- 1Instituto de Microbiologia Paulo de Góes, Universidade Federal do Rio de Janeiro, Rio de Janeiro, Brazil
- 2Departamento de Ciência do Solo, Escola Superior de Agricultura Luiz de Queiroz, Universidade de São Paulo, Piracicaba, Brazil
- 3Instituto de Biofísica, Universidade Federal do Rio de Janeiro, Rio de Janeiro, Brazil
- 4Departamento de Fitotecnia, Centro de Ciências Agrárias, Universidade Federal de Viçosa, Viçosa, Brazil
- 5Empresa Brasileira de Pesquisa Agropecuária, Centro Nacional de Pesquisas de Milho e Sorgo, Sete Lagoas, Brazil
Maize is an important food source worldwide and is of considerable industrial importance. Low maize yields are mostly due to low soil fertility, so expensive mineral fertilizers are often used to offset the lack of nutrients. Poultry litter (PL) is one of the most valuable and phosphorous-rich animal wastes. However, PL usually contains veterinary antibiotic residues, particularly fluoroquinolones (FQs), which may alter soil microorganism diversity and resistance patterns. In this study, we aimed to understand the impact of applying mineral (triple superphosphate–STP) or organomineral (STP with PL and reactive Bayovar phosphate with PL) fertilizers (130 or 260 kg/ha of total P2O5) on the structure and composition of the soil bacteriome and on phosphate-mineralizing bacteria associated with the maize rhizosphere. Maize plants were sampled at 60 and 90 days after sowing and a clear rhizosphere effect was observed in all samples. No specific groups of bacterial genera predominated (>3% relative abundance) according to the different fertilizer treatments and most of the genera were shared among samples. Multivariate analyses of 16S rRNA sequences revealed clear clustering based on sampling time and distinct separation from bulk soil samples. Abundances of phosphate-mineralizing bacteria varied depending on the sampling time. We observed a positive effect on phytase activity under the 260 kg STP with PL treatment. Although the FQ enrofloxacin and its main metabolite ciprofloxacin were detected in PL, their concentrations in fertilized soils were below quantification thresholds. Quinolone resistance genes were not detected in the maize rhizosphere or bulk soil. Together, these results suggest that the rhizosphere effect, plant age and applied amounts of fertilizer are more influential on bacterial communities than the type of fertilizer used. Thus, application of PL as an organomineral fertilizer does not appear to have extensive impacts on the bacterial diversity of maize rhizosphere, so it could be an excellent option for enhancing maize production.
Introduction
Maize (Zea mays L.) is an important human food source (mainly in southern and eastern Africa, Central America and Mexico), a major component of livestock feed, and it has several industrial uses, such as starch, sweeteners, oil, beverages, glue and industrial alcohol (Ranum et al., 2014). A significant proportion of maize production is dedicated to generating ethanol fuel (ethyl alcohol), frequently used as a biofuel additive for gasoline (Gaurav et al., 2017; Eckert et al., 2018).
Although maize production has grown steadily over the years, low yields in many countries are mostly due to poor fertility of the cultivated lands. Low phosphorus (P) and nitrogen (N) availability are the major constraints to maize production, so mineral fertilizers are applied to offset these nutrient deficiencies. Although usually effective, mineral fertilizers are quite expensive because they often utilize non-renewable raw materials and/or involve high production costs (Novais and Mello, 2007).
Water-soluble phosphates (superphosphates, diammonium phosphate) and rock phosphates are among the most applied inorganic phosphate fertilizers (Ramachandra, 2006). The Bayovar phosphate mine located in Peru is one of the biggest rock deposits in South America, producing 3.9 million metric tons per year. Although direct application of phosphate rock is an interesting low-cost option for supplying phosphorus to soil, different studies have already demonstrated that consequent crop yield is not enhanced to levels obtained for soluble phosphates (Chien and Menon, 1995; Franzini et al., 2009).
An alternative way to improve the physical and biological properties of soil and consequently to increase crop yields is to use organic fertilizers (alone or in combination with mineral fertilizers), such as animal litter, green manure or compost (Melkamu, 2012). The efficacy of organic fertilizers for improving maize growth, grain and dry matter yields, and/or harvest index has previously been demonstrated (Ademba et al., 2014, 2015; Kidinda et al., 2015; Martins et al., 2017; Baghdadi et al., 2018).
Poultry litter (PL) is a mixture of feces, food waste, bedding material and feathers (Chen and Jiang, 2014). Millions of tons of PL are produced every year worldwide, and it is considered one of the most valuable organic fertilizers (Wilkinson, 1979). PL is usually recycled and spread on arable land, minimizing the problem of its disposal in the environment and instead adding value to it. However, PL usually contains veterinary antibiotic residues, mainly fluoroquinolones (FQs) (Picó and Andreu, 2007; Zhao et al., 2010; Nõlvak et al., 2016), which may alter ecosystems. These residues may negatively affect the large and diverse population of microorganisms in PL or in PL-based organic fertilizers or PL-fertilized soils, changing the diversity and resistance patterns of the original microbiome (Kemper, 2008; Leal et al., 2012). Moreover, a variety of human pathogens can be introduced into fertilized soil when treated with PL (Chinivasagam et al., 2010; Chen and Jiang, 2014).
PL-based organic fertilization can have extensive impacts on microbial ecology. Microorganisms involved in nitrogen or phosphorus cycles may be positively or negatively affected by such fertilizers, particularly in terms of their enzymatic activities. However, few studies have compared the individual or synergistic impacts of inorganic or organic fertilizers on bacterial communities (Poulsen et al., 2013; Nõlvak et al., 2016). In this study, we used high-throughput sequencing of the bacterial 16S rRNA-encoding gene to examine the responses of bacterial communities in maize rhizosphere to different fertilizer regimes (mineral alone and a combined mineral and organic fertilizer, applied at two doses of total P2O5). Moreover, we determined the presence of FQs in the soil and the influence of fertilizers on the activity of enzymes involved in soil phosphorus cycles.
The overall objective of this study is to understand the effects of different fertilization strategies (inorganic alone or inorganic plus organic fertilizers) on the diversity and composition of rhizosphere soil bacteriomes and on phosphate-mineralizing bacteria. We used PL as a constituent of the organomineral fertilizer we applied. Assuming that each soil fertilization treatment has a different influence on maize rhizosphere, we hypothesized that: (i) maize rhizosphere is affected by the type of fertilization strategy and, consequently, affects the composition of the soil bacterial communities associated with it; and (ii) these variations in bacterial communities reflect changes in the abundance and activity of phosphate-mineralizing bacteria.
Materials and Methods
Experimental Area and Soil Sampling
The samples used in this study were collected from an experimental field located at “EMBRAPA Milho e Sorgo,” in the city of Sete Lagoas (geographical coordinates: 19° 28′ 36″ South; 44° 11′ 53″ West), State of Minas Gerais, Brazil. The soil of this area is characterized as dark-red acid distrophic latosol (Typic Hplustox), with a clayey texture. The chemical characteristics of the soil are: pH in water−5.9; organic matter−3.6 dag/kg; contents of P (Mehlich-1)−6 mg/dm3, K (Mehlich-1)−105 mg/dm3, Ca−4.4 cmolc/dm3, Mg−0.8 cmolc/dm3, Al−0.0 cmolc/dm3, H + Al−4.3 cmolc/dm3 and cation-exchange capacity (CTC) potential−9.8 cmolc/dm3. We used the maize genotype DKB 390 PRO obtained from EMBRAPA. The experimental area consisted of a completely randomized 4-fold casual block design in a 3 × 2 + 1 factorial scheme. The first factor corresponded to three different sources of phosphorus: triple superphosphate (STP, total P2O5 = 44%), organomineral based on PL plus triple superphosphate (STP_with_PL, total P2O5 = 13.4%), and organomineral with reactive Bayovar phosphate (BAY_with_PL, total P2O5 = 16.3%), each of which was applied at doses of 130 or 260 kg/ha of total P2O5 (second factor). All treatments were compared to a control based on absence of phosphate fertilization. N and/or K fertilizer was applied manually using 300 kg/ha of an NPK formula (20-00-20). Details of the experimental set-up have been described previously (Martins et al., 2017).
Plants were harvested at two time-points: at flowering (60 days after sowing) and during grain-filling and maturity (90 days after sowing). At each time-point, four sets of four plants were harvested, and their roots were shaken to remove loosely attached soil. The adhering soil from the four plants was pooled and was considered rhizosphere soil. Corresponding bulk soil samples were taken from the same field in areas free of root activity. Samples were transported to the laboratory on ice and kept at −20°C before DNA extraction.
