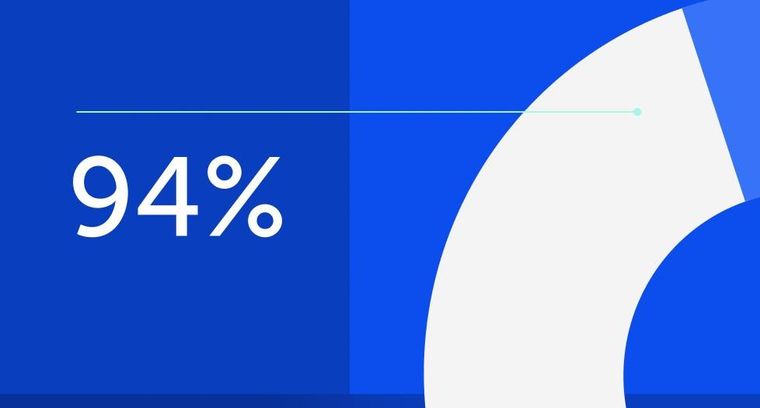
94% of researchers rate our articles as excellent or good
Learn more about the work of our research integrity team to safeguard the quality of each article we publish.
Find out more
ORIGINAL RESEARCH article
Front. Environ. Sci., 12 December 2018
Sec. Freshwater Science
Volume 6 - 2018 | https://doi.org/10.3389/fenvs.2018.00150
This article is part of the Research TopicAchieving Water-Energy-Food Nexus Sustainability: A Science and Data Need or a Need for Integrated Public Policy?View all 26 articles
Globally, freshwater is unevenly distributed, both in space and time. Climate change, land use alteration, and increasing human exploitation will further increase the pressure on water as a resource for human welfare and on inland water ecosystems. Water transfer megaprojects (WTMP) are defined here as large-scale engineering interventions to divert water within and between river basins that meet one of the following criteria: construction costs >US$ 1 billion, distance of transfer >190 km, or volume of water transferred exceeds 0.23 km3 per year. WTMP represent an engineered solution to cope with water scarcity. These projects are most commonly associated with large-scale agricultural and energy development schemes, and many of them serve multiple purposes. Despite numerous case studies that focus on the social, economic, and environmental impacts of individual water transfer megaprojects, a global inventory of existing, planned and proposed projects is lacking. We carried out the first comprehensive global inventory of WTMP that are planned, proposed or under construction. We collected key information (e.g., location, distance, volume, costs, purpose) on 34 existing and 76 future (planned, proposed or under construction) WTMP. If realized, the total volume of water transferred by future projects will reach 1,910 km3 per year with a total transfer distance of more than twice the length of the Earth's equator. The largest future WTMP are located in North America, Asia, and Africa and the predicted total investment will exceed 2.7 trillion US$. Among future projects, 42 are for agricultural development, 13 for hydropower development and 10 combine both purposes. Future megaprojects are also planned to support mining, ecosystem restoration and navigation. Our results underscore the extent to which humans have and are planning to re-engineer the global hydrological network and flows through WTMP, creating a network of “artificial rivers.” They emphasize the need to ensure the inclusion of these projects in global and basin hydrological models, and to develop internationally agreed criteria to assess the ecological, social and economic impacts of WTMP.
Water is an essential resource for human well-being and the functioning of ecosystems. At the same time, increasing water scarcity is among the biggest challenges humanity is facing (Haddeland et al., 2014; Brauman et al., 2016). By 2030, the world will experience a 40% water deficit or a supply-demand gap under a business-as-usual scenario (2030 WRG, 2009). The global distribution of freshwater is uneven both in space and time (Rodell et al., 2018), and becomes further exacerbated through changes in total precipitation, seasonality, interannual variability, and the magnitude and frequency of extreme meteorological events (Rockström et al., 2014; Schewe et al., 2014). Water quality is deteriorating, too, due to industrial, agricultural and municipal pollution, further constraining water resources for humans and nature alike (Vörösmarty et al., 2010).
While the global availability of freshwater remains relatively constant, the demand is growing. This increasing demand is tightly linked to securing food and energy for a growing human population (UNESCO-WWAP, 2014; UNSD, 2018). Water and energy are necessary for all stages of food production, from irrigation to processing. Currently, irrigation accounts for 70% (or 2,710 km3) of the water resources withdrawn by humans globally from rivers and aquifers, although the exact value significantly varies between continents and regions (FAO, 2011). Together, food production and supply chains are responsible for 30% of the total global energy consumption (UNESCO-WWAP, 2012). At the same time, water is required for power generation and cooling as well as the production of biofuels. In 2010, global water withdrawals for energy consumption accounted for 15% of the world's total withdrawals; and this withdrawal rate is expected to increase by 20% until 2035 (UNESCO-WWAP, 2014). Hence, the “water-food-energy nexus” was identified by the World Economic Forum as a key development challenge for the increasing human population (WEF, 2011). By 2050, the human population is projected to reach 9.8 billion (UN, 2017), with 66% living in urban areas (UN, 2014). In addition, food demand will increase by 50% (FAO, IFAD, UNICEF, WFP and WHO, 2017), energy demand by up to 61% (WEC, 2013), and water demand by 55% (UNESCO-WWAP, 2014). Therefore, ensuring sufficient water resources, in the required quality as well as sustainable energy and food supply are essential and interconnected goals for sustaining human well-being (UNSD, 2018; Vörösmarty et al., 2018).
High water demand increases the risk that water of the required amount and quality will not be available at the time and place it is needed (Gupta and van der Zaag, 2008; Rodell et al., 2018). This calls for large-scale engineering solutions to store, redistribute and treat water resources. Hard infrastructure and engineering solutions are often considered as a first option, not considering viable alternatives or combinations of gray and green (natural or seminatural features) infrastructure that may ensure a more sustainable use of water resources (Palmer et al., 2015; Vörösmarty et al., 2018).
Megaprojects are often high-risk projects because they require major financial investments, demand long time frames from planning to completion, and may have major socio-economic and environmental ramifications (Flyvbjerg, 2014; Sternberg, 2016). In the water sector, megaprojects include transfer projects, large dams, navigation schemes, desalination plants, treatment plants, and ecosystem restoration projects (Sternberg, 2016; Tockner et al., 2016). Megaprojects are often initiated as an expression of national and political power and expected to trigger economic and social development (Sternberg, 2016). Concurrently, the social, economic and environmental consequences of these projects do not receive adequate attention in the decision-making process (Sternberg, 2016; Zhuang, 2016).
