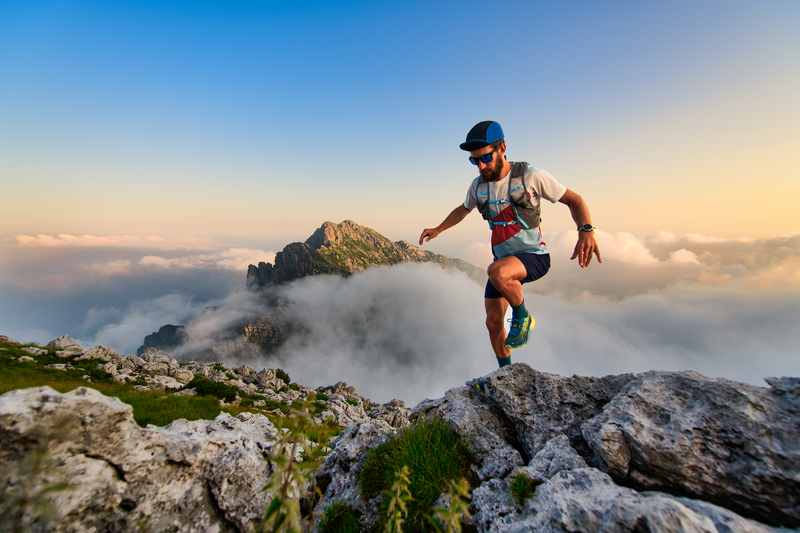
95% of researchers rate our articles as excellent or good
Learn more about the work of our research integrity team to safeguard the quality of each article we publish.
Find out more
SYSTEMATIC REVIEW article
Front. Environ. Sci. , 12 May 2020
Sec. Water and Wastewater Management
Volume 8 - 2020 | https://doi.org/10.3389/fenvs.2020.00044
Microbial electrochemical systems (MES) can be described as a combination of technologies with the unique ability to use microorganisms to conduct the transformation between chemical and electric energy. This property holds the promise to offer an alternative to the use of fossil fuels in the generation of electricity and hazardous compounds in the production of chemical products. In this review, the fundamental aspects to describe the mechanisms involved in the MES microbe–electrode interaction are presented. Furthermore, a detailed discussion on the current classification strategies is performed including the techniques used at distinctive characterization levels. Also, the implementation of a dual-iterative bibliographic analysis was conducted. The first iteration of the bibliographic analysis was considered from a historical perspective, allowing one to identify, in a systematic manner, the main research areas related to MES. In the second iteration, the previously identified areas were surveyed in order to obtain a representative sample for the analysis and identification of trends and main research objectives of MES technologies. The MES areas that displayed the highest growth rate value are those related to “wastewater,” “wastewater treatment,” and “extracellular electron transfer,” while “system (configuration/application)” and “microbial fuel cell” reported the highest number of related documents.
The ability of microorganisms to derive electricity from their vital activities was initially described by English botanist Potter (1911). In this work, he measured the electric potential of Saccharomyces cerevisiae and Escherichia coli, using a galvanic cell with platinum electrodes, attributing his observations to the disintegration of organic compounds under proper conditions. A similar principle was employed by Cohen (1931) to demonstrate that the introduction of a suitable substance, such as potassium ferricyanide or benzoquinone, in a bacterial electrical half-cell, would maintain a reduction–oxidation system on the medium, thus producing an increase in the overall electrical capacity and intensity. Three decades later, the works of Sisler (1961, 1962); Davis and Yarbrough (1962), and Davis (1963) provided a proof of concept for the generation of electricity using different microorganisms. These authors coincided that the reduction–oxidation potential was related to the enzymatic activity of the culture, as a response to the interaction with the medium, this system was denominated microbial fuel cell (MFC). Upon the premise of these works, the promise to exploit microorganisms as a viable source of electricity gained academic, political, and economic interest.
However, it was not until the early 1990s that MFC ensuing systems and technologies—usually referred to as bio-electrochemical systems/technologies (BES/BET) or microbial electrochemical systems/technologies (MES/MET)—achieved public attention, boosting advances in the identification and use of microorganisms, systems configuration, and material science. While in this work, MES is used as the generic term encompassing all of the above concepts, it is important to establish that the notion of system is related to advances oriented at an application-level perspective, and technology deals with the development of tools directed to a domain not specific to a knowledge area, with implications in the conception, evaluation, and development of systems. In this sense, a technology can be used in a broad definition spectrum, while a system is a specific concept which is defined by a combination of technologies characterized by the specific pursued application. Within the last two decades, MES have experienced an exponential increase in the amount of scientific publications, covering diverse domains such as energy production, chemical synthesis/catalysis, water treatment, sensors, among others. Despite current progress, MES are still far-fetched from integration to real-life and commercial application (Holtmann and Harnisch, 2018; Mohan et al., 2019). This is particularly challenging due to the complexity of the multidisciplinary nature of the MES approach (Koch and Harnisch, 2016b), requiring efforts on the standardization of terms and characterization processes (Schröder et al., 2015).
From this perspective, the present review provides a conceptual approach of (i) the mechanisms involved in MES, (ii) classification and characterization techniques, (iii) current trends in MES research, and (iv) future perspectives of MES development.
The underlying principle of MES relies in the ability of microorganisms to perform the transformation between chemical energy and electrical energy. In this transformation, electrons are used as the exchange currency between the cell and the electrical interface; this capability is derived from the mechanisms involved in the respiration process. Microorganisms have evolved different strategies to respond to the energetic demands required for survival (Schoepp-Cothenet et al., 2013); in aerobic respiration, microorganisms allow the access of soluble molecules within the cell structure that act as electron acceptor/donor (AD). Inside the cell, different electron transfer chains interact at the ionic level to complete the biological energetic cycle; moreover, some microorganisms are able to exchange electrons with molecules that are non-permeable for the cell membrane, thus, cannot be introduced into the cell, through a process known as extracellular/external electron transfer (EET) (White et al., 2016; Kumar et al., 2017).
From the perspective of electrochemistry, the interactions between an electrode and a microorganism can be of either capacitive (microorganism as a dielectric interface) or faradaic (microorganism performs an EET process) nature; while sole capacitive interactions are rarely studied in microbial electrochemistry (although convenient for detection and characterization of biological systems), the combination of capacitive and faradaic interactions are used to define the electron dynamics in MES. Usually, the rationale of the EET processes involved in MES include exclusively the faradic interactions; this is because the energetic overtake allowed by the EET can be further used for degradation of substrate, microbial electrocatalysis (MECat), or microbial electrosynthesis (MESyn), to produce complex compounds through microbially catalyzed reactions.
In MES, the electron transfer chains are extended outside the cell boundaries to allow the discharge and uptake of electrons to a conductive surface (electrode) (Lovley, 2012; Kracke et al., 2015; Pankratova and Gorton, 2017). This process can be analogously described to that of a galvanic cell: two chambers separated by an ion-exchange membrane, in which an electrochemical cell retrieves electrical energy from redox reactions occurring through the electrode's closed-loop system. Microorganisms that are equipped with EET mechanisms, coupled with their energetic cycle, are known as electroactive (Koch and Harnisch, 2016b).
The EET mechanisms developed at the MES electrode–microorganism interface (Figure 1) can be of anodic (substrate oxidation) (Schröder, 2007) or cathodic (substrate reduction) (Rosenbaum et al., 2011) nature. Anodic processes in MES, such as the process executed in MFCs, have been largely recognized due to the resultant electric output (Kumar et al., 2016). Generally, in environments with lower availability of soluble electron acceptors, microorganisms catalyze reactions toward the anode by transferring the produced electrons to the electrode, the positive electron flux generates a measurable electrical current, these microorganisms are known as electricigens or exoelectrogens (Kumar et al., 2016). In contrast, in the cathodic processes, electrons are transferred in the direction of the microorganism. In this case, microorganisms can be perceived as to “feed” from electricity, which have gained them the name electrotrophs (Lovley, 2011).
Figure 1. Schematic representation of the different mechanisms of EET in MES. (A) Direct EET. (B) Nanowire (Direct) EET. (C) Mediated EET. (D) No EET.
The ionic interplay required for the EET process in MES has been assumed to be guided by specialized redox molecules (Liu et al., 2018). Whenever those molecules are directly bounded with the cell structure, the process is considered as direct electron transfer (DEET). In the case of attachment of the molecules to the cell membrane (Figure 1A), the close contact of the electrode and the cell is required. This limits the EET to be performed only by the cells distributed directly on the surface of the electrode; thus, the EET process cannot be extended beyond the first layer of a (multilayered) cell aggregate, a common situation in biofilms. Some microorganisms, such as Shewanella oneidensis, have been observed to develop membrane-attached appendages, these highly conductive pili-like structures, known as nanowires (Reguera et al., 2005; Gorby et al., 2006; El-Naggar et al., 2010), have been proposed to be accountable for the electron transport along various cell lengths (Figure 1B).