DNA Extraction of Soil Samples
Total DNA from the microbial community was extracted directly from the rhizosphere and bulk soil samples (0.5 g of each sample) using the DNeasy PowerSoil Kit (QIAGEN, USA). DNA preparations were visualized by electrophoresis in a 0.8% agarose gel in 1× TBE buffer (Sambrook et al., 1989) to assess their integrity. Samples were then stored at −20°C prior to PCR amplifications.
Detection of Quinolone-Resistance (qnr) Genes
The DNA extracted from soil samples was subjected to PCR amplification of quinolone-resistance genes (qnrA, qnrB and qnrS) in separate reactions. PCR was performed in 25-μl reactions containing 1× PCR buffer, 2 U of Taq DNA polymerase (Promega), 200 μM each deoxynucleotide triphosphate (dNTP), 5 pmol of each forward and reverse primer (listed in Kraychete et al., 2016), 1.5 mM MgCl2, and 2 μl template DNA (20–40 ng). PCR conditions were: 10 min at 95°C; 25 cycles of amplification consisting of 45 s at 95°C, 45 s at 58°C and 15 s at 72°C; and then 3 min at 72°C (described in detail by Kraychete et al., 2016). DNA fragments were analyzed by electrophoresis in a 1.4% agarose gel at 80 V for 1 h in 1× TBE buffer followed by staining with ethidium bromide.
Quantification of Fluoroquinolones (FQs) in Poultry Litter and in Soil Samples
Enrofloxacin (ENR) and ciprofloxacin (CIP) standards, both ≥98% purity (HPLC), were purchased from Sigma-Aldrich (Saint Louis, MO, USA). Extraction of FQs from soil and PL was performed as described in Turiel et al. (2006). FQs were determined using a HPLC system (CBM-20A) with a quaternary pump (LC-10ATVP) and a fluorescence detector (RF-10AXL–Shimadzu Corp., Japan). We used a C18 analytical column of dimensions 250 × 4.6 mm and 5 μm (Kromasil®, Sweden). The mobile phase (flow 1.0 ml/min) was composed of 0.02 M o-H3PO4:ACN (80:20; Uslu et al., 2008). For recovery tests, five replicates of PL and of soil were spiked with ENR and CIP before the extraction procedure. For PL, recoveries and coefficients of variation were 81.7% and 5.4 for ENR and 75.4% and 3.4 for CIP, respectively. For soil samples, recoveries and coefficients of variation were 77.2% and 2.9 for ENR and 77.5% and 2.6 for CIP, respectively. The limits of quantification (LOQ) in PL were 171 μg/kg (ENR) and 271 μg/kg (CIP), and for soil they were 116 μg/kg (ENR) and 189 μg/kg (CIP).
High-Throughput Sequencing and Data Analysis
The DNA extracted from soil samples was used for PCR amplification using the forward primer 515F (5′-GTGCCAGCMGCCGCGGTAA-3′) and the reverse primer 806R (5′-GGACTACHVGGGTWTCTAAT-3′) that target the V4 region of the rrs gene (Caporaso et al., 2011). Amplification, pooling, and purification were performed by Macrogen (South Korea). All samples were sequenced using a MiSeq Platform and the MiSeq Reagent kit v3 (Illumina, USA). All subsequent analyses were carried out with the software package QIIME 1 (Quantitative Insights into Microbial Ecology toolkit; Caporaso et al., 2010a). All sequences were quality-filtered as described by Bokulich et al. (2013). The remaining sequences were clustered in operational taxonomic units (OTUs) at 97% sequence identity using USEARCH 6.1 (v6.1.544), followed by selection of a representative sequence for each OTU (Edgar, 2010). Chimeric sequences were identified using USEARCH 6.1 (v6.1.544) and removed (Edgar, 2010). Representative sequences from each OTU were aligned with the Greengenes Core Set (DeSantis et al., 2006) using PyNAST (Caporaso et al., 2010b). Taxonomy was assigned to sequences using the BLAST tool (Altschul et al., 1997). Before further analysis, singletons, chloroplast plastids, mitochondria, and archaeal sequences were manually removed from the dataset. The OTU-generated matrices were then used to calculate α diversity and β diversity. Species diversity was calculated using Chao1 estimators (Chao, 1987) and the Shannon-Weaver diversity index (Shannon and Weaver, 1949). All sequences have been deposited to the Sequence Read Archive (SRA) of the National Center for Biotechnology Information (NCBI) under the accession number SRP151585.
Statistical Analyses
Statistical analyses were performed with PAST 3.16 software (Hammer et al., 2001). Analysis of variance (ANOVA) followed by Tukey's pairwise test was used to determine significant differences among diversity indexes (Chao1 and Shannon) obtained for each treatment. Differences were considered significant when p < 0.05. For β-diversity metrics, non-metric multidimensional scaling (NMDS–Bray-Curtis distance) analyses were performed based on OTU-generated matrices exported into PAST software.
Abundances of Phosphate-Mineralizing Bacteria
Absolute quantifications for each sample were carried out twice using the StepOne Real-time System (Applied Biosystems, USA). Abundances of phosphate mineralizing communities were estimated by using the phoD (alkaline phosphatase) gene as a proxy. We chose this gene as representative of the phosphate-mineralizing process. The amplification reaction was carried out using the primers ALPS-F730 (5′ CAG TGG GAC GAC CAC GAG GT 3′) and ALPS-R1101 (5′ GAG GCC GAT CGG CAT GTC G 3′; Sakurai et al., 2008) in a 25-μl PCR reaction containing 12.5 μl of 2-fold PCR master mix (SYBR Green PCR Master mix; Applied Biosystems), 0.2 μM of each primer, and 1 μl of sample DNA. Thermal cycling was as follows: 94°C for 15 min; 35 cycles of 94°C for 1 min, 57°C for 1 min, and 72°C for 2 min. We established a dissociation curve after the final cycle. For the construction of a standard curve, we amplified a soil DNA sample (soil previously cultivated with maize) using the above-mentioned primers. The resulting amplicon was cloned into the pGEM-T vector (Promega, The Netherlands). Randomly selected clones were Sanger-sequenced with the M13F primer at the facilities of the University of São Paulo, Brazil (http://genfis40.esalq.usp.br/multi/) and the resulting sequences were compared to database sequences using the BLAST tool. After the confirmation of the insertion of phoD gene sequence in different clones, we used a representative one to construct the standard curve for quantitative PCR. The serial dilution ranged from 1011 to 104 gene copy numbers/μl. Specificity of the amplification products was confirmed by melting curve analyses, and the expected sizes of the amplified fragments were checked in a 1% agarose gel.
Phosphatase and Phytase Activity Assays
Phosphatase activities at pH 6.5 and 11 were determined for soil samples corresponding to all treatments according to the protocol presented in Tabatabai (1994). Briefly, 4 ml of modified universal buffer (MUB pH 6.5) and 1 ml (concentration of 10 mM/g soil) of para-nitrophenol phosphate (pNPP) (Sigma-Aldrich, USA) were added to 1 g of fresh soil and incubated at 37°C for 1 h. After this period, the samples were filtered through Whatman 42 filter paper and the resulting color intensities were measured at 420 nm using a Nova 2000 spectrophotometer (Nova Instruments, Brazil). Values are expressed as μM of pNPP produced per kg of soil in 1 h.
The amount of available phosphorus using calcium phytate as substrate was measured to establish phytase activity. To do that, we incubated 1 g of fresh soil (in triplicate) with sodium acetate buffer and 0.1 M of calcium phytate at 37°C for 1 h. We then added 0.5 ml of trichloroacetic acid (10%) and incubated the mixture for 20 min. The samples were then filtered through Whatman 42 filter paper and the filtrate was mixed with ammonium molybdate plus ammonium metavanadate solution for 10 min. The resulting color intensities were measured at 420 nm using a Nova 2000 spectrophotometer. Values are expressed as mg/ml of available P per kg of soil (Ames, 1966; Chen et al., 2006).
The correlation between phosphatase activity and phoD gene abundance was determined by linear regression using the R project for Statistical Computing (R Core Team, 2013).
Results
Composition of Bacterial Communities in Maize Rhizosphere
The diversity and composition of the bacterial communities in the maize rhizosphere subjected to different fertilization treatments were determined using direct-extraction of bacterial community DNA (60 and 90 days after sowing maize). High-throughput sequencing (Illumina MiSeq) of the V4 region of 16S rRNA generated 2,203,160 sequences. The sequencing data were rarified to 5,100 reads per sample based on the lowest number of sequences obtained in one sample. OTU numbers and the diversity measures obtained from each treatment are shown in Table 1. We assessed significant differences among diversity values based on Tukey's pairwise comparisons (p < 0.05). The number of OTUs varied on average from 1,196 (bulk soil_60d) to 1,491 (130 kg_BAY_with_PL_90d; Table 1). Values for the richness and diversity indices increased between the two sampling time-points (from 60 to 90 days of sowing). We also observed a statistically significant difference in bacterial richness (Chao1) for bulk soil samples (taken after 90 days) compared with all fertilizer-treated samples taken at the same time-point. Differences in diversity indices among samples are presented in Table 1.
