- 1School of Engineering, RMIT University, Melbourne, VIC, Australia
- 2Department of Civil Engineering, University of the Philippines, Los Baños, Philippines
At present, the recovery and utilization of methane from anaerobic wastewater treatment systems as a source of energy are well-researched and widely adopted for a more sustainable system approach. However, not all methane produced in an anaerobic treatment system is completely recovered; subsequently, dissolved methane present in the effluent can be released into the environment and contribute to greenhouse gas accumulation in the atmosphere and reduce the system's methane yield. Many studies have already investigated and discussed the factors affecting the production of dissolved methane, as well as the techniques for its recovery. Among the recovery techniques, the use of degassing membrane contactor is most preferred for wastewater treatment application. However, reported data in the literature is limited to certain types of wastewater characteristics and anaerobic systems. Studies on membrane-based recovery of dissolved methane from AnMBR effluents are reviewed in this paper. For the case of the degassing membrane contactor, porous, or micro-porous membranes provides higher dissolved methane recovery efficiency than non-porous. However, porous membranes are more susceptible to pore wetting problem. Among the different operating conditions of degassing membrane contactors, liquid velocity, or flow rate greatly affects the recovery, wherein higher velocity decreases the recovery efficiency of dissolved methane. Consequently, research priorities aimed at development of degassing membrane to accommodate higher liquid velocity and to reduce pore wetting. Moreover, energy analysis of the AnMBR with degassing membrane system should be analyzed for performance in full-scale applications.
Introduction
Methane is a hydrocarbon compound resulting from the anaerobic degradation of organic materials. It is flammable and explosive gas, producing carbon dioxide and water vapor (Encyclopædia Britannica, 2018). The sources of global methane are a result of both natural and anthropogenic activities. The latter provides 60% of methane sources which are further classified as agriculture, energy, waste, and industrial sectors. The majority of methane produced from agriculture sector is released during the enteric fermentation in animal raising; methane from the energy sector is mainly produced from the production and processing of oil; from the waste sector it is primarily generated from the solid waste and wastewater processing; and lastly, the industrial source of methane comes from chemicals and metal productions (Karakurt et al., 2012).
As part of the focus of this study, almost 9% of the methane released into the environment comes from the activities involved in wastewater systems—from its collection, treatment, and disposal (Karakurt et al., 2012; Hu et al., 2017; Short et al., 2017). Methane is released into the wastewater through the metabolism of methanogens in an anaerobic condition of wastewater treatment system (Crone et al., 2016). Water or wastewater treatment is an inevitable part of the human community to abate the negative impacts of its disposal on the environment and living beings. However, the high energy requirement for the collection and treatment of wastewater is a major concern. This emphasizes the need for processes which will allow recovery of methane and its utilization as a source of energy for the wastewater treatment plant to improve its energy efficiency (Rongwong et al., 2018). Theoretically, about 0.35 liters of methane is produced per grams of chemical oxygen demand (COD) removed from the wastewater (Tchobanoglous et al., 2003) and about one cubic meter of methane has an estimated energy potential of 9 kWh (Crone et al., 2016). As cited in the study of Molino et al. (2013), if methane produced from the wastewater treatment system is used as automotive fuel, around 97% of potential carbon dioxide emission can be reduced compared to the use of fossil fuel, provided that the methane content of biogas is at least 90% (Harasimowicz et al., 2007). A critical review study on nine pilot-scale AnMBR systems (treating domestic wastewater) estimated that five of these systems have positive energy balance, which proved the potential of AnMBR to be an energy producer (Shin and Bae, 2018). Aside from this energy impact, recovery and utilization of methane have an environmental impact, too. According to the Intergovernmental Panel on Climate Change in 2014, methane has 28 times global warming potential than carbon dioxide (IPCC, 2014). This establishes the need for the control on the release of methane into the environment.