Water transfer megaprojects (WTMP) may play an important role in sustaining the water-food-energy nexus, as they can provide water for irrigation, domestic supply, energy production, navigation, and industrial development (Sternberg, 2016). The common term is interbasin water transfer, defined as “the transfer of water from one geographically distinct river basin to another, or from one river reach to another”; hereafter called “donor” and “recipient” system, respectively (Davies et al., 1992; Gupta and van der Zaag, 2008). According to the International Commission on Irrigation and Dams (ICID, 2005), interbasin water transfer accounted for 540 km3 a−1 or 14% of the global water withdrawals as for 2005, although these values should be used with caution due to major uncertainties in the underlying data. Global water withdrawal through transfer schemes is expected to increase by 25% until 2025 (Gupta and van der Zaag, 2008), primarily through an expansion of water transfer schemes. In the USA, for example, the number of interbasin water transfer schemes (primarily ordinary transfer projects of small scale) has increased by an order-of-magnitude, from 256 in 1985/1986 to 2,161 in 2017 (Dickson and Dzombak, 2017).
Concern about the environmental, societal and economic consequences of interbasin water transfers has been raised in recent periods (WWF, 2007; Zhang et al., 2015; Zhuang, 2016 and examples therein). While it has been shown that water transfer schemes can reduce the pressure on groundwater resources (Poland, 1981), improve water quality (Hu et al., 2008; Rivera-Monroy et al., 2013), and support ecosystem restoration measures (Snedden et al., 2007; Dadaser-Celik et al., 2009); there are concerns about their impacts. For example, WTMP may cause high levels of evaporative losses and rates of leakage due to poor maintenance of infrastructure (Davies et al., 1992), provoke salinization due to reduced water flow (Zhuang, 2016), increase nutrient concentrations due to inputs from nutrient-rich basins (Fornarelli and Antenucci, 2011; Jin et al., 2015), facilitate the spreading of pollutants and invasive species (Murphy and Rzeszutko, 1977; O'Keeffe and DeMoor, 1988; Snaddon and Davies, 1998; Clarkson, 2004), and change species composition (Grant et al., 2012; Lin et al., 2017).
From a social point-of-view, WTMP can alter the water balance in the affected basins, with potential beneficial or negative effects for human well-being in the donating and receiving basins. Due to increased water supply, residents in receiving basins may benefit from boosted agricultural and industry development, while environmental deterioration in donating basins may lead to a reduction in income and lead to involuntary or uncompensated resettlement of local communities (Sternberg, 2016; Yu et al., 2018).
Water transfer may also increase the probability of conflicts between countries that share water basins. For example, water transfer from non-renewable waters of the Disi aquifer by Jordan and Saudi Arabia led to concerns related to over-exploitation of commonly shared groundwater and a potential “tragedy of commons” (Müller et al., 2017). Inappropriate planning of water transfer schemes can also lead to major economic failures; for example, when high construction costs lead to increased water prices that exceed the paying ability of target groups (Sternberg, 2016).
Comprehensive data and information on the global extent of future WTMP are currently lacking (Tockner et al., 2016). Design, construction, and commencement of megaprojects require time, money and technical skills (Flyvbjerg, 2014). WTMP that are currently in the proposing, planning or construction stages may require decades until completion. Indeed, some projects may stay on the stage of a preliminary proposal, without any plan actually developed or funding assigned. However, knowing their distribution and key characteristics will help coping with the challenges humans and freshwater ecosystems are facing, and support appropriate, and alternative, strategies for managing water resources and ecosystem processes under rapidly changing conditions (Shumilova, 2018).
The aim of this study was to collate data and information about WTMP that are currently proposed, planned or under construction globally, and to be potentially completed by the year 2050.
The key research questions are:
(1) What is the global distribution of WTMP proposed, planned or under construction?
(2) Which purposes will future WTMP fulfill, particularly in meeting the water-food-energy nexus?
(3) How much water will be transferred across which distances?
(4) What are the estimated financial costs of future WTMP realization (including design and construction)?
In addition, we collected information on the distribution and key characteristics of existing WTMP, in order to put both existing and future WTMP into context. Finally, we discuss the consequences WTMP may cause in affecting humans and nature alike.
Water transfer projects include any type of infrastructure that transfers water from one river catchment to another, from one river reach to another, or from any freshwater body (river, lake, groundwater source) to a place where it will be utilized by humans (Davies et al., 1992; Gupta and van der Zaag, 2008). Megaprojects are generally defined based on actual construction costs, with a threshold of about one billion US$ per project (Flyvbjerg, 2014). We extended that definition for water transfer megaprojects to include projects that meet one, or more, of the following criteria: construction costs amount to one billion US$ or more, distance of transfer is 190 km or more, or volume of water transferred exceeds 0.23 km3 a−1 (Shumilova, 2018). To set these criteria we first selected a sample of 13 WTMP planned or under construction with the estimated construction cost of 1 ± 0.5 billion US$. Then, we calculated the median water transfer distance and volume of these projects (Table S1). These criteria were used to identify existing megaprojects, too.
We collected data and information on all megaprojects based on peer-reviewed publications, official web-sites of water transfer projects, environmental impact assessments, reports of non-governmental organizations, and information available in online newspapers. Data and information were collected between January and December 2017. We searched for the English terms “water transfer,” “water diversion,” “water megaproject,” and “water redistribution schemes,” using the following search engines: www.webofscience.com; https://scholar.google.com/; and www.google.com. In order to improve the data quality, we used multiple sources for each project for cross-validation (the full list of information sources for each project planned and under construction is provided in the Supplementary Material).
For each project, we compiled the following data and information: geographic location of the project (continent, country), project status (proposed, planned, under construction), donor and recipient system, total water transfer distance, total water transfer volume (i.e., maximum annual capacity), estimated financial construction costs (future WTMP), and main purpose(s) of the project. In case information sources provided different values on water transfer distance, volume and costs, we used the largest values found in the literature. We visualized the location of each project using QGIS software (version 2.12). Identification of the location and course of the planned WTMP was based on available project plans, terrain topography, or depicted as the shortest connection between donating and receiving water body in case no other information was available.
A total of 34 existing WTMP were identified, with the majority of projects located in North America (17) and Asia (10) (Figure 1A, Table S2). A total of 76 WTMP are either under construction (25 projects) or in the planning phase (51) (Figure 1B; Table S3). The majority of future WTMP will be located in North America (33 projects) and Asia (18) (Figure 1B; Table 1). In Europe, only three WTMP are expected so far, of which two are under construction.
Figure 1. Global distribution of (A) existing water transfer megaprojects (black lines) (N = 34) and (B) future water transfer megaprojects that are under construction (red lines) or in the planning phase (green lines) (Ntotal = 76). Blue lines show major rivers.
Table 1. Summary information (per continent) on water transfer megaprojects, either proposed, planned or under construction (see text for further explanation).
Two of the future projects will transfer water from aquifers (Disi Water Conveyance Project in Jordan and a pipeline from an aquifer in Eastern Nevada to Las Vegas, USA), and all others will transfer water from river systems through canals or pipelines. Among future projects we also distinguished 24 projects defined as “proposed,” without further commitments at this stage (Table S4); although data should be treated with caution (see description of “zombie-projects” in section Global scale inventory on WTMP). Most of the proposed projects are located in North America (20), three in Australia, and one in Asia.