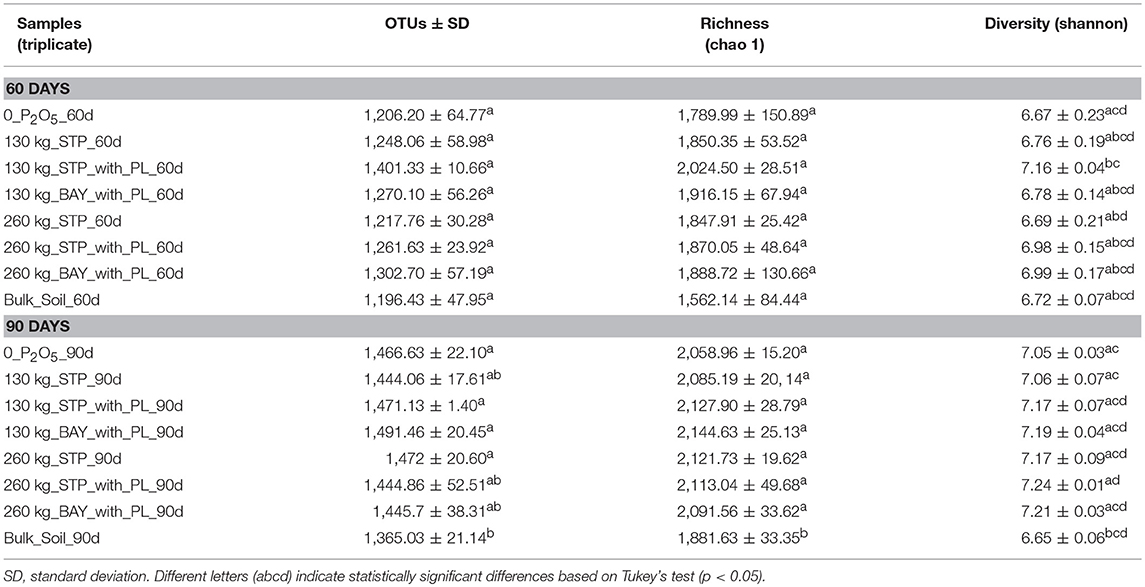
Table 1. Estimated OTUs, as well as richness and diversity indices based on the OTU generated matrices.
We considered each replicate separately for OTU analyses. Only taxonomic groups representing >3% of relative abundance were considered for further analyses, with the remainder (all replicates with <3% of relative abundance) being denoted as “others.” Considering taxonomic groups with >3% of the relative abundance, the same phyla and/or classes were shared in samples obtained after 60 and 90 days of sowing (with the exception of Deltaproteobacteria only found in samples of 60 days; Figures 1A,B). The phylum Proteobacteria predominated in all rhizosphere samples (more than 40 and 30% of relative abundances in samples taken 60 and 90 days after sowing, respectively). In bulk soil, ~31% (60 days) and 16% (90 days) of the OTUs represented this phylum. Alphaproteobacteria and Betaproteobacteria constituted the major classes, followed by Gammaproteobacteria. Comparing the relative abundances of OTUs found for our two time-periods, we observed a slight decrease of Betaproteobacteria and Gammaproteobacteria and the absence of Deltaproteobacteria in all fertilizer treatments analyzed after 90 days of maize sowing (Figures 1A,B).
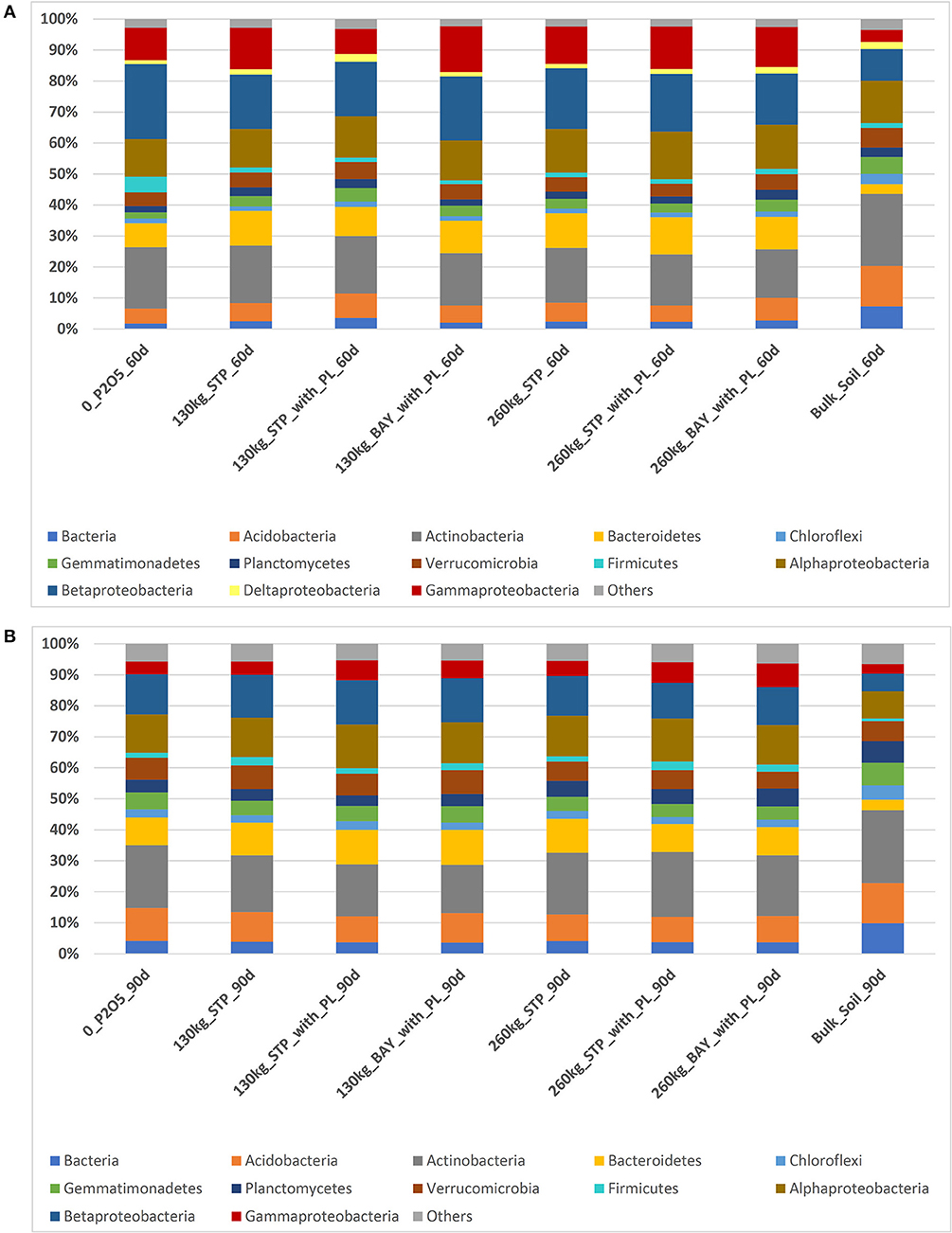
Figure 1. Relative abundances of the most abundant bacterial phyla and Proteobacterial classes found in maize rhizosphere subjected to different phosphorus fertilizers and in bulk soil, as determined by high-throughput sequencing. (A) Samples taken 60 days after sowing maize, and (B) Samples taken 90 days after sowing maize.
No specific groups of bacterial genera predominated according to the different fertilizer treatments. Moreover, most genera were shared among samples obtained after 60 and 90 days of sowing (considering only those with >3% of relative abundance; Figures 2A,B). More than 60 and 70% of taxonomic groups represented <3% of the relative abundances in samples obtained after 60 and 90 days of sowing, respectively. A slight increase of Acidobacteria, Rhodoplanes and Gaiellaceae were observed after 90 days of sowing, as was a decrease of Sphingobium (Figures 2A,B). Genera, such as Kaistobacter and Rhodoplanes were observed in all samples and for both sampling time-periods (Figures 2A,B).