However, not all methane produced in a wastewater treatment system is recovered, which in turn will be discharged with the effluent in the form of dissolved methane and released into the environment. Liu et al. (2014) provided correlations among the solubility of methane in water and the temperature and salinity of the water (Figure 1). From Figure 1, the theoretical dissolved methane present in municipal wastewater effluent (with an average influent soluble COD concentration of 200 mg/L and a COD removal efficiency of at least 90%) at 30°C is around 45% of the total methane produced (Liu et al., 2014). In support of this, Smith et al. (2013) found that the percentage of dissolved methane in the effluent is 40–50% at 15°C. This dissolved methane can be utilized as an additional energy source for the operation of wastewater treatment facilities. Rongwong et al. (2018) calculated that optimum net electricity energy of 0.178 MJ could be recovered from the dissolved methane per cubic meter of effluent from an AnMBR coupled with a degassing membrane. This is still around 85% of the total energy recovered from the dissolved methane.
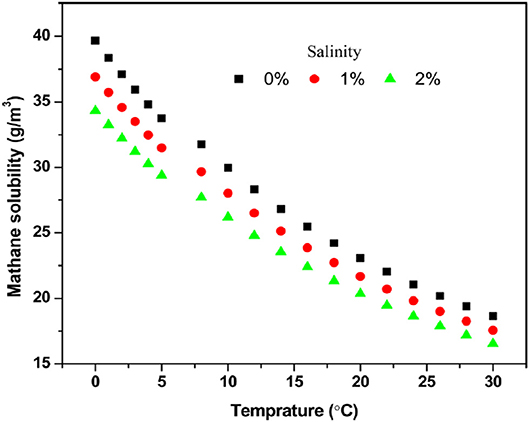
Figure 1. Solubility of methane in water under different temperature and salinity (Adapted with permission from Dissolved Methane: A Hurdle for Anaerobic Treatment of Municipal Wastewater Liu et al., 2014. Copyright 2014 American Chemical Society).
Anaerobic Membrane Bioreactor (AnMBR): Applications and Challenges in Wastewater Treatment
Anaerobic membrane bioreactor (AnMBR) is a type of biological wastewater treatment system that operates in the absence of oxygen and utilizes membranes to provide solid-liquid separation (Lin et al., 2013). AnMBR is usually favored over other conventional aerobic and anaerobic treatment systems because it provides effluent with high quality, requires a smaller footprint, provides long solid retention time (SRT) while having low hydraulic retention time (HRT), and allows complete retention of biomass. Also, it has lesser start-up time and can be applied as either complete treatment or pre-treatment. However, the major challenge with AnMBR is to maintain the permeate flux which will tend to reduce over time due to the fouling of membrane. But with the addition of membrane fouling control, such as gas sparging and use of chemicals, the operating and maintenance expenditures will increase (Lin et al., 2013; Berkessa et al., 2018).
The configuration of AnMBR can either be an external crossflow AnMBR, where the membrane module is separated from the reactor, or submerged AnMBR (SAnMBR), where the membrane is submerged in the reactor. Although external AnMBR provides more direct control of fouling and easier replacement of membrane module, studies confirm that SAnMBR has lower energy consumption, fewer cleaning procedures, and lower tangential velocities (Lin et al., 2013; Dvorák et al., 2015). Furthermore, membrane materials used in AnMBR is categorized as polymeric, metallic, and inorganic, and the membrane module configuration as flat sheet, hollow fiber, and tubular. Aside from the material and configuration of the membrane, its pore size also determines the treatment efficiency and the capability of AnMBR (Lin et al., 2013).
Based on the review by Lin et al. (2013), the applicability of AnMBR in wastewater treatment is described by the influent concentration, influent particulate characteristics, and extreme conditions, such as very high or very low temperature and pH. It was then concluded that AnMBR is applicable to treat all types of wastewater except those with high organic strength, low particulate concentration, and less extreme conditions. With this, new designs for AnMBR have emerged to improve the applicability of AnMBR to other environments, such as high strength and industrial wastewaters, with lesser problems with fouling (Liu et al., 2016; Hu et al., 2017; Berkessa et al., 2018).
