- 1Population Health and Reproduction Department, School of Veterinary Medicine, University of California, Davis, Davis, CA, United States
- 2California Department of Parks and Recreation, Aquatic Invasive Species Branch, Division of Boating and Waterways, Sacramento, CA, United States
- 3Environmental Water Quality and Estuarine Studies, California Department of Water Resources, Sacramento, CA, United States
- 4Aquatic Health Program, Department of Anatomy, Physiology, and Cell Biology, School of Veterinary Medicine, University of California, Davis, Davis, CA, United States
- 5Division of Environmental Services, California Department of Water Resources, Sacramento, CA, United States
- 6Aquatic Ecology Section, California Department of Water Resources, Sacramento, CA, United States
Purpose: In recreational water bodies, herbicides are widely used for controlling unwanted weeds, and impacts of herbicide residues on health risks to aquatic ecosystem is a serious concern. This study was aimed to improve the existing understanding of the deposition of herbicides from water column to bed sediment and leachate of herbicides from bed sediment to water column. We investigated the attachment of two herbicides with sediment and release from sediment: (1) Glyphosate; and (2) Fluridone. The goal of this study was to determine the deposition and release of Glyphosate and Fluridone in bed sediment of the Sacramento–San Joaquin River Delta.
Materials and Methods: Field sampling was performed to collect water and sediment samples from Sacramento–San Joaquin River Delta. Bottom dredge sampler was used for collecting sediment samples and horizontal water bottle sampler was used for collecting water samples. A series of experiments were conducted to determine the attachment and release of Fluridone and Glyphosate from sediment at a different level of initial concentrations. For analyzing Fluridone and Glyphosate in sediment leachate and water, samples were processed using enzyme-linked immunosorbent assay (ELISA) based method.
Results and Discussion: Observations showed that proportions of Glyphosate concentrations in water were higher than Fluridone concentrations in water, when both herbicides were inoculated in water in same quantity. On the contrary, the concentrations of Fluridone in sediment-bound leachate were higher than Glyphosate concentrations in sediment-bound leachate, regardless of the initial concentrations. Fluridone and Glyphosate concentrations in water column samples differed significantly (p < 0.05) over the time even initial concentrations of these herbicides were kept similar, which indicates that Fluridone interaction with water column was considerably different than the interaction of Glyphosate with the water column.
Conclusions: Bed sediment can be an important sink and source for release of Fluridone and Glyphosate from bed sediment to the water column of an ambient water body. Significant concentrations of herbicides were deposited in bed sediment of Sacramento-San Joaquin Delta, and eventually the high concentrations of herbicides were observed in sediment leachate. Improved understanding of this important release pathway can provide much needed information to adequately address the impacts of particle attached herbicides on aquatic and ecological environment of a water body.
Introduction
The uses of herbicides for controlling unwanted plants in land and water have increased enormously over the past few decades in both developing and developed countries (Wang et al., 2012; Stone et al., 2014; Souza et al., 2017). However, in order to understand the resultant risks to environment and population health requires improvement in existing knowledge with regards to the fate of herbicides residues in ambient water bodies. The ability of these compounds to accumulate in the environment and their toxicity to aquatic lives, humans, and wildlife is a concern (Amweg et al., 2006; Parsons et al., 2009; Buah-Kwofie and Humphries, 2017; Gama et al., 2017; Morales et al., 2017). As an example, a 20 year dataset (1992–2011) showed that more than a billion pounds of herbicides were used annually between 1992 and 2011 in the United States of America (USA), and the levels of herbicides exceeded aquatic-life benchmarks in multiple streams in the USA, which drains agricultural and urban land (Stone et al., 2014).
Similarly, an industrialized agricultural region of the state of Sinaloa in Mexico reported pesticide usage of 700 t/year (including 17 herbicides with moderately to highly toxicity) (Arellano-Aguilar et al., 2017). The results showed that many organochlorines and organophosphorus herbicides were present in water samples in sites related with agriculture, and seawater from the Gulf of California (Arellano-Aguilar et al., 2017).
Over the past few decades, a great deal of research has focused on understanding the negative effects of endocrine active compounds on fish production (Kidd et al., 2007; Buah-Kwofie and Humphries, 2017; Scott et al., 2017). As an example, the findings of feminized male western mosquitofish (Gambusia holbrooki) downstream of a wastewater treatment plant (WWTP) in Australia, which showed the evidence of endocrine disruption, received wide-scale attention around the world (Batty and Lim, 1999; Scott et al., 2017). Results showed that the growth and development of the modified anal fin (the gonopodium) was reduced in males in streams receiving the water from WWTP, suggesting the presence of endocrine disruptors in the water (Batty and Lim, 1999). The levels of organochlorine herbicides (OCP) in fish such as Tigerfish (Hydrocynus vittatus) collected from Kruger National Park (South Africa) sections of Luvuvhu, Olifants, and Letaba rivers showed more than 15 herbicides in fish muscle tissues, and the concentrations of the majority of the OCPs were considerably higher than aquatic-life benchmarks (Gerber et al., 2016).
The occurrences of synthetic organic herbicides, insecticides, and fungicides in streams and rivers, used for agriculture in the USA, pose widespread concerns for aquatic life based on the benchmark exceedances (Stone et al., 2014). The adverse impacts of storm water runoff, and urban and agricultural herbicides on threatened Delta Smelt (Hypomesus transpacificus) and larvae of Sacramento splittail (Pogonichthys macrolepidotus) is reported (Teh et al., 2005; Weston et al., 2014). The increased levels of herbicides in ambient water bodies including OCPs, which were banned decades ago, and the accumulation of herbicides in predator and benthic fish species poses a potential health risks to human consumers and fish predators (Abrantes et al., 2010; Net et al., 2015; Gama et al., 2017).
While elevated levels of herbicides in the water column of an ambient water body (i.e., streams, rivers, lakes, delta, estuaries) is a concern, the presence of herbicides in low lying bed sediment is a particular concern because many fish (and other aquatic organisms) lay eggs in the sandy and sediment environment (Milne, 1974; Hebert et al., 2013; Henry et al., 2016; Bauer et al., 2017). Thus, increased pesticide concentrations in bed sediment adversely affect the habitat and breeding grounds (Batty and Lim, 1999; Wepener et al., 2012; Cayla, 2014).
In general, it is well established that many herbicides and derivatives can persist in the environment, including water bodies for decades, however the fate and dynamics of movement of particle-attached herbicides in water bodies remain unclear (Wepener et al., 2012; Cayla, 2014). The increased load of herbicides in bed sediment is a serious concern, which poses the risk of environmental contamination with legacy. As an example, many herbicides including OCPs and new contaminants (pharmaceuticals) were found in the Salton Sea, California, USA, and sediments of urban rivers in Florida and California, USA (Amweg et al., 2006; Wang et al., 2012; Yang et al., 2015). A study showed that 12 out of 15 urban creeks in California were toxic, and sediment pyrethroid pesticide (a replacement for organophosphates for many urban applications) concentrations were sufficient to cause toxicity in amphipod, Hyalella azteca (Amweg et al., 2006). Understanding the pesticide presence and its degradation in sediment environment can provide improved information with regards to the ecological health of a waterbody (Pesce et al., 2013).
