- School of Natural Sciences, Bangor University, Bangor, United Kingdom
Environmental pollution associated with metal-contaminated waters discharging from abandoned mine sites is a global issue. Remediation using passive systems, such as constructed wetlands, has several significant detractions which active treatment systems that harness the abilities of hydrogen sulfide-generating bacteria to immobilize transition metals and ameliorate pH can obviate, including the potential for recovering and recycling metals. Here we describe the commissioning and testing of a laboratory-scale, continuous flow “hybrid” sulfidogenic bioreactor (HSB) where both elemental (zero-valent) sulfur (ZVS) and sulfate were provided as potential electron acceptors and glycerol as the primary electron donor for the bacterial consortium immobilized in the bioreactor vessel. The consortium included several species of acid-tolerant bacteria that catalyze the dissimilatory reduction of both ZVS and sulfate, and a novel acidophilic ZVS-reducing Firmicute, distantly related to known sulfidogens. The HSB was used to remediate synthetic and actual circum-neutral pH, zinc-contaminated water bodies from two abandoned metal mining sites in the United Kingdom. In both cases, zinc was successfully (>99%) removed from solution as a sulfide (ZnS) phase using both in-line (where mine water was pumped directly into the bioreactor) and off-line (where hydrogen sulfide was transferred from the HSB to a separate contactor vessel containing the mine waters) configurations. Both mine waters contained sufficient alkalinity to effectively neutralize the generation of acidity resulting from ZnS formation. A potential scenario for full-scale treatment of one of the mine waters using a HSB is described.
Introduction
Metal and coal mining has provided a legacy of contaminated watercourses in many post-industrial mining countries around the world. Metal contamination associated with many thousands of abandoned mine sites worldwide (e.g., ∼45,000 in North America, >5,500 in Japan, and >10,000 in the United Kingdom; Mayes et al., 2009) originates from both point and diffuse sources, such as discharges from drainage levels or adits and runoff waters from mine wastes (rock piles and tailings). This gives rise to a severe and pervasive form of pollution, generally referred to as acid mine, or rock, drainage (Blowes et al., 2014). Mayes et al. (2009, 2013) estimated that several hundred kilometers of streams and rivers and 6% of all surface waters in the United Kingdom were impacted by discharges from abandoned metal mines, accounting for hundreds of tons of soluble metals per annum flowing into receiving water bodies. Many of these have circum-neutral pH and, in contrast to extremely acidic mine waters, contain relatively little soluble iron, though concentrations of other transition metals, such as zinc, lead, and cadmium can exceed water quality guidelines. Various treatments options have been suggested and trialed for remediating mine water of this kind, including constructed wetlands and compost bioreactors which use microbiological processes, in tandem with chemical adsorption, to remove potentially toxic metals (Younger et al., 2003; Gandy et al., 2016). A primary role of microorganisms in this context is to generate hydrogen sulfide from the dissimilatory reduction of more oxidized forms of sulfur (such as sulfate), which in turn reacts with many metals that commonly occur in mine discharge waters to form insoluble sulfide phases (Johnson and Hallberg, 2005). However, these “passive” systems have many potential drawbacks (Johnson and Hallberg, 2002). Protracted hydraulic residence times and large land surface areas, which may not always be available, are often required for these systems to be effective, and abandoned mines located in upland areas with steep topographies often make passive systems a non-feasible option. In the short term, rapid mineralization of the more labile organic components in compost-based systems can generate drainage waters with very elevated concentrations of dissolved organic carbon (DOC), which may even more of a pollution threat than the untreated mine water (e.g., Jarvis et al., 2015). Longer term problems include variable performances of passive systems (which are affected by seasonal parameters, aging of composts, etc.) and the possibility of re-mobilization of captured metals caused, for example, by ingress of oxygen. Residual solid materials used to construct passive systems end up as inorganic and organic waste material-containing spent composts that ultimately have to be removed and disposed of in suitable landfill sites. Moreover, the metals themselves, which might have some commercial value, form part of the waste product and are not recovered or recycled.
An alternative approach is to utilize an “active” microbiological approach in which hydrogen sulfide generated in bioreactors is used to immobilize transition metals, usually in off-line contact vessels. This approach has the advantage of being far more controllable and predictable than passive remediation systems, though the often much greater capital and operating costs of using bioreactors are significant detractions. Full-scale systems, developed by the Dutch company Paques and the Canadian company BioteQ, operate in various global locations (Cline et al., 2003; Bratty et al., 2006). Various electron donors metabolized by sulfidogenic bacteria, such as hydrogen and ethanol, have been used in full-scale bioreactors, while the electron acceptor used is either sulfate or elemental (zero-valent) sulfur (ZVS), and any excess H2S generated can be readily converted back to ZVS in off-line vessels. In thermodynamic terms, ZVS is a superior electron acceptor due to having no requirement for ATP-consuming activation as a first step in its reduction (Rabus et al., 2006) and because the redox potential of the S0/H2S couple (−270 mV) is more positive than that of the SO42–/H2S couple (−303 mV). In theory, smaller amounts of electron donor are required to generate H2S from ZVS (a two-electron reduction) than from sulfate (an eight-electron reduction). However, biosulfidogenic systems that use ZVS (such as the BioSulphide® process) do not lower the sulfate concentrations in the water being processed, which may be an important objective of the remediation process. In addition, the cost of ZVS can negatively impact the economics of the process, whereas sulfate is usually already present in elevated concentrations in waters draining metal and coal mines.
Current commercial systems comprise bioreactors that house the hydrogen sulfide-generating bacteria, and separate agitated contactor tanks that contain the metal-rich wastewaters being treated. One reason for this is to shield the bacteria from the potentially toxic effects of the mine waters, which may be acidic and contain inhibitory concentrations of one or more soluble metal or metalloid (such as arsenic). This adds complexity and cost to the system. To circumvent this problem, novel sulfidogenic bioreactors have been developed that use acidophilic and acid-tolerant consortia of sulfate-reducing bacteria, which are tolerant of moderate and extreme acidity and elevated concentrations of metals (Ňancucheo and Johnson, 2012; Santos and Johnson, 2018). These have the additional advantage of (partially) neutralizing the pH of acidic mine waters, since dissimilatory sulfate reduction is a proton-consuming reaction at low pH, due to the end products being primarily H2S and CO2 rather than HS– and HCO3– (Johnson and Sánchez-Andrea, 2019). However, these bioreactors are not suitable for remediating circum-neutral pH mine waters, as they require they influent liquid to have a lower pH than the effluent generated. In the current study, a variant of the low pH sulfidogenic bioreactor was developed in which both sulfate and ZVS were the potential electron acceptors, and glycerol was provided as the electron donor. In contrast to sulfate reduction, the dissimilatory reduction of ZVS is pH neutral, or acid-generating at higher pH values (Johnson and Sánchez-Andrea, 2019) facilitating its use for remediating neutral pH and alkaline mine waters. The novel system housed a microbial consortium that included novel species of acidophilic sulfidogens that were able to utilize both forms of sulfur (sulfate and ZVS), and is referred to as a “hybrid” sulfidogenic bioreactor (HSB). Experiments carried out with the HSB demonstrated that it could be effective at removing soluble zinc from two circum-neutral pH metal-contaminated mine waters in the United Kingdom.
Materials and Methods
Mine Waters
Metal-contaminated waters from two abandoned mining areas, one in central England and the other in northern England, were used in laboratory experiments. One site was Snailbeach Farm (52° 37′ 4.7172″ N; 2° 55′ 33.726″ W) in the Minsterley Brook catchment area, which receives water draining mine wastes from several abandoned small, primarily lead-zinc-barite mines that were worked up until the 1940s. Approximately 60 L of mine-impacted water (MIW) was collected from this site in October 2016. The second site, Force Crag, was located in the north-west of England (54° 35′ 0.6036″ N, 3° 14′ 23.1972″ W); this was also an abandoned lead-zinc-barite mine and operated intermittently for 157 years until its closure in 1992. Sixty liters of MIW was collected from this site in April 2017.
