regards to valuation) the sustainability linkages that comprise the conceptual model can be divided into three broad groups (grouped by their ease of monetization):
• Those linked already to some form of investment cost or return – which can be valued under a direct financial model.
• Linkages that can be readily and broadly agreed to be linked to wider effects that are economically tangible and so more readily valued, for example, surrounding property value uplift, and benefits to local businesses.
• Those linkages related to wider effects that at least one stakeholder considers economically intangible, or not easy to value, such as public health benefit, value of access to nature, improved visual amenity etc.
While direct monetization of sustainability benefits was not possible in the example given by Li et al. (2019), the conceptual site model based on sustainability linkages provides a clearer basis for understanding cause and effect for benefits and disbenefits, while also therefore providing a rationale for grouping individual effects based on their ease of valuation. This potentially provides a road map for cost-benefit assessments for different integrated coastal management approaches by (1) being able to match specific sustainability linkages to their most appropriate means of valuation, and (2) connecting the sustainability assessment and cost benefit assessment processes in a transparent (and defensible) manner. Moreover, where stakeholders have concerns about the valuation the process would be sufficiently transparent that they could precisely zero in on the points of concern and perhaps then be better able to make their own arguments.
Concluding Remarks
The sustainability linkages approach and framework discussed here provides a potential method for achieving a better understanding and design of combined coastal brownfields management approaches to maximize benefits and minimize risks, benchmarked against standard sustainability assessment criteria. This framework can be applied to explore the opportunities for synergy and realization of wider environmental, economic and societal benefits between coastal protection, dredged material re-use and the management of brownfield land, as well as to support planning and options appraisal to realize maximum benefit and value from integrated coastal management strategies. The approach uses engagement with core and wider stakeholders throughout the preparation, definition and execution phases, which is critical as the effectiveness of soft re-use depends on the public’s perceptions of risk and their willingness to support new uses of the sites (Levi and Kocher, 2006). There are strong synergies for these integrated soft re-use management approaches to interface with coastal flood protection and coastal habitat creation initiatives, and emerging areas such as landfill mining and resource re-use, green infrastructure approaches and city carbon neutrality targets. The unique strengths of natural infrastructure are that it can be self-maintaining, has the potential to self-repair after major damaging events, and (in the case of marsh/mangrove systems) has the ability to grow and keep pace with sea level rise (Sutton-Grier et al., 2015). Low-input approaches, appropriately designed, can generate wide environmental, economic and social benefits along post-industrial coasts, and can show enhanced resilience to sea level rise and other hydroclimatic effects induced by climate change (O’Connor et al., 2019), and can leverage a number of current brownfields resilience initiatives. In the United States, for example these include the ASTM guide (in development) for Resilient remedies, and the Interstate Technology and Regulatory Council – [a 50 US state-led coalition – (ITRC)] project to develop principles, practices and case studies for Resilient Sustainable Remediation. For the latter, a recent survey by the ITRC RSR team noted that brownfields provided the best opportunity for sustainable clean up and re-use of contaminated sites: the states surveyed identified the most valuable metrics as job creation and preservation and creation of open space, including parks and marshlands.
Author Contributions
PB, KS, and AC conceived the initial idea, and outlined the brief, which was then co-developed by all authors. PB and AC led on the gentle remediation and sustainability linkages approaches. KS led on coastal landfills and dredged sediment management. RW led on coastal management and ecology. BM led on renewables and US-based examples. All authors contributed to the manuscript writing, revision, read, and approved the submitted version. AC finalized and co-ordinated submission of the manuscript. The opinions given in this manuscript are those of the authors, and do not necessarily reflect those of their employers.
Funding
Preparation of this manuscript was undertaken using the authors’ own resources, or as part of their standard employment.
Conflict of Interest
PB was employed by the company r3 Environmental Technology Ltd.
The remaining authors declare that the research was conducted in the absence of any commercial or financial relationships that could be construed as a potential conflict of interest.
Footnotes
- ^ Sites that have been affected by the former uses of the site and surrounding land, are derelict or underused, may have real or perceived contamination problems, are mainly in developed urban areas and require intervention to bring them back to beneficial use. CABERNET (2007).
