- Departamento de Ecología y Biología Animal, Universidad de Vigo, Vigo, Spain
Soil fauna is crucial to soil formation, litter decomposition, nutrient cycling, biotic regulation, and for promoting plant growth. Yet soil organisms remain underrepresented in soil processes and in existing modeling exercises. This is a consequence of assuming that much of the below-ground diversity is just ecologically “redundant” and that soil food webs exhibit a higher degree of omnivory. However, evidence is accumulating on the strong influence of abiotic filters (temperature, moisture, soil pH) and soil habitat characteristics in controlling their spatial and temporal patterns. From this, new emerging concepts such as “hot moments,” “biological accessibility,” and “trophic cascades” have been coined to enable plausible explanations of the observed faunal responses to environmental changes. Here, I argue that many of these findings are indeed “happy accidents” (i.e., “eureka discoveries”) that remain disjointed between disciplines, impeding us from making significant breakthroughs. Therefore, here I provide some new perspectives on soil fauna research and highlight some experimental approaches to better explore the great variety of organisms living in soils and their complex interactions. A more comprehensive and dynamic holistic approach is needed to couple soil pedological and biological processes and to combine current experimental and theoretical knowledge if we aim to improve our predictive capacities in determining the persistence of soil organic matter and soil ecosystem functioning.
Introduction
Soils are complex systems and their complexity resides in their heterogeneous nature: a mixture of air, water, minerals, organic compounds, and living organisms. The spatial variation, both horizontal and vertical, of all these constituents is related to soil forming agents varying at different scales (from micro- to macro-scales; Lin et al., 2005). Consequently, the horizontal patchy distribution of soil properties (soil temperature, moisture, pH, litter/nutrient availability, etc.) also drives the patchiness of the soil organisms across the landscape (Berg, 2012), and has been one of the main arguments for explaining the great diversity observed in soil communities (Nielsen et al., 2010). Furthermore, because soils also show vertical stratification of their elemental constituents (along the soil profile) as result of microclimate, soil texture, and resource quantity and quality differing between soil horizons, soil communities also change in abundance and structure with soil depth (Berg and Bengtsson, 2007).
In addition, because the majority of these organisms are aerobic, the amount of porous space, pore-size distribution, surface area, and oxygen levels are crucial to their life cycles and activities. The smallest creatures (microbes) use the micropores filled with air to grow, whereas other bigger animals require bigger spaces (macropores) or the water film surrounding the soil particles to move in search for food. Therefore, soil textural properties together with the depth of the water table are also important factors regulating their diversity, population sizes, and their vertical stratification. Ultimately, the structure of the soil communities strongly depends not only on the natural soil forming factors but also on human activities (agriculture, forestry, urbanization) and determines the shape of our landscapes, in terms of healthy or contaminated, pristine or degraded soils.
Since all these drivers of biodiversity changes also operate above-ground, it is expected that there must be some concordance of mechanisms regulating the spatial patterns and structure of both above- and below-ground communities. In support of this, a small-scale field study revealed that the relationships between environmental heterogeneity and species richness might be a general property of ecological communities (Nielsen et al., 2010). In contrast, the molecular examination of 17,516 environmental 18S rRNA gene sequences representing 20 phyla of soil animals covering a range of biomes and latitudes around the world indicated otherwise, and the main conclusion from this study was that below-ground animal diversity may be inversely related to above-ground biodiversity (Wu et al., 2011).
The lack of distinct latitudinal gradients in soil biodiversity contrasts with those clear global patterns observed for plants above-ground and has led to the assumption that they are indeed controlled by different factors (Bardgett and van der Putten, 2014). For example, Lozupone and Knight (2007) found that salinity was the major environmental determinant of bacterial diversity composition across the globe (rather than extremes of temperature, pH, or other physical and chemical factors). Similarly, in another global scale study, Tedersoo et al. (2014) concluded that fungal richness is causally unrelated to plant diversity and is better explained by climatic factors, followed by edaphic and spatial patterns. Global patterns of the distribution of macroscopic organisms are far poorer documented. However, the little evidence available appears to indicate that, at large scales, soil metazoans respond to altitudinal, latitudinal or area gradients in the same way as those described for above-ground organisms (Decaëns, 2010). In contrast, at local scales, the high diversity of microhabitats commonly found in soils provides the required niche portioning to create “hot spots” of diversity in just a gram of soil (Bardgett and van der Putten, 2014).
Not only spatial patterns of soil biodiversity are difficult to explain, but also its potential linkages to many soil processes and the overall ecosystem functioning remains under debate. For example, while some studies have found that reductions in the abundance and presence of soil organisms results in the decline of multiple ecosystem functions (e.g., Wagg et al., 2014), others concluded that above-ground plant diversity alone is a better predictor of ecosystem multi-functionality than soil biodiversity (Jing et al., 2015). Soil organisms exhibit a wide array of feeding preferences, life-cycles and survival strategies and they interact within complex food webs [reviewed by Briones (2014)]. Consequently, “species richness” per se has very little influence on soil processes and “functional dissimilarity” can have stronger impacts on ecosystem functioning (Heemsbergen et al., 2004). Therefore, besides the difficulties in linking above- and below-ground diversities at different spatial scales, gaining a better understanding of the biotic effects on ecosystem processes might require incorporating a great number of components together with several multi-trophic levels (Scherber et al., 2010) as well as the much less considered non-trophic interactions (e.g., phoresy, passive consumption; Goudard and Loreau, 2008). In addition, if soil systems are indeed self-organized, and soil organisms concentrate their activities within a selected set of discrete scales with some form of overall coordination (Lavelle et al., 2016), there is no need for looking for external factors controlling the assemblages of soil constituents. Instead we might just need to recognize the “unexpected” and that the linkages between above-ground and below-ground diversity and soil processes are difficult to predict.
The last three decades of soil ecology research has evidenced that the initial focus on distributions of specific faunal groups has turned significantly into understanding their activity roles, plant-soil interactions, and ecosystem functions. In addition, the studies accumulated so far clearly illustrate that, as soil ecologists, we have been very efficient in gathering information and proposing new hypothesis and ideas. Therefore, can we then assume that we have thoroughly explored all the possible research questions arisen when trying to obtain a more complete view of how the soil systems are organized, how their different components interact and how they respond to changes in the belowground environment but also to those in the one above?
The most recent literature seems to indicate that further advances will emerge from studying sub-organism level responses and thus environmental DNA (Thomsen and Willerslev, 2015) and various “omics” approaches (mainly metagenomics, metatranscriptomics, proteomics, and proteogenomics) are rapidly advancing, at least for the microbial world (Nannipieri, 2014 and references therein). Furthermore, recently “metaphenomics” has been proposed as a better way to encompass the entire omics and the environmental constraints (Jansson and Hofmockel, 2018). Should macroscopic organisms then follow?
In this overview, I argue that before we become overly involved with these new promising tools, which we do not know what they exactly do or to what extent they can be applied to bigger organisms, soil ecology might benefit from looking at available information from a different perspective, re-interpreting and integrating what we have learnt. There are many basic physiological and behavioral aspects of soil organisms, interactive biotic relationships (below-below and above-below ground), functional roles and responses to the abiotic environment that are consistently ignored or less explored, despite being aware of their existence. By bringing the available information (old and/or new) together and breaking the bridges with other disciplines, we could start fitting all these puzzle pieces together and aim at a “eureka moment” in which a more complete picture of the importance of soil fauna in ecosystem functioning becomes revealed.
Describing Soil Biodiversity: From Broad Groups to Molecules
The vast array of different organisms inhabiting the soil makes it very difficult to establish broad groups where they can be lumped in and in turn, make life easier for soil ecologists and modelers (Briones, 2014). Despite the great progress achieved in taxonomical diversity thanks to advances in molecular techniques (e.g., DNA sequencing and fingerprinting of different organisms, but also direct DNA extraction from a soil sample, i.e., environmental DNA or e-DNA), their applicability to soil ecology studies remains difficult. This is due to the existence of relic DNA that could overestimate the amount of biodiversity (Carini et al., 2016), the lack of standardization in these methodological procedures (Orgiazzi et al., 2015), the limited information that they provide on the activity and viability (dead or alive) of many groups (Cangelosi and Meschke, 2014), and the high number of habitats and animal groups that still remain undersampled.