Prior to using actual MIW in laboratory experiments, synthetic versions of each were prepared, based on data provided by the Coal Authority (United Kingdom). The chemical compositions of these and of the MIWs that were collected on site are shown in Table 1. The synthetic mine waters were amended with glycerol (electron donor) and yeast extract (to provide growth factors for the bacteria) and heat-sterilized (120°C; 30 min), while the MIWs were not sterilized and the organic feeds were provided separately, as described below.
Bioreactor Set Up
An upflow biofilm bed sulfidogenic bioreactor (Ňancucheo and Johnson, 2012) was set up, using a FerMac 310/60 unit (Electrolab., United Kingdom) to monitor temperature (maintained at 30°C during the entire test period), pH, and agitation (50 rpm) of the 2.2 L (working volume) reactor vessel. The HSB was populated with microorganisms immobilized on 1–2 mm diameter porous glass beads (Poraver Dennert GmbH, Germany), obtained from an operating sulfidogenic bioreactor and included species of both acidophilic sulfate reducing bacteria (aSRB) and non-sulfidogenic acidophilic bacteria (Santos and Johnson, 2018). Approximately 300 g of wet beads was mixed with 100 g (dry weight equivalent) of sterile hydrophilic ZVS and placed into the bioreactor vessel, and an additional 100 g (dry weight equivalent) of ZVS placed on top. The ZVS had been modified from being hydrophobic to hydrophilic by short-term (7 days) contact with the sulfur-oxidizing acidophilic bacterium Acidithiobacillus thiooxidans in a liquid culture, after which it was removed and sterilized (110°C; 60 min). The bioreactor was also inoculated with a novel sulfidogenic bacterium that reduces ZVS but not sulfate (isolate I2511; Holanda and Johnson, unpublished). The liquid volume in the bioreactor vessel was topped up to ∼2.1 L with a liquid medium comprising basal salts, trace elements, 4 mM glycerol, and 0.01% (w/v) yeast extract, pH adjusted to 4.0 (Ňancucheo et al., 2016). The bioreactor was gassed with a continuous stream of oxygen-free nitrogen (at ∼200 mL min–1) in order to maintain a slight positive pressure within the reactor vessel and to remove and deliver the hydrogen sulfide (H2S) produced to an off-line vessel containing 50 mM copper sulfate. Once the production of H2S by the HSB had been confirmed (indicated by the production and deposition of CuS in the off-line vessel), the bioreactor was operated in continuous flow mode (455 mL h–1) using a modified version of the liquid medium added initially, which contained 0.8–1.0 mM ZnSO4 and 1–2 mM glycerol (pH adjusted to 6.5).
Bioreactor Operation
Following confirmation that the newly commissioned HSB was actively generating H2S and that near steady-state conditions had been established (approximately 50 days), the bacterial growth medium was replaced, first with synthetic and later actual MIW (Minsterley Brook, followed by Force Crag water). Two modus operandi for removing transition metals (primarily zinc) from water samples were compared simultaneously: (i) in-line, where the mine waters were pumped directly into the bioreactor vessel and metals removed (as sulfide phases) in situ; (ii) off-line, where the HSB was constrained to generate H2S in above that required to precipitate metals in-line, with the excess being transferred to a contactor vessel containing synthetic or actual mine water. When sterile synthetic mine waters were used, organic materials were added directly to the feed liquor reservoirs [1 mM glycerol and 0.005% (w/v) yeast extract for synthetic Minsterley Brook mine water, and 50 μM glycerol and 0.0025% (w/v) yeast extract for synthetic Force Crag mine water]. However, this was not possible when actual MIWs were used, as the indigenous microorganisms would have metabolized these labile organic materials within the reservoirs themselves. Therefore, a separate vessel was prepared containing more concentrated feed liquors (207 mM glycerol and 0.5% w/v yeast extract with Minsterley Brook MIW, and 20–50 mM glycerol and 0.25% w/v yeast extract for Force Crag MIW). Minsterley Brook synthetic and actual MIW were pumped into the bioreactor vessel at 455 mL h–1 (equivalent to a dilution rate of 0.22 h–1) and the organic feed liquor at 3.6–4.0 mL h–1. In the case of Force Crag, the initial mine water feed rate was 455 mL h–1, but this was later increased to up to 1,520 mL h–1 (dilution rate equivalents of 0.22–0.72 h–1) and the organic feed was supplied initially at 6 mL h–1, which was progressively increased to 11 mL h–1. The off-line mine water reservoir contained with 1 L (Minsterley Brook) or 500 mL (Force Crag) of mine water, and the gas stream from this was delivered to a third vessel containing copper sulfate solution, in order to remove residual H2S. Additional peristaltic pumps (Ecoline VC-M/CA8-6, ISMATEC) were used where necessary, and the liquid volume level in the bioreactor vessel was maintained at a constant level throughout all experiments. A schematic showing the operational set up of the HSB is shown in Figure 1.
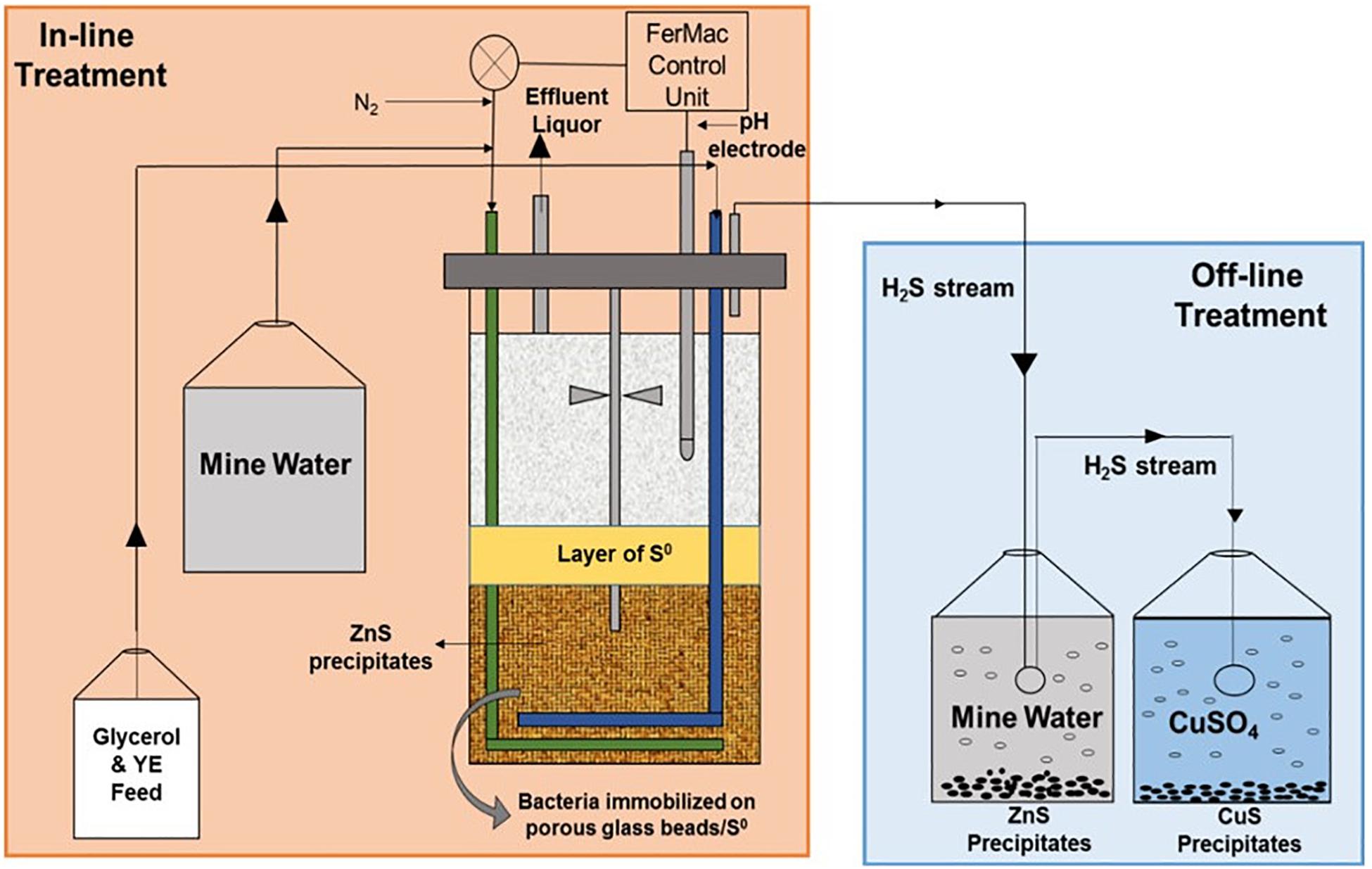
Figure 1. Schematic representation of the set up used to assess removal of soluble zinc from circum-neutral pH, metal-contaminated mine waters.