- ^ Specifically, managing the risks to receptors so that there is no longer a risk of unacceptable harm. This may be via some form of intervention at the level of source, pathway or receptor.
- ^ For example, as described by the World Health Organization: https://www.who.int/globalchange/ecosystems/en
- ^ For example, as described by Land contamination: risk management, https://www.gov.uk/guidance/land-contamination-how-to-manage-the-risks
- ^ https://news.trust.org/packages/zero-carbon-cities/
- ^ https://www.mcecleanenergy.org/news/press-releases/mce-solar-one-thinking-globally-building-locally/
- ^ http://www.timbre-project.eu/
- ^ www.claire.co.uk/surfuk
References
Adelaja, S., Shaw, J., Beyea, W., and McKeown, J. C. (2010). Renewable energy potential on brownfield sites: a case study of Michigan. Energy Policy 38, 7021–7030. doi: 10.1016/j.enpol.2010.07.021
Aiken, C., Chase, N., Hellendrung, J., and Wormser, J. (2014). Designing with Water, Preparing for the Rising Tide. Boston, MA: Boston Harbor Association.
Apitz, S. E. (2010). Waste or resource? Classifying and scoring dredged material management strategies in terms of the waste hierarchy. J. Soils Sediments 10, 1657–1668. doi: 10.1007/s11368-010-0300-9
Ausden, M., Dixon, M., Lock, L., Miles, R., Richardson, N., and Scott, C. (2018). Sea Change in the Beneficial Use of Dredged Sediment (Technical Report). Bedfordshire: Royal Society for the Protection of Birds.
Bardos, P., Bone, B., Boyle, R., Ellis, D., Evans, F., Harries, N. D., et al. (2011). Applying sustainable development principles to contaminated land management using the SuRF-UK framework. Remediation 21, 77–100. doi: 10.1002/rem.20283
Bardos, P., Jones, S., Stephenson, I., Menger, P., Beumer, V., Neonato, F., et al. (2016). Optimising value from the Soft Re-use of Brownfield Sites. Sci. Total Environ. 563–564, 769–782. doi: 10.1016/j.scitotenv.2015.12.002
Bartke, S., Martinát, S., Klusáček, P., Pizzol, L., Alexandrescu, F., Frantál, B., et al. (2016). Targeted selection of brownfields from portfolios for sustainable regeneration: user experiences from five cases testing the Timbre Brownfield Prioritization tool. J. Environ. Manage. 184, 94–107. doi: 10.1016/j.jenvman.2016.07.037
Beaven, R. P., Stringfellow, A. M., Nicholls, R. J., Haigh, I. D., Kebede, A. S., and Watts, J. (2020). Future challenges of coastal landfills exacerbated by sea level rise. Waste Manage. 105, 92–101. doi: 10.1016/j.wasman.2020.01.027
Bert, V., Lors, C., Laboudigue, A., Tack, K., Damidot, D., and Bureau, J. (2008). “Use of phytostabilisation to remediate metal polluted dredged sediment,” in Proceedings of the International Symposium on Sediment Management (I2SM), Jul 2008: ffineris-00973314f, Lille, 275–279.