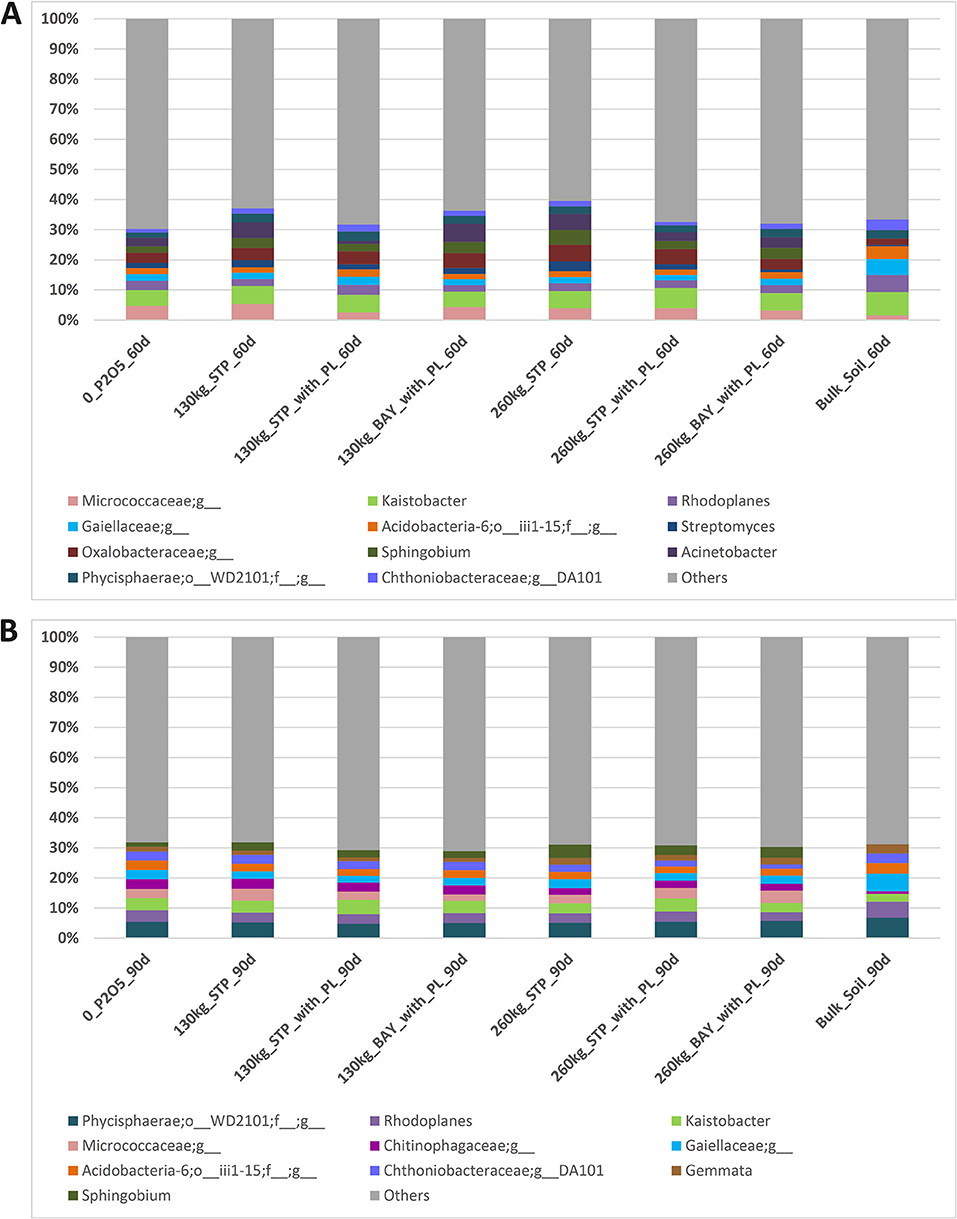
Figure 2. Relative abundances of the most abundant bacterial genera found in maize rhizosphere subjected to different phosphorus fertilizers and in bulk soil, as determined by high-throughput sequencing. (A) Samples taken 60 days after sowing maize, and (B) Samples taken 90 days after sowing maize.
Multivariate Analyses of 16S rRNA-Based OTUs
When we analyzed all samples together, NMDS of 16S rRNA-based OTUs revealed clear clustering based on sampling time (60 or 90 days after maize sowing) and the distinctiveness of bulk soil samples (Figure 3A). We could not distinguish a pattern for OTUs from the 60-day time-point based on fertilizer type or amount of fertilizer applied (Figure 3B). However, OTUs of the samples taken 90 days after sowing could be grouped according to fertilizer dosage (130 × 260 kg), but not by the type of fertilizer used (Figure 3C). The same groupings were observed when we assessed separately taxonomic groups representing more or <3% of relative abundance (Figures S1A–F).
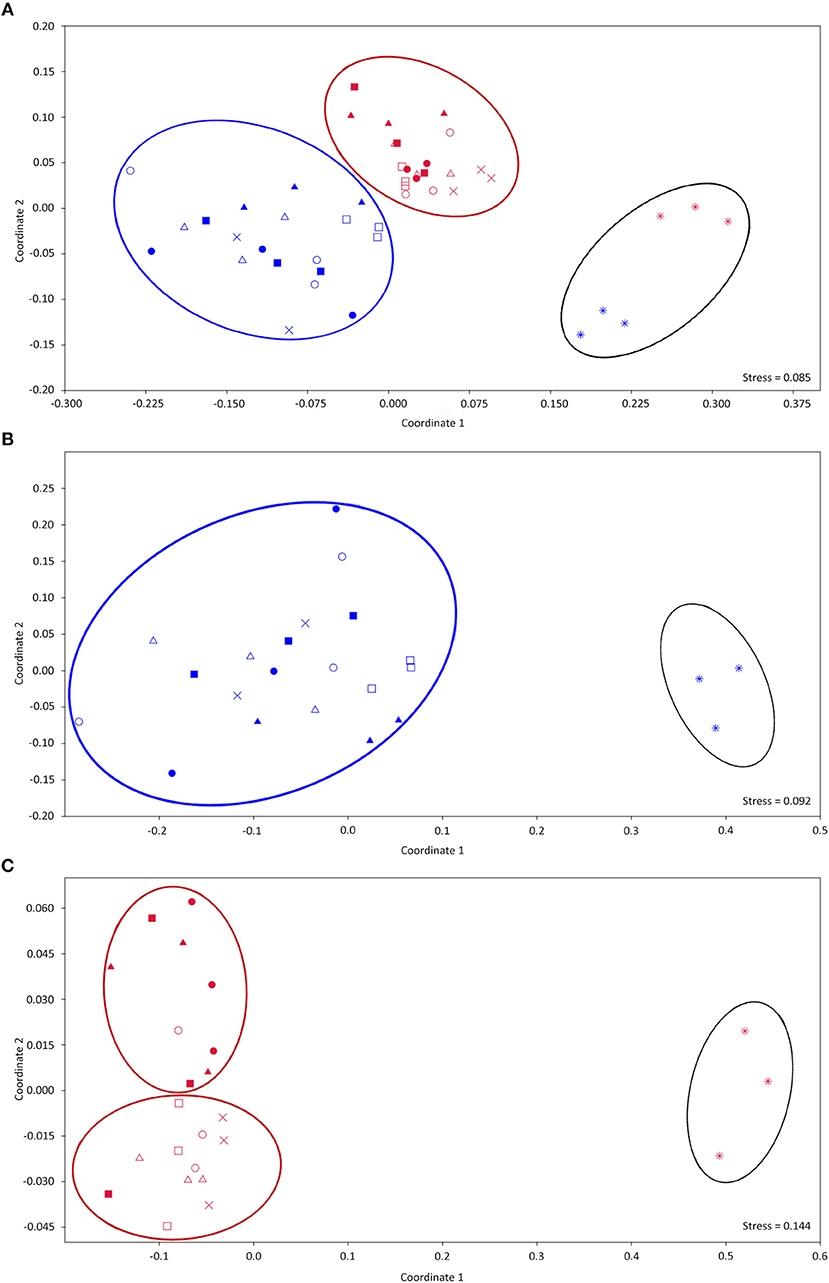
Figure 3. Non-metric multidimensional scaling (NMDS) ordination diagram based on the OTU-generated matrices obtained after sequencing using primers for the 16S rRNA-coding gene. (A) All samples analyzed together, (B) Samples taken 60 days after sowing, and (C) Samples taken 90 days after sowing. *, Bulk soil; ×, 0_P2O5; □, 130 kg_STP_PL; ■, 260 kg_STP_PL; Δ, 130 kg_BAY_PL; ▴, 260 kg_BAY_PL; ◦, 130 kg_STP; •, 260 kg_STP; blue and red colors represent 60 and 90 days after sowing, respectively.
