- Department of Natural Resources, Cornell University, Ithaca, NY, United States
Plant litter provides a fresh source of energy and nutrients to fuel microbial activity in soil, and in northern peatlands this can be leaf litter from mosses, graminoids, shrubs, and/or trees. Because Sphagnum and other mosses decompose slowly, vascular plant litter assumes a principal role, but its role in microbial methane production is unclear. Therefore, we examined decomposition of leaf litter from nine species, including trees, shrubs, and graminoids, using litterbags positioned for up to 2.5 years in two raised bogs and in two rich fens. Across species leaf litter quality varied for concentrations of nitrogen, soluble organic matter, and cell wall composition. After 2.5 years of decay the amount of leaf litter mass remaining ranged from 43 to 63% in the bogs vs. 17 to 71% in the rich fens. Thus, site conditions interacted with litter quality to determine decay rates but with species-specific patterns. Leaf mass remaining after 0.5, 1.5, and 2.5 years of decay was incubated in vitro, without soil, to assess its ability to support methane production and concomitant anaerobic carbon dioxide respiration. Residue from all nine species supported methane production, with the greatest rates (up to 5,000 nmol g−1 day−1) in tissue with high concentrations of pectin. Rates were 2- to 700-times greater for the leaf material that decomposed in the rich fens than in the bogs. Production rates were more variable for methane than for anaerobic respiration. As seen for mass loss, differences in litter quality predicted variation in gas production rates but differently in the bogs than in the rich fens. The results underscore the importance of vascular plant litter in the biogeochemistry of carbon and methane in peatlands and why vegetation, plant species composition, and peatland type must be described to put peatland ecosystems into global budgets of carbon and methane.
Introduction
Plant litter provides a fresh source of energy and nutrients for microorganisms in soil, and in northern peatland ecosystems, microorganisms can choose among a diversity of plant growth forms, including mosses, graminoids, shrubs, and trees. Because most Sphagnum and other mosses decompose at a slower rate than vascular plant leaves (Lang et al., 2009), partially decayed moss tissue is a major component of peat soil (Van Breemen, 1995), and studies show that decaying vascular plant tissue assumes a principal role in energy flow and soil microbial activity (Straková et al., 2011; Del Giudice and Lindo, 2017). This is especially important for anaerobic methane (CH4) production (methanogenesis) in peat soil (Kotsyurbenko et al., 2019).
Not all vascular plant leaves decompose at the same rate, and many studies show that species-specific leaf traits extend to decomposability. This is summarized in the concept of litter quality, which has three aspects. One is structural traits, such as specific leaf area (SLA) and leaf dry matter content (LDMC), that influence microbial colonization of the tissue. Second are nutrients, primarily nitrogen (N) and phosphorous, that support growth of microbial decomposers. Third are the organic polymers that provide energy for microbial decomposers. These three aspects of litter are especially important in northern peatlands, because the diversity of plant growth forms display a wide range of litter types and decay rates (Belyea, 1996; Dorrepaal et al., 2005; Bragazza et al., 2007; Moore et al., 2007; Ward et al., 2015).
Soil properties also influence litter decay rates. Again, this is especially important for northern peatlands, which range from nutrient-rich fens that have a hydrologic connection with groundwater to nutrient-poor fens and bogs that become increasingly isolated from groundwater inputs (Siegel and Glaser, 1987). As a result, peat soil from poor fens and bogs can rise above the persistent water table level, be drier and more acidic (McLaughlin and Webster, 2010; Webster and McLaughlin, 2010), and have slower rates of microbial activity than in richer fens (Moore et al., 2007). However, the better-aerated conditions in bogs might enhance aerobic decomposition of plant litter to the detriment of anaerobic microorganisms that predominate in the water-saturated portion of the peat soil.
It is well-known that peatland systems support microbial methanogenesis, but there is still uncertainty about the depth at which it occurs in the peat soil. Most of the debate is whether methanogens occur at the top of the persistent water table to take advantage of freshly decaying litter coming from above (Franchini et al., 2015) vs. deeper in the fully anoxic peat soil to avoid aeration (Sundh et al., 1994; Glaser et al., 2016). On the other hand, studies have shown that bacteria and decomposer fungi do colonize decaying litter at the peat soil surface (Andersen et al., 2013), but whether this includes anaerobic methanogens is less well-known. Furthermore, there is the question whether anaerobes colonize the decaying litter at the onset of the process vs. latter as the residue sinks into the surface peat (Corteselli et al., 2017). Showing that anaerobic methanogens can colonize and produce CH4 in leaf litter that sits well above the water table would add a new dimension to the spatial patterns of methanogenesis in peatland ecosystems.
Therefore, the aims of this study were to examine: (1) the relative importance of plant growth form and peatland type for leaf litter decomposition, (2) how variation in leaf litter quality helps to explain variation in decay rates, and (3) the ability of decaying leaves to support microbial activity, in particular, methanogenesis. The main findings of the study were: (1) leaf litter from broad-leaf trees and graminoids decayed faster in rich fens than in bogs, whereas leaf litter from shrubs and needle-leaf trees had faster decay rates in bogs, (2) several aspects of litter quality helped to explain variation in litter decay rates in rich fens, but the same aspects were less effective predictors in bogs, and (3) the decaying residue supported methanogenesis, especially, in the rich fens.
Materials and Methods
Study Sites and Experimental Design
We examined leaf litter decomposition in peatlands located near Ithaca, NY. Mean annual temperature in the region is 8.9°C, with monthly mean temperatures ranging from −4.8°C in January to 20.4°C in July. Mean annual precipitation is 890 m.
Dryden Bog (42°26′51.5″N 76°15′33.3″W) is a small ombrotrophic bog ~150 m across (1.75 ha area). The maximum peat depth is 8 m. The surface consists of well-developed hummocks, dominated by the shrub Chamaedaphne calyculata (leatherleaf) and Sphagnum mosses (S. capillifolium, S. fuscum). Hollows occur between hummocks and have a mixture of the graminoid Eriophorum vaginatum (cotton grass), Vaccinium macrocarpon (cranberry), and Sphagnum mosses (S. magellanicum, S. angustifolium, and S. fallax). The water table is close to the surface of hollows following spring snowmelt and it drops about 15 cm below the surface of the hollows by mid-summer.
McLean Bog (42°32′55.4″N 76°15′58.2″W) is an ombrotrophic kettle hole bog ~70 m across (0.4 ha area). Maximum peat depth is 8 m. The bog surface has a lawn-type microtopography. Evergreen shrubs include leatherleaf, Rhododendron groenlandicum (Labrador tea), and Kalmia angustifolia (sheep laurel). Cotton grass and Dulichium arundinaceum (three-way sedge) have moderate cover. Sphagnum mosses include: S. capillifolium, S. fallax, S. magellanicum, and S. angustifolium.
McLean Fen (42°32′39.5″N 76°16′05.8″W) is rich fen ~75 m across (0.5 ha area). Maximum peat depth is 3 m. The wetland is underlain by glacio-fluvial stratified sand and gravel with a highly calcareous matrix. The fen receives water from three ground-water springs. We used an area dominated by Carex stricta (tussock sedge).
Salt Road Fen (42°35′53.5″N 76°19′17.8″W) is a rich fen ~250 m across (6.25 ha area). Maximum peat depth is 2 m. The site has a mixture of sedges and shrub cover, including Cornus racemose (gray dogwood) and Rhamnus alnifolia (swamp buckthorn). We used an area with Typha latifolia (cattail) and several sedge species: Carex flava, C. prairiea, and C. atlantica.
We selected nine plant species (Table 1). Two species were broad-leaf deciduous trees: Acer rubrum (red maple) and Alnus glutinosa (common alder). Two species were shrubs: Myrica gale (bog myrtle) and leatherleaf. Two species were graminoids: cattail and Carex lacustris (lake sedge). Two species were needle-leaf evergreen trees: Pinus strobus (white pine) and Pinus rigida (pitch pine). We also selected a needle-leaf deciduous tree species: Larix laracina (larch). We refer to the species by common names in the paper.
Two sets of leaves were collected from mature plants growing in close proximity to each other in the Cornell Botanical Gardens. The close proximity ensured exposure to the same soil and climate. Bog myrtle does not occur in the Cornell Botanical Gardens, and thus leaf material was collected from a nearby wetland (Lake Como, NY, outlet stream). Leatherleaf does not occur in the Cornell Botanical Gardens, and thus leaf material was collected from the Dryden Bog study site.
One set consisted of green leaves collected in August 2015 by-hand from fully sun lit parts of five individual plants per species. The other set consisted of leaf litter collected at the end of the growing season in October 2015 by shaking branches gently or with a light touch indicating a well-formed abscission zone. Fallen leaves were gathered from a sheet of plastic placed beneath the canopy. Portions from both collections were air-dried at 24°C to a constant mass before being ground for chemical analyses.
Decomposition Experiment
The senesced leaf litter was used for the decomposition study. For each species, 10–20 g (dry mass equivalent) of leaf litter was sealed within 1.2 mm mesh fiberglass screen measuring 20 × 20 cm. This mesh size allowed access to microbial decomposers as well as oribatid mites and ants, which are the dominant invertebrates in the food web in peat soils (Barreto and Lindo, 2018). One bag of each species was fixed to a piece of 1.65 mm monofilament trimmer line in a random arrangement, resulting in nine bags on each line. Twenty replicate lines of litterbags were placed in each site in November 2015. All bags were placed at the soil surface, about 2–5 cm below the tops of moss plants if present.