The broad classification into micro-, meso-, and macrofauna, although having some conceptual advantages [e.g., it is assumed that the bigger the animal the bigger the effects on soil processes, e.g., Bradford et al. (2007), and that the bigger the animal the most susceptible to environmental perturbations (e.g., Tsiafouli et al., 2015; Briones and Schmidt, 2017)], also poses some inconveniences. Among them, the difficulty in placing many organisms into a specific group, partly because many of them can vary considerably in size. For example, several mesofaunal groups (such as mites, collembolans, enchytraeids) include species that span from small specimens (around microfauna body width values) to large ones (close to the values observed for macrofauna). In addition, the methodologies to collect these organisms are not group-specific and for example, protozoa (1–2 μm) are still extracted from the soil using microbiological techniques. Not only that, from the systematic point of view, should protozoa be considered as fauna, when they actually consist of several phyla that do not belong to the Animal kingdom? Or would it be better to lump them with microflora (since most species are < 50 μm in size)? And what about considering fungi as “microflora” when their mycelium can extend over kilometers? The same can be said about trying to link this classification to specific microhabitats and functional roles, such as the traditional description of “mesofauna” as those organisms that cannot create their own biogenic structures, when for example enchytraeids can tunnel the soil profile and even reach soil depths beyond the capabilities of many earthworm epigeic species (“true ecosystem engineers”).
One alternative option is the use of functional classifications and functional traits, i.e., instead of putting the focus on the morphology of the soil organisms to split them into different groups, the target would be to quantify their functional role in the ecosystem (e.g., decomposition processes, soil physical structure maintenance) or their responses to changes in the environment (e.g., behavioral or life-history traits). Trait-based approaches are becoming more widely used in soil community ecology and standardized protocols are now available for the most representative taxa (Moretti et al., 2017). These might help us to gain a better understanding of why taxonomic diversity and functional diversity do not often show the same responses to habitat changes (Pey et al., 2014). Importantly, both functional and trait-based approaches are based on activities rather than on the presence of a certain organism and therefore, enabling us to identify who are the true players, what they exactly do and to what extent. Although, in many cases, some of these features can be linked to morphology and taxonomical identity, it avoids the inclusion of inactive states (e.g., cocoons, cysts, letarged/diapaused specimens, carrion) that will be unavoidably extracted in a DNA sample. Furthermore, the fact that the bioturbation activities of some organisms can also re-distribute the genetic material through the soil matrix (Prosser and Hedgpeth, 2018) also pose more difficulties to the interpretations derived from environmental DNA analyses.
Plants Talking and Rhizospheric Fauna Responding
In many ecological studies, the term primary production is typically associated to above-ground plant biomass, usually referred to “net primary production” or NPP, and completely ignores “below-ground plant productivity.” This is due to plant productivity being commonly referred to in agriculture context. Consequently, for several decades, research on rhizospheric fauna mainly concentrated on agricultural pests (Bonkowski et al., 2009), and only from around 1990s onwards a more complex invertebrate community was included (Lavelle, 1996). However, the role of plant roots in soil processes cannot be dissociated from the vast array of organisms that proliferate around them. These include, besides parasites, herbivores and predators, free-living microbes feeding on root exudates, and microbial grazers such as nematodes, collembolans or worms.
While the association between plant roots and mycorrhiza is known to be very old since they co-evolved together, the interactions between soil fauna and plant roots are just starting to be revealed and seem to be more complex than anticipated (Bonkowski et al., 2009; Puga-Freitas and Blouin, 2015; Xiao et al., 2018). Plants produce a variety of secondary metabolites, such as iridoid glycosides (through root exudates) and volatile organic compounds (emitted by green leaves and roots) for above-ground (e.g., to attract pollinators; Dudareva and Pichersky, 2000) and below-ground communication (e.g., to deter herbivores; Wurst et al., 2010). Accordingly, plants are not just merely suppliers of litter for decomposers and instead, they play an active role in attracting beneficial soil invertebrates (e.g., attracting entomopathogenic nematodes to kill the herbivore), providing bacterial inoculum, disturbing the communication between harmful bacteria and also, in modifying rhizodeposition and root architecture (for a more detailed description of the intimate interactions of soil fauna with plant roots see Bonkowski et al., 2009). All these chemical signals released by the plants are directed to benefit their own growth and increase their viability and vigorousity.
However, not only below-ground herbivore suppression can indirectly result in a positive feedback on plant performance, predator-induced shift in detritivore habitat can also help to increase plant biomass. For example, the presence of a carabid beetle (Agonum impressum) that commonly feeds on earthworms resulted in a vertical movement of the prey from the upper to lower soil layer, leading to improved soil properties and enhanced plant biomass (Zhao et al., 2013). Interestingly, the positive effects of this predator-driven response were only significant for above-ground plant biomass but not for root biomass (although a non-significant positive trend toward higher values in the treatment with predators was detected). Hence, the next question will be why despite the actions occurring below-ground the positive response is only being detected above-ground? Could plants become more efficient in taking up nutrients without increasing their root surface area?. According to the reported results, the predators did not significantly affect the overall earthworm densities, but the proportion of the “larger” species (Pheretima aspergillum) present in the top layer. This is an anecic species that lives in permanent vertical burrows (Chang et al., 2009) and hence, it could simply retreat to bottom end of its burrow to avoid predation. In contrast, the burrow system of the second species investigated here (Aporrectodea nocturna) comprises a few long (>100 mm) vertical burrows, exhibiting few branches and low sinuosity (Capowiez et al., 2015). Since this latter species do not possess a “located” home, it will find refuge anywhere in the soil profile. Burrow temporal stability is known to affect not only the amount of organic matter deposited in the lining of the walls (e.g., Hoang et al., 2016), but also the activity of other organisms using these tunnels (Butt and Lowe, 2007; Han et al., 2015) and intra-specific competition (Grigoropoulou et al., 2009). Therefore, are the observed results a reflection of a higher burrowing activity of the “smaller” species or to a greater inactivity of the “larger” one?
One clue to solve this puzzle is the fact that plants are more efficient in foraging N in earthworm casts than in the bulk soil (Agapit et al., 2018) and that root growth could be limited through large increases in soil bioturbation as a result of increased earthworm activity (Arnone and Zaller, 2014). From this, it could be concluded that plant roots would benefit from earthworm casting but not from their burrowing, which is exactly what the predatory beetle achieved.
From this, it would be interesting to know whether plants could stimulate a similar response without relying on an above-ground predator and release any kind of “alarming secretions” that would encourage soil organisms to produce more casting material or to tunnel less, or even more in some cases, so they can access nutrients or water more easily. A new promising tool, which enables recording the acoustic signals emitted by plant roots growing and earthworms burrowing (Lacoste et al., 2018), might help us to decipher whether plant bioturbating activities could also drive soil fauna responses.
Soil Fauna Passing by and Microorganisms Waking Up
Soil microbial activities are hampered by the fact that they are strongly limited by C and N availabilities and their low dispersal abilities prevents them from moving to a more favorable patch with a better nutrient supply. The concept of the “sleeping beauty paradox” coined by Lavelle et al. (1995) perfectly describes the discrepancy between potentially high metabolic capabilities and slow turnover rates by stating that microbial communities are largely dormant and need a “Prince Charming,” either a macroorganism, a physical process or an environmental factor, which “awakens them” by facilitating their contact with the nutrient pools.
As a result, new microsite areas (biopores, aggregates) are created, where soil processes occur at a much faster rate at least during short periods (hours to days) while the food resources last. From this, another new concept in soil ecology has emerged, “hot spots and hot moments” described by Kuzyakov and Blagodatskaya (2015). This close link between these pulses of microbial activity and nutrient availability explains the contradictory estimates of active microorganisms in the soil obtained in the laboratory and in the field. This is a consequence of the use of indirect techniques that rely on substrate additions and bioassays or that are based on static approaches (for a full discussion of the current methods see Blagodatskaya and Kuzyakov, 2013). This together with the high temporal and spatial heterogeneity exhibited by soil microbial communities, both across latitudes and vertically in the complex soil matrix, clearly demonstrate that soil ecology urgently requires more advances in this field.