Recovery of Dissolved Methane From Anaerobic Treatment Effluents
One of the major challenges associated with the methane recovery from anaerobic processes is the high concentration of dissolved methane in the effluent (Liu et al., 2014). Many studies link this high concentration to the supersaturation index of dissolved methane which is defined as the ratio between the actual dissolved methane concentration and the theoretical concentration, based on Henry's Law (Crone et al., 2016). Supersaturation is caused by shock conditions and entrapped bubbles in the sludge (Smart Water Fund, 2013). Rongwong et al. (2018) reported that this supersaturation index varied in the different reactors, wherein upflow anaerobic sludge blanket has a higher supersaturation of methane (at most 6.9) than in AnMBR (at most 1.5). This low supersaturation index of AnMBR is due to the ability of the system to retain the biomass in the reactor (Crone et al., 2016).
Apart from the supersaturation, the release of methane gas in the headspace of the AnMBR would initially determine the concentration of the dissolved methane in the effluent. Guo et al. (2016) listed and analyzed the factors that affect the stability and transfer of methane in the headspace of AnMBR, namely temperature, pH, solid retention time (SRT), organic loading rate (OLR), and hydraulic retention time (HRT). For temperature, Henry's Law states that higher temperature lowers the solubility of gases. Thus, thermophilic conditions (50–60°C) are generally favorable for methane production since at psychrophilic temperatures (3–15°C), the dissolved methane in the effluent increases (Lin et al., 2013; Smith et al., 2015; Guo et al., 2016). However, the thermophilic condition is not widely utilized due to the additional energy requirement. The study emphasized the need for further research for optimal temperature condition and the effect of temperature shocks in biogas production. In the study of Gao et al. (2011), it is worthwhile to note that submerged AnMBR can tolerate temperature changes with little to no effect on the recovery of biogas. In the case of pH, methane production is higher within the optimal range of 6.0–8.0, which provides favorable pH condition for the growth of methanogenic bacteria (Huang et al., 2008; Ward et al., 2008; Weiland, 2010). Subsequently, a study by Gao et al. (2010) of submerged AnMBR for thermomechanical whitewater treatment with varying pH shocks found that it lowers the methane recovery, increases fouling, and lowers effluent quality. This lower methane recovery could be attributed to the increase in the supersaturation of methane due to shock condition. Lastly, longer SRT and HRT as well as higher OLR (since 0.35 liters of methane can be recovered for every gram of COD removed) provide higher methane production (Roh et al., 2006; Saddoud and Sayadi, 2007; Wijekoon et al., 2011; Guo et al., 2016). From the study of Yeo and Lee (2013), the production of methane gas is 45% higher for SRT of 40 days compared to that of 20 days. Also, they found out that supersaturation of dissolved methane occurred for 20 days and none for 40 days. However, lower HRT and higher OLR could induce fouling (Guo et al., 2016).
There are several techniques for the recovery of dissolved methane in the anaerobic wastewater treatment effluent. The most common techniques for these systems are aeration, gas stripping, and degassing membrane. The use of membranes provides the highest potential for dissolved methane recovery due to its ease of operation and high mass transfer area (Rongwong et al., 2018). Moreover, agitation provides the lowest methane recovery among the list, while sparging and degassing membrane produce the best methane recovery with medium to high capital and operating costs (Smart Water Fund, 2013).
The mechanism of the membrane to separate the gas from the liquid is from the concentration difference defined by Fick's Law and pressure drop across the membrane (Gabelman and Hwang, 1999; Crone et al., 2016). Moreover, the hydrophobicity of the membranes acts as a barrier between the gas and liquid phases (Wongchitphimon et al., 2017). The hollow fiber membrane is the most used configuration for the membrane due to its high gas-liquid separation efficiency, compactness, ease of scaling-up, and very high surface area as compared to flat sheet membranes. There is a wide range of commercially available hollow fiber membranes that varies from the type of hydrophobic polymer used, the porosity (non-porous, porous, microporous), and the inner and outer diameters, length, thickness, and the number of the fibers. However, membrane wetting, which is the penetration of liquid into the pores of the membrane, is the major issue in degassing due to the additional mass transfer resistance it poses (Wongchitphimon et al., 2017).