Bert, V., Seuntjens, P., Dejonghe, W., Lacherez, S., Thuy, H. T., and Vandecasteele, B. (2009). Phytoremediation as a management option for contaminated sediments in tidal marshes, flood control areas and dredged sediment landfill sites. Environ. Sci. Pollut. Res. Int. 16, 745–764. doi: 10.1007/s11356-009-0205-6
Blanco-Canqui, H. (2016). Growing dedicated energy crops on marginal lands and ecosystem services. Soil Sci. Soc. Am. J. 80, 845–858. doi: 10.2136/sssaj2016.03.0080
Brand, J. H., and Spencer, K. L. (2019). Potential contamination of the coastal zone by eroding historic landfills. Mar. Pollut. Bull. 146, 282–291. doi: 10.1016/j.marpolbul.2019.06.017
Brand, J. H., and Spencer, K. L. (2020). Will flooding or erosion of historic landfills result in a significant release of soluble contaminants to the coastal zone? Sci. Total Environ. 724:138150. doi: 10.1016/j.scitotenv.2020.138150
Brand, J. H., Spencer, K. L., O’Shea, F. T., and Lindsay, J. (2017). Potential pollution risks of historic landfills on low-lying coasts and estuaries. WIREs Water 5:e1264. doi: 10.1002/wat2.1264
Burges, A., Alkorta, I., Epelde, L., and Garbisu, C. (2018). From phytoremediation of soil contaminants to phytomanagement of ecosystem services in metal contaminated sites. Int. J. Phytoremediation 20, 384–397. doi: 10.1080/15226514.2017.1365340
Burges, A., Epelde, L., Blanco, F., Becerril, J. M., and Garbisu, C. (2017). Ecosystem services and plant physiological status during endophyte-assisted phytoremediation of metal contaminated soil. Sci. Total Environ. 584, 329–338. doi: 10.1016/j.scitotenv.2016.12.146
CABERNET (2007). “Concerted action on brownfield and economic regeneration network (CABERNET),” in Proceedings of the 2nd International CABERNET Conference — Managing Urban Land, Stuttgart.
CL:AIRE (2011). The SuRF-UK Indicator Set for Sustainable Remediation Assessment. ISBN: 978-1-905046-1292-5. Available online at: https://www.claire.co.uk/phocadownload//SuRF-UK%20Framework%20Annex%201%20-%20FINAL_web.pdf (accessed January 3, 2020).
CL:AIRE (2020). Supplementary Report 2 of the SuRF-UK Framework: Selection of Indicators / Criteria for Use in Sustainability Assessment for Achieving Sustainable Remediation. Available online at: www.claire.co.uk/surfuk (accessed June, 2020).
Converse, A. O. (2007). Renewable energy in the United States. Appl. Biochem. Biotechnol. 137, 611–624.
Costa-Pierce, P., and Weinstein, M. P. (2002). Use of dredge materials for coastal restoration. Ecol. Eng. 19, 181–186. doi: 10.1016/s0925-8574(02)00076-9
Cox, M. E., and Preda, M. (2005). Trace metal distribution within marine and estuarine sediments of Western Moreton Bay, Queensland, Australia: relation to land use and setting. Geogr. Res. 43, 173–193. doi: 10.1111/j.1745-5871.2005.00312.x
Cross, M. (2017). Wallasea island wild coast project, UK: circular economy in the built environment. Proc. Inst. Civil Eng. Waste Resour. Manag. 170, 3–14. doi: 10.1680/jwarm.16.00006
Cundy, A. B., Bardos, R. P., Church, A., Puschenreiter, M., Friesl-Hanl, M., Müller, I., et al. (2013). Developing principles of sustainability and stakeholder engagement. J. Environ. Manage. 129, 283–291.
Cundy, A. B., Bardos, R. P., Puschenreiter, M., Mench, M., Bert, V., Friesl-Hanl, W., et al. (2016). Brownfields to green fields: realising wider benefits from practical contaminant phytomanagement strategies. J. Environ. Manage. 184, 67–77. doi: 10.1016/j.jenvman.2016.03.028
Cundy, A. B., and Croudace, I. W. (2017). The fate of contaminants and stable Pb isotopes in a changing estuarine environment: 20 years on. Environ. Sci. Technol. 51, 9488–9497. doi: 10.1021/acs.est.7b00973
Defra (2012). Tackling Water Pollution From the Urban Environment. Consultation Outcome. London: Defra.