The goal of this study was to assess the Glyphosate and Fluridone attachment to sediment, and understand the release of herbicides from sediment through leachate to the water column. The specific objectives were to: (1) assess the attached concentrations of two herbicides (Fluridone and Glyphosate) in sediment; (2) compare the concentrations of Fluridone and Glyphosate in the water column and sediment; and (3) evaluate the effects of sediment leachate/washing on the particle-attached Fluridone and Glyphosate concentrations. Fluridone and Glyphosate are widely used herbicides for controlling unwanted aquatic plants and phytoplankton in ambient water, and the presence of these herbicides in water and sediment environment has a potential to affect the habitat of aquatic lives (Fox et al., 1994; Parsons et al., 2009; Antunes et al., 2010; Crowe et al., 2011; Scott et al., 2017; Souza et al., 2017).
Materials and Methods
Sediment Sample Collection, Characterization, and Standard Addition
To conduct an herbicide attachment study, three different set of sediment samples were collected and homogenized from Liberty Island of the Sacramento-San Joaquin Delta, California, USA. A shallow water bottom dredge sampler (15 × 15 cm opening, Forestry Suppliers Inc., Mississippi, U.S.) was used for collecting bed sediment samples (≈ 2 kg). Approximately, 500–800 g of sediment was dried overnight at 70°C to remove the moisture. Subsequently, 20 g of dried sediment sample was mixed with 20 mL of Glyphosate and Fluridone standards. The 5 levels of standards [0 ppb (control); 100 ppb; 500 ppb; 1,000 ppb; and 5,000 ppb] were prepared immediately before starting the experiments. The physio-chemical characteristics of sediment were determined using standard methods. Organic matter was determined using Loss on Ignition Method. Cation exchange capacity of sediment was determined using barium-calcium exchange method. Particle size was analyzed using the hydrometer method. The details of methods applied for physio-chemical analysis are listed elsewhere (UC Davis Analytical Laboratory, 2017).
Herbicides Standard Preparation
To prepare the solutions of standards, Fluridone [(CAS Number 59756-60-4; C19H14F3NO; Molecular Weight 329.32)] Pestanal Standard was purchased from Sigma-Aldrich, USA. Glyphosate analytical standard [CAS Number: 1071-83-6; (HO)2P(O)CH2NHCH2CO2H; Molecular Weight 169.07)] was also purchased from Sigma-Aldrich, USA. Least detectable dose (LDD) of Fluridone was 0.15 ppb, and LDD of Glyphosate was 0.05 ppb. For both herbicides (Fluridone, and Glyphosate), the 5 levels of standards (0 ppb; 100 ppb; 500 ppb; 1,000 ppb; and 5,000 ppb) were prepared using Milli-Q Water.
Experiment Setup
To assess the herbicide attachment with sediment, two sets of experiments were conducted. The first set of experiments was conducted with 20 mL of herbicide standard mixed with 20 g of sediment. Three replicates of each standard were used for the study. The second set of experiments was conducted on water only (40 mL of standard without sediment) with three replicates of each standard. Each experiment was conducted in Corning® 50 mL PP centrifuge tubes (CLS430897 Sigma), Sigma-Aldrich, USA. During the first stage of experiment (termed as the First Wash), the mixture (sediment with herbicide) was prepared, and after vortexing the mixture in corning tubes for 2 min, the mixture in tube was kept in room temperature (22°C) for an hour. After an hour, the mixture was centrifuged at 5,000 rpm for 5 min, and supernatant was collected for analysis.
During the second stage of experiment one (termed as the Second Wash), fresh 20 mL of Milli-Q Water was added into Corning Tube with previously washed sediment (gone through the first wash), and the corning tube was kept in room temperature for one more hour. To simulate ambient water body conditions, instead of using solvents, aqueous media was used for washing the sediment. After an hour, the mixture was centrifuged at 5,000 rpm for 5 min, and the 20 mL of supernatant was collected for the analysis. During the third stage (termed as the Third Wash), fresh 20 mL of Milli-Q Water was added into Corning Tubes with sediment (gone through previous two washes), and the samples were placed in room temperature for 2 h in order to allow sufficient settling time for depositing particle bound herbicides. Subsequently, supernatant was collected, and analyzed for herbicides.
In the second set of experiments, three Corning Tubes for each level of herbicide standards were filled with 20 mL of herbicide standards and 20 mL of DI water. The both type of experiments (with and without sediments) were ran together, and similar process (centrifugation) and ambient conditions (temperature) were applied during the experiments and sample analysis. These water experiments were also subjected to washing and dilutions. During 1, 2, and 4 h washes, water samples were centrifuged at 5,000 rpm for 5 min, and 20 mL supernatant was taken out of Corning Tubes for the analysis. The fresh 20 mL of Milli-Q Water was added into the Corning Tubes (before the next stage of experiment) for equaling the volume (40 mL) used for sediment experiments. The changes in herbicide levels in these two sets of experiment (with sediment and without sediment) were used to assess the herbicide attachment and release at ambient temperature condition (≈22°C). All the experiments were triplicated.
Sample Analysis for Fluridone
For analyzing Fluridone concentrations in sediment and water sample, the water and leachate samples were processed using enzyme-linked immunosorbent assay (ELISA) based method. The ELISA kits for Fluridone (PN 500511, Magnetic Particle, 100 T), and Glyphosate (PN 500081, Magnetic Particle ELISA, 120T) were obtained from Abraxis Inc, Warminster, PA. The Abraxis Fluridone Kit uses the principles of ELISA to determine Fluridone in samples. The method is described in detail elsewhere (Fluridone, 2017) [PN 500511, Abraxis]. In brief, the sample to be tested was added, along with an enzyme conjugate, to a disposable test tube, followed by paramagnetic particles attached with antibodies specific to Fluridone.
A reaction (competitive) occurred between the Fluridone (which is in the sample) and the enzyme labeled Fluridone analog. This reaction prolonged for 20 min. At the end of the reaction, a magnetic field is used. The magnetic field allows the unbound reagents to be decanted. Subsequently, washing solution is used to wash particles. In order to detect Fluridone in the samples, a color solution is added. The color solution consists of enzyme substrate (hydrogen peroxide) and the chromogen (3,3′, 5,5′-tetramethylbenzidine). The conversion of substrate/chromogen is catalyzed by the enzyme-labeled Fluridone bound to the Fluridone antibody. After incubation, the reaction was stopped and stabilized by adding a diluted acid (stopping solution). Since the labeled Fluridone (conjugate) was in competition with the unlabeled Fluridone (sample) for the antibody sites, the color developed is inversely proportional to the concentration of Fluridone in the sample needs to be tested. The sample color was read at 450 nm within 15 min after adding the stopping solution.