The experimental design consisted of four plant growth forms, two plant species per growth form, plus larch, and two peatland types, each with two replicates, with five replicate sets of litterbags per site to be collected after 0.5, 1.5, and 2.5 years in the field (N = 540 litterbags). Although we did collect the five replicates per site after 0.5 year, a frisky bear decided to munch on some of the litterbags in McLean Bog and in McLean Fen after that, destroying several replicates. Thus, we reduced the sample size to two replicates per peatland for the 1.5-years collection. However, the bear was apparently not done, and it ate the remaining bags in McLean Bog. Thus, the 2.5-years collection consisted of five replicates from Dryden Bog, three replicates from Salt Road Fen, and two replicates from McLean Fen.
Weather conditions in the 0.5 year prior to the first collection were warmer (+0.38°C) and drier (−121 mm) than normal. Between the first and second collections, weather was warmer (+1.1°C) and wetter (+76 mm) than normal. Air temperature was cooler (+0.3°C) whereas precipitation was normal between the second and third collections.
Upon collection, the litterbags were cleaned of moss and roots before being opened, and the remaining leaf litter residue in each bag was weighed and divided into three portions. One was used to determined water content as the ratio of wet mass to dry mass, following oven drying to a constant mass. The second portion was placed in a plastic bag and stored for a short time (<3 days) in a cold room before being incubated in vitro to measure production rates for CH4 and CO2 (see below). The third portion was dried at 60°C until constant mass and used for chemical analyses.
Leaf Traits and Litter Quality
The green leaves were used for measures of specific leaf area (SLA) and leaf dry matter content (LDMC). Specific leaf area was calculated as the ratio between projected leaf area (cm2) and leaf oven-dried mass (g). The adaxial side of needle leaves from coniferous species was determined using microscopy. Leaf dry matter content was calculated as the ratio of oven-dried mass (g) to water-saturated mass (g). Leaves were considered water saturated after being stored in a plastic bag between wet paper towels at 4°C for 2 days.
The chemical composition of leaf litter was determined on samples collected before decay and on the residue from litterbag collections. Portions were analyzed sequentially for neutral detergent fiber (NDF), acid detergent fiber (ADF), and acid-detergent lignin on an ANKOM fiber analyzer (ANKOM Technology, Macedon, New York, USA). The analyses are used to approximate hemicellulose (NDF–ADF), cellulose (ADF–acid-detergent lignin), and lignin (acid-detergent lignin) (Van Soest, 1994). Protocols are available at: https://www.ankom.com/analytical-methods-support/fiber-analyzer-a2000. Total nitrogen (N) was determined by dry combustion on a LECO CN analyzer.
We further characterized cell wall composition by a sequential extraction procedure described in McLeod et al. (2007). Briefly, tissue (0.2 g) is extracted sequentially to: remove free sugars and polysaccharides (fraction 1, 10% formic acid); remove loosely bound pectin (fraction 2, phosphate buffer (200 mM NaH2PO4, 0.5% w/v chlorobutanol, 10 mM Na2S2O3, adjusted to pH 7); remove calcium, which releases pectin from the middle lamella (fraction 3, cyclohexanediamine tetra acetic acid (CDTA at pH 7.5; Chapman et al., 1987); break hydrogen bonds and separate pectin from cellulose, and to remove cell wall proteins (fraction 4, urea; Chapman et al., 1987); unmask pectin in the primary cell wall (fraction 5, sodium carbonate (200 mM Na2CO3 at 5°C); liberate hemicellulose (fraction 6, sodium hydroxide (6 M NaOH, 1% w/v NaBH4 at 37°C; Carpita, 1984); and, finally remove all remaining non-structural sugars (fraction 7, 5% formic acid). Each extraction took place in a 50 ml centrifuge tube with 6 ml of solvent added and incubated for 24 h. Each solvent was removed by centrifugation and saved for colorimetric analysis.
Total carbohydrates in the solvents were quantified using an o-phenanthroline colorimetric assay (Dubois et al., 1956). Before the colorimetric assay, the extract was diluted with de-ionized water and de-salted through a 10-mL plastic syringe containing around 1-mL of microfiber glass wool and 9-mL exchange resin (Mixed Bed IONAC NM-60 H+/OH− Form, Type I, Beads 16–50 Mesh). De-salting was repeated until the remaining salt concentration was below 50 mS m−1. The colorimetric assay was done by mixing 1 mL solution with 50 uL 0.1 M K3Fe(CN)6 solution, 100 μL alkaline reagent and 1 mL color reagent (o-phenanthroline solution), and quantified by 505 nm wavelength. Hereafter we refer to these as soluble sugars (fractions 1 + 7), middle lamella pectin (fractions 2 + 3), cell wall pectin (fractions 4 + 5), and hemicellulose (fraction 6).
Methanogenesis and Anaerobic Respiration
Portions of the leaf litter residue per litterbag (ca. 5 g at field moisture levels) were placed into 236-mL glass jars containing 10 mL of distilled water, and the jars were sealed with a lid that had a gas-impermeable rubber septum to facilitate taking a sample of the headspace gas for analysis. Each jar was made anoxic by removing the headspace with a vacuum pump for 1 min and replacing with O2-free N2, repeated three times. The jars remained closed during a 40-days period, which is an accepted time-period to examine anaerobic processes in peat soils (Yavitt et al., 1997). Periodically, 5-mL gas samples were from the jar headspace, replaced with 5 mL of N2, and analyzed for CH4 and CO2 as described below.
In addition, we examined rates of methanogenesis and anaerobic respiration by peat soils with an addition of leaf litter residue from the 0.5- or 1.5-years decay classes. Bulk collection of peat soil from Dryden Bog and McLean Fen was homogenized under constant stream of N2, and 30 g (fresh mass) portions plus 30 mL of degassed, de-ionized water were placed into 236-mL glass jars, made anoxic as described above. The jars were incubated for 14 days to determine a baseline level of methanogenesis and anaerobic respiration. After this pre-incubation period, the lids were removed and 5 g of air-dried leaf litter residue from individual litterbags was added and pressed down lightly to ensure soil-residue contact. The jars of soil and added litter were resealed, made anoxic, and samples of the jar headspace were collected periodically for 40 days and analyzed for concentrations CH4 and CO2.
Methane in the gas samples was analyzed using a Shimadzu GC-14AP Greenhouse Gas Analyzer. This model is equipped with a Flame Ionization Detector (FID) for CH4. The instrument parameters include a 10-mL sample loop, run time of 5.5-min, ECD temperature of 240°C, column temperature of 60°C, and injection temperature of 65°C. We used 20 mL of each sample to flush and fill the sample loop. Methane peaks are detected by the FID at 1.667-min and compared to known gas standards. Carbon dioxide in the gas samples was analyzed using an open gas exchange system (Li-Cor 6400, Li-Cor Inc., Lincoln, Nebraska, USA) with an in-line septum.
Calculations
Samples of air-dried leaf litter and residue were weighed before and after oven-drying to develop conversion factors to express mass of leaf litter on a dry mass basis. We determined the percentage of leaf litter mass remaining in litterbags at each collection point and fit the data to a single exponential decay equation y = e−kt (Olson, 1963), where y is the percentage of mass remaining, k is the decay rate constant, and t is time in years. We estimated the value for k as the slope of the linear relationship between the natural logarithm of the percentage mass remaining vs. time. We did not include a value for mass prior to placement in the field (100% mass remaining) to account for rapid mass loss due to leaching of soluble compounds.
The analysis of biochemical components in leaf litter, both for the material before decomposition as well as the residue from the litterbags, had to be scaled back due to funding limitations, which precluded analysis of individual samples. Therefore, we did the analyses on samples pooled by species and site across replicate litterbags. Thus, independent tests of differences in biochemical composition were not possible. The results for biochemical composition should be regarded as suggestive rather than conclusive.
Data Analysis
The low statistical power in the experimental design main effects (n = 4 plant growth forms; n = 2 species per growth; n = 2 peatland types) and interactions constrains our ability to draw real biological inferences with traditional statistics, such as Analysis of Variance (ANOVA). Furthermore, the loss of litterbags from McLean Bog during the decay period and lack of true replication in leaf litter quality makes it difficult to determine if non-significant results were caused by a true lack of differences or simply an artifact of limited sample size.
Therefore, we evaluated the magnitude and direction of treatment effects by calculating the Effect Size, as Cohen's d (Nakagawa and Cuthill, 2007). To overcome limitation of small sample size, we compared mass loss and gas production rates in bogs vs. rich fens, with the replicate litterbags used to estimate variability. Rather than claim that differences are statistically significant, we follow the suggestion of Amrhein et al. (2019) and report means, estimates of variation, sample size, and P-values.
Relationships among decay rates and rates of methanogenesis and anaerobic respiration with predictors, leaf traits and litter quality, were examined using Pearson Correlation.