The inventory of WTMP purposes showed that both existing and future projects represent an important infrastructure in supporting many of the water-food-energy nexus developments. Among existing WTMP, twelve projects provide water for irrigation, seven for hydropower generation, four for both purposes, and one project serves ecosystem restoration (Table S2). Among future projects, 42 projects will transfer water for agriculture development (19 in North America, 8 in Asia and Africa, 3 in Australia and South America, 1 in Europe), 13 for hydropower generation (7 in North America, 3 in Africa, 2 in Asia, 1 in Europe), and ten for both purposes (Figure 2). Furthermore, six future WTMP will meet the needs of the mining industry, five will support ecosystem restoration, and three projects will serve as navigation canals (Table S3).
Figure 2. Distribution of future WTMP according to their purposes: water supply for purposes of agriculture (green lines, N = 43), hydropower development (orange lines, N = 13) or both (orange-green stripped lines, N = 10). Blue lines show major rivers.
For existing WTMP, the water transfer volume ranged from 0.06 to 51 km3 a−1 (median: 2.4 km3 a−1), with a combined volume of 204 km3 a−1 (Table S2). The “James Bay Project” (Canada; 51 km3 a−1) and the “Goldfields Water Supply Scheme” (Australia; 33 km3 a−1) transfer the largest volumes. For future WTMP, the estimated water volume transferred per project will range from 0.05 to 317 km3 a−1 (median: 2.2 km3 a−1), with a combined volume of 1,910 km3 a−1 (Table 1). The proposed “North American Water and Power Alliance” (NAWAPA) megaproject is estimated to transfer 193 km3 a−1 across the entire continent, and the proposed “Great Recycling and Northern Development (GRAND) Canal of North America” may transfer 317 km3 a−1.
The water transfer distance of existing WTMP ranged from 0.4 to 2,820 km (median: 358 km) with a combined length of 13,049 km (Table 1). The longest distance of water transfer amounts to 2,820 km for the “Great Manmade River” (Libya) and the California State Water Project (USA; 1,128 km). The calculated water transfer distance of future WTMP will range from 17 km to 14,900 km (median: 482 km) (Table S3). The combined length of all megaprojects proposed or planned (56,115 km) or under construction (24,281 km) will amount to 80,396 km. Thereof, the “National River Linking Project” (India), which is under construction, will stretch a total length of 14,900 km, and the proposed “NAWAPA” megaproject (North America) will cover 10,620 km.
The construction costs (actual estimates) of future WTMP range from 0.095 to 1,500 billion US$ per project (median: 5.2 billion US$) (Table 1). The construction of all future 76 WTMP will require a combined investment of around 2.7 trillion US$. The construction of the proposed “NAWAPA” megaproject is estimated to cost 1.5 trillion US$. Regarding the projected costs per km of water transfer, the most expensive projects currently in the planning phase are the “California Water Fix and Eco Restore” project (USA; 479 million US$ per km), the Acheloos River diversion project (Greece; 339 million US$ per km) and the New Valley Project (Toshka Project) (Egypt; 290 million US$ per km). Regarding the costs of transfer in relation to the water volume transferred, i.e., costs per millions of m3 a−1, the calculated costs are highest for the channel connecting Lake Baikal (Russia) with the Chinese city Lanzhou (325 million US$ per million m3 a−1), the pipeline connecting the underground aquifer in eastern Nevada with Las Vegas (USA; 97 million US$ per million m3 a−1), and the Kimberley-Perth canal (Australia; 73 million US$ per million m3 a−1); all of which are in the planning phase.
In this paper, we presented the most comprehensive global synthesis on future WTMP, which are expected to be completed by around 2050 as well as on the key characteristics of each of these projects. The inventory shows that WTMP already are and will become even more of a global phenomenon. They are planned across all continents and in countries that are both developed (e.g., USA) and developing (e.g., India, China) in terms of industrial status and per capita income.
By building massive water transfer infrastructures, humans are creating “artificial rivers” on Earth. If all planned projects are completed, the water transferred will encompass a total volume of up to 1,910 km3, equivalent to over 26 times the mean annual flow of the Rhine River, and will travel a total distance of twice the length of Earth's equator. For comparison: the mean annual flow at the mouth of the Rhine River, one of the longest (total length: 1,250 km) and economically most important rivers in Western Europe, amounts to 72 km3 a−1 (Uehlinger et al., 2009). While the median water transfer distance per individual project will be around one third of the Rhine River length, 17 projects will exceed the length of the river Rhine. The scale of these interventions means that they may fundamentally transform the global water cycle. The total volume of transferred water will account for up to 48 % of the global water withdrawal (based on the recent total withdrawal rate of around 4,000 km3 year−1 FAO, 2010), and to about 5 % of the total global continental discharge to oceans (Table 2). Indeed, we can expect an even greater increase because our analysis includes megaprojects only. For example, in the USA we identified nine existing megaprojects, while a recent inventory of the total number of interbasin transfer projects includes 2,161 smaller projects (Dickson and Dzombak, 2017; Table S2).
Table 2. Water volumes transferred in future WTMP vs. volumes of continental water withdrawals and total discharge to oceans (per continent).
In most cases, water transfer occurs between hydrologically very different regions, i.e., from water rich to xeric areas, reconfiguring the conception and use of desert lands (e.g., Sternberg, 2016). Water is taken to serve demands of distant populations. Among such projects are the New Valley (Toshka) Project (water transfer from Lake Naser) and El Salam Project (water transfer from Nile) in Egypt for the needs of agriculture and industry in xeric areas, the Disi Water Conveyance Project (water transfer from Disi Aquifer to Amman, the capital of Jordan), and the water transfer pipeline from the aquifer in Eastern Nevada for water needs in Las Vegas. Water is also transferred to develop agricultural and economic resources, like the proposed Bradfield Scheme in Australia (water transfer from Tully, Herbert and Burdekin rivers to irrigate dry parts of Queensland and to create a lake in the middle of the continent) or the proposed Sibaral canal that aims to refill the Aral Sea. Such a redistribution of water can exacerbate disparities between water rich and water poor areas, especially in view of projected changes in freshwater availability under climate change (Rodell et al., 2018).
A significant number of future megaprojects (15 in total, Figure 3) are transboundary and will transfer water across longer distances compared to existing projects. The median water transfer distance of future WTMP will exceed those of existing projects by more than 100 km, although the median water transfer volume of existing and future WTMP is very similar (2.4 vs. 2.2 km3 a−1, respectively). Among 76 future projects, 23 will transfer water further than 1,000 km, compared to two out of 34 existing projects. The volume and in particular the distance of future WTMP emphasize that these projects must be considered as integral parts of the global hydrosystem network, and therefore included in hydrological models.