Sample Analysis for Glyphosate
Similar to the Fluridone analysis, the Glyphosate ELISA Kit applies the principles of ELISA to the determine Glyphosate in samples. The details of method are available elsewhere (Glyphosate, 2017) [PN 500081, Abraxis]. The samples, which require testing were derivatized, and added with paramagnetic particles attached with antibodies. Subsequently, the Glyphosate enzyme conjugate was added. A competitive reaction occurred between the Glyphosate in the sample and the enzyme labeled Glyphosate analog. The reaction prolonged for thirty min. At the end of the incubation period, a magnetic field is applied to hold in the test tube para-magnetic particles (with Glyphosate and labeled Glyphosate bound to the antibodies). This allowed the unbound reagents to be decanted. After decanting, washing solution was used for washing the particles. The color solution was added to detect the presence of Glyphosate in the samples. The color solution consists of the enzyme substrate (hydrogen peroxide) and the chromogen (3,3′, 5,5′-tetramethylbenzidine). Subsequently, the conversion of the substrate/ chromogen mixture to a colored product was catalyzed by the enzyme-labeled Glyphosate. After incubation, the reaction is stopped and stabilized by adding a diluted acid (stopping solution). Since the labeled Glyphosate (conjugate) was in competition with the unlabeled Glyphosate (of sample) for the antibody sites, the color developed was inversely proportional to the concentration of Glyphosate in the samples to be tested. The color of samples was read at 450 nm within 15 min after adding the stopping solution.
Results and Discussion
Changes in Fluridone concentrations in water are shown in Figure 1. Variations in Glyphosate concentrations are shown in Figure 2. Table 1 showed the result of one-way ANOVA with Tukey's pairwise comparison for Fluridone and Glyphosate in sediment and water.
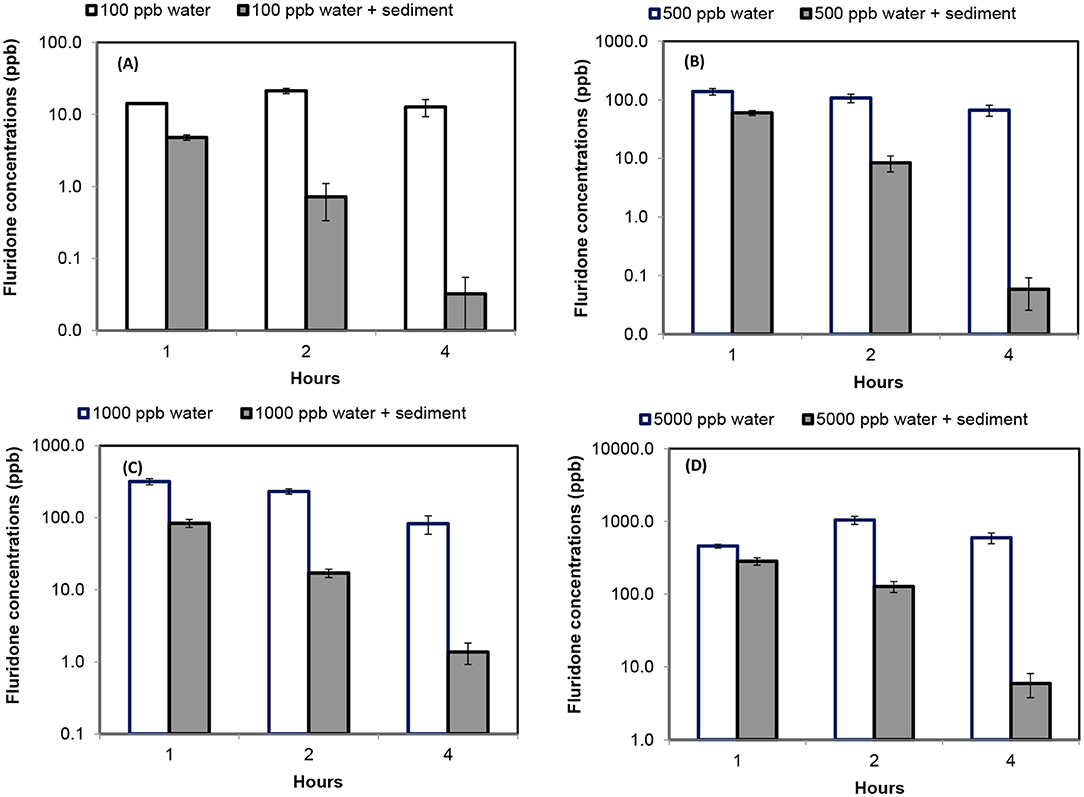
Figure 1. Fluridone concentration determined in water and sediment feedstock after consecutive washes [error bars indicate standard deviation of 3 replicates]. (A) Determination of Fluridone concentrations in water and sediment environment at initial concentrations of 100 ppb; (B) Determination of Fluridone concentrations in water and sediment environment at initial concentrations of 500 ppb; (C) Determination of Fluridone concentrations in water and sediment environment at initial concentrations of 1,000 ppb; and (D) Determination of Fluridone concentrations in water and sediment environment at initial concentrations of 5,000 ppb.
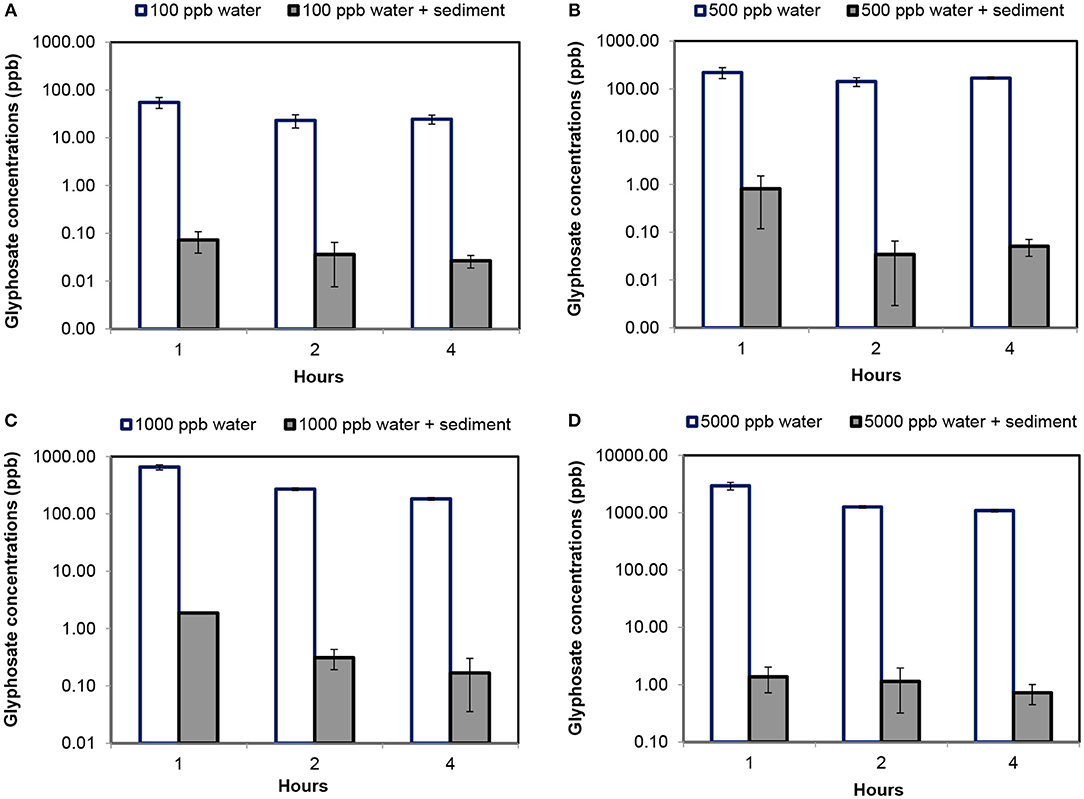
Figure 2. Glyphosate concentrations determined in water and sediment feedstock after consecutive washes. (A) Determination of Glyphosate concentrations in water and sediment environment at initial concentrations of 100 ppb; (B) Determination of Glyphosate concentrations in water and sediment environment at initial concentrations of 500 ppb; (C) Determination of Glyphosate concentrations in water and sediment environment at initial concentrations of 1,000 ppb; and (D) Determination of Glyphosate concentrations in water and sediment environment at initial concentrations of 5,000 ppb [error bars indicate standard deviation of 3 replicates].