Furthermore, the roles played by different soil invertebrates in the dispersal of soil microorganisms deserve further consideration. Both mesofauna (microarthropods) and macrofauna (earthworms) are known to carry cells, spores and mycelium attached to their bodies and in their guts and then released out again via egestion in their feces. While phoresy will help transported microorganisms in colonizing new areas, gut passage could result in either activation or destruction of the microbial cells (e.g., Schoënholzer et al., 1999; Renker et al., 2005; Buse et al., 2014). In other cases, although spore/propagule viability is retained, germination might be delayed (Talbot, 1952). Whether these dispersal mechanisms are stochastic (awaiting for a passing by invertebrate) or there is some attraction mechanism involved is another interesting aspect that deserves more experimental research. For example, earthworm skin secretes mucus, a rather attractive source of labile C for microbes, which could stimulate microbial activities and accelerate the mineralization of soil organic matter (Scheu, 1991; Bernard et al., 2012). “Fecal attraction” on earthworm casts and middens or microarthropod fecal pellets is another way of congregating a high number of microorganisms (Bohlen et al., 2002; Tagger et al., 2008), and bacteria living in feces can serve a way of intra-specific communication among certain insects (Wada-Katsumata et al., 2015). On the other hand, microorganisms can also attract soil invertebrates, and it has been shown that fungal odor attracts collembolans (Bengtsson et al., 1988) which might enhance the dispersal of the fungi, but also the movement of the fungivorous collembolan (Bengtsson et al., 1994). From these studies, it is clear that many of these faunal-microbial interactions are not always random and that finding how and when a macroorganism can “switch on” a hot moment could enhance our understanding of ecosystem functioning.
Furthermore, it would be interesting to know if any of these mechanisms could also represent a hot spot for gene transfer as it has been demonstrated that fungal hyphae are useful infrastructures for bacteria to move toward more accessible food sources but also for horizontal transfer of genes between differing bacteria (Berthold et al., 2016). Could a soil invertebrate wake up a fungal species and as a result, facilitate the movement of bacteria through the soil and consequently, their functional attributes (e.g., gene expression for nitrification or denitrification)?
Soil Food Webs Revisited: Dining at the Soil Restaurant
The first description of a topological food web appeared in 1912 and was produced by Pierce and Cushman (1912), who were investigating the insect enemies of the cotton boll weevil. This seminal work progressed by linking detrital biotic interactions with other components of the aquatic and terrestrial ecosystems [reviewed by Pimm et al. (1991)]. Unfortunately, our current understanding on trophic interactions is far from complete and we need a more refined picture of the number and identity of the potential consumers at the different trophic levels. For example, soil viruses and enchytraeids are usually omitted in these food web simulations and nematodes and mites are the only groups that might be subdivided into different feeding groups, whereas the different ecological groupings of earthworms or the feeding guilds of collembolans are typically ignored. This is important because the inclusion or omission of a certain trophic level or food source could change the overall interpretation of the soil food web. For example, omitting the grazing activities of certain groups, such as protozoa and nematodes could result in underestimations of total N mineralisation rates, with reductions of 28 and 12%, respectively (De Ruiter et al., 1993).
However, even in the case of a well-defined trophic classification, we can also find some species “breaking the rules.” For example, in the case of nematodes, by looking at their head structures (i.e., presence/absence of stylets, teeth, etc.) we can obtain information about their feeding habits (i.e., whether they typically feed on bacteria, fungi, plants, other nematodes or on a mixture of food sources); however, some species from any of these groups can switch to cyanobacteria (Yeates, 1998). Similarly, collembolans, which are generally considered to be fungivorous, include species feeding on nematodes (Chamberlain et al., 2005). And what about coprophagy exhibited by mites, isopoda, enchytraeids and earthworms? This particular case represents a special way of soil fauna interacting with microorganisms and functions as an “external rumen” (Swift et al., 1979) allowing many soil organisms to obtain extra nutrients from suboptimal foods (Ponge, 1991). All these behavioral patterns complicate their placement into a particular trophic/functional group.
Furthermore, the number of trophic levels in terrestrial food webs rarely exceed three levels (Hairston and Hairston, 1993), due to the low efficiency of their trophic groups in assimilating their preferred food and transferring energy from one level to the next (i.e., fraction of the food below that is contributing to the biomass production of the trophic level above). This has led to the suggestion that complex soil food webs are not stable, which contrasts with the pioneering work by earlier researchers (Svensson and Rosswall, 1980; Parker et al., 1984; Hunt et al., 1987; De Ruiter et al., 1995) and more recent work (Digel et al., 2014; Van Altena et al., 2016) that described soil food webs with 4–8 trophic levels. For example, Digel et al. (2014) analyzed 48 forest soil food webs ranging from 89 to 168 taxa and found 729 to 3344 feeding interactions. The results from these studies indicate that long and dynamically stable soil food webs are possible. The key variables controlling the functioning of these more complex web structures are the “number of species involved,” the “degrees of connectedness“ and the “strengths of species interactions,” which were identified by May (1972) and tested by De Ruiter et al. (1995). They found that the omnivorous links from the higher predators in the web were crucial in terms of preserving stability, confirming May's theory that the most densely connected species, that is where the trophic connections were most complex, were crucial for their stability [see also review by (Manne and Pimm (1996)]. Indeed, compared to other food webs, soil food webs are characterized by exhibiting a higher degree of omnivory, with a high number of species feeding on different trophic levels, as well as cannibalism or “intra-guild predation” (Digel et al., 2014).
Omnivory can represent a problem when classifying organisms according to their feeding habits and in quantifying the effects of predation on the biomass of those organisms placed at lower trophic levels. This, in turn, has implications on the magnitude and extent of “trophic cascades” (sensu Scheu and Setälä, 2002 and Wardle, 2002) and/or on the overall dominance of “top-down vs. bottom-up regulation processes” (sensu Moore et al., 2003). In relation to this, Neutel et al. (2002) described the unequal effects of top-down regulations exhibited by predators that feed on preys that belong to different trophic levels and showed that prey density could determine their preferential feeding on a particular prey and consequently, have a significant effect on the trophic level where that consumed prey is concentrating its activities.
However, is it really a omnivory/generalist feeding behavior that characterizes the trophic relationships between soil organisms or do soil animals actually rather prefer to choose what to eat by looking at the whole menu, instead of just merely going for their local basic food source? That is what I have called “feeding flexibility” (Briones et al., 2010) to better describe how soil mesofauna could switch from one diet to another in response to changes in the environmental conditions (abiotic or biotic). This concept differs from “biological accessability,” which has been proposed as a better predictor of soil organic matter turnover than recalcitrance (Dungait et al., 2012). The accessibility of the organic sources to decomposers could be mediated by physical (e.g., by a macroorganism facilitating the close contact) or chemical factors (e.g., by microbial pre-conditioning of the plant material, as an “external rumen”). I argue that besides cooperation between soil fauna and microorganisms, some invertebrates are more selective than currently assumed and they could feed on more labile or more recalcitrant substrates depending on what is available on the menu or what is easier to get under certain circumstances. For example, the observed priming effects on soil fauna (e.g., Nieminen and Pohjola, 2014; Eck et al., 2015) explain how organisms that typically feed on more humified organic matter (e.g., enchytraeids and endogeic worms) could suddenly find a labile substrate irresistible. However, this does not mean that their diet naturally consists of a mixture of substances of different nature (omnivory) because this variety of foods is not always available and hence, they cannot rely on their regular supply nor spend their energy in searching insistently for them. Intra-specific competition can also drive changes in feeding behaviors, and a beautiful example of this can be extracted from the work by Anderson (1975), who showed that when two species of oribatid mites were grown in isolation they preferred to feed on similar sources, but when put together in competition they changed their feeding habits by moving to a different layer (litter or fermented). Similarly, if the soil at the surface becomes too wet or too dry and the organisms are able to escape from those adverse conditions by migrating down, their survival could only be guaranteed if they can feed on any of the food choices available at the deeper below-ground menu (and this might lead to “compensatory feeding,” as it has been seen in root herbivores in response to lower nutrient quality of the source; Johnson et al., 2014). Perhaps, these feeding choices are “context-dependent” and, under different abiotic and biotic pressures, the same species could exhibit different feeding strategies.
Other factors that are currently impeding us from gaining a full understanding of the functioning of the soil food webs are: (i) redundancy (several species feeding on the same resource) and complementarity within functional groups (Setälä et al., 2005), which will also have implications on top-down and bottom-up relationships; (ii) the fact that some soil organisms feed on different diets or exhibit different feeding rates during their lifetime (e.g., Briones et al., 2005) and hence, their tissue turnover and feeding efficiencies/diets might also change with age, and (iii) density-dependent effects on their feeding activities, which could lead to positive or negative (direct and indirect) effects on their prey (Kaneda and Kaneko, 2008). They all need to be integrated in food web analyses to provide a more realistic (dynamic) quantification of energy flows across the different trophic levels.