Physico-Chemical Techniques and Calculations
The pH of the liquid phase above the ZVS layer in the HSB was measured continuously with a glass electrode (Figure 1) and that of the effluent liquor was measured regularly using a pHase combination glass electrode coupled to an Accumet 50 pH meter. Concentrations of zinc, glycerol, sulfate, and acetic acid were determined by ion chromatography (Ňancucheo and Johnson, 2012). Concentrations of soluble copper were measured using a colorimetric-based assay (Anwar et al., 2000). Since the stoichiometry H2S generated to both ZnS and CuS precipitated is 1:1, rates of H2S production were calculated by combining the rates of ZnS formation inside the bioreactor and those of ZnS and CuS formation in the external vessels (determined from changes in soluble zinc and copper concentrations), and assumed that there was no leakage of H2S gas from the system. The relative percentage of sulfate and ZVS used as electron acceptors were calculated from differences in sulfate concentrations in liquors entering and draining the bioreactor, and comparing these with the total amounts of H2S generated. Sulfate removal was presumed to be predominantly due to dissimilatory reduction to H2S, with only very small amounts being assimilated by the indigenous microorganisms. The relative amounts of ZVS used for dissimilatory reduction were calculated from differences in H2S generation and sulfate removal. DOC concentrations were measured using a Protoc DOC analyzer (Pollution and Process Monitoring Ltd., United Kingdom). To determine alkalinity, 100 mL of MIW was titrated with 0.1 M HCl until the pH decreased from ∼7.0 to 4.0. Alkalinity was calculated in mg of CaCO3 L–1 using the equation (A × M × 50,000)/V, where A is the volume (mL) of HCl used, M is the molarity of HCl, and V is the volume (mL) of the MIW (Thomas and Lynch, 1960).
Biomolecular Analysis
Various water and slurry samples were analyzed for their microbial populations. These were: (i) actual MIW samples (50 mL of each, filtered on site); (ii) 15 mL of liquor from the surface of the bioreactor; and (iii) a slurry sample taken from the ZVS layer in the bioreactor. Samples were filtered through sterile 0.2 μm (pore size) cellulose nitrate membrane filters (Whatman, United Kingdom), flowing agitation to dislodge cells in the case of the ZVS slurry. DNA from biomass collected on the filter membranes was extracted using PowerSoil UltraClean microbial DNA isolation Kits (QIAGEN, Denmark), following manufacturer’s instructions. Prokaryotic 16S rRNA genes were amplified and analyzed by terminal restriction enzyme fragment length polymorphism (T-RFLP) (Kay et al., 2013).
Results
The newly commissioned HSB began to generate H2S very soon after it had been set up, producing ∼300 μmoles hydrogen sulfide L–1 h–1, and >99% of the zinc in the influent (growth medium) liquor was being removed continuously within the bioreactor vessel from 13 days after commissioning. The initial phase continued for a further 37 days before the HSB was challenged with synthetic and actual mine waters. Data files from each of the experiments are included in Supplementary Tables S1–S5.
Minsterley Brook Mine Waters
While there were differences in some of the metabolites (e.g., chloride and net alkalinity) in synthetic and actual MIW from Minsterley Brook, the zinc concentrations and pH values were similar in both (Table 1). As shown in Figure 2, zinc was effectively (>96%) removed from synthetic Minsterley Brook mine water throughout the 102 h test period, using the in-line configuration, and that figure increased to >99% when actual MIW was used (during a 123 h test period; the lower figure at 95 h was due to a short-term disruption of the organic feed). The pH within the bioreactor vessel was, however, much lower (3.27–3.55) with synthetic than with actual MIW (6.72–6.95) from Minsterley Brook. Similar differences in pH were found with off-line treatment of synthetic and actual MIW from this site (Figure 3). This paralleled the relative efficiencies of zinc removal from these waters, which was far more rapid and more effective in the case of the actual MIW. Whereas only ∼20% of the zinc in the synthetic mine water had been precipitated after 4 h off-line, no soluble zinc was detected in the actual MIW after 2 h contact (Figure 3). This was not due to an inadequate supply of H2S, as copper was precipitated in the second off-line vessel that received off-gas that had already passed through the synthetic mine water. With more protracted (up to 100 h) contact with the off-gas from the HSB, 75% of soluble zinc was removed from the synthetic mine water, and the solution pH fell to 2.9 (data not shown).
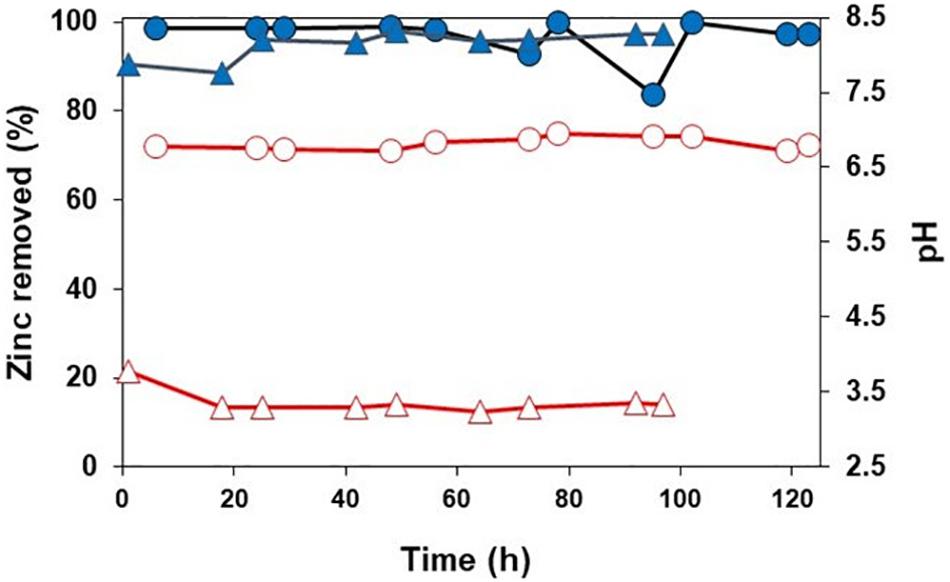
Figure 2. Precipitation of zinc (closed symbols) and changes in bioreactor pH (open symbols) during in-line treatment of synthetic (,
) and actual MIW (
,
) from Minsterley Brook.