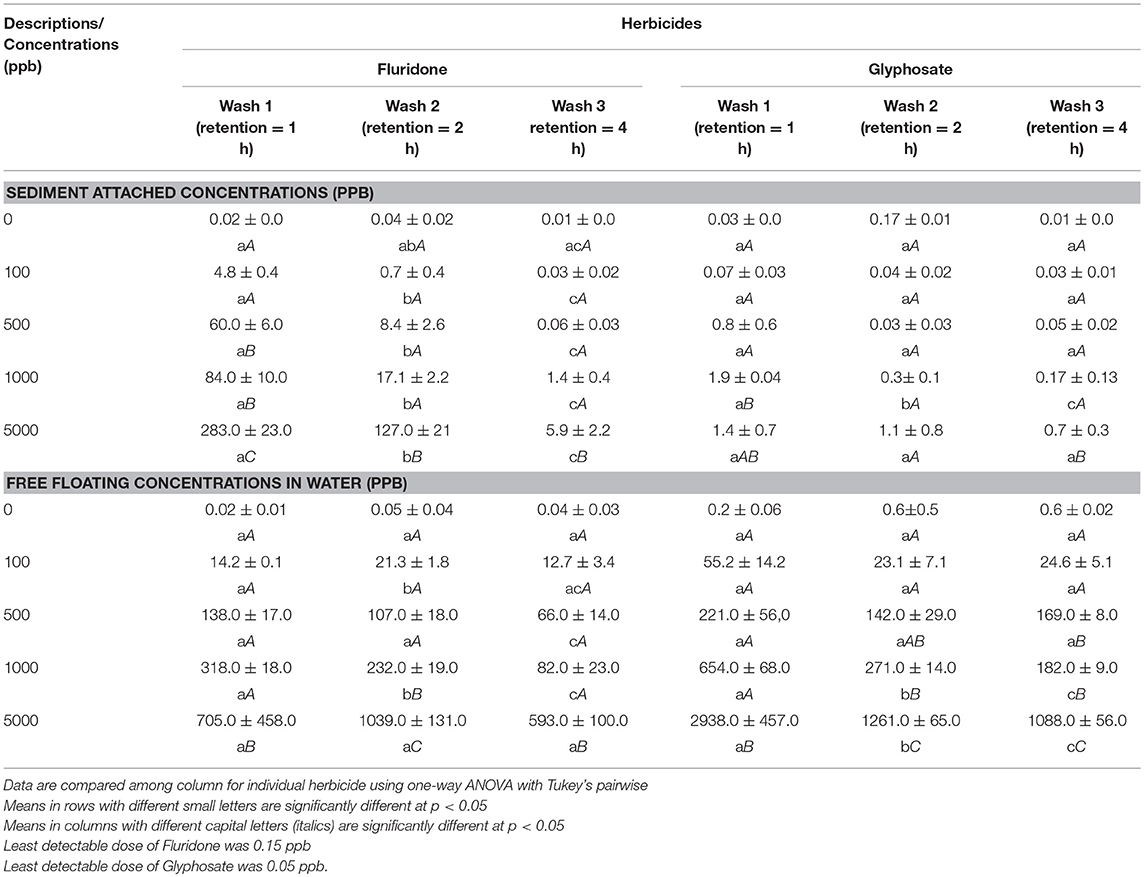
Table 1. Determination of Fluridone and Glyphosate concentrations (ppb) in sediment and water after consecutive washes.
Fluridone concentrations in water samples differed significantly (p < 0.05) among Wash 1, Wash 2, and Wash 3. The effect of wash was significantly different among various initial Fluridone concentrations (Table 1). When initial Fluridone concentration in water was 100 ppb, the first and third wash resulted in Fluridone concentrations of 14.16 ppb and 12.72 ppb, respectively. At initial Fluridone concentration of 5,000 ppb, the first and third wash resulted in a final concentration of 705.59 ppb and 593.35 ppb, respectively.
Similar to Fluridone, Glyphosate concentrations in water changed significantly (p < 0.05) as a result of Wash. Further, the effect of wash was significantly different (p < 0.05) among various levels of initial Glyphosate concentrations. Compared to Fluridone, the concentrations of Glyphosate in water were higher after the first wash. Even after the third wash (i.e., Wash 3), the concentration of Glyphosate in water was higher than Fluridone for each initial level.
The effects of wash on Fluridone concentrations in sediment are shown in Figure 1. The results of sediment characteristics analysis showed sediment was consisting of 4.6% (±0.7%) organic matter. Organic carbon was 2.7% (±0.4%). Sand, silt, and clay content was 13.7% (± 8.3%), 51.7% (± 0.6%), 34.7% (± 8.5%), respectively. Cation exchange capacity of sediment was 38.6% (± 6.1%).
Fluridone concentrations among washes were significant different (p < 0.05). The effect of wash was significantly different (p < 0.05) among various levels of initial Fluridone (0–5,000 ppb) concentrations in sediment (Table 1). Fluridone concentration in sediment was reduced during each consecutive wash. As an example, when initial concentration of Fluridone in sediment was 100 ppb, the first wash resulted in a concentration of only 4.79 ppb, while the third wash resulted in a concentration of 0.03 ppb. When initial concentration of Fluridone in sediment was 5,000 ppb, the first wash resulted in a concentration of only 283.26 ppb, and the third wash resulted in a concentration of 5.94 ppb.
The effects of washing on Glyphosate concentrations of sediment leachate and water are shown in Figure 2. Compared to Fluridone, the concentrations of Glyphosate in sediment leachate were considerably low. When initial concentration of Glyphosate in feedstock was 100 ppb, the first wash resulted in a reduction of only 0.07 ppb in sediment, while the third wash resulted in a reduction of 0.03 ppb. At initial Glyphosate concentrations of 500 ppb, the first wash resulted in a reduction of glyphosate determination of 0.81 ppb, and the third wash resulted in decrease of 0.05 ppb. Even when initial Glyphosate concentration was increased to 1,000 and 5,000 ppb, the first wash of sediment resulted in a final Glyphosate concentration of 83.93 ppb and 283.26 ppb, respectively. The third wash of these sediment resulted in Glyphosate concentrations of 0.17 ppb and 0.73 ppb, respectively.
A comparative analysis between Fluridone and Glyphosate determination (shown in Figure 3) indicates that the concentrations of Glyphosate in water were higher than that observed for Fluridone during each wash. At initial concentrations of 100 ppb (each herbicide), the first wash of water yielded Glyphosate in water higher than Fluridone (Figure 3A). At initial level of 100 ppb, the Glyphosate in water was 289% greater than that of Fluridone. This pattern was similar for all treatments and washes. Fluridone concentrations changed significantly during each wash. Even during the third wash, the Glyphosate concentration was 93% greater than that of Fluridone (Figure 3).