Abundance of Phosphate-Mineralizing Bacteria
Abundances of phosphate-mineralizing bacteria were evaluated in rhizosphere soil subjected to the different fertilizer treatments and in bulk soil, for both sampling periods. Generally, abundances of these microorganisms differed between sampling time-points. Samples taken 90 days after sowing showed higher abundances of phosphate-mineralizing bacteria than those taken 60 days after sowing. Copy numbers of the phoD gene per g of soil varied from 5.4 × 105 to 3.2 × 106 (60 days) and from 1.4 × 108 to 1.1 × 109 (90 days; Figure 4). There was no clear effect of fertilizer addition on the rhizosphere in terms of abundance of phosphate-mineralizing bacteria 60 days after sowing. We recorded a lower abundance of phoD gene copies in bulk soil compared to 260 kg_STP_with_PL, 130 kg_BAY_with_PL, 130 kg_STP and 0_P2O5 (Figure 4A). In samples taken 90 days after sowing, we noted a trend of increasing number of phoD gene copies for higher dosage fertilizer treatments (260 kg STP, 260 kg STP_with_PL and 260 kg BAY_with_PL). However, there was no statistically significant difference among all treatments, including among the bulk and rhizosphere soils (Figure 4B).
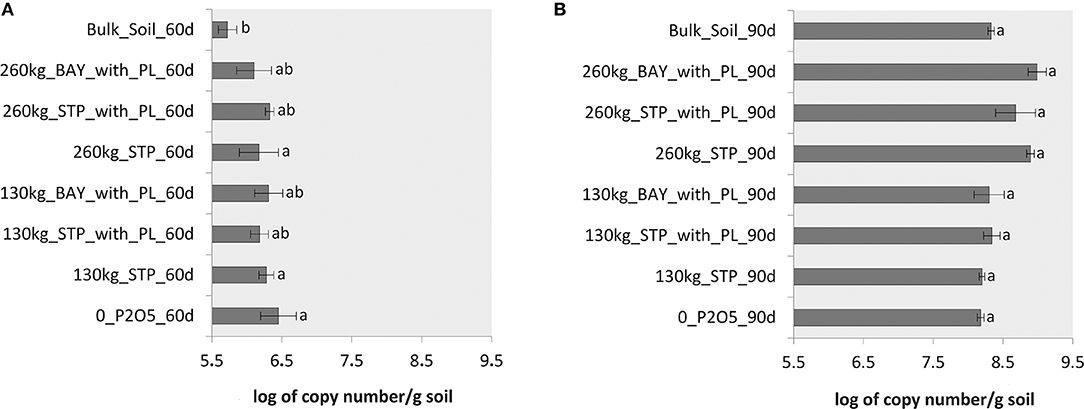
Figure 4. Abundances of phosphate-mineralizing bacteria (phoD gene). (A) Samples taken 60 days after sowing, and (B) Samples taken 90 days after sowing maize. Treatments (in A or B) that do not share a letter have a mean difference that is statistically significant based on Tukey's pairwise test (p < 0.05).
Enzymatic Activities: Phytase and Phosphatases
We found an effect on phytase activity of the amount of inorganic phosphate fertilizer added with PL (260 kg STP_with_PL) for both time-periods (60 and 90 days after sowing). Sole addition of 260 kg STP did not enhance phytase activity (Figures 5A,B). Phosphatase activities (pH 6.5) at 60 days after sowing varied from 367.17 to 1,009.02 mg pNPP/kg of soil/h (Figure 5C), whereas phosphatase activities 90 days after sowing varied from 303.23 to 620.53 mg pNPP/kg of soil/h (Figure 5D). We found no evidence for differences between rhizosphere and bulk samples, nor among the different fertilizers applied, for samples taken 90 days after sowing (Figure 5D). Phosphatase activity at pH 6.5 was positively correlated with phoD gene abundance for samples taken 60 days after sowing (r2 = 0.4903; p = 0.0001; Figure S2A). Phosphatase activities at pH 11 were lower than those at pH 6.5, with values ranging from 219.8 to 451.2 (60 days after sowing–Figure 5E) and from 120.2 to 364 mg pNPP/kg of soil/h (90 days after sowing–Figure 5F). The lowest phosphatase activity at pH 11 was observed for bulk soil samples. No increase in phosphatase activities was observed relating to the addition of the different fertilizers (Figures 5E,F). However, this phosphatase activity was positively correlated with phoD gene abundance at 60 days (r2 = 0.3217 and p = 0.03; Figure S2B). No correlation between phoD gene abundance and phosphatase activity (both acid and alkaline) was observed at 90 days (data not shown).
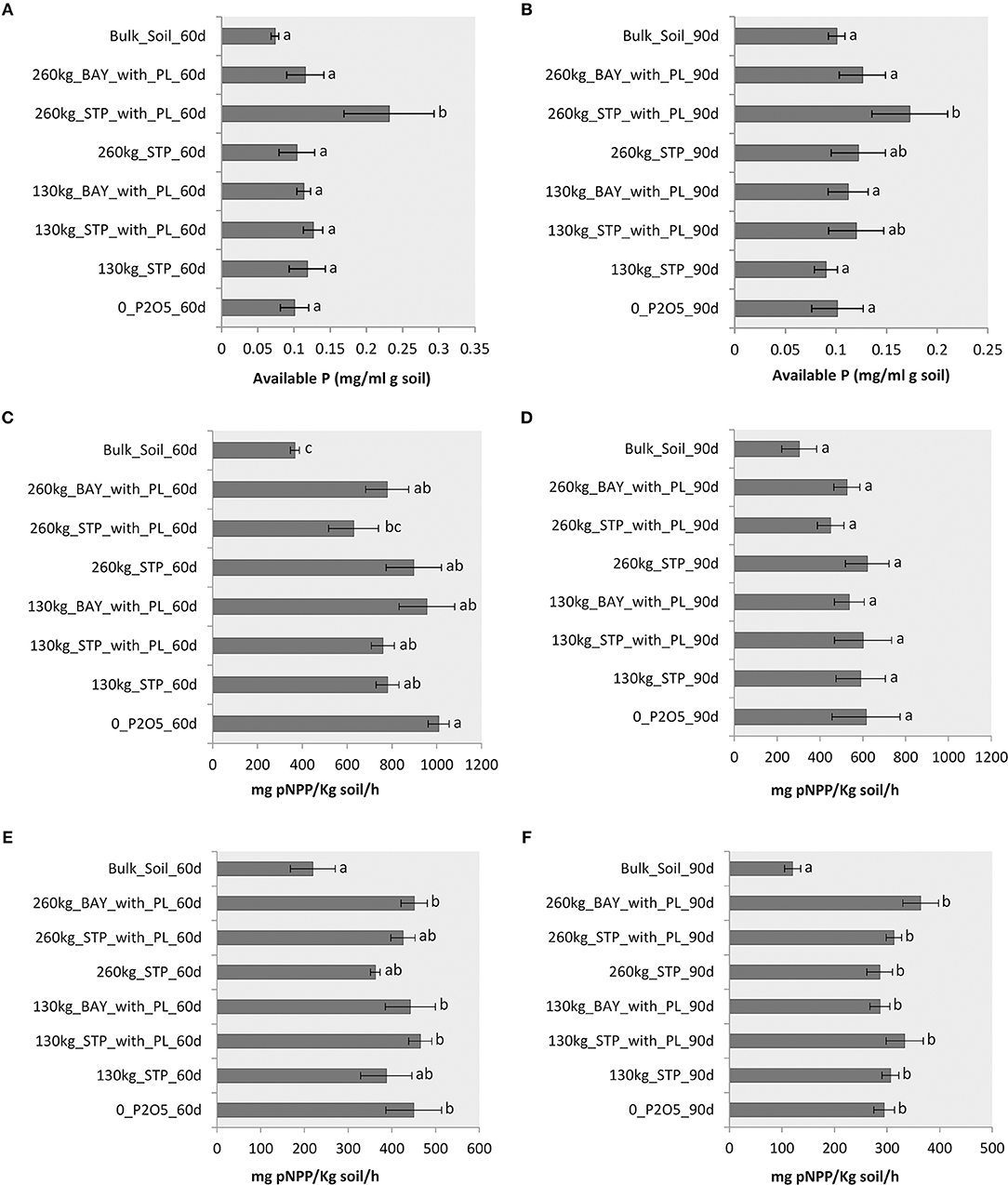
Figure 5. (A) Phytase activity, measured 60 days after sowing maize; (B) Phytase activity, measured 90 days after sowing maize; (C) Phosphatase activity in pH 6.5, measured 60 days after sowing maize; (D) Phosphatase activity in pH 6.5, measured 90 days after sowing maize; (E) Phosphatase activity in pH 11.0, measured 60 days after sowing maize; and (F) Phosphatase activity in pH 11, measured 90 days after sowing maize. Treatments (in each panel) that do not share a letter have a mean difference that is statistically significant based on Tukey's pairwise test (p < 0.05).
Concentration of Fluoroquinolones in Poultry Litter and in Soil Samples
We determined the concentrations of ENR and CIP in PL prior to preparing the fertilizer mixtures with BAY or STP. The combined concentration of these two antibiotics in PL was 3,620 μg/kg (comprising 424 μg/kg of ENR and 3,196 μg/kg of CIP). Concentrations of FQs were below the limits of quantification (<LOQ) in fertilized soil samples.