Table 1 summarizes the different studies which used a degassing membrane contactor unit to improve the recovery of dissolved methane recovery from the effluent of the reactor. From these studies, use of a degassing unit improved the recovery of dissolved methane from the effluent of the reactors used to almost 99%. All of these studies concluded that low liquid velocity or flux, high transmembrane pressure, and porous/micro-porous membranes increase the recovery efficiency of dissolved methane from the effluent. At lower liquid flux, the membrane contact time increases the probability of methane to be diffused into the membrane (Cookney et al., 2012). In the study of Cookney et al. (2016), the recovery of dissolved methane decreased from 98.9 to 63.3% when the liquid velocity was increased from 0.0004 to 0.045 m/s. This decrease in the recovery efficiency is more pronounced for the case of non-porous membrane used, wherein the efficiency decreased from 92.6% (0.0004 m/s) to 10.8% (0.047 m/s). This shows that porous/micro-porous membrane is better than non-porous membrane in terms of dissolved methane recovery at high liquid flux.
A study by Cookney et al. (2016) found that the mass transfer resistance in porous membrane is 0.2% at high liquid velocity and 91% with non-porous membrane. However, in the study of Henares et al. (2016), the micro-porous membrane has lower recovery efficiency than non-porous membrane at higher liquid flux (for 90 L/h/m2 at a vacuum pressure of 50 kPa). This is due to the pore wetting problem typically experienced with porous/micro-porous membranes at higher liquid flux (Henares et al., 2016). On the other hand, flow in lumen side of the hollow fiber membranes (flow is through the inside of the fiber) rather than its shell side (flow through the outside) is favorable for recovery of dissolved methane. This is because the lumen side provides higher mass transfer efficiency (Crone et al., 2016). Finally, these degassing units did not show any negative impact on the effluent quality.
Research Priorities
Several studies proved that AnMBR showed a more stable supersaturation index (1.0–1.5) than the upflow anaerobic sludge blanket reactor (1.34–6.9), as summarized in the review study of Crone et al. (2016). However, only a few studies have been carried out for AnMBR with the focus on the connection between the supersaturation and the dissolved methane recovery. As presented in the study of Cookney et al. (2016), although the supersaturation is almost 1.0, the percentage of dissolved methane in the effluent is 88%. In comparison to the other AnMBR study of Smith et al. (2013), the index is 1.5 while the percentage of dissolved methane in the effluent is at most 50%. This could be attributed to the operating condition of the reactor wherein biogas sparging or bubbling was employed and the organic loading rate was very high.
Aside from this, all of the studies presented in Table 1 did not measure the actual dissolved methane concentration in the effluent; rather the concentration was computed based on temperature and partial pressure in the still headspace from Henry's Law. With the advancement of technology, now the actual methane concentration in the effluent can be measured for accurate quantification. At present, only two studies used a commercially available probe to measure the actual dissolved methane (Rongwong et al., 2017; Wongchitphimon et al., 2017). Other studies for AnMBR showed that the higher methane recovery is expected from hybrid system (two-stage/two-phased reactors) and with sparging (Roh et al., 2006; Saddoud and Sayadi, 2007; Huang et al., 2008; Lin et al., 2009; Gao et al., 2010; Xie et al., 2010, and Wijekoon et al., 2011). This is supported by the study of Shoener et al. (2016), wherein a cross-flow multi-tube and submerged hollow fiber with granular activated carbon (GAC) configurations were suggested. Moreover, as seen in Table 1, there are very limited studies on the use of degassing membrane contactor in recovering dissolved methane from the AnMBR effluent. Further studies should be aimed at the optimization of the different operating conditions of degassing membrane contactor, such as liquid flux and transmembrane pressure, inclusion of carbon dioxide desorption, and development of new membrane that is durable and resistant to pore wetting (Rongwong et al., 2017; Wongchitphimon et al., 2017). Finally, energy analysis on the use of degassing membrane contactor is a must to justify its economic and environmental impact.