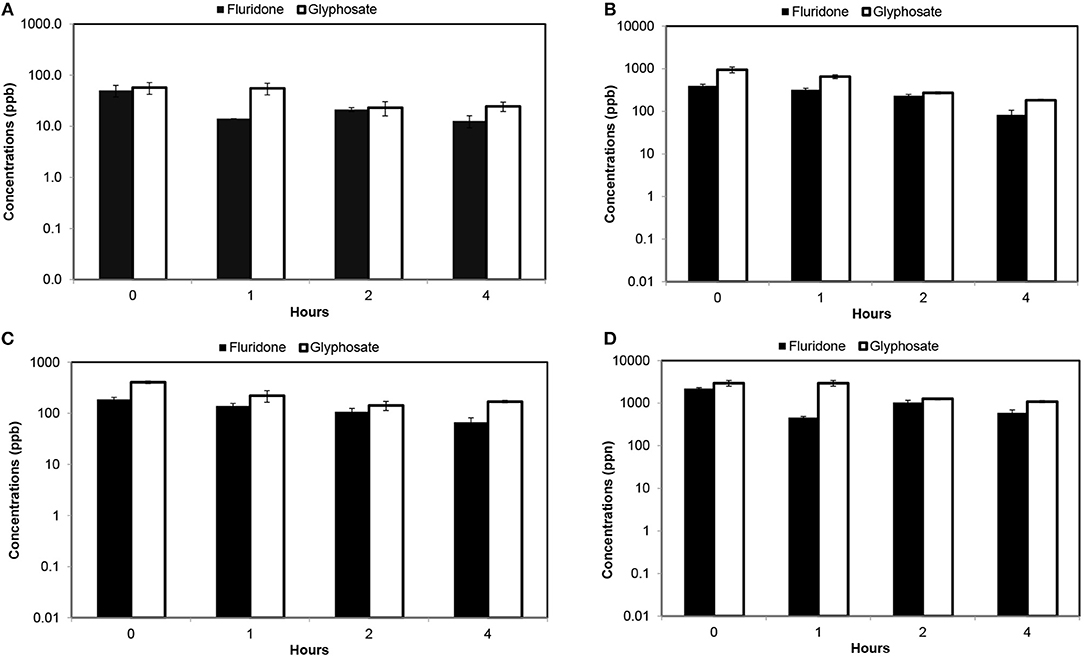
Figure 3. Fluridone and Glyphosate concentrations determined in water after consecutive washes [error bars indicate standard deviation of 3 replicates]. (A) Determination of Fluridone and Glyphosate at initial concentrations of 100 ppb; (B) Determination of Fluridone and Glyphosate at initial concentrations of 1,000 ppb; (C) Determination of Fluridone and Glyphosate at initial concentrations of 500 ppb; and (D) Determination of Fluridone and Glyphosate at initial concentrations of 5,000 ppb.
At 5,000 ppb initial concentration of each, Glyphosate in water was 541% (after first wash) and 83% higher than Fluridone (after third wash) (Figure 3D). The effects of wash were significant (p < 0.05) on both Fluridone and Glyphosate in water at 5,000 ppb initial level (Table 1).
In contrast to water column, Glyphosate concentrations were lower than Fluridone in sediment leachate (Table 1). At each level of initial concentrations, the sediment leachate showed relatively higher level of Fluridone compared to Glyphosate during the first wash. This fact signifies that, Fluridone can have prolonged impacts on sediment and water interphase. Fluridone was released easily from sediment to the water column during the first wash. When initial concentrations of herbicides were 500 ppb, the Fluridone concentration in leachate was 73.74 times higher than the Glyphosate concentrations (first wash). During the third wash, Fluridone concentration was 14.92% higher than that of Glyphosate concentrations in leachate (Figure 4C). At higher initial concentrations (1,000 ppb), the first wash of sediment yielded Fluridone concentration in leachate 45.10 times higher than that of Glyphosate. The third wash resulted Fluridone concentrations 8 times higher than Glyphosate concentrations (Figure 4B). When initial concentration was 5,000 ppb, the first wash of sediment resulted in Fluridone concentrations in leachate of 283.26 ppb, while leachate Glyphosate concentration was only 1.37 ppb. During the third wash, leachate Fluridone concentration was 717% higher than Glyphosate concentrations (Figure 4D).
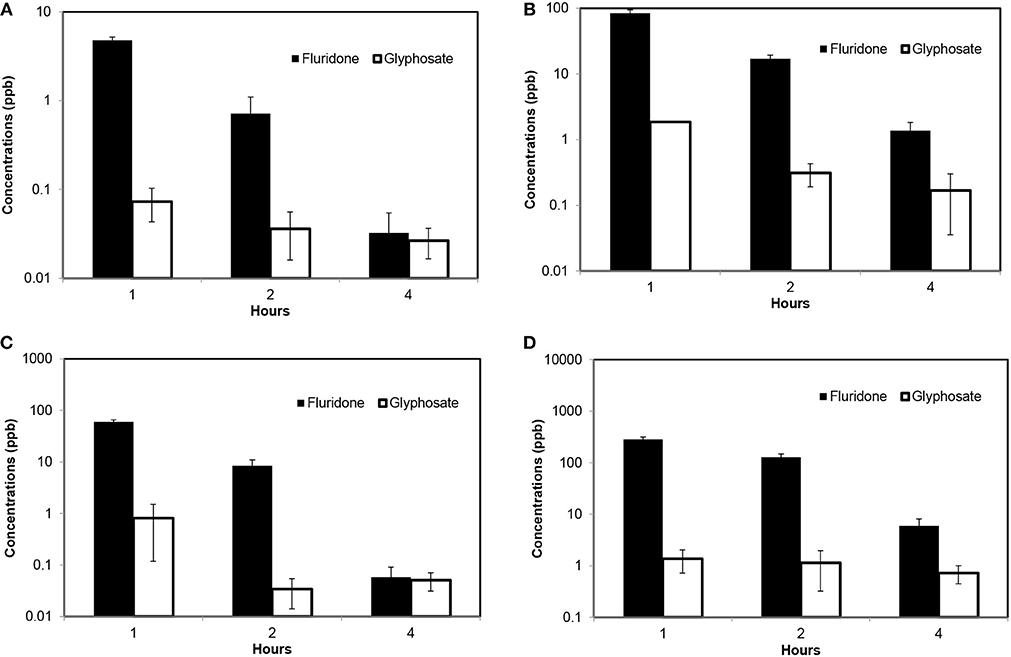
Figure 4. Determination of sediment attached Fluridone and Glyphosate concentrations in leachate after consecutive washes [error bars indicate standard deviation of 3 replicates]. (A) Determination of Fluridone and Glyphosate at initial concentrations of 100 ppb; (B) Determination of Fluridone and Glyphosate at initial concentrations of 1,000 ppb; (C) Determination of Fluridone and Glyphosate at initial concentrations of 500 ppb; and (D) Determination of Fluridone and Glyphosate at initial concentrations of 5,000 ppb.