Detection of Quinolone-Resistance (qnr) Genes
The prevalence of three plasmid-mediated quinolone resistance (PMQR) determinants—qnrA, qnrB, qnrS— were determined in PL, in maize rhizosphere under different fertilizer treatments, and in bulk soil. We only detected the expected PCR products in our positive controls (samples of different quinolone-resistant bacteria; data not shown), suggesting that these genes are not present or, if they are present in our soil samples and in the PL we used, they occur in copy numbers below the detection limit of our PCR technique.
Discussion
The effects of different types of fertilizers on the composition of soil microbial communities remain a hotly debated topic. Various studies have linked changes in soil bacterial community structure and composition to nutrient availability, so applications of different types of fertilizers may distinctly alter the predominant microbial taxa (Hartmann et al., 2015; Li et al., 2015, and others). However, other studies have demonstrated that inorganic and/or organic fertilizers have had relatively little or no effect on soil microbial diversity or activities (Okano et al., 2004; Treseder, 2008; Dong et al., 2014). Here, we investigated the effects of adding mineral or organomineral (constituting PL) phosphorus fertilizers at different dosages (130 and 260 kg/ha) on the composition of the soil bacteriome and on phosphate-mineralizing bacteria associated with maize rhizosphere. We found that the type of phosphorus in fertilizers did not greatly influence the bacterial community present in maize rhizosphere. Instead, observed changes in bacterial composition were due to either plant age or the amount of the fertilizer used. Our NMDS revealed an evident rhizosphere effect (Figure 3), which was independent of the fertilizer type applied.
One of our primary objectives was to assess the microbiological safety of using PL-based organic fertilizers to enhance soil nutrients. PL usually contains a large and diverse population of primarily Gram-positive bacteria, such as Actinomycetes, Clostridia/Eubacteria, and Bacilli/Lactobacilli (Bolan et al., 2010). Some food-borne pathogens may survive for long periods in raw PL (Chen and Jiang, 2014), and the presence of a variety of human pathogens (members of the orders Clostridiales and Pseudomonadales) has been demonstrated in soil treated with poultry manure (Faissal et al., 2017). When we examined the predominant genera found in maize rhizospheres under different fertilizer treatments, we did not detect any known potential pathogens. Instead, OTUs related to genera, such as Sphingobium, Kaistobacter, and Rhodoplanes, all belonging to the Alphaproteobacteria, predominated in all of our soil samples (Figures 1, 2).
A major problem with using PL as a phosphorous fertilizer is the presence of FQs, which represent one of the major groups of antibiotics applied in animal husbandry (Zhang et al., 2015). FQs can threaten ecosystems by encouraging the selection of resistant bacteria and increased expression of resistance genes (Luo et al., 2010). Authorities from a variety of countries have expressed concern regarding the development of resistance in microorganisms that are pathogenic to both humans and animals, such as Campylobacter spp. and Salmonella spp. (Gouvêa and Santos, 2015). For example, Hou et al. (2015) reported more than 800 μg/kg of CIP in animal manure samples in China, and CIP is usually prescribed as a treatment against a range of diseases in humans (Leal et al., 2012). Numerous pathogens now exhibit enhanced resistance due to the widespread veterinary usage of FQs (Fair and Tor, 2014). Fortunately, in our fertilized soil samples, we found that the concentrations of two antibiotics (ENR and CIP) were below the limits of quantification (<LOQ), minimizing the abovementioned risks. However, the soil microbiota was definitely exposed to both ENR and CIP, as their concentrations in PL were high. At the end of our experimental period, we assessed the presence of quinolone-resistance determinants (qnrA, qnrB, qnrS) in all samples, but no respective PCR products were observed either in the PL-fertilized or control samples, including bulk soil. This result suggests that gene copy numbers of qnrA, qnrB, and qnrS (and, consequently, numbers of quinolone-resistant bacteria) did not increase considerably in the PL-fertilized samples.
Concomitantly to the current study, Martins et al. (2017) determined the effects of added fertilizer on different plant parameters, such as plant height, stem diameter and number of grains per row in the same experimental area. That study showed that application of organomineral fertilizer (STP with PL) increased grain production, resulting in mean productivity equal to or greater than that obtained when STP was exclusively applied. The same effect was not observed when Bayovar was used together with PL. Fontoura et al. (2010) observed that STP was more efficient than reactive natural phosphates in a study of different phosphorus regimes in a non-tillage system.
We observed a positive effect on phytase activity when 260 kg STP with PL was used, at both sampled time-periods (i.e., 60 and 90 days after sowing). This higher phytase activity (shown in Figures 5A,B) may have contributed to the increased productivity reported by Martins et al. (2017). However, no clear differences were observed among the different fertilizer regimes in terms of phosphatase activity at either pH 6.5 or 11 (Figures 5C–F). Although soil phosphatase activity can be a good indicator of the organic P mineralization potential and biological activity of soils (Sakurai et al., 2008), production and secretion of phosphatase are stimulated under conditions of low inorganic P concentration (Tan et al., 2013; Fraser et al., 2015). Therefore, inorganic fertilizers added to PL may have disrupted microbial phosphatase activity, perhaps explaining why we did not observe an increase in phosphatase activities.
Furthermore, we did not observe a strong effect of fertilizer addition on the abundance of phosphate-mineralizing bacteria. Although we only used ALPS primers—which are specific to the phoD alkaline phosphatase gene (Tan et al., 2013), and extracellular alkaline phosphatase (EC 3.1.3.1–ALP) is a non-specific enzyme that catalyses the hydrolysis of ester-phosphate bonds of many orthophosphate monoesters except phytate (Nannipieri et al., 2011)—PhoD is widespread in both terrestrial and aquatic ecosystems (Tan et al., 2013), and different studies have reported increases in ALP activity following manure application (Saha et al., 2008). ALP activity has previously been significantly correlated with phoD gene abundance, providing an indication of bacterial ALP production in the analyzed soils (Fraser et al., 2015). However, since high rates of mineral P may inhibit transcription by the Pho regulon (Oshima et al., 1996), thereby suppressing bacterial ALP activity (Saha et al., 2008; Zhang et al., 2012), the inorganic phosphate present in PL may have also hampered phosphatase activity, perhaps explaining why we did not record any differences in the abundance of phosphate-mineralizing bacteria that could be linked to fertilizer type.
In conclusion, we found that maize rhizosphere is not differentially affected by the type of fertilizer applied and, instead, alterations observed in the diversity of the soil bacterial communities studied here can be associated with plant age (during flowering and grain-filling and at maturity) and the amount of fertilizer used (130 or 260 kg). Changes in the abundance of phosphate-mineralizing bacteria were also related to sampling time (plant age) and, to a lesser extent, to a rhizosphere effect. We report that the use of PL mixed with inorganic fertilizers: (i) does not affect the total bacterial community; (ii) does not increase the concentration of FQs in soil; and (iii) does not increase the copy number of genes related to quinolone-resistant bacteria. All these results demonstrate that PL can be applied to agricultural fields as a source of nutrients, primarily phosphorus, to enhance crop production. In future, a more deep functional approach could be used to understand the implications of the use of PL mixed with inorganic fertilizers on soil microbial processes not only essential to ecosystem function but also for the sustainable management of agricultural ecosystems.
Author Contributions
RV and SC performed the experiments, interpreted the data, wrote the manuscript, and revised it until its final version. DM, AR, and IM participated in designing the study and made significant contributions to maize cultivation. DL and CP participated during the experiments. OM and DJ participated in revising the manuscript. LS participated in designing the study, interpreted the data, and writing of the manuscript. All of the authors examined and agreed with the final version of the manuscript.
Conflict of Interest Statement
The authors declare that the research was conducted in the absence of any commercial or financial relationships that could be construed as a potential conflict of interest.
Acknowledgments
This study was supported by grants from the National Research Council of Brazil (CNPq), Coordenação de Aperfeiçoamento de Pessoal de Nível Superior (CAPES) and Fundação de Amparo à Pesquisa do Estado do Rio de Janeiro (FAPERJ). We thank Dr. Renata Picão for providing the quinolone resistant bacterial strains.