Figure 3. Distribution of future WTMP under construction (red lines) or planned (yellow lines) across major river basins. Dark blue color shows major basins affected by water transfer, light blue shows non-affected basins. Black lines show countries boundaries.
Currently, there is no dedicated agency responsible for maintaining a database on water transfer projects, not even in countries where water transfer already is an important component of water supply, such as in the United States and China (Dickson and Dzombak, 2017; Yu et al., 2018). Furthermore, we lack internationally agreed standards to evaluate water transfer project design, performance and impacts on people and ecosystems, as have been created for large dams (World Comission on Dams, 2000; HSAP, 2010; Roman, 2017).
Our dataset contains the most comprehensive existing global collation of information on existing and future WTMP. However, we are aware that the quality and completeness of information should be treated with caution because of the heterogeneity of information on projects' characteristics. Only English search terms were applied for data acquisition, which potentially may lead to an incomplete representation of existing and future projects in certain regions, in particular in Asia and Latin America. In addition, in our database we included projects that have been proposed, but have not become a subject of further commitments, and their realization is still questionable.
Several future projects included in our inventory are so-called “zombie-projects” (Gleick et al., 2014). They were once proposed, were put on hold or set aside, but then brought back to life. According to our database, most of such projects were proposed in North America in the late 1950s and early 1960s with the aim to transfer water from northern regions of the continent (particularly in Canada) to southern parts in the United States and Mexico by building canals (Forest and Forest, 2012). For example, the NAWAPA project in North America was first proposed in 1954 and discussed again in 2010s (Nuclear NAWAPAXXI, 2013). Another example is the Sibaral Project (2,500 km long of water transfer from Siberian rivers to the Aral Sea), which was proposed during the Soviet Union era, stopped in 1986, and recently discussed again among various actors in Central Asia and Russia (Pearce, 2004). Their realization cannot be dismissed, however, as extreme droughts, natural disasters, or famines may open so-called “windows-of-opportunities” to move forward on their construction (Tockner et al., 2016). At the same time, these projects are connected with massive environmental, social, and economic interventions and therefore in most cases environmentally and economically unsustainable (Flyvbjerg, 2014; Sternberg, 2016; Zhuang, 2016).
Data on expected costs of WTMP show that these projects will require enormous and in most cases underestimated investments. The construction costs of all future WTMP (with information on costs available) will amount to more than 2.7 trillion US$ (actual estimates), which exceeds the calculated investments for constructing 3,700 large hydropower dams, either planned or under construction (Zarfl et al., 2015). The median costs of a single WTMP (5.2 billion US$) can comprise a significant proportion of the annual GDP of individual countries (for comparison, the total annual GDP of Greece is 196 billion US$ World Economic Outlook Database, 2017). In China, the estimated expenses on water transfer projects, both completed and planned as for 2015, accounted for around 1% of the country's GDP in 2014, corresponding to more than 150 billion US$ (average costs per project: 3.5 billion US$; Yu et al., 2018). High costs together with cost overruns, however, can lead to financial failures of megaprojects (Sternberg, 2016). For example, the Central Arizona Project (USA), completed in 1992, provided farmers with irrigation waters for very high fees, but investments in the project have still not been covered (Sternberg, 2016). Estimated expenses of WTMP increase while projects are under construction. The costs of the Sao Francisco irrigation project (Brazil), currently under construction, have increased from initially 4.5 to more than 10 billion US$, and may further increase until completion; while running costs are not yet included (Roman, 2017). Expenses on water transfer often compete with other societal requirements. For example, 4% of the GDP of Saudi Arabia are dedicated to sustaining water resources, compared to 8% for health and social affairs (Ministry of Finance, Saudi Arabia, 2013). Apart from financial costs related to project construction, costs related to environmental damage and social issues need to be considered too. For example, the construction of the 1,000 km long Yettinahole Diversion Project in India will lead to the deterioration of one of the world's biodiversity hot spots (Krishnadas and Jumani, 2017). Furthermore, diversion projects will also affect the water supply of downstream communities. Therefore, overall megaproject benefits should be compared to costs under different scenarios for the use of water and resources in view of multiple values dimensions (e.g., Hansjürgens et al., 2016).
WTMP offer engineering solutions in meeting increasing water needs (Gupta and van der Zaag, 2008) and are part of national water management plans. The development of future WTMP is mainly driven by geographical or temporal limitations in water availability (e.g., large water volumes planned to be transferred from water secure areas to arid regions) as well as by existing deficits in water supply that limit further economic development (e.g., transfer schemes to provide water for mining schemes in Chile and Australia). Future WTMP are also proposed to facilitate the economic linkage of regions (e.g., navigation canals in South America and Africa). Some projects aim to provide water supply for particular cities (e.g., water transfer from the aquifer in East Nevada to Las Vegas, water transfer from Lake Baikal to the Chinese city Lanzhou). Currently, 12% of the largest cities in the world (with a population larger than 750,000 people) are dependent on interbasin water transfer, and the number of cities relying on transferred water is increasing (McDonald et al., 2014). In the next decades, further expansion of urban infrastructure is expected, particularly in developing countries (McDonald et al., 2014). The fastest growing large cities dependent on water transfer are located in China, India, and Mexico (McDonald et al., 2014).
Future WTMP will play a significant role in the water-food-energy nexus and this approach therefore could facilitate the resolution of some of the approval processes regarding realization of projects and their expected dimensions. We identified that the majority of projects is supporting the agricultural sector. The Aquatacama Project, which will transfer around 1.5 km3 a−1 over a distance of 2,500 km from the south to the north of Chile, is expected to double its area of agricultural land and food production (Dourojeanni et al., 2013). Very large-scale projects proposed in North America as NAWAPA, PLHINO, and PLHIGON will jointly form a single water transfer network, boosting food production in Mexico. The area of irrigated land in Mexico will increase by 75% and grain production will be doubled (Small, 2007). Finally, the South-to-North water transfer project in China provides water for agriculture and domestic use in the densely populated areas in Northern China. A number of projects will also serve multiple purposes including providing water for agriculture, energy supply and domestic purposes. For example, Turkey, a country with the second largest hydropower potential in Europe (following Norway; Yuksel, 2015), demonstrates how water transfer schemes will support both the energy and agricultural sectors. Within the Southeastern Greater Anatolian Project (GAP), for example, 22 dams and 19 hydroelectric power plants will be constructed along the Tigris and Euphrates Rivers. After completion, the project will provide 308 MW for electricity production (45% of the total economically exploitable hydroelectric potential in Turkey) and irrigate 1.8 million ha of land, with a total length of irrigation channels of 1,032 km (Yuksel, 2015). In Egypt and Sudan, within the scope of the New Nile Project, a 2,500 km long canal will be built to provide water for agriculture and to provide a capacity of 18 GW for electricity production (Al-Naggar, 2014).