Can we therefore conclude that at least some soil invertebrates are selective (eating their preferable food when available), but others are opportunistic (eating whatever is abundant at that particular moment) or generalist feeders (eating whatever is easy to obtain in order to avoid competition)? Could the soil food webs also exhibit temporal “feeding pulses” during “hot moments” (at one or several trophic levels), with a measurable effect on the trophic levels below (“cascade trophic effects”)?
Soil Fauna Linkages to Soil Processes and Ecosystem Functioning
Despite our yet limited knowledge on the identity of the different organisms inhabiting our soils, what is the usefulness of having such a huge diversity? Is it truly necessary? Do every existing species have a role in their lives? And, more importantly, is this high richness always accompanied by a better performance of the ecosystems where they live?
It could be expected that in those extreme environments (such as cold and arid ecosystems) where nutrients are scarce and/or supply discontinuous, due to environmental pressures (climatic, soil conditions, etc.), soil biodiversity will be low and food chains short. Under these conditions, any nutritional surplus will lead to a “hot moment” (sensu Kuzyakov and Blagodatskaya, 2015) in which the soil food web will re-activate and hence, soil processes rates will increase (Figure 1). In contrast, those systems with a regular supply of nutritious substrates will be able to sustain a higher number of different taxonomical and functional entities. In this case, not only a greater variety of trophic niches will be available, but also the co-existence of several groups feeding on the same sources could be maintained as long as nutrient availability persist. This is expected to result in a higher number of “hot spots” (sensu Kuzyakov and Blagodatskaya, 2015) across the soil matrix (Figure 1). The resulting increases in foraging activities and reproductive rates will “cascade” up and down along the soil food web bringing other pressures into action (predation, competition) which will modulate the initial responses to increased nutrient availability. On the other hand, this also means that those “hot spots” in the more limiting arid and cold ecosystems could be more intense, and thereby exponentially increase the role of soil fauna and trophic cascades in those systems.
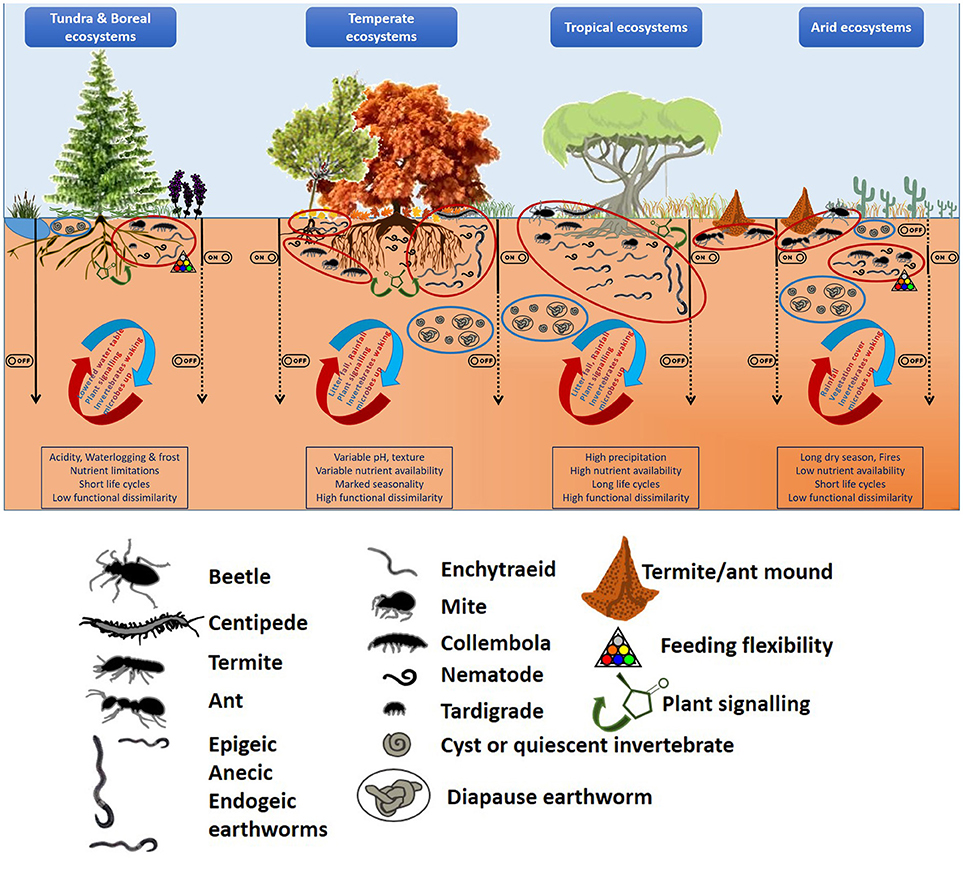
Figure 1. Linking hotspots and hot moments of soil fauna to climatic gradients and soil heterogeneity: Historical factors (climate, parent material) shape our landscapes (both above- and below-ground), but the regional/local abiotic conditions constraint biological activities. These operate at different spatial and temporal scales and can switch on and off different organisms at different microsites resulting in a hot moment in a particular hotspot. Since each of their responses can have effects on others, their effects could then cascade up and down in the food web. Soil invertebrates are depicted not in scale, just for illustrative purposes (see pictorial legend for major taxonomical groups). Ellipses indicate hot (red) or cold spots (blue), with the curved arrows giving some examples of the factors that could switch on/off a hot moment and the straight black arrows (continuous black line = on, dashed = off) showing the implications for soil processes along the soil profile. In the boxes, the main ecosystem characteristics are listed.
Since feeding is a primary need, it is not surprising that soil organic matter quantity and quality has always been considered the main driver of decomposition and nutrient cycling. Indeed, much research focus has been placed on C resource quality (recalcitrance and chemical protection), following the works by Hooper et al. (2000) and Ponge (2003, 2013). However, another important aspect that should be considered here is that besides the “chemical heterogeneity” of the C substrates deposited by the primary producers, there is also considerable “physical heterogeneity” in the soil systems that needs to be accounted for. The existence of physical gradients (pH, moisture, aggregates, porosity, etc.) together with the horizontal patchy distributions exhibited by soil organisms [even at local (plot) level] hinders any attempt to link all attributes present in soils, i.e., soil biodiversity, soil properties, and soil functions. Therefore, despite the success in finding a gradient of increasing biodiversity with increasing humification of soil organic matter (Ponge, 2003), different outcomes can be anticipated depending on the influence of environmental filters and anthropogenic forces acting upon decomposition rates (Zanella et al., 2017).
This could explain why litter decomposition rates show such a great variability across biomes, elevations and soil types, highlighting that, besides temperature and moisture, there must be other important factors controlling decomposition rates. Sadly, despite being widely known that decomposition has been intimately associated to soil fauna since prehistoric times (Labandeira et al., 1997), they are continuously ignored in recent modeling and perspective studies (e.g., Schmidt et al., 2011) or lumped together in a big box, whereas bacteria and fungi are given their own individual compartments and full roles. As a soil fauna ecologist, I can only stress the need to increase their visibility and fight for “their own rights” so they become considered as least at the same level of prokaryotes. In support of this, a global meta-analysis showed that soil fauna consistently enhanced litter decomposition across biomes by 27% (García-Palacios et al., 2013). In addition, the classical work by Coûteaux et al. (1991) nicely demonstrated that increasing soil web complexity (by adding invertebrates at higher trophic levels) accelerates litter decomposition more than expected from a simple sum of their individual activities, so their omission is unforgettable.
Similarly, the majority of the so-called “soil quality indexes” are merely based on soil properties (phosphorus runoff potential, nitrogen availability, metal contamination) or microbial activities (Cmic/Corg, soil respiration, litter decomposition, etc.), and completely ignore those based on soil fauna parameters, such as the eco-morphological index (EMI) proposed by Parisi (2001) and the abundance-based fauna index (FAI) proposed by Yan et al. (2012). For example, the inclusion of a soil biodiversity index that included measures of richness and abundance of functional guilds has allowed to relate soil community characteristics to ecosystem multifunctionality (Wagg et al., 2014) and another study concluded that higher diversity at different trophic levels is necessary to maintain ecosystem multi-function performance (Soliveres et al., 2016). More advances in linking the responses of species assemblages, biotic interactions and ecosystem processes to global change are envisaged from recent efforts in standardizing protocols measuring ecological traits in soil invertebrates (Moretti et al., 2017). This will also allow us to rationalize the use of the soils for cultivation/urbanization and for allowing a better use of the natural sources (such as zero- carbon and circular economies).