Supplementary Material
The Supplementary Material for this article can be found online at: https://www.frontiersin.org/articles/10.3389/fenvs.2018.00118/full#supplementary-material
References
Ademba, J. S., Kwach, J. K., Esilaba, A. O., and Ngari, S. M. (2015). The effects of phosphate fertilizers and manure on maize yields in South Western Kenya. E. Afr. Agr. Forestry J. 81, 1–11. doi: 10.1080/00128325.2015.1040640
Ademba, J. S., Kwach, J. K., Ngari, S. M., Esilaba, A. O., and Kidula, N. L. (2014). Evaluation of organic and inorganic amendments on nutrient uptake, phosphorus use efficiency and yield of maize in Kisii County, Kenya. Afr. J. Agr. Res. 9, 1571–1578. doi: 10.5897/AJAR2012.709
Altschul, S. F., Madden, T. L., Schäffer, A. A., Zhang, J., Zhang, Z., Miller, W., et al. (1997). Gapped BLAST and PS I-BLAST: a new generation of protein database search programs. Nucleic Acids Res. 25, 3389–3402. doi: 10.1093/nar/25.17.3389
Ames, B. N. (1966). Assay of inorganic phosphate, total phosphate and phosphatases. Meth. Enzymol. 8, 115–118. doi: 10.1016/0076-6879(66)08014-5
Baghdadi, A., Halim, R. A., Ghasemzadeh, A., Ramlan, M. F., and Sakimin, S. Z. (2018). Impact of organic and inorganic fertilizers on yield and quality of silage corn in intercropped system with soybean. PeerJ. Preprints 6:e26905v1. doi: 10.7287/peerj.preprints.26905v1
Bokulich, N. A., Subramanian, S., Faith, J. J., Gevers, D., Gordon, J. I., Knight, R., et al. (2013). Quality-filtering vastly improves diversity estimates from Illumina amplicon sequencing. Nat. Methods 10, 57–59. doi: 10.1038/nmeth.2276
Bolan, N. S., Szogi, A. A., Chuasavathi, T., Seshadri, B., Rothrock, M. J. Jr., and Panneerselvam, P. (2010). Uses and management of poultry litter. Worlds Poult. Sci. J. 66, 673–698. doi: 10.1017/S0043933910000656
Caporaso, J. G., Bittinger, K., Bushman, F. D., DeSantis, T. Z., Andersen, G. L., and Knight, R. (2010b). PyNAST: a flexible tool for aligning sequences to a template alignment. Bioinformatics 26, 266–267. doi: 10.1093/bioinformatics/btp636
Caporaso, J. G., Kuczynski, J., Stombaugh, J., Bittinger, K., Bushman, F. D., Costello, E. K., et al. (2010a). QIIME allows analysis of high-throughput community sequencing data. Nat. Methods 7, 335–336. doi: 10.1038/nmeth0510-335
Caporaso, J. G., Lauber, C. L., Walters, W. A., Berg-Lyons, D., Lozupone, C. A., Turnbaugh, P. J., et al. (2011). Global patterns of 16S rRNA diversity at a depth of millions of sequences per sample. Proc. Natl. Acad. Sci. U.S.A. 108, 4516–4522. doi: 10.1073/pnas.1000080107
Chao, A. (1987). Estimating the population size for capture-recapture data with unequal catchability. Biometrics 43, 783–791. doi: 10.2307/2531532
Chen, Y., Rekha, P., Arun, A., Shen, F., Lai, W., and Young, C. (2006). Phosphate solubilizing bacteria from subtropical soil and their tricalcicum phosphate solubilizing abilities. Agric. Ecosyst. Environ. Appl. Soil Ecol. 34, 33–41. doi: 10.1016/j.apsoil.2005.12.002
Chen, Z., and Jiang, X. (2014). Microbiological safety of chicken litter or chicken litter-based organic fertilizers: a review. Agriculture 4, 1–29. doi: 10.3390/agriculture4010001
Chien, S. H., and Menon, R. G. (1995). Factors affecting the agronomic effectiveness of phosphate rock for direct application. Fert. Res. 41, 227–234. doi: 10.1007/BF00748312
Chinivasagam, H. N., Redding, M., Runge, G., and Blackall, P. J. (2010). Presence and incidence of food-borne pathogens in Australian chicken litter. Br. Poult. Sci. 51, 311–318. doi: 10.1080/00071668.2010.499424
DeSantis, T. Z., Hugenholtz, P., Larsen, N., Rojas, M., Brodie, E. L., Keller, K., et al. (2006). Greengenes, a chimera-checked 16S rRNA gene database and workbench compatible with ARB. Appl. Environ. Microbiol. 72, 5069–5072. doi: 10.1128/AEM.03006-05
Dong, W. -Y., Zhang, X. -Y, Dai, X. -Q, Fu, X. -L, Yang, F. -T., Liu, X. -Y., et al. (2014). Changes in soil microbial community composition in response to fertilization of paddy soils in subtropical China. Agric. Ecosyst. Environ. Appl. Soil Ecol. 84, 140–147. doi: 10.1016/j.apsoil.2014.06.007
Eckert, C. T., Frigo, E. P., Albrecht, L. P., Albrecht, A. J. P., Christ, D., Santos, W. G., et al. (2018). Maize ethanol production in Brazil: characteristics and perspectives. Renew. Sust. Energ. Rev. 82, 3907–3912. doi: 10.1016/j.rser.2017.10.082
Edgar, R. C. (2010). Search and clustering orders of magnitude faster than BLAST. Bioinformatics 26, 2460–2461. doi: 10.1093/bioinformatics/btq461
Fair, R. J., and Tor, Y. (2014). Antibiotics and bacterial resistance in the 21st century. Perspect. Medicin. Chem. 6, 25–64. doi: 10.4137/PMC.S14459
Faissal, A., Ouazzani, N., Parrado, J. R., Dary, M., Manyani, H., Morgado, B. R., et al. (2017). Impact of fertilization by natural manure on the microbial quality of soil: molecular approach. Saudi J. Biol. Sci. 24, 1437–1443. doi: 10.1016/j.sjbs.2017.01.005
Fontoura, S. M. V., Vieira, R. C. B., Bayer, C., Ernani, P. R., and Moraes, R. P. (2010). Eficiência técnica de fertilizantes fosfatados em latossolo sob plantio direto. Rev. Bras. Ciênc. Solo 34, 1907–1914. doi: 10.1590/S0100-06832010000600015
Franzini, V., Muraoka, T., and Mendes, F. L. (2009). Ratio and rate effects of 32P-triple superphosphate and phosphate rock mixtures on corn growth. Sci. Agric. 66, 71–76. doi: 10.1590/S0103-90162009000100010
Fraser, T., Lynch, D. H., Entz, M. H., and Dunfield, K. E. (2015). Linking alkaline phosphatase activity with bacterial phoD gene abundance in soil from a long-term management trial. Geoderma 257–258, 115–122. doi: 10.1016/j.geoderma.2014.10.016
Gaurav, N., Sivasankari, S., Kiran, G. S., Ninawe, A., and Selvin, J. (2017). Utilization of bioresources for sustainable biofuels: a review. Renew. Sust. Energy Rev. 73, 205–214. doi: 10.1016/j.rser.2017.01.070
Gouvêa, R., Santos, F. F. dos, Aquino, M. H. C. de, and Pereira, V. L. de A. (2015). Fluoroquinolones in industrial poultry production, bacterial resistance and food residues: a review. Rev. Bras. Cienc. Avic. 17, 1–10. doi: 10.1590/1516-635x17011-10
Hammer, Ø., Harper, D. A. T., and Ryan, P. D. (2001). Paleontological statistics software package for education and data analysis. Palaeontol. Electron. 4, 9–18.
Hartmann, M., Frey, B., Mayer, J., Mader, P., and Widmer, F. (2015). Distinct soil microbial diversity under long-term organic and conventional farming. ISME J. 9. 1177–1194. doi: 10.1038/ismej.2014.210
Hou, J., Wan, W., Mao, D., Wang, C., Mu, Q., Qin, S., et al. (2015). Occurrence and distribution of sulfonamides, tetracyclines, quinolones, macrolides, and nitrofurans in livestock manure and amended soils of Northern China. Environ. Sci. Pollut. Res. Int. 22, 4545–4554. doi: 10.1007/s11356-014-3632-y
Kemper, N. (2008). Veterinary antibiotics in the aquatic and terrestrial environment. Ecol. Indic. 8, 1–13. doi: 10.1016/j.ecolind.2007.06.002
Kidinda, L. K., Kasu-Bandi, B. T., Mukalay, J. B., Kabemba, M. K., Ntata, C. N., Ntale, T. M., et al. (2015). Impact of chicken manure integration with mineral fertilizer on soil nutriments balance and maize (Zea mays) yield: a case study on degraded soil of Lubumbashi (DR Congo). Am. J. Plant Nutr. Fert. Technol. 5, 71–78. doi: 10.3923/ajpnft.2015.71.78
Kraychete, G. B., Botelho, L. A., Campana, E. H., Picão, R. C., and Bonelli, R. R. (2016). Updated multiplex PCR for detection of all six plasmid-mediated qnr gene families. Antimicrob. Agents Chemother. 60, 7524–7526. doi: 10.1128/AAC.01447-16
Leal, R. M., Figueira, R. F., Tornisielo, V. L., and Regitano, J. B. (2012). Occurrence and sorption of fluoroquinolones in poultry litters and soils from São Paulo State, Brazil. Sci. Total Environ. 432, 344–349. doi: 10.1016/j.scitotenv.2012.06.002
Li, J., Cooper, J. M., Lin, Z. A., Li, Y., Yang, X., and Zhao, B. (2015). Soil microbial community structure and function are significantly affected by long-term organic and mineral fertilization regimes in the North China Plain. Agric. Ecosyst. Environ. Appl. Soil Ecol. 96, 75–87. doi: 10.1016/j.apsoil.2015.07.001
Luo, Y., Mao, D. Q., Rysz, M., Zhou, Q. X., Zhang, H. J., Xu, L., et al. (2010). Trends in antibiotic resistance genes occurrence in the Haihe River, China. Environ. Sci. Technol. 44, 7220–7225. doi: 10.1021/es100233w
Martins, D. C., De Resende, A. V., Galvão, J. C. C., Simão, E. P., Ferreira, J. P. C., and Almeida, G. O. (2017). Organomineral phosphorus fertilization in the production of corn, soybean and bean cultivated in succession. Am. J. Plant Sci. 8, 2407–2421. doi: 10.4236/ajps.2017.810163
Melkamu, J. (2012). “Impact of mineral fertilizer integration with farmyard manure on crop yield, nutrient use efficiency and soil fertility in a long-term trial,” in Crop Production Technologies, eds P. Sharma, and V. Abrol (Shanghai: InTech), 153–168.