However, WTMP can cause undesirable social and economic consequences, particularly when projects with underestimated costs and overestimated benefits are approved (Flyvbjerg, 2007). Water usage can be unsustainable when water is transferred to promote agriculture in water-poor areas. For example, the Central Arizona Project (USA) supports water-intensive cotton growth in the semiarid Phoenix region. Another example is the Great Manmade River Project (Libya), which transfers groundwater from the Sahara to the Mediterranean coast, facilitating the migration of people to the desert, further increasing the pressure on already scarce water resources there (Sternberg, 2016). In addition, many of the future WTMP are transboundary and are planned in countries that are less stable politically and economically. This may lead to international disputes in water issues (Tockner et al., 2016). For example, the current conflict between the Russian Federation and the Ukraine led to the closure of the existing North-Crimean canal in 2014, which was playing a crucial role for sustaining agriculture and domestic water supply on the Crimean peninsula, supplying 85% of water needs (Vasilenko, 2017). This resulted not only in the failure of agriculture and other sectors of the local economy, but also in significant ecological damages of aquatic ecosystems in Crimea, namely the salinization of the Sivash Bay after water transfer was stopped (Shadrin et al., 2018). Another example is the Southeastern Greater Anatolian Project (GAP). Although it will support water development in Turkey, water security will be negatively affected in downstream countries such as Syria and Iraq, causing economic impacts, large-scale migration, and thus affecting the geopolitical situation in the region, especially in combination with climate change (Feitelson and Tubi, 2017; Rodell et al., 2018).
Environmental impacts of individual interbasin transfer projects have been analyzed in multiple studies (Zhuang, 2016 and references therein), and the impacts of megaprojects in general are likely to be similar, albeit at a grander scale given their size. Most of the projects have already raised various discussions among stakeholders, pointing out that benefits of water transfer projects are overestimated, while costs are underestimated (WWF, 2007). An example of a future project that has caused concern about potential impacts is the “Acheloos Diversion” project (Greece; under construction) that was dubbed a “Modern Greek Drama” (Tyralis et al., 2017) and which may cause irreversible damage to ecosystems containing internationally protected species (WWF, 2007). Another example is the Sao Francisco irrigation project (Brazil), which is expected to increase desertification and cause salinization of irrigated soils due to increased evapotranspiration, lead to biodiversity loss, fragmentation of native vegetation, and disrupt fishing due to more dams (Stolf et al., 2012). Although the National Integration Ministry claimed that environmental impacts of the Sao Francisco project will be minimal, opponents of the project included state government institutions of the proposed donor basins, technical councils, and churches. On the other hand, some of the future WTMP have the objective of restoring ecosystems. For example the “Transaqua” project is expected to refill Lake Chad and the “Comprehensive Everglades Restoration Plan” is expected to restore the hydrology of one of the most important wetlands globally (Ifabiyi, 2013; CERP, 2015).
Globally, WTMP will redistribute large volumes of water between distantly located catchments, in particular in Asia and North America (Figure 3), thereby changing the hydrological balance. Large water withdrawals can lead to a flow reduction in donating basins. For example, the annual flow of the Yellow River in China was reduced by 10% in 2013, compared to the average flows within the last 60 years due to average withdrawal of 3.3 km3 a−1 (Yu et al., 2018). In many cases, however, extraction of streamflow from the donating basins is not significant. For example, half of the interbasin transfer schemes that existed in the US in 1973–1982 extracted 0.04%, and 78% of the projects < 1% of annual streamflow from the donating basins (Emanuel et al., 2015). However, under drought condition the percentage of withdrawal can be significantly higher. Overall, water transfer between wet and dry catchments will lead to a flow homogenization at regional and continental scales, but solid data to underpin this observation are still missing (McDonald et al., 2014).
Overall, the effects on freshwater ecosystems need to be estimated individually for each project. In general, the extent of the effects will depend on the physical and biological characteristics of the donating and recipient systems, the types of connecting and storage structures (pipelines or open canals, dams or natural infrastructure), the volume of water transferred and the frequency of transfers (Soulsby et al., 1999; Gibbins et al., 2001; Fornarelli and Antenucci, 2011). The current inventory of future WTMP (see Table S3) can serve to identify potential impacts on freshwaters by overlapping the WTMP data with other datasets (e.g., with hot-spots of biodiversity, water quality in donating, and receiving basins).
Within the next decades, we may expect up to a 9-fold increase in the volume of water transferred by WTMP if all planned projects are completed. As water scarcity becomes a global phenomenon, WTMP are currently considered to be an engineering solution to meet increasing water demands in both developed and developing countries. While these projects may play a fundamental role in food and energy production, there are concerns about their social, environmental and economic costs. Even projects which seem to be both environmentally and economically unsustainable could be implemented if the facilitating economic and political conditions prevail.
Presently, the lack of reliable data does not allow a full evaluation of the environmental, social, and economic potential impacts of future WTMP. Projects costs need to be integrated into the context of estimated benefits. The size of these WTMP suggests, however, that their impacts will cover regional and continental scales and will be irreversible. Thus, it is recommended that natural or green infrastructure solutions be seriously considered as alternatives or part of a gray-green infrastructure combined solution (e.g., Palmer et al., 2015). For example measures such as using recycled water, improving piping, and distribution in existing systems, using natural wetlands or groundwater systems as storage systems and increasing the efficiency of irrigation for agricultural purposes should come first in addressing the challenges of water shortage, although they may not eliminate the problem completely considering its scale.
Overall, the results of the inventory of WTMP emphasize the need to include these projects in global hydrological models and to develop internationally agreed criteria for their multiple assessments. Otherwise, we are facing an engineered water future, which may constrain alternative solutions to cope with an increasingly uneven distribution, both in space and time, of the global water resources. We need to manage our hydrological systems as hybrid systems–as regional water resources for human use as well as highly valuable ecosystems, for the benefit of people and nature alike.
OS, KT, and CZ designed the study. OS, AK, and CZ collected information. OS compiled the manuscript and all co-authors contributed to the text.
The authors declare that the research was conducted in the absence of any commercial or financial relationships that could be construed as a potential conflict of interest.
This work had been carried out within the SMART Joint Doctorate Programme Science for the Management of Rivers and their Tidal systems, funded by the Erasmus Mundus programme of the European Union (http://www.riverscience.it). OS is thankful for a partial support from IGB equal opportunity fund for young female scientists and DFG (SU 405/10-1). We also acknowledge support by the German Research Foundation (DFG) and the Open Access Publishing Fund of the University of Tübingen. The authors thank Dominik Reiner for his support in cross-checking the compiled data on future WTMP, and three reviewers for their very helpful comments. The content of this manuscript is also part of the doctoral thesis of OS as listed in the reference list. The submitted manuscript is published as a preprint version on the EarthArXiv preprint server (Shumilova, O., Tockner, K., Thieme, M., Koska, A., Zarfl, C. (2018). Global water transfer megaprojects: A solution for the water-food-energy nexus? EarthArXiv [Preprint]. doi: 10.31223/osf.io/ymc87).