Is Further Research Needed?
Soil fauna ecologists have profusely explored soils around the world trying to determine the number of species, abundance, and temporal patterns of the organisms that live in it, the interactive roles of plant-soil-fauna on soil processes and ecosystem functioning. Here, I provide several stimulating ideas that might lead to a more refined understanding of the heterotrophic component of the soil system or help in looking at the information available from a different perspective:
1. Only a proportion of all the species living in soils have been described and, although advances are expected thanks to rapid DNA sequencing, very little is known about their community structure and dynamics across different ecosystems. If we are unable to clearly relate ecosystem functions to ecosystem diversity, then the relationships found in several studies might not be causal correlations and hence, much of the diversity present might just be “redundant.” To answer this question, we might need not only further refinement of our experimental approaches and more taxonomical efforts and ecological work on soil biota, but also “activity proxies” and “response traits” rather than abundance and biomass ones.
2. If soils sustain a high diversity, how could the little attention they receive compared to plants or birds be justified? Measures to preserve and promote higher biodiversity in soils should come into force, including the implementation of management policies at National and International levels. Biodiversity is protected under various directives and international commitments (e.g., Habitats Directive, Natura 2000, Convention on Biological Diversity, CITES), but without explicit mention of soil biodiversity. For organisms living above-ground, the current species' extinction rates have prompted the need for preserving endangered species. Thus, in the case of plants, seed banks have been built in selected parts of the world to keep unique and valuable plant species (e.g., Svalbard Global Seed Vault). Similarly, cells, tissues and embryos of different vertebrates have been frozen to open the possibility of “resurrecting” extinct species or saving nearly extinct species in the future (e.g., Frozen Zoo® in San Diego). Large culture collections of fungi (including yeasts), bacteria and plasmid exist (CBS-KNAW Collections in The Netherlands) for commercial value. Should not a “soil biota zoo” be constructed to safeguard our soil biodiversity and serve as a home to a World Data Archive?
3. Re-defining soil quality/soil health goes along with including soil biological indicators that integrate parameters measuring soil biodiversity functionality. For example, the humus index developed by Ponge et al. (2002), besides providing a framework to integrate soil biodiversity, soil conditions, and humus forms, also allows a quantitative assessment of soil formation and development and plant-soil biodiversity co-evolution across different ecosystems.
4. Feeding preferences of soil organisms are not yet clearly established, and in the particular case of burrowing forms that move through the soil by ingesting it, can we say that they are selective feeders or just ingesting accidentally? Moreover, if they do select what they eat, what are the implications for soil functioning? For example, some studies have shown that earthworms and collembolans can be highly selective when grazing on fungi (Moody et al., 1995; Jørgensen et al., 2005) and, in some cases, decrease the crop damage caused by fungal infections (Stephens et al., 1994; Sabatini and Innocenti, 2001). Obviously, spreading or reducing the incidence of fungal disease in successive crops will depend on the survival through the gut passage (Moody et al., 1995), which might be different for different species. Answering these questions may open new research options and, for example, elucidating the level of specificity of the grazer (i.e., major groups or species-specific selection) and assessing the overall impacts of grazing in shaping the fungal communities (e.g., increases in fungal diversity as a result of reducing the presence of the dominant fungus; for more impacts of fungi grazing fauna see McGonigle, 2007) could have different implications for crop performance. And finally, could these aspects be extended to other soil organisms (pseudoscorpions, predatory mites and beetles) and hence, by inoculating certain species (or species combinations) reduce the effect of soil-borne pathogens (not only fungi, also bacteria and viruses)?
5. When linking soil biodiversity and soil processes we also need to re-define “recalcitrance” beyond merely chemical terms and “biological accessibility” beyond microbial attack. An additional difficult task will be to combine the spatial and temporal heterogeneity of food substrates together with the “functional dissimilarity” of soil organisms (Heemsbergen et al., 2004) in modeling exercises. Importantly, can we provide mathematical formulations of “seasonal pulses of litter fall and nutrients” and “hot spots of biological activities,” including hyphal horizontal and vertical transfers between leaf litters and soil layers together with microsites of preferential nutrient flow paths and high biological densities that could lead to immobilization or mobilization of certain elements?
6. Since in the last three decades soil ecology research has turned its interest to the functional role of soil organisms, can we say we have identified and/or accounted for all their possible roles? The current literature is dominated by descriptions of the processes that occur at the root-soil interface. However, there are other soil fauna effects that can modulate the breaking down of organic inputs and yet, are very rarely included in ecological investigations: “zoological weathering” (i.e., mobilizing inorganic elements from rocks by the action of soil organisms), “zoological retarding” (e.g., the presence of a peritrophic membrane that encapsulates mite's feces and that slows down their degradation), “zoological bioturbation” (movement of organic and mineral particles and other organisms by soil fauna), “zoological bonding” (chemical binding of C and P induced by soil fauna). Could they become emerging concepts in future soil ecology studies?
7. A final challenge is the integration of abiotic (climate, soil texture) and biotic factors (litter quality and biological accessibility) influencing decomposition across spatial and temporal scales and to understand how their effects could change under environmental perturbations such as land use and climate changes. The fact that many of these factors do no work in isolation, understanding the interactions between soil and climatic factors, plant and soil organisms, microbes and soil fauna, and among them all is crucial.
These are only few examples that illustrate the complex nature of soil communities living in a heterogeneous soil matrix, consisting of a mosaic of microsites with different soil conditions and resource availabilities. The structure and degree of connectivity between these patches together with their temporal dynamics determines the number and composition of species assemblages. However, because they do not conform to closed loops and their responses to short-term changes in soil abiotic conditions are usually stochastic, it is difficult to underpin the mechanisms that allows their shelf-organization (sensu Lavelle et al., 2016). Soil biodiversity is at the core of the International agendas (GSBI, IPBES), and in the UN Sustainable Development Goals, and only with a more refined view of all the potential contributions of soil organisms to soil processes their full integration in sustainable management and climate change mitigation policies will be possible.
Author Contributions
The author confirms being the sole contributor of this work and has approved it for publication.
Conflict of Interest Statement
The author declares that the research was conducted in the absence of any commercial or financial relationships that could be construed as a potential conflict of interest.
The handling editor and reviewer JC declared their involvement as co-editors in the Research Topic, and confirms the absence of any other collaboration.
References
Agapit, C., Gigon, A., Puga-Freitas, R., Zeller, B., and Blouin, M. (2018). Plant-earthworm interactions: influence of age and proportion of casts in the soil on plant growth, morphology and nitrogen uptake. Plant Soil 424, 49–61. doi: 10.1007/s11104-017-3544-y
Anderson, J. M. (1975). Succession, diversity and trophic relationships of some soil animals in decomposing leaf litter. J. Anim. Ecol. 44, 475–495. doi: 10.2307/3607
Arnone, J. A. III., and Zaller, J. G. (2014). Earthworm effects on native grassland root system dynamics under natural and increased rainfall. Front. Plant Sci. 5:art152. doi: 10.3389/fpls.2014.00152
Bardgett, R. D., and van der Putten, W. H. (2014). Belowground biodiversity and ecosystem functioning. Nature 515, 505–511. doi: 10.1038/nature13855
Bengtsson, G., Erlandsson, A., and Rundgren, S. (1988). Fungal odour attracts soil Collembola. Soil Biol. Biochem. 20, 25–30. doi: 10.1016/0038-0717(88)90122-8
Bengtsson, G., Hedlund, K., and Rundgren, S. (1994). Food- and density-dependent dispersal: evidence from a soil collembolan. J. Anim. Ecol. 63, 513–520. doi: 10.2307/5218
Berg, M. P. (2012). “Patterns of biodiversity at fine and small spatial scales,” in Soil Ecology and Ecosystem Services, eds D. H. Wall, R. D. Bardgett, V. Behan-Pelletier, T. H. Jones, J. E. Herrick, K. Ritz, J. Six, D. R. Strong and W. H. van der Putten (New York, NY: Oxford Academic Press), 136–152.