Studied scenarios of Fluridone and Glyphosate showed that these two herbicides interact differently with the sediment. The first wash of sediment resulted considerably higher amount of Fluridone than Glyphosate in leachate. These results are relevant to understand the effectiveness of various herbicides, when applied in an ambient water body for controlling aquatic weeds such as Hydrilla sp. A previous study suggest that the growth of Hydrilla sp can be restricted, when it is exposed for 8–12 weeks with Fluridone concentrations of 10–15 ppb (Andrew et al., 2003). Fluridone concentrations of 60–90 ppb in ponds and 10–20 ppb for whole lake is recommended doses for controlling the hydrilla (Andrew et al., 2003). Previous research showed that when Fluridone was applied over a period of 13 weeks (at a theoretical concentration of 10–12 ppb) to several lakes in central Florida for controlling hydrilla, actual Fluridone concentrations in water bodies was much lower than the theoretical concentrations (Fox et al., 1994). A potential reason for low Fluridone concentration in water could be a result of sediment and water interaction (i.e., deposition of particle bound Fluridone in bed sediment). The deposition of particle bound Fluridone can reduce Fluridone concentrations in water column. As shown in this study, these particles bound Fluridone has a potential to get resuspended over the time from bed sediment to the water column. The effect of sediment leachate can be significant on water borne herbicide concentrations. Regardless of initial concentrations, substantial amount of Fluridone was released from sediment (Figure 4) to water during Wash 1- Wash 3. This may have impacts on the rate of Fluridone dissipation from the water and sediment, and also on the half-life of Fluridone in water, which is suggested to be 21–26 days (West and Parka, 1981). However, another study suggested Fluridone half-life of 97 days (Fox et al., 1996).
Although it is reported that hydrilla is susceptible to even low concentrations of Fluridone (Fox et al., 1994, 1996; Andrew et al., 2003). In addition to hydrilla control, Fluridone and Glyphosate are used for controlling a range of fresh water species of phytoplankton including Scenedesmus acutus, Scenedesmus subspicatus, Chlorella vulgaris, and Chlorella saccharophila. As an example, Glyphosate concentration of 24.5–41.7 mg/L resulted in a 50% growth reduction of phytoplankton over 72 h, while 1.6–3.0 mg/L resulted in a 10% growth reduction (Vendrell et al., 2009). Further, the effect of Glyphosate was found to be species specific (Vendrell et al., 2009). Fluridone is used for controlling flowering rush (Butomus umbellatus L.), an emerging aquatic weed, and a dose of 30 μg/L was found to be effective in reducing the growth of flowering rush (Madsen et al., 2016). The impacts of deposition and resuspension of particle attached herbicides on reductions of aquatic weeds are yet to be determined. The attachment of Fluridone with sediment particles may influence its effectiveness in controlling aquatic plants and macrophytes (Jacob et al., 2016). Subsequent deposition of particle-attached Fluridone into bed sediment has also a potential to reduce the expected Fluridone concentrations in the water column. The resuspension and disturbance of streambed sediment can release particle-attached Fluridone back into the water column. Although many eradication programs rely on Fluridone to control hydrilla, the uncertain response of Fluridone in terms of effective concentrations and exposure is not uncommon (Netherland, 2015), which may be attributed to the less understood phenomenon of attachment, deposition, and resuspension. The deposition of particle-attached Fluridone in bed sediment may also have consequential impacts on aquatic lives coming into contact with the bed sediment.
Glyphosate is an intensively used herbicide, and increased levels of Glyphosate for extended periods may pose risks to environmental health (Hanke et al., 2008; Vendrell et al., 2009; Antunes et al., 2010; Daouk et al., 2013). It is reported that Glyphosate has both positive and negative influences on phytoplankton communities (i.e., it can serve as a nutrient source to microbes able to tolerate the herbicidal effects of the compound, and also kills those less tolerant) (Saxton et al., 2011). In many countries, Glyphosate is widely used in the agricultural lands located in the drainage basins for curbing grass growth (Abrantes et al., 2010; Cayla, 2014). Results of this study showed that the concentrations of Glyphosate in sediment leachate were relatively lower than Fluridone concentrations in leachate regardless of initial concentrations (Figure 4). The high affinity and miscibility of Glyphosate to water may result in a relatively quicker dissemination and transport of the Glyphosate and its metabolites into surface and ground water. Previous studies showed elevated Glyphosate in ground water (Abrantes et al., 2010; Crowe et al., 2011). Studies have shown that Glyphosate can easily leach from ground surface to the water column (Vereecken, 2005; Crowe et al., 2011). Agricultural subsurface drains and preferential flow potentially increases Glyphosate concentrations in surface and subsurface waters (Stone and Wilson, 2006; Borggaard and Gimsing, 2008).
Considering the wide application of Fluridone and Glyphosate as herbicides for controlling aquatic weeds in water bodies and grasses in agricultural land, it is important to improve our existing understanding how the transport and effectiveness of these herbicides are influenced under particle-attached conditions. In scenarios, when herbicides are applied into water bodies to control aquatic weeds, a substantial amount of these compounds are attached to sediment particles and deposited into bed sediment. The impacts of deposition and resuspension of herbicides on environment water bodies, aquatic lives, and plants are not fully understood. The results of the preliminary study presented here show that concentrations of herbicides in water column may be influenced by their affinity to water and sediment particles. Substantial amount of herbicides can be deposited or attached with sediment. Further, leachate of sediment can be a source of herbicides, which can increase the concentrations of herbicides in the water column. The release and deposition of herbicides in ambient waterbodies, and quantitative effects of leachate on water column herbicide levels is not yet understood very well. Future studies for gaining additional insights in terms of herbicides affinity to particles, deposition, and resuspension, and consequential impacts on the health of water bodies are needed.
Conclusions
We determined deposition and release of Fluridone and Glyphosate concentrations in aquatic environment by conducting a series of lab-scale experiment, which involved monitoring Fluridone and Glyphosate in water column, and sediment leachate. The study used bed sediment and water collected from multiple locations of Sacramento-San Joaquin Delta. The impacts of changes in initial concentrations of Fluridone and Glyphosate on the concentrations of these herbicides in water and sediment leachate were determined. Results showed that the regardless of initial concentrations, over the time Glyphosate concentrations in water were significantly higher than Fluridone concentrations in water (p < 0.05). The concentrations of Fluridone were higher in sediment leachate than Glyphosate concentrations in sediment leachate (p < 0.05). Fluridone and Glyphosate concentrations in water and sediment leachate changed significantly (p < 0.05) over the time, when samples were subjected to a series of centrifugation and washing processes, however, the impacts of these processes on Glyphosate in sediment leachate was different than the impacts on Fluridone in sediment leachate. We anticipate that the results of Fluridone and Glyphosate concentrations in water column and sediment leachate provided here will help in decision making of herbicide application in ambient water bodies, and understanding the potential impacts of herbicides application on bed sediment.
Author Contributions
PP was involved in writing the manuscript with the help of JC, SL, MS, KH, LC, and ST. PG and MK helped in field sample collection and reviewing the manuscript. YZ, and YW were involved in data analysis, and method development. All authors read and approved the final manuscript.
Funding
We thank Department of Parks and Recreation, Division of Boating and Waterways, and Department of Water Resources, Sacramento, California for supporting this work (Grant/Contract Number-C1670803; and # C1370030). Authors also thank Yi Wang (graduate student, UC Davis) for providing support toward herbicide analysis. Any results, findings, opinions, interpretation, conclusions or recommendations are those of authors and do not necessarily reflect the views of the funding supporting agencies.