Results
Decomposition
Across the nine species and four sites, an average of 57% of the leaf litter mass remained after 2.5 years of decomposition (Figure 1). Fitting a single-exponential decomposition model to the mass remaining, species-specific decomposition rate constants (k) ranged from 0.06 to 0.54 y−1. Decomposition rate constants were fairly similar (p = 0.7194) for decay in the bogs (k = 0.22 ± 0.075 y−1 [mean ± standard deviation SD], n = 18) vs. the rich fens (k = 0.24 ± 0.16 y−1, n = 18). Ranking leaf litter decay rates among plant growth forms from fastest to slowest was: broad-leaf deciduous trees, 0.34 y−1 > graminoids, 0.26 y−1 > shrubs, 0.20 y−1 > needle-leaf trees, 0.16 y−1). Peatland type affected decay rates as a function of plant growth form (Table 2); leaf litter from the broad-leaf deciduous tree species and the graminoid species decayed faster in the rich fens than in the bogs (p = 0.0425), whereas leaf litter from the shrub species and needle-leaf tree species decomposed faster in the bogs than in the rich fens (p = 0.0688). Although the two species per plant growth form showed some differences in their temporal patterns of mass loss during the 2.5 years period (Figure 1), pairwise comparison of a species effect calculated as Cohen's d showed no effect for the four plant growth forms, i.e., 95% C.I. of Cohen's d comparing the two species crossed zero.
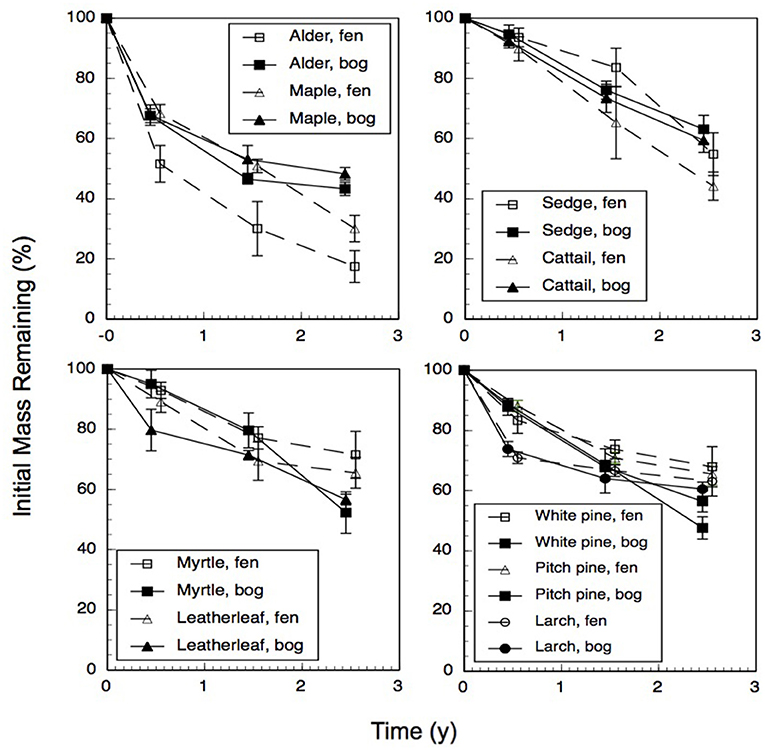
Figure 1. Percentage of initial mass remaining for leaf litter from nine plant species having decayed in bogs or in rich fens for three amounts of time. Values are mean + standard error; n = 10 for 0.5 year, n = 4 for 1.5 years, n = 5 for 2.5 years.
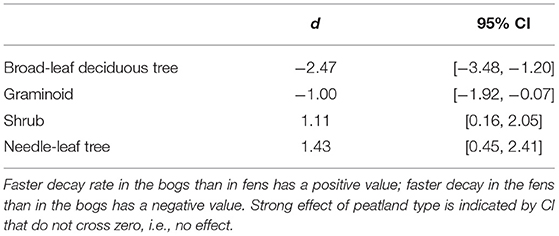
Table 2. Standardized mean effect sizes Cohen's d and 95% confidence intervals (CI) of leaf litter decay rate in bogs vs. fens.
Leaf Traits and Litter Quality
Traits of green leaves varied among the nine species (Table 1): the variation was greater for SLA (Coefficient of Variation, CV = 52% among the nine species) than for LDMC (CV = 25%). In general, green leaves with longer life span (LLS) had smaller values for SLA, whereas LDMC was lower for the broad-leaf deciduous tree species and graminoid species than for the shrub species and needle-leaf tree species.
The cell wall composition of leaf litter (Table 3) revealed greater concentrations of pectin and cellulose than concentrations of lignin, hemicellulose, and soluble components. Overall cell wall pectin was the least variable component among the nine species (CV = 13%). Pectin in the middle lamella (CV = 32%), cellulose (CV = 41%), and lignin (CV = 36%) were moderately variable, whereas N (CV = 64%), soluble components (CV = 60%), and hemicellulose (CV = 74%) were the most variable. There were relative differences among components as a function of plant growth form. For example, the two broad-leaf deciduous tree species were characterized by a relatively large fraction of soluble components; the two graminoid species were characterized by a relatively large fraction of cellulose; and, the shrub species and larch were characterized by a relatively small fraction of hemicellulose but large concentrations of lignin. A large concentration of N was evident in the two species associate with microbial N fixation and in cattail.
We used the fraction of the initial amount of each cell wall component that remained in the residue after 2.5 years of decay to show how components of cell walls changed during decomposition (Table 4). For N, leaf litter with a large amount initially, due to N fixation (alder, bog myrtle) or N uptake from the soil (cattail), lost N during decomposition, whereas maple, sedge, and leatherleaf accumulated N during decay period. Ranking mass loss of cell wall components from the most to the least lost was: soluble > pectin = cellulose > hemicellulose > lignin. The broad-leaf deciduous leaf litter was distinguished by large loss of soluble components; the graminoids were distinguished by slower loss of soluble components; the shrubs and needle-leaf litter had average patterns of mass loss for components. Although these patterns were fairly similar between bogs and rich fens, mass loss rates for hemicellulose was much less in the bogs than in the rich fens.
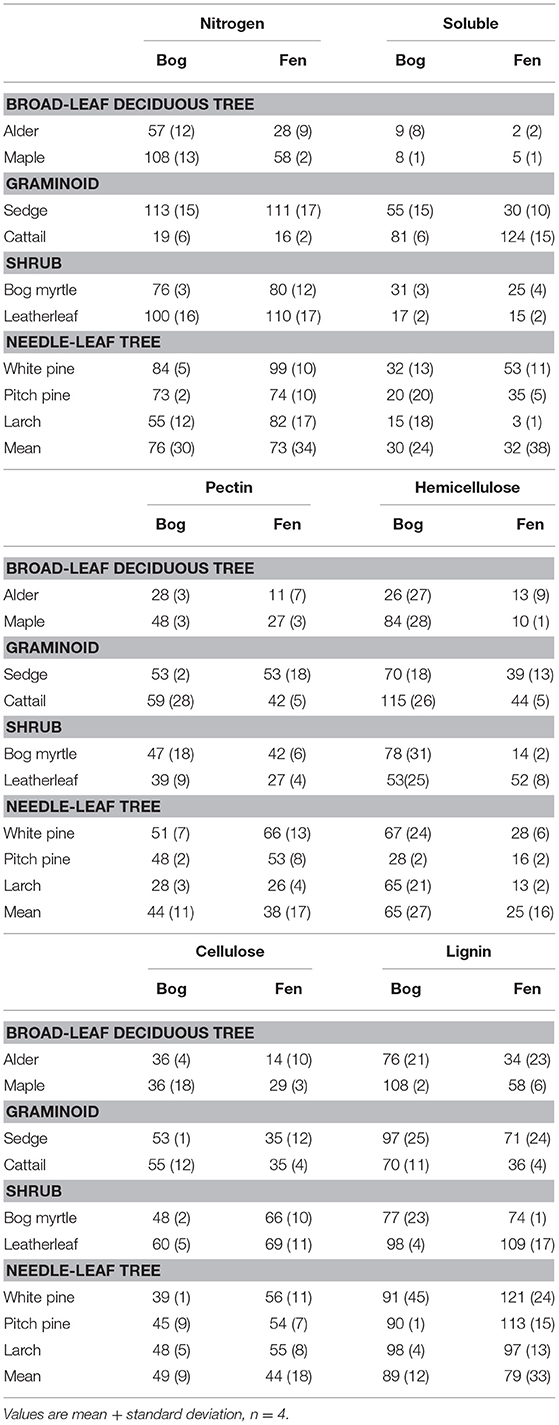
Table 4. Fraction remaining (%) of original mass of cell wall components after 2.5 years of decomposition.
Methanogenesis and Concomitant Anaerobic CO2 Production
Portions of leaf litter residue incubated in vitro without oxygen to assess methanogenesis showed rates that varied by a factor of 5,000, from 1 to 5,172 nmol g−1 dry residue d−1 (Figure 2). Across the nine species and four sites, rates of methanogenesis were greater for the 0.5-year old residue (67 + 84, nmol g−1 dry residue d−1, n = 180) and the 1.5-years old residue (304 + 658 nmol g−1 dry residue d−1, n = 72) than for the older 2.5-years old residue (6 + 7, nmol g−1 dry residue d−1, n = 90). Across the nine species and ages of the residues, rates were greater for leaf litter that decomposed in the rich fens (234 + 545 nmol g−1 dry residue d−1, n = 276) than in the bogs (18 + 28 nmol g−1 dry residue d−1, n = 276), with the ranking from greatest to least: broad-leaf deciduous trees > graminoids > shrubs = needle leaf trees. The effect of peatland type on rates of different plant growth forms (Table 5) was stronger for graminoids and shrubs than for the other two growth forms.