Berg, M. P., and Bengtsson, J. (2007). Temporal and spatial variability in soil food web structure. Oikos 116, 1789–1804. doi: 10.1111/j.0030-1299.2007.15748.x
Bernard, L., Chapuis-Lardy, L., Razafimbelo, T., Razafindrakoto, M., Pablo, A. L., Legname, E., et al. (2012). Endogeic earthworms shape bacterial functional communities and affect organic matter mineralization in a tropical soil. ISME J. 6, 213–222. doi: 10.1038/ismej.2011.87
Berthold, T., Centler, F., Hübschmann, T., Remer, R., Thullner, M., Harms, H., et al. (2016). Mycelia as a focal point for horizontal gene transfer among soil bacteria. Sci. Rep. 6:36390. doi: 10.1038/srep36390
Blagodatskaya, E., and Kuzyakov, Y. (2013). Active microorganisms in soil: critical review of estimation criteria and approaches. Soil Biol. Biochem. 67, 192–211. doi: 10.1016/j.soilbio.2013.08.024
Bohlen, P. J., Edwards, C. A., Zhang, Q., Parmelee, R. W., and Allen, M. (2002). Indirect effects of earthworms on microbial assimilation of labile carbon. Appl. Soil Ecol. 20, 255–261. doi: 10.1016/S0929-1393(02)00027-6
Bonkowski, M., Villenave, C., and Griffiths, B. (2009). Rhizosphere fauna: the functional and structural diversity of intimate interactions of soil fauna with plant roots. Plant Soil 321, 213–233. doi: 10.1007/s11104-009-0013-2
Bradford, M. A., Tordoff, G. M., Black, H. I. J., Cook, R., Eggers, T., Garnett, M. H., et al. (2007). Carbon dynamics in a model grassland with functionally different soil communities. Funct. Ecol. 21, 690–697. doi: 10.1111/j.1365-2435.2007.01268.x
Briones, M. J. I. (2014). Soil fauna and soil functions: a jigsaw puzzle. Front. Environ. Sci. 2:art7. doi: 10.3389/fenvs.2014.00007
Briones, M. J. I., Garnett, M. H., and Ineson, P. (2010). Soil biology and warming play a key role in the release of ‘old C' from organic soils. Soil Biol. Biochem. 42, 960–967. doi: 10.1016/j.soilbio.2010.02.013
Briones, M. J. I., Garnett, M. H., and Piearce, T. G. (2005). Earthworm ecological groupings based on 14C analysis. Soil Biol. Biochem. 37, 2145–2149. doi: 10.1016/j.soilbio.2005.03.001
Briones, M. J. I., and Schmidt, O. (2017). Conventional tillage decreases the abundance and biomass of earthworms and alters their community structure in a global meta-analysis. Glob. Change Biol. 23, 4396–4419. doi: 10.1111/gcb.13744
Buse, T., Ruess, L., and Filser, J. (2014). Collembola gut passage shapes microbial communities in faecal pellets but not viability of dietary algal cells. Chemoecology 24, 79–84. doi: 10.1007/s00049-013-0145-y
Butt, K. R., and Lowe, C. N. (2007). Presence of earthworm species within and beneath Lumbricus terrestris (L.) middens. Eur. J. Soil Biol. 43, S57–S60. doi: 10.1016/j.ejsobi.2007.08.002
Cangelosi, G. A., and Meschke, J. S. (2014). Dead or alive: molecular assessment of microbial viability. Appl. Environ. Microbiol. 80, 5884–5891. doi: 10.1128/AEM.01763-14
Capowiez, Y., Bottinelli, N., Sammartino, S., Michel, E., and Jouquet, P. (2015). Morphological and functional characterisation of the burrow systems of six earthworm species (Lumbricidae). Biol. Fertil. Soils 51, 869–877. doi: 10.1007/s00374-015-1036-x
Carini, P., Marsden, P. J., Leff, J. W., Morgan, E. E., Strickland, M. S., and Fierer, N. (2016). Relic DNA is abundant in soil and obscures estimates of soil microbial diversity. Nat. Microbiol. 2:16242. doi: 10.1101/043372
Chamberlain, P. M., Bull, I. D., Black, H. I. J., Ineson, P., and Evershed, R. P. (2005). Fatty acid composition and change in Collembola fed differing diets: identification of trophic biomarkers. Soil Biol. Biochem. 37, 1608–1624. doi: 10.1016/j.soilbio.2005.01.022
Chang, C., Shen, H.-P., and Chen, J.-H. (2009). Earthworm Fauna of Taiwan. Taipei: National Taiwan University Press.
Coûteaux, M.-M., Mousseau, M., Célérier, M.-L., and Bottner, P. (1991). Increased atmospheric CO2 and litter quality: decomposition of sweet chestnut leaf litter with animal food webs of different complexities. Oikos 61, 54–64. doi: 10.2307/3545406
De Ruiter, P. C., Moore, J. C., Zwart, K. B., Bouwman, L. A., Hassink, J., Bloem, J., et al. (1993). Simulation of nitrogen mineralization in the below-ground food webs of two winter wheat fields. J Appl. Ecol. 30, 95–106. doi: 10.2307/2404274
De Ruiter, P. C., Neutel, A.-M., and Moore, J. C. (1995). Energetics, patterns of interaction strengths, and stability in real ecosystems. Science 269, 1257–1260. doi: 10.1126/science.269.5228.1257
Decaëns, T. (2010). Macroecological patterns in soil communities. Global Ecol. Biogeogr. 19, 287–302. doi: 10.1111/j.1466-8238.2009.00517.x
Digel, C., Curtsdotter, A., Riede, J., Klarner, B., and Brose, U. (2014). Unravelling the complex structure of forest soil food webs: higher omnivory and more trophic levels. Oikos 123, 1157–1172. doi: 10.1111/oik.00865
Dudareva, N., and Pichersky, E. (2000). Biochemical and molecular genetic aspects of floral scents. Plant Physiol. 122, 627–634. doi: 10.1104/pp.122.3.627
Dungait, A. J., Hopkins, D. W., Gregory, A., and Whitmore, A. (2012). Soil organic matter turnover is governed by accessibility not recalcitrance. Glob. Change Biol. 18, 1781–1796. doi: 10.1111/j.1365-2486.2012.02665.x
Eck, T., Potthoff, M., Dyckmans, J., Wichern, F., and Joergensend, R. G. (2015). Priming effects of Aporrectodea caliginosa on young rhizodeposits and old soil organic matter following wheat straw addition. Eur. J. Soil Biol. 70, 38–45. doi: 10.1016/j.ejsobi.2015.07.002
García-Palacios, P., Maestre, F. T., Kattge, J., and Wall, D. H. (2013). Climate and litter quality differently modulate the effects of soil fauna on litter decomposition across biomes. Ecol. Lett. 16, 1045–1053. doi: 10.1111/ele.12137
Goudard, A., and Loreau, M. (2008). Nontrophic interactions, biodiversity, and ecosystem functioning: an interaction web model. Am Nat. 171, 91–106. doi: 10.1086/523945
Grigoropoulou, N., Butt, K. R., and Lowe, C. N. (2009). Interactions of juvenile Lumbricus terrestris with adults and their burrow systems in a two-dimensional microcosm. Pesq. Agropec. Bras. 44, 964–968. doi: 10.1590/S0100-204X2009000800025
Hairston, N. G. Jr., and Hairston, N. G. Sr. (1993). Cause-effect relationships in energy flow, trophic structure, and interspecific interactions. Amer. Nat. 142, 379–411. doi: 10.1086/285546
Han, E., Kautz, T., Perkons, U., Uteau, D., Peth, S., Huang, N., et al. (2015). Root growth dynamics inside and outside of soil biopores as affected by crop sequence determined with the profile wall method. Biol. Fertil. Soils 51, 847–856. doi: 10.1007/s00374-015-1032-1