On the other hand, improvement of methane concentration in biogas recovered from wastewater should also be looked into for the better utilization of biogas. Huertas et al. (2011) stated that for energy management there should at least 70% methane, at most 10% carbon dioxide, and negligible hydrogen sulfide be present in the biogas. However, it should be noted that removal of carbon dioxide is only recommended if the recovered biogas is used as a vehicle fuel and as natural gas for the grid; removal of carbon dioxide is usually not required for boiler, kitchen stove, and combined heat and power (CHP) applications (Petersson and Wellinger, 2009). For the cases which require biogas upgrade, techniques such as absorption (at least 97% methane), adsorption (95–99% methane), membrane separation (at least 96% methane), and cryogenic separation (90–99% methane) can be employed (Chen et al., 2015). Among these techniques, the use of membranes showed the most benefits such as low energy consumption, good selectivity, ease of engineering (Chen et al., 2015).
Conclusion
The use of the membranes in wastewater treatment is a well-established technology and currently used for the treatment of high strength or industrial wastewater. Moreover, the membrane can also be used as a technique to separate the dissolved methane from the effluent of an anaerobic treatment reactor. However, there are limited studies regarding the use of membranes for this kind of application, i.e., combining AnMBR and a degassing membrane contactor, with a focus on the recovery of dissolved methane. The studies reviewed showed that dissolved methane recovery using a degassing membrane contactor was higher under the following conditions: low liquid velocity or flux, higher transmembrane pressure, use of porous or micro-porous membrane, flow of liquid from the lumen side of the membrane, presence of carbon dioxide desorption, longer retention time, and low temperature. Moreover, the highest reported recovery of dissolved methane from an AnMBR effluent was around 99% obtained using a polypropylene micro-porous membrane with a liquid velocity of 0.0004 m/s. Therefore, further studies with an aim to optimize recovery and development of new membranes, while considering membrane fouling and pore wetting problem. Finally, this optimization should consider the economy of the system as well.
Author Contributions
PV synthesized the whole review paper, as part of her on-going doctorate study. This was then reviewed and edited by VJ and MO.
Funding
This review is part of the on-going doctorate study of the first author under the grant from the Commission on Higher Education, Philippines, the University of the Philippines, and the RMIT University, Australia.
Conflict of Interest Statement
The authors declare that the research was conducted in the absence of any commercial or financial relationships that could be construed as a potential conflict of interest.
Acknowledgments
The authors would like to acknowledge RMIT University, Australia and the government of the Philippines for the academic and financial support.
Abbreviations
AnMBR, anaerobic membrane bioreactor; COD, chemical oxygen demand; EGSB, expanded granular sludge bed; HRT, hydraulic retention time; OLR, organic loading rate; PDMS, polydimethylsiloxane; PE, polyethylene; PP, polypropylene; PU, Polyurethane; PVDF, Polyvinylidene difluoride; SAF-MBR, staged anaerobic fluidized membrane bioreactor; SRT, solids retention time; SAnMBR, submerged anaerobic membrane bioreactor; UASB, upflow anaerobic sludge blanket.
References
Bandara, W. M., Satoh, H., Sasakawa, M., Nakahara, Y., Takahashi, M., and Okabe, S. (2011). Removal of residual dissolved methane gas in an upflow anaerobic sludge blanket reactor treating low-strength wastewater at low temperature with degassing membrane. Water Res. 45, 3533–3540. doi: 10.1016/j.watres.2011.04.030
Berkessa, Y. W., Yan, B., Li, T., Tan, M., She, Z., Jegatheesan, V., et al. (2018). Novel anaerobic membrane bioreactor (AnMBR) design for wastewater treatment at long HRT and high solid concentration. Bioresour. Technol. 250, 281–289. doi: 10.1016/j.biortech.2017.11.025
Chen, X. Y., duc Vinh, H., Ramirez, A. A., Rodrigue, D., and Kaliaguine, S. (2015). Membrane gas separation technologies for biogas upgrading. RSC Adv. 5, 24399–24448. doi: 10.1039/C5RA00666J
Cookney, J., Cartmell, E., Jefferson, B., and McAdam, E., J (2012). Recovery of methane from anaerobic process effluent using poly-di-methyl-siloxane membrane contactors. Water Sci. Technol. 65, 604–610. doi: 10.2166/wst.2012.897
Cookney, J., McLeod, A., Mathioudakis, V., Ncube, P., Soares, A., Jefferson, B., et al. (2016). Dissolved methane recovery from anaerobic effluents using hollow fibre membrane contactors. J. Memb. Sci. 502, 141–150. doi: 10.1016/j.memsci.2015.12.037
Crone, B. C., Garland, J.L, Sorial, G.,A, and Vane, L.,M (2016). Significance of dissolved methane in effluents of anaerobically treated low strength wastewater and potential for recovery as an energy product: a review. Water Res. 104, 520–531. doi: 10.1016/j.watres.2016.08.019
Dvorák, L., Gómez, M., Dolina, J., and Cernín, A. (2015). Anaerobic membrane bioreactors—a mini review with emphasis on industrial wastewater treatment: applications, limitations and perspectives. Desalin. Water Treat. 57, 19062–19076. doi: 10.1080/19443994.2015.1100879
Encyclopædia Britannica (2018). Encyclopædia Britannica. Available online at: https://www.britannica.com/science/methane (Accessed September 17, 2018).