The Supplementary Material for this article can be found online at: https://www.frontiersin.org/articles/10.3389/fenvs.2018.00150/full#supplementary-material
2030 WRG (2009). 2030 Water Resources Group. Charting our water future: Economic frameworks to inform decision-making. Available online at: https://www.mckinsey.com/~/media/mckinsey/dotcom/client_service/sustainability/pdfs/charting%20our%20water%20future/charting_our_water_future_full_report_.ashx
Al-Naggar, A. (2014). Fact and Fiction: Diverting Water From the Congo to the Nile. Available online at: http://english.ahram.org.eg/News/100748.aspx
Brauman, K. A., Richter, B. D., Postel, S., Malsy, M., and Flörke, M. (2016). Water depletion: an improved metric for incorporating seasonal and dry-year water scarcity into water risk assessments. Elem. Sci. Anth. 4:p.000083. doi: 10.12952/journal.elementa.000083
CERP (2015). Comprehensive Everglades Restoration Plan. Everglades National Park Service. Available online at: https://www.nps.gov/ever/learn/nature/cerp.htm
Clarkson, R. W. (2004). Effectiveness of electrical fish barriers associated with the central arizona project. North Am. J. Fish. Manage. 24, 94–105. doi: 10.1577/M02-146
Dadaser-Celik, F., Coggins, J. S., Brezonik, P. L., and Stefan, H. G. (2009). The projected costs and benefits of water diversion from and to the Sultan Marshes (Turkey). Ecol. Econ. 68, 1496–1506. doi: 10.1016/j.ecolecon.2008.10.012
Davies, B. R., Thoms, M., and Meador, M. (1992). The ecological impacts of inter-basin water transfers and their threats to river basin integrity and conservation. Aquatic Conserv. Marit. Freshwater Ecosyst. 2, 325–349. doi: 10.1002/aqc.3270020404
Dickson, K. E., and Dzombak, D. A. (2017). Inventory of interbasin transfers in the United States. J. Am. Water Resour. Assoc. 53, 1121–1132. doi: 10.1111/1752-1688.12561
Dourojeanni, A., Jadue, N., León, G., Osborne, K., and Serra, D. (2013). Aquatacama Project: Preliminary Socio-Economic Analysis. Fundacion Chile. Available online at: http://www.acquatacama.cl/sites/default/files/AQUATACAMA%20REPORT%20FINAL%20-%20F.%20Chile.pdf
Emanuel, R. E., Buckley, J. J., Caldwell, P. V., McNulty, S. G., and Sun, G. (2015). Influence of basin characteristics on the effectiveness and downstream reach of interbasin water transfers: displacing a problem. Environ. Res. Lett. 10, 124005–124013. doi: 10.1088/1748-9326/10/12/124005
FAO (2010). Food and Agriculture Organization of the United Nations. Water Withdrawal By Sector, Around 2010. Available online at: http://www.fao.org/nr/water/aquastat/tables/WorldData-Withdrawal_eng.pdf
FAO (2011). Food and Agriculture Organization of the United Nations: The State of the World's Land and Water Resources for Food and Agriculture(SOLAW) – Managing Systems at Risk. Available online at: http://www.fao.org/3/a-i1688e.pdf
FAO, IFAD, UNICEF, WFP WHO. (2017). The State of Food Security and Nutrition in the World 2017. Building Resilience for Peace and Food Security. Available online at: http://www.fao.org/3/a-I7695e.pdf
Feitelson, E., and Tubi, A. (2017). A main driver or an intermediate variable? Climate change, water and security in the Middle East. Glob. Environ. Change 44, 39–48. doi: 10.1016/j.gloenvcha.2017.03.001
Fekete, B. M., Vörösmarty, C. J., and Grabs, W. (2002). High-resolution fields of global runoff combining observed river discharge and simulated water balances. Glob. Biogeochem. Cycles 16, 1–6. doi: 10.1029/1999GB001254
Flyvbjerg, B. (2007). Policy and planning for large-infrastructure projects: problems, causes, cures. Environ. Plann. B Plann. Des. 34, 578–597 doi: 10.1068/b32111
Flyvbjerg, B. (2014). What you should know about megaprojects and why: an overview. Project Manage. J. 45, 6–19. doi: 10.1002/pmj.21409
Forest, B., and Forest, P. (2012). Engineering the North American waterscape: the high modernist mapping of continental water transfer projects. Polit. Geogr. 31, 167–183. doi: 10.1016/j.polgeo.2011.11.005
Fornarelli, R., and Antenucci, J. P. (2011). The impact of transfers on water quality and the disturbance regime in a reservoir. Water Res. 45, 5873–5885. doi: 10.1016/j.watres.2011.08.048
Gibbins, C. N., Soulsby, C., Jeffries, M. J., and Acornley, R (2001). Developing ecological acceptable river flow regimes: a case study of Kielder reservoir and the Kielder water transfer system. Fish. Manag. Ecol. 8, 463–485. doi: 10.1046/j.1365-2400.2001.00274.x
Gleick, P., Heberger, M., and Donnelly, K. (2014). “Zombie water projects,” in The World's Water Volume 8: The Biennial Report on Freshwater Resources, ed Gleick, P (Washington/Covelo/London: Island Press/Center for Resource Economics), 123–146.
Grant, E. H., Lynch, H. J., Muneepeerakul, R., Arunachalam, M., Rodriguez-Iturbe, I., et al. (2012). Interbasin water transfer, riverine connectivity, and spatial controls on fish biodiversity. PLoS ONE 7:e34170. doi: 10.1371/journal.pone.0034170
Gupta, J., and van der Zaag, P. (2008). Interbasin water transfers and integrated water resources management: where engineering, science and politics interlock. Phys. Chem. Earth 33, 28–40. doi: 10.1016/j.pce.2007.04.003
Haddeland, I., Heinke, J., Biemans, H., Eisner, S., Flörke, M., Hanasaki, N., et al. (2014). Global water resources affected by human interventions and climate change. PNAS 111, 3251–3256. doi: 10.1073/pnas.1222475110
Hansjürgens, B., Droste, N., and Tockner, K. (2016). “Neglected values of major water engineering projects: ecosystem services, social impacts, and economic valuation,” in Society-Water-Technology, eds R. F. Hüttl, O. Bens, C. Bismuth, S. Hoechstetter (Heidelberg: Springer), 65–78
HSAP (2010). Hydropower Sustainability Assessment Protocol. Available online at: http://www.hydrosustainability.org/Protocol/The-Protocol-Documents.aspx
Hu, W., Zhai, S., Zhu, Z., and Han, H. (2008). Impacts of the Yangtze River water transfer on the restoration of Lake Taihu. Ecol. Eng.34, 30–49. doi: 10.1016/j.ecoleng.2008.05.018
ICID (2005). International Commission on Irrigation and Drainage. Experiences in inter-basin water transfers for irrigation, drainage or flood management (3rd draft 15 August 2005). Unpublished report.