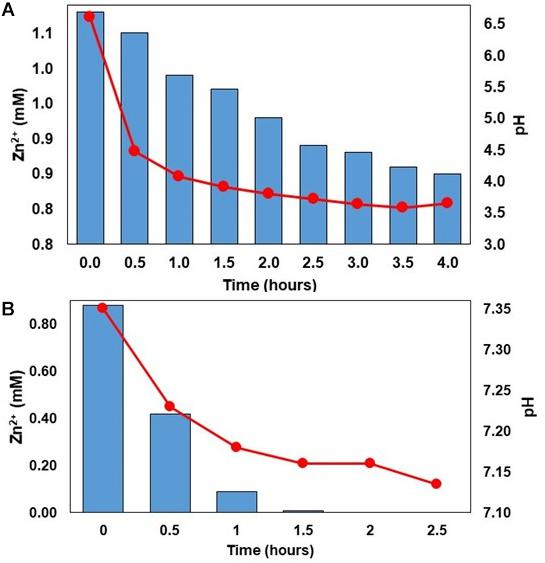
Figure 3. Changes in concentrations of soluble zinc (bars) and pH (lines) in off-line vessels containing synthetic mine water (A) and actual MIW (B) from Minsterley Brook, contacted with H2S generated by the HSB.
There were some variations in the rates of H2S generated by the HSB when synthetic Minsterley Brook mine water was used as the feed liquor, but this was less apparent in the case of actual MIW (Figure 4, top). On each sampling occasion, the rate of H2S generation exceeded that of sulfate reduced, confirming that both sulfate and ZVS were being used simultaneously as electron acceptors in the HSB. Calculations of the relatively proportions of these being reduced to H2S when the HSB was fed with actual MIW (Figure 4, bottom) indicated that 41 ± 17% of the H2S produced derived from the dissimilatory reduction of sulfate, with the remainder coming from the dissimilatory reduction of ZVS, over the time course of this experiment. The corresponding figure for the synthetic mine water was 48 ± 26% (data not shown).
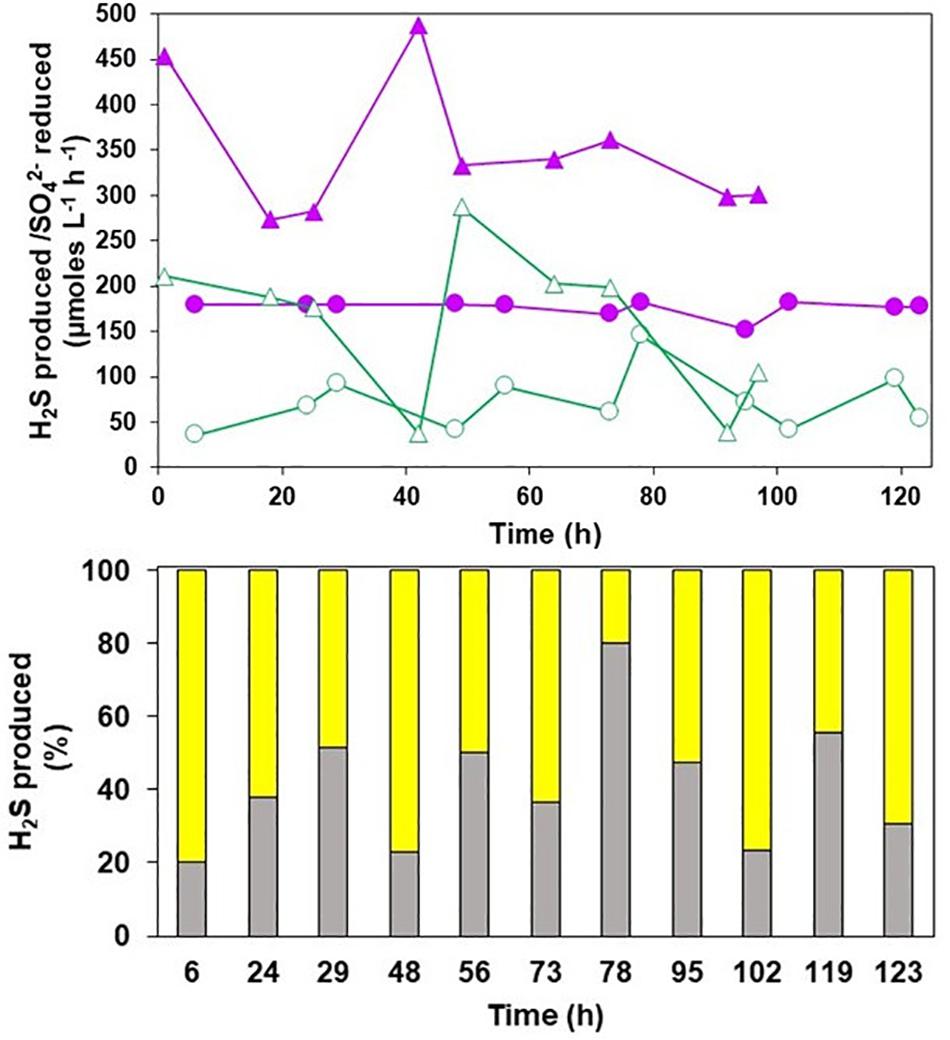
Figure 4. (Top) Rates of hydrogen sulfide production (closed symbols) and sulfate reduction (open symbols) during in-line treatment of synthetic mine water (,
) and actual MIW (
,
) from Minsterley Brook. (Bottom) Relative amounts of hydrogen sulfide produced via the dissimilatory reduction of sulfate (
) and ZVS (
) during treatment of actual MIW from Minsterley Brook.
Acetate was detected in effluent solutions draining the HSB throughout the experiments using both synthetic mine water and actual MIW from Minsterley Brook (Figure 5). However, the stoichiometry of glycerol oxidized to acetate produced was always > 1:1, confirming that some of the glycerol had been completely oxidized (to CO2) while the rest had been incompletely oxidized to acetate and CO2. Similar results have been observed using low pH sulfate-reducing bioreactors (Santos and Johnson, 2018).
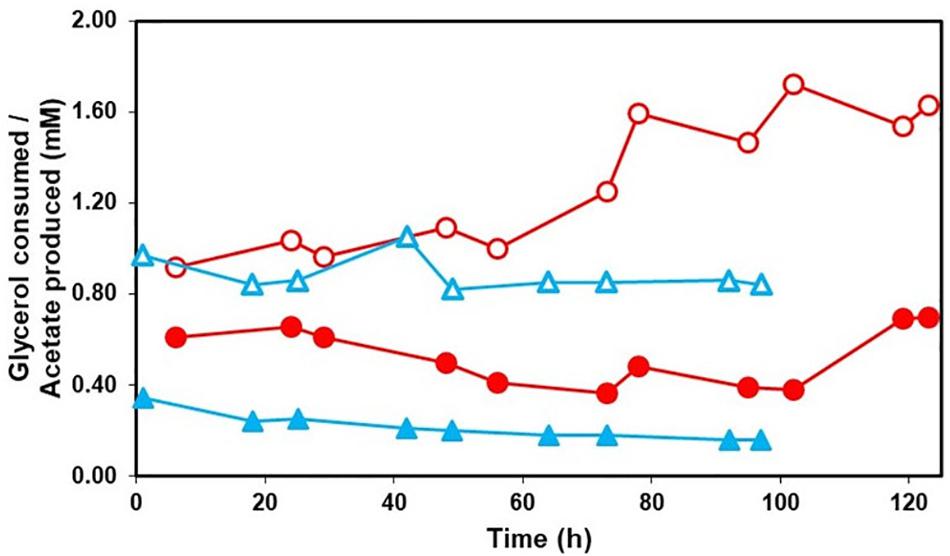
Figure 5. Consumption of glycerol (open symbols) and production of acetate (closed symbols) by the HSB when either synthetic mine water (,
) or actual MIW (
,
) was used as the feed liquor.