Department of Conservation (2019). Fox Landfill Riverbed Clean-up, Department for Conservation. Available online at: https://www.doc.govt.nz/news/stories/2019/fox-landfill-riverbed-cleanup/ (accessed January 3, 2020)Google Scholar
Dixon, M., Morris, R. K. A., Scott, C. R., Birchenough, A., and Colclough, S. (2008). Managed realignment–lessons from Wallasea, UK. Proc. Inst. Civil Eng. Marit. Eng. 161, 61–71. doi: 10.1680/maen.2008.161.2.61
Faivre, N., Fritz, M., Freitas, T., Boissezon, B., and Vandewoestijne, S. (2017). Nature-based solutions in the EU: innovating with nature to address social, economic and environmental challenges. Environ. Res. 159, 509–518. doi: 10.1016/j.envres.2017.08.032
Ferronato, N., and Torretta, V. (2019). Waste mismanagement in developing countries: a review of global issues. Int. J. Environ. Res. Public Health 16:1060. doi: 10.3390/ijerph16061060
Gooddy, D. C., Macdonald, D. M. J., Lapworth, D. J., Bennett, S. A., and Griffiths, K. J. (2014). Nitrogen sources, transport and processing in peri-urban floodplains. Sci. Total Environ. 494–495, 28–38. doi: 10.1016/j.scitotenv.2014.06.123
Gu, B., Jiang, S., Wang, H., Wang, Z., Jia, R., Yang, J., et al. (2017). Characterization, quantification and management of China’s municipal solid waste in spatiotemporal distributions: a review. Waste Manage. 61, 67–77. doi: 10.1016/j.wasman.2016.11.039
Gupta, N., Yadav, K. K., and Kumar, V. (2015). A review on current status of municipal solid waste management in India. J. Environ. Sci. 37, 206–217. doi: 10.1016/j.jes.2015.01.034
Hakstege, P. (2013). Climate Change Adaptation as it Affects the Dredging Community. Port Planning, Design and Construction, 56 th Edn, February 2013. Available online at: https://www.porttechnology.org/technical-papers/climate_change_adaptation_as_it_affects_the_dredging_community/ (accessed January 3, 2020)Google Scholar
Harrison, C., and Davies, G. (2002). Conserving biodiversity that matters: practitioners’perspectives on brownfield development and urban nature conservation in London. J. Environ. Manage. 65, 95–108. doi: 10.1006/jema.2002.0539
Hartig, J. H., Krueger, A., Rice, K., Niswander, S. F., Jenkins, B., and Norwood, G. (2012). Transformation of an Industrial Brownfield into an ecological buffer for Michigan’s only Ramsar Wetland of international importance. Sustainability 4, 1043–1058. doi: 10.3390/su4051043
Hassan, A., Pariatamby, A., Ahmed, A., Shnada Auta, H., and Hamid, F. S. (2019). Enhanced bioremediation of heavy metal contaminated landfill soil using filamentous fungi consortia: a demonstration of bioaugmentation potential. Water Air Soil Pollut. 230:215. doi: 10.1007/s11270-019-4227-5
Hoornweg, D., Bhada-Tata, P., and Kennedy, C. (2013). Environment: waste production must peak this century. Nature 502, 615–617. doi: 10.1038/502615a
International Organization for Standardization [ISO] (2017). Soil Quality–Sustainable Remediation. ISO 18504:2017. Available online at: www.iso.org/standard/62688.html (accessed January 3, 2020)Google Scholar
Jambeck, J. R., Geyer, R., Wilcox, C., Siegler, T. R., Perryman, M., Andrady, A., et al. (2015). Plastic waste inputs from land into the ocean. Science 347, 768–771. doi: 10.1126/science.1260352
Kennish, M. J. (2002). Environmental threats and environmental future of estuaries. Environ. Conserv. 29, 78–107. doi: 10.1017/S0376892902000061
Latham, D., Milburn, C., Munro, B., Wilson, J., Sherry, S., and Baker, K. (2016). Creating inter-tidal and freshwater habitat on a brownfield site. Proc. Inst. Civil Eng. 169, 185–192. doi: 10.1680/jcien.16.00001
Leger, C., Balch, C., and Essex, S. J. (2016). Understanding the planning challenges of brownfield development in coastal urban areas of England. Plan. Pract. Res. 31, 119–131. doi: 10.1080/02697459.2016.1146428
Levi, D., and Kocher, S. (2006). The use of coastal brownfields as nature preserves. Environ. Behav. 38, 802–819. doi: 10.1177/0013916505285217
Li, X., Bardos, R. P., Cundy, A. B., Harder, M. K., Doick, K. J., Norrman, J., et al. (2019). Using a conceptual site model for assessing the sustainability of brownfield regeneration for a soft reuse: a case study of Port Sunlight River Park (U.K.). Sci. Total Environ. 652, 810–821. doi: 10.1016/j.scitotenv.2018.10.278
Lord, R. A. (2015). Reed canary grass (Phalaris arundinacea) outperforms Miscanthus or willow on marginal soils, brownfield and non-agricultural sites for local, sustainable energy crop production. Biomass Bioenergy 78, 110–125. doi: 10.1016/j.biombioe.2015.04.015
Lord, R. A., Atkinson, J., Lane, A., Scurlock, J., and Street, G. (2008). “Biomass, remediation, re-generation (BioReGen Life Project): reusing Brownfield sites for renewable energy crops,” in Proceedings of the 08th GeoCongress, March 9–12, Vol. 177, (New Orleans, LA: ASCE Geotechnical Special Publication), 527–534.