Ifabiyi, I. P. (2013). Recharging the Lake Chad: the hydro-politics of national security and regional integration in Africa. Afr. Res. Rev. 7, 196–216. doi: 10.4314/afrrev.v7i3.15
Jin, Z., Chen, L., Li, F., Pan, Z., and Jin, M. (2015). Effects of water transfer on water quality and estimation of the pollutant fluxes from different sources into West Lake, Hangzhou City, China. Environ. Earth Sci. 73, 1091–1101. doi: 10.1007/s12665-014-3456-6
Krishnadas, M., and Jumani, S. (2017). The Wire. Why Diverting Yettinahole River is Both Ecologically Damaging and Economically Unsound. Available online at: https://thewire.in/102253/yettinahole-karnataka-bengaluru-chikkaballapur/ (Accessed September 28, 2018).
Lin, M. L., Lek, S., Ren, P., Li, S. H., and Li, W. (2017). Predicting impacts of South-to-North water transfer project on fish assemblages in Hongze Lake, China. J. Appl. Ichthyol. 33, 395–402. doi: 10.1111/jai.13251
McDonald, R. I., Weber, K., Padowski, J., Flörke, M., Schneider, C., et al. (2014). Water on an urban planet: urbanization and the reach of urban water infrastructure. Glob. Environ. Change 27, 96–105. doi: 10.1016/j.gloenvcha.2014.04.022
Ministry of Finance Saudi Arabia. (2013). Recent Economic Developments and Highlights of Fiscal Years 1434/1435 (2013) 2 & 1435/1436. Available online at: www.mof.gov.sa/english/downloadscenter/pages/budget.aspx (Accessed January 13, 2017).
Müller, M. F., Müller-Itten, M. C., and Gorelick, S. M. (2017). How Jordan and Saudi Arabia are avoiding a tragedy of the commons over shared groundwater. Water Resour. Res. 53, 5451–5468. doi: 10.1002/2016WR020261
Murphy, T. J., and Rzeszutko, C. P. (1977). Precipitation inputs of PCBs to Lake Michigan. J. Great Lakes Res. 3, 305–312.
Nuclear NAWAPAXXI (2013). Nuclear NAWAPA XXI: Gateway to the Fusion Economy. 21st century science and technology special report. Available online at: http://21stcenturysciencetech.com/Nuclear_NAWAPA_XXI/Nuclear_NAWAPA_sm.pdf
O'Keeffe, J. H., and DeMoor, F. C. (1988). Changes in the physico-chemistry and benthic invertebrates of the Great Fish River, South Africa, following an interbasin transfer of water. Regulat. Rivers Res. Manage. 2, 39–55.
Palmer, M. A., Liu, J., Matthews, J. H., Mumba, M., and D'Odorico, P. (2015). Manage water in a green way. Science 349, 584–585. doi: 10.1126/science.aac7778
Pearce, F. (2004). Russia Reviving Massive River Diversion Plan. New Scientist, 9 February 2004. Available online at: www.newscientist.com/article/dn4637 (Accessed July 22, 2018).
Poland, J. F. (1981). The Occurrence and Control of Land Subsidence Due to Ground-Water Withdrawal With Special Reference to the San Joaquin and Santa Clara Valleys, California. Ph. D. dissertation. Stanford University, USA.
Rivera-Monroy, V. H., Branoff, B., Meselhe, E. A., McCorquodale, A., Dortch, M., Steyer, G. D., et al. (2013). Landscape-level estimation of nitrogen loss in coastal Louisiana wetlands: potential sinks under different restoration scenarios. J. Coastal Res. 67, 75–87. doi: 10.2112/SI_67_6
Rockström, J., Falkenmark, M., Allan, T., Folke, C., and Gordonet, L. (2014). The unfolding water drama in the Anthropocene: towards a resilience-based perspective on water for global sustainability. Ecohydrology 7, 1249–1261. doi: 10.1002/eco.1562
Rodell, M., Famiglietti, J. S., Wiese, D. N., Reager, J. T., Beaudoing, H. K., Landerer, F. W., et al. (2018). Emerging trends in global freshwater availability. Nature 557, 651–659. doi: 10.1038/s41586-018-0123-1
Roman, P. (2017). The São Francisco Interbasin Water Transfer in Brazil: Tribulations of a Megaproject Through Constraints and Controversy. Water Alternatives 10, 395–419. Available online at: http://www.water-alternatives.org/index.php/alldoc/articles/vol10/v10issue2/361-a10-2-11/file
Schewe, J., Heinke, J., Gerten, D., Haddeland, I., Arnell, N. W., Clark, D. B., et al. (2014). Multimodel assessment of water scarcity under climate change. Proc. Natl. Acad. Sci. U.S.A. 111, 3245–3250. doi: 10.1073/pnas.1222460110
Shadrin, N. V., Anufriieva, E. V., Kipriyanova, L. M., Kolesnikova, E. A., Latushkin, A. A., Romanov, R. E., et al. (2018). The political decision caused the drastic ecosystem shift of the Sivash Bay (the Sea of Azov). Q. Int. 475, 4–10. doi: 10.1016/j.quaint.2017.12.009
Shiklomanov, I. A. (2000). Appraisal and assessment of world water resources. Water Int. 25, 11–32. doi: 10.1080/02508060008686794
Shumilova, O. (2018). Neglected Aspects in the Alteration of River Flow and Riverine Organic Matter Dynamic: A Global Perspective. [Doctoral dissertation]: Freie Universität Berlin.
Small, D. (2007). U.S. and Mexico: Cooperate On Great Water Projects. Executive Intelligence Review. Available online at: http://www.larouchepub.com/eiw/public/2007/eirv34n47-48-20071207/eirv34n47-48-20071207.pdf
Snaddon, C. D., and Davies, B. R. (1998). A preliminary assessment of the effects of a small South African inter-basin water transfer on discharge and invertebrate community structure. Regulat. Rivers Res. Manage. 14, 421–441.
Snedden, G. A., Cable, J. E., Swarzenski, C., and Swenson, E. (2007). Sediment discharge into a subsiding Louisiana deltaic estuary through a Mississippi River diversion. Estuar. Coast. Shelf Sci. 71, 181–193. doi: 10.1016/j.ecss.2006.06.035
Soulsby, C., Gibbins, C. N., and Robins, T. (1999). Inter-basin water transfers and drought management in the Kielder/Derwent system. J. Chart. Inst. Water Environ. Manage. 13, 213–223.