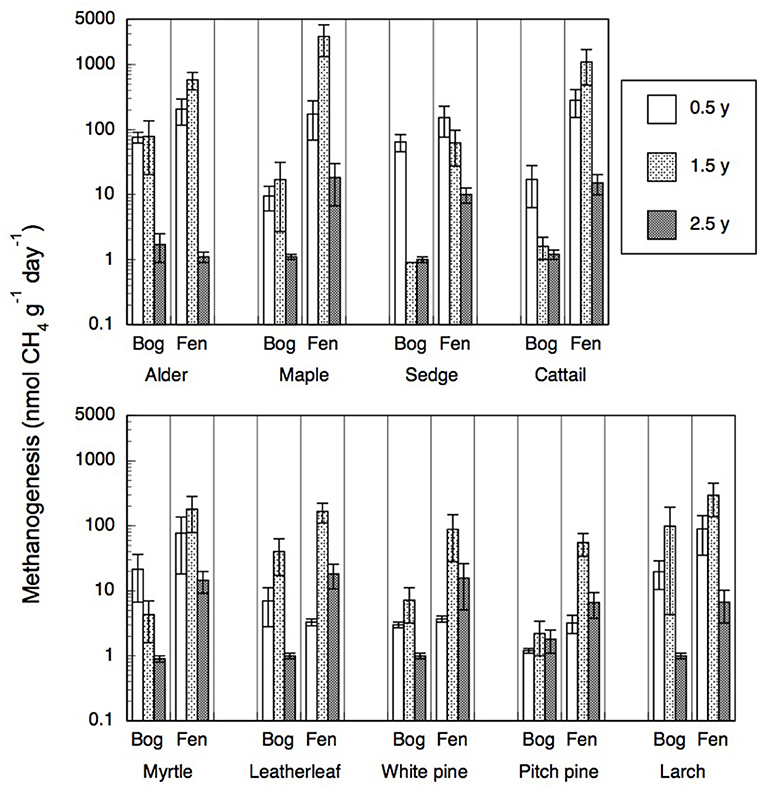
Figure 2. Rates of methanogenesis (nmol CH4 g−1 dry residue d−1) by leaf litter residue from nine species having decayed in bogs or in rich fens for three amounts of time. Values are mean + standard error; n = 10 for 0.5 year, n = 4 for 1.5 years, n = 5 for 2.5 years.
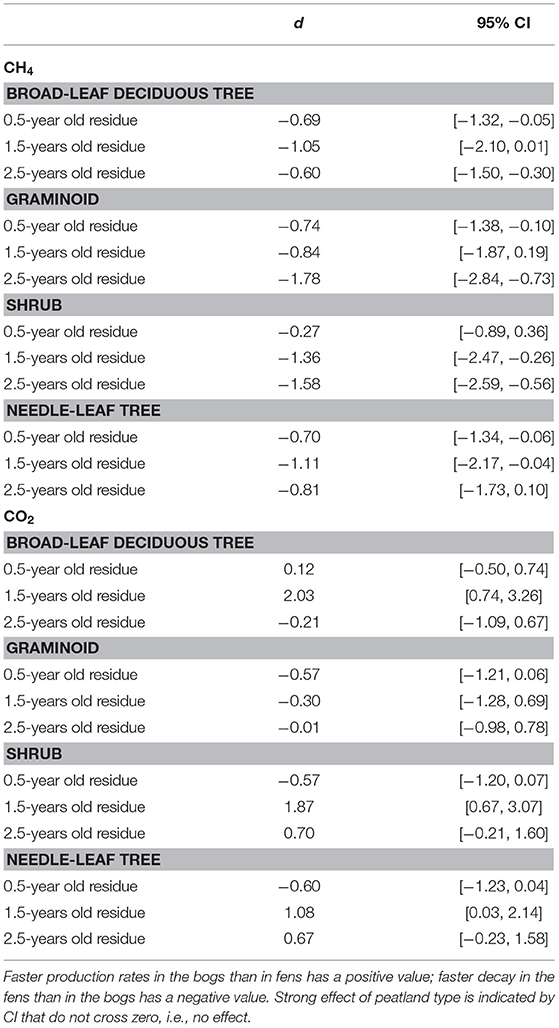
Table 5. Standardized mean effect sizes Cohen's d and 95% confidence intervals (CI) of leaf litter CH4 production and CO2 production in bogs vs. fens.
In contrast, anaerobic respiration varied by a factor of 25, from 4.2 to 106.7 μmol g−1 dry residue d−1 (Figure 3). Across the nine species and four sites, rates increased with increasing state of decay from the 0.5-years old residue (26 + 7 μmol g−1 dry residue d−1, n = 180) to the 1.5-years old residue (28 + 12 μmol g−1 dry residue d−1, n = 72) to the 2.5-years old residue (31 + 11 μmol g−1 dry residue d−1, n = 90). As a function of growth form, the ranking was: graminoids > broad-leaf deciduous trees > shrubs = needle leaf trees. Although rates were greater for leaf litter that decayed in the bogs (31 + 10 μmol g−1 dry residue d−1, n = 276) than in the rich fens (26 + 10 μmol g−1 dry residue d−1, n = 276), the effect of peatland type was evident only for 1.5-years old residue with greater rates for residue from the bogs (Table 5).
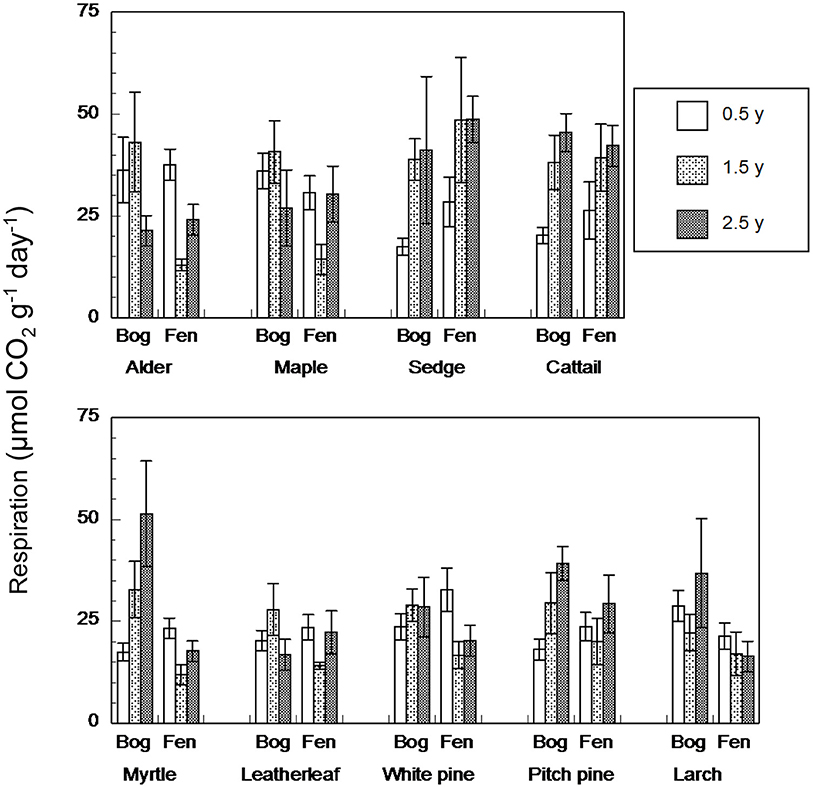
Figure 3. Rates of anaerobic respiration (μmol CO2 g−1 dry residue d−1) by leaf litter residue from nine species having decayed in bogs or in rich fens for three amounts of time. Values are mean + standard error; n = 10 for 0.5 year, n = 4 for 1.5 years, n = 5 for 2.5 years.
Portions of leaf litter residue added to soil resulted in rates of methanogenesis that ranged from 6 to 9,312 nmol g−1 d−1 (Figure 4). In contrast, the peat soils without added leaf litter had rates of <20 nmol g−1 d−1. Across the nine species and two types of soil, rates were greater with older 1.5-years old residue (1,634 + 2,235 nmol g−1 d−1, n = 72) than with the less decomposed 0.5-year old residue (337 + 879 nmol g−1 d−1, n = 72). Also, across the nine species and two types of soil, rates were greater with residue from decay in the bogs (1,405 + 2,272 nmol g−1 d−1, n = 72) than from decay in the rich fens (566 + 1,049 nmol g−1 d−1, n = 72). For most of the plant growth forms, the largest rates occurred with residue from decay in the bogs placed on the surface of fen soil.