Heemsbergen, D. A., Berg, M. P., Loreau, M., van Hal, J. R., Faber, J. H., and Verhoef, H. A. (2004). Biodiversity effects on soil processes explained by interspecific functional dissimilarity. Science 306, 1019–1020. doi: 10.1126/science.1101865
Hoang, D. T. T., Razavi, B. S., Kuzyakov, Y., and Blagodatskaya, E. (2016). Earthworm burrows: kinetics and spatial distribution of enzymes of C-, N- and P- cycles. Soil Biol. Biochem. 99, 94–103. doi: 10.1016/j.soilbio.2016.04.021
Hooper, D. U., Bignell, D. E., Brown, V. K., Brussard, L., Dangerfield, J. M., Wall, D. H., et al. (2000). Interactions between aboveground and belowground biodiversity in terrestrial ecosystems: patterns, mechanisms, and feedbacks. BioScience 50, 1049–1061. doi: 10.1641/0006-3568(2000)050[1049:IBAABB]2.0.CO;2
Hunt, H. W., Coleman, D. C., Ingham, E. R., Ingham, R. E., Elliott, E. T., Moore, J. C., et al. (1987). The detrital food web in a shortgrass prairie. Biol. Fertil. Soils 3, 57–68. doi: 10.1007/BF00260580
Jansson, J. K., and Hofmockel, K. S. (2018). The soil microbiome—from metagenomics to metaphenomics. Curr. Opin. Microbiol. 43, 162–168. doi: 10.1016/j.mib.2018.01.013
Jing, X., Sanders, N. J., Shi, Y., Chu, H., Classen, A. T., Zhao, K., et al. (2015). The links between ecosystem multifunctionality and above- and belowground biodiversity are mediated by climate. Nat. Commun. 6:8159. doi: 10.1038/ncomms9159
Johnson, S. N., Lopaticki, G., and Hartley, S. E. (2014). Elevated atmospheric CO2 triggers compensatory feeding by root herbivores on a C3 but not a C4 grass. PLoS ONE 9:e90251. doi: 10.1371/journal.pone.0090251
Jørgensen, H. B., Johansson, T., Canbäck, T., Hedlund, K., and Tunlid, A. (2005). Selective foraging of fungi by collembolans in soil. Biol. Lett. 1, 243–246. doi: 10.1098/rsbl.2004.0286
Kaneda, S., and Kaneko, N. (2008). Collembolans feeding on soil affect carbon and nitrogen mineralization by their influence on microbial and nematode activities. Biol. Fertil. Soils 44, 435–442. doi: 10.1007/s00374-007-0222-x
Kuzyakov, Y., and Blagodatskaya, E. (2015). Microbial hotspots and hot moments in soil: concept and review. Soil Biol. Biochem. 83, 184–199. doi: 10.1016/j.soilbio.2015.01.025
Labandeira, C. C., Phillips, T. L., and Norton, R. A. (1997). Oribatid mites and the decomposition of plant tissues in Paleozoic coal-swamp forest. Palaios 12, 319–353. doi: 10.2307/3515334
Lacoste, M., Ruis, S., and Or, D. (2018). Listening to earthworms burrowing and roots growing – acoustic signatures of soil biological activity. Sci. Rep. 8:10236. doi: 10.1038/s41598-018-28582-9
Lavelle, P., Lattaud, C., Trigo, D., and Barois, I. (1995). Mutualism and biodiversity in soils. Plant Soil 170, 23–33. doi: 10.1007/BF02183052
Lavelle, P., Spain, A., Blouin, M., Brown, G., Decaëns, T., Grimaldi, M., et al. (2016). Ecosystem engineers in a self-organized soil: a review of concepts and future research questions. Soil Sci. 181, 91–109. doi: 10.1097/SS.0000000000000155
Lin, H., Wheeler, D., Bell, J., and Wilding, L. (2005). Assessment of soil spatial variability at multiple scales. Ecol. Modell. 182, 271–229. doi: 10.1016/j.ecolmodel.2004.04.006
Lozupone, C. A., and Knight, R. (2007). Global patterns in bacterial diversity. PNAS 104, 11436–11440. doi: 10.1073/pnas.0611525104
Manne, L., and Pimm, S. L. (1996). Ecology: engineered food webs. Curr. Biol. 6, 29–31. doi: 10.1016/S0960-9822(02)00414-1
McGonigle, T. P. (2007). “Effects of animals grazing on fungi,” in Environmental and Microbial Relationships, The Mycota, Vo.l 4, eds C. Kubicek and I. Druzhinina (Berlin: Springer-Verlag), 201–212.
Moody, S. A., Briones, M. J. I., Piearce, T. G., and Dighton, J. (1995). Selective consumption of decomposing wheat straw by earthworms. Soil Biol. Biochem. 27, 1209–1213. doi: 10.1016/0038-0717(95)00024-9
Moore, J. C., McCann, K., Setälä, H., and De Ruiter, P. C. (2003). Top-down is bottom-up: does predation in the rhizosphere regulate aboveground dynamics? Ecology 84, 846–857. doi: 10.1890/0012-9658(2003)084[0846:TIBDPI]2.0.CO;2
Moretti, M., Dias, A. T. C., de Bello, F., Altermatt, F., Chown, S. L., Azcárate, F. M., et al. (2017). Handbook of protocols for standardized measurement of terrestrial invertebrate functional traits. Funct. Ecol. 31, 558–567. doi: 10.1111/1365-2435.12776
Nannipieri, P. (2014). Soil as a biological system and omics approaches. Int. J. Environ. Qual. 13, 61–66. doi: 10.6092/issn.2281-4485/4541
Neutel, A. M., Heesterbeek, J. A., and De Ruiter, P. C. (2002). Stability in real food webs: weak links in long loops. Science 296, 1120–1123. doi: 10.1126/science.1068326
Nielsen, U. N., Osler, G. H., Campbell, C. D., Neilson, R., Burslem, D. F., and van der Wal, R. (2010). The enigma of soil animal species diversity revisited: the role of small-scale heterogeneity. PLoS ONE 5:e11567. doi: 10.1371/journal.pone.0011567
Nieminen, J. K., and Pohjola, P. (2014). Labile carbon addition affects soil organisms and N availability but not cellulose decomposition in clear-cut Norway spruce forests. Boreal Env. Res. 19, 257–266. Available online at: http://www.borenv.net/BER/pdfs/ber19/ber19-257.pdf
Orgiazzi, A., Dunbar, M. B., Panagos, P., de Groot, G. A., and Lemanceau, P. (2015). Soil biodiversity and DNA barcodes: opportunities and challenges. Soil Biol. Biochem. 80, 244–250. doi: 10.1016/j.soilbio.2014.10.014
Parisi, V. (2001). La qualità biologica del suolo. Un metodo basato sui microartropodi. Acta Nat Ateneo Parmense 37, 105–114. Available online at: http://www.isprambiente.gov.it/it/temi/biodiversita/lispra-e-la-biodiversita/attivita-e-progetti/progetto-speciale-funghi-1/pdf/ACTA_NATURALIA_PARMAEParisi_QBS_ridotto.pdf
Parker, L. W., Santos, P. F., Phillips, J., and Whitford, W. G. (1984). Carbon and nitrogen dynamics during the decomposition of litter and roots of a Chihuahuan desert annual, Lepidiurn lasiocarpum. Ecol. Monogr. 54, 339–360. doi: 10.2307/1942501
Pey, B., Nahmanic, J., Auclercd, A., Capowiez, Y., Cluzeau, D., Cortet, J., et al. (2014). Current use of and future needs for soil invertebrate functional traits in community ecology. Basic Appl. Ecol. 15, 194–206. doi: 10.1016/j.baae.2014.03.007
Pierce, W. D., and Cushman, R. A. (1912). The Insect Enemies of the Cotton Boll Weevil. U.S. Department of Agriculture, Vol. 100. Washington, DC: Governance Printing Office.
Pimm, S. L., Lawton, J. H., and Cohen, J. E. (1991). Food web patterns and their consequences. Nature 350, 669–674.