Machado, A. A., Spencer, K., Werner, K., Toffolon, M., and Zarfl, C. (2016). Metal fate and effects in estuaries: a review and conceptual model for better understanding of toxicity. Sci. Total Environ. 541, 268–281. doi: 10.1016/j.scitotenv.2015.09.045
Maco, B., Bardos, R. P., Coulon, F., Erickson−Mulanax, E., Hansen, L. J., Harclerode, M., et al. (2018). Resilient remediation: addressing extreme weather and climate change, creating community value. Remediation 29, 7–18. doi: 10.1002/rem.21585
Mahar, A., Wang, P., Ali, A., Awasthi, M. K., Lahori, A. H., Wang, Q., et al. (2016). Challenges and opportunities in the phytoremediation of heavy metals contaminated soils: a review. Ecotoxicol. Environ. Safety 126, 111–121. doi: 10.1016/j.ecoenv.2015.12.023
Martin, J., Baptist, T., Gerkema, B. C., van Prooijen, D. S., van Maren, M., van Regteren, K., et al. (2019). Beneficial use of dredged sediment to enhance salt marsh development by applying a ‘Mud Motor’. Ecol. Eng. 127, 312–323. doi: 10.1016/j.ecoleng.2018.11.019
Michalak, A. M., and Kitanidis, P. K. (2002). “Application of Bayesian inference methods to inverse modeling for contaminant source identification at Gloucester Landfill, Canada,” in Computational Methods in Water Resources XIV, Vol. 2, eds S. M. Hassanizadeh, R. J. Schotting, W. G. Gray, and G. F. Pinder (New York, NY: Elsevier Sci.), 1259–1266.
Mitchell, R. J., Richardson, E. A., Shortt, N. K., and Pearce, J. R. (2015). Neighborhood environments and socioeconomic inequalities in mental well-being. Am. J. Prev. Med. 49, 80–84. doi: 10.1016/j.amepre.2015.01.017
Nicholls, R. J., Beaven, R., Stringfellow, A., Spencer, K. L., Tarrant, O., and Cope, S. (2020). “Coastal landfills, rising sea levels and shoreline management: a challenge for the 21st Century,” in Proceedings of Coastal Management 2019: Joining Forces to Shape our Future Coasts: Coastal Management 2019, eds N. Hardiman, and Institute of Civil Engineers (London: Institute of Civil Engineers), 391–404.
NICOLE, and COMMON FORUM (2013). Risk-Informed and Sustainable Remediation. Joint Position Statement by NICOLE and COMMON FORUM 2013. Available online at: www.commonforum.eu/Documents/DOC/PositionPapers/1177_DDC_FLYER_SR_Joint_snijlijn_ def_2.pdf (accessed June 9, 2013).