Gabelman, A., and Hwang, S-T. (1999). Hollow fiber membrane contactors. J. Memb. Sci. 159, 61–106. doi: 10.1016/S0376-7388(99)00040-X
Gao, Jane, W.,J, Lin, H.J., Leung, K.T., and Liao, B.,Q. (2010). Influence of elevated pH shocks on the performance of a submerged anaerobic membrane bioreactor. Proc. Biochem. 45, 1279–1287. doi: 10.1016/j.procbio.2010.04.018
Gao, W. J., Leung, K.,T, Qin, W.,S, and Liao, B.,Q (2011). Effects of temperature and temperature shock on the performance and microbial community structure of a submerged anaerobic membrane bioreactor. Bioresour. Technol. 102, 8733–8740. doi: 10.1016/j.biortech.2011.07.095
Giménez, J. B., Marti, N., Ferrer, J., and Seco, A. (2012). Methane recovery efficiency in a submerged anaerobic membrane bioreactor (SAnMBR) treating sulphate-rich urban wastewater: evaluation of methane losses with the effluent. Bioresour. Technol. 118, 67–72. doi: 10.1016/j.biortech.2012.05.019
Guo, W., Ngo, H. H., Chen, C., Pandey, A., Tung, K-L., and Lee, D. J. (2016). “Anaerobic membrane bioreactors for future green bioprocesses,” in Green Technologies for Sustainable Water Management (Reston: ASCE Library), 867–901. doi: 10.1061/9780784414422.ch25
Harasimowicz, M., Orluk, P., Zakrzewska-Trznadel, G., and Chmielewski, A.G (2007). Application of polyimide membranes for biogas purification and enrichment. J. Hazard. Mater. 144, 698–702. doi: 10.1016/j.jhazmat.2007.01.098
Henares, M., Izquierdo, M., Penya-Roja, J.M., V., and Martínez-Soria (2016). Comparative study of degassing membrane modules for the removal of methane from Expanded Granular Sludge Bed anaerobic reactor effluent. Sep. Purificat. Technol. 170, 22–29. doi: 10.1016/j.seppur.2016.06.024
Hu, D., Su, H., Chen, Z., Cui, Y., Ran, C., Xu, J., et al (2017). Performance evaluation and microbial community dynamics in a novel AnMBR for treating antibiotic solvent wastewater. Bioresour. Technol. 243, 218–227. doi: 10.1016/j.biortech.2017.06.095
Huang, Z., Ong, S.,L, and Ng, H.Y. (2008). Feasibility of submerged anaerobic membrane bioreactor (SAMBR) for treatment of low-strength wastewater. Water Sci. Technol. 58, 1925–1931. doi: 10.2166/wst.2008.749
Huertas, J. I., Giraldo, N., and Izquierdo, S. (2011). “Removal of H2S and CO2 from biogas by amine absorption,” in Mass Transfer in Chemical Engineering Processes (London, UK: IntechOpen), 133–150. doi: 10.5772/20039
IPCC (2014). Climate Change 2014: Synthesis Report. Contribution of Working Groups I, II and III to the Fifth Assessment Report of the Intergovernmental Panel on Climate Change, eds R. K. Pachauri, M. R. Allen, V. R. Barros, J. Broome, W. Cramer, R. Christ, J. A. Church, L. Clarke, Q. Dahe and P. Dasgupta: IPCC.