Nannipieri, P., Giagnoni, L., Landi, L., and Renella, G. (2011). “Role of phosphatase enzymes in soil,” in Phosphorus in Action, Soil Biology, eds E. K. Bünemann, A. Oberson, and E. Frossard (Berlin; Heidelberg: Springer-Verlag), 215–243.
Nõlvak, H., Truu, M., Kanger, K., Tampere, M., Espenberg, M., Loit, E., et al. (2016). Inorganic and organic fertilizers impact the abundance and proportion of antibiotic resistance and integron-integrase genes in agricultural grassland soil. Sci. Total Environ. 562, 678–689. doi: 10.1016/j.scitotenv.2016.04.035
Novais, R. F., and Mello, J. W. V. (2007). “Relação solo-planta,” in Fertilidade Do Solo, eds R. F. Novais, V. H. Alvarez, N. F. Barros, R. L. F. Fontes, R. B. Cantarutti, and J. C. L. Neves (Viçosa: Sociedade Brasileira de Ciência do Solo), 133–204.
Okano, Y., Hristova, K. R., Leutenegger, C. M., Jackson, L. E., Denison, R. F., Gebreyesus, B., et al. (2004). Application of real-time PCR to study effects of ammonium on population size of ammonia-oxidizing bacteria in soil. Appl. Environ. Microbiol. 70, 1008–1016. doi: 10.1128/AEM.70.2.1008-1016
Oshima, Y., Ogawa, N., and Harashima, S. (1996). Regulation of phosphatase synthesis in Saccharomyces cerevisiae—a review. Gene 179, 171–177. doi: 10.1016/S0378-1119(96)00425-8
Picó, Y., and Andreu, V. (2007). Fluoroquinolones in soil–risks and challenges. Anal. Bioanal. Chem. 387, 1287–1299. doi: 10.1007/s00216-006-0843-1
Poulsen, P. H., Al-Soud, W. A., Bergmark, L., Magid, J., Hansen, L. H., and Sørensen, S. J. (2013). Effects of fertilization with urban and agricultural organic wastes in a field trial–prokaryotic diversity investigated by pyrosequencing. Soil Biol. Biochem. 57, 784–793. doi: 10.1016/j.soilbio.2011.12.023
R Core Team (2013). R: A Language and Environment for Statistical Computing. Vienna: R Foundation for Statistical Computing. Available online at: http://www.R-project.org/
Ramachandra, T. V. (2006). Soil and Groundwater Pollution from Agricultural Activities. New Delhi: TERI Press. 347.
Ranum, P., Pena-Rosas, J. P., and Garcia-Casal, M. N. (2014). Global maize production, utilization, and consumption. Ann. N. Y. Acad. Sci. 1312, 105–112. doi: 10.1111/nyas.12396
Saha, S., Prakash, V., Kundu, S., Kumar, N., and Lal Mina, B. (2008). Soil enzymatic activity as affected by long term application of farm yard manure and mineral fertilizer under rainfed soybean-wheat system in N-W Himalaya. Eur. J. Soil Biol. 44, 309–315. doi: 10.1016/j.ejsobi.2008.02.004
Sakurai, M., Wasaki, J., Tomizawa, Y., Shinano, T., and Osaki, M. (2008). Analysis of bacterial communities on alkaline phosphatase genes in soil supplied with organic matter. Soil Sci. Plant Nutr. 54, 62–71. doi: 10.1111/j.1747-0765.2007.00210.x
Sambrook, J., Fritsch, E. F., and Maniatis, T. (1989). Molecular Cloning: A Laboratory Manual, 2nd Edn. New York, NY: Cold Spring Harbor Laboratory Press.
Shannon, C. E., and Weaver, W. (1949). The Mathematical Theory of Communication. Chicago, IL: University of Illinois Press. 144.
Tabatabai, M. A. (1994). “Soil enzymes,” in Methods of Soil Analysis, ed J. M. Bigham (Madison, WI: Soil Science Society of America, Inc. and American Society of Agronomy, Inc.), 775–834.
Tan, H., Matthieu, B., Mooij, M. J., Rice, O., Morrissey, J. P., Dobson, A., et al. (2013). Long-term phosphorus fertilisation increased the diversity of the total bacterial community and the phoD phosphorus mineraliser group in pasture soils. Biol. Fertil. Soils 49, 661–672. doi: 10.1007/s00374-012-0755-5
Treseder, K. K. (2008). Nitrogen additions and microbial biomass: a meta-analysis of ecosystem studies. Ecol. Lett. 11, 1111–1120. doi: 10.1111/j.1461-0248.2008.01230.x
Turiel, E., Martín-Esteban, A., and Tadeo, J. L. (2006). Multiresidue analysis of quinolones and fluoroquinolones in soil by ultrasonic-assisted extraction in small columns and HPLC-UV. Anal. Chim. Acta 562, 30–35. doi: 10.1016/j.aca.2006.01.054
Uslu, M. Ö., Yediler, A., Balcioglu, I. A., and Schulte-Hostede, S. (2008). Analysis and sorption behavior of fluoroquinolones in solid matrices. Water Air Soil Pollut. 190, 55–63. doi: 10.1007/s11270-007-9580-0
Wilkinson, S. R. (1979). Plant nutrient and economic value of animal manures. J. Anim. Sci. 48, 121–133.
Zhang, A., Chen, Z., Zhang, G., Chen, L., and Wu, Z. (2012). Soil phosphorus composition determined by 31P NMR spectroscopy and relative phosphatase activities influenced by land use. Eur. J. Soil Biol. 52, 73–77. doi: 10.1016/j.ejsobi.2012.07.001
Zhang, Q. Q., Ying, G. G., Pan, C. G., Liu, Y. S., and Zhao, J. L. (2015). Comprehensive evaluation of antibiotics emission and fate in the river basins of China: source analysis, multi-media modeling, and linkage to bacterial resistance. Environ. Sci. Technol. 49, 6772–6782. doi: 10.1021/acs.est.5b00729
Keywords: maize rhizosphere, poultry litter, organomineral fertilizer, bacterial community, fluoroquinolones
Citation: Vollú RE, Cotta SR, Jurelevicius D, Leite DCA, Parente CET, Malm O, Martins DC, Resende AV, Marriel IE and Seldin L (2018) Response of the Bacterial Communities Associated With Maize Rhizosphere to Poultry Litter as an Organomineral Fertilizer. Front. Environ. Sci. 6:118. doi: 10.3389/fenvs.2018.00118
Received: 08 August 2018; Accepted: 21 September 2018;
Published: 16 October 2018.
Edited by:
Victor Satler Pylro, Universidade Federal de Lavras, BrazilReviewed by:
Leandro Nascimento Lemos, Universidade de São Paulo, BrazilGilberto de Oliveira Mendes, Federal University of Uberlandia, Brazil
Acacio Aparecido Navarrete, Universidade Federal de São Carlos, Brazil
Copyright © 2018 Vollú, Cotta, Jurelevicius, Leite, Parente, Malm, Martins, Resende, Marriel and Seldin. This is an open-access article distributed under the terms of the Creative Commons Attribution License (CC BY). The use, distribution or reproduction in other forums is permitted, provided the original author(s) and the copyright owner(s) are credited and that the original publication in this journal is cited, in accordance with accepted academic practice. No use, distribution or reproduction is permitted which does not comply with these terms.
*Correspondence: Lucy Seldin, bHNlbGRpbkBtaWNyby51ZnJqLmJy