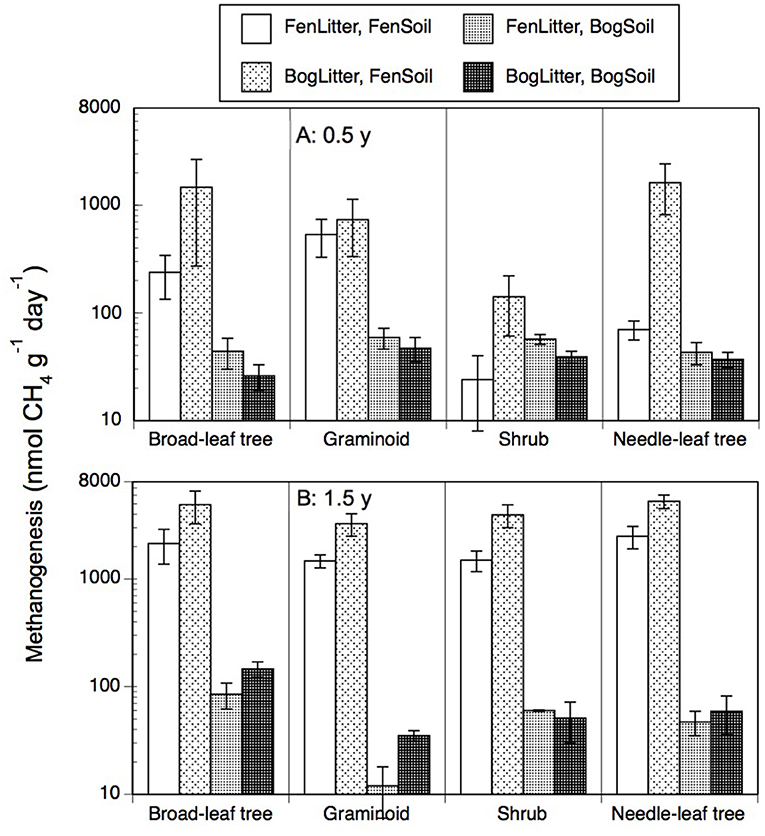
Figure 4. Rates of methanogenesis (nmol CH4 g−1 dry residue d−1) by leaf litter residue from four plant growth forms having decayed in bogs or in rich fens (A: 0.5 year, B: 1.5 years) mixed with peat soil from a bog or rich fen. Values are mean + standard error; n = 4 per plant growth form, 6 for needle-leaf trees.
Rates of anaerobic respiration with leaf litter residue and peat soil ranged from 20 to 232 μmol g−1 d−1 (Figure 5). Across the nine species and two types of soil, rates were 2-times greater with older 1.5-years old residue (99 + 58 mmol g−1 d−1, n = 72) than with the less decomposed 0.5-year old residue (54 + 22 μmol g−1 d−1, n = 72). However, across the nine species and two types of soil, rates were similar with residue from decay in the bogs (79 + 46 μmol g−1 d−1, n = 72) as with residue from decay rich fens (74 + 52 μmol g−1 d−1, n = 72). In general, the greatest rates occurred bog soil and 1.5-years old residue regardless where it had decayed.
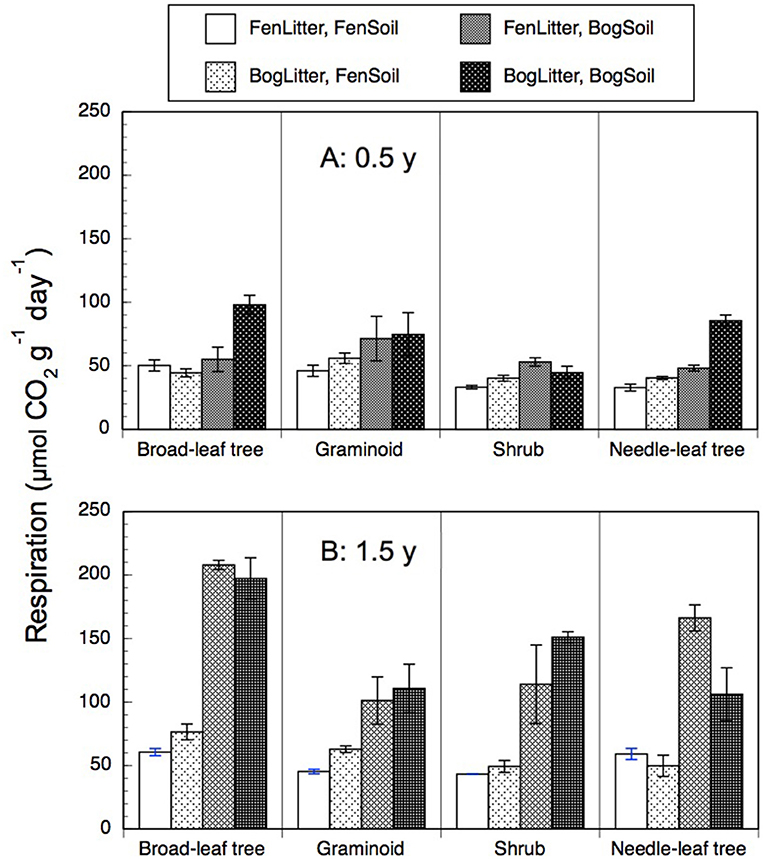
Figure 5. Rates of anaerobic respiration (μmol CO2 g−1 dry residue d−1) by leaf litter residue from four plant growth forms having decayed in bogs or in rich fens (A: 0.5 year, B: 1.5 years) mixed with peat soil from a bog or rich fen. Values are mean + standard error; n = 4 per plant growth form, 6 for needle-leaf trees.
Leaf litter decay rates showed positive relationships with SLA and the Sum of Cell Wall components (Sum CW) and negative relationships with LDMC and the lignin/N ratio, but only for decay in the rich fens (Table 6). None of the aspects of litter provided strong prediction of decay rates in the bogs. For gas production, correlations were calculated between the rate in the residue and the aspect of leaf litter quality measured in the residue at the same time (Table 6). Most of the cell wall components predicted rates of CH4 production by the 2.5-years old residue in the rich fens, with positive relationships for CWP, hemicellulose, and cellulose, whereas the relationship was negative for lignin. The best predictor for CH4 production in the bogs was for the 0.5-year old residue with a positive relationship for N and a negative relationship for the lignin/N ratio. Hemicellulose was the best predictor of CO2 production by leaf litter residue from the rich fens.
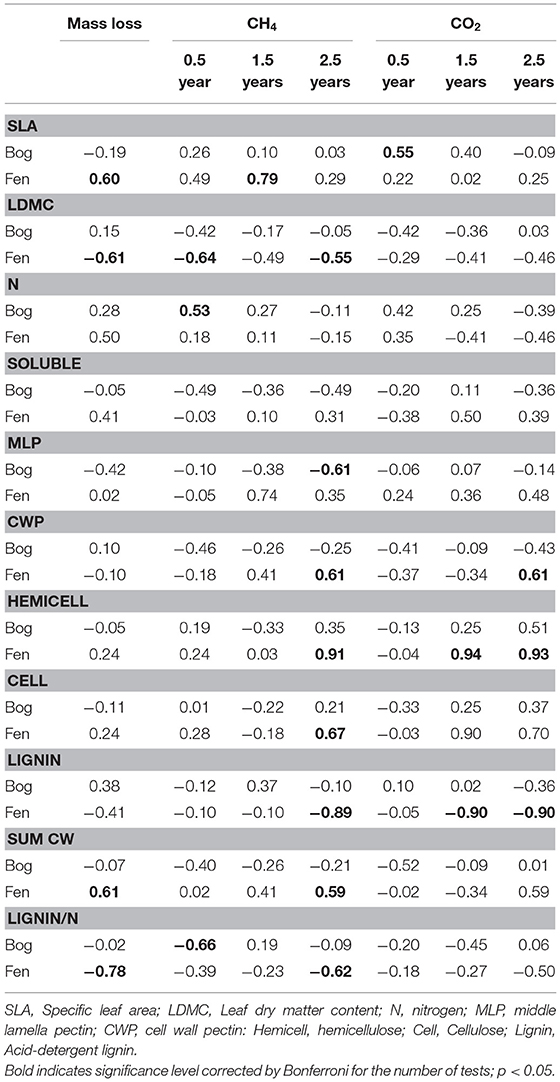
Table 6. Pearson correlation between aspects of leaf litter quality and mass loss and rates of CH4 production and CO2 by decayed residue.
Discussion
Decomposition
The interaction in leaf litter decay rates between plant growth form and peatland type adds a new dimension to explanations of ecosystem processes in peatlands. Previous studies have shown that leaf litter decay rates vary among plant growth forms (Dorrepaal et al., 2005; Lang et al., 2009) and types of peatlands (Szumigalski and Bayley, 1996; Moore et al., 2007). However, the interaction illustrates why it is necessary to specify vegetation, plant species, and peatland type when putting peatlands into broad cycles of carbon. One hypothesis of a vegetation × site interaction is that leaf litter decays faster in sites in which it came from, the so-called home-field advantage (Gholz et al., 2000). Here for example, sedge and cattail decomposed faster in rich fens where they occur than in bogs, and leatherleaf decomposed faster in bogs where it occurs than in the rich fens (Schwintzer, 1981). However, home-field advantage did not apply to bog myrtle, which despite its name occurs in fens (Schwintzer, 1984), and to larch, an indicator species for rich fens (Slack et al., 1980), both of which decomposed faster in the bogs. An alternative hypothesis for a vegetation × site interaction is that all litter species decompose at a fast rate in sites dominated by plants with decay-resistant litter, the so-called functional breadth hypothesis (Keiser et al., 2011). The idea is that microbial decomposers can deal with any litter quality when adapted to decay resistant litter, which is Sphagnum moss in bogs in this case (Van Breemen, 1995), whereas fastest-decomposing litter shows no preference for site. This applied here to the slowest decaying leaf litter from needle-leaf trees with faster decay rates in the bogs. However, slow-decaying litter from graminoids decayed faster in the rich fens, as opposed to the functional breadth hypothesis. Thus, neither hypothesis for a vegetation × site interaction in litter decay rates applied in toto to the plant species used here in the bog, rich fen contrast.