Ponge, J. F. (1991). Food resources and diets of soil animals in a small area of Scots pine litter. Geoderma 49, 33–62. doi: 10.1016/0016-7061(91)90090-G
Ponge, J. F. (2003). Humus forms in terrestrial ecosystems: a framework to biodiversity. Soil Biol. Biochem. 35, 935–945. doi: 10.1016/S0038-0717(03)00149-4
Ponge, J. F. (2013). Plant-soil feedbacks by humus forms: a review. Soil Biol. Biochem. 57, 1048–1060. doi: 10.1016/j.soilbio.2012.07.019
Ponge, J. F., Chevalier, R., and Loussot, P. (2002). Humus Index: an integrated tool for the assessment of forest floor and topsoil properties. Soil Sci. Soc. Am. J. 66, 1996–2001. doi: 10.2136/sssaj2002.1996
Prosser, C. M., and Hedgpeth, B. M. (2018). Effects of bioturbation on environmental DNA migration through soil media. PLoS ONE 13:e0196430. doi: 10.1371/journal.pone.0196430
Puga-Freitas, R., and Blouin, M. (2015). A review of the effects of soil organisms on plant hormone signalling pathways. Environ. Exp. Bot. 114, 104–116. doi: 10.1016/j.envexpbot.2014.07.006
Renker, C., Otto, P., Schneider, K., Zimdars, B., Maraun, M., and Buscot, F. (2005). Oribatid mites as potential vectors for soil microfungi: study of mite-associated fungal species. Microb. Ecol. 50, 518–528. doi: 10.1007/s00248-005-5017-8
Sabatini, M. A., and Innocenti, G. (2001). Effects of Collembola on plant-pathogenic fungus interactions in simple experimental systems. Biol. Fertil. Soils 33, 62–66. doi: 10.1007/s003740000290
Scherber, C., Eisenhauer, N., Weisser, W. W., Schmid, B., Voigt, W., Fischer, M., et al. (2010). Bottom-up effects of plant diversity on multitrophic interactions in a biodiversity experiment. Nature 468, 553–556. doi: 10.1038/nature09492
Scheu, S. (1991). Mucus excretion and carbon turnover of endogeic earthworms. Biol. Fertil. Soils 12, 217–220 doi: 10.1007/BF00337206
Scheu, S., and Setälä, H. (2002). “Multitrophic interactions in decomposer food-webs,” in Multitrophic Level Interactions, eds T. Tscharntke, B.A. Hawkins (Cambridge, MA: Cambridge University Press), 223–264.
Schmidt, M. W., Torn, M. S., Abiven, S., Dittmar, T., Guggenberger, G., Janssens, I. A., et al. (2011). Persistence of soil organic matter as an ecosystem property. Nature 478, 49–56. doi: 10.1038/nature10386
Schoënholzer, F., Hahn, D., and Zeyer, J. (1999). Origins and fate of fungi and bacteria in the gut of Lumbricus terrestris L. studied by image analysis. FEMS Microbiol. Ecol. 28, 235–248. doi: 10.1111/j.1574-6941.1999.tb00579.x
Setälä, H., Berg, M. P., and Jones, T. H. (2005). “Trophic structure and functional redundancy in soil communities,” in Biological Diversity and Function in Soils, eds R. Bardgett, M. Usher and D. Hopkins (Cambridge: Cambridge University Press), 236–249.
Soliveres, S., van der Plas, F., Manning, P., Prati, D., Gossner, M. M., Renner, S. C., et al. (2016). Biodiversity at multiple trophic levels is needed for ecosystem multifunctionality. Nature 536, 456–459. doi: 10.1038/nature19092
Stephens, P. M., Davoren, C. W., Ryder, M. H., and Doube, B. M. (1994). Ability ofthe lumbricid earthworms Aporrectodea rosea and Aporrectodea trapezoides to reduce the severity of take-all under greenhouse and field conditions. Soil Biol. Biochem. 26, 1291–1297. doi: 10.1016/0038-0717(94)90209-7
Svensson, B. H., and Rosswall, T. (1980). Energy flow through the subarctic mire at Stordaien. Ecol. Bull. 30, 283–301.
Swift, M. J., Heal, O. W., and Anderson, J. M. (1979). Decomposition in Terrestrial Ecosystems. Oxford: Blackwell Science.
Tagger, S., Périssol, C., Criquet, S., Aubert, G., Neville, P., Le Petit, J., et al. (2008). Characterization of an amphimull under Mediterranean evergreen oak forest (Quercus ilex): micromorphological and biodynamic descriptions. Can. J. For. Res. 38, 268–277. doi: 10.1139/X07-165
Talbot, P. H. B. (1952). Dispersal of fungus spores by small animals inhabiting wood and bark. Trans. Brit. Mycol. Soc. 35, 123–128. doi: 10.1016/S0007-1536(52)80018-X
Tedersoo, L., Bahram, M., Põlme, S., Kõljalg, U., Yorou, N. S., Wijesundera, R., et al. (2014). Fungal biogeography. Global diversity and geography of soil fungi. Science 346:1256688. doi: 10.1126/science.1256688
Thomsen, P. F., and Willerslev, E. (2015). Environmental DNA – an emerging tool in conservation for monitoring past and present biodiversity. Biol. Conserv. 183, 4–18. doi: 10.1016/j.biocon.2014.11.019
Tsiafouli, M. A., Thébault, E., Sgardelis, S. P., De Ruiter, P. C., Van der Putten, W. H., Birkhofer, K., et al. (2015). Intensive agriculture reduces soil biodiversity across Europe. Glob. Change Biol. 21, 973–985. doi: 10.1111/gcb.12752
Van Altena, C., Hemerik, L., and de Ruiter, P. C. (2016). Food web stability and weighted connectance: the complexity-stability debate revisited. Theor. Ecol. 9, 49–58. doi: 10.1007/s12080-015-0291-7
Wada-Katsumata, A., Zurek, L., Nalyanya, G., Roelofs, W. L., Zhang, A., and Schal, C. (2015). Gut bacteria mediate aggregation in the German cockroach. Proc. Natl. Acad. Sci. USA. 112, 15678–15683. doi: 10.1073/pnas.1504031112
Wagg, C., Bender, S. F., Widmer, F., and van der Heijde, M. G. (2014). Soil biodiversity and soil community composition determine ecosystem multifunctionality. PNAS 111, 5266–5270. doi: 10.1073/pnas.1320054111
Wardle, D. A. (2002). Communities and Ecosystems: Linking the Aboveground and Belowground Components. Princeton: Princeton University Press.
Wu, T., Ayres, E., Bardgett, R. D., Wall, D. H., and Garey, J. R. (2011). Molecular study of worldwide distribution and diversity of soil animals. Proc.Natl Acad. Sci. USA. 108, 17720–17725. doi: 10.1073/pnas.1103824108
Wurst, S., Wagenaar, R., Biere, A., and der Putten, W. H. (2010). Microorganisms and nematodes increase levels of secondary metabolites in roots and root exudates of Plantago lanceolate. Plant Soil 329, 117–126. doi: 10.1007/s11104-009-0139-2
Xiao, Z., Wang, X., Koricheva, J., Kergunteuil, A., Le Bayon, R.-C., Liu, M., et al. (2018). Earthworms affect plant growth and resistance against herbivores: a meta-analysis. Funct. Ecol. 32, 150–160. doi: 10.1111/1365-2435.12969
Yan, S., Singh, A. N., Fu, S., Liao, C., Wang, S., Li, Y., et al. (2012). A soil fauna index for assessing soil quality. Soil Biol. Biochem. 47, 158–165. doi: 10.1016/j.soilbio.2011.11.014
Yeates, G. W. (1998). “Feeding in free-living soil nematodes: a functional approach,” in The Physiology and Biochemistry of Free-Living and Plant-Parasitic Nematodes, eds R. N. Perry and D. J. Wright (Wallingford: CAB international), 245–269.
Zanella, A., Ponge, J. F., and Briones, M. J. I. (2017). Humusica 1, article 8: terrestrial humus systems and forms – biological activity and soil aggregates, space-time dynamics. Appl. Soil Ecol. 122, 103–137. doi: 10.1016/j.apsoil.2017.07.020
Keywords: soil ecology, plant-soil interactions, soil fauna-microbial interactions, soil food web, non-trophic interactions, functional diversity
Citation: Briones MJI (2018) The Serendipitous Value of Soil Fauna in Ecosystem Functioning: The Unexplained Explained. Front. Environ. Sci. 6:149. doi: 10.3389/fenvs.2018.00149
Received: 30 August 2018; Accepted: 23 November 2018;
Published: 07 December 2018.
Edited by:
Philippe C. Baveye, AgroParisTech Institut des Sciences et Industries du Vivant et de L'environnement, FranceReviewed by:
David Coleman, University of Georgia, United StatesJulia Clause, University of Poitiers, France
Copyright © 2018 Briones. This is an open-access article distributed under the terms of the Creative Commons Attribution License (CC BY). The use, distribution or reproduction in other forums is permitted, provided the original author(s) and the copyright owner(s) are credited and that the original publication in this journal is cited, in accordance with accepted academic practice. No use, distribution or reproduction is permitted which does not comply with these terms.
*Correspondence: Maria J. I. Briones, bWJyaW9uZXNAdXZpZ28uZXM=