Karakurt, I., Aydin, G., and Aydiner, K. (2012). Sources and mitigation of methane emissions by sectors: a critical review. Renew. Energy 39, 40–48. doi: 10.1016/j.renene.2011.09.006
Lin, H., Peng, W., Zhang, M., Chen, J., Hong, H., and Zhang, Y. (2013). A review on anaerobic membrane bioreactors: applications, membrane fouling and future perspectives. Desalination 314, 169–188. doi: 10.1016/j.desal.2013.01.019
Lin, H. J., Xie, K., Mahendran, B., Bagley, D.,M, Leung, K.,T, Liss, S.,N., et al. (2009). Sludge properties and their effects on membrane fouling in submerged anaerobic membrane bioreactors (SAnMBRs). Water Res. 43, 3827–3837. doi: 10.1016/j.watres.2009.05.025
Liu, J., Jia, X., Gao, B., Bo, L., and Wang, L. (2016). Membrane fouling behavior in anaerobic baffled membrane bioreactor under static operating condition. Bioresour. Technol. 214, 582–588. doi: 10.1016/j.biortech.2016.05.016
Liu, Z. H., Yin, H., Dang, Z., and Liu, Y. (2014). Dissolved methane: a hurdle for anaerobic treatment of municipal wastewater. Environ. Sci. Technol. 48, 889–890. doi: 10.1021/es405553j
Luo, G., Wang, W., and Angelidaki, I. (2014). A new degassing membrane coupled upflow anaerobic sludge blanket (UASB) reactor to achieve in-situ biogas upgrading and recovery of dissolved CH4 from the anaerobic effluent. Appl. Energy 132, 536–542. doi: 10.1016/j.apenergy.2014.07.059
Molino, A., Migliori, M., Ding, Y., Bikson, B., Giordano, G., and Braccio, G. (2013). Biogas upgrading via membrane process: modelling of pilot plant scale and the end uses for the grid injection. Fuel 107, 585–592. doi: 10.1016/j.fuel.2012.10.058
Petersson, A., and Wellinger, A. (2009). Biogas Upgrading Technologies Developments and Innovations. Technical Brochure, IEA Bioenergy 20, 1–19.
Roh, S., Chun, Y. N., Nah, J.-W., Shin, H.-J., and Kim, S.-I. (2006). Wastewater treatment by anaerobic digestion coupled with membrane processing. J. Indust. Chem. 12, 489–493.
Rongwong, W., Goh, K., and Bae, T-H. (2018). Energy analysis and optimization of hollow fiber membrane contactors for recovery of dissolve methane from anaerobic membrane bioreactor effluent. J. Memb. Sci. 554, 184–194. doi: 10.1016/j.memsci.2018.03.002
Rongwong, W., Wongchitphimon, S., Goh, K., Wang, R., and Bae, T-H. (2017). Transport properties of CO2 and CH4 in hollow fiber membrane contactor for the recovery of biogas from anaerobic membrane bioreactor effluent. J. Memb. Sci. 541, 62–72. doi: 10.1016/j.memsci.2017.06.090
Saddoud, A., and Sayadi, S. (2007). Application of acidogenic fixed-bed reactor prior to anaerobic membrane bioreactor for sustainable slaughterhouse wastewater treatment. J. Hazard. Mater. 149, 700–706. doi: 10.1016/j.jhazmat.2007.04.031
Shin, C., and Bae, J. (2018). Current status of the pilot-scale anaerobic membrane bioreactor treatments of domestic wastewaters: a critical review. Bioresour. Technol. 247, 1038–1046. doi: 10.1016/j.biortech.2017.09.002
Shoener, B. D., Zhong, C., Greiner, A. D., Khunjar, W. O., Hong, P-Y., and Guest, J. S. (2016). Design of anaerobic membrane bioreactors for the valorization of dilute organic carbon waste streams. Energy Environ. Sci. 9, 1102–1112. doi: 10.1039/C5EE03715H
Short, M. D., Daikeler, A., Wallis, K., Peirson, W.,L, and Peters, G.,M (2017). Dissolved methane in the influent of three Australian wastewater treatment plants fed by gravity sewers. Sci. Tot. Environ. 599–600, 85–93. doi: 10.1016/j.scitotenv.2017.04.152
Smart Water Fund (2013). Literature Review of Technology for the Recovery of Methane From Wastewater.