Sternberg, T. (2016). Water megaprojects in deserts and drylands. Int. J. Water Resour. Dev. 32, 301–320. doi: 10.1080/07900627.2015.1012660
Stolf, R., Piedade, S. M. D., Da Silva, J. R., Da Silva, L. C. F., and Maniero, M. A. (2012). Water transfer from Sao Francisco River to semiarid northeast of Brazil: technical data, environmental impacts, survey of option about the amount to be transferred. Engenharia Agricola 32, 998–1010. doi: 10.1590/S0100-69162012000600001
Tockner, K., Bernhardt, E. S., Koska, A., and Zarfl, C. (2016). “A global view on future major water engineering projects,” in Society-Water-Technology, eds R. F. Hüttl, O. Bens, C. Bismuth, S. Hoechstetter (Springer: Heidelberg), 47–64.
Tyralis, H., Tegos, A., Delichatsiou, A., Mamassis, N., and Koutsoyiannis, D. (2017). A perpetually interrupted interbasin water transfer as a modern Greek drama: assessing the Acheloos to Pinios interbasin water transfer in the context of integrated water resources management. Open Water 1, 113–128. Available online at: http://scholarsarchive.byu.edu/openwater/vol4/iss1/11
Uehlinger, U., Wantzen, K. M., Leuven, R. S. E. W., and Arndt, H. (2009). “The Rhine River Basin,” in Rivers of Europe, ed K. Tockner (London: Academic Press), 199–245.
UN (2014). World Urbanization Prospects: The 2014 Revision, Highlights. Available online at: https://esa.un.org/unpd/wup/publications/files/wup2014-highlights.pdf
UN (2017). World Population Prospects: The 2017 Revision, Key Findings and Advance Tables. Available online at: https://esa.un.org/unpd/wpp/publications/Files/WPP2017_KeyFindings.pdf
UNESCO-WWA (2012). The United Nations World Water Development Report 4: Managing Water under Uncertainty and Risk. Available online at: http://unesdoc.unesco.org/images/0021/002156/215644e.pdf#page=812
UNESCO-WWA (2014). The United Nations World Water Development Report: Water and Energy. Available online at: http://unesdoc.unesco.org/images/0022/002257/225741E.pdf
UNSD (2018). United Nations. The sustainable development goals report. Available online at: https://unstats.un.org/sdgs/files/report/2018/TheSustainableDevelopmentGoalsReport2018-EN.pdf
Vasilenko, V. A. (2017). Hydro-economic problems of Crimea and their solutions. Reg. Ekonom. Sotsiol. 4, 198–219. doi: 10.1134/S2079970516040146
Vörösmarty, C. J., Osuna, V. R., Cak, A. D., Bhaduri, A., Bunn, S. E., Corsi, F., et al. (2018). Ecosystem-based water security and the Sustainable Development Goals (SDGs). Ecohydrol. Hydrobiol. doi: 10.1016/j.ecohyd.2018.07.004. [Epub ahead of print].
Vörösmarty, C. J., McIntyre, P. B., Gessner, M. O., Dudgeon, D., Prusevich, A., Green, P., et al. (2010). Global threats to human water security and river biodiversity. Nature 467, 555–561. doi: 10.1038/nature09440
WEC (2013). World Energy Scenarios: Composing Energy Futures to 2050. Available online at: https://www.worldenergy.org/wp-content/uploads/2013/09/World-Energy-Scenarios_Composing-energy-futures-to-2050_Full-report.pdf
WEF (2011). World Economic Forum: Global Risks 2011, 6th Edn. Available online at: http://reports.weforum.org/wp-content/blogs.dir/1/mp/uploads/pages/files/risk-report-barometers-2011.pdf
World Comission on Dams (2000). Dams and Developemnt. A New Framamework for Decision-Making. Available online at: https://www.internationalrivers.org/sites/default/files/attached-files/world_commission_on_dams_final_report.pdf
World Economic Outlook Database (2017). Report for Selected Countries and Subjects. International Monetary Fund. Available online at: https://www.imf.org/external/pubs/ft/weo/2017/02/weodata/index.aspx
WWF (2007). Pipedream? Inter Basin Water Transfer and Water Shortages. Available online at: http://d2ouvy59p0dg6k.cloudfront.net/downloads/pipedreams_ibts_final_report_27_june_2007_1.pdf
Yu, M., Wang, C., Liu, Y., Olsson, G., and Wang, C. (2018). Sustainability of mega water diversion projects: experience and lessons from China. Sci. Total Environ. 619–620, 721–731. doi: 10.1016/j.scitotenv.2017.11.006
Yuksel, I. (2015). South-eastern anatolia project (GAP) factor and energy management in Turkey. Ener. Rep. 1, 151–155. doi: 10.1016/j.egyr.2015.06.002
Zarfl, C., Lumsdon, A. E., Berlekamp, J., Tydecks, L., and Tockner, K. (2015). A global boom in hydropower dam construction. Aquat. Sci. 77, 161–170. doi: 10.1007/s00027-014-0377-0
Zhang, L., Li, S., Loáiciga, H. A., Zhuang, Y., and Du, Y. (2015). Opportunities and challenges of interbasin water transfers: a literature review with bibliometric analysis. Scientometrics 105, 279–294. doi: 10.1007/s11192-015-1656-9
Keywords: water transfer, megaprojects, hydrology, water balance, water-food-energy nexus, biodiversity, water management
Citation: Shumilova O, Tockner K, Thieme M, Koska A and Zarfl C (2018) Global Water Transfer Megaprojects: A Potential Solution for the Water-Food-Energy Nexus? Front. Environ. Sci. 6:150. doi: 10.3389/fenvs.2018.00150
Received: 01 August 2018; Accepted: 28 November 2018;
Published: 12 December 2018.
Edited by:
Richard George Lawford, Morgan State University, United StatesReviewed by:
Balazs Miklos Fekete, City College of New York (CUNY), United StatesCopyright © 2018 Shumilova, Tockner, Thieme, Koska and Zarfl. This is an open-access article distributed under the terms of the Creative Commons Attribution License (CC BY). The use, distribution or reproduction in other forums is permitted, provided the original author(s) and the copyright owner(s) are credited and that the original publication in this journal is cited, in accordance with accepted academic practice. No use, distribution or reproduction is permitted which does not comply with these terms.
*Correspondence: Oleksandra Shumilova, c2h1bWlsb3ZhQGlnYi1iZXJsaW4uZGU=
Christiane Zarfl, Y2hyaXN0aWFuZS56YXJmbEB1bmktdHVlYmluZ2VuLmRl
†Present Address: Klement Tockner, Austrian Science Fund, Vienna, Austria
Disclaimer: All claims expressed in this article are solely those of the authors and do not necessarily represent those of their affiliated organizations, or those of the publisher, the editors and the reviewers. Any product that may be evaluated in this article or claim that may be made by its manufacturer is not guaranteed or endorsed by the publisher.
Research integrity at Frontiers
Learn more about the work of our research integrity team to safeguard the quality of each article we publish.