A key finding of our study is that aspects of litter quality were better predictors of litter decay rates in the rich fens than in the bogs (Table 6). For example, SLA and LDMC had strong relationships with litter decay rates but only in the rich fens. One explanation for such relationships is rapid colonization by microbial decomposers (Makita and Fujii, 2015). For example, a large value for SLA presents a large surface area for colonization, resulting in a positive relationship with decay rate. Decomposition in the rich fens also was negatively related to the ratio of acid-detergent lignin to N (Table 6). Lignin and N are well-documented predictors of decomposition in terrestrial ecosystems (Melillo et al., 1982; Berg and McClaugherty, 2014) but did not generalize to litter decay rates in the bogs, perhaps because of N mineralization per se in the bogs that complicates that relationship. One hypothesis is that N released from decaying tissue is lost quickly from the bog soil, and this seems to slow the decay of vascular plant litter (Reuter et al., 2019).
Pectin in the middle lamella emerged as a stronger predictor of leaf litter decay rates in the bogs (Table 6). Pectin is a major component of the middle lamellae (and primary plant cell wall) in vascular plant species and is typically 30–35% of cell wall dry weight (Pelloux et al., 2007), although lower levels of 5–10% are found in some graminoid species (Smith and Harris, 1999). Our finding pectin percentages of 29–42% (mean = 34%) among the nine species here is in line with others for trees, shrubs, and sedges. Because middle lamella pectin plays a functional role gluing cells to each other (Bou Daher and Braybrook, 2015), the negative relationship with litter decay rates suggests that the glue effectively slowed the rate of decay in bogs. Although the functional breadth hypothesis, mentioned above, would predict faster litter decay rates in in the bogs (Keiser et al., 2011; Fanin et al., 2016), and bogs typically harbor microorganisms with pectin-degrading enzymes (Thormann, 2006), these enzymes tend to be deactivated when bound to Sphagnum (Børsheim et al., 2001). Therefore, the unique chemistry of Sphagnum complicates litter decay in bogs (Van Breemen, 1995).
Our experimental design included two plant species associated with N-fixing microorganisms and cattail that initially had N-rich leaf litter, but these species did not have the most rapid decay rates. This finding is in line with other studies where leaf litter from plants with N-fixers does not decompose faster than leaf litter from non-N-fixing plants (Cornwell et al., 2008; Prescott, 2010). The experimental design also included plant species with a broad range of hemicellulose content. Schädel et al. (2010) examined hemicellulose contents in leaves for a range of plant growth forms and found that the ratio of cellulose + lignin to hemicellulose had a mean value of ca. 1.4 for graminoids, 1.75 for broad-leaf trees, and 2.7 for needle-leaf trees. Values here are larger, but with the same ranking among plant growth forms, with 2.4 for graminoids, 3.1 for broad-leaf trees, 4.9 for needle-leaf trees, and a notable value of 12.4 for the shrubs. The much larger values here, along with typical values for lignin and cellulose (Preston et al., 2009), point to the importance of pectin rather than hemicellulose in the leaf litter of vascular plant species that occur in peatlands.
After 2.5 years of decay, persistence of lignin in the residue of decaying litter is a well-established phenomenon (Preston et al., 2009). How chemistry of litter residue changes during decomposition has been much debated (cf., Wickings et al., 2012). For example, Soong et al. (2015) argued that lignin and cellulose decompose at similar rates maintaining a constant proportion in the residue over time, but this was not true for all nine of plant species we studied. Likewise, there is a general belief that about 50% of hemicellulose is lost quickly from decaying litter, within the first few months, but what remains does not decompose as easily (Machinet et al., 2011). However, the retention of hemicellulose during litter decay in the bogs vs. 75% of the initial hemicellulose being lost from decaying litter in the rich fens deviated from this conclusion. Overall, pectin is thought to be easily degradable (Jung and Engels, 2002). However, it is well-known that pectin accumulates in peat soils (Waksman and Reuszer, 1932), which is thought to be derived mostly from Sphagnum mosses. Thus, our data show that decomposing leaf litter from vascular plants is an additional source of pectin. These findings are important for the link to methanogenesis, as lignin and pectin contain methyl groups that can be fermented to methanol and acetate, which are substrates for methanogenesis (Lever et al., 2010).
Methanogenesis and Anaerobic Respiration
The maximum rates of methanogenesis attained by decaying leaf litter were impressive. Although there is no accepted level that constitutes an active rate vs. a less-active rate, using an arbitrary value of >100 nmol g−1 d−1 as active, all of the species, except pitch pine, were able to support active CH4 production. Indeed, upper values achieved by decaying leaf litter are in line with rates observed in peat soils that are known sources of atmospheric CH4 (Yavitt et al., 2018). This means that leaf litter from species across a broad range of plant growth forms, when decaying on the surface of peat soils, provides a suitable habitat for methanogenic microorganisms and associated anaerobes.
The wide range in rates of methanogenesis by decaying leaf litter, albeit from the nine species of plants, decayed in two types of peatlands, and with three ages of residue is not surprising, given that rates of methanogenesis are notoriously variable in peat soils (Yavitt et al., 1997; Treat et al., 2015; Kotsyurbenko et al., 2019). Many studies have sought the explanation(s) for variation in rates of methanogenesis in peatlands, but the multiple processes acting over a variety of spatial and temporal scales suggest that any one aspect might not be a stronger predictor of the process. For example, much greater rates of methanogenesis by leaf litter residue retrieved from the rich fens than the bogs in this study points to wetter soil conditions year-round in the rich fens that enable the growth and persistence of anaerobic methanogens. However, Godin et al. (2012) found greater diversity in the methanogenic community and active rates of methanogenesis in rich fens across a gradient of fen types, but soil wetness was not a good predictor. Several studies have singled out graminoids in peatlands for their role in methanogenesis (cf., Treat et al., 2015; Strack et al., 2017), given that they assume dominance in rich fens (Schwintzer, 1981).
It is noted that the two species of graminoids examined here were not associated with particularly large rates of methanogenesis. Other studies have attributed the positive effect of graminoids to rapid decay rates (Treat et al., 2015), but that does not apply to the two species here that had relatively slow rates of leaf litter decay. Another hypothesis is that graminoids lack phenolic compounds in litter (Emilson et al., 2018) that are toxic to methanogens. However, leaves from shrubs and needle-leaf trees are notoriously full of phenolics (Dorrepaal et al., 2005), yet decaying leaf litter from shrubs, and from needle-leaf trees here and in other studies (Yavitt and Williams, 2015; Corteselli et al., 2017) supported methanogenesis, which runs counter to the toxicity argument. Moreover, because most phenolics are water soluble (Min et al., 2015), they are likely leached away making the residue a less toxic habitat than expected from initial litter chemistry. Thus, the overwhelming prevalence of methanogenesis associated with litter decay in the rich fens challenges a single explanation.
The mostly negative relationships between aspects of litter quality and rates of methanogenesis for decay in the bogs (Table 6) seems to presuppose that leaf litter residue is a poorer substrate for methanogens in bogs. However, we tested this by mixing leaf litter residue retrieved from the bogs with fen soil. Even though it was a short-term in vitro study, the large rates of methanogenesis by bog-derived residue indicates no inherent inhibition of CH4 producers by the residue per se. Therefore, low rates of methanogenesis observed by decaying leaf litter in the bogs could be attributed to a relatively depauperate methanogenic community in the bogs vs. that in the rich fens (Dettling et al., 2007; Godin et al., 2012). Likewise, the positive link between pectin and rates of methanogenesis for decaying litter in rich fens but not in the bogs might be related to more active pectin-degrading microorganisms in fens than in bogs (Børsheim et al., 2001). The positive links between hemicellulose and rates of methanogenesis in the rich fens but not in the bogs are notable with further studies needed to pinpoint the mechanistic link.
Also evident in Table 6 is that the strength a correlation between a particular aspect of leaf litter quality and rates of methanogenesis changed as the residue aged. In some cases, the strength of the relationship weakened with age of the residue, such as with SLA, in other case it strengthened. Explanations for each aspect of litter quality are, of course, complicated by likely changes in the size and activity of different populations of methanogens associated with residue of different age (Sun et al., 2012).
Fairly constant rates of anaerobic respiration as the leaf litter residue aged can be explained by the combination of microbial growth on the residue (Witkamp, 1966) and greater respiration per unit of mass loss as microbes need to work harder to decompose components that accumulate as the residue ages (Wardle, 1993). This effect of greater CO2 production associated with decaying litter has been shown in a few studies (McLaren et al., 2017), and the results here extended it to anaerobic respiration. Since our findings include leaf litter that decomposes slowly as well as more quickly, and for decay in bogs and in rich fens, greater microbial respiration on aged litter residue might be a common feature of peatland ecosystems. The implication is that CO2 produced in peat soil is not always coming from decay of the freshest litter, but rather it is supported by microbial activity on aged litter.
Conclusions
During 2.5-years of decomposition, we found that decaying leaf litter provided a habitat for anaerobic CH4-producing microorganisms. This conclusion extended to leaves from trees, shrubs, and graminoids decomposing in bogs and in rich fens. With anaerobic microorganisms invading quickly at the onset of the decomposition process, and persisting in the residue, the results have broadly implications to spatial patterns of methanogenesis within the soil profile of peatland ecosystems. The initial decay rate of leaf litter is relatively slow in these ecosystems, and the decay rate further slows as the litter residue becomes progressively buried in the soil profile (Frolking et al., 2001). Thus, the species-specific responses will help to define whole-ecosystem CH4 production as the composition of plant species wax and wane over time in peatland ecosystems (Williams and Yavitt, 2003).
Data Availability Statement
The datasets generated for this study are available on request to the corresponding author.