Conflict of Interest Statement
The authors declare that the research was conducted in the absence of any commercial or financial relationships that could be construed as a potential conflict of interest.
References
Abrantes, N., Pereira, R., and Gonçalves, F. (2010). Occurrence of pesticides in water, sediments, and fish tissues in a lake surrounded by agricultural lands: concerning risks to humans and ecological receptors. Water Air Soil Pollut. 212, 77–88. doi: 10.1007/s11270-010-0323-2
Amweg, E. L., Weston, D. P., You, J., and Lydy, M. J. (2006). Pyrethroid insecticides and sediment toxicity in urban creeks from California and Tennessee. Environ. Sci. Technol. 40, 1700–1706. doi: 10.1021/es051407c
Andrew, W., Haller, W., and Shilling, D. G. (2003). Response of St. augustinegrass to fluridone in irrigation water. J. Aquat. Plant Manage. 41, 61–63. Available online at: http://aquaticcommons.org/1786/1/v41p61.pdf
Antunes, S. C., Pereira, J. L., Cachada, A., Duarte, A. C., Gonçalves, F., Sousa, J. P., et al. (2010). Structural effects of the bioavailable fraction of pesticides in soil: suitability of elutriate testing. J. Hazard. Mater. 184, 215–225. doi: 10.1016/j.jhazmat.2010.08.025
Arellano-Aguilar, O., Betancourt-Lozano, M., Aguilar-Zárate, G., and de Leon-Hill, C. P. (2017). Agrochemical loading in drains and rivers and its connection with pollution in coastal lagoons of the Mexican Pacific. Environ. Monit. Assess. 189, 270. doi: 10.1007/s10661-017-5981-8
Batty, J., and Lim, R. (1999). Morphological and reproductive characteristics of male mosquitofish (Gambusia affinis holbrooki) inhabiting sewage-contaminated waters in New South Wales, Australia. Arch. Environ. Contam. Toxicol. 36, 301–307. doi: 10.1007/s002449900475
Bauer, A. E., Frank, R. A., Headley, J. V., Peru, K. M., Farwell, A. J., and Dixon, D. G. (2017). Toxicity of oil sands acid-extractable organic fractions to freshwater fish: pimephales promelas (fathead minnow) and Oryzias latipes (Japanese medaka). Chemosphere 171, 168–176. doi: 10.1016/j.chemosphere.2016.12.059
Borggaard, O. K., and Gimsing, A. L. (2008). Fate of glyphosate in soil and the possibility of leaching to ground and surface waters: a review. Pest Manage. Sci. 64, 441–456. doi: 10.1002/ps.1512
Buah-Kwofie, A., and Humphries, M. S. (2017). The distribution of organochlorine pesticides in sediments from iSimangaliso Wetland Park: ecological risks and implications for conservation in a biodiversity hotspot. Environ. Pollut. 229, 715–723. doi: 10.1016/j.envpol.2017.07.031
Cayla, N. (2014). Reconstructing pesticide use from lake sediments. Proc. Natl. Acad. Sci. U.S.A. 111, 15599–15600. doi: 10.1073/pnas.1411512111/DCSupplemental
Crowe, A. S., Leclerc, N., Struger, J., and Brown, S. (2011). Application of a glyphosate-based herbicide to Phragmites australis: impact on groundwater and near-shore lake water at a beach on Georgian Bay. J. Great Lakes Res. 37, 616–624. doi: 10.1016/j.jglr.2011.08.001
Daouk, S., Copin, P. J., Rossi, L., Chèvre, N., and Pfeifer, H. R. (2013). Dynamics and environmental risk assessment of the herbicide glyphosate and its metabolite AMPA in a small vineyard river of the Lake Geneva catchment. Environ. Toxicol. Chem. 32, 2035–2044. doi: 10.1002/etc.2276
Fluridone (2017). Fluridone, ELISA Kits. PN 500511, Magnatic Particle ELISA (100T). Available online at: http://www.abraxiskits.com/wp-content/uploads/2017/03/Fluridone-Users-Guide.pdf (Accessed April 9, 2018).
Fox, A. M., Haller, W., and Shilling, D. (1994). Use of fluridone for hydrilla management in the Withlacoochee river, Florida. J. Aquat. Plant Manage. 32, 47–55.
Fox, A. M., Haller, W. T., and Shilling, D. G. (1996). Hydrilla control with split treatments of fluridone in Lake Harris, Florida. Hydrobiologia 340, 235–239. doi: 10.1007/BF00012761
Gama, A. F., Cavalcante, R. M., Duaví, W. C., Silva, V. P. A., and Nascimento, R. F. (2017). Occurrence, distribution, and fate of pesticides in an intensive farming region in the Brazilian semi-arid tropics (Jaguaribe river, Ceará). J. Soils Sediments 17, 1160–1169. doi: 10.1007/s11368-016-1597-9
Gerber, R., Smit, N. J., Van Vuren, J. H. J., Nakayama, S. M. M., Yohannes, Y. B., Ikenaka, Y., et al. (2016). Bioaccumulation and human health risk assessment of DDT and other organochlorine pesticides in an apex aquatic predator from a premier conservation area. Sci. Total Environ. 550(Suppl. C), 522–533. doi: 10.1016/j.scitotenv.2016.01.129
Glyphosate, H. S. (2017). Glyphosate, ELISA Kits. PN 500081, Magnatic Particle ELISA (120T). Available online at: https://www.abraxiskits.com/wp-content/uploads/2017/12/Glyphosate-Magnetic-Particle-Users-Guide.pdf (Accessed April 9 2018).