Force Crag Mine Waters
The most notable difference between the chemical composition of the synthetic Force Crag mine water (based on previous analyses) and the MIW collected on site was that the former contained 4 mg L–1 of copper, while this metal was below detection limits in the MIW collected on site (Table 1). The alkalinity of the synthetic water was again less than the MIW sampled, though this difference was far less than that of the Minsterley Brook mine waters.
More than 99% of soluble copper and up to 90% of soluble zinc was removed by in-line treatment of synthetic Force Crag mine water (Supplementary Figure S1). Removal of soluble zinc was more rapid and effective in the case of actual MIW from Force Crag, with >99% precipitated during the first 38 h of testing, at a flow rate of 455 mL h–1 and a dilution rate of 0.21 h–1. Increased flow rates (up to 1,520 mL h–1, equivalent to a dilution rate of 0.69 h–1) were then imposed to ascertain whether the performance of the HSB could be further improved. Data, shown in Figure 6, indicated that increasing dilution rates resulted initially in less effective removal of soluble zinc, but this increased again when the rate was lowered slightly (to 0.54 h–1). When the dilution rate was subsequently increased once more to 0.69 h–1, removal of soluble zinc was maintained at >99%. The pH of the bioreactor with synthetic Force Crag mine water as feed liquor was consistently 3.6, while with the actual MIW it varied between 5.8 and 6.6.
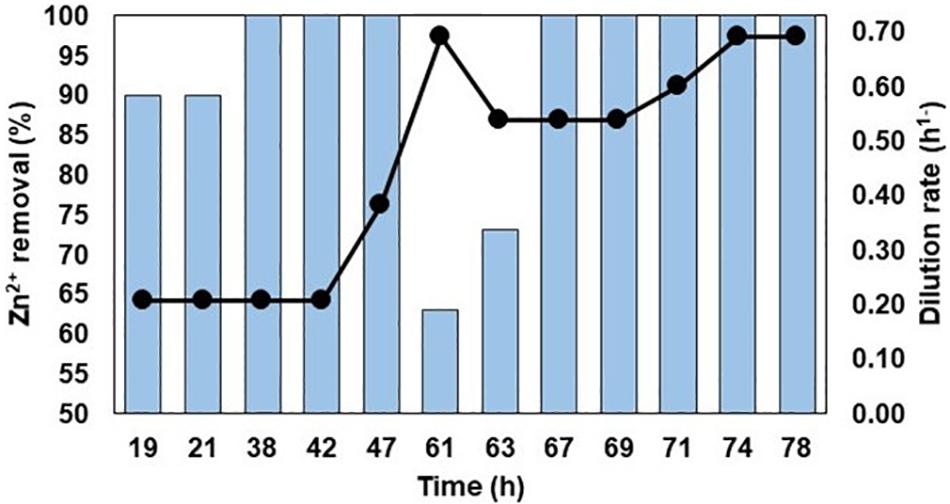
Figure 6. In-line treatment of MIW from Force Crag. The bars represent percentage removal of Zn2+, and the line graph depicts changes in dilution rates.
Both transition metals present in significant concentrations in synthetic Force Crag mine water were removed (>99%) from solution in the off-line vessel contacted with H2S generated by the HSB, copper much more rapidly than zinc (Figure 7). Copper was not detected in actual MIW collected from Force Crag, and zinc was more rapidly removed from solution than was the case with the synthetic mine water. As was the case with Minsterley Brook, the final pH of the off-line water was much more acidic (pH 3.7) with the synthetic than with the actual (pH 6.8) mine water (Figure 7).
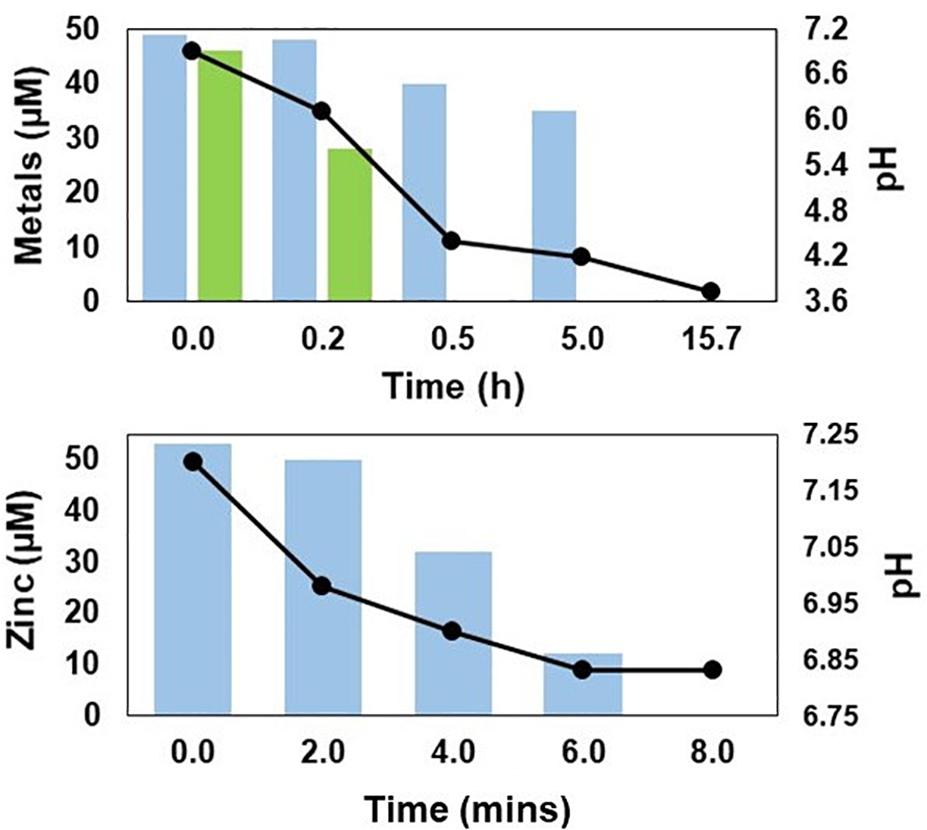
Figure 7. Changes in concentrations of soluble zinc () and copper (
) (bar graphs), and pH (line graphs) in off-line vessels containing synthetic mine water (top) and actual MIW (bottom) from Force Crag, contacted with H2S generated by the HSB.
Hydrogen sulfide was generated by the HSB when either synthetic or actual Force Crag mine water was used as the influent liquor (Supplementary Figure S2). However, no decrease in sulfate concentrations was detected when synthetic or actual mine Force Crag mine waters were pumped through the HSB, the implication being that the H2S was generated solely via the dissimilatory reduction of ZVS. Whereas in the case of synthetic Force Crag mine water H2S generation was noted to decline after ∼ 50 h of continuous flow, the opposite was observed (after about 65 h) with the actual MIW (Supplementary Figure S2).
Over 99% of glycerol added to synthetic Force Crag mine water was consumed within the bioreactor and acetate was produced in near 1:1 stoichiometric amounts relative to the glycerol consumed (Supplementary Figure S3). With actual Force Crag MIW, more acetate was detected in the effluent liquor than could be accounted for by incomplete oxidation of glycerol between 18 and 47 h, though this was reversed during the latter stages of this experiment.
Microbial Populations in Mine Waters and the HSB
Biomolecular analysis of bacterial 16S rRNA genes on mine water collected at both abandoned mines sites showed that the bacterial population in that from Minsterley Brook was highly diverse [indicated by the large number of terminal restriction fragments (T-RFs) detected] with no T-RF exceeding > 10% relative abundance (Supplementary Figure S4), while the T-RFLP profile Force Crag mine water had fewer peaks, with one T-RF (220 nt length) accounting for 33% of the summated T-RFs.