Author Contributions
AK and JY designed the experiment. AK, MH, GP, and AR carried out the experiment. JY analyzed the data and wrote the paper with help from all co-authors.
Funding
This work was supported by a USDA National Institute of Food and Agriculture, Hatch project (1007286). Any opinions, findings, conclusions, or recommendations expressed in this publication are those of the author(s) and do not necessarily reflect the view of the National Institute of Food and Agriculture (NIFA) or the United States Department of Agriculture (USDA).
Conflict of Interest
The authors declare that the research was conducted in the absence of any commercial or financial relationships that could be construed as a potential conflict of interest.
Acknowledgments
We thank Alexis Heinz for indispensable assistance with logics in the laboratory.
References
Amrhein, V., Greenland, S., and McShane, B. (2019). Scientists rise up against statistical significance. Nature 567, 305–307 doi: 10.1038/d41586-019-00857-9
Andersen, R., Chapman, S. J., and Artz, R. R. (2013). Microbial communities in natural and disturbed peatlands: a review. Soil Biol. Biochem. 57, 979–994. doi: 10.1016/j.soilbio.2012.10.003
Barreto, C., and Lindo, Z. (2018). Drivers of decomposition and the detrital invertebrate community differ across a hummock-hollow microtopology in boreal peatlands. Écoscience 25, 39–48. doi: 10.1080/11956860.2017.1412282
Belyea, L. R. (1996). Separating the effects of litter quality and microenvironment on decomposition rates in a patterned peatland. Oikos 77, 529–539. doi: 10.2307/3545942
Berg, B., and McClaugherty, C. (2014). Plant Litter: Decomposition, Humus Formation, Carbon Sequestration. Berlin: Springer Verlag.
Børsheim, K. Y., Christensen, B. E., and Painter, T. J. (2001). Preservation of fish by embedment in Sphagnum moss, peat or holocellulose: experimental proof of the oxopolysaccharidic nature of the preservative substance and of its antimicrobial and tanning action. Innov. Food Sci. Emerg. 2, 63–74. doi: 10.1016/S1466-8564(00)00029-1
Bou Daher, F., and Braybrook, S. A. (2015). How to let go: pectin and plant cell adhesion. Front. Plant Sci. 6:523. doi: 10.3389/fpls.2015.00523
Bragazza, L., Siffi, C., Iacumin, P., and Gerdol, R. (2007). Mass loss and nutrient release during litter decay in peatland: the role of microbial adaptability to litter chemistry. Soil Biol. Biochem. 39, 257–267. doi: 10.1016/j.soilbio.2006.07.014
Carpita, N. C. (1984). Fractionation of hemicelluloses from maize cell walls with increasing concentrations of alkali. Phytochemistry 23, 1089–1093. doi: 10.1016/S0031-9422(00)82615-1
Chapman, H. D, Morris, V. J., Selvendran, R. R., and O'Neill, M. A. (1987). Static and dynamic light-scattering studies of pectic polysaccharides from the middle lamellae and primary cell walls of cider apples. Carbohyd. Res. 165, 53–68. doi: 10.1016/0008-6215(87)80077-0
Cornwell, W. K., Cornelissen, J. H., Amatangelo, K., Dorrepaal, E., Eviner, V. T., Godoy, O., et al. (2008). Plant species traits are the predominant control on litter decomposition rates within biomes worldwide. Ecol. Lett. 11, 1065–1071. doi: 10.1111/j.1461-0248.2008.01219.x
Corteselli, E. M., Burtis, J. C., Heinz, A. K., and Yavitt, J. B. (2017). Leaf litter fuels methanogenesis throughout decomposition in a forested peatland. Ecosystems 20, 1217–1232. doi: 10.1007/s10021-016-0105-9
Del Giudice, R., and Lindo, Z. (2017). Short-term leaching dynamics of three peatland plant species reveals how shifts in plant communities may affect decomposition processes. Geoderma 285, 110–116. doi: 10.1016/j.geoderma.2016.09.028
Dettling, M. D., Yavitt, J. B., Cadillo-Quiroz, H., Sun, C., and Zinder, S. H. (2007). Soil–methanogen interactions in two peatlands (bog, fen) in Central New York State. Geomicrobiol. J. 24, 247–259. doi: 10.1080/01490450701456651
Dorrepaal, E., Cornelissen, J. H., Aerts, R., Wallen, B. O., and Van Logtestijn, R. S. (2005). Are growth forms consistent predictors of leaf litter quality and decomposability across peatlands along a latitudinal gradient? J. Ecol. 93, 817–828. doi: 10.1111/j.1365-2745.2005.01024.x
Dubois, M., Gilles, K. A., Hamilton, J. K., Rebers, P. A., and Smith, F. (1956). Colorimetric method for determination of sugars and related substances. Anal. Chem. 28, 350–356. doi: 10.1021/ac60111a017
Emilson, E. J., Carson, M. A., Yakimovich, K. M., Osterholz, H., Dittmar, T., Gunn, J. M., et al. (2018). Climate-driven shifts in sediment chemistry enhance methane production in northern lakes. Nat. Commun. 9:1801. doi: 10.1038/s41467-018-04236-2
Fanin, N., Fromin, N., and Bertrand, I. (2016). Functional breadth and home-field advantage generate functional differences among soil microbial decomposers. Ecology 97, 1023–1037. doi: 10.1890/15-1263
Franchini, A. G., Henneberger, R., Aeppli, M., and Zeyer, J. (2015). Methane dynamics in an alpine fen: a field-based study on methanogenic and methanotrophic microbial communities. FEMS Microbiol. Ecol. 91:fiu032. doi: 10.1093/femsec/fiu032
Frolking, S., Roulet, N. T., Moore, T. R., Richard, P. J., Lavoie, M., and Muller, S. D. (2001). Modeling northern peatland decomposition and peat accumulation. Ecosystems 4, 479–498. doi: 10.1007/s10021-001-0105-1
Gholz, H. L., Wedin, D. A., Smitherman, S. M., Harmon, M. E., and Parton, W. J. (2000). Long-term dynamics of pine and hardwood litter in contrasting environments: toward a global model of decomposition. Glob. Change Biol. 6, 751–765. doi: 10.1046/j.1365-2486.2000.00349.x
Glaser, P. H., Siegel, D. I., Chanton, J. P., Reeve, A. S., Rosenberry, D. O., Corbett, J. E., et al. (2016). Climatic drivers for multidecadal shifts in solute transport and methane production zones within a large peat basin. Glob. Biogeochem. Cycles 30, 1578–1598. doi: 10.1002/2016GB005397
Godin, A., McLaughlin, J. W., Webster, K. L., Packalen, M., and Basiliko, N. (2012). Methane and methanogen community dynamics across a boreal peatland nutrient gradient. Soil Biol. Biochem. 48, 96–105. doi: 10.1016/j.soilbio.2012.01.018
Jung, H. G., and Engels, F. M. (2002). Alfalfa stem tissues. Crop Sci. 42, 524–534. doi: 10.2135/cropsci2002.5240
Keiser, A. D., Strickland, M. S., Fierer, N., and Bradford, M. A. (2011). The effect of resource history on the functioning of soil microbial communities is maintained across time. Biogeosciences 8, 1477–1486. doi: 10.5194/bg-8-1477-2011
Kotsyurbenko, O. R., Glagolev, M. V., Merkel, A. Y., Sabrekov, A. F., and Terentieva, I. E. (2019). “Methanogenesis in soils, wetlands, and peat,” in Biogenesis of Hydrocarbons, Handbook of Hydrocarbon and Lipid Microbiology, eds A. J. M. Stams and D. Z. Sousa (Cham: Springer), 1–18. doi: 10.1007/978-3-319-53114-4_9-1
Lang, S. I., Cornelissen, J. H., Klahn, T., Van Logtestijn, R. S., Broekman, R., Schweikert, W., et al. (2009). An experimental comparison of chemical traits and litter decomposition rates in a diverse range of subarctic bryophyte, lichen and vascular plant species. J. Ecol. 97, 886–900. doi: 10.1111/j.1365-2745.2009.01538.x
Lever, M. A., Heuer, V. B., Morono, Y., Masui, N., Schmidt, F., Alperin, M. J., et al. (2010). Acetogenesis in deep subseafloor sediments of the Juan de Fuca Ridge Flank: a synthesis of geochemical, thermodynamic, and gene-based evidence. Geomicrobiol. J. 27, 183–211. doi: 10.1080/01490450903456681
Machinet, G. E., Bertrand, I., Barrière, Y., Chabbert, B., and Recous, S. (2011). Impact of plant cell wall network on biodegradation in soil: role of lignin composition and phenolic acids in roots from 16 maize genotypes. Soil Biol. Biochem. 43, 1544–1552. doi: 10.1016/j.soilbio.2011.04.002
Makita, N., and Fujii, S. (2015). Tree species effects on microbial respiration from decomposing leaf and fine root litter. Soil Biol. Biochem. 88, 39–47. doi: 10.1016/j.soilbio.2015.05.005
McLaren, J. R., Buckeridge, K. M., van de Weg, M. J., Shaver, G. R., Schimel, J. P., and Gough, L. (2017). Shrub encroachment in Arctic tundra: Betula nana effects on above-and belowground litter decomposition. Ecology 98, 1361–1376. doi: 10.1002/ecy.1790