Hanke, I., Singer, H., and Hollender, J. (2008). Ultratrace-level determination of glyphosate, aminomethylphosphonic acid and glufosinate in natural waters by solid-phase extraction followed by liquid chromatography–tandem mass spectrometry: performance tuning of derivatization, enrichment and detection. Anal. Bioanal. Chem. 391, 2265–2276. doi: 10.1007/s00216-008-2134-5
Hebert, C. E., Campbell, D., Kindopp, R., MacMillan, S., Martin, P., Neugebauer, E., et al. (2013). Mercury trends in colonial waterbird eggs downstream of the oil sands region of Alberta, Canada. Environ. Sci. Technol. 47, 11785–11792. doi: 10.1021/es402542w
Henry, L. A., Stehmann, M., De Clippele, L. S., Findlay, H., Golding, N., and Roberts, J. (2016). Seamount egg-laying grounds of the deep-water skate Bathyraja richardsoni. J. Fish Biol. 89, 1473–1481. doi: 10.1111/jfb.13041
Jacob, A. P., Culver, D. A., Lanno, R. P., and Voigt, A. (2016). Ecological impacts of fluridone and copper sulphate in catfish aquaculture ponds. Environ. Toxicol. Chem. 35, 1183–1194. doi: 10.1002/etc.3258
Kidd, K. A., Blanchfield, P. J., Mills, K. H., Palace, V. P., Evans, R. E., Lazorchak, J. M., et al. (2007). Collapse of a fish population after exposure to a synthetic estrogen. Proc. Natl. Acad. Sci. U.S.A. 104, 8897–8901. doi: 10.1073/pnas.0609568104
Madsen, J., Turnage, G., and Getsinger, K. (2016). Efficacy of combinations of diquat or triclopyr with fluridone for control of flowering rush. J. Aquat. Plant Manage. 54, 68–71. Available online at: http://www.apms.org/wp/wp-content/uploads/japm-54-02-68.pdf
Milne, H. (1974). Breeding numbers and reproductive rate of eiders at the Sands of Forvie National Nature Reserve, Scotland. Ibis 116,135–152. doi: 10.1111/j.1474-919X.1974.tb00233.x
Morales, J. B. L., Torres, J. B. V., Bastidas, P. J. B., Escalante, M. A. A., Sánchez, J. I. S., Lobo, A. L. B., et al. (2017). Monitoring of pesticides residues in northwestern Mexico rivers. Acta Universitaria 27, 45–54. doi: 10.15174/au.2017.1203
Net, S., Rabodonirina, S., Sghaier, R. B., Dumoulin, D., Chbib, C., Tlili, I., et al. (2015). Distribution of phthalates, pesticides and drug residues in the dissolved, particulate and sedimentary phases from transboundary rivers (France–Belgium). Sci. Total Environ. 521, 152–159. doi: 10.1016/j.scitotenv.2015.03.087
Netherland, M. D. (2015). Laboratory and greenhouse response of monoecious hydrilla to fluridone. J. Aquat. Plant Manage. 53, 178–184. Available online at: http://www.apms.org/wp/wp-content/uploads/japm-53-02-178.pdf
Parsons, J. K., Couto, A., Hamel, K., and Marx, G. (2009). Effect of fluridone on macrophytes and fish in a coastal Washington lake. J. Aquat. Plant Manage. 47, 31–40. Available online at: https://fortress.wa.gov/ecy/publications/documents/0903033.pdf
Pesce, S., Margoum, C., Rouard, N., Foulquier, A., and Martin-Laurent, F. (2013). Freshwater sediment pesticide biodegradation potential as an ecological indicator of microbial recovery following a decrease in chronic pesticide exposure: a case study with the herbicide diuron. Ecol. Indic. 29, 18–25. doi: 10.1016/j.ecolind.2012.12.014
Saxton, M. A., Morrow, E. A., Bourbonniere, R. A., and Wilhelm, S. W. (2011). Glyphosate influence on phytoplankton community structure in Lake Erie. J. Great Lakes Res. 37, 683–690. doi: 10.1016/j.jglr.2011.07.004
Scott, P. D., Coleman, H. M., Colville, A., Lim, R., Matthews, B., McDonald, J. A., et al. (2017). Assessing the potential for trace organic contaminants commonly found in Australian rivers to induce vitellogenin in the native rainbowfish (Melanotaenia fluviatilis) and the introduced mosquitofish (Gambusia holbrooki). Aquat. Toxicol. 185(Suppl. C), 105–120. doi: 10.1016/j.aquatox.2017.02.008
Souza, E. L., Foloni, L. L., Filho, J. T., Velini, E. D., Siono, L. M., and Silva, J. R. (2017). Half-life of glyphosate on the control of water hyacinths in water tanks. JWARP 9, 470–480. doi: 10.4236/jwarp.2017.95030
Stone, W. W., Gilliom, R. J., and Ryberg, K. R. (2014). Pesticides in US streams and rivers: occurrence and trends during 1992–2011. Environ. Sci. Technol. 48, 11025–11030. doi: 10.1021/es5025367
Stone, W. W., and Wilson, J. T. (2006). Preferential flow estimates to an agricultural tile drain with implications for glyphosate transport. J. Environ. Qual. 35, 1825–1835. doi: 10.2134/jeq2006.0068
Teh, S. J., Deng, D., Werner, I., Teh, F., and Hung, S. S. (2005). Sublethal toxicity of orchard stormwater runoff in Sacramento splittail (Pogonichthys macrolepidotus) larvae. Mar. Environ. Res. 59, 203–216. doi: 10.1016/j.marenvres.2003.12.005
UC Davis Analytical Laboratory (2017). Method of Analysis. Available online at: http://anlab.ucdavis.edu/methods-of-analysis (Accessed February 15 2018).
Vendrell, E., de Barreda Ferraz, D. G., Sabater, C., and Carrasco, J. (2009). Effect of glyphosate on growth of four freshwater species of phytoplankton: a microplate bioassay. Bull. Environ. Contam. Toxicol. 82, 538–542. doi: 10.1007/s00128-009-9674-z
Vereecken, H. (2005). Mobility and leaching of glyphosate: a review. Pest Manage. Sci. 61, 1139–1151. doi: 10.1002/ps.1122
Wang, W., Delgado-Moreno, L., Conkle, J. L., Anderson, M., Amrhein, C., Ye, Q., et al. (2012). Characterization of sediment contamination patterns by hydrophobic pesticides to preserve ecosystem functions of drainage lakes. J. Soils Sediments 12, 1407–1418. doi: 10.1007/s11368-012-0560-7
Wepener, V., Smit, N., Covaci, A., Dyke, S., and Bervoets, L. (2012). Seasonal bioaccumulation of organohalogens in Tigerfish, Hydrocynus vittatus Castelnau, from Lake Pongolapoort, South Africa. Bull. Environ. Contam. Toxicol. 88, 277–282. doi: 10.1007/s00128-011-0439-0
West, S. D., and Parka, S. J. (1981). Determination of the aquatic herbicide fluridone in water and hydrosoil: effect of application method on dissipation. J. Agric. Food Chem. 29, 223–226. doi: 10.1021/jf00104a004
Weston, D. P., Asbell, A. M., Lesmeister, S. A., Teh, S. J., and Lydy, M. J. (2014). Urban and agricultural pesticide inputs to a critical habitat for the threatened delta smelt (Hypomesus transpacificus). Environ. Toxicol. Chem. 33, 920–929. doi: 10.1002/etc.2512
Keywords: fluridone and glyphosate in ambient water, particle attached herbicides, herbicides leachate from sediment, sediment and water column interaction, deposition and resuspension
Citation: Pandey P, Caudill J, Lesmeister S, Zheng Y, Stillway M, Hoffmann K, Gilbert P, Kwong M, Conrad L and Teh S (2019) Assessing Glyphosate and Fluridone Concentrations in Water Column and Sediment Leachate. Front. Environ. Sci. 7:22. doi: 10.3389/fenvs.2019.00022
Received: 28 December 2017; Accepted: 07 February 2019;
Published: 03 April 2019.
Edited by:
Youji Wang, Shanghai Ocean University, ChinaReviewed by:
Sara Cristina Antunes, Universidade do Porto, PortugalZhao Chen, University of Maryland, College Park, United States
Copyright © 2019 Pandey, Caudill, Lesmeister, Zheng, Stillway, Hoffmann, Gilbert, Kwong, Conrad and Teh. This is an open-access article distributed under the terms of the Creative Commons Attribution License (CC BY). The use, distribution or reproduction in other forums is permitted, provided the original author(s) and the copyright owner(s) are credited and that the original publication in this journal is cited, in accordance with accepted academic practice. No use, distribution or reproduction is permitted which does not comply with these terms.
*Correspondence: Pramod Pandey, cGtwYW5kZXlAdWNkYXZpcy5lZHU=