Njue, C. N., Cundy, A. B., Smith, M., Green, I. D., and Tomlinson, N. (2012). Assessing the impact of historical coastal landfill sites on sensitive ecosystems: a case study from Dorset, Southern England. Estuarine Coastal Shelf Sci. 114, 166–174. doi: 10.1016/j.ecss.2012.08.022
O’Connor, D., Zheng, X., Hou, D., Shen, Z., Li, G., Miao, G., et al. (2019). Phytoremediation: climate change resilience and sustainability assessment at a coastal brownfield redevelopment. Environ. Int. 130:104945. doi: 10.1016/j.envint.2019.104945
O’Shea, F. T., Cundy, A. B., and Spencer, K. L. (2018). The contaminant legacy from historic coastal landfills and their potential as sources of diffuse pollution. Mar. Pollut. Bull. 128, 446–455. doi: 10.1016/j.marpolbul.2017.12.047
Peer, W. A., Baxter, I. R., Richards, E. L., Freeman, J. L., and Murphy, A. S. (2005). “Phytoremediation and hyperaccumulator plants,”in Molecular Biology of Metal Homeostasis and Detoxification, eds M. J. Tamas and E. Martinoia. Berlin: Springer, 299–340. doi: 10.1007/4735_100
Pinch, P., and Munt, I. (2002). Blue belts: an agenda for ‘Waterspace’ planning in the UK. Plan. Pract. Res. 17, 159–174. doi: 10.1080/02697450220145922
Premier, V., de Sousa Machado, A. A., Mitchell, S., Zarfl, C., Spencer, K. L., and Toffolon, M. (2019). A model-based analysis of metal fate in the Thames Estuary. Estuaries Coasts 42:1185. doi: 10.1007/s12237-019-00544-y
Sam, K., and Zabbey, N. (2018). Contaminated land and wetland remediation in Nigeria: opportunities for sustainable livelihood creation. Sci. Total Environ. 639, 1560–1573. doi: 10.1016/j.scitotenv.2018.05.266
Sarkis, J., Bai, C., Lopes, de Sousa Jabbour, A. B., Chiappetta Jabbour, C. J., and Sobreiro, V. A. (2016). Connecting the pieces of the puzzle toward sustainable organizations: a framework integrating OM principles with GSCM. Benchmark. Int. J. 23, 1605–1623. doi: 10.1108/bij-04-2015-0033
SedNet (2004). in Contaminated Sediments in European River Basins. Report, Deliverable D5.1 From the SedNet (European Sediment Network) Project, eds W. Salomons and J. Brils 69. Available online at: https://sednet.org/wp-content/uploads/2016/03/Sednet_booklet_final_2.pdf (accessed January 3, 2020).
Sellers, G., Hutchings, T. R., and Moffat, A. J. (2006). Learning From Experience: Creating Sustainable Urban Greenspaces From Brownfield Sites. WIT Transactions on Ecology and the Environment, Vol. 94. London: WIT Press, 163–172. doi: 10.2495/BF060161
Solecki, W. D., Rosenzweig, C., Parshall, L., Pope, G., Clark, M., Cox, J., et al. (2005). Mitigation of the heat island effect in urban New Jersey. Glob. Environ. Chang. Part B Environ. Hazard. 6, 39–49. doi: 10.1016/j.hazards.2004.12.002
Song, Y., Hou, D., Zhang, J., O’Connor, D., Li, G., Gu, Q., et al. (2018). Environmental and socio-economic sustainability appraisal of contaminated land remediation strategies: a case study at a mega-site in China. Sci. Total Environ. 610–611, 391–401. doi: 10.1016/j.scitotenv.2017.08.016
Spencer, K. L., Dewhurst, R. E., and Penna, P. (2006). Potential impacts of water injection dredging on water quality and ecotoxicity in Limehouse Basin, River Thames, SE England, UK. Chemosphere 63, 509–521. doi: 10.1016/j.chemosphere.2005.08.009
Su, Y., Zhang, Z., Wu, D., Zhan, L., Shi, H., and Xie, B. (2019). Occurrence of microplastics in landfill systems and their fate with landfill age. Water Res. 164:114968. doi: 10.1016/j.watres.2019.114968
Sutton-Grier, A. E., Wowk, K., and Bamford, H. (2015). Future of our coasts: the potential for natural and hybrid infrastructure to enhance the resilience of our coastal communities, economies and ecosystems. Environ. Sci. Policy 51, 137–148. doi: 10.1016/j.envsci.2015.04.006
Tack, F. M. G., and Bardos, P. (2020). “Overview of soil and groundwater remediation,” in Soil and Groundwater Remediation Technologies – A Practical Guide: ISBN 9780367337407, eds S. O. Yong, J. Rinklebe, D. Hou, D. C. W. Tsang, and F. M. G. Tack (London: CRC Press).