T-RFLP analysis of microbial populations within the HSB detected bacterial species that had been reported previously for low pH sulfidogenic bioreactors (Desulfosporosinus acididurans; Sánchez-Andrea et al., 2015) and Peptococcaceae CEB3 (both SRB), Acidocella aromatica, actinobacterium sp. IR1, and Acidithiobacillus ferrooxidans (Ňancucheo and Johnson, 2012; Santos and Johnson, 2018). During treatment of Minsterley Brook synthetic mine water, D. acididurans and strain CEB3 accounted for >50% of the bacterial populations (from summated T-RFs) with both sulfur-attached and planktonic cells, and D. acididuransT (35 and 56%, respectively) was the more abundant sulfidogen (Supplementary Figure S5). When the influent liquor was changed to actual MIW from this site, both these strains became less abundant and novel unidentified T-RFs appeared in T-RFLP profiles. Other bacteria identified were a Clostridium sp. (that shared 98% identity of 16S rRNA gene with Clostridium drakei) (which increased greatly in relative abundance in both attached and planktonic populations when actual MIW was used as the feed liquor), Ac. aromatica, actinobacterium sp. IR1 and At. ferrooxidans, though the latter was detected only in sulfur-attached populations (Supplementary Figure S5).
Corresponding bacterial populations when synthetic mine water and actual MIW from Force Crag are shown in Supplementary Figure S6. Changes in the composition of cells attached to ZVS as a result of changing from synthetic to actual mine water were less pronounced than those of planktonic populations; only D. acididurans was detected, and at low relative abundance (4%) as a known SRB. However, the ZVS-reducing Firmicute strain I2511 was detected in both sulfur-attached and planktonic bacterial populations during synthetic mine water treatment (at 8 and 13% relative abundance, respectively) and in the ZVS bacterial community (13% relative abundance) at the end of the trial using actual MIW from Force Crag. The facultative anaerobes At. ferrooxidans and Ac. aromatica were both far more abundant following the trial with actual MIW, and other changes in the relative abundances of unknown bacteria were also found during testing with Force Crag mine water (Supplementary Figure S6). All attempts to amplify archaeal 16S rRNA genes from bioreactor samples proved negative (in contrast to positive controls using archaeal DNA).
Discussion
Precipitating transition metals as sulfides is particularly appropriate when concentrations of the former are relatively small, as in the mine waters used in this study, as the low solubility products of many metal sulfides (Monhemius, 1977) means that concentrations can be lowered to very low levels. Another benefit is that the precipitates generated are more dense and readily collected than, for example, hydroxide phases. Sulfidogenic bioreactors, using either sulfate or ZVS as the electron acceptor and inorganic (hydrogen) or organic electron donors, have been demonstrated for this purpose in both laboratory and full-scale systems. The low pH sulfate-reducing bioreactors, on which the HSB was based, have previously been shown to operate over a wide pH range (2.5–6) (Ňancucheo and Johnson, 2012; Santos and Johnson, 2018) and contain a consortium of acidophilic and acid-tolerant bacteria including species that can reduce both sulfate and ZVS, generating hydrogen sulfide (Sánchez-Andrea et al., 2015). The modification of this system to the HSB described in the current publication involved both adding hydrophilic ZVS to the reactor and inoculating with a novel Firmicute (strain I2511) that catalyses the dissimilatory reduction of ZVS, but not sulfate, but awaits full characterization. The difference in proton consumption (at pH < 7) associated with sulfate and ZVS reduction facilitated the removal of metals from circum-neutral pH mine waters by the HSB, while the advantages previously demonstrated with the low pH sulfate-reducing bioreactors, including being able to operate under acidic conditions, e.g., as a consequence of mineralization of ZnS, and in being tolerant of transition metals such as zinc (Ňancucheo and Johnson, 2012) were retained. This robustness of the HSB can be a major attribute in pilot- and full-scale systems operated in the field, where perturbations in mine water chemistries could be anticipated.
Removal of zinc was both more rapid and effective when using actual mine waters (from both sites) rather than the synthetic versions prepared using the available chemical analyses of discharges at the abandoned mine sites. The reason for this was mainly the difference in alkalinities of the waters used, which were lower in the synthetic versions of the mine waters, especially in the case of Minsterley Brook. Metal sulfide precipitation is a proton (hydronium ion)-generating reaction (e.g., Zn2+ + H2S → ZnS + 2 H+); treating both synthetic and actual MIW from Minsterley Brook generated ∼2 mM of protons as a consequence of fully precipitating the ∼1 mM Zn2+ as sulfide. The alkalinity of the actual waste water was 148 mg L–1 (CaCO3 equivalent) which corresponds to 3 mM HCO3–. Since each bicarbonate anion can neutralize one proton (HCO3– + H+ → CO2 + H2O), the alkalinity of the waste water was greater than that of the acidity generated by complete mineralization of zinc as ZnS, so there was little change in water pH as a consequence of zinc removal. In contrast, the synthetic version of the waste water contained only 12.5 mg L–1 alkalinity (0.25 mM HCO3–) which is far less than that of the mineral (Zn2+) acidity of the synthetic waste water, and consequently the pH of this water fell significantly as zinc was removed. At pH ∼3.5, zinc does not readily form a sulfide phase due to its solubility product (log Ksp = −24.5 at 25°C; Monhemius, 1977) dictating that the concentration of the reacting species (S2–) required to precipitate ZnS is far greater than that possible under the experimental conditions used (the pKa values of H2S/HS– and HS–/S2– are 6.9 and ∼13, respectively; Johnson and Sánchez-Andrea, 2019). This explains why, for example, only ∼75% of the soluble zinc had been removed offline from synthetic Minsterley Brook mine water after 4 h treatment, while ∼99% had been removed from actual MIW within 1.5 h.
In the case of Force Crag, previous chemical analysis had detected significant (4 mg L–1) soluble copper in the mine water, but copper in the sample collected on site was below detectable limits, though zinc concentrations were 3 mg L–1 in both synthetic and actual mine waters. Copper sulfide has a lower solubility product (log Ksp = −35.9 at 25°C) than zinc sulfide, which is why copper was removed more rapidly and effectively from synthetic Force Crag mine water, as shown with other mine waters (Johnson and Sánchez-Andrea, 2019). The combination of greater alkalinity and lower transition metal (zinc + copper) concentrations in the actual compared to synthetic Force Crag mine water meant (as with Minsterley Brook) that there was little pH change consequential on complete removal of the metals (as sulfides) in the former, while pH fell significantly with the latter.
Mass balance calculations suggested that both sulfate and ZVS were being used as terminal electron acceptors for hydrogen sulfide production when Minsterley Brook mine waters were tested, but there was no evidence for net sulfate reduction in the case of Force Crag mine waters. These calculations were based on differences in sulfate concentrations in influent and effluent liquors. In the case of Force Crag mine waters, this may have been due to the much lower concentrations of sulfate in both synthetic and actual MIW, and also due to the higher flow rates used, resulting in possible re-oxidation of the hydrogen sulfide (to sulfate) generated due to greater ingress of dissolved oxygen present in the feed liquors, which were not deoxygenated. Most of the primary electron donor (glycerol) provided was used was consumed within the HSB in all experiments. Acetate was detected in effluent liquors, confirming that some, at least, of the glycerol had been oxidized incompletely, rather fully to carbon dioxide. While this did not impact zinc removal, incomplete oxidation is not desirable as it lowers the efficiency of the bioreactor and the labile acetate released from the bioreactor contributes to the biological oxygen demand of discharged liquors.
Biomolecular analysis confirmed that that mixed bacterial communities were present both in the liquid phase and attached to the solid ZVS within the HSB. In the case of Minsterley Brook, changing from synthetic to actual MIW resulted in lower relative abundance of known sulfate-reducers (both free-swimming and attached) and a higher relative abundance of a Clostridium sp. (a non-sulfidogenic anaerobe). With Force Crag mine waters, known sulfate-reducers were less relatively abundant, but the ZVS-reducing Firmicute strain I2511 was detected in significant relative abundance in three of the four samples analyzed, suggesting that this bacterium was an important sulfidogen in those tests.
A full-scale passive system, a downward-flow compost bioreactor comprising two vertical flow-through ponds (each with a HRT of 15–20 h) operated in parallel, and used to precipitate zinc and other metals within the compost substrate (woodchips and dried activated sewage sludge) as sulfides and other phases, was installed at the Force Crag site in 2014. Jarvis et al. (2015) reported that during the first days of operation the passive system was a source of secondary contamination in the receiving River Coledale Beck, generating effluents with elevated biochemical oxygen demand (up to 100 mg L–1) and chemical oxygen demand (up to 3,084 mg L–1), and elevated concentrations of ammonium (82 mg L–1) and phosphate (28 mg L–1). However, concentrations of soluble organic and inorganic contaminants subsequently decreased and, within one year, values were in below discharge consent levels (Coal Authority U.K., personal communication). The passive system removed ∼ 97% of zinc in the mine water and sulfate concentrations decreased during treatment, but zinc sulfide was not identified in the solid phase of the compost. Results from the Force Crag mine experiments provided a good illustration of the major differences between using sulfidogenic bioreactors and passive systems to treat contaminated mine waters. The HSB was superior in terms of zinc removal (>99%), lower HRTs (1.5–5 h), no generation of secondary contaminants (apart from small concentrations (0.09–0.53 mM) of acetate) and in generating zinc sulfide precipitates that would be a potentially secondary resource, though was inferior in terms of sulfate removal (which is not an issue with Force Crag, as concentrations are very low).
Alternative Engineering Designs
Comparing the two possible configurations (on-line and off-line treatment), while both approaches were effective at removing zinc from MIWs from both sites, generating processed waters with only minor changes in pH, adopting a 100% in-line treatment would consume a great deal of energy in order to maintain a pilot- or full-scale HSB at a temperature conducive for the sulfidogenic bacteria. In addition, the metal sulfide precipitates formed within the bioreactor are intimately mixed with ZVS, biomass, and other solid materials in the in-line system, complicating their extraction and metal recovery. The system proposed for Force Crag therefore involves taking a small proportion (1–5%) of the mine water drainage and using it as a direct feed for an on-site HSB, which would generate hydrogen sulfide for removing zinc in the remaining bulk of the water discharged in off-line contactor vessels. Extrapolating data from the laboratory system used in the present study (with rates of hydrogen sulfide production of ∼300 μmoles L–1 h–1), a 1 m3 HSB could produce sufficient hydrogen sulfide each hour to treat 6.5 m3 of mine water at Force Crag. Rates of mine water discharge average about 15 L s–1 (∼54 m3 h–1) at Force Crag (Jarvis et al., 2015). This suggests that a 10 m3 bioreactor would be required for full-scale mine water treatment at this site, though optimizing rates of hydrogen sulfide production could significantly increase the efficiency of the direct biological treatment protocol that is proposed. Based on the current mine water chemistry, over 2 t ZnS would be produced per annum, as a potentially saleable product that could partly offset operating costs of the system.
Economic Considerations
The operating costs (OPEX) of using a scaled-up HSB to remediate waters at the two sites can be estimated in terms of the three consumables required, using the costs of these commodities quoted in various on-lines sites in 2018 (0.48 for glycerol, 3.00 for yeast extract, and 0.83 for ZVS; all as $(US) kg–1). Snailbeach Farm waste water contains ∼1 mM (65 mg L–1) zinc (65 g m–3) and each cubic meter would require 1 mole of H2S to completely remove this metal. If this was generated exclusively from complete glycerol oxidation being coupled to the reduction of ZVS, this would require 14.3 moles (1.32 kg) of glycerol for each 100 m3 of water treated (the stoichiometry being 1 glycerol:7 ZVS) which would cost ∼ $0.63. One hundred moles (3.2 kg) of ZVS would be consumed [at a cost of ∼$2.66/100 m3], making a combined cost of $3.29. If yeast extract was added at a similar rate as in the current experiments (equivalent to about 300 g/100 m3), this would add a further $0.90 to the consumables cost, which then totals $4.19/100 m3 waste water. If, on the other hand, all of the H2S was generated from reduction of sulfate present (in excess of that required) in the waste water, then the glycerol costs increase [to $2.54/100 m3 water, since the stoichiometry of glycerol:sulfate is 4:7] but there is no cost for ZVS consumed. Assuming a similar amount of yeast extract was used as in the previous scenario, the cost of treating 100 m3 of water is now slightly lower [$3.44]. In the experiments carried out, the roughly similar amounts of H2S were generated from reduction of sulfate and ZVS, and an average OPEX (consumable) cost of $3.81/100 m3 water would therefore be more realistic. This figure assumes, however, that 100% of the principle electron donor (glycerol) is used to generate H2S. Assuming a more realistic figure of 50% efficiency, the estimated consumable cost would be adjusted to $7.63/100 m3 water treated. Since the same amount of H2S would be required to precipitate zinc from Snailbeach Farm waste water off-line, consumable costs would be similar to in-line treatment.
Force Crag contains ∼0.05 mM zinc (3.25 g m–3) and requires 0.05 mole of H2S to completely remove the zinc from each cubic meter of mine water. Considering complete glycerol oxidation being coupled solely to the reduction of ZVS (there was no evidence of net reduction of sulfate during Force Crag experiments), this requires 0.7 moles (0.06 kg) of glycerol for each 100 m3 of water treated (stoichiometry of 1 glycerol:7 ZVS) which would cost ∼ $0.03. Five moles (0.16 kg) of ZVS would be consumed, at a cost of ∼$0.13/100 m3. The yeast extract required (equivalent to about 14 g/100 m3) would add a further $0.04, which then totals $0.20 for each 100 m3 of treated mine water. Assuming that the efficiency to generate H2S, in terms of glycerol utilization, would be possibly ∼50%, the estimated consumable costs would need to be adjusted to $0.40 for each 100 m3 of water treated.
A major advantage of the HBS system described over conventional chemical treatment is that metal is recovered (as a sulfide phase) which could be recovered, affording some revenue that could at least partly off-set OPEX. Using a zinc metal commodity price of $3.5 kg–1, and concentrations of 65 mg L–1 Zn2+ in Snailbeach Farm and 3 mg L–1 in Force Crag mine waters, 6.5 and 0.3 kg of ZnS could in theory be recovered from 100 m3 of each of these, respectively, equivalent to estimated metal values of $22.75 and $1.05, in both cases exceeding (marginally in the case of Force Crag) OPEX. While other costs, including capital costs (CAPEX) for equipment, maintenance, and energy charges also need to be considered, these figures nonetheless suggest that the HSB system (both in-line and off-line configurations) could be an effective alternative approach for remediating both of these circum-neutral pH waters.
Conclusion
Circum-neutral pH, zinc-contaminated mine waters at two geographically distant abandoned mining areas in England were successfully remediated using a novel HSB, and zinc recovered as a solid sulfide precipitate that would allow it to be reprocessed (e.g., in a smelter) and recycled. Up to >99% of soluble zinc was removed from synthetic and actual mine waters, and processed MIWs remained circum-neutral pH and contained concentrations of zinc that were well below discharge consent levels (10.9 μg bioavailable Zn L–1; Environmental Quality Standards of the European Environment Agency). The economics of applying the proposed technology are encouraging.
Data Availability Statement
The datasets generated for this study are available on request to the corresponding author.
Author Contributions
RH carried out the experimental work, and contributed to writing the manuscript and preparing the tables and figures. DJ wrote the manuscript and designed the experiments.
Funding
RH is grateful to the National Council of Technological and Scientific Development (CNPq—Brazil) for provision of a research studentship (Grant. No. 248980/2013-0).
Conflict of Interest
The authors declare that the research was conducted in the absence of any commercial or financial relationships that could be construed as a potential conflict of interest.
Acknowledgments
We thank the Coal Authority (United Kingdom) for providing mine water data and facilitating mine water collection.
Supplementary Material
The Supplementary Material for this article can be found online at: https://www.frontiersin.org/articles/10.3389/fenvs.2020.00022/full#supplementary-material
References
Anon (2005). Standard Methods for the Examination of Water and Wastewater, 21st Edn, Washington D.C: American Public Health Association.
Anwar, M. A., Iqbal, M., Qamar, M. A., Rehman, M., and Khalid, A. M. (2000). Technical communication: determination of cuprous ions in bacterial leachates and for environmental monitoring. World J. Microbiol. Biotechnol. 16, 135–138.
Blowes, D. W., Ptacek, C. J., Jambor, J. L., Weisener, C. G., Paktunc, D., Gould, W. D., et al. (2014). The Geochemistry of acid Mine Drainage’ in Treatise on Geochemistry, 2nd Edn, Oxford, UK: Elsevier, 131–190.
Bratty, M., Lawrence, R., Kratochvil, D., and Marchant, B. (2006). “Applications of biological H2S production from elemental sulfur in the treatment of heavy metal pollution including acid rock drainage,” in Proceedings of the 7th International Conference on Acid Rock Drainage, St. Louis, MO.
Cline, C., Hoksberg, A., Abry, R., and Janssen, A. (2003). “Biological process for H2S removal from gas streams the Shell-Paques/THIOPAQTM gas desulfurization process,” in Proceedings of the Laurance Reid Gas Conditioning Conference, Norman, OK.
Gandy, C. J., Davis, J. E., Orme, P. H. A., Potter, H. A. B., and Jarvis, A. P. (2016). Metal removal mechanisms in a short hydraulic residence time subsurface flow compost wetland for mine drainage treatment. Ecol. Eng. 97, 179–185. doi: 10.1016/j.ecoleng.2016.09.011
Jarvis, A., Gandy, C., Bailey, M., Davis, J., Orme, P., Potter, H., et al. (2015). “Metal removal and secondary contamination in a passive metal mine drainage treatment system,” in Proceedings of the 10th International Conference on Acid Rock Drainage/International Mine Water Association, Santiago.
Johnson, D. B., and Hallberg, K. B. (2002). Pitfalls of passive mine treatment. Rev. Environ. Sci. Biotechnol. 1, 335–343. doi: 10.1023/a:1023219300286
Johnson, D. B., and Hallberg, K. B. (2005). Biogeochemistry of the compost bioreactor components of a composite acid mine drainage passive remediation system. Sci. Tot. Environ. 338, 81–93. doi: 10.1016/j.scitotenv.2004.09.008
Johnson, D. B., and Sánchez-Andrea, I. (2019). Dissimilatory reduction of sulfate and zero-valent sulfur at low pH and its significance for bioremediation and metal recovery. Adv. Microb. Physiol. 75, 205–231. doi: 10.1016/bs.ampbs.2019.07.002
Kay, C., Rowe, O., Rocchetti, L., Coupland, K., Hallberg, K. B., and Johnson, D. B. (2013). Evolution of microbial “streamer” growths in an acidic, metal-contaminated stream draining an abandoned underground copper mine. Life 3, 189–210. doi: 10.3390/life3010189
Mayes, W. M., Johnston, D., Potter, H. A. B., and Jarvis, A. P. (2009). A national strategy for identification, prioritisation and management of pollution from abandoned non-coal mine sites in England and Wales. Sci. Total Environ. 407, 5435–5447. doi: 10.1016/j.scitotenv.2009.06.019
Mayes, W. M., Potter, H. A. B., and Jarvis, A. P. (2013). Riverine flux of metals from historically mined orefields in England and Wales. Water Air Soil Pollut. 224, 1425–1429.
Monhemius, A. J. (1977). Precipitation diagrams for metal hydroxides, sulphides, arsenates and phosphates. Trans. Inst. Min. Metall. 86, 202–206.
Ňancucheo, I., and Johnson, D. B. (2012). Selective removal of transition metals from acidic mine waters by novel consortia of acidophilic sulfidogenic bacteria. Microb. Biotechnol. 5, 34–44. doi: 10.1111/j.1751-7915.2011.00285.x
Ňancucheo, I., Rowe, O. F., Hedrich, S., and Johnson, D. B. (2016). Solid and liquid media for isolating and cultivating acidophilic and acid-tolerant sulfate-reducing bacteria. FEMS Microbiol. Lett. 363:fnw083. doi: 10.1093/femsle/fnw083
Rabus, R., Hansen, T. A., and Widdel, F. (2006). “Dissimilatory sulfate- and sulfur-reducing prokaryotes,” in The Prokaryotes, eds M. Dworkin, S. Falkow, E. Rosenberg, K. H. Schleifer, and E. Stackebrandt (New York, NY: Springer), 659–768. doi: 10.1007/0-387-30742-7_22
Sánchez-Andrea, I., Stams, A. J. M., Hedrich, S., Ňancucheo, I., and Johnson, D. B. (2015). Desulfosporosinus acididurans sp. nov.: an acidophilic sulfate-reducing bacterium isolated from acidic sediments. Extremophiles 19, 39–47. doi: 10.1007/s00792-014-0701-6
Santos, A. L., and Johnson, D. B. (2018). Design and application of a low pH upflow biofilm sulfidogenic bioreactor for recovering transition metals from synthetic waste water at a Brazilian copper mine. Front. Microbiol. 9:2051. doi: 10.3389/fmicb.2018.02051
Thomas, J. F. J., and Lynch, J. J. (1960). Determination of carbonate alkalinity in natural waters. J. Am. Water Works Assoc. 52, 259–268. doi: 10.1002/j.1551-8833.1960.tb00473.x
Keywords: bioreactors, mine waters, remediation, sulfidogenesis, sulfur, zinc
Citation: Holanda R and Johnson DB (2020) Removal of Zinc From Circum-Neutral pH Mine-Impacted Waters Using a Novel “Hybrid” Low pH Sulfidogenic Bioreactor. Front. Environ. Sci. 8:22. doi: 10.3389/fenvs.2020.00022
Received: 16 October 2019; Accepted: 19 February 2020;
Published: 10 March 2020.
Edited by:
C. Marjorie Aelion, University of Massachusetts Amherst, United StatesReviewed by:
Gordon Southam, The University of Queensland, AustraliaSusan Anne Baldwin, The University of British Columbia, Canada
Xiangke Wang, North China Electric Power University, China
Pavlina Kousi, National Technical University of Athens, Greece
Copyright © 2020 Holanda and Johnson. This is an open-access article distributed under the terms of the Creative Commons Attribution License (CC BY). The use, distribution or reproduction in other forums is permitted, provided the original author(s) and the copyright owner(s) are credited and that the original publication in this journal is cited, in accordance with accepted academic practice. No use, distribution or reproduction is permitted which does not comply with these terms.
*Correspondence: David Barrie Johnson, ZC5iLmpvaG5zb25AYmFuZ29yLmFjLnVr
†Present address: Roseanne Holanda, SENAI Innovation Institute for Mineral Technologies, National Service for Industrial Training, Belém, Brazil