However, aggregation of cells over an electrode surface is not always possible, in this case, the cells may secrete membrane-soluble molecules that serve as electron shuttles (Glasser et al., 2017), these molecules act as redox mediators between the cell and the electrode, avoiding the need of a direct contact with the electrode (Figure 1C); this process is known as indirect electron transfer (IEET). Additionally, AD in MES can be produced and recovered through redox processes originating in the electrode; electroactive molecules, naturally present or artificially introduced in the environment, are oxidized or reduced by the electrode and can be used by the cell though respiration or EET processes (Figure 1D). Similarly, the ionic interaction between microorganisms and electrodes can be achieved by the modification of environmental parameters, such as pH or oxygen pressure, using redox reactions controlled by the electrode.
The internal machinery behind the EET mechanisms involved in MES remains only partially understood. Most of current knowledge on this phenomenon has been obtained from the study of model organisms within the genus Shewanella and Geobacter (Lovley, 2012; Liu et al., 2018). The role of different complexes in EET, such as multi-heme cytochromes (Chong et al., 2018), quinones (Newman and Kolter, 2000), and flavins (Von Canstein et al., 2008), among others, has been extensively studied; however, none of these mechanisms extensively explain the intricate process of EET; the increasing diversity of characterized microorganisms poses an enormous challenge, as new processes can be discovered; thus, a definitive model of electroactivity needs to be discussed (Koch and Harnisch, 2016b).
The following section provides an overview of different classification approaches and highlights the complexity of the concepts used to understand electroactivity in microorganisms.
The diversity of MES, in terms of applications and involved disciplines, has propitiated the use of multiple characterization parameters and motley terminologies (Schröder et al., 2015). Counterintuitively, this multidimensional approach burdens the determination of a generally accepted definition to describe the sense of electroactivity in a microorganism (Koch and Harnisch, 2016b).
Different strategies for classification of MES have been proposed; for instance, Schröder et al. (2015) suggest a designation relying upon the identification of the technology in which a system is based (Figure 2). The definition of an electrochemical microbial technology (MET) implies the functional (ionic) connection between microorganism and (at least one) electrode. Two categories are recommended under this classification, primary MET, in which the direct ionic interaction is achieved, including both EET mechanisms DEET and IEET; and secondary MET, which refers to MES that allow a more indirect interaction, such as the modification of environmental conditions. From a methodological ground, this classification can be understood to be determined by the applied electrode potential; for primary MET, the electrode potential stands within the thermodynamic range of the microorganism, contrary to secondary MET, which requires highly positive or negative electrode potentials. The identification of potential physiological windows are specific to each microorganism; for that reason, the recognition of the proposed primary and secondary MET as a classification, whereas methodologically well founded, requires that the researcher would be able to identify, almost unequivocally, the mechanism responsible for the EET process, but with the risk of incurring in misclassification and overestimation of the electrochemical properties.
Figure 2. Schematic organization of the MES classification based on the technology used in the system.
From a methodological perspective, MES can be identified based on the direction of the electron flux of the system (Rosenbaum and Franks, 2014). Thus, MES that provide a positive electron flux toward an electrode can be considered as part of a power-producing system, in opposition to MES that use energy to increase the performance of a catalytic process, identified as a power-consuming system (Figure 3). A further classification layer can be considered from the context of the desired application objective (Figure 4), as proposed by Mohan et al. (2019) and Bajracharya et al. (2016), for instance, electrogenesis systems, termed microbial fuel cells (MFC), in which microorganisms are used to generate electrical energy (Bagchi and Behera, 2019); microbial electrosynthesis (MESyn) systems, in which microorganisms are used to promote the conversion rates of diverse compounds by directly providing them with electrical energy, such as electro-fermentation (EF) systems (Kumar et al., 2018); microbial electrolysis system/cell (MEC) (Kadier et al., 2019), in which microorganisms are used to generate chemical products from their native state, generally used as chemical or fuel, through an electrochemical process, such as electro-hydrogenesis (production of hydrogen by an electrochemical process) systems and electro-methanogenesis (production of methane by an electrochemical process) systems; microbial desalination system/cell (MDC) (Sharma et al., 2019), in which salt molecules are separated from water, based in the transfer of ionic species in proportion to current flux either applied to or generated by the microorganism; and bio-electrochemical treatment systems (Xu et al., 2016), in which microorganisms are used to degrade organic/inorganic compounds or to separate chemical species from water, this reaction can be driven by an electrical energy input or can provide energy as an output product of the process. In this sense, some MES could be assigned to diverse classification categories; for example, it is usual that in MES that are designed to generate electrical energy, parallel processes are expected to, at the same time, recover value products (Mohanakrishna et al., 2010; Bajracharya et al., 2016). In this case, the comparison with systems designed for a single application might be undesirable, as the focus of the system can be diverted to an efficiency discussion, neglecting the contribution when multiple outcomes are possible.
Figure 3. Schematic organization of the MES classification based in the direction of the electron flux.
Figure 4. Schematic organization of the MES classification based in the application objective and exemplary diagrams for each application.
Besides these functionality-driven parameters, a mechanistic evaluation for characterization of MES should be considered toward comparison and assessment of the different systems. To this extent, the use of a common language framework is needed. This issue was openly addressed by Schröder et al. (2015). In that work, the authors recommended to limit the use of the prefix bio to the broad group (field) comprising all aspects where the interface of a biological element and conventional electrochemistry is present, suggesting that, for every specific biological component (subfield), the prefix bio should be avoided and, in turn, the identifier of the component should be used (e.g., tissue electrochemistry, enzyme electrochemistry, microbial electrochemistry, etc.). Following this recommendation, the subfield of microbial electrochemistry is referred to describe the study of the fundamental interactions between microorganisms and electrodes. In a strict sense, every term derived from a specific subfield should be designated using the identifier assigned to the specific subfield; for instance, systems subordinated to the subfield of microbial electrochemistry ought to be referred to as microbial electrochemical systems (MES); similarly, the production and degradation of products subordinated to an electrochemical reaction driven by microorganisms should be, respectively, addressed as microbial electrosynthesis (MESyn) and microbial electrocatalysis (MECat). Here, further caution should be considered, as the acronym MES is also used in different reports to refer to microbial electrosynthesis; thus, we recommend the use of the proposed acronyms MESyn and MECat to identify such processes.
Therefore, the characterization of MES can be derived from system-level performance indicators, classically defined by parameters such as current density, exchange current density, resistance, capacitance, impedance, etc. In MES, these parameters are influenced by the interaction area between microorganisms and electrodes, conventionally addressed by using geometric factors. However, special attention should be taken when using more complex 3D structures, such as porous electrodes, conductive fabrics, and machined microstructures (Moß et al., 2019b); the specific structure of the electrodes can potentially increase the contact area available for the electrochemical interaction (Champigneux et al., 2018); thus, an appropriate methodology should be used to assess and calculate correction factors based on the topology of the electrode (Sharma et al., 2014). This representation requires that the characterized system supports the effective attachment of bacteria to the electrode and is prone to neglect the effects of multilayer aggregation; moreover, it needs prior knowledge of the electrochemical microorganism-electrode electron transfer mechanisms.
From this perspective, a system-level approach toward characterization and classification of MES is advantageous from an instrumentation viewpoint and, in principle, could be extrapolated to a cell-level perspective. Furthermore, the characterization involved in the system-level approach can be useful to assess application-specific characteristics, which can be used for economic feasibility studies (Zhang and Angelidaki, 2016), as the recent work presented by Trapero et al. (2017). However, the electrochemical behavior displayed by a single cell can differ considerably within MES, depending on factors such as the distance of the microorganism to the electrode (electron transfer mechanism), environmental parameters (e.g., temperature, salinity, pH), formation of biofilm, among others, as well as specific system characteristics, such as materials, architecture, and configuration. This could cause one to ignore important mechanisms of EET, for example, layered aggregation of cells or early substrate depletion, moreover, when the different aspects regarding system performance and intrinsic microbial metabolism are considered.
In the opinion of Koch and Harnisch (2016b), a proper description would be determined by individual characterization of cells. Howbeit this approach has been demonstrated to be achievable by a few works (Jiang et al., 2010; Liu et al., 2010; Mclean et al., 2010; Gross and El-Naggar, 2015), the technical challenges to replicate these experiences are a major pitfall. As a rationale to that limitation, Koch and Harnisch (2016b) considered the use of production rates, associated to the electron flux in MES, to offer a suitable strategy to approach a basic set of characteristics to report in a MES setting, for instance, electron per time unit (current density), and product or cell yield per electron. These characteristics can be related (although not necessarily linked) to the electroactivity of a given microorganism. Furthermore, the authors highlighted that such measurements might lean on the interests of the researcher; thus, different parameters would fit the needs of different fields and would not represent the univocal identification of a species as electroactive.
Given this complex scenario on the current discussion toward an unambiguous definition of electroactivity in microorganisms, it might be considered that efforts to establish a rule of thumb to classify MES are, to some extent, futile. Rather, a synergistic approach should be considered, which, far from determining if a microorganism is electroactive—or not—should provide an indexed structure of characteristics to evaluate the properties of the different interactions involved in a MES setup that could account for the divergence of the multiple disciplinary approaches (Koch and Harnisch, 2016b).
To this extent, a correct identification of the microorganisms that are used in any MES setup must be performed. Different methods are currently used in systems biology to identify and classify microbial samples using well-established parameters. For instance, specific characteristics, such as the cell wall physical and chemical composition, biofilm structure, organelle identification, and viability assessment, can be evaluated by diverse staining methods. Furthermore, the determination of molecular markers and genetic structures of microorganisms can be achieved using diverse DNA/RNA sequencing techniques, which allows high-throughput identification and analysis of complex data, as well as to gauge information from highly diverse microbial populations, for example, the phylogenetical relatedness of a multi-sample population (Logan et al., 2019).
The identification of characterization techniques for MES can be hierarchically identified by the resolution of the applied measurement, this model was proposed by Harnisch and Rabaey (2012) for the study of methods to characterize electrochemically active biofilms. In MES, the lowermost layer is determined by the basic molecular interaction, that is, characterization at a molecular-level resolution (Figure 5A); the functional and structural characterization of these molecular naturally produced compounds can help to determine possible mechanisms of EET in different microorganisms (Millo, 2012). However, available research is currently mostly limited to outer membrane cytochromes (Urban and Klinqenberg, 1969; Hartshorne et al., 2007; Schuetz et al., 2009; Fonseca et al., 2019). Further characterization can be identified by the interactions and evaluation at the cell-level resolution (Figure 5B), which can be considered as the most accurate descriptive standard (Koch and Harnisch, 2016b). Despite this property, only few works have been able to effectively achieve this measurement resolution (Jiang et al., 2010; Liu et al., 2010; Mclean et al., 2010; Ryu et al., 2010; Gross and El-Naggar, 2015), which prompts researchers to question the technical advantages of this approach.
Figure 5. Schematic characterization of resolution hierarchical level characterization. (A) Molecule-level characterization. (B) Cell-level characterization. (C) Aggregation-level characterization. (D) System-level characterization.
An alternative to single-cell characterization can be suggested as an aggregation- or community-level characterization (Figure 5C); in this case, performance parameters, describing electron transfer mechanisms, are recommended to be calculated according to topological properties of the electrodes (Sharma et al., 2014; Champigneux et al., 2018; Moß et al., 2019b). To complete this concept, it should be acknowledged that not every MES setup, or every microorganism, is able to aggregate on an electrode surface; consequently, a surrogate to consider is the cell density indicator per volume unit. At the uppermost layer of the resolution identification, it would be reasonable to locate the system-level characterization (Figure 5D), in reference to system specific parameters, such as loading rates, conversion efficiency, production capacity, among others. Arguably, system-level characterization has been disregarded in the light of its inconvenience to assess the numerous interactions involved in MES; however, from an engineering perspective, characterization of system specific parameters allows a more pragmatic insight toward the evaluation of a real-life implementation, alleviating efforts toward economic and life cycle assessment exercises. Furthermore, some considerations are yet to be established in MES environments, such as the limiting growth rate of bacteria (Moß et al., 2019a), for instance, due to nutrient availability or growth-limiting factors such as quorum-sensing mechanisms.
From a technical context, different methods can be used in the characterization of MES (Ikeda and Kano, 2001; Logan et al., 2006; Sadik et al., 2009; Harnisch and Rabaey, 2012; Sharma et al., 2014; Sydow et al., 2014; Zhi et al., 2014; Schröder et al., 2015; Mozneb et al., 2019; Saito et al., 2019); some of these methods use techniques that allow the characterization at multiple resolution levels and can be considered as transversal, as identified in Table 1; these techniques are related but not limited to microorganism identification (Logan et al., 2019), imaging characterization (Hernandez et al., 2019), and electrochemical evaluation (Saito et al., 2019). Therefore, the specific strategy considered for the evaluation of MES at the different resolution levels might be approached from the perspective of the combination of such techniques; currently, different strategies have been used to evaluate specific characteristics of MES at the each of the proposed levels (Table 2); in most of the used strategies, different methods are employed to achieve the assessment of the parameters of interest. Some systems are used to monitor the development between two different levels (Mclean et al., 2010), while others are specific at the different resolution levels and might require special conditions for measurement.
In conclusion, the discussion toward a univocal definition of electroactivity, or even a basic set of characteristics, remains an unsolved issue. It must be emphasized that there is not a definitive classification procedure; however, the identification of the resolution characterization level might serve to denote specific features of diverse experimental and real-life application setups (Figure 5). The basic mechanistic implications of electroactivity in microorganisms can be typified under the parameters provided at the higher-resolution levels, i.e., molecule, cell, and aggregation level; such definitions need yet to be adopted by researchers. Current advances toward high-resolution systems have appeared as a promising alternative to identify microbe-specific properties; however, different aspects must be carefully evaluated at every experimental stage (Logan, 2012; Thomas, 2015). Furthermore, a basic revision of parameters for an appropriate report methodology must be considered (Harnisch and Rabaey, 2012; Sharma et al., 2014; Schröder et al., 2015; Koch and Harnisch, 2016b). Contrary to some of these opinions, reporting on the overall consumption or generation of energy and products of a MES setup might be useful as a basis for the technical evaluation of real-life applications, and can be subsumed within the last two levels of the characterization resolution identification, i.e., aggregation and system levels; however, such parameters do not suffice to explain specific characteristics of the EET mechanism of a microorganism; thus, generalizations derived from these levels should be avoided.
From this standpoint, additionally to a proper identification of the microorganism, or microorganism consortia, that is used in any individual work, researchers can profit from characterization systems that allow rapid and facile evaluation of microorganisms, and such systems should comply with specifications for the assessment of parameters at the cell or aggregation characterization resolution levels, such as electrochemical reaction or production rates, and, most importantly, ensure comparable environments for microorganisms that thrive in similar environmental conditions. Such a system not only would provide insights into the behavior of microorganisms at the electrode–microorganism interface but also will allow one to interpret the results of specific system and molecule resolution level parameters, as it would allow one to contrast the contribution of the microorganism and the individual properties of the system design or molecule interactions to the electrochemical reaction.
The diversification of MES and related technologies can be approached through an analysis of the historical evolution of scientific publications; to this end, a bibliometric mapping method based on co-occurrence of keywords (Noyons, 1999) was performed and analyzed for the present systematic review. Bibliometrics is a set of quantitative tools to describe different attributes of a (usually large) collection of bibliographic data (i.e., information about authors, organizations, countries, documents, sources, references and keywords) (Noyons, 1999). This collection of data is generally correlated; that is, two different documents can share various attributes; for instance, references, authors, or keywords, which are individually referred to as nodes. The connection of multiple nodes can be represented as a network, which, in turn, can be interpreted as a domain map to identify related works in relevant research areas (Börner et al., 2005).
Bibliometric analysis of MES (Wang et al., 2015) and MFC-related technologies (Md Khudzari et al., 2018) have been previously conducted. In both of these works, different perspectives of main bibliographic characteristics of academic publications were used to describe the status of MES research, providing information about leading journals productivity, as well as country and institution of MES research activities and collaborations; furthermore, the authors of these publications provided an overview of MES relevant technologies. These works can be considered complementary to each other, as they use different databases to retrieve information (i.e., Web of Science and Scopus).
The limitation of these preceding studies can be considered from the perspective of the methodology used for the identification of research topics, which is usually dependent on the researcher expertise and not a systematic approach. For this reason, in this work, we propose the evaluation of up-to-date publications based on the derived documents from the seminal publication on microbial electroactivity of Potter (1911) to establish a syntactic approach in order to describe current trends of MES.
To this extent, a bibliometric analysis was performed based on publications listed as directly citing the work of Potter, consigned in the Web of Science (WoS) core collection database. Data identified as author keyword was extracted from 329 documents. The different variants of source keywords were merged in order to avoid duplicity derived from plural forms and equivalent terms (e.g., microbial fuel cell = [microbial fuel cells, MFC, MFCs, microbial fuel cell (MFC), microbial fuel cells (MFC)]); in this way, 457 individual keywords were identified from the initial 607 excerpted (The complete dataset can be found in Table 1 of the Supplementary Material). The most frequent keywords were selected based on the occurrence of each, that is, the number of documents in which each word was used; keywords with more than 10 occurrences are summarized in Figure 6, which represented 25% of the total keyword occurrences of the surveyed documents (main keywords).
Figure 6. Summary of the occurrence of the most common author keywords derived from works citing the work of Potter (1911).
On the other hand, 62% of the identified keywords, with 9 or less occurrences each, were grouped in 11 different categories (category keywords), as shown in Table 3. The remaining 12% of keywords were related to other terms that were not directly connected to the electrochemical processes (e.g., mathematical model, challenges, low cost, etc.). This initial approach allows one to identify that current efforts toward water resources care and protection are among one of the most important topics in MES research, further analysis can be found in Table 1 of the Supplementary Material; additionally, fundamental studies oriented to the study of the fundamental processes involved in the bio-electrochemical interaction of bacteria remain occupying a pivotal role in MES research efforts. The integrative nature of current research activities can be recognized from Table 3, in which very well-defined roles are related to the identified categories.
Table 3. Categories related to the author keywords derived from works citing the work of Potter (1911) and the related keywords included in each category.
Further analysis of the identified keywords was conducted to establish prevailing research areas; for this purpose, the association strength of each keyword was evaluated, using proprietary software VOSViewer (Centre for Science and Technology Studies, Leiden University, The Netherlands) (Van Eck and Waltman, 2007, 2010). Figure 7 illustrates the resulting concept map to visualize the association relationships between main keywords and category keywords. In this map, four different clusters were identified.
Keywords arranged in the first cluster can be correlated to descriptors of architectural characteristics and application-oriented research works of MES; in a broad sense, this cluster can be directly associated to properties measurable using a system-level characterization approach. The second cluster can be identified with parameters attributed to cell and aggregation characterization resolution levels; in other words, it can be assumed that these works were designed to describe basic mechanisms of the cellular structure and physiology of microorganisms. Moreover, although different microbial species have been recognized as important targets in MES (Logan et al., 2019), items identified in this cluster are focused in the study of bacteria. The third cluster gathers documents represented by keywords specific to environmental applications, thus relevant to the resolution of system-level characterization. However, characterization strategies at the cell and aggregation resolution level were used in various studies; in consequence, another layer could be added, related to environmental interaction, viz., the symbiotic behavior of microorganisms, at different levels, with host ecosystems. Finally, the fourth cluster can be described by approaches pertaining to subcellular properties, which can be evaluated using molecule-level characterization strategies.
The cluster analysis suggests that the identified keywords represent multiple technical and methodological approaches and, thus, could be further used to trace the main research trends involved in MES; under this premise, a new bibliographic survey was performed using the WoS core collection.
Using the advanced search tool, provided by Clarivative analytics and available at the WoS Web page, a new iteration for the identification of relevant works was conducted. The main dataset was built with documents published before October 2019. The search query for this dataset identified 3,332 different documents labeled with the topic field tag bio-electrochemical systems/technologies or microbial electrochemical systems/technologies; acronyms were not considered for this selection. Deriving out of the main dataset, 17 different search result sets were created, based upon each of the identified keywords; each of the search query strings and the resulting number of documents are presented in Table 4. It is important to clarify that the number of publications in this study is only representative, although exhaustive; thus, it cannot be claimed that every publication related to MES is included, as some works might have been excluded due to the fact that some relevant works do not use the terms bio-electrochemical systems/technologies specifically as a topic tag identifier or are not included in the WoS database.
From the outcome of this survey, it can be observed that the query used to generate the dataset for system (configuration/application) returned the highest number of related documents, with 1,213 associated results, followed by microbial fuel cell (1,165), bacteria (keyword/strains) (981), and system (material/fabrication) (928). Figure 8 shows the cumulative number of publications per year during the interval between 1998 and 2018. Based on this information, it is clear that the number of publications per year, related to MES, exhibits a continuously increasing trend. The number of accumulated publications in 2018 was 60-fold the reported for 1998; furthermore, only in the last 4-year period, from 2014 to 2018, does the surveyed number of publications produced match all the works published before 2014.
Interestingly, the growth trends observed for the different areas revealed appealing information about the evolution of research interests during the given period; for instance, the accumulated number of publications for microbial fuel cell features a prominent growth rate in comparison with its counterparts. This demeanor can be numerically evaluated by calculating the ratio of the publications for a given period, for example, for a research area with slow growth rate, such as other microorganisms, the number of accumulated publications for 2008 was 25, and completed a total of 203 accumulated publications for 2018, compared to 31 publications for microbial fuel cell in 2008 and 987 in 2018; the growth rates for other microorganisms in this case is 8.12, contrasting with the growth rate of 31.84 for microbial fuel cell during the same period. The publication growth rate between 2008 and 2018 for the documents with the tag bio-electrochemical systems/technologies or microbial electrochemical systems/technologies subsumed in the main dataset is equal to 8.35, while for system (configuration/application) 9.14, bacteria (keyword/strains) 8.26, system (materials/fabrication) 10, analysis tools 7.58, biosensor 5.36, biofilm 12.36, synthesis/catalysis 14.79, other biomolecules 6.31, electron transfer 8.43, wastewater treatment 36.5, environmental 9.1, extracellular electron transfer 34.71, wastewater 38.33, renewable energy 11, and energy generation 13.5. Another interesting feature can be noticed when comparing the growth rate of system (configuration/application), which yielded the highest amount of documents accumulated in one area, with that of microbial fuel cell; the lower value of the former and the high number of found documents indicate that this research area can be considered as less active than the latter. These publication dynamics can also be noticed in other areas, such as analysis tools and bacteria (keyword/strains); for these cases, some level of research maturity can be assumed; consequently, despite the importance of such areas on the field, they are not expected to attract considerable new research. Another case that is especially considered is the area biosensor, which displayed the lowest growth rate; this indicates a low interest, which can be assumed to be related to the non-competitive results compared to well-established sensing technologies.
On the other hand, areas that displayed a very high growth rate value and a low accumulated publication number, i.e., wastewater, wastewater treatment, and extracellular electron transfer, can be assumed to be in a nascent stage; this means that they are expected to gather increasing attention in the next years. Complimentarily, areas with a very low number of publications and a relatively high growth rate, such as energy generation and renewable energy, are yet to be evaluated and cannot be assumed as nascent; this is due to the fact that the number of publications is not representative and the present trend can probably change. In Figure 9, the distribution of the yearly relative frequency of these publications per research area is represented as a percentual equivalent of the total amount of publications for each year, showing the specific trends in the evolution of research interests during the last two decades (Detailed analysis can be found in Table 2 of the Supplementary Material).
Within this context, it can be affirmed that MES is composed of a dynamic set of research areas that continue attracting attention from diverse fields. During the last decade, MES have transformed from the concept related to the development of biological batteries, to a complex group of technologies that integrates advanced methods in biology, chemistry, materials science, and engineering with the potential to radically change the traditional production industry.
Current trends in MES are oriented following two main objectives; the first objective is related to the management, treatment, and exploitation of wastewater and water resources affected by pollution. In this manner, characterization at the system-level resolution can provide valuable insights into the economic viability to integrate different technologies in real-life scenarios (Trapero et al., 2017; Batlle-Vilanova et al., 2019); such efforts will be of vital importance to validate the competitiveness of MES to stakeholders from the public and private sector, and such validation will be crucial toward the advancement of technology directed to complete the necessary readiness level to be qualified for use in an operational environment (Holtmann and Harnisch, 2018). The second objective is associated with the development of strategies and tools designed to understand and characterize the fundamental functioning of the processes involved in the microbial electron transfer mechanisms; this competence is the basis to accomplish some long-awaited purposes, such as the efficient, clean production of energy, and to conceive thoughtful methodologies to envision new applications.
The current advances in MES provide adequate phenomenological evidence of the capacity of some microorganisms to energetically interphase their environment using a multiplicity of mechanisms (Lovley, 2012; Kracke et al., 2015; Pankratova and Gorton, 2017). Although only few model microorganisms have been studied in detail (Lovley, 2012; Liu et al., 2018), it has been suggested that microbial electroactivity can be a more widespread characteristic than predicted (Cournet et al., 2010; Koch and Harnisch, 2016a). Furthermore, recent advances had demonstrated that it is possible to engineer microorganisms to develop electron transfer conduits (Zhang et al., 2019a); however, there is still a lack of fundamental knowledge to model these capacities into applications beyond the lab bench (Koch and Harnisch, 2016b).
The analysis of the dynamics in the different research area interest evolution revealed that, although MFC technologies are considered as the harbinger of current research efforts in MES, there is a strong interest to unveil the processes that underlie behind the electron transfer mechanisms that govern the microbe–electrode interactions in MES. The existing characterization strategies provide fundamental tools to describe such interactions, ranging from system-level resolution to aggregation-, cell- and molecule-level detailed characterization.
While empirical studies can be conducted to evaluate the suitability of microorganisms and microbial consortia for the transformation of products, either by synthesis of compounds or by production of energy, there is no standard methodology to address the fundamental questions of the EET capacity of microbial cells. The improvement in the design and use of advanced strategies of current technologies, such as nano-manipulation and fabrication, altogether with judicious multidisciplinary work, might open new possibilities for harvesting microorganisms to power MES progress.
The compelling interest in new MES applications anticipates a major role of these technologies for alleviating the current global ecological pressure. Furthermore, the commission of MES technologies, for relevant operational applications, will provide invaluable tools to breach economic and social disparities, serving as a foundation for sustainable industrial processes. Recent reports indicate the economic competitiveness of MES when compared with other industrial systems (Trapero et al., 2017; Batlle-Vilanova et al., 2019). In order to satisfy these demands, an integrated effort of research and industrial endeavors is required. This is challenging, due to a lack of assertive communication between current capacities of MES and a better understanding of them by stakeholders and industrial partners. MES research should also consider that, for the system to achieve a wider impact, the definition of standard terminology is required; by achieving this fundamental consensus, it would be easier to transfer the studied phenomenon of electron transfer to practical applications.
Currently, the achievements in microbial electrochemistry have allowed researchers to dramatically expand the performance of MES in terms of energy generation capacity, with a recorded range maxima between 15 and 17 W/m2 (Logan et al., 2019) for pure cultures, as well as recovery and synthesis of value products (heavy metals, nutrients, industrial chemicals, and gaseous fuels) (Jadhav et al., 2017; Kumar et al., 2018), with realistic economic potential (Harnisch et al., 2015); different reaction pathways have been described, which can contribute to the development of ecologically sustainable production and treatment of industrial compounds (Harnisch and Urban, 2018; Srikanth et al., 2018); this allows one to profile MES as an important alternative for future technologies, with advantages that can be well-regarded for stakeholders that include the diminishing of overall production and operational costs, associated with lower chemicals and energy consumption, and the reduction of the environmental impact and carbon footprint (Trapero et al., 2017).
Some of the advances that are expected in the following years pertain to the domain of wastewater treatment (Velvizhi, 2019) and hybrid systems for value product recuperation and energy production (Zhang et al., 2019b); novel technological approaches, such as microbial electro-Fenton systems (Hassan et al., 2019), have already proven enhanced efficiency for the treatment and removal of persistent organic pollutants from various wastewater effluents. The achievement of useful power densities has also been established by means of stack architectures (various MFCs connected in series or parallel) (Zhuang et al., 2012) as well as new architectures for generation and storage of energy (Fischer et al., 2018) that could compete within a segment of the power generation market, such as the generation of energy to charge personal electronic devices (e.g., cellphones or tablets). Recent advances in the development of low-cost tools (Nejatimoharrami et al., 2017; Segura and Osma, 2017) might accelerate the development of systems directed to evaluate, monitor, detect, and harvest the power of microorganisms.
Despite these achievements, some technical challenges are still required to be fulfilled in order to guide MES into a commercial application. Microbial resistance to antibiotics during exposure of microorganisms to pharmaceutical and medical wastewaters has not been thoughtfully investigated (Guo et al., 2018). Research in MES should be compelled to explore the advantages of different system architectures (Chen et al., 2019) and the use of cheaper materials (Palanisamy et al., 2019; Pareek and Mohan, 2019), as well as to continue the efforts for microorganism identification and characterization (Logan and Regan, 2006). Furthermore, as discussed in this work, systems that allow rapid and facile evaluation of microorganisms with comparable conditions will provide long-required tools to assess the contribution of each of the MES components in the overall system performance.
The future developments in MES demand advancement in the negotiation of a universal unified language. This approach would allow the mitigation of the effects of the use of confusing terminology, which would ultimately allow researchers to focus in specific design and experimentation tasks. Future applications of MES will include well-characterized components and properties; such descriptors will allow the systematic assessment to boost applications, wiring technologies for wastewater treatment, bioproduction, bioremediation, bioelectricity, and biocomputing.
All datasets generated for this study are included in the article/Supplementary Material.
All authors listed have made a substantial, direct, and intellectual contribution to the work, and approved it for publication.
This research was funded by the Colombian Ministry of Education and the Administrative Department of Science, Technology and Innovation, Colciencias, through the program for national doctorates, grant 567.
The authors declare that the research was conducted in the absence of any commercial or financial relationships that could be construed as a potential conflict of interest.
The Supplementary Material for this article can be found online at: https://www.frontiersin.org/articles/10.3389/fenvs.2020.00044/full#supplementary-material
Babauta, J., Renslow, R., Lewandowski, Z., and Beyenal, H. (2012). Electrochemically active biofilms: facts and fiction. A review. Biofouling 28, 789–812. doi: 10.1080/08927014.2012.710324
Bagchi, S., and Behera, M. (2019). “Microbial fuel cells,” in Bioelectrochemical Interface Engineering, eds R. Navanietha Krishnaraj and R. K. Sani (Hoboken, NJ: John Wiley & Sons, Inc.), 91–116.
Bajracharya, S., Sharma, M., Mohanakrishna, G., Dominguez Benneton, X., Strik, D. P. B. T. B., Sarma, P. M., et al. (2016). An overview on emerging bioelectrochemical systems (BESs): technology for sustainable electricity, waste remediation, resource recovery, chemical production and beyond. Renew. Energy 98, 153–170. doi: 10.1016/j.renene.2016.03.002
Batlle-Vilanova, P., Rovira-Alsina, L., Puig, S., Balaguer, M. D., Icaran, P., Monsalvo, V. M., et al. (2019). Biogas upgrading, CO2 valorisation and economic revaluation of bioelectrochemical systems through anodic chlorine production in the framework of wastewater treatment plants. Sci. Total Environ. 690, 352–360. doi: 10.1016/j.scitotenv.2019.06.361
Bellin, D. L., Sakhtah, H., Zhang, Y., Price-Whelan, A., Dietrich, L. E. P., and Shepard, K. L. (2016). Electrochemical camera chip for simultaneous imaging of multiple metabolites in biofilms. Nat. Commun. 7:10535. doi: 10.1038/ncomms10535
Börner, K., Chen, C., and Boyack, K. W. (2005). Visualizing knowledge domains. Annu. Rev. Inf. Sci. Technol. 37, 179–255. doi: 10.1002/aris.1440370106
Champigneux, P., Delia, M. L., and Bergel, A. (2018). Impact of electrode micro- and nano-scale topography on the formation and performance of microbial electrodes. Biosens. Bioelectron. 118, 231–246. doi: 10.1016/j.bios.2018.06.059
Chen, S., Patil, S. A., Brown, R. K., and Schröder, U. (2019). Strategies for optimizing the power output of microbial fuel cells: transitioning from fundamental studies to practical implementation. Appl. Energy 233–234, 15–28. doi: 10.1016/j.apenergy.2018.10.015
Chong, G. W., Karbelkar, A. A., and El-Naggar, M. Y. (2018). Nature's conductors: what can microbial multi-heme cytochromes teach us about electron transport and biological energy conversion?. Curr. Opin. Chem. Biol. 47, 7–17. doi: 10.1016/j.cbpa.2018.06.007
Cournet, A., Délia, M.-L., Bergel, A., Roques, C., and Bergé, M. (2010). Electrochemical reduction of oxygen catalyzed by a wide range of bacteria including Gram-positive. Electrochem. Commun. 12, 505–508. doi: 10.1016/j.elecom.2010.01.026
Davis, J. B. (1963). Generation of electricity by microbial action. Adv. Appl. Microbiol. 5, 51–64. doi: 10.1016/S0065-2164(08)70006-6
Davis, J. B., and Yarbrough, H. F. (1962). Preliminary experiments on a microbial fuel cell. Virology 137, 615–616.
El-Naggar, M. Y., Wanger, G., Leung, K. M., Yuzvinsky, T. D., Southam, G., Yang, J., et al. (2010). Electrical transport along bacterial nanowires from Shewanella oneidensis MR-1. Proc. Natl. Acad. Sci. U.S.A 107, 18127–18131. doi: 10.1073/pnas.1004880107
Fischer, F., Sugnaux, M., Savy, C., and Hugenin, G. (2018). Microbial fuel cell stack power to lithium battery stack: pilot concept for scale up. Appl. Energy 230, 1633–1644. doi: 10.1016/j.apenergy.2018.09.030
Fonseca, B. M., Silva, L., Trindade, I. B., Moe, E., Matias, P. M., Louro, R. O., et al. (2019). Optimizing electroactive organisms: the effect of orthologous proteins. Front. Energy Res. 7, 1–13. doi: 10.3389/fenrg.2019.00002
Glasser, N. R., Saunders, S. H., and Newman, D. K. (2017). The colorful world of extracellular electron shuttles. Annu. Rev. Microbiol. 71, 731–751. doi: 10.1146/annurev-micro-090816-093913
Gorby, Y. A., Yanina, S., McLean, J. S., Rosso, K. M., Moyles, D., Dohnalkova, A., et al. (2006). Electrically conductive bacterial nanowires produced by Shewanella oneidensis strain MR-1 and other microorganisms. Proc. Natl. Acad. Sci. U.S.A 103, 11358–11363. doi: 10.1073/pnas.0604517103
Gross, B. J., and El-Naggar, M. Y. (2015). A combined electrochemical and optical trapping platform for measuring single cell respiration rates at electrode interfaces. Rev. Sci. Instrum. 86:064301. doi: 10.1063/1.4922853
Guo, N., Wang, Y., Tong, T., and Wang, S. (2018). The fate of antibiotic resistance genes and their potential hosts during bio-electrochemical treatment of high-salinity pharmaceutical wastewater. Water Res. 133, 79–86. doi: 10.1016/j.watres.2018.01.020
Harnisch, F., and Freguia, S. (2012). A basic tutorial on cyclic voltammetry for the investigation of electroactive microbial biofilms. Chem. An Asian J. 7, 466–475. doi: 10.1002/asia.201100740
Harnisch, F., and Rabaey, K. (2012). The diversity of techniques to study electrochemically active biofilms highlights the need for standardization. ChemSusChem 5, 1027–1038. doi: 10.1002/cssc.201100817
Harnisch, F., Rosa, L. F. M., Kracke, F., Virdis, B., and Krömer, J. O. (2015). Electrifying white biotechnology: engineering and economic potential of electricity-driven bio-production. ChemSusChem 8, 758–766. doi: 10.1002/cssc.201402736
Harnisch, F., and Urban, C. (2018). Electrobiorefineries: unlocking the synergy of electrochemical and microbial conversions. Angew. Chem. Int. Ed. 57, 10016–10023. doi: 10.1002/anie.201711727
Hartshorne, R. S., Jepson, B. N., Clarke, T. A., Field, S. J., Fredrickson, J., Zachara, J., et al. (2007). Characterization of Shewanella oneidensis MtrC: a cell-surface decaheme cytochrome involved in respiratory electron transport to extracellular electron acceptors. J. Biol. Inorg. Chem. 12, 1083–1094. doi: 10.1007/s00775-007-0278-y
Hassan, M., Olvera-Vargas, H., Zhu, X., Zhang, B., and He, Y. (2019). Microbial electro-fenton: an emerging and energy-efficient platform for environmental remediation. J. Power Sources 424, 220–244. doi: 10.1016/j.jpowsour.2019.03.112
Hernandez, C. A., Beni, V., and Osma, J. F. (2019). Fully automated microsystem for unmediated electrochemical characterization, visualization and monitoring of bacteria on solid media; E. coli K-12: a case study. Biosensors 9:131. doi: 10.3390/bios9040131
Holmes, D. E., Chaudhuri, S. K., Nevin, K. P., Mehta, T., Methé, B. A., Liu, A., et al. (2006). Microarray and genetic analysis of electron transfer to electrodes in Geobacter sulfurreducens. Environ. Microbiol. 8, 1805–1815. doi: 10.1111/j.1462-2920.2006.01065.x
Holtmann, D., and Harnisch, F. (2018). “Electrification of biotechnology: Quo vadis?,” in Bioelectrosynthesis. Advances in Biochemical Engineering/Biotechnology, eds. D. Holtmann and F. Harnisch (Cham: Springer), 167, 395–411. doi: 10.1007/10_2018_75
Ikeda, T., and Kano, K. (2001). An electrochemical approach to the studies of biological redox reactions and their applications to biosensors, bioreactors, and biofuel cells. J. Biosci. Bioeng. 92, 9–18. doi: 10.1016/S1389-1723(01)80191-2
Jadhav, D. A., Ghosh Ray, S., and Ghangrekar, M. M. (2017). Third generation in bio-electrochemical system research – a systematic review on mechanisms for recovery of valuable by-products from wastewater. Renew. Sustain. Energy Rev. 76, 1022–1031. doi: 10.1016/j.rser.2017.03.096
Jiang, X., Hu, J., Fitzgerald, L. A., Biffinger, J. C., Xie, P., Ringeisen, B. R., et al. (2010). Probing electron transfer mechanisms in Shewanella oneidensis MR-1 using a nanoelectrode platform and single-cell imaging. Proc. Natl. Acad. Sci. U.S.A 107, 16806–16810. doi: 10.1073/pnas.1011699107
Jiang, X., Hu, J., Petersen, E. R., Fitzgerald, L. A., Jackan, C. S., Lieber, A. M., et al. (2013). Probing single- to multi-cell level charge transport in Geobacter sulfurreducens DL-1. Nat. Commun. 4:2751. doi: 10.1038/ncomms3751
Kadier, A., Kalil, M. S., Rai, P. K., Kumar, S. S., Abdeshahian, P., Sivagurunathan, P., et al. (2019). “Microbial electrolysis cells (MECs),” in Bioelectrochemical Interface Engineering, eds R. Navanietha Krishnaraj and R. K. Sani (Hoboken, NJ: John Wiley & Sons, Inc.), 209–234.
Koch, C., and Harnisch, F. (2016a). Is there a specific ecological niche for electroactive microorganisms?. ChemElectroChem 3, 1282–1295. doi: 10.1002/celc.201600079
Koch, C., and Harnisch, F. (2016b). What is the essence of microbial electroactivity? Front. Microbiol. 7:1890. doi: 10.3389/fmicb.2016.01890
Kracke, F., Vassilev, I., and Krömer, J. O. (2015). Microbial electron transport and energy conservation – the foundation for optimizing bioelectrochemical systems. Front. Microbiol. 6:575. doi: 10.3389/fmicb.2015.00575
Kumar, A., Hsu, L. H. H., Kavanagh, P., Barrière, F., Lens, P. N. L., Lapinsonnière, L., et al. (2017). The ins and outs of microorganism-electrode electron transfer reactions. Nat. Rev. Chem. 1:0024. doi: 10.1038/s41570-017-0024
Kumar, P., Chandrasekhar, K., Kumari, A., Sathiyamoorthi, E., and Kim, B. S. (2018). Electro-fermentation in aid of bioenergy and biopolymers. Energies 11:343. doi: 10.3390/en11020343
Kumar, R., Singh, L., and Zularisam, A. W. (2016). Exoelectrogens: recent advances in molecular drivers involved in extracellular electron transfer and strategies used to improve it for microbial fuel cell applications. Renew. Sustain. Energy Rev. 56, 1322–1336. doi: 10.1016/j.rser.2015.12.029
Liu, H., Newton, G. J., Nakamura, R., Hashimoto, K., and Nakanishi, S. (2010). Electrochemical characterization of a single electricity-producing bacterial cell of Shewanella by using optical tweezers. Angew. Chem. Int. Ed. Engl. 49, 6596–6599. doi: 10.1002/anie.201000315
Liu, X., Shi, L., and Gu, J. D. (2018). Microbial electrocatalysis: redox mediators responsible for extracellular electron transfer. Biotechnol. Adv. 36, 1815–1827. doi: 10.1016/j.biotechadv.2018.07.001
Logan, B. E. (2012). Essential data and techniques for conducting microbial fuel cell and other types of bioelectrochemical system experiments. ChemSusChem 5, 988–994. doi: 10.1002/cssc.201100604
Logan, B. E., Hamelers, B., Rozendal, R., Schröder, U., Keller, J., Freguia, S., et al. (2006). Microbial fuel cells: methodology and technology. Environ. Sci. Technol. 40, 5181–5192. doi: 10.1021/es0605016
Logan, B. E., and Regan, J. M. (2006). Microbial fuel cells - challenges and applications. Environ. Sci. Technol. 40, 5172–5180. doi: 10.1021/es0627592
Logan, B. E., Rossi, R., Ragab, A., and Saikaly, P. E. (2019). Electroactive microorganisms in bioelectrochemical systems. Nat. Rev. Microbiol. 17, 307–319. doi: 10.1038/s41579-019-0173-x
Lovley, D. R. (2011). Powering microbes with electricity: direct electron transfer from electrodes to microbes. Environ. Microbiol. Rep. 3, 27–35. doi: 10.1111/j.1758-2229.2010.00211.x
Lovley, D. R. (2012). Electromicrobiology. Annu. Rev. Microbiol. 66, 391–409. doi: 10.1146/annurev-micro-092611-150104
Lyautey, E., Cournet, A., Morin, S., Boulêtreau, S., Etcheverry, L., Charcosset, J.-Y., et al. (2011). Electroactivity of phototrophic river biofilms and constitutive cultivable bacteria. Appl. Environ. Microbiol. 77, 5394–5401. doi: 10.1128/AEM.00500-11
Malvankar, N. S., Mester, T., Tuominen, M. T., and Lovley, D. R. (2012). Supercapacitors based on c-type cytochromes using conductive nanostructured networks of living bacteria. Chemphyschem 13, 463–468. doi: 10.1002/cphc.201100865
Mclean, J. S., Wanger, G., Gorby, Y. A., Wainstein, M., Mcquaid, J., Ishii, S. I., et al. (2010). Quantification of electron transfer rates to a solid phase electron acceptor through the stages of biofilm formation from single cells to multicellular communities. Environ. Sci. Technol. 44, 2721–2727. doi: 10.1021/es903043p
Md Khudzari, J., Kurian, J., Tartakovsky, B., and Raghavan, G. S. V. (2018). Bibliometric analysis of global research trends on microbial fuel cells using Scopus database. Biochem. Eng. J. 136, 51–60. doi: 10.1016/j.bej.2018.05.002
Millo, D. (2012). Spectroelectrochemical analyses of electroactive microbial biofilms. Biochem. Soc. Trans. 40, 1284–1290. doi: 10.1042/BST20120115
Mohan, S. V., Sravan, J. S., Butti, S. K., Krishna, K. V., Modestra, J. A., Velvizhi, G., et al. (2019). “Microbial Electrochemical Technology: Emerging and Sustainable Platform,” in Microbial Electrochemical Technology, eds. S. V. Mohan, S. Varjani, and A. Pandey (Amsterdam: Elsevier), 3–18. doi: 10.1016/B978-0-444-64052-9.00001-7
Mohanakrishna, G., Venkata Mohan, S., and Sarma, P. N. (2010). Bio-electrochemical treatment of distillery wastewater in microbial fuel cell facilitating decolorization and desalination along with power generation. J. Hazard. Mater. 177, 487–494. doi: 10.1016/j.jhazmat.2009.12.059
Moß, C., Behrens, A., and Schröder, U. (2019a). The limits of three-dimensionality - systematic assessment of effective anode macro-structure dimensions for mixed culture electroactive biofilms. ChemSusChem. 13, 582–589. doi: 10.1002/cssc.201902923
Moß, C., Patil, S. A., and Schröder, U. (2019b). Scratching the surface—how decisive are microscopic surface structures on growth and performance of electrochemically active bacteria? Front. Energy Res. 7, 1–10. doi: 10.3389/fenrg.2019.00018
Mozneb, M., Smothers, C., Rodriguez, P., and Li, C. (2019). “Electrochemical analysis of single cells,” in Bioelectrochemical Interface Engineering, eds. R. Navanietha Krishnaraj and R. K. Sani (Hoboken, NJ: John Wiley& Sons, Inc.), 55–76.
Nejatimoharrami, F., Faina, A., and Stoy, K. (2017). New capabilities of evobot: a modular, open-source liquid-handling robot. SLAS Technol. Transl. Life Sci. Innov. 22, 500–506. doi: 10.1177/2472630316689285
Newman, D. K., and Kolter, R. (2000). A role for excreted quinones in extracellular electron transfer. Nature 405, 94–97. doi: 10.1038/35011098
Noyons, E. C. M. (1999). Bibliometric Mapping as a Science Policy and Research Management Tool. Available online at: http://hdl.handle.net/1887/38308 (accessed October 02, 2019).
Palanisamy, G., Jung, H.-Y., Sadhasivam, T., Kurkuri, M. D., Kim, S. C., and Roh, S.-H. (2019). A comprehensive review on microbial fuel cell technologies: processes, utilization, and advanced developments in electrodes and membranes. J. Clean. Prod. 221, 598–621. doi: 10.1016/j.jclepro.2019.02.172
Pankratova, G., and Gorton, L. (2017). Electrochemical communication between living cells and conductive surfaces. Curr. Opin. Electrochem. 5, 193–202. doi: 10.1016/j.coelec.2017.09.013
Pareek, A., and Mohan, S. V. (2019). “Progress in development of electrode materials in microbial fuel cells,” in Bioelectrochemical Interface Engineering, eds. R. Navanietha Krishnaraj and R. K. Sani (Hoboken, NJ: John Wiley& Sons, Inc.), 165–186.
Parot, S., Nercessian, O., Delia, M. L., Achouak, W., and Bergel, A. (2009). Electrochemical checking of aerobic isolates from electrochemically active biofilms formed in compost. J. Appl. Microbiol. 106, 1350–1359. doi: 10.1111/j.1365-2672.2008.04103.x
Patil, S. A., Hägerhäll, C., and Gorton, L. (2012). “Electron transfer mechanisms between microorganisms and electrodes in bioelectrochemical systems,” in Advances in Chemical Bioanalysis, ed F. M. Matysk (Cham: Springer International Publishing), 71–129.
Potter, M. C. (1911). Electrical effects accompanying the decomposition of organic compounds. Proc. R. Soc. B Biol. Sci. 84, 260–276. doi: 10.1098/rspb.1911.0073
Reguera, G., McCarthy, K. D., Mehta, T., Nicoll, J. S., Tuominen, M. T., and Lovley, D. R. (2005). Extracellular electron transfer via microbial nanowires. Nature 435, 1098–1101. doi: 10.1038/nature03661
Rosenbaum, M., Aulenta, F., Villano, M., and Angenent, L. T. (2011). Cathodes as electron donors for microbial metabolism: which extracellular electron transfer mechanisms are involved? Bioresour. Technol. 102, 324–333. doi: 10.1016/j.biortech.2010.07.008
Rosenbaum, M. A., and Franks, A. E. (2014). Microbial catalysis in bioelectrochemical technologies: status quo, challenges and perspectives. Appl. Microbiol. Biotechnol. 98, 509–518. doi: 10.1007/s00253-013-5396-6
Ryu, W., Bai, S. J., Park, J. S., Huang, Z., Moseley, J., Fabian, T., et al. (2010). Direct extraction of photosynthetic electrons from single algal cells by nanoprobing system. Nano Lett. 10, 1137–1143. doi: 10.1021/nl903141j
Sadik, O. A., Aluoch, A. O., and Zhou, A. (2009). Status of biomolecular recognition using electrochemical techniques. Biosens. Bioelectron. 24, 2749–2765. doi: 10.1016/j.bios.2008.10.003
Saito, J., Murugan, M., Deng, X., Guionet, A., Miran, W., and Okamoto, A. (2019). “Electrochemical techniques and applications to characterize single- and multicellular electric microbial functions,” in Bioelectrochemical Interface Engineering, eds. R. Navanietha Krishnaraj and R. K. Sani (Hoboken, NJ: John Wiley& Sons, Inc.), 37–53.
Schoepp-Cothenet, B., van Lis, R., Atteia, A., Baymann, F., Capowiez, L., Ducluzeau, A.-L., et al. (2013). On the universal core of bioenergetics. Biochim. Biophys. Acta Bioenerg. 1827, 79–93. doi: 10.1016/j.bbabio.2012.09.005
Schröder, U. (2007). Anodic electron transfer mechanisms in microbial fuel cells and their energy efficiency. Phys. Chem. Chem. Phys. 9, 2619–2629. doi: 10.1039/B703627M
Schröder, U., Harnisch, F., and Angenent, L. T. (2015). Microbial electrochemistry and technology: terminology and classification. Energy Environ. Sci. 8, 513–519. doi: 10.1039/C4EE03359K
Schuetz, B., Schicklberger, M., Kuermann, J., Spormann, A. M., and Gescher, J. (2009). Periplasmic electron transfer via the c-type cytochromes Mtra and Fcca of Shewanella oneidensis Mr-1. Appl. Environ. Microbiol. 75, 7789–7796. doi: 10.1128/AEM.01834-09
Segura, C. C., and Osma, J. F. (2017). Miniaturization of cyclic voltammetry electronic systems for remote biosensing. Int. J. Biosens. Bioelectron. 3, 297–299. doi: 10.15406/ijbsbe.2017.03.00068
Sharma, M., Bajracharya, S., Gildemyn, S., Patil, S. A., Alvarez-Gallego, Y., Pant, D., et al. (2014). A critical revisit of the key parameters used to describe microbial electrochemical systems. Electrochim. Acta 140, 191–208. doi: 10.1016/j.electacta.2014.02.111
Sharma, S., Hammed, A., and Simsek, H. (2019). “Microbial desalination cells,” in Bioelectrochemical Interface Engineering, eds. R. Navanietha Krishnaraj and R. K. Sani (Hoboken, NJ: John Wiley& Sons, Inc.), 235–249.
Sisler, F. D. (1962). Electrical energy from microbiological processes. J. Washington Acad. Sci. 52, 181–187.
Srikanth, S., Kumar, M., and Puri, S. K. (2018). Bio-electrochemical system (BES) as an innovative approach for sustainable waste management in petroleum industry. Bioresour. Technol. 265, 506–518. doi: 10.1016/j.biortech.2018.02.059
Sydow, A., Krieg, T., Mayer, F., Schrader, J., and Holtmann, D. (2014). Electroactive bacteria—molecular mechanisms and genetic tools. Appl. Microbiol. Biotechnol. 98, 8481–8495. doi: 10.1007/s00253-014-6005-z
Thomas, E. (2015). Microbial growth and physiology: A call for better craftsmanship. Front. Microbiol. 6:287. doi: 10.3389/fmicb.2015.00287
Trapero, J. R., Horcajada, L., Linares, J. J., and Lobato, J. (2017). Is microbial fuel cell technology ready? an economic answer towards industrial commercialization. Appl. Energy 185, 698–707. doi: 10.1016/j.apenergy.2016.10.109
Urban, P. F., and Klinqenberg, M. (1969). Redox potentials of ubiquinone and cytochrome. Eur. J. Biochem. 9, 519–525. doi: 10.1111/j.1432-1033.1969.tb00640.x
Van Eck, N. J., and Waltman, L. (2007). Bibliometric mapping of the computational intelligence field. Int. J. Uncertainty, Fuzziness Knowl. Based Syst. 15, 625–645. doi: 10.1142/S0218488507004911
Van Eck, N. J., and Waltman, L. (2010). Software survey: VOSviewer, a computer program for bibliometric mapping. Scientometrics 84, 523–538. doi: 10.1007/s11192-009-0146-3
Velvizhi, G. (2019). “Overview of bioelectrochemical treatment systems for wastewater remediation,” in Microbial Electrochemical Technology, eds. S. V. Mohan, S. Varjani, and A. Pandey (Amsterdam: Elsevier), 587–612.
Von Canstein, H., Ogawa, J., Shimizu, S., and Lloyd, J. R. (2008). Secretion of flavins by Shewanella species and their role in extracellular electron transfer. Appl. Environ. Microbiol. 74, 615–623. doi: 10.1128/AEM.01387-07
Wang, J., Zheng, T., Wang, Q., Xu, B., and Wang, L. (2015). A bibliometric review of research trends on bioelectrochemical systems. Curr. Sci. 109:2204. doi: 10.18520/cs/v109/i12/2204-2211
White, G. F., Edwards, M. J., Gomez-Perez, L., Richardson, D. J., Butt, J. N., and Clarke, T. A. (2016). “Mechanisms of Bacterial Extracellular Electron Exchange,” in Advances in Microbial Physiology, ed. R. K. Poole (London, UK: Elsevier Ltd.), 87–138. doi: 10.1016/bs.ampbs.2016.02.002
Xu, L., Zhao, Y., Doherty, L., Hu, Y., and Hao, X. (2016). The integrated processes for wastewater treatment based on the principle of microbial fuel cells: a review. Crit. Rev. Environ. Sci. Technol. 46, 60–91. doi: 10.1080/10643389.2015.1061884
Zhang, T., Ghosh, D., and Tremblay, P. (2019a). “Synthetic biology strategies to improve electron transfer rate at the microbe–anode interface in microbial fuel cells,” in Bioelectrochemical Interface Engineering, eds R. Navanietha Krishnaraj and R. K. Sani (Hoboken, NJ: John Wiley & Sons, Inc.), 187–208.
Zhang, Y., and Angelidaki, I. (2016). Microbial electrochemical systems and technologies: it is time to report the capital costs. Environ. Sci. Technol. 50, 5432–5433. doi: 10.1021/acs.est.6b01601
Zhang, Y., Liu, M., Zhou, M., Yang, H., Liang, L., and Gu, T. (2019b). Microbial fuel cell hybrid systems for wastewater treatment and bioenergy production: synergistic effects, mechanisms and challenges. Renew. Sustain. Energy Rev. 103, 13–29. doi: 10.1016/j.rser.2018.12.027
Zhi, W., Ge, Z., He, Z., and Zhang, H. (2014). Methods for understanding microbial community structures and functions in microbial fuel cells: a review. Bioresour. Technol. 171, 461–468. doi: 10.1016/j.biortech.2014.08.096
Zhuang, L., Zheng, Y., Zhou, S., Yuan, Y., Yuan, H., and Chen, Y. (2012). Scalable microbial fuel cell (MFC) stack for continuous real wastewater treatment. Bioresour. Technol. 106, 82–88. doi: 10.1016/j.biortech.2011.11.019
Microbial electrochemical systems (MES):
Combination of technologies, configurations, and materials that use microorganisms to mediate electricity driven processes to transform between chemical energy and electrical energy.
Microbial electrochemical technologies (MET):
Group of tools, techniques, and methods that individually contribute to evaluate or harness the electrochemical contribution of microorganisms at the electron-exchange interface with electronic devices in which a functional (ionic) connection is present.
Microbial fuel cell (MFC):
Device to produce an electron flux in an electric circuit from the chemical reactions mediated by microorganisms.
Bio-electrochemical systems (BES):
Combination of technologies, configurations, and materials that use a biological component to mediate electricity driven processes to transform between chemical energy and electrical energy.
Bio-electrochemical technologies (BET):
Group of tools, techniques, and methods that individually contribute to evaluate or harness the electrochemical contribution of biological components at the electron-exchange interface with electronic devices.
Microbial electro-synthesis (MESyn):
Process in which microorganisms are used to produce or mediate the reactions required to obtain chemical compounds.
Microbial electro-catalysis (MECat):
Process in which a chemical reaction rate is increased by microbial-driven processes used for the degradation of substrates.
Electron acceptor/donor (AD):
Ions or molecules that act as oxidizing/reducing agents in an electrochemical reaction.
External/extracellular electron transfer (EET):
Process by which some microorganisms can exchange electrons with solid substrates that are not soluble to the microbial cell membrane.
Keywords: microbial electrochemical system, characterization, classification, bibliographic analysis, review
Citation: Hernandez CA and Osma JF (2020) Microbial Electrochemical Systems: Deriving Future Trends From Historical Perspectives and Characterization Strategies. Front. Environ. Sci. 8:44. doi: 10.3389/fenvs.2020.00044
Received: 17 January 2020; Accepted: 31 March 2020;
Published: 12 May 2020.
Edited by:
Haluk Beyenal, Washington State University, United StatesReviewed by:
Iwona Gajda, University of the West of England, United KingdomCopyright © 2020 Hernandez and Osma. This is an open-access article distributed under the terms of the Creative Commons Attribution License (CC BY). The use, distribution or reproduction in other forums is permitted, provided the original author(s) and the copyright owner(s) are credited and that the original publication in this journal is cited, in accordance with accepted academic practice. No use, distribution or reproduction is permitted which does not comply with these terms.
*Correspondence: Johann F. Osma, amYub3NtYTQzQHVuaWFuZGVzLmVkdS5jbw==
Disclaimer: All claims expressed in this article are solely those of the authors and do not necessarily represent those of their affiliated organizations, or those of the publisher, the editors and the reviewers. Any product that may be evaluated in this article or claim that may be made by its manufacturer is not guaranteed or endorsed by the publisher.
Research integrity at Frontiers
Learn more about the work of our research integrity team to safeguard the quality of each article we publish.