Smith, A. L., Skerlos, S.,J., and Raskin, L. (2013). Psychrophilic anaerobic membrane bioreactor treatment of domestic wastewater. Water Res. 47, 1655–1665. doi: 10.1016/j.watres.2012.12.028
Smith, A. L., Skerlos, S.,J., and Raskin, L. (2015). Anaerobic membrane bioreactor treatment of domestic wastewater at psychrophilic temperatures ranging from 15°C to 3°C. Environ. Sci. Water Res. Technol. 1, 56–64. doi: 10.1039/C4EW00070F
Tchobanoglous, G., Burton, F. L., Stensel, D. H., and Metcalf Eddy, Inc. eds. (2003). Wastewater Engineering: Treatment and Reuse, 4th Edn, New York, NY: McGraw-Hill.
Ward, A. J., Hobbs, P.,J, Holliman, P.,J, and Jones, D.,L (2008). Optimisation of the anaerobic digestion of agricultural resources. Bioresour. Technol. 99, 7928–7940. doi: 10.1016/j.biortech.2008.02.044
Weiland, P. (2010). Biogas production: current state and perspectives. Appl. Microbiol. Biotechnol. 85, 849–860. doi: 10.1007/s00253-009-2246-7
Wijekoon, K. C., Visvanathan, C., and Abeynayaka, A. (2011). Effect of organic loading rate on VFA production, organic matter removal and microbial activity of a two-stage thermophilic anaerobic membrane bioreactor. Bioresour. Technol. 102, 5353–5360. doi: 10.1016/j.biortech.2010.12.081
Wongchitphimon, S., Rongwong, W., Chuah, C. Y., Wang, R., and Bae, T-H. (2017). Polymer-fluorinated silica composite hollow fiber membranes for the recovery of biogas dissolved in anaerobic effluent. J. Memb. Sci. 540, 146–154. doi: 10.1016/j.memsci.2017.06.050
Xie, K., Lin, H.,J, Mahendran, B., Bagley, D.,M, Leung, K.,T, Liss, S.,N., et al. (2010). Performance and fouling characteristics of a submerged anaerobic membrane bioreactor for kraft evaporator condensate treatment. Environ. Technol. 31, 511–521. doi: 10.1080/09593330903527898
Keywords: methane, wastewater, anaerobic membrane bioreactor, dissolved methane, degassing membrane
Citation: Velasco P, Jegatheesan V and Othman M (2018) Recovery of Dissolved Methane From Anaerobic Membrane Bioreactor Using Degassing Membrane Contactors. Front. Environ. Sci. 6:151. doi: 10.3389/fenvs.2018.00151
Received: 03 August 2018; Accepted: 29 November 2018;
Published: 17 December 2018.
Edited by:
Obulisamy Parthiba Karthikeyan, University of Michigan, United StatesReviewed by:
Gaurav Saxena, Babasaheb Bhimrao Ambedkar University, IndiaSuyun Xu, University of Shanghai for Science and Technology, China
Copyright © 2018 Velasco, Jegatheesan and Othman. This is an open-access article distributed under the terms of the Creative Commons Attribution License (CC BY). The use, distribution or reproduction in other forums is permitted, provided the original author(s) and the copyright owner(s) are credited and that the original publication in this journal is cited, in accordance with accepted academic practice. No use, distribution or reproduction is permitted which does not comply with these terms.
*Correspondence: Perlie Velasco, czM2ODY1ODZAc3R1ZGVudC5ybWl0LmVkdS5hdQ==