McLaughlin, J. W., and Webster, K. L. (2010). Alkalinity and acidity cycling and fluxes in an intermediate fen peatland in northern Ontario. Biogeochemistry 99, 143–155. doi: 10.1007/s10533-009-9398-5
McLeod, A. R., Newsham, K. K., and Fry, S. C. (2007). Elevated UV-B radiation modifies the extractability of carbohydrates from leaf litter of Quercus robur. Soil Biol. Biochem. 39, 116–126. doi: 10.1016/j.soilbio.2006.06.019
Melillo, J. M., Aber, J. D., and Muratore, J. F. (1982). Nitrogen and lignin control of hardwood leaf litter decomposition dynamics. Ecology 63, 621–626. doi: 10.2307/1936780
Min, K., Freeman, C., Kang, H., and Choi, S. U. (2015). The regulation by phenolic compounds of soil organic matter dynamics under a changing environment. Biomed Res. Int. 2015:825098. doi: 10.1155/2015/825098
Moore, T. R., Bubier, J. L., and Bledzki, L. (2007). Litter decomposition in temperate peatland ecosystems: the effect of substrate and site. Ecosystems 1, 949–963. doi: 10.1007/s10021-007-9064-5
Nakagawa, S., and Cuthill, I. C. (2007). Effect size, confidence interval and statistical significance: a practical guide for biologists. Biol. Rev. 82, 591–605. doi: 10.1111/j.1469-185X.2007.00027.x
Olson, J. S. (1963). Energy storage and the balance of producers and decomposers in ecological systems. Ecology 44, 322–331. doi: 10.2307/1932179
Pelloux, J., Rusterucci, C., and Mellerowicz, E. J. (2007). New insights into pectin methylesterase structure and function. Trends Plant Sci. 12, 267–277. doi: 10.1016/j.tplants.2007.04.001
Prescott, C. E. (2010). Litter decomposition: what controls it and how can we alter it to sequester more carbon in forest soils?. Biogeochemistry 101, 133–149. doi: 10.1007/s10533-010-9439-0
Preston, C. M., Nault, J. R., Trofymow, J. A., Smyth, C., and CIDET Working Group (2009). Chemical changes during 6 years of decomposition of 11 litters in some Canadian forest sites. Part 1. Elemental composition, tannins, phenolics, and proximate fractions. Ecosystems 12, 1053–1077. doi: 10.1007/s10021-009-9266-0
Reuter, H., Gensel, J., Elvert, M., and Zak, D. (2019). Preferential protein depolymerization as a preservation mechanism for vascular plant decomposing in Sphagnum peat. Biogeosciences 2019, 1–22. doi: 10.5194/bg-2019-176
Schädel, C., Blöchl, A., Richter, A., and Hoch, G. (2010). Quantification and monosaccharide composition of hemicelluloses from different plant functional types. Plant Physiol. Biochem. 48, 1–8. doi: 10.1016/j.plaphy.2009.09.008
Schwintzer, C. R. (1981). Vegetation and nutrient status of northern Michigan bogs and conifer swamps with a comparison to fens. Can. J. Bot. 59, 842–853. doi: 10.1139/b81-118
Schwintzer, C. R. (1984). Production, decomposition, and nitrogen dynamics of Myrica gale litter. Plant Soil 78, 245–258. doi: 10.1007/BF02277855
Siegel, D. I., and Glaser, P. H. (1987). Groundwater flow in a bog-fen complex, Lost River Peatland, northern Minnesota. J. Ecol. 75, 743–754. doi: 10.2307/2260203
Slack, N. G., Vitt, D. H., and Horton, D. G. (1980). Vegetation gradients of minerotrophically rich fens in western Alberta. Can. J. Bot. 58, 330–350. doi: 10.1139/b80-034
Smith, B. G., and Harris, P. J. (1999). The polysaccharide composition of Poales cell walls: Poaceae cell walls are not unique. Biochem. Syst. Ecol. 27, 33–53. doi: 10.1016/S0305-1978(98)00068-4
Soong, J. L., Parton, W. J., Calderon, F., Campbell, E. E., and Cotrufo, M. F. (2015). A new conceptual model on the fate and controls of fresh and pyrolized plant litter decomposition. Biogeochemistry 124, 27–44. doi: 10.1007/s10533-015-0079-2
Strack, M., Mwakanyamale, K., Fard, G. H., Bird, M., Bérubé, V., and Rochefort, L. (2017). Effect of plant functional type on methane dynamics in a restored minerotrophic peatland. Plant Soil 410, 231–246. doi: 10.1007/s11104-016-2999-6
Straková, P., Niemi, R. M., Freeman, C., Peltoniemi, K., Toberman, H., Heiskanen, I., et al. (2011). Litter type affects the activity of aerobic decomposers in a boreal peatland more than site nutrient and water table regimes. Biogeosciences 27, 2741–2755. doi: 10.5194/bg-8-2741-2011
Sun, C. L., Brauer, S. L., Cadillo Quiroz, H., Zinder, S. H., and Yavitt, J. B. (2012). Seasonal changes in methanogenesis and methanogenic community in three peatlands, New York State. Front. Microbiol. 3:81. doi: 10.3389/fmicb.2012.00081
Sundh, I., Nilsson, M., Granberg, G., and Svensson, B. H. (1994). Depth distribution of microbial production and oxidation of methane in northern boreal peatlands. Microb. Ecol. 27, 253–265. doi: 10.1007/BF00182409
Szumigalski, A. R., and Bayley, S. E. (1996). Decomposition along a bog to rich fen gradient in central Alberta, Canada. Can. J. Bot. 74, 573–581. doi: 10.1139/b96-073
Thormann, M. N. (2006). Diversity and function of fungi in peatlands: a carbon cycling perspective. Can. J. Soil Sci. 86, 281–293. doi: 10.4141/S05-082
Treat, C. C., Natali, S. M., Ernakovich, J., Iversen, C. M., Lupascu, M., McGuire, A. D., et al. (2015). A pan-Arctic synthesis of CH4 and CO2 production from anoxic soil incubations. Glob. Change Biol. 21, 2787–2803. doi: 10.1111/gcb.12875
Van Breemen, N. (1995). How Sphagnum bogs down other plants. Trends Ecol. Evol. 10, 270–275. doi: 10.1016/0169-5347(95)90007-1
Waksman, S. A., and Reuszer, H. W. (1932). On the origin of the uronic acids in the humus of soil, peat, and composts. Soil Sci. 33:135. doi: 10.1097/00010694-193202000-00005
Ward, S. E., Orwin, K. H., Ostle, N. J., Briones, M. J., Thomson, B. C., Griffiths, R. I., et al. (2015). Vegetation exerts a greater control on litter decomposition than climate warming in peatlands. Ecology 96, 113–123. doi: 10.1890/14-0292.1
Wardle, D. A. (1993). Changes in the microbial biomass and metabolic quotient during leaf litter succession in some New Zealand forest and scrubland ecosystems. Funct. Ecol. 7, 346–355. doi: 10.2307/2390215
Webster, K. L., and McLaughlin, J. W. (2010). Importance of the water table in controlling dissolved carbon along a fen nutrient gradient. Soil Sci. Soc. Am. J. 74, 2254–2266. doi: 10.2136/sssaj2009.0111
Wickings, K., Grandy, A. S., Reed, S. C., and Cleveland, C. C. (2012). The origin of litter chemical complexity during decomposition. Ecol. Lett. 15, 1180–1188. doi: 10.1111/j.1461-0248.2012.01837.x
Williams, C. J., and Yavitt, J. B. (2003). Botanical composition of peat and degree of peat decomposition in three temperate peatlands. Ecoscience 10, 85–95. doi: 10.1080/11956860.2003.11682755
Witkamp, M. (1966). Decomposition of leaf litter in relation to environment, microflora, and microbial respiration. Ecology 47, 194–201. doi: 10.2307/1933765
Yavitt, J. B., Burtis, J. C., Smemo, K. A., and Welsch, M. (2018). Plot-scale spatial variability of methane, respiration, and net nitrogen mineralization in muck-soil wetlands across a land use gradient. Geoderma 315, 11–19. doi: 10.1016/j.geoderma.2017.11.038
Yavitt, J. B., and Williams, C. J. (2015). Conifer litter identity regulates anaerobic microbial activity in wetland soils via variation in leaf litter chemical composition. Geoderma 243, 141–148. doi: 10.1016/j.geoderma.2014.12.023
Keywords: anaerobic respiration, leaf litter decomposition, leaf litter quality, New York State, soil methane production
Citation: Yavitt JB, Kryczka AK, Huber ME, Pipes GT and Rodriguez AM (2019) Inferring Methane Production by Decomposing Tree, Shrub, and Grass Leaf Litter in Bog and Rich Fen Peatlands. Front. Environ. Sci. 7:182. doi: 10.3389/fenvs.2019.00182
Received: 19 June 2019; Accepted: 31 October 2019;
Published: 19 November 2019.
Edited by:
Colin McCarter, University of Toronto Scarborough, CanadaCopyright © 2019 Yavitt, Kryczka, Huber, Pipes and Rodriguez. This is an open-access article distributed under the terms of the Creative Commons Attribution License (CC BY). The use, distribution or reproduction in other forums is permitted, provided the original author(s) and the copyright owner(s) are credited and that the original publication in this journal is cited, in accordance with accepted academic practice. No use, distribution or reproduction is permitted which does not comply with these terms.
*Correspondence: Joseph B. Yavitt, amJ5MUBjb3JuZWxsLmVkdQ==