Tan, Z., Ka-Lun Lau, K., and Ng, E. (2016). Urban tree design approaches for mitigating daytime urban heat island effects in a high-density urban environment. Energy Build. 114, 265–274. doi: 10.1016/j.enbuild.2015.06.031
Tang, Y., Chen, A., and Zhao, S. (2016). Carbon storage and sequestration of urban street trees in Beijing, China. Front. Ecol. Evol. 4:53. doi: 10.3389/fevo.2016.00053
Todaro, F., De Gisi, S., and Notarnicola, M. (2016). Contaminated marine sediments: waste or resource? An overview of treatment technologies. Proc. Environ. Sci. Eng. Manage. 3, 157–164.
Tolnai, G. (2018). Budapest’s fragmented riverfront renewal: western trends interspersed with post-socialist characteristics. Belgian. J. Geogr. 4. doi: 10.4000/belgeo.21210
U.S. Environmental Protection Agency (2019). RE-Powering America’s Land Initiative: December 2019 Benefits Matrix. Available online at: https://www.epa.gov/re-powering/re-powering-benefits-matrix (accessed December 2019).
U.S. Government Accountability Office [GAO] (2019). SUPERFUND: EPA Should Take Additional Actions to Manage Risks from Climate Change GAO-20-73. Washington, DC: GAO, 66.
Vane, C., Beriro, D., and Turner, G. (2015). Rise and fall of mercury (Hg) pollution in sediment cores of the Thames Estuary, London, UK. Earth Environ. Sci. Trans. R. Soc. Edinb. 105, 285–296. doi: 10.1017/S1755691015000158
Vegter, J., Lowe, J., and Kasamas, H. (eds) (2002). Sustainable Management of Contaminated Land: An overview report. Wien: Austrian Federal Environment Agency on behalf of CLARINET.
Waldschläger, K., Lechthaler, S., Stauch, G., and Schüttrumpf, H. (2020). The way of microplastic through the environment – application of the source-pathway-receptor model (review). Sci. Total Environ. 713:136584. doi: 10.1016/j.scitotenv.2020.136584
Wilkes, P., Disney, M., Vicari, M. B., Calders, K., and Burt, A. (2018). Estimating urban above ground biomass with multi-scale LiDAR. Carbon Balance Manag. 13:10. doi: 10.1186/s13021-018-0098-0
Wille, E. (2018). “Flooding risks at old landfill sites: linear economy meets climate change,” in Proceedings of the 4th International Symposium on Enhanced Landfill Mining, Mechelen.
Woods, R. (2012). Brownfield sites and moth diversity in the Tees Estuary. Entomologist’s Rec. J. Var. 124, 89.
Zhao, M., Kong, Z.-H., Escobedo, F. J., and Gao, J. (2010). Impacts of urban forests on offsetting carbon emissions from industrial energy use in Hangzhou, China. J. Environ. Manage. 91, 807–813. doi: 10.1016/j.jenvman.2009.10.010
Keywords: risk management, coastal management, gentle remediation options, sustainable remediation, sustainability linkage, coastal landfill sites, phytomanagement, brownfield
Citation: Bardos P, Spencer KL, Ward RD, Maco BH and Cundy AB (2020) Integrated and Sustainable Management of Post-industrial Coasts. Front. Environ. Sci. 8:86. doi: 10.3389/fenvs.2020.00086
Received: 02 March 2020; Accepted: 26 May 2020;
Published: 16 June 2020.
Edited by:
Andrew Hursthouse, University of the West of Scotland, United KingdomReviewed by:
Stanislav Martinat, Cardiff University, United KingdomRichard Alastair Lord, University of Strathclyde, United Kingdom
Copyright © 2020 Bardos, Spencer, Ward, Maco and Cundy. This is an open-access article distributed under the terms of the Creative Commons Attribution License (CC BY). The use, distribution or reproduction in other forums is permitted, provided the original author(s) and the copyright owner(s) are credited and that the original publication in this journal is cited, in accordance with accepted academic practice. No use, distribution or reproduction is permitted which does not comply with these terms.
*Correspondence: Andrew B. Cundy, QS5DdW5keUBzb3Rvbi